- 1College of Enology, Northwest A&F University, Yangling, Shaanxi, China
- 2College of Life Science, Langfang Normal University, Langfang, Hebei, China
- 3Shaanxi Engineering Research Center for Viti-Viniculture, Yangling, Shaanxi, China
- 4China Wine Industry Technology Institute, Yinchuan, Ningxia, China
- 5Engineering Research Center for Viti-Viniculture, National Forestry and Grassland Administration, Yangling, Shaanxi, China
The goals of this work were to screen physiological and biochemical indexes to assess a set of V. vinifera germplasm resources, to compare evaluation methods for cold hardiness, and to establish a comprehensive method that can be used for more accurate screening for cold hardiness in V. vinifera. Four single methods were used to evaluate the cold hardiness of 20 germplasms resources and 18 physiological and biochemical indexes related to cold hardiness were determined. The LT50 values determined by electrical conductivity (EL), 2,3,5-triphenyltetrazolium chloride staining (TTC), differential thermal analysis (DTA), and recovery growth (RG) methods showed extremely significant positive correlation. Bound water content (BW), proline content (Pro), total soluble sugar content (TSS), malondialdehyde content (MDA), catalase content (CAT), and ascorbic acid content (ASA) exhibited significant correlation with LT50 values measured by different evaluation methods. The comprehensive cold hardiness index calculated by principal component analysis (PCA) combined with subordinate function (SF) was negatively correlated with LT50 values measured by different evaluation methods. Meili and Ecolly exhibited the highest cold hardiness, indicating their potential for use as parents for cold hardiness breeding. EL, DTA, TTC, and RG methods successfully distinguished cold hardiness among different V. vinifera germplasm lines. Measurements of BW, Pro, TSS, MDA, CAT, and ASA in dormant shoots also can be used as main physiological and biochemical indexes related to cold hardiness of V. vinifera. Comprehensive evaluation by PCA combined with SF can accurately screen cold hardiness in V. vinifera. This study provides a reference and accurate identification method for the selection of cold hardiness parents and the evaluation of cold hardiness of germplasm of V. vinifera.
1 Introduction
As a main cultivar, V. vinifera grapes have extremely high economic value and are used to produce grapes consumed fresh or dried or used to make wine or juice. V. vinifera varieties are often used for as starting material to generate improved varieties and as parent materials for high-quality hardiness breeding (Wang et al., 2021b). However, the mature shoots of V. vinifera can only tolerate a low temperature of about -15°C during winter dormancy, so in some cold regions, such as northern China and the Russian far East, soil-burial over-wintering of vines has become the main cultivation mode of V. vinifera (Wang et al., 2021b). Although effective, this practice is labor intensive, thus restricts the sustainable development of viticulture (Han et al., 2021). Therefore, it is important to breed cold-resistant new varieties that can be cultivated in these regions without the requirement for vine burial during winter. To successfully breed cold resistant varieties, effective methods are required to evaluate cold hardiness.
There have been many studies of the physiological and biochemical aspects of grapevine cold hardiness and different methods have been proposed to evaluate cold hardiness (Avanci et al., 2010; Jeon et al., 2010; Jin et al., 2012; Zhang F. et al., 2012; Hu et al., 2013). The classical evaluation method of grapevine cold hardiness uses electrical conductivity (EL) to measure the electrolyte leakage of shoot tissues at low temperatures (Sutinen et al., 1992; Elisa et al., 2009; Wang et al., 2021b). The semi-lethal temperature (LT50) can then be calculated from these measurements using the logistic equation (Elisa et al., 2009). Another technique, differential thermal analysis (DTA), detects and records the exothermic process at low temperature, and then analyzes and evaluates the cold hardiness of plant tissues (Chai et al., 2015; Kaya and Köse, 2017; Londo and Kovaleski, 2017; Muhammed and Cafer, 2021). The DTA method has been applied to the study of cold hardiness of grape shoots, roots, and buds (Chen et al., 2020a). The tissue browning method is another objective and reliable method used to study cold hardiness of grapevine (Niu and He, 1993). In this method, the browning of shoot slices is observed under a microscope after freezing and classifying freezing damage based on the browning area of secondary xylem (Niu and He, 1993). Another method uses 2,3,5-Triphenyltetrazolium chloride (TTC) staining to measure tissue viability and cold hardiness of plants (Thomas and Ahlers, 2010). Zhao et al. successfully identified the cold hardiness of V. amurensis using TTC staining index and logistic equation (Zhao et al., 2018). Restoration growth (RG) may be the most intuitive and reliable method of cold hardiness measurement of grapevine as it directly observes the germination, rooting, and callus production rates after low temperature treatment (Fennell, 2004; Chen et al., 2014; Liu Z. et al., 2021).
There are many V. vinifera cultivars and the cold hardiness of different varieties varies, with few V. vinifera varieties are suitable for cultivation in any specific country or region (Zhan and Li, 2010; Wang et al., 2021b). Most research on V. vinifera has focused on cultivation management (Ju et al., 2019; Yue et al., 2020; Wang et al., 2021a; Yue et al., 2021), fruit quality regulation (Bohan et al., 2020; Li et al., 2021; Yang N. et al., 2021), wine-making characteristics (Sun et al., 2021; Wei et al., 2022), grape and wine nutrition (Cheng et al., 2021; Xu et al., 2021; Yang C. et al., 2021), and hardiness improvement (Liu R. et al., 2021; Wan et al., 2021; Wang X. et al., 2021; Wang et al., 2021c; Wang et al., 2022), but there has been little comprehensive work to determine the most appropriate methods for the evaluation of cold hardiness in V. vinifera. Most methods used thus far to investigate cold hardiness are relatively simple methods that are not targeted, so the results obtained using different cold hardiness evaluation methods will be different (He, 2015). This lack of comprehensive and effective evaluation limits the full utilization of V. vinifera germplasm resources.
The goal of this study was to test the effectiveness of four cold hardiness evaluation methods to screen the cold hardiness of 20 V. vinifera lines. The correlations between various related physiological and biochemical indexes and cold hardiness were analyzed and indexes of cold hardiness were screened, allowing the establishment of a comprehensive evaluation method for cold hardiness in V. vinifera. The results will facilitate the identification of cold hardiness to screen existing varieties for cultivation in specific regions and for the breeding of improved varieties.
2 Materials and methods
2.1 Materials and experimental field
Shoots of experimental materials (20 V. vinifera grape varieties) were sampled from an experimental vineyard of the Northwest Agriculture and Forestry University (NWAFU) located in Yangling of Shanxi Province (lat. 34°N, long. 108°E), China. This area has a semiarid continental monsoon climate, and the soil type is bauxite. Self-rooted vines of V. vinifera. were planted in 2013. Vine rows were oriented west - east, with vines spaced in 1.0 × 2.5 m rows. The vines were cordon-trained and pruned to two buds per spur. All viticultural practices were performed according to local standards.
2.2 Experimental design
Sampling was conducted on January 10, 2021, and 35 vines of each variety with good growth condition were randomly selected. Ten dormant shoots (one-year-old shoots, the number of dormant buds on each shoot is not less than six) were collected for a total of 350 shoots collected from each variety. The collected shoots were rinsed three times with tap water, three times with deionized water, and then the water was adsorbed using filter paper. The shoots were divided in seven plastic bags, with fifty shoots for each variety per bag. Six bags were placed in a chamber with controlled temperature and humidity for freeze-thaw treatment for evaluation of cold hardiness by EL, TTC, and RG methods. By EL and TTC methods, the amounts of shoots treated at each temperature was nine, including three biological replicates and three technical replicates; by the RG method, the amounts of dormant buds treated at each temperature was 90, including three biological replicates and three technical replicates. Samples were freeze-thaw treated in accordance with the method of Zhang et al., with slight modifications (Zhang et al., 2013). Six temperatures were used: 4°C (control temperature treatment), -10°C, -14°C, -18°C, -22°C, and -26°C for the six treatment groups. The freezing treatment lasted 12 h and the recovery period was 4 h at room temperature to thaw the samples. The cooling and heating rates were both set at 4°C.h-1. A sample of fifty shoots for each variety was stored in a refrigerator at 4°C and used as test materials were used to evaluate cold hardiness by DTA method and to determine physiological and biochemical indexes related to cold hardiness. by the DTA method, the amounts of one-year-old shoots and dormant buds were performed 54 points, including three biological replicates and three technical replicates.
2.3 Evaluation of cold hardiness
2.3.1 Evaluating cold hardiness by EL
EL was performed as described by Wang et al. (2021b). Take three shoots of each variety were prepared for each freezing temperature, remove the epidermis, avoid the buds, select the stems and cut them into 1∽2 mm slices and mix evenly. Samples weighing 2 g were transferred into a 25 ml test tube with a stopper, and then 20 mL deionized water was added and shaken well. This was done three times for each treatment. After shaking for 12 hours in a shaker, a conductivity meter (DDS‐11C, Shanghai optical instrument fac‐ tory, Shanghai, China) was used to determine the initial conductivity value. Each test tube was then boiled for 40 min, allowed to stand for 2 hours, and then the final conductivity value was determined. Experiments were repeated three times with three biological replicates. The relative conductivity was calculated as follows. Relative conductivity (%) = (initial conductivity value/final conductivity value) × 100. LT50 value was calculated using the logistic regression equation: Y = K/(1 + ae−bx). Y is the relative conductivity, x is the processing temperature, and K is the maximum leakage (K = 100). In practical application: Y´ = ln [(K − Y)/Y]. Y´ = lna − bx, that is, the relative conductivity Y is converted into Y´, and the relation‐ ship between it and the processing temperature is expressed by linearity. The parameter a and b of the equation were obtained by linear regression. The inflection point temperature is the LT50.
2.3.2 Evaluating cold hardiness by DTA
Refer to the method of Kaya and Köse, with slight modifications (Kaya and Köse, 2017; Küpe and Köse, 2020). Nine shoots of each variety were randomly selected from the samples that have not been freeze-thaw treated for DTA. Temperature exotherms of shoots, including dormant buds and internodes of shoots, were determined by observing temperature recording for sudden temperature deflections from dormant buds and internodes of shoots. Platinum hardiness temperature needle (Model: PT100, Anwei Jujie Technology Co., Ltd., Wuhu, China) were inserted in the intact dormant buds and internodes of shoots and fixed with elastic band. Silicon grease was used to cover the thermocouple junction to obtain maximum heat transfer. Then the samples were wrapped with aluminium foil and placed in Dewar Flaks which was prechilled to 4°C. Dewar Flaks were taken into a Programmable Temperature & Humidity Chamber (Model: YSGJS-408, Shanghai Lanhao Instrument & Equipment Co., Ltd., Shanghai, China), equipped with a temperature controller, to achieve a constant cooling rate that was 4°C·h-1. Cooling started at 4°C in all freezing tests and ended at -26°C. Low temperature exotherms (LTEs) were identified from temperature data recorded at 2-s intervals using 48-channel data acquisition system, Data Acquisition System (Model: R8000, Anwei Jujie Technology Co., Ltd., Wuhu, China) in computer. As the temperature drops, an inflection point appears in the cooling curve. The starting point of the inflection point is the supercooling point, and the peak value is the freezing point. Lethal temperatures for shoot were expressed as LTE50 (the temperature of the median LTE’s), the temperatures at which 50% of dormant buds or internodes of shoots were killed. DTA was performed on 18 points (3 repeats, 6 points for each repeat) for an independent experiment between the dormant buds and internodes of shoots and repeated three independent experiments.
2.3.3 Evaluating cold hardiness by TTC
TTC was performed as described by Zhao et al. (2018). After the freeze-thaw treatment, 10 5-mm thick sections were cut from shoot internodes and placed in 10 mL 0.5% TTC in the dark in a 30°C incubator for 4 d. Then, the samples were dissected to obtain longitudinal sections, which were projected onto a LA2400 scanner having image analysis software (Win RHIZO™; Regent Instruments Inc. Quebec City, Quebec, Canada), and the areas stained by TTC on the longitudinal sections were measured. The level of cold injury for each shoot was recorded as one of five levels based on the area of staining of the whole longitudinal section. Level 1 = 0% – 5.0%, 2 = 5.1% – 40.0%, 3 = 40.1% – 70.0%, 4 = 70.1% – 90.0%, 5 = 90.1% – 100% staining area. Then, the staining index was calculated at related levels as follows: Staining index=Σ (staining level × shoot sections at the level)/(4 × total shoot sections). The LT50 value was determined using a logistic equation of staining indices. Experiments were repeated three times with three biological replicates.
2.3.4 Evaluating cold hardiness by RG
Refer to the method of Liu Z. et al. (2021) slightly modified. The shoots treated with different low temperatures were soaked in water at room temperature for 12 hours and cut into single-bud stem cuttings. The cross section of the cut was wrapped with plastic film to avoid water loss. The single-bud stem cuttings were inserted into the foam board, and the bottom cross section was exposed 1~3cm, which was put into the test box of 30*20*8 cm with tap water and cultured in the room. Cultured under the light intensity of 2000~3000 Lx for 12 h, in the dark for 12 h, the temperature was 25-28°C. Each variety is divided into 3 parts, each with 30 single-bud cuttings. The dynamics of shoots were observed every day, and the germination rate was counted after 30 days. The formula of germination rate: germination rate (%) = (number of budding shoots/number of cuttings) × 100%. According to the sprouting changes of single bud cuttings of different varieties under low temperature treatments, combined with logistic equation and to determine LT50.
2.4 Determination of physiological and biochemical indicators
2.4.1 Water contents
Total water (%, TW) in shoots was determined as (fresh weight -dry weight) *100, the dry weight after oven-drying at 90°C for 16 h until a constant weight. Free water (%, FW) was determined using a digital display saccharimeter in accordance with the method of Chen et al. (2014). Bound water (%, BW) = TW – FW. Three shoots (only one bud per shoot) were used for water measurements in each replication.
2.4.2 Osmoregulation substances
Samples of shoots (1.0 g) from all treatment groups were ground in a chilled mortar with 1% (w/v) polyvinylpolypyrrolidone, homogenized with 15 mL of 50 mM potassium phosphate buffer (pH 7.8), then centrifuged at 10,000 × g for 15min. The resulting supernatant was used for assays. Soluble protein content was determined by Coomassie brilliant blue method, using bovine serum albumin as a standard (Bradford, 1976). The protein content was detected at 525 nm. Proline content was measured based on the method of Bates et al. (1973). Shoot sample (0.2 g) was placed into 5 mL of aqueous sulfosalicylic acid (3%) and kept in a boiling water bath for 30 min. After the mixture was cooled to room temperature, 2.0 mL supernatant extract was mixed with 2.0 mL ninhydrin and 2 mL acetic acid. Then, the mixture was maintained for 30 min in a boiling water bath and cooled in an ice bath. Next, 5 mL of toluene was added, and the mixture was placed in the dark for 5 h. Readings of the colored product were then taken at 520 nm.
2.4.3 Carbohydrate contents
Collected shoot samples were dried to a constant weight and then crushed for the determination of sugar content. Samples of dry powder (0.5 g) were put into 10 mL centrifuge tubes, 8 mL of 80% ethanol solution was added, and extraction was performed for 30 min in a water bath at 80°C with constant stirring. The solution was cooled to room temperature and then centrifuged at 3500 × g for 10 min. The supernatant was transferred to a 25 mL volumetric flask and then 6 mL of 80% ethanol was added to the precipitate to repeat the extraction. The supernatants of three extractions were combined and assayed. Reducing sugar content was measured using the 3,5-dinitrosalicylic acid method to determine the absorbance at 520 nm and calculated based on the standard curve of glucose (Miller, 1959). The soluble sugar content was determined by anthrone colorimetry at 620 nm and calculated according to the standard curve of glucose (Yemm and Willis, 1954).
To measure the sucrose content, a sample (10 mL) of the reducing sugar extract was transferred to a 100 mL Erlenmeyer flask, mixed with 10 mL of 6 mol/L hydrochloric acid, boiled in a water bath for 10 min, titrated with 10% NaOH to neutrality after cooling, and then diluted to 50 mL with water to produce the sucrose extract. This extract was then subjected to the 3,5-dinitrosalicylic acid method to determine the sucrose content. After extracting the reducing sugar, the residue was transferred to a 100 mL Erlenmeyer flask, 10 mL 6 mol/L hydrochloric acid was added and mixed well before boiling in a water bath for 10-30 min (Gao, 2006). After cooling, 20 mL water was added and then the supernatant was filtered into a 50 mL volumetric flask. The residue was washed three times and filtered again, and then the volume was adjusted to 50 mL to obtain the starch extract. The extract was subjected to centrifugation at 4000 × g for 10 min and then the supernatant was diluted twice before determination of the starch content using the 3,5-dinitrosalicylic acid method, as was done to measure reducing sugar content (Gao, 2006).
2.4.4 Oxidative stress indices
Shoots (0.1 g) were ground in liquid nitrogen after removing the epidermis and buds, and extracted with 2ml of 5% (w/v) trichloroacetic acid, then centrifuged at 10,000 × g for 20 min. The supernatant was used for the assay of H2O2, as described by Patterson et al. (1984). Superoxide anions () production was estimated as described by Elstner and Heupel (Elstner and Heupel, 1976). After the sample (1 g) was added to the 65 mM phosphate buffer to polish, the mixture was centrifuged for 10 min at 10,000 × g before 10 mM hydroxylamine hydrochloride was heated for 20 min at 25°C. After 17 mM amino benzene sulfonamide acid α-naphthylamine was added, the reaction solution was placed in a water bath (30°C) for 30 min and then analyzed with a colorimetric spectrophotometer at 530 nm. Results were compared to standard curves. Malondialdehyde (MDA) content was determined by thiobarbituric acid-reactive substances methods (Hodges et al., 1999). Shoot sample (2.0 g) was homogenized in 15 mL 0.1% TCA and then centrifuged at 5,000 × g for 10min. Five milliliters of 5% TCA containing 0.5% TBA were added to 1 mL of the supernatant then incubated in boiling water for 10min. Then the reaction tubes were transferred to ice water to stop the reaction. MDA absorption was measured spectrophotometrically at 450, 532, and 600 nm.
2.4.5 Antioxidant enzymes
Samples of shoot tissues (0.1 g) from all tested varieties were ground in a chilled mortar with 2% (w/v) polyvinylpolypyrrolidone, homogenized with 10 mL of 50 mM potassium phosphate buffer (pH 7.8) containing1 mM EDTA-Na2 and 0.3% Triton X-100, then centrifuged at 12,000 × g for 20 min. The resulting supernatant was used for enzyme assays. Protein content was determined according to Bradford (Bradford, 1976), using bovine serum albumin as a standard. Superoxide dismutase (SOD; EC 1.15.1.1) activity was estimated by the method of Giannopolittis and Ries and was expressed as units/g FW min (Giannopolites and Ries, 1977). Catalase (CAT; EC 1.11.1.6) activity was evaluated according to Aebi and was expressed as units/g FW min (Aebi, 1984). Peroxidase (POD; EC 1.11.1.7) activity was measured using the method of Rao (1996) and was expressed as units/g FW s.
2.4.6 Antioxidant metabolites
Shoot sample (0.4 g) was ground with a mortar and pestle in 2 mL of 0.5 mM EDTA solution containing 3% trichloroacetic acid and centrifuged at 15,000 × g for 10 min at 4°C. The supernatant was used for assays of the levels of ascorbic acid (ASA) and glutathione (GSH). The amount of GSH was evaluated following the method of Monostori et al. and was expressed as mg/g FW (Monostori et al., 2009). The amount of ASA was estimated using the method of Foyer et al. and was expressed as mg/g FW (Foyer and Halliwell, 1976).
2.5 Statistical analysis
The standardized data for the substances measured were analyzed with SPSS 17.0 and processed using the subordinative function to evaluate the level of cold hardiness of the range of wild grape germplasm examined here. Evaluation of cold hardiness is based on the evaluation of the various subordinative function indices in the form,
(Positive correlation, including BW, Pro, TSS, CAT, and ASA.)
(Negative correlation: including MDA.)
Here, i is a particular accession, j is a particular index, Xij is the testing value of the index j of accession i, Xjmin is the minimum value of index j for all accessions, Xjmax is the maximum value of index j of all accessions, Uij is the SF value of accession i, and index j that relates to cold hardiness.
Microsoft Excel 2013 was used to record and process the original data. Origin 9.0 (OriginLab, Northampton, MA, USA) software was used to fit the logistic equation, and LT50 values were obtained. Heat Map with Dendrogram and Correlation Plot were performed using Origin 9.0 software. Descriptive statistics were analyzed via SPSS 17.0 (Statistical Product and Service Solutions, Inc., Chicago, IL, USA). Values correspond to the mean interval of three independent experiments. Principal component analysis and weight of cold hardiness were performed using SPSSAU, an online platform for data analysis (https://spssau.com).
3 Results
3.1 Evaluation of cold hardiness of V. vinifera by different methods
3.1.1 Evaluation of cold hardiness of V. vinifera based on EL
As shown in Table 1, the relative electrolyte leakage of one-year-old shoots of different varieties increased with the decrease of temperature. The LT50 values of the 20 tested V. vinifera varieties determined by EL ranged from -19.42°C ~ -11.07°C. Meili, Ecolly, Italian Riesling, and Riesling varieties had LT50 values below -17°C: -17.76°C, -19.42°C, -17.16°C, and -17.44°C, respectively. Merlot, Petit Verdot, Chardonnay, Sauvignon Blanc, Cabernet Sauvignon, Syrah, and Gewurztraminer varieties had LT50 values higher than -13°C: -11.08°C, -10.58°C, -12.28°C, -11.07°C, -12.23°C, -11.78°C, and -12.31°C, respectively. Granoir, Cabernet Sauvignon, Marselan, Dunkelfelder, Pinot Noir, Viognier, Petit Manseng, Yan73, and Ugni Blanc had LT50 values that ranged from -13°C to -17°C.
3.1.2 Evaluation of cold hardiness of V. vinifera based on DTA
DTA was also used to evaluate cold hardiness of the tested varieties. As shown in Table 2, Ecolly shoots (one-year-old shoots) and winter buds exhibited the lowest supercooling point and freezing point, -11.57°C and -10.97°C respectively for shoots, and -10.72°C and -10.02°C, respectively for winter buds. The shoots and winter buds of Dunkelfelder have the strongest supercooling ability. For shoots, the LT50 values for the 20 V. vinifera varieties determined by DTA ranged from -11.60°C ~ -8.20°C. Shoots of Meili, Ecolly, Italian Riesling, and Riesling exhibited LT50 values lower than -10°C, with -11.20°C, -11.60°C, -11.10°C and -10.10°C respectively. Shoots of Dunkelfelder, Merlot, Petit Verdot, Sauvignon Blanc, Cabernet Sauvignon, Syrah, and Gewurztraminer had LT50 values higher than -9°C: -8.20°C, -8.80°C, -8.70°C, -8.60°C, -8.40°C, -8.45°C and -8.35 °C, respectively. For buds, the LT50 values determined by DTA were in the range of -10.60°C ~ -6.70°C. Buds of Meili, Ecolly, Italian Riesling, Riesling, and Ugni Blanc had LT50 values lower than -9°C: -10.50°C, -10.60°C, -9.80°C, -9.70°C and -9.40°C, respectively. Buds of Merlot, Sauvignon Blanc, Syrah, and Gewurztraminer exhibited LT50 values higher than -8°C: -6.70°C, -6.80°C, -7.95°C, and -7.60°C, respectively.
3.1.3 Evaluation of cold hardiness of V. vinifera based on TTC
As shown in Table 3, the staining index of one-year-old shoots of different varieties decreased with the decrease of temperature. The LT50 values of the 20 tested V. vinifera varieties determined by TTC ranged from -23.74°C ~ -15.87°C. Meili, Ecolly, Italian Riesling, Riesling, and Ugni Blanc varieties had LT50 values below -20°C: -20.31°C, -21.07°C, -23.74°C, -20.48°C, and -20.07°C, respectively. Merlot, Petit Verdot, Cabernet Franc, and Syrah varieties had LT50 values higher than -18°C: -16.21°C, -17.57°C, -15.87°C, and -17.98°C, respectively. Granoir, Cabernet Sauvignon, Marselan, Dunkelfelder, Pinot Noir, Viognier, Petit manseng, Chardonnay, Sauvignon Blanc, Yan73, and Gerwurztraminer had LT50 values that ranged from -20°C∽-18°C.
3.1.4 Evaluation of cold hardiness of V. vinifera based on RG
As shown in Table 4, the germination rates of buds of different varieties decreased with the decrease of temperature. The LT50 values range of 20 tested V. viniferae varieties determined by RG ranged from -21.71°C ~ -12.54°C. Meili, Ecolly, Italian Riesling and Riesling varieties had LT50 values below -18°C: -19.67°C, -20.71°C, -20.33°C, and -18.78°C, respectively. Granoir, Sauvignon Blanc, Cabernet Franc, Syrah, and Gewurztraminer varieties had LT50 values higher than -15°C: -13.11°C, -13.22°C, -12.88°C, -13.98°C, and -12.54°C, respectively. Cabernet Sauvignon, Marselan, Dunkelfelder, Pinot Noir, Merlot, Viognier, Petit Verdot, Petit Manseng, Chardonnay, Yan73, and Ugni Blanc had LT50 values that ranged from -15°C to -18°C.
3.1.5 Analysis of results obtained by different evaluation methods
The LT50 values obtained through the different evaluation methods were analyzed by clustering, and the results are shown in Figure 1. The cold hardiness of the 20 tested V. vinifera varieties can be clustered into five categories. Meili and Ecolly clustered together with the highest cold hardiness. Pinot Noir, Viognier, Yan73, Riesling, and Petit Manseng clustered together with cold hardiness. Cabernet Sauvignon, Italian Riesling, Marselan, Dunkelfelder, and Cabernet Franc clustered together with moderate cold hardiness. Merlot, Petit Verdot, Chardonnay, and Ugni Blanc clustered together with low cold hardiness. Granoir, Gewurztraminer, Sauvignon Blanc, and Syrah clustered together with the lowest cold hardiness. The four evaluation methods can be divided into three categories: DTA (shoots and buds) and TTC clustered into one group, and EL and RG clustered into separate groups.
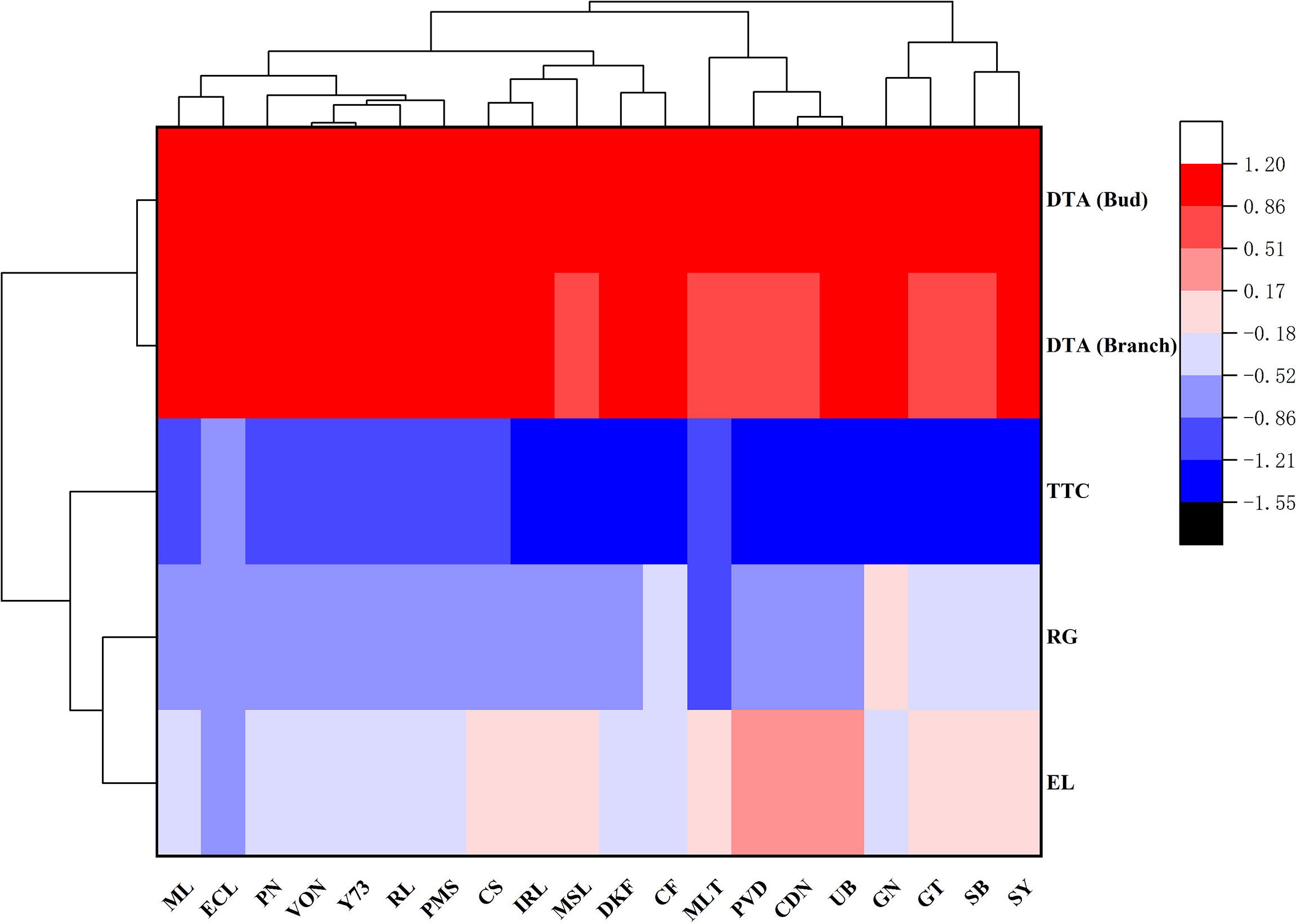
Figure 1 Clustering of LT50 values of V. vinifera varieties subjected to different evaluation methods. Note: The results (LT50 values) of different evaluation methods were quantified by the software SPSS17.0. ML (Meili), ECL (Ecolly), GN (Granoir), CS (Cabernet Sauvignon), MSL (Marselan), DKF (Dunkelfelder), PN (Pinot Noir), MLT (Merlot), VON (Viognier), PVD (Petit Verdot), PMS (Petit Manseng), CDN (Chardonnay), SB (Sauvignon Blanc), IRL (Italian Riesling), CF (Cabernet Franc), RL (Riesling), Y73 (YAN73), SY (Syrah), GT (Gewurztraminer), UB (Ugni Blanc).
Correlation analysis was performed of the different evaluation methods and the results are shown in Figure 2. There was a positive correlation among different evaluation methods, and all reached a very significant level. The correlation coefficients of DTA measured in shoots with DTA measured in buds, TTC, RG, and EL were 0.76, 0.85, 0.70 and 0.78 respectively. The correlation coefficients between bud DTA and TTC, RG, and shoot DTA measurements were 0.85, 0.73, and 0.85 respectively. The correlation coefficients between TTC and RG and DTA of buds were 0.69 and 0.78 respectively. The correlation coefficient between RG and TTC was 0.77.
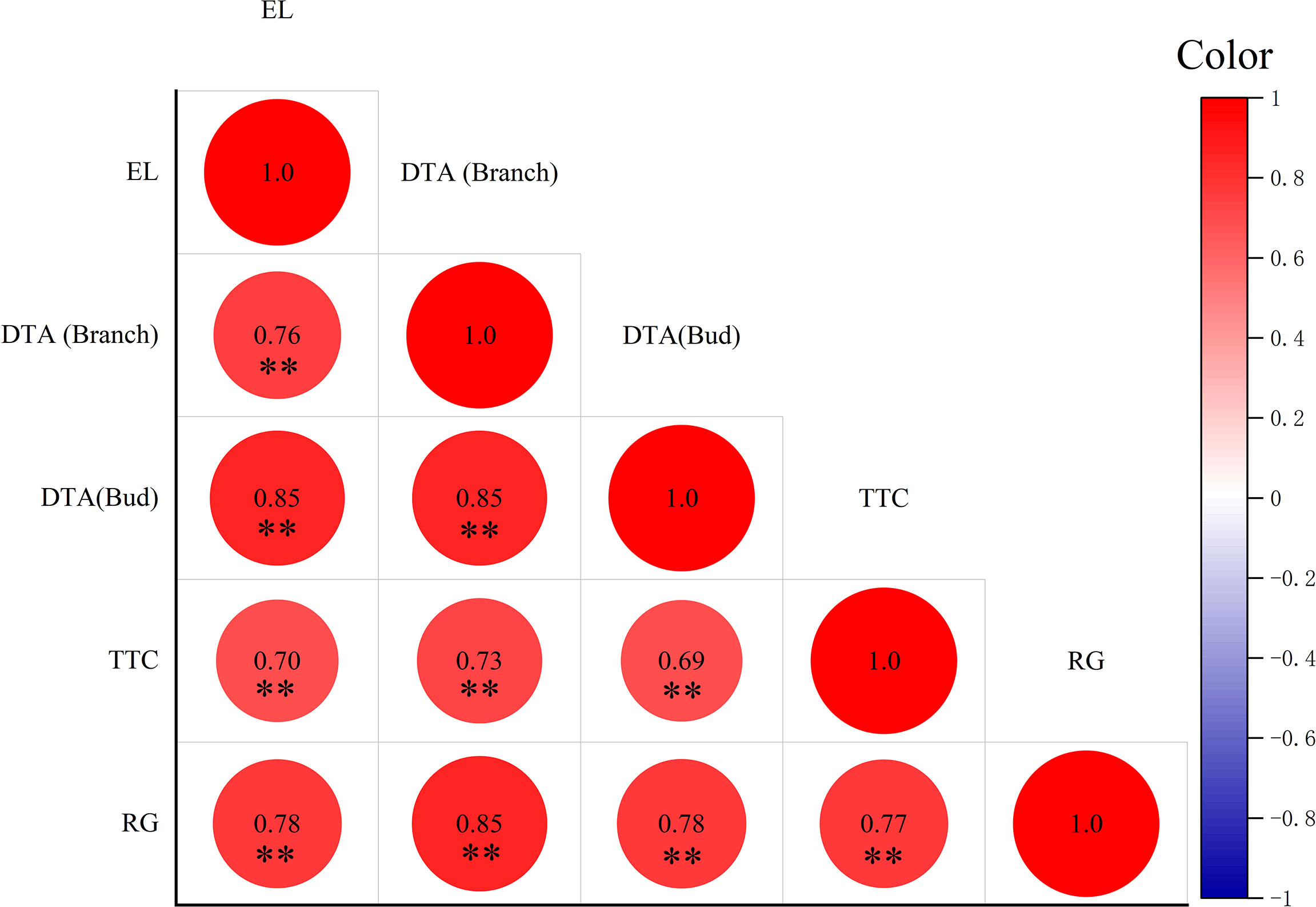
Figure 2 Correlation of LT50 values estimated by different methods. Data were tested by Student’s t-test, **p < 0.01 represent significant differences between methods. The larger the circle, the larger the correlation coefficient. Red is positive, blue is negative.
3.2 Analysis and screening of cold hardiness indexes
3.2.1 Correlation analysis of cold hardiness indexes
As shown in Figure 3, correlation analysis of the cold hardiness indicators revealed a high positive correlation between free water content (FW) and total water content (TW). The ratio of free water to bound water (FW/BW) was highly positively correlated with FW. Proline content (Pro) was highly positively correlated with bound water (BW). Total soluble sugar content (TSS) was highly positively correlated with BW and Pro. Malondialdehyde content (MDA) was highly positively correlated with FW and FW/BW. Catalase content (CAT) was highly positively correlated with BW, Pro, and TSS. Ascorbic acid content (ASA) was highly positively correlated with BW, Pro, soluble protein content (SPro), TSS, catalase (CAT), and peroxidase content (POD). BW was highly negatively correlated with FW. FW/BW was highly negatively correlated with BW. Pro was highly negatively correlated with FW and FW/BW. TSS was highly negatively correlated with FW and FW/BW. Sucrose content (Suc) was highly negatively correlated with FW/BW. Starch content (Sta) was highly negatively correlated with BW. MDA was highly negatively correlated with BW, Pro, and TSS. CAT was highly negatively correlated with FW and FW/BW. ASA was highly negatively correlated with FW, FW/BW, and MDA. Reduced glutathione content (GSH) was highly negatively correlated with TW and FW.
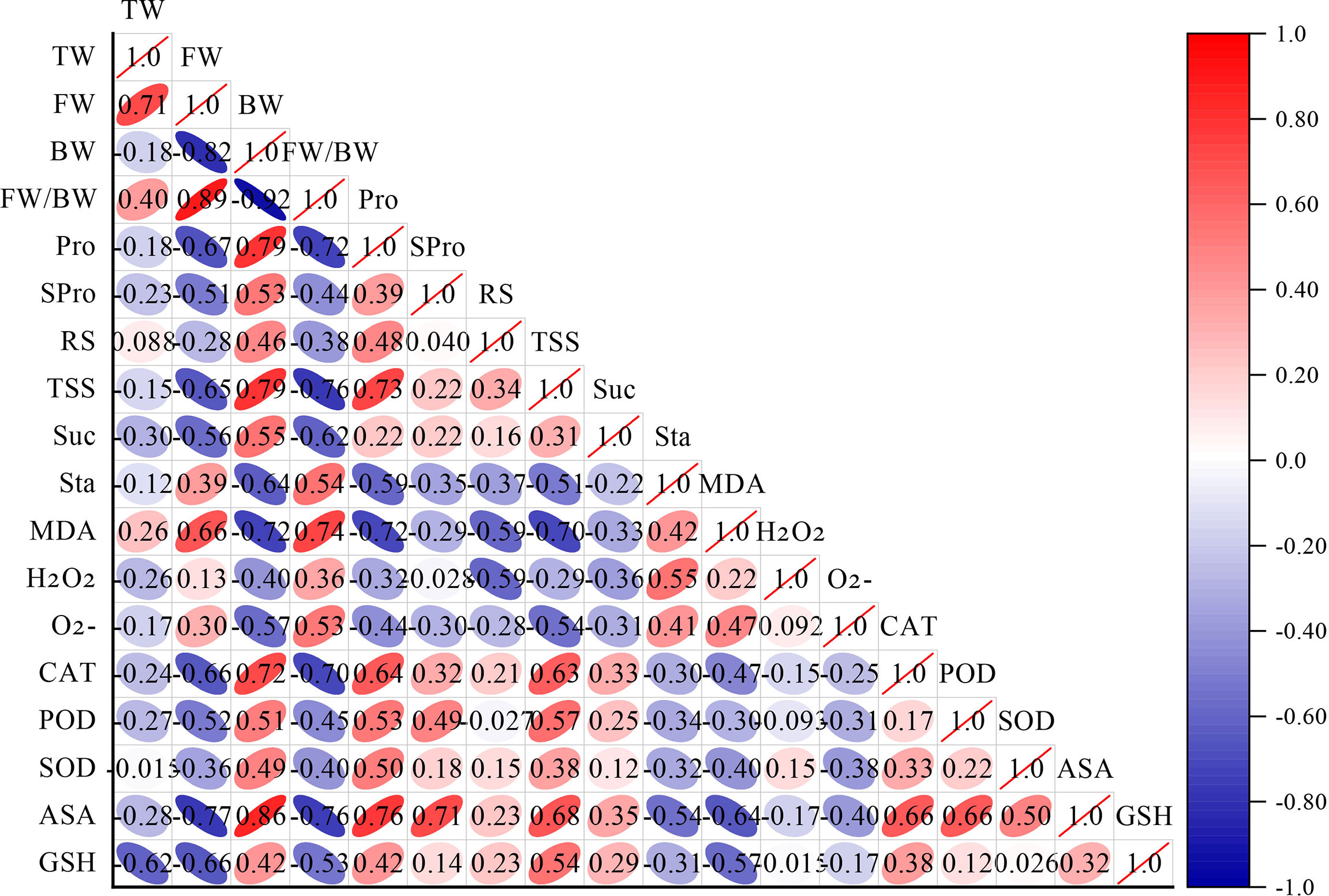
Figure 3 Correlation analysis of cold resistance indexes of different V. vinifera varieties. The more slender the ellipse, the larger the correlation coefficient. Red is positive, blue is negative. TW (Total water content), FW (Free water content), BW (Bound water content), FW/BW (The ratio of FW/BW), Pro (Proline content), SPro (Soluble protein content), RS (Reducing sugar content), TSS (Total soluble sugar content), Suc (Sucrose content), Sta (Starch content), MDA (Malondialdehyde content), H2O2 (hydrogen peroxide content), (Superoxide anions content), CAT (Catalase content), POD (Peroxidase content), SOD (Superoxide dismutase content), ASA (Ascorbic acid content), GSH (Reduced glutathione content).
3.2.2 Selection and weight of cold hardiness index
Correlation analysis of LT50 values measured by different evaluation methods and different cold hardiness indicators are shown in Figure 4. The amounts of free water (FW), bound water (BW), proline (Pro), total soluble sugar (TSS), malondialdehyde (MDA), catalase (CAT), and ascorbic acid (ASA), and, as well as the ratio of free water to bound water (FW/BW) of grapevine were significantly correlated with LT50 values measured by different evaluation methods. Among the water indexes, FW and LT50 values showed a high correlation coefficient overall, with a negative correlation. For osmoregulatory substances, Pro and LT50 values showed high overall correlation with a negative correlation. Among carbohydrate indexes, TSS and LT50 values were highly and negatively correlated. For the oxidative metabolites, MDA and LT50 values showed a high positive correlation coefficient. Of the antioxidant enzymes, CAT and LT50 values showed a high negative correlation coefficient. Among the antioxidant metabolites, ASA and LT50 values showed a high negative correlation coefficient. The high degree of correlation suggests that BW, Pro, TSS, MDA, CAT, and ASA can be used as primary indicators to evaluate cold hardiness in V. vinifera.
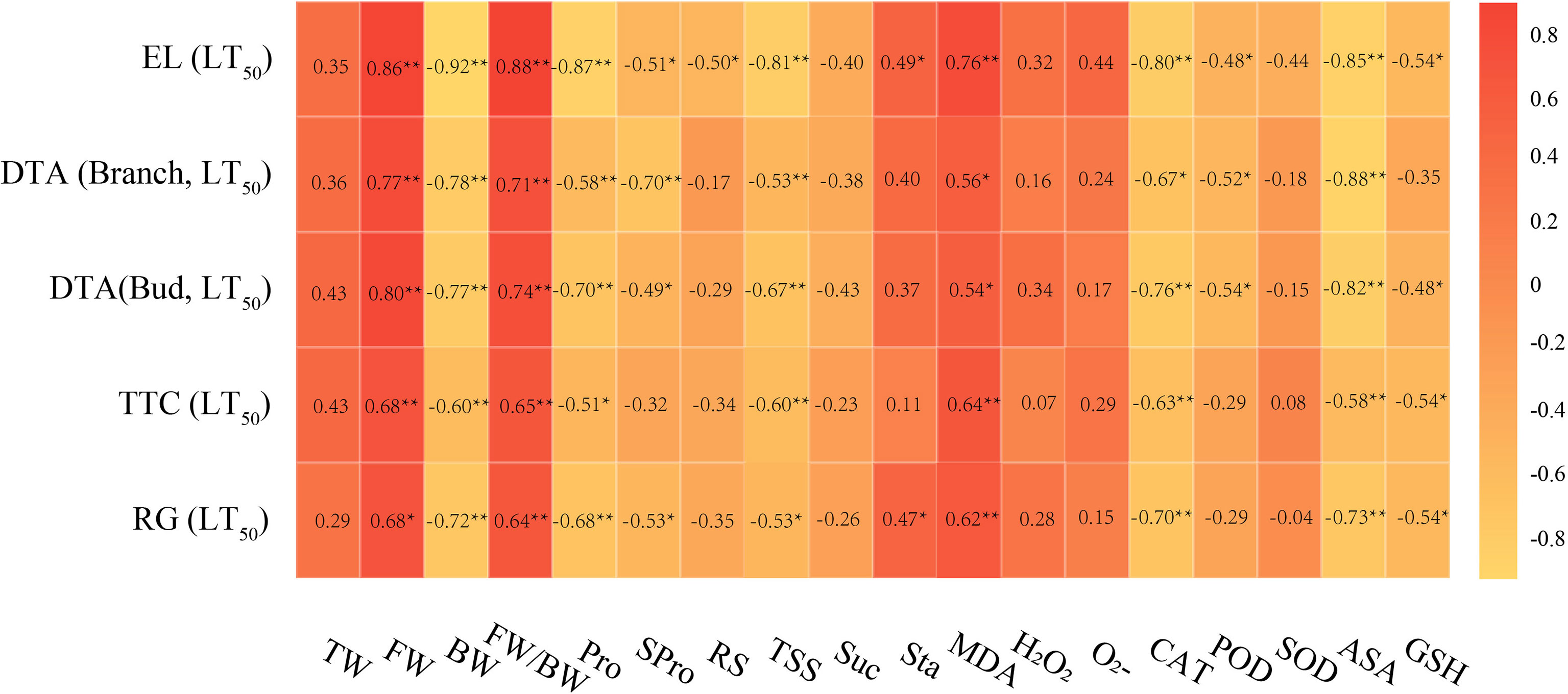
Figure 4 Correlation analysis of LT50 values and cold resistance indicators. Data were tested by Student’s t-test, **p < 0.01 represent significant differences between LT50 values measured by different evaluation methods and different cold resistance indicators.
The six cold hardiness indexes were standardized and then make principal component analysis (PCA) was performed. The results are shown in Table 5. PCA extracted one principal component, with characteristic root value greater than 1. The variance explanation rate of this one principal component was 75.274%, so the cumulative variance explanation rate was 75.274%. This single component reflects most grapevine cold hardiness information. To confirm the result, the principal component was removed from this analysis, and its corresponding weighted variance explanation rate is 75.274/75.274 = 100.00%.
Table 6 shows the extracted information of cold hardiness index by principal component, and the corresponding relationship between the principal component and the cold hardiness index. The communalities of all cold hardiness indexes are higher than 0.4, indicating strong correlation between indexes and principal components so principal components can be used to effectively extract information. Component score 1 = 0.208*BW + 0.198*Pro + 0.192*TSS – 0.181*MDA + 0.174*CAT + 0.197*ASA. The indexes that influence grape cold hardiness from strongest to weakest are BW, Pro, ASA, TSS, MDA, and CAT, and the corresponding weights are 18.08%, 17.22%, 17.09%, 16.73%, 15.71%, and 15.16%, respectively.
3.3 Establishment of comprehensive evaluation method of cold hardiness
3.3.1 Subordination function method and comprehensive evaluation of grapevine cold hardiness index
The different indexes have different units, nature, and quantity, making it necessary to carry out standard quantification. The change of each index is continuous, so the subordination function method (SF) can be used for standard quantification. The ascending and descending order of the subordination function is determined according to the positive and negative loading of the principal component factors. According to the subordination function value and weight of each index, the addition and multiplication rule Ci= Wij × R(Xij) can be applied, where Wij is the weight of each index and R (Xij) is the subordination function value of each index. By the calculation of each index, the comprehensive index of cold hardiness of grape varieties (Ci) was obtained, as shown in Table 7. This comprehensive index of cold hardiness was then applied, and the order of cold hardiness of different varieties is as follows: Ecolly > Meili > Viognier > Riesling > Petit Manseng > Italian Riesling > Yan73 > Pinot Noir > Dunkelfelder > Marselan > Cabernet Sauvignon > Granoir > Ugni Blanc > Gewurztraminer > Chardonnay >Syrah > Cabernet Franc > Sauvignon Blanc > Petit Verdot > Merlot.
3.3.2 Correlation analysis between the composite index and LT50 values calculated by different methods
LT50 is the main ecological factor characterizing plant cold hardiness. Correlation analysis between the composite index and LT50 measured by different evaluation methods is shown in Table 8. The composite index showed a very significant negative correlation with the five sets of LT50 measurements using the four evaluation methods. The composite index is most highly correlated with the LT50 values measured by EL, with a correlation coefficient of -0.938. The correlation coefficients between the composite index and DTA measurement of shoots and buds are -0.738 and -0.808 respectively. The correlation coefficients between the composite index and LT50 values measured by TTC and RG are -0.709 and -0.763, respectively.
4 Discussion
4.1 Evaluation of cold hardiness in V. vinifera using different methods
EL is the classical method to evaluate cold hardiness and was used here to screen several varieties (Sutinen et al., 1992). The results showed that cold hardiness was in the order of Ecolly > Meili > Riesling > Italian Riesling > Pinot Noir > Yan73 > Viognier > Petit Manseng > Dunkelfelder > Cabernet Sauvignon > Granoir > Marselan > Ugni Blanc > Gewurztraminer > Chardonnay > Sauvignon Blanc > Syrah > Merlot > Cabernet Franc > Petit Verdot. This order is basically consistent with that of previous work to measure cold hardiness, but the measured LT50 values are lower than other published values (Chen et al., 2020b; Wang et al., 2021b). This difference may be related to specifics of our sampling location or sampling time. There is a certain correlation between the cold hardiness of grapevines and the degree of dormancy and the local temperature during overwintering. In the northeast region of China, the cold hardiness of V. amurensis increased in October, was highest in November, and then gradually decreased (Zhao et al., 2020).
DTA, also known as low temperature exotherm (LTE), depends on the exothermic process when plant tissue freezes at low temperature (Kaya and Köse, 2017). Cold hardiness can be evaluated by detecting and recording the exothermic heat released by DTA (Mills et al., 2006). Median LTE temperatures approximate field temperatures that are lethal to 50% of buds (LT50), and thus DTA has become a preferred technique to characterize grapevine cold hardiness (Kaya and Köse, 2017). In this study, we collected the LTE of shoots and buds by DTA. The cold hardiness measurements of the two different parts (LT50 of shoot and LT50 of bud) were highly correlated, with stronger cold hardiness of shoots than that of buds; this is consistent with the results of previous studies (Chen et al., 2020a). Cold hardiness can vary in different parts of grapevine or during different growth degrees, with the strongest cold hardiness typically seen in shoots, followed by that in the main roots and the secondary roots (Chen et al., 2020a). The frost hardiness of unopened buds is relatively stronger, followed by opened buds, and then by young sprouts (Duan et al., 2017).
There can be significant observational error using TTC, as it is difficult to accurately visualize and quantify the results (Tian et al., 2013). The visual evaluation of a TTC stained image combined with application of the logistic equation is a simple and reliable method for the evaluation of grapevine cold hardiness (Zhao et al., 2018). In this study, the TTC measurements and use of the logistic equation allowed determination of cold hardiness in the following order: Italian Riesling > Ecolly > Riesling > Meili > Ugni Blanc > Pinot Noir > Chardonnay > Marselan > Yan73 > Dunkelfelder > Viognier > Cabernet Sauvignon > Petit Manseng > Sauvignon Blanc > Granoir > Gewurztraminer > Syrah > Petit Verdot > Merlot > Cabernet Franc. This differs from the previous ranking results (Wang et al., 2021b), and the variation may be experimental error related to the easy accumulation of triphenyl formazan in the cross section during the test (Zhao et al., 2018). Overall, the accuracy of this method may not be the highest, but the correlation analysis indicates that this method is sufficiently effective to evaluate the cold hardiness of grapevine.
RG is a more intuitive method in which the germination rate, rooting rate, and callus ratio of shoots are measured to ask how well plants survive after being frozen (Cao et al., 2010). Here, we used the germination rate of cuttings at different low temperatures combined with the logistic equation to provide quantifiable evaluation results for RG. The RG classification has high correlation with the results of other evaluation methods, indicating that RG combined with the logistic equation can be used as a quantitative method for the evaluation of grapevine cold hardiness.
LT50 value is used as an indicator of plant stress injury and has been widely applied to measure cold hardiness in grapevine (Zeng et al., 2017; Yang et al., 2020). The clustering results of LT50 values determined by different evaluation methods indicated that Meili and Ecolly varieties exhibit the highest cold hardiness; Pinot Noir, Viognier, Yan73, Riesling, and Petit Manseng show cold hardiness; Marselan, Dunkelfelder, Italian Riesling, Cabernet Sauvignon, and Cabernet Franc exhibit moderate cold hardiness; Merlot, Petit Verdot, Chardonnay, and Ugni Blanc show low cold hardiness; and Granoir, Gewurztraminer, Sauvignon Blanc, and Syrah exhibit the lowest cold hardiness. These results are not completely consistent with previous assessments. Ecolly is widely cultivated without soil burial for over-wintering in many areas where this practice is required for other varieties, suggesting this variety and related hybrid breeding technology may help solve this important challenge to viticulture in China. In clustering of 124 V. vinifera by Wang et al., Ecolly is in hardiness zone 1, Meili, Italian Riesling, Riesling, Cabernet Sauvignon, Dunkelfelder, and Petit Manseng belong to hardiness zone 2, Ugni Blanc, Marselan, Yan73, Sauvignon Blanc, Petit Verdot Chardonnay, and Pinot Noir belong to hardiness zone 3, Gewurztraminer, Cabernet Franc, and Merlot belong to hardiness zone 4 (Wang et al., 2021b). The differences between the two sets of results may be related to the number of germplasm resources tested and the range of LT50 values that characterize the cold hardiness of V. vinifera. The cold hardiness of V. vinifera is generally poor and the range of LT50 values was relatively small, with LT50 values determined by EL that are between -22°C∽-13°C (Wang et al., 2021b).
The correlation analysis of the LT50 values calculated using the four evaluation methods showed very significant positive correlations among the different evaluation methods. This suggests that EL, DTA, TTC, and RG methods can all be used to evaluate cold hardiness and distinguish the cold hardiness of different varieties. However, for practical measurement, these methods rely on different basic principles, so there are advantages and disadvantages of each approach. During measurement, EL is easily affected by temperature, soaking time, and the dissolution of CO2 in the air, leading to poor repeatability of the results (Su et al., 2015a). EL is also time-consuming and requires significant materials (Jiang et al., 1999). As a non-destructive evaluation method of cold hardiness, DTA is vulnerable to any changes in the external environment or altered states of dormancy of plants under natural conditions, decreasing the accuracy of the measured results (Chai et al., 2015). TTC can easily be affected by human subjectivity when visually measuring the degree of browning and coloring, which leads to a large error. To achieve good repeatability, the operator must have proficient experimental skills, as it is difficult for beginners to obtain accurate results (Guo et al., 2018). As an intuitive method to evaluate the cold hardiness of grapevine, RG is limited by requiring a long test period and a large amount of materials (Liu Z. et al., 2021). The germination rate of grapevine is also affected by the water retention of buds, which can be another source of variation (Wang et al., 2020). In practical application, the appropriate evaluation method of cold hardiness should be selected according to practical needs.
4.2 Correlation of the cold hardiness index with cold hardiness in V. vinifera
Cold hardiness of grapevine is a quantitative characteristic controlled by multiple genes (Wang et al., 2020; Wang Z. L. et al., 2021). Given this, it makes sense that evaluation using a single index is not sufficiently comprehensive to accurately reflect cold hardiness in grapevine (Zhang J. et al., 2012). In this study, 18 physiological and biochemical indexes of cold hardiness were determined, and the correlations between different indexes and LT50 values determined by EL were analyzed. The FW, BW, FW/BW, Pro, TSS, MDA, CAT, and ASA of dormant shoots were significantly correlated with the LT50 values, suggesting these physiological and biochemical factors are sufficient to evaluate cold hardiness of V. vinifera during overwintering. This conclusion is not completely consistent with previous research results. Zhao et al. studied the correlation between physiological and biochemical indexes of V. amurensis and the LT50 values measured by EL and found significant correlation of FW, BW and TSS with LT50 values (Zhao et al., 2020). Su et al. examined the correlation between physiological and biochemical indexes of V. amurensis and V. vinifera and the LT50 values measured by EL and found that TSS, SPro, MDA, and Pro were significantly correlated with LT50 measurements (Su et al., 2015b). Cao et al. analyzed the correlation between physiological and biochemical indexes of rootstock, V. labrusca, V. vinifera, and wild species and found that TSS, Spro, MDA, and Pro exhibited significant correlation with the EL-based LT50 values (Cao et al., 2010). The different findings reported by different groups may vary due to the use of different populations of tested materials. This study focused on V. vinifera, so the cold hardiness model developed here may be most suitable for the evaluation of cold hardiness of this species.
Further analysis of the correlation between different indexes shows a high correlation between different indexes, which is consistent with previous research (Zhang J. et al., 2012; He, 2015; Zhao et al., 2020). This shows that the information reflected by these cold hardiness indexes is super-imposed, so one or two indexes cannot be used alone to evaluate the cold hardiness of grapevine. Instead, SF should be adopted to more accurately evaluate the cold hardiness of different germplasm resources. Zhang et al. comprehensively evaluated the cold hardiness of 25 wild grape varieties by SF (Zhang J. et al., 2012). Su et al. established a comprehensive evaluation method of cold hardiness through PCA and SF, showing that the average subordinate function value obtained by SF could be used as a comprehensive evaluation index of cold hardiness of V. amurensis germplasm (Su et al., 2015b). Accurate evaluation of cold hardiness of V. vinifera is essential for cold hardiness breeding using V. vinifera as parents.
The acquisition of plant cold hardiness is a complex physiological response process, with contributions from many factors altering cold acclimation capabilities (Strimbeck et al., 2015). In response to low temperature stress, plants will launch a series of signal transduction reactions, the tissue water content decreases, osmoregulative substances accumulate, and antioxidant enzyme activity levels change to increase the cold-hardiness capabilities (Chinnusamy et al., 2007). The water content and state of water in plants significantly determine the cold hardiness of plants. The freezing point of FW is 0°C, but the freezing point of BW is -20°C ~ -25°C. At a low ratio of FW/BW, the freezing temperature will decrease correspondingly, which increases frost hardiness (Wu, 2011). Under cold stress, the increase of SPro can further increase the proportion of bound intracellular water. SPro can also regulate the expression of cold hardiness genes to enhance a plant’s cold hardiness (Dionne et al., 2001). Pro helps maintain osmotic equilibrium between the symplasts and apoplasts, so helps prevent low temperature damage by maintaining the functional integrity of the membrane (Dionne et al., 2001). Sugar can improve the cold hardiness of plants by increasing the concentration of cell sap and lowering the freezing point (Cao et al., 2010). Under cold stress, polysaccharides are hydrolyzed to soluble sugars that increase the osmotic potential of the cytoplasm and lower the freezing temperature (Zhang J. et al., 2012). Generally, cold stress can initiate the accumulation of reactive oxygen species (ROS) such as superoxide radical (), hydroxyl radical (OH-), and hydrogen peroxide (H2O2) to increase oxidative stress in the plant (Bajguz and Hayat, 2009; Liu et al., 2011). To protect against low temperature-induced oxidative damage, all plants have developed processes to scavenge ROS by enzymatic antioxidant techniques such as POD, SOD and CAT, as well as non-enzymatic methods (Lee and Lee, 2000). SOD scavenges toxic superoxide radicals and catalyzes the reduction of two superoxide anions into H2O2 and O2 (Miyake and Yokota, 2000). POD is another antioxidant enzyme that converts H2O2 into O2 and H2O (Sudhakar et al., 2001). ASA and GSH are important nonenzymatic antioxidants in plant cells. They can directly scavenge and reduce the level of H2O2 (Meng et al., 2014). In this study, according to the mechanism of the comprehensive cold hardiness index, BW of the water indexes, Pro of the osmoregulation substances, TSS of the carbohydrate indexes, MDA of the oxidation metabolites, CAT of the antioxidant enzyme system, and ASA of the antioxidant metabolites are the main indexes of cold hardiness of V. vinifera. These six indexes were given weight in cold hardiness by PCA, allowing the construction of the comprehensive evaluation method of V. vinifera by SF. The correlation was analyzed between the composite index and the LT50 values calculated using the other evaluation methods. The results showed that there was a very significant negative correlation between the composite index and the LT50 values measured by different evaluation methods. The composite index has the highest correlation with the LT50 values determined by EL, with a coefficient of -0.938. The results show the comprehensive evaluation method of grapevine cold hardiness constructed by PCA combined with SF can accurately evaluate the cold hardiness of V. vinifera germplasm resources.
5 Conclusions
Our results showed that Ecolly and Meili have the highest cold hardiness among the 20 V. vinifera germplasms tested and can be used as parent materials for cold hardiness breeding. EL, DTA, TTC, and RG can be used to screen cold hardiness of V. vinifera and can distinguish the cold hardiness of different varieties. During overwintering, measurements of BW, Pro, TSS, MDA, CAT, and ASA in shoots can be used as the main cold hardiness indexes of V. vinifera germplasm. The comprehensive evaluation method constructed by PCA combined with SF can be accurately applied for the evaluation of cold hardiness of V. vinifera germplasm resources.
Data availability statement
The original contributions presented in the study are included in the article/supplementary material. Further inquiries can be directed to the corresponding authors.
Author contributions
Conceptualization, Z-LW, DW, HW and HL; Data curation, Z-LW, DW, XC and YW; Formal analysis, MH and XH; Funding acquisition, HW and HL; Methodology, FY and Y-HL; Project administration, HW and HL. All authors have read and agreed to the published version of the manuscript.
Funding
This work was supported by the National Key Research and Development Project (2019YFD1002500), the Key Research and Development Project of Shaanxi Province (2020ZDLNY07-08), the Ningxia Hui Nationality Autonomous Region Major Research and Development Project (2020BCF01003), the Research and application of key technologies for sustainable development of wine industry (LYNJ202110), and the Science and technology plan major project of Yinchuan, Ningxia (YCKJ2020-ZD04).
Acknowledgments
We would like to thank Rachel M for improving the English in this paper.
Conflict of interest
The authors declare that the research was conducted in the absence of any commercial or financial relationships that could be construed as a potential conflict of interest.
The reviewers YJ and YW declared a shared affiliation with the authors to the handling editor at the time of review.
Publisher’s note
All claims expressed in this article are solely those of the authors and do not necessarily represent those of their affiliated organizations, or those of the publisher, the editors and the reviewers. Any product that may be evaluated in this article, or claim that may be made by its manufacturer, is not guaranteed or endorsed by the publisher.
References
Aebi, H. (1984). Catalase in vitro. Methods Enzymol. 105, 121–126. doi: 10.1016/S0076-6879(84)05016-3
Avanci, N. C., Luche, D. D., Goldman, G. H., Goldman, M. (2010). Jasmonates are phytohormones with multiple functions, including plant defense and reproduction. Genet. Mol. Res. 9 (1), 484–505. doi: 10.4238/vol9-1gmr754
Bajguz, A., Hayat, S. (2009). Effects of brassinosteroids on the plant responses to environmental stresses. Plant Physiol. Biochem. 47 (1), 1–8. doi: 10.1016/j.plaphy.2008.10.002
Bates, L. S., Waldren, R. P., Teare, I. D. (1973). Rapid determination of free proline for water-stress studies. Plant Soil 39 (1), 205–207. doi: 10.1007/BF00018060
Bohan, Y., Shuang, H., Yuan, L., Buchun, L., Yanlun, J., Dengzhao, K., et al. (2020). Transcriptomics integrated with metabolomics reveals the effect of regulated deficit irrigation on anthocyanin biosynthesis in Cabernet sauvignon grape berries. Food Chem. 314 (9), 126170. doi: 10.1016/j.foodchem.2020.126170
Bradford, M. M. (1976). A rapid and sensitive method for the quantitation of microgram quantities of protein utilizing the principle of protein-dye binding. Anal. Biochem. 72 (1-2), 248–254. doi: 10.1016/0003-2697(76)90527-3
Cao, J., Chen, B., Wang, L., Mao, J., Zhao, X. (2010). Cold resistance indexes identification and comprehensive evaluation of grape varieties. Acta Botanica Boreali Occidentalia Sin. 30 (11), 2232–2239. doi: 10.3724/SP.J.1142.2010.40521
Chai, F., Zhu, W., Xiang, Y., Xin, H., Li, S. (2015). Optimized method for detecting the cold hardiness of grape dormant bud by temperature exotherms (LTE) analysis and its utilization. Acta Hortic. Sin. 42 (1), 140–148. doi: 10.16420/j.issn.0513-353x.2014-0651
Cheng, G., Ma, T., Deng, Z., GutiérrezGamboa, G., Ge, Q., Xu, P., et al. (2021). Plant-derived melatonin from food: a gift of nature. Food Funct. 12 (7), 2829–2849. doi: 10.1039/D0FO03213A
Chen, R., Zhang, X., Ding, Q., Yang, Y., Nan, X., Hu, H., et al. (2020a). Comprehensive evaluation of cold resistance in different parts of four wine grape varieties based on different thermal analysis. Chin. J. Eco Agriculture 28 (7), 1022–1032. doi: 10.13930/j.cnki.cjea.200151
Chen, B., Zhang, B., Mao, J., Hao, Y., Yang, R., Cai, X., et al. (2014). The relationship between the changing of water content and the cold resistance of grape branches. Plant Physiol. J. 50 (04), 535–541. doi: 10.13592/j.cnki.ppj.2013.0441
Chen, R., Zhang, X., Yang, Y., Li, F. (2020b). Comparison of cold resistance of six wine-making grape varieties at the eastern foot of helan mountain. Northern Horticult. 06, 43–48. doi: 10.11937/bfyy.20192619
Chinnusamy, V., Zhu, J., Zhu, J. K. (2007). Cold stress regulation of gene expression in plants. Trends Plant Sci. 12 (10), 444–451. doi: 10.1016/j.tplants.2007.07.002
Dionne, J., Castonguay, Y., Nadeau, P., Desjardins, Y. (2001). Freezing tolerance and carbohydrate changes during cold acclimation of green-type annual bluegrass (Poa annua l.) ecotypes. Crop Sci. 41 (2), 443–451. doi: 10.2135/cropsci2001.412443x
Duan, X., Zhang, L., Li, H., Guo, X., Wang, J., Ma, G. (2017). Laboratory frost simulation experiment of wine grape at germination period of young sprouts at eastern foot of helan mountain. Nonwood For. Res. 35 (04), 171–176. doi: 10.14067/j.cnki.1003-8981.2017.04.025
Elisa, A., Sergio, M., Camilla, P., Elisa, M., Elettra, M. (2009). Comparing image (fractal analysis) and electrochemical (impedance spectroscopy and electrolyte leakage) techniques for the assessment of the freezing tolerance in olive. Trees- Structure&Function 23 (1), 159–167. doi: 10.1007/s00468-008-0264-1
Elstner, E. F., Heupel, A. (1976). Inhibition of nitrite formation from hydroxylammoniumchloride: a simple assay for superoxide dismutase. Anal. Biochem. 70 (2), 616–620. doi: 10.1016/0003-2697(76)90488-7
Fennell, A. (2004). Freezing tolerance and injury in grapevines. J. Crop Improvement 10 (1-2), 201–235. doi: 10.1300/J411v10n01_09
Foyer, C. H., Halliwell, B. (1976). The presence of glutathione and glutathione reductase in chloroplasts: A proposed role in ascorbic acid metabolism. Planta 133 (1), 21–25. doi: 10.1007/BF00386001
Gao, J. (2006). Experimental guidance for plant physiology (Beijing, China: Higher Education Press).
Giannopolites, C. N., Ries, S. K. (1977). Superoxide dismutase occurrence in higher plants. Plant Physiol. 59, 309–314. doi: 10.1104/pp.59.2.309
Guo, Y., Zhang, S., Li, Y., Zhang, X., Wang, G., Yang, Y., et al. (2018). Screening of cold resistance related indexes and establishment of evaluation method for Chinese chestnut. J. Northwest A&F Univ. (Nat. Sci. Ed.) 46 (10), 40–48. doi: 10.13207/j.cnki.jnwafu.2018.10.006
Han, X., Xue, T., Liu, X., Wang, Z., Zhang, L., Wang, Y., et al. (2021). A sustainable viticulture method adapted to the cold climate zone in China. Horticulturae 7 (6), 150. doi: 10.3390/horticulturae7060150
He, W. (2015). Development of evaluation method and utilization for cold resistance of germplasm resources of vitis amurensis (Yangling, Shaanxi Province: Master Master, Chinese Academy of Agricultural Science Dissertation).
Hodges, D. M., Delong, J. M., Prange, F. (1999). Improving the thiobarbituric acid-reactive-substances assay for estimating lipid peroxidation in plant tissues containing anthocyanin and other interfering compounds. Planta 207 (4), 604–611. doi: 10.1007/s004250050524
Hu, Y., Jiang, L., Wang, F., Yu, D. (2013). Jasmonate regulates the inducer of cbf expression-c-repeat binding factor/DRE binding factor1 cascade and freezing tolerance in arabidopsis. Plant Cell 25 (8), 2907–2924. doi: 10.1105/tpc.113.112631
Jeon, J., Kim, N. Y., Kim, S., Na, Y. K., Kim, J. (2010). A subset of cytokinin two-component signaling system plays a role in cold temperature stress response in arabidopsis. J. Biol. Chem. 285, 23371–23386. doi: 10.1074/jbc.M109.096644
Jiang, H., Howell, G. S., Flore, J. A. (1999). Efficacy of chlorophyll fluorescence as a viability test for freeze-stressed woody grape tissues. Can. J. Plant Sci. 79 (3), 401–409. doi: 10.4141/P98-088
Jin, P., Lv, M., Sun, C., Zheng, Y., Sun, M. (2012). Effects of methyl jasmonate in combination with low temperature conditioning on chilling injury and active oxygen metabolism in loquat fruits. Acta Hortic. Sin. 39 (2), 461–468. doi: 10.16420/j.issn.0513-353x.2012.03.010
Ju, Y.-l., Yang, B.-h., He, S., Tu, T.-y., Min, Z., Fang, Y.-l., et al. (2019). Anthocyanin accumulation and biosynthesis are modulated by regulated deficit irrigation in Cabernet sauvignon (Vitis vinifera l.) grapes and wines. Plant Physiol. Biochem. 135, 469–479. doi: 10.1016/j.plaphy.2018.11.013
Kaya, Ö., Köse, C. (2017). Determination of resistance to low temperatures of winter buds on lateral shoot present in karaerik (Vitis vinifera l.) grape cultivar. Acta Physiologiae Plantarum 39 (9), 209. doi: 10.1007/s11738-017-2513-7
Küpe, M., Köse, C. (2020). The relationship between bud size and exotherm formation in dormant buds of grapevine. Atatürk Üniversitesi Ziraat Fakültesi Dergisi 51 (3), 243–248. doi: 10.17097/ATAUNIZFD.678624
Lee, D. H., Lee, C. B. (2000). Chilling stress-induced changes of antioxidant enzymes in the leaves of cucumber: in gel enzyme activity assays. Plant Sci. 159 (1), 75–85. doi: 10.1016/s0168-9452(00)00326-5
Li, Z., Jiao, Y., Zhang, C., Dou, M., Weng, K., Wang, Y., et al. (2021). VvHDZ28 positively regulate SA biosynthesis during seed abortion in Thompson seedless. Plant Biotechnol. J. 19 (9), 1824–1838. doi: 10.1111/pbi.13596
Liu, R., Chen, T., Yin, X., Xiang, G., Xu, Y. (2021). A plasmopara viticola RxLR effector targets a chloroplast protein PsbP to inhibit ROS production in grapevine. Plant J. 106 (6), 1557–1570. doi: 10.1111/tpj.15252
Liu, Z., Dong, Z., Li, X., Tan, M., Yang, R., Yang, Z., et al. (2021). Analysis of cold resistance of wine grape based on recovery growth method. J. Fruit Resour. 2 (2), 5–8. doi: 10.16010/j.cnki.14-1127/s.2021.02.002
Liu, D., Pei, Z., Naeem, M., Ming, D., Liu, H., Khan, F., et al. (2011). 5-aminolevulinic acid activates antioxidative defence system and seedling growth in brassica napus l. under water-deficit stress. J. Agron. Crop Sci. 197, 284–295. doi: 10.1111/j.1439-037X.2011.00465.x
Londo, J. P., Kovaleski, A. P. (2017). Characterization of wild north American grapevine cold hardiness using differential thermal analysis. Am. J. Enology Viticulture 68 (2), 203–212. doi: 10.5344/ajev.2016.16090
Meng, J. F., Xu, T. F., Wang, Z. Z., Fang, Y. L., Xi, Z. M., Zhang, Z. W., et al. (2014). The ameliorative effects of exogenous melatonin on grape cuttings under water-deficient stress: antioxidant metabolites, leaf anatomy, and chloroplast morphology. J. Pineal. Res. 57 (2), 200–212. doi: 10.1111/jpi.12159
Miller, G. (1959). Use of dinitrosalicyclic acid reagent for determination of reducing sugars. Anal. Chem. 31, 426–428. doi: 10.1021/ac60147a030
Mills, L., Ferguson, J., Keller, M. (2006). Cold-hardiness evaluation of grapevine buds and cane tissues. Am. J. Enology Viticulture 57 (2), 194–200. doi: 10.1016/j.scienta.2005.07.007
Miyake, C., Yokota, A. (2000). Determination of the rate of photoreduction of O2 in the water-water cycle in watermelon leaves and enhancement of the rate by limitation of photosynthesis. Plant Cell Physiol. 41 (3), 335–343. doi: 10.1093/pcp/41.3.335
Monostori, P., Wittmann, G., Karg, E., Túri, S. (2009). Determination of glutathione and glutathione disulfide in biological samples: An in-depth review. J. Chromatogr. B Anal. Technol. Biomed. Life Sci. 877 (28), 3331–3346. doi: 10.1016/j.jchromb.2009.06.016
Muhammed, K., Cafer, K. (2021). Determination of the characteristic structures of low temperature exotherm in grapevine buds and their relationship with the bud structure. Erwerbs-Obstbau 63, 123–129. doi: 10.1007/s10341-021-00590-6
Niu, L., He, P. (1993). Comparative study on three identification methods of grape cold resistance. Sino-Overseas Grapevine Wine 2, 5–7. doi: 10.13414/j.cnki.zwpp.1993.02.003
Patterson, B., Macrae, E., Ferguson, I. (1984). Estimation of hydrogen peroxide in plant extracts using titanium (IV). Anal. Biochem. 134, 487–497. doi: 10.1016/0003-2697(84)90039-3
Rao, M. (1996). Ultraviolet-b- and ozone-induced biochemical changes in antioxidant enzymes of arabidopsis thaliana. Plant Physiol. 110 (1), 125–136. doi: 10.1104/pp.110.1.125
Strimbeck, G. R., Schaberg, P. G., Fossdal, C. G., Schröder, W. P., Kjellsen, T. D. (2015). Extreme low temperature tolerance in woody plants. Front. Plant Sci. 6. doi: 10.3389/fpls.2015.00884
Su, L., Dai, Z., Li, S., Xin, H. (2015a). A novel system for evaluating drought-cold tolerance of grapevines using chlorophyll fluorescence. BMC Plant Biol. 15, 82. doi: 10.1186/s12870-015-0459-8
Sudhakar, C., Lakshmi, A., Giridarakumar, S. (2001). Changes in the antioxidant enzyme efficacy in two high yielding genotypes of mulberry (Morus alba l.) under NaCl salinity. Plant Sci. 161 (3), 613–619. doi: 10.1016/S0168-9452(01)00450-2
Su, L., Li, S., Ma, S., Dai, C., Zhenzhen, S., Tang, B., et al. (2015b). A comprehensive assessment method for cold resistance of grape vines. Acta Prataculturae Sin. 24 (03), 70–79. doi: 10.11686/cyxb20150307
Sun, X., Zhang, F., Gutiérrez-Gamboa, G., Ge, Q., Ma, T. (2021). Real wine or not? protecting wine with traceability and authenticity for consumers: chemical and technical basis, technique applications, challenge, and perspectives. Crit. Rev. Food Sci. Nutr. 2, 1–27. doi: 10.1080/10408398.2021.1906624
Sutinen, M. L., Palta, J. P., Reich, P. B. (1992). Seasonal differences in freezing stress resistance of needles of pinus nigra and pinus resinosa: evaluation of the electrolyte leakage method. Tree Physiol. 11 (3), 241–254. doi: 10.1093/treephys/11.3.241
Thomas, F. M., Ahlers, U. (2010). Effects of excess nitrogen on frost hardiness and freezing injury of above-ground tissue in young oaks (Quercus petraea and q. robur). New Phytol. 144 (1), 73–83. doi: 10.1046/j.1469-8137.1999.00501.x
Tian, J., Wang, H., Gao, Y., Zhang, Z. (2013). Assessment of freezing tolerance of juglans germplasms by using annual dormant branches. Acta Hortic. Sin. 40 (06), 1051–1060. doi: 10.16420/j.issn.0513-353x.2013.06.005
Wang, X., Tu, M., Wang, Y., Yin, W., Zhang, Y., Wu, H., et al. (2021a). Ecosystem service function and assessment of the value of grape industry in soil-burial over-wintering areas. Horticulturae 7 (7), 202. doi: 10.3390/HORTICULTURAE7070202
Wang, Z., Chai, F., Zhu, Z., Elias, G. K., Xin, H., Liang, Z., et al. (2020). The inheritance of cold tolerance in seven interspecific grape populations. Scientia Hortic. 266, 109260. doi: 10.1016/j.scienta.2020.109260
Wang, Z.-L., Hui, M., Shi, X.-Q., Wu, D., Wang, Y., Han, X., et al. (2022). Characteristics of the seed germination and seedlings of six grape varieties (V. vinifera). Plants 11 (4), 479. doi: 10.3390/plants11040479
Wang, X., Tu, M., Wang, Y., Yin, W., Zhang, Y., et al. (2021). Whole-genome sequencing reveals rare off-target mutations in CRISPR/Cas9-edited grapevine. Horticult. Res. 8 (1), 114. doi: 10.1038/S41438-021-00549-4
Wan, R., Guo, C., Hou, X., Zhu, Y., Wang, X. (2021). Comparative transcriptomic analysis highlights contrasting levels of resistance of Vitis vinifera and Vitis amurensis to botrytis cinerea. Horticult. Res. 8 (1), 103. doi: 10.1038/S41438-021-00537-8
Wang, Z., Wang, Y., Wu, D., Hui, M., Han, X., Wang, Y., et al. (2021b). Identification and regionalization of cold resistance of wine grape germplasms (V. vinifera). Agriculture 11 (11), 1117. doi: 10.3390/agriculture11111117
Wang, Z., Wang, Y., Wu, D., Hui, M., Han, X., Xue, T., et al (2021c). GRAS-domain transcription factor PAT1 regulates jasmonic acid biosynthesis in grape cold stress response. Plant Physiol. 186, 1–43. doi: 10.1093/plphys/kiab142
Wang, Z. L., Xue, T. T., Gao, F. F., Zhang, L., Han, X., et al. (2021). Intraspecific recurrent selection in V. vinifera: an effective method for breeding of high quality, disease-, cold-, and drought -resistant grapes. Euphytica 217, 111. doi: 10.1007/s10681-021-02851-7
Wei, R., Ding, Y., Gao, F., Zhang, L., Wang, L., Li, H., et al. (2022). Community succession of the grape epidermis microbes of cabernet sauvignon (Vitis vinifera l.) from different regions in China during fruit development. Int. J. Food Microbiol. 362, 109475. doi: 10.1016/J.IJFOODMICRO.2021.109475
Wu, X. (2011). Study on cold resistance of grape resources (Yangling, Shaanxi Province: Master, Northwest A&F University).
Xu, Q., Fu, Q., Li, Z., Liu, H., Sun, Y. (2021). Procyanidin C1 is a natural agent with senolytic activity against aging and age-related diseases. Nat. Metab. 04 (14), 1101. doi: 10.1101/2021.04.14.439765
Yang, C., Shang, K., Lin, C., Wang, C., Shi, X., Wang, H., et al. (2021a). Processing technologies, phytochemical constituents, and biological activities of grape seed oil (GSO): A review. Trends Food Sci. Technol. 116, 1074–1083. doi: 10.1016/j.tifs.2021.09.011
Yang, Y., Zhang, X., Chen, R., Liu, Z., Li, F., Feng, R., et al. (2020). Comparing the cold resistance of roots of different wine grape varieties. Chin. J. Eco Agriculture 28 (04), 558–565. doi: 10.13930/j.cnki.cjea.190787
Yang, N., Zhou, Y., Wang, Z., Zhang, Z., Xi, Z., Wang, X. (2021). Emerging roles of brassinosteroids and light in anthocyanin biosynthesis and ripeness of climacteric and non-climacteric fruits. Crit. Rev. Food Sci. Nutr. 18, 1–13. doi: 10.1080/10408398.2021.2004579
Yemm, E. W., Willis, A. J. (1954). The estimation of carbohydrates in plant extracts by anthrone. Biochem. J. 57 (3), 508–514. doi: 10.1042/bj0570508
Yue, X., Ma, X., Tang, Y., Wang, Y., Wu, B., Jiao, X., et al. (2020). Effect of cluster zone leaf removal on monoterpene profiles of sauvignon blanc grapes and wines. Food Res. Int. 131, 109028. doi: 10.1016/j.foodres.2020.109028
Yue, X., Wei, S., Liu, W., Lu, J., Fang, Y., Zhang, Z., et al. (2021). Effect of rain-shelter cultivation on the monoterpenes profile of Muscat Hamburg grapes and wines. Scientia Hortic. 285, 110136. doi: 10.1016/j.scienta.2021.110136
Zeng, L., Zheng, X., Zhang, T., Luo, J. (2017). Comparison of cold resistance of 6 apple rootstocks by conductance method and logistic equation. Jiangsu Agric. Sci. 45 (10), 119–121. doi: 10.15889/j.issn.1002-1302.2017.10.034
Zhang, F., Wang, X. Q., Zhang, H. Q. (2012). The effect of cold stress on endogenous hormones and CBF1 homolog in four contrasting bamboo species. J. For. Res. 17 (1), 72–78. doi: 10.1007/s10310-011-0253-x
Zhang, J., Wu, X., Niu, R., Liu, Y., Liu, N., Xu, W., et al. (2012). Cold-resistance evaluation in 25 wild grape species. Vitis 51 (4), 153–160. doi: 10.5073/VITIS.2012.51.153-160
Zhang, N., Zhao, B., Zhang, H.-J., Weeda, S., Yang, C., Yang, Z.-C., et al. (2013). Melatonin promotes water-stress tolerance, lateral root formation, and seed germination in cucumber (Cucumis sativus l.). J. Pineal. Res. 54 (1), 15–23. doi: 10.1111/J.1600-079X.2012.01015.X
Zhao, Y., Ai, J., Yang, Y., Wang, Z., Liu, Y., He, W., et al. (2018). Identification of cold resistance in vitis amurensis germplasms base on TTC staining index and logistic equation. Trans. Chin. Soc. Agric. Eng. 34 (11), 174–180. doi: 10.11975/j.issn.1002-6819.2018.11.022
Keywords: grapevine, cold hardiness, physiological and biochemical indexes, comprehensive evaluation, V. vinifera germplasm resources
Citation: Wang Z-L, Wu D, Hui M, Wang Y, Han X, Yao F, Cao X, Li Y-H, Li H and Wang H (2022) Screening of cold hardiness-related indexