- 1College of Agronomy, Northwest A&F University, Yangling, Shaanxi, China
- 2Key Laboratory of Crop Physic–ecology and Tillage Science in Northwestern Loess Plateau, Ministry of Agriculture, Northwest A&F University, Yangling, Shaanxi, China
- 3State Key Lab of Soil Erosion and Dryland Farming on the Loess Plateau, Institute of Soil and Water Conservation, Northwest A&F University, Yangling, Shaanxi, China
- 4Department of Agronomy, University of Agriculture, Faisalabad, Pakistan
- 5Department of Zoology, College of Science, King Saud University, Riyadh, Saudi Arabia
- 6Wroclaw University of Environmental and Life Sciences, Institute of Soil Science, Plant Nutrition and Environmental Protection, Wroclaw, Poland
Improving cropping systems together with suitable agronomic management practices can maintain dry farming productivity and reduce water competition with low N inputs. The objective of the study was to determine the photosynthetic and yield responses of maize and peanut under six treatments: sole maize, sole peanut, maize–peanut intercropping, maize–peanut rotation–intercropping, 20% and 40% N reductions for maize in the maize–peanut rotation–intercropping. Maize–peanut intercropping had no land-use advantage. Intercropped peanut is limited in carboxylation rates and electron transport rate (ETR), leading to a decrease in hundred-grain weight (HGW) and an increase in blighted pods number per plant (NBP). Intercropped peanut adapts to light stress by decreasing light saturation point (Isat) and light compensation point (Icomp) and increasing the electron transport efficiency. Intercropped maize showed an increase in maximum photosynthetic rate (Pnmax) and Icomp due to a combination of improved intercellular CO2 concentration, carboxylation rates, PSII photochemical quantum efficiency, and ETR. Compare to maize–peanut intercropping, maize–peanut rotation–intercropping alleviated the continuous crop barriers of intercropped border row peanut by improving carboxylation rates, electron transport efficiency and decreasing Isat, thereby increasing its HGW and NBP. More importantly, the land equivalent ratio of maize–peanut rotation–intercropping in the second and third planting years were 1.05 and 1.07, respectively, showing obvious land use advantages. A 20% N reduction for maize in maize–peanut rotation–intercropping does not affect photosynthetic character and yield for intercropped crops. However, a 40% N reduction decreased significantly the carboxylation rates, ETR, Icomp and Pnmax of intercropped maize, thereby reducing in a 14.83% HGW and 5.75% lower grain number per spike, and making land-use efficiency negative.
1 Introduction
China has a big population and little land, relatively sparse vacancy of land resources, and inadequate cultivated land reserve resources (Yang and Li, 2000; Wu et al., 2017). In order to safeguard food security, China has historically advocated intensive cultivation with high input and high output (Yu and Lu, 2006; Jiang et al., 2013). The long-term implementation of the intensive cultivation strategy inevitably brings a negative influence on soil, results in a huge waste of water and nitrogen, and leads to soil desiccation and pollution (Gianfreda et al., 2005), especially in dry farming areas with weak environmental carrying capacity (Yan et al., 2017). Wheat (Triticum aestivum L.) and maize (Zea mays L.) rotation are the main cropping system in China’s dry farming areas. Studies have shown that the multi-year continuous cropping of maize and wheat declines the quality of cultivated land (Mrabet et al., 2001; Parihar et al., 2017). At present, many farmers in dry farming areas report various degrees of land degradation, including reduced soil fertility, shallow cultivation layers, and poor soil tillage, leading to over-reliance on chemical fertilizers, less risk tolerance, and a decline in farming efficiency.
Land fallow is an important measure to restore fertility, improve grain production capacity, and resist natural disasters in dry farming areas (Gozubuyuk et al., 2014; Nittaya et al., 2017). In addition, land fallow during the dry season in dry crop areas reduces irrigation pressure on farmland in other cropping systems, which can contribute to ensuring regional food security. However, the fall in farm income has hindered the spread of land fallow. Combining cereal-legume intercropping with land fallow will improve farm output due to low agricultural inputs and high economic benefits (Kermah et al., 2017; Rahman et al., 2021). More importantly, intercropping in dry farming can reduce N pollution at the source, as fallow and legumes require far less chemical N fertilizer than cereal. After harvest, legumes leave a large amount of easily decomposable residues and improve the soil microbial environment and soil N mineralization capacity for use by subsequent crops (Stagnari et al., 2017; Kakraliya et al., 2018). It is important to develop a promising and easy-to-operate cropping system with the advantages of crop intercropping and land fallow is of great significance to realize the balanced development of economic and ecological benefits.
Peanut (Arachis hypogaea L.) is a widely grown oilseed and cash crop in tropical and subtropical regions, and a major source of oil for human consumption. Maize–peanut intercropping can exert the advantages of marginal effect and peanut biological N fixation, improve the absorption and utilization of nutrient elements in the intercropping population, and reduce replant disease (Li et al., 2009; Guo et al., 2021). Maize makes the largest contribution to the high yield advantage, the adaptation of peanuts to low light is key to determining the economic benefits of intercropping. In a meta-analysis, the first and the third quartile for partial land equivalent ratios (PLER) for peanuts were 0.38 and 0.63 (Feng et al., 2021). Overall, besides the border-row proportion (Wang et al., 2020), the cause of the great differences among PLER was environmental conditions and the N application rate. However, few studies of maize–peanut intercropping focused on dryland farming. In addition, most N reduction studies in intercropping were based on simultaneous reduction of base fertilizer and top dressing, but the key to N reduction in cereal-legume intercropping systems is the efficient use of N by the cereal and the increased nitrogen fixation of the legume in the later growth stages of the crops (Kermah et al., 2017; Yong et al., 2018; Li et al., 2019). Premature N reduction at basal fertilizer is likely to affect maize and peanut growth. It is worth noting that intercropped peanuts are equivalent to long-term continuous cropping in the same area if the planting strips are not changed, and there are barriers to the risk of continuous cropping. To address the above issues, we rotated the maize planting strip (MPS) and peanut planting strip (PPS) and reduce N in maize top dressing based on the previous annual maize–peanut intercropping.
Plants accumulate biomass through photosynthesis, and the photosynthetic utilization capacity of crops is closely related to water, N application rate, and intensity of light interception (Sims et al., 1998b; Coruzzi and Bush, 2001; Flexas et al., 2006). The complex community structure of intercropping systems can alter the distribution and intensity of light and nutrient utilization (Gong et al., 2015; Yang et al., 2017). Lack of water in dry farming areas and N reduction can fundamentally alter photosynthesis in crops, directly affecting dry matter production (Escalona et al., 2012; Chai et al., 2016). We hypothesize that (i) maize-peanut intercropping could increase the farmland yield by modifying the adaptation of peanuts and maize to different light radiation, (ii) rotating the planting strips in intercropping could increase the photosynthetic capacity and yield of peanuts by alleviating continuous cropping obstacles, and (iii) properly decreasing the application of chemical N fertilizer in MPS has no significant effect on photosynthetic capacity and yield of peanuts and maize in maize–peanut intercropping. In testing the hypothesis, we measured (i) soli total N (TN) content in MPS and PPS before planting, 30 days after top dressing (AT30d), 60 days after top dressing (AT60d), and after harvest, (ii) light interception, light response curve, Rubisco-related enzyme activity, photosynthetic and fluorescence characteristics of peanuts and maize, (iii) yield and yield-related traits under different planting patterns and N application rates. This study provides a theoretical basis for promoting a new intercropping system for peanut and maize in dry farming areas.
2 Materials and methods
2.1 Site description and experimental design
A three-year field experiment was conducted at the Yangling (108°08′E, 34°17′N), Shaanxi Province, China, from 2018 to 2020. This area has a cold climate with a dry winter and a hot summer. The mean annual temperature was 15°C with mean growing degree days from April to September, an annual sunshine duration of 2440 h, a frost-free period of 200 d, and total annual rainfalls of 993 mm. The climatic data during the study period were presented in Figure 1A. The soil was formed from alluvial deposits of the Yellow River and was classified as a sandy loam (Haplic Chernozems classification according to FAO–UNESCO 1988). The soil was sampled for the 0–20 cm depth before sowing in 2018 with 10.01 g kg−1 soil organic matter, 0.78 mg kg−1 total N, 7.21 mg kg−1 available P, and 178.76 mg kg−1 available K.
The experiment was established with a randomized, incomplete block design and with three replicates. Seeds of maize (cv. ‘Zhengdan 958’) and peanut (cv. ‘Fenghua No. 5’) were planted by hand on 7 May in rows oriented east to west. In 2018, the experiment comprised five treatments: sole maize, sole peanut, maize–peanut intercropping (I), 20% N and 40% N reductions for maize in the maize–peanut intercropping. In 2019, we rotated the maize planting strip (MPS) and peanut planting strip (PPS) in half of the area in maize–peanut intercropping (I) and all areas in 20% N and 40% N reductions in maize planting strip in the maize–peanut intercropping, and record the numbers RI, RI2, and RI3. In 2020, rotate the MPS and PPS of all RI, RI2, and RI3. Videlicet, 2019 and 2020, there were six treatments: sole maize (M), sole peanut (P), maize–peanut intercropping (I), maize–peanut rotation–intercropping (RI), 20% N (RI2), and 40% N (RI3) reductions in maize planting strip in the maize–peanut rotation–intercropping. The sole maize treatment received N fertilizer at 260 kg N ha−1 as urea, of which 90 kg N ha−1 of the total N was evenly spread and incorporated into the top 15 cm of soil as base fertilizer, and the remaining N fertilizer was as top dressing at the six-leaf stage of maize. The sole peanut treatment received N fertilizer at 90 kg ha−1 N as urea and all were as base fertilizer. Intercropped maize and peanut received the same area–based fertilizer as the corresponding sole crop. For RI2 and RI3, the 20% and 40% N reductions in MPS only took place at the top dressing, that is, 90 kg ha−1 was applied to both basal and 118 (RI2) and 66 kg (RI3) ha−1 N to the top dressing, respectively.
To soften the soil and eliminate weeds, the soil is mechanically ploughed about 15 cm deep before sowing. The plant spacings were 22.5 and 15 cm for sole maize and sole peanut, and the row spacing for all crops was 45 cm. The stand density was 148,000 plants ha−2 for sole peanut and 74,000 plants ha−2 for sole maize. The plant spacings and row spacing for intercropped peanut and maize were the same as the sole crop. Therefore, intercropped peanuts and maize had equal row spacing which leads to convenient rotation of planting strips. Two seeds of maize and peanut were planted per hole, and the seedlings were thinned to one vigorous plantlet after the seedling stage. Single plot size was 54 m2 (10 m × 5.4 m). Weeds were controlled by manual removal at once when needed. No irrigation water was applied during the growing season.
2.2 Measurements
2.2.1 Soil total N content
At preplant, AT30d, AT60d and harvest, soil samples were taken with a 9 mm inner–diameter soil drill at 0–20 and 20–40 cm. The intercropping treatment soil samples were divided into peanut and maize planting strip, and each planting strip was sampled between intercropped border and middle row and between intercropped middle rows (Figure 1B). Kjeldahl method were employed to measure TN content in the soil (Bremner and Mulvaney, 1996).
2.2.2 Light interception and light response curve
At the R1 stage of maize (full pod formation stage of peanut), the radiation transmission was recorded with a 1 m long line quantum sensor (AccuPAR LP–80, Meter, USA). A set of readings was taken from each crop strip starting at 7:30 and obtained every half hour until 17:30, including the incident and reflected light flux density at the top (canopy), spike leaf, bottom (earth surface) layer of maize, and the canopy, bottom layer of peanuts. Intercropped crops were divided into the intercropped middle row and intercropped border row to record data separately. The integral equation is used to calculate the total received light for the measurement period, while the light interception accumulation (II) is calculated using Eq. 1.
where ITI means incident I accumulation at the top of crop planting strip, ITR means reflected I accumulation at the top of crop planting strip, IBI means incident I accumulation at the bottom of crop planting strip, IBR means reflected I accumulation at the bottom of crop planting strip.
The light response of photosynthesis for spike leaves of maize and top third leaf of peanut main stems were selected to measure the light response using a portable photosynthetic system (LI–6400, Inc., Logan, NE, USA). In the leaf chamber, the CO2 concentration of the sample chamber was stabilized at 400 μmol mol–1, and the PPFD was controlled at 0, 50, 100, 200, 300, 400, 600, 800, 1000, 1200, 1400, 1600, 1800, 2000 μmol m–2 s–1. Selected leaves were acclimated for at least 2 min at each level of PPFD before switching. The Ye model characterizes the Pn–I relationship was Eq. 2 (Ye, 2007; Ye et al., 2013).
where α means the initial slope of light response curve, Rd means the dark respiration rate (μmol (CO2) m–2 s–1), and β and γ are the photoinhibition coefficient and saturation coefficient, respectively (Ye et al., 2013).
The light saturation point (Isat, μmol (photon) m–2 s–1) can be calculated when dPn/dI = 0 as Eq. 3. The light compensation point (Icomp, μmol (photon) m–2 s–1) can be calculated when Pn = 0 as Eq. 4. The asymptotic estimate of the maximum photosynthetic rate (Pnmax, μmol(CO2) m–2 s–1) can be calculated when I = Isat as Eq. 5 (Zhu et al., 2020).
2.2.3 Photosynthetic and fluorescence characteristics
At the R1 stage (full pod formation stage of peanut), spike leaves of maize and top third leaf of peanut main stems were selected to measure photosynthetic parameters using a Li-6400 portable photosynthesis system (LI–COR Inc., Lincoln, NE, USA). All measurements were conducted from 9:30 to 11:00 h under a CO2 concentration of 400 μmol mol−1. Intercropped crops were divided into the intercropped middle row and intercropped border row to record data separately. Specific parameters measured included photosynthetic rate (Pn, μmol CO2 m-2 g-1), intercellular CO2 concentration (Ci, μmol mol-1), and stomatal conductance (Gs, mmol m-2 g-1).
The leaves for the determination of photosynthesis parameters were taken to determine the Chlorophyll fluorescence parameters with a pulse amplitude modulation 2000 fluorometer (MFMS-2, Hansatech, Kings Lynn, UK) by the method of Zhao and Wang (2002). Specific parameters measured included maximum light energy conversion efficiency of PSII (Fv/Fm), effective quantum efficiency of PSII (ΦPSII), photochemical fluorescence quenching coefficient (qP), non-photochemical fluorescence quenching coefficient (qN), and electron transport rate (ETR).
2.2.4 Rubisco-related enzyme activity
At the R1 stage of maize (full pod formation stage of peanut), 0.1 g fresh leaf samples used for photosynthetic rate determination of 2.2.3 were ground to a powder in liquid nitrogen and then mixed with 2 mL of extraction solution (100 mmol L-1 Hepes–Na (pH 8. 0), 10 mmol L-1 MgCl2, 0.4 mmol L-1 EDTA–Na2, 1% PVP, 100 mmol L-1 Na-ascorbate, 0.1% BSA, and 50 mmol L-1 DTT). All extraction fluid was centrifuged for 15 minutes at 12000 × g for 10 min. The supernatant was collected to measure the enzymatic activity.
For Rubisco initial activity, reaction system included 0.1 mL of 5 mmol L-1 NADH, 0.1 mL of 50 mmol L-1 ATP, 0.1 mL of 0.2 mol L-1 NaHCO3, 0.7 mL of reactive medium (100 mmol L-1 Tris-HCl, 10 mmol L-1 MgCl2 and 0.4 mmol L-1 EDTA–Na2), 0.05 mL of 160 U mL-1 creatine kinase, 0.05 mL of160 U mL-1 phosphoglycerate kinase, 0.05 mL of160 U mL-1 Glyceraldehyde–3–phosphate dehydrogenase, 0.15 mL distilled water. The prepared reaction system was shaken well and 630μL was poured into the cuvette. After zeroing with reaction system at 340 nm, 50 μL RuBP and 20 μL enzyme solution were poured into the cuvette and the absorbance was immediately measured every 30 s at 340 nm (Ultraviolet–2600; UNICO Instruments, Shanghai, China) for 3 min. The absolute value of the decrease in absorbance per minute was used to calculate the rubisco initial activity as Eq. 6:
where ARubisco (μmol min-1 g FW-1) means the Rubisco activity; ΔA means the absolute value of the change in absorbance at 340 nm over 3 min; d (cm) means the optical path of cuvette; 6.22 means the absorbance at 340 nm per μmol NADH; 2 means fixation of 1 mol CO2 requires 2 mol NADH; Δt means the measurement time; VE means Total volume of the reaction system; m means the fresh sample weight.
For Rubisco total activity, 50 μL of enzyme solution was added to the 100 μL of reactive medium (0.2 mol L-1 NaHCO3, 100 mmol L-1 Tris-HCl, 10 mmol L-1 MgCl2, and 0.4 mmol L-1 EDTA-Na2) for 15 min at 30°C. Then, the absorbance was recorded according to the method for determining Rubisco initial activity. Rubisco activation state was expressed as the initial activity divided by the total activity (Butz and Sharkey, 1989).
For Rubisco activase (RCA), 50 μL of enzyme solution and 50 μg of Rubisco passivated with 0.4 mmol L-1 RuBP were added to 450 μL of the following reaction solution: 100 mmol L-1 Hepes-Na (pH 8. 0), 10 mol L-1 NaHCO3, 1 mmol L-1 EDTA–Na2, 10 mmol L-1 MgCl2, 2.5 mmol L-1 DDT, 5 mmol L-1 ATP, 5 mmol L-1 phosphocreatine, 5 U L-1 Creatine Kinase. By adding 25 μL of 12 mmolL-1 RuBP after reacting for 10 minutes, the absorbance was determined according to the Rubisco initial activity. RCA activity was the difference between Rubisco activity with and without RuBP passivation.
2.2.5 Yield and yield-related traits
A 43.2 m2 (8 m × 5.4 m) plant sample was collected to measure yield per plant, hundred-grain weight (HGW), pods number per plant and blighted pods number per plant (NBP) of peanut and yield per plant, hundred-grain weight (HGW) and grain number per spike of maize. LER was used to evaluate the land-use efficiency using a concept proposed by Willey (1979). It is expressed as:
where PLERM and PLERP are partial land equivalent ratios of maize and peanut, respectively. YSM and YIM are the sole and intercropped maize yields, respectively. YSP and YSP are the sole and intercropped peanut yields, respectively.
2.3 Data analysis
A factorial set of treatments was arranged within a randomized complete block design. Each treatment was repeated three times for triplicates of sampling. Data were analyzed using Origin 2022. The IBM SPSS Statistic Version 21.0 (StatSoft. Inc.) was used for statistical analysis. Significant differences were tested using an ANOVA in combination with Fisher’s LSD test at P< 0.05 levels. The TN, Rubisco-related enzyme activity, photosynthetic and fluorescence characteristics, yield, and yield-related traits were analyzed independently each year. The values presented in the figures and table were mean + standard errors. In the case of heteroscedasticity, data were analyzed by the Kruskal-Wallis H test and Tamhane’s T2 (P = 0.05) for post hoc multiple comparisons.
3 Results
3.1 Soli total N content
Because the top dressing in the intercropping was only in MPS, the soil TN content in MPS in intercropping decreased significantly than sole maize after AT30d, and PPS in intercropping increased significantly due to the movement of N in the soil (Figure 2). However, at AT60d, there was no significant difference between the intercropped MPS and the sole maize, while the soil TN content in MPS was also higher than in the sole peanut.
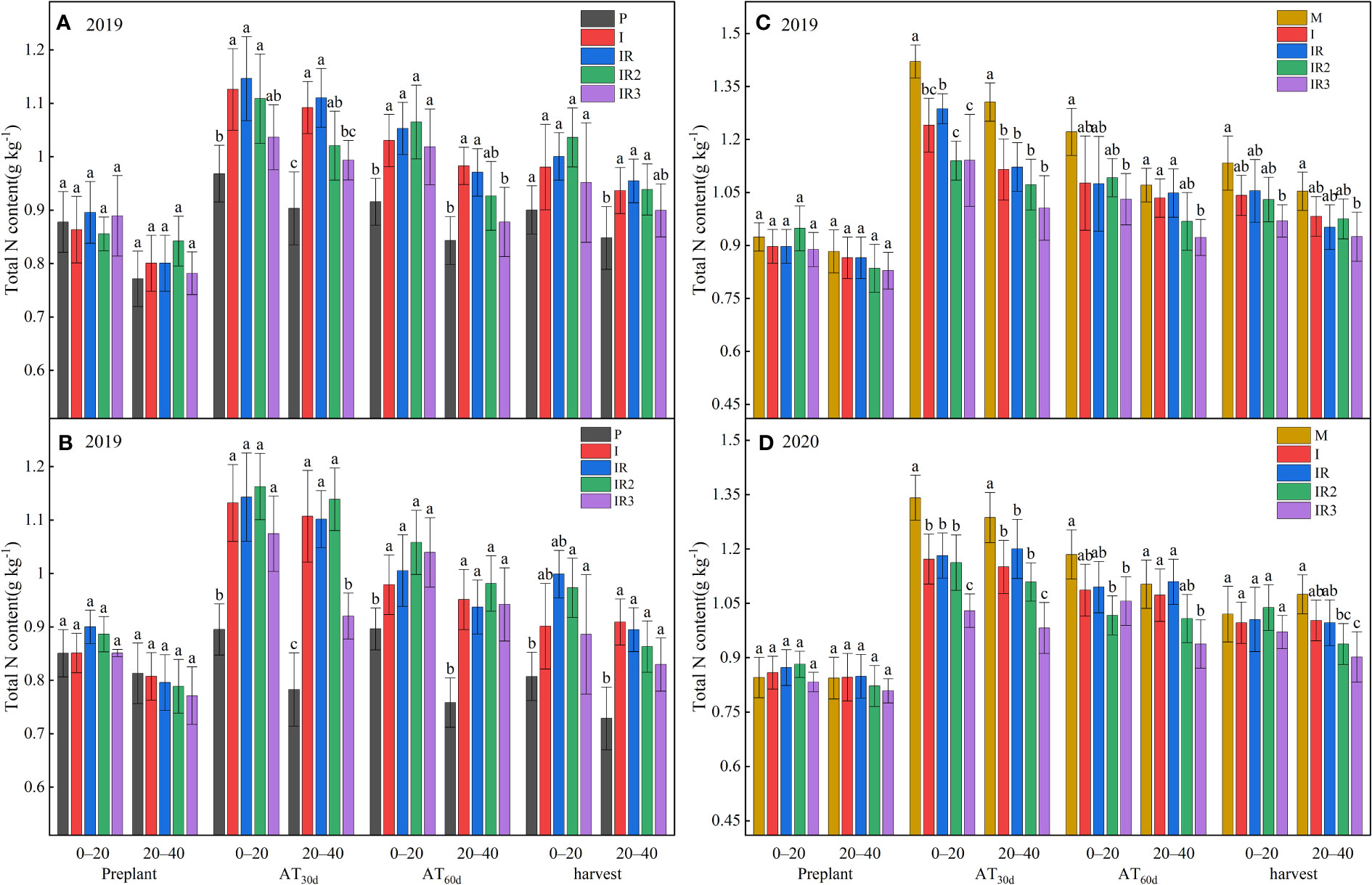
Figure 2 Total N content in 0–20 and 20–40 cm soil in peanut planting strip (A, B) and maize planting strip (C D). P, sole peanut; M, sole maize; I, maize–peanut intercropping; RI, maize–peanut rotation–intercropping, RI2, 20% N reduction for maize of RI; ICN3, 40% N reductions for maize of RI. Different lowercase letters represent significant (P<0.05).
Rotating the planting strips had no effect on soil TN content (Figure 2). A 40% N reduction significantly reduced the soil TN content of MPS in the 0–40 cm at AT30d and the 20–40 cm at AT60d. It was also found that the 20–40 cm soil TN content of PPS was reduced by 10.51%–16.48% and 9.56%–9.46% at AT30d and AT60d after 40% N reduction, respectively. A 20% N reduction was only found to reduce the TN content of the MPS by 11.44% at AT30d in 2019.
3.2 Photosynthetic character of peanut
During the maize R1 (stem and leaves had finished growing), there was an average reduction of 106.78% and 65.47% in top incident light, 120.40% and 61.28% in bottom incident light and 110.50% and 66.99% in light interception between 2019 and 2020 for intercropped border and middle row peanut compared to sole peanut (Figures S1, S3; Table 1). The intercropped disadvantage caused a significant reduction in Pn and Ci in peanuts (Figure 3). The Pnmax of the peanut in intercropped middle and border rows in 2019 and intercropped middle row in 2020 were not affected after intercropping, but Icomp and Isat showed a decrease, and the decreased degree was greater in intercropped border row of the peanut than in the middle row (Table 1). For chlorophyll fluorescence, intercropping increased the ΦPSII and qP, and decreased qN and ETR in peanuts (Table 2).
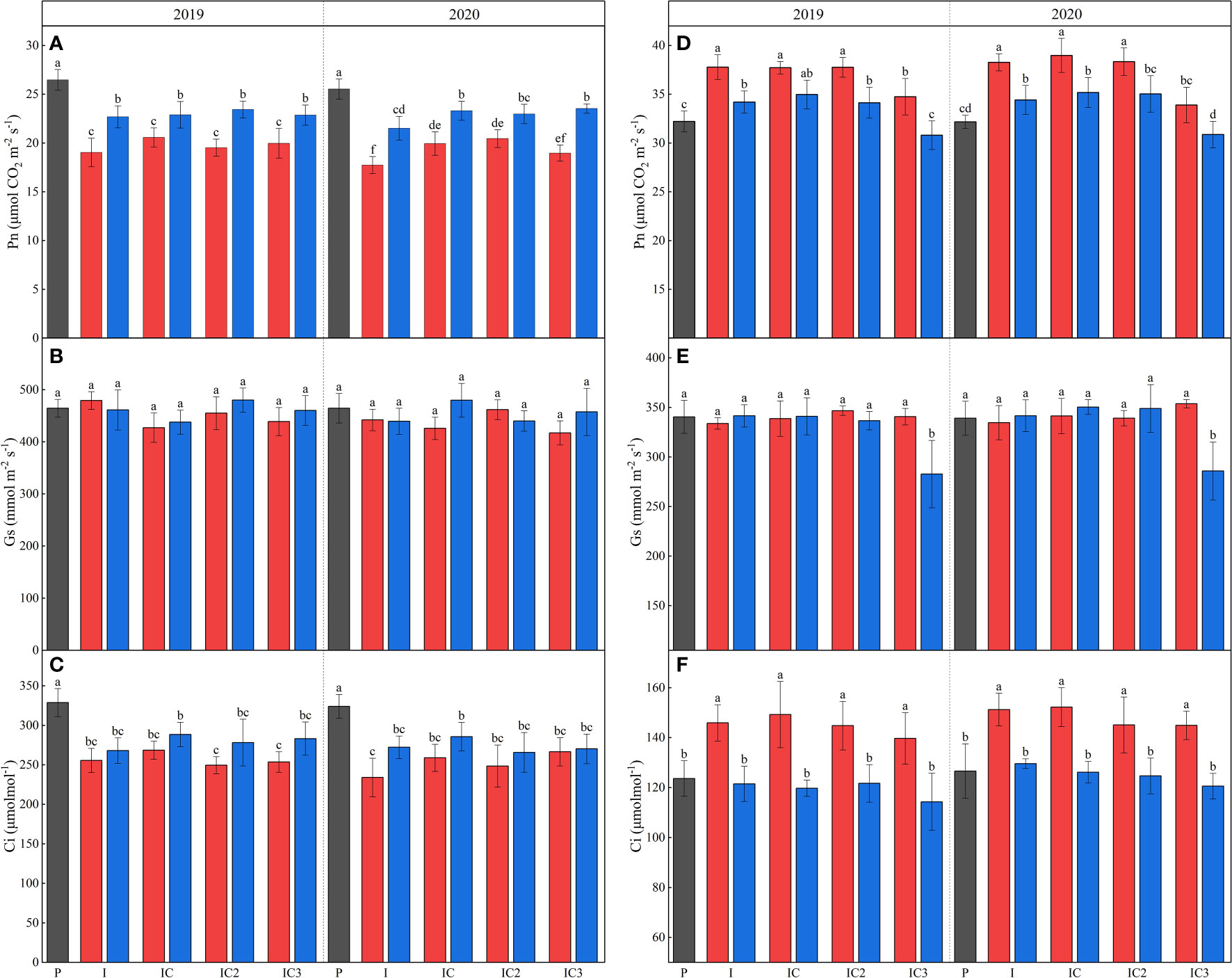
Figure 3 Pn (A, D), Gs (B, E) and Ci (C, F) for peanut and maize in 2019 and 2020. P, sole peanut; M, sole maize; I, maize–peanut intercropping; RI, maize–peanut rotation–intercropping, RI2, 20% N reduction for maize of RI; ICN3, 40% N reductions for maize of RI. Different lowercase letters represent significant (P<0.05).
Rotating the peanut and maize planting strips had no effect on peanut light interception, but significantly impacted light response curve (Table 1). In 2020, after rotating the planting strip, intercropped border row peanut had a significant 10.55% increase in Pnmax and a 7.76% decrease in Isat. Similar phenomenon was not observed in 2019. And in 2020, a significant increase in qP and ETR and a significant decrease in qN in the intercropping border row were found after rotating the planting strip. In this experiment, N reduction for maize in the maize–peanut intercropping systems had no significant effect on the incident light, photosynthetic parameters and fluorescence parameters of intercropped peanut.
3.3 Photosynthetic character of maize
In the maize–peanut intercropping systems, maize is the dominant crop due to its higher plant height. The incident light in the top and middle of intercropped maize was always higher than that of sole maize before 16:00 and 14:00, while the bottom incident light was always higher than that of sole maize (Figures S3, S4). The incident light in the top and spike leaf of intercropped border row maize was higher than those of the middle row maize before 13:30. During the testing period, the intercropped border and middle row maize compared to the sole maize showed an average increase in top incident light accumulation by 6.05% and 5.54%, spike leaf incident light accumulation by 64.04% and 52.03%, bottom incident light accumulation by 53.70% and 18.80%, light interception accumulation by 11.53% and 4.84% (Table 3). Pn, Ci, Pnmax, ΦPSII, and ETR increased and Rd decreased in intercropped maize compared to sole maize (Figure 3 and Table 3).
Rotating the planting strip and a 20% N reduction had no significant effect on the photosynthetic character of intercropped maize (Figure 3 and Table 3). A 40% N reduction led to a significant reduction in Pn by 7.86% and 13.03%, 11.92% and 12.27% in the intercropped middle row and border row in 2019 and 2020 respectively. For intercropped middle row maize, the Gs, Pnmax, Icomp, qP, ΦPSII, and ETR significantly decreased after a 40% N reduction; but for intercropped border row maize, only the decrease of qP was found to significantly decreased.
3.4 Rubisco-related enzyme activity
Rubisco is the key enzyme that catalyzes the initial steps of photosynthetic CO2 fixation. After intercropping, Rubisco initial activity, Rubisco activation rate, and RCA were significantly reduced in peanuts, with the reduction being much greater in the intercropped border row than in the intercropped middle row (Figure 4). Rotation strips did not affect Rubisco total activity and Rubisco activation rate in intercropped peanut, but significantly increased the Rubisco initial activity by 7.85% and Rubisco activation rate by 7.97% respectively in intercropped border row peanut in 2020. N reduction had no significant effect on Rubisco-related enzyme activity for peanut.
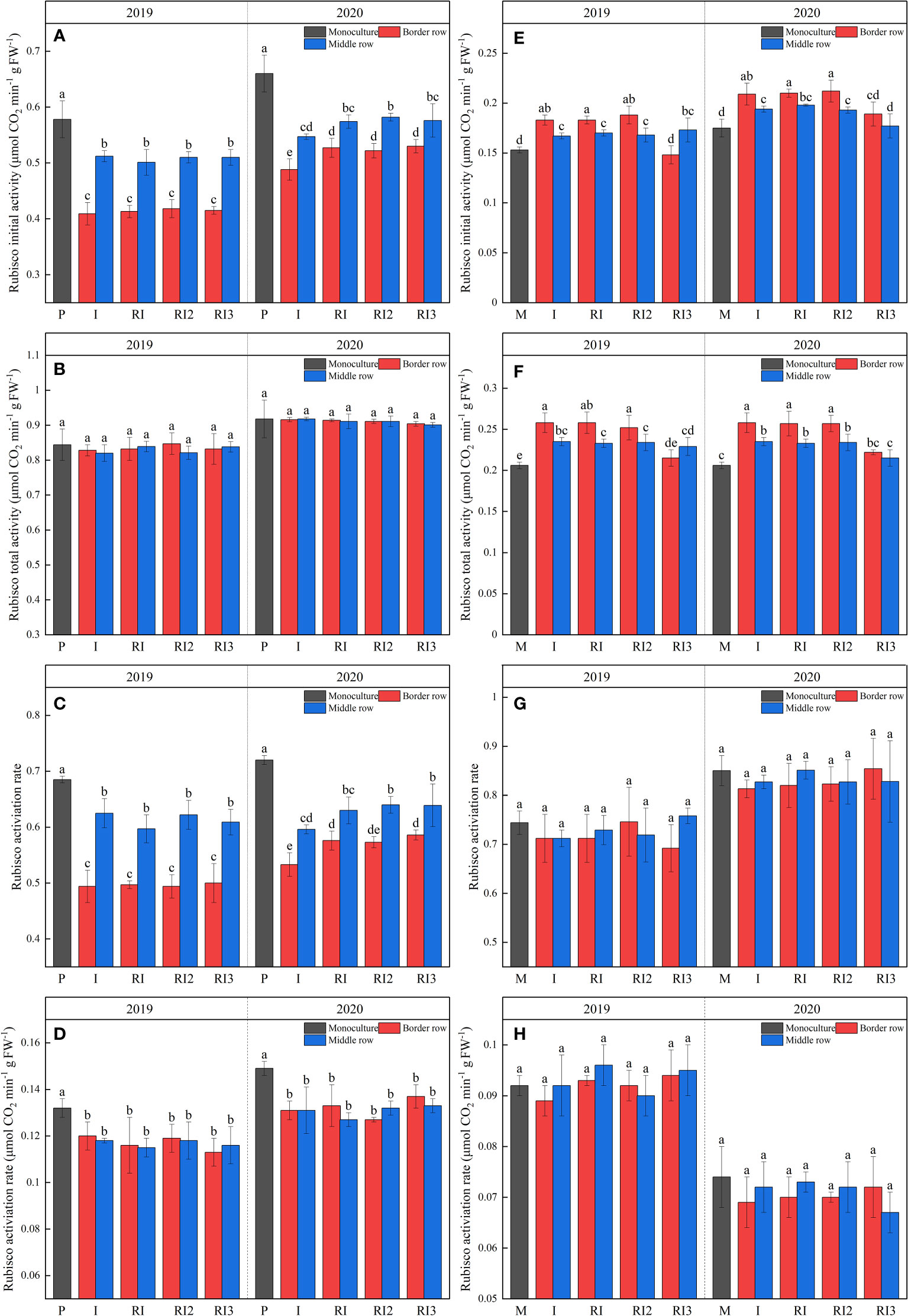
Figure 4 Rubisco initial activity (A, E), Rubisco total activity (B, F), Rubisco activation rate (C, G), and Rubisco activase (D, H) of peanuts and maize. P, sole peanut; M, sole maize; I, maize–peanut intercropping; RI, maize–peanut rotation–intercropping, RI2, 20% N reduction for maize of RI; ICN3, 40% N reductions for maize of RI. Different lowercase letters represent significant (P<0.05).
After intercropping, Rubisco initial activity and Rubisco total activity in the intercropped maize increased significantly due to light dominance (Figure 4). Rotating the planting strip does not affect maize Rubisco-related enzyme activity. A 40% N reduction reduced Rubisco initial activity and Rubisco total activity in the intercropped maize. It is noteworthy that Rubisco initial activity and Rubisco total activity were significantly higher in the I, RI, and RI2 border row than in the middle row, while this phenomenon was not observed in RI3.
3.5 Yield and yield-related traits
Intercropping peanut is a disadvantageous crop. After intercropping, peanuts pods number per plant remains unchanged, while the hundred-grain weight (HGW) decrease significantly and blighted pods number per plant (NBP) increase significantly, resulting in 26.26% and 17.58%, 35.71% and 16.67% reduction in yield per plant in 2019 and 2020 for intercropped border and middle rows, respectively (Figure 5). After rotating the planting strips, there was no significant difference in peanut yield per plant in 2019, but the yield per plant of intercropped border row peanut increased by 27.70% in 2020. Significant decreases in yield per plant and HGW were observed in sole peanut in 2020 compared to 2019. Importantly, the decreases in yield per plant and HGW of intercropped peanut in maize–peanut intercropping but not in maize–peanut rotation–intercropping. N reduction did not affect peanut yield and yield-related traits.
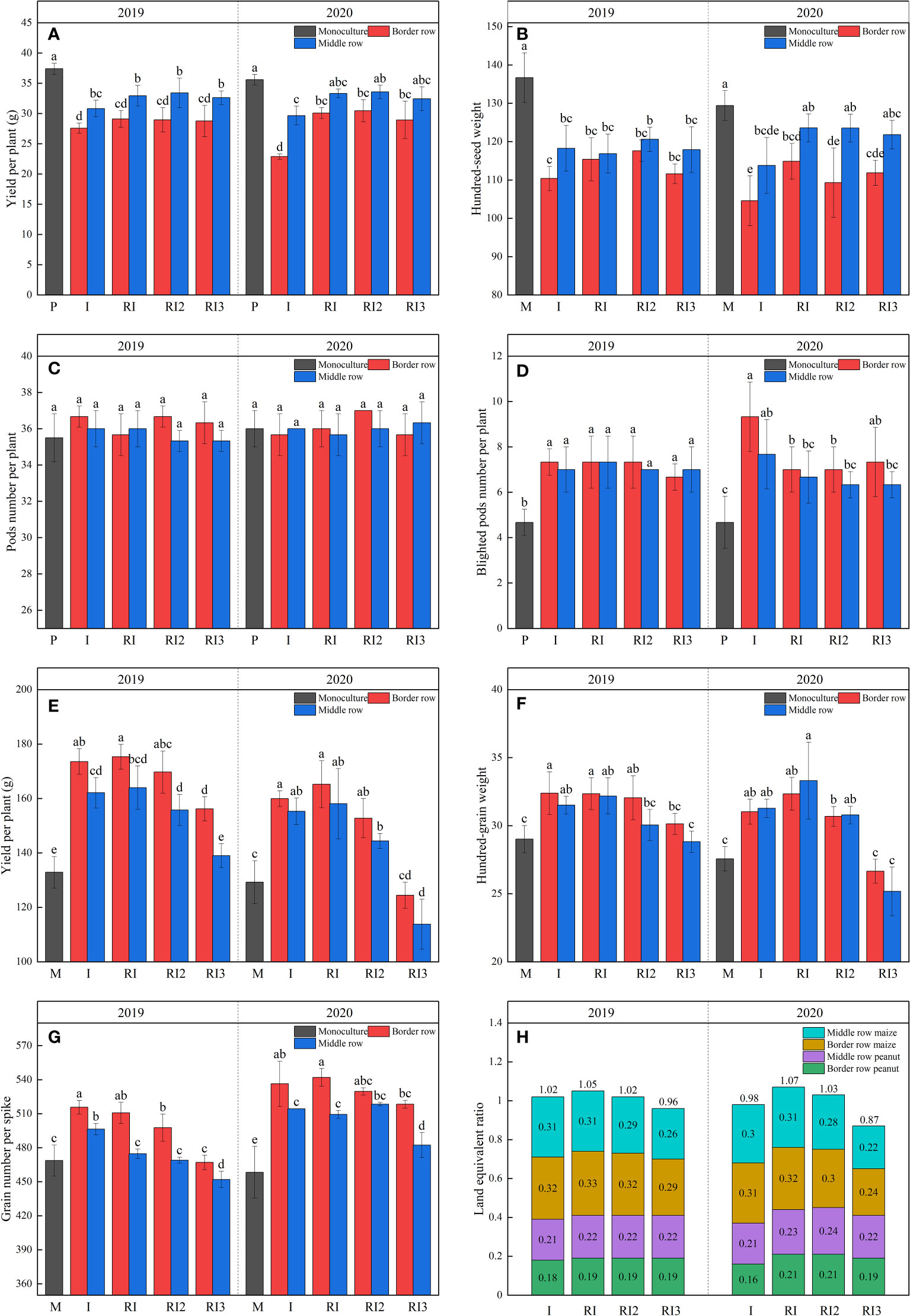
Figure 5 Yield, yield–related traits and land equivalent ratio. (A), yield per plant of peanut; (B), hundred-grain weight of peanut; (C), full pods number per plant of peanut; (D), blighted pods number per plant of peanut; (E), yield per plant of maize; (F), hundred-grain weight of maize; (G), grain number per spike of maize; (H) land equivalent ratio. P, sole peanut; M, sole maize; I, maize–peanut intercropping; RI, maize–peanut rotation–intercropping, RI2, 20% N reduction for maize of RI; ICN3, 40% N reductions for maize of RI. Different lowercase letters represent significant (P<0.05).
After intercropping, the yield per plant, HGW, and grain number per spike increased significantly in the intercropped maize (Figure 5). Rotating the planting strips and a 20% N reduction does not affect maize yield and yield-related traits. But after reducing N by 40%, the yield per plant, grain number per spike, and HGW of maize decreased significantly. Interannual variation in maize yield and yield-related properties for all treatments was not significant in 2019 and 2020.
The LER for all the intercropping treatments ranged from 0.88 to 1.07. LER for maize–peanut intercropping in 2019 and 2020 were 1.02 and 0.98. In 2019, rotating the planting strip increased the LER by 2.94%, but in 2020 it increased the LER by 9.18% by increasing the peanut yield in intercropped border row. A 40% N reduction for maize can reduce the LER of intercropping below 1 by decreasing the maize yield. Significant reductions in LER were observed in maize–peanut intercropping compared to 2019, while this was not found in maize–peanut rotation–intercropping.
4 Discussion
4.1 The photosynthetic responses of peanut adaptation for intercropping
The degree of interspecific competition between different crops affects the morphology and yield formation of crops within the intercropping systems (Lv et al., 2014). In this study, there are two primary factors that influence the photosynthesis of intercropped peanut, one is an increase in soil N in the intercropped peanut planting strip caused by top dressing in the maize planting strips (Figure 2), and the second is light disadvantage (Table 1) by tall intercropped maize. Videlicet, the increase in soil nitrogen for intercropped peanuts cannot compensate for the loss of light. A significant reduction was measured for incident light interception and Pn in intercropped peanut (Figure 3). In addition, the Ci of intercropped peanut were also found to be significantly lower, which also indicates that lower CO2 concentrations were also important in reducing the effects of photosynthesis in intercropped peanuts. This may be attributable to tall intercropped maize affecting air movement (especially CO2), especially for C3 crops such as peanut with a low affinity of Rubisco for CO2 (von Caemmerer and Quick, 2000).
Plants are highly light-adapted, and changes in the light conditions received by the intercropping population under intercropping systems have led to significant changes in the photosynthetic character of the crop. In general, plant leaves grown in shade condition was thinner and large, had a thinner fence tissue thickness and chloroplast (Terashima et al., 2006; Gong et al., 2015), the more light-harvesting complexes per unit, a lower CO2 assimilation rate saturated (Huang et al., 2011; Zhu et al., 2012). The light response curve can reflect the photosynthetic character of plants. According to Yao et al. (2017), the intercropped soybean (Glycine max L.) showed a 16.91%, 40.00%, and 9.62% reduction in Pnmax, Icomp, and Isat, and a 30.41% increase in Rd compared to the sole soybean. In this study, similar results for Icomp, Isat, and Rd were obtained for intercropped peanut, but not the Pnmax (Table 1). This suggests that the maximum photosynthetic potential of peanut is not inhibited, which would be different from soybean. Previous research has shown that Peanuts are extremely sensitive to soil Fe content. A shortage of Fe drastically disrupted photosynthesis, and caused a marked reduction in small and large subunits of Rubisco content, chlorophyll a/b-binding proteins content, and RNA synthesis in leaf (Abadía, 1992; Winder and Nishio, 1995). Therefore, we suspected that the absence of a decrease in Pnmax in intercropped peanut is attributed to the improvement in iron uptake caused by the low Fe requirement of intercropped maize than peanut (Zuo et al., 2000).
Rubisco is the key enzyme to determine the direction and efficiency of photosynthetic carbon metabolism in plants (Andersson and Backlund, 2008), and the initial and total Rubisco activity are widely used to estimate both in vivo activation state and full carboxylation potential (Paulilo et al., 1994; Parry et al., 1997). In past reports, researchers have confirmed that the Rubisco activity is significantly affected by changes in light and decreases with the reduction in light availability (Paulilo et al., 1994; Andersson and Backlund, 2008). In this study, intercropped peanuts showed a significant reduction in carboxylation rates under light stress, but still maintained a strong full carboxylation potential (Figures 3, 4), which is also consistent with the low Pn and constant Pnmax. Intercropped peanuts showed substantial potential to increase yield. The higher carbon sequestration potential of photosynthetic enzymes in intercropped peanut is also reported in the protein analysis of Xiong et al. (2013). The low Rubisco activation state of intercropped peanut was due to a decrease in RCA activity caused by light stress (Butz and Sharkey, 1989; Andersson and Backlund, 2008), while RCA is necessary for catalytic Rubisco (Parry et al., 1997).
During photosynthesis, the function of the photosystem II (PSII) reaction center is crucial for limiting light energy use and the proper functioning of photosynthesis, and its activity is particularly sensitive to light stress (Schreiber et al., 1995). Compared with sole peanut, intercropped peanuts had higher electron transport efficiency and effective quantum efficiency of PSII to adapt to less light. However, these light-adapted responses still could not compensate for the light inhibition, and the electron transport rate was significantly reduced. This is also an important reason for the reduction of the photosynthetic rate of intercropping peanut.
4.2 The photosynthetic responses of maize adaptation for cropping system
As a cereal crop with a high stem, high-water-consuming, deep roots system C4 crop, intercropped maize has always been in a dominant ecological position in the maize–peanut intercropping (Lv et al., 2014; Li et al., 2019). At the same time, in this study, intercropped maize was subjected to a long period of N stress after top dressing (Figure 2) (due to a soil N transfer from MPS to PPS). Obviously, the growth advantages received by intercropped maize can compensate for the soil N disadvantage, and the Pn and yield per plant of intercropped maize were significantly greater than those of sole maize (Figure 5). In the study of Lv et al. (2014), competition for nutrients was of more importance than the competition for light through root separation experiment in maize–soybean intercropping, which doesn’t correspond to our study. A possible explanation for this result is that maize growth was not subjected to sufficient N stress to affect growth in this study due to (i) the sufficient water and light advantages received by intercropped maize than sole maize can increase the uptake and use of soil N (Sims et al., 1998a; Good et al., 2004); (ii) a large amount of N lost through leaching and nitrous oxide in dry farming areas soil (Shaver et al., 2002; Gao et al., 2009); (iii) the underlying nutrient sharing by peanut with maize (Searle et al., 1981). Several scientists have claimed that intercellular CO2 concentration and stomatal conductance are correlated, but not in this study, which may be a response strategy for maize to avoid excessive transpiration in the face of water deficiency in arid agricultural areas. This also suggests that stomata are not a factor for the increased photosynthetic rate (Yang et al., 2017).
Increasing Pnmax and Icomp were common responses of many plants, including most crop species, to higher light, having the effect of improving the efficiency of carbon assimilation (Givnish et al., 2004; Pfanz et al., 2007). Similar results were found in intercropped maize. The higher photosynthetic rates and photosynthetic potential of intercropped maize compared to sole maize are mainly due to the high carboxylation rates, light energy conversation efficiency primary, and electron transport rate (Table 4). The carboxylation capacity of intercropped maize increased by increasing initial Rubisco activity rather than total Rubisco activity, and the Isat of intercropped maize was not found to increase, which may be due to the soil N content limitation.
4.3 The photosynthetic responses of peanut and maize adaptation after rotating planting strips
After harvest, leguminous crops leave a lot of root nodules and residues as organic N for the following crop (Fischer et al., 2002). Crops grown after legumes tend to have a high Pn and yield. Contrary to our hypothesis that there were no significant differences between intercropped maize after rotating the planting strips compared to maize in the non-rotating planting strips (Tables 3, 4). The most probable explanation is that organic N are consumed during the winter fallow period (McGuire et al., 1998). As this study was only carried out for three years, long-term maize–peanut rotation–intercropping is expected to improve soil quality and intercropped maize yields.
Peanut is highly susceptible to continuous cropping obstacles due to soil nutrient imbalance, allelopathy, microbial community imbalance and increased soil pathogenic microorganisms (Li et al., 2020). The main factor affecting the photosynthesis of intercropped peanut is the soil after the rotation of the planting strips because the growth of intercropped maize is not affected. After rotating the planting strips, in the second planting year (2019), the photosynthetic rate and yield of intercropped peanut did not change significantly (Table 1 and Figures 3, 5). But in the third planting year (2020), after rotating the planting strips, the Pnmax, Pn and yield per plant of intercropping border row peanut increased significantly. On the one hand, this indicated that intercropped fringe border peanut would suffer from a continuous cropping obstacle enough to affect Pn and yield in the third planting year, and on the other hand, it confirmed that rotating the planting strip in maize–peanut intercropping can enhance the resistance of peanuts to continuous cropping obstacles. The increase in Pn and yield are the consequence of the combined increase in carboxylation rates, electron transport rate, and electron transport efficiency (Tables 1, 2). In addition, continuous cropping obstacle primarily occurs in intercropped border row rather than in intercropping middle row, it also shows that better light radiation can alleviate the continuous crop barrier.
4.4 The photosynthetic responses of peanut and maize adaptation after N fertilizer reduction
N is an essential nutrient and in addition it is a basic input in dry farming. Generally, N fertilization helps reduce the intraspecific competition between intercrops (Zhang et al., 2021). To achieve the maximum economic benefit, N fertilizer rates need to be carefully adjusted (Parihar et al., 2017; Yong et al., 2018) In this study, N reduction did not affect the light interception in intercropped maize and peanut (Table 3), and the factor affecting crop growth was the change in soil N content. Similarly, although the N reduction was only in the maize planting strip, the peanut planting strip was necessarily subject to N stress due to N transport in the soil. However, in this study, no effect of this N stress on photosynthesis and yield of peanut was observed. The most probable explanation was the low soil N requirement of intercropped peanuts.
Maize is more sensitive to soil N stress. A 20% N reduction had no effect on the photosynthetic character, Rubisco-related enzyme activity, and yield of intercropped maize (Tables 3, 4 and Figures 3–5), indicating that maize was not under sufficient N stress to affect normal photosynthesis and production. This is attributed to: (i) a 20% N reduction had a small and short-term effect on soil N content and as not sufficient to affect photosynthesis in maize (Figure 2); (ii) the increased N uptake and utilization capacity of intercropped maize can compensate for the reduced soil N content (Sims et al., 1998a; Gao et al., 2009); (iii) intercropped maize can use the nitrogen fixed by peanuts. After a 40% N reduction, the N compensating and light utilization ability based on intercropping advantage was limited (Table 3). The reduction in photosynthesis in intercropped maize is a combination of reduced Gs and carboxylation capacity, light conversion capacity and electron transfer rate. It is normal that the synthesis of Rubisco requires the involvement of N (Andersson and Backlund, 2008). The increase of initial and total Rubisco activity in intercropped border row maize than in intercropped middle row was not found after 40% N reduction, but in the 20% N reduction and non-reduction treatments, which is important evidence that the rate of carboxylation in intercropped maize is more strongly N limited.
4.5 Yield under different planting patterns and N application rates
The main reason for farmers to implement intercropping is that it can improve land productivity with a small investment (Zhang et al., 2021). There is conflicting evidence regarding the advantages of the land-use advantage of maize–peanut intercropping (Feng et al., 2021). These apparent discrepancies may arise from the fact that the different studies were performed under different environmental conditions and border–row proportions (Wang et al., 2020; Feng et al., 2021). In our study, the LER for maize–peanut intercropping in 2019 and 2020 was 1.02 and 0.98, respectively, indicating that there are no benefits from land use efficiency in dry farming areas (Figure 5). The increase in maize yield was attributable to an increase in HGW and grain number per spike, while the decrease in peanut yield was mainly attributable to a decrease in HGW and an increase in the NBP.
After rotating the planting strip, intercropped border row yield increased significantly in maize–peanut rotation–intercropping than in maize–peanut intercropping due to an increase in hundred-grain weight (HGW) and blighted pods number per plant (NBP). This supports our conclusion that the rotation of the planting strip can alleviate the continuous crop barrier of peanuts in the maize–peanut intercropping. Although the increase in productivity of intercropped middle row peanut in maize–peanut rotation–intercropping did not reach significant levels (p ≤ 0.05), it was evident that yield per plant, HGW and NBP in the intercropped border rows peanut had also reached the level of sole peanut. This is also evidence of the alleviation of the continuous crop barrier in the intercropped middle row peanut. More importantly, in both 2019 and 2020, the LER for maize–peanut rotation–intercropping was greater than 1, showing a significant land-use advantage. A 20% N reduction had no effect on LER of maize–peanut rotation–intercropping in dry farming areas. A 40% N reduction in the maize planting strip reduced the LER to less than 1, and decreased by 8.33% in 2020 compared with 2019, owing to lower HGW and grain number per spike of intercropped maize. The lower LER in 2020 can be explained by excessively reduced N application leading to a long-term lower N input to farmland than N output, resulting in lower soil farm fertility. Hereby, to improve photosynthetic production capacity and economic benefits in dry farming areas, first of all, optimize cropping patterns and choose maize–peanut rotation–intercropping to improve the photosynthetic production capacity of maize and alleviate the continuous crop barriers of peanut; secondly, reduce nitrogen without affecting maize growth.
5 Conclusions
In maize–peanut intercropping, the intercropped peanut adapts to light inhibition by decreasing light saturation point (Isat), reducing light compensation point (Icomp), and increasing electron transport efficiency, but exhibits low intercellular CO2 concentration (Ci), carboxylation rates, and electron transport rate, resulting in lower hundred-grain weight (HGW) and higher blighted pods per plant. For intercropped maize, Ci, electron transfer rate, electron transfer rate, Pn, maximum photosynthetic rate (Pnmax) and Icomp increased simultaneously, resulting in higher HGW and grain number per spike. But there was no land-use advantage to maize-peanut intercropping in dry farming areas. Rotating the planting strips in maize–peanut intercropping (maize–peanut rotation–intercropping) had no effect on intercropped maize, but mitigated succession barriers in intercropped border peanut through increased carboxylation rates, electron transport rate and transport efficiency, which in turn leads to an increase in the yield of intercropped peanuts per plant and an improvement in farmland land use efficiency. In maize–peanut rotation–intercropping, a moderate N reduction has no significant effect on photosynthetic capacity and yield of peanuts and maize, but excessive N reduction decreased the carboxylation rates, photoconversion ability, electron transport rate and Pnmax in intercropped maize, leading to a significant reduction in Pn, HGW and grain number per spike, as well as land equivalent ratio. Therefore, maize–peanut rotation–intercropping with N reduction for maize can be recommended as an excellent cropping system to improve agricultural productivity in dry farming areas. The method and results of this study can be generalized to provide guidance to the optimization of cropping systems in dry farming areas.
Data availability statement
The original contributions presented in the study are included in the article/Supplementary Material, further inquiries can be directed to aGFuZmVpeWFuemhvdUAxNjMuY29t.
Author contributions
XC and XR: Conceptualization. SG: Methodology. SW: software. XC and XR: Validation. RG and SG: Formal analysis. FH and ZJ: Investigation. TC and PZ: Resources. FH: Data curation. FH: Writing-Original draft preparation. XC, XR, SH, TJ and PS: Writing-Reviewing and Editing. XC and XR. Project administration. BP and PS: Funding acquisition. All authors have read and agreed to the published version of the manuscript.
Funding
We thank the Program of Shaanxi Province Key R&D Program (No. 2021NY-073 and No. 2022NY-196), National Natural Science Foundation of China (No. 31871580, 31871562), Key R&D program of Ningxia Hui Autonomous Region (No. 2019BBF03011) for generous financial support. We also thanks Researchers Supporting Project Number (RSP2022R410), King Saud University, Riyadh, Saudi Arabia for funding. The Article Processing Charge is financed by Wroclaw University of Environmental and Life Science. The Tax Idenfication Number (NIP) is PL8960005354.
Acknowledgments
The authors would like to extend their sincere appreciation to the Researchers Supporting Project Number (RSP2022R410), King Saud University, Riyadh, Saudi Arabia.
Conflict of interest
The authors declare that the research was conducted in the absence of any commercial or financial relationships that could be construed as a potential conflict of interest.
Publisher’s note
All claims expressed in this article are solely those of the authors and do not necessarily represent those of their affiliated organizations, or those of the publisher, the editors and the reviewers. Any product that may be evaluated in this article, or claim that may be made by its manufacturer, is not guaranteed or endorsed by the publisher.
Supplementary material
The Supplementary Material for this article can be found online at: https://www.frontiersin.org/articles/10.3389/fpls.2022.1014631/full#supplementary-material
References
Abadía, J. (1992). Leaf responses to fe deficiency: A review. J. Plant Nutr. 15 (10), 1699–1713. doi: 10.1080/01904169209364432
Andersson, I., Backlund, A. (2008). Structure and function of rubisco. Plant Physiol. Biochem. 46 (3), 275–291. doi: 10.1016/j.plaphy.2008.01.001
Bremner, J. M., Mulvaney, C. S. (1996). “Nitrogen total,” in Methods of soil analysis, part 3: Chemical methods. Eds. Sparks, D. L., Page, A. L., Helmke, P. A., Loeppert, R. H., Soltanpour, P. N., Tabatabai, M. A., Johnston, C. T., Sumner, M. E. (Madison, WI: Inc. Am. Soc. Agron., Inc.), 1085–1121.
Butz, N. D., Sharkey, T. D. (1989). Activity ratios of ribulose-1, 5-bisphosphate carboxylase accurately reflect carbam.ylation ratios. Plant Physiol. 89 (3), 735–739. doi: 10.1104/pp.89.3.735
Chai, Q., Gan, Y., Zhao, C., Xu, H. L., Waskom, R. M., Niu, Y., et al. (2016). Regulated deficit irrigation for crop production under drought stress. A. review. Agron. Sustain. Dev. 36 (1), 1–21. doi: 10.1007/s13593-015-0338-6
Coruzzi, G., Bush, D. R. (2001). Nitrogen and carbon nutrient and metabolite signaling in plants. Plant Physiol. 125 (1), 61–64. doi: 10.1104/pp.125.1.61
Escalona, J. M., Tomàs, M., Martorell, S., Medrano, H., Ribas-Carbo, M., Flexas, J. (2012). Carbon balance in grapevines under different soil water supply: importance of whole plant respiration. Aust. J. Grape Wine Res. 18 (3), 308–318. doi: 10.1111/j.1755-0238.2012.00193.x
Feng, C., Sun, Z., Zhang, L., Feng, L., Zheng, J., Bai, W., et al. (2021). Maize/peanut intercropping increases land productivity: A meta-analysis. Field Crop Res. 270, 108208. doi: 10.1016/j.fcr.2021.108208
Fischer, R. A., Santiveri, F., Vidal, I. R. (2002). Crop rotation, tillage and crop residue management for wheat and maize in the sub-humid tropical highlands: I. wheat and legume performance. Field Crop Res. 79 (2-3), 107–122. doi: 10.1016/S0378-4290(02)00157-0
Flexas, J., Bota, J., Galmes, J., Medrano, H., Ribas-Carbó, M. (2006). Keeping a positive carbon balance under adverse conditions: responses of photosynthesis and respiration to water stress. Physiol. Plant 127 (3), 343–352. doi: 10.1111/j.1399-3054.2006.00621.x
Gao, Y., Li, Y., Zhang, J., Liu, W., Dang, Z., Cao, W., et al. (2009). Effects of mulch, n fertilizer, and plant density on wheat yield, wheat nitrogen uptake, and residual soil nitrate in a dryland area of China. Nutr. Cycl. Agroecosyst. 85 (2), 109–121. doi: 10.1007/s10705-009-9252-0
Gianfreda, L., Rao, M. A., Piotrowska, A., Palumbo, G., Colombo, C. (2005). Soil enzyme activities as affected by anthropogenic alterations: intensive agricultural practices and organic pollution. Sci. Total. Environ. 341 (1-3), 265–279. doi: 10.1016/j.scitotenv.2004.10.005
Givnish, T. J., Montgomery, R. A., Goldstein, G. (2004). Adaptive radiation of photosynthetic physiology in the Hawaiian lobeliads: light regimes, static light responses, and whole-plant compensation points. Am. J. Bot. 91 (2), 228–246. doi: 10.3732/ajb.91.2.228
Gong, W. Z., Jiang, C. D., Wu, Y. S., Chen, H. H., Liu, W. Y., Yang, W. Y. (2015). Tolerance vs. avoidance: Two strategies of soybean (Glycine max) seedlings in response to shade in intercropping. Photosynthetica 53 (2), 259–268. doi: 10.1007/s11099-015-0103-8
Good, A. G., Shrawat, A. K., Muench, D. G. (2004). Sources and fates of nitrogen in plants and the environment. Trends Plant Sci. 12 (9), 597–605. doi: 10.1016/j.tplants.2004.10.008
Gozubuyuk, Z., Sahin, U., Ozturk, I., Celik, A., Adiguzel, M. C. (2014). Tillage effects on certain physical and hydraulic properties of a loamy soil under a crop rotation in a semi-arid region with a cool climate. Catena 118, 195–205. doi: 10.1016/j.catena.2014.01.006
Guo, F., Wang, M., Si, T., Wang, Y., Zhao, H., Zhang, X., et al. (2021). Maize-peanut intercropping led to an optimization of soil from the perspective of soil microorganism. Arch. Agron. Soil Sci. 67 (14), 1986–1999. doi: 10.1080/03650340.2020.1818725
Huang, D., Wu, L., Chen, J. R., Dong, L. (2011). Morphological plasticity, photosynthesis and chlorophyll fluorescence of Athyrium pachyphlebium at different shade levels. Photosynthetica. 49 (4), 611–618. doi: 10.1007/s11099-011-0076-1
Jiang, L., Deng, X., Seto, K. C. (2013). The impact of urban expansion on agricultural land use intensity in China. Land. Use Pol. 35, 33–39.
Kakraliya, S. K., Singh, U., Bohra, A., Choudhary, K. K., Kumar, S., Meena, R. S., et al. (2018). “Nitrogen and legumes: a meta-analysis,” in Legumes for soil health and sustainable management, 277–314. doi: 10.1007/978-981-13-0253-4_9
Kermah, M., Franke, A. C., Adjei-Nsiah, S., Ahiabor, B. D., Abaidoo, R. C., Giller, K. E. (2017). Maize-grain legume intercropping for enhanced resource use efficiency and crop productivity in the Guinea savanna of northern Ghana. Field Crop Res. 213, 38–50. doi: 10.1016/j.fcr.2017.07.008
Li, Y. H., Shi, D. Y., Li, G. H., Zhao, B., Zhang, L. W., Liu, P., et al. (2019). Maize/peanut intercropping increases photosynthetic characteristics, 13C-photosynthate distribution, and grain yield of summer maize. J. Integr. Agric. 18 (10), 2219–2229. doi: 10.1016/S2095-3119(19)62616-X
Li, Z., Xiong, F., Guo, W., Mo, C., Wu, H., Du, L. (2020). The root transcriptome analyses of peanut wild species Arachis correntina (Burkart) krapov. & WC Gregory and cultivated variety xiaobaisha in response to benzoic acid and p-cumaric acid stress. Genet. Resour. Crop Evol. 67 (1), 9–20. doi: 10.1007/s10722-019-00859-6
Li, Y. Y., Yu, C. B., Cheng, X., Li, C. J., Sun, J. H., Zhang, F. S., et al. (2009). Intercropping alleviates the inhibitory effect of n fertilization on nodulation and symbiotic N2 fixation of faba bean. Plant Soil. 323 (1), 295–308. doi: 10.1007/s11104-009-9938-8
Lv, Y., Francis, C., Wu, P., Chen, X., Zhao, X. (2014). Maize–soybean intercropping interactions above and below ground. Crop Sci. 54 (3), 914–922. doi: 10.2135/cropsci2013.06.0403
McGuire, A. M., Bryant, D. C., Denison, R. F. (1998). Wheat yields, nitrogen uptake, and soil moisture following winter legume cover crop vs. fallow. Agron. J. 90 (3), 404–410. doi: 10.2134/agronj1998.00021962009000030015x
Mrabet, R., Saber, N., El-Brahli, A., Lahlou, S., Bessam, F. (2001). Total, particulate organic matter and structural stability of a calcixeroll soil under different wheat rotations and tillage systems in a semiarid area of Morocco. Soil Tillage. Res. 57 (4), 225–235. doi: 10.1016/S0167-1987(00)00180-X
Nittaya, C., Amnat, C., Kazuyuki, Y., Shigeto, S., Sirintornthep, T. (2017). Greenhouse gas emissions, soil carbon sequestration and crop yields in a rain-fed rice field with crop rotation management. Agron. Sustain. Dev. 237, 109–120. doi: 10.1016/j.agee.2016.12.025
Parihar, C. M., Jat, S. L., Singh, A. K., Ghosh, A., Rathore, N. S., Kumar, B., et al. (2017). Effects of precision conservation agriculture in a maize-wheat-mungbean rotation on crop yield, water-use and radiation conversion under a semiarid agro-ecosystem. Agric. Water Manage. 192, 306–319. doi: 10.1016/j.agwat.2017.07.021
Parry, M. A. J., Andralojc, P. J., Parmar, S., Keys, A. J., Habash, D., Paul, M. J., et al. (1997). Regulation of rubisco by inhibitors in the light. Plant Cell Environ. 20 (4), 528–534. doi: 10.1046/j.1365-3040.1997.d01-85.x
Paulilo, M. T. S., Besford, R. T., Wilkins, D. (1994). Rubisco and PEP carboxylase responses to changing irradiance in a Brazilian cerrado tree species, qualea grandiflora mart. (Vochysiaceae). Tree Physiol. 14 (2), 165–177. doi: 10.1093/treephys/14.2.165
Pfanz, H., Vodnik, D., Wittmann, C., Aschan, G., Batic, F., Turk, B., et al. (2007). Photosynthetic performance (CO2-compensation point, carboxylation efficiency, and net photosynthesis) of timothy grass (Phleum pratense l.) is affected by elevated carbon dioxide in post-volcanic mofette areas. Environ. Exp. Bot. 61 (1), 41–48. doi: 10.1016/j.envexpbot.2007.02.008
Rahman, N. A., Larbi, A., Kotu, B., Asante, M. O., Akakpo, D. B., Bedi, S. M., et al. (2021). Maize–legume strip cropping effect on productivity, income, and income risk of farmers in northern Ghana. Agron. J., 1–12. doi: 10.1002/agj2.20536
Schreiber, U. B. W. N., Bilger, W., Neubauer, C. (1995). “Chlorophyll fluorescence as a nonintrusive indicator for rapid assessment of in vivo photosynthesis,” in Ecophysiology of photosynthesis, vol. 100. (Berlin, Heidelberg: Springer), 49–70. doi: 10.1007/978-3-642-79354-7_3
Searle, P. G. E., Comudom, Y., Shedden, D. C., Nance, R. A. (1981). Effect of maize+ legume intercropping systems and fertilizer nitrogen on crop yielding and residual nitrogen. Field Crop Res. 4, 133–145. doi: 10.1016/0378-4290(81)90063-0
Shaver, T. M., Peterson, G. A., Ahuja, L. R., Westfall, D. G., Sherrod, L. A., Dunn, G. (2002). Surface soil physical properties after twelve years of dryland no-till management. Soil Sci. Soc Am. J. 66 (4), 1296–1303. doi: 10.2136/sssaj2002.1296
Sims, D. A., Luo, Y., Seemann, J. R. (1998a). Comparison of photosynthetic acclimation to elevated CO2 and limited nitrogen supply in soybean. Plant Cell Environ. 21 (9), 945–952. doi: 10.1046/j.1365-3040.1998.00334.x
Sims, D. A., Seemann, J. R., Luo, Y. (1998b). The significance of differences in the mechanisms of photosynthetic acclimation to light, nitrogen and CO2 for return on investment in leaves. Funct. Ecol. 12 (2), 185–194. doi: 10.1046/j.1365-2435.1998.00194.x
Stagnari, F., Maggio, A., Galieni, A., Pisante, M. (2017). Multiple benefits of legumes for agriculture sustainability. Chem. Biol. Technol. Agric. 4 (1), 1–13. doi: 10.1186/s40538-016-0085-1
Terashima, I., Hanba, Y. T., Tazoe, Y., Vyas, P., Yano, S. (2006). Irradiance and phenotype: comparative eco-development of sun and shade leaves in relation to photosynthetic CO2 diffusion. J. Exp. Bot. 57 (2), 343–354. doi: 10.1093/jxb/erj014
von Caemmerer, S., Quick, W. P. (2000). Rubisco: Physiology in vivo. Photosynthesis 9, 85–113. doi: 10.1007/0-306-48137-5_4
Wang, R., Sun, Z., Zhang, L., Yang, N., Feng, L., Bai, W., et al. (2020). Border–row proportion determines strength of interspecific interactions and crop yields in maize/peanut strip intercropping. Field Crop Res. 253, 107819. doi: 10.1016/j.fcr.2020.107819
Willey, R. W. (1979). Intercropping-its importance and research needs. Part I. Competition and yield advantages. Field Crop Abstr. 31(1), 1–10.
Winder, T. L., Nishio, J. N. (1995). Early iron deficiency stress response in leaves of sugar beet. Plant Physiol. 108 (4), 1487–1494. doi: 10.1104/pp.108.4.1487
Wu, Y., Shan, L., Guo, Z., Peng, Y. (2017). Cultivated land protection policies in China facing 2030: Dynamic balance system versus basic farmland zoning. Habitat. Int. 69, 126–138. doi: 10.1016/j.habitatint.2017.09.002
Xiong, H., Shen, H., Zhang, L., Zhang, Y., Guo, X., Wang, P., et al. (2013). Comparative proteomic analysis for assessment of the ecological significance of maize and peanut intercropping. J. Proteomics 78, 447–460. doi: 10.1016/j.jprot.2012.10.013
Yang, H., Li, X. (2000). Cultivated land and food supply in China. Land. Use Pol. 17 (2), 73–88. doi: 10.1016/S0264-8377(00)00008-9
Yang, F., Liao, D., Wu, X., Gao, R., Fan, Y., Raza, M. A., et al. (2017). Effect of aboveground and belowground interactions on the intercrop yields in maize-soybean relay intercropping systems. Field Crop Res. 203, 16–23. doi: 10.1016/j.fcr.2016.12.007
Yan, H., Liu, F., Liu, J., Xiao, X., Qin, Y. (2017). Status of land use intensity in China and its impacts on land carrying capacity. J. Geograph. Sci. 27 (4), 387–402. doi: 10.1007/s11442-017-1383-7
Yao, X., Zhou, H., Zhu, Q., Li, C., Zhang, H., Wu, J. J., et al. (2017). Photosynthetic response of soybean leaf to wide light-fluctuation in maize-soybean intercropping system. Front. Plant Sci. 8. doi: 10.3389/fpls.2017.01695
Ye, Z. P. (2007). A new model for relationship between irradiance and the rate of photosynthesis in Oryza sativa. Photosynthetica 45 (4), 637–640. doi: 10.1007/s11099-007-0110-5
Ye, Z. P., Suggett, D. J., Robakowski, P., Kang, H. J. (2013). A mechanistic model for the photosynthesis–light response based on the photosynthetic electron transport of photosystem II in C3 and C4 species. New Phytol. 199 (1), 110–120. doi: 10.1111/nph.12242
Yong, T. W., Chen, P., Dong, Q., Yang, F., Wang, X. C., Liu, W. G., et al. (2018). Optimized nitrogen application methods to improve nitrogen use efficiency and nodule nitrogen fixation in a maize-soybean relay intercropping system. J. Integr. Agric. 17 (3), 664–676. doi: 10.1016/S2095-3119(17)61836-7
Yu, B., Lu, C. (2006). Change of cultivated land and its implications on food security in China. Chin. Geograph. Sci. 16 (4), 299–305. doi: 10.1007/s11769-006-0299-4
Zhang, W. P., Gao, S. N., Li, Z. X., Xu, H. S., Yang, H., Yang, X., et al. (2021). Shifts from complementarity to selection effects maintain high productivity in maize/legume intercropping systems. J. Appl. Ecol. 58 (11), 2603–2613.
Zhao, C., Wang, G. (2002). Effects of drought stress on the photoprotection in ammopiptanthus mongolicus leaves. J Integr Plant Biol. 44, 1309–1313. Available at: https://www.jipb.net/EN/Y2002/V44/I11/1309.
Zhu, T. T., Li, J., Liu, Y. Y., Tong, X. J., Yu, Q. (2020). Leaf photosynthetic light response of summer maize: comparison of models and analysis of parameters. Photosynthetica. 58 (1). doi: 10.32615/ps.2019.134
Zhu, J. J., Qiang, P., Liang, Y. L., Wu, X., Hao, W. L. (2012). Leaf gas exchange, chlorophyll fluorescence, and fruit yield in hot pepper (Capsicum anmuum l.) grown under different shade and soil moisture during the fruit growth stage. J. Integr. Agric. 11 (6), 927–937. doi: 10.1016/S2095-3119(12)60083-5
Keywords: dry farming areas, maize-peanut intercropping, rotation of crop planting strip, N reducing, light adaptation
Citation: Han F, Guo S, Wei S, Guo R, Cai T, Zhang P, Jia Z, Hussain S, Javed T, Chen XL, Ren X, Al-Sadoon MK and Stępień P (2022) Photosynthetic and yield responses of rotating planting strips and reducing nitrogen fertilizer application in maize–peanut intercropping in dry farming areas. Front. Plant Sci. 13:1014631. doi: 10.3389/fpls.2022.1014631
Received: 09 August 2022; Accepted: 23 September 2022;
Published: 16 November 2022.
Edited by:
Min Huang, Hunan Agricultural University, ChinaReviewed by:
Baizhao Ren, Shandong Agricultural University, ChinaEnke Liu, Institute of Environment and Sustainable Development in Agriculture (CAAS), China
Zhuzhu Luo, Gansu Agricultural University, China
Copyright © 2022 Han, Guo, Wei, Guo, Cai, Zhang, Jia, Hussain, Javed, Chen, Ren, Al-Sadoon and Stępień. This is an open-access article distributed under the terms of the Creative Commons Attribution License (CC BY). The use, distribution or reproduction in other forums is permitted, provided the original author(s) and the copyright owner(s) are credited and that the original publication in this journal is cited, in accordance with accepted academic practice. No use, distribution or reproduction is permitted which does not comply with these terms.
*Correspondence: XiaoLi Chen, Y3hscnhsQGFsaXl1bi5jb20=; Xiaolong Ren, cnhsY3hsQGFsaXl1bi5jb20=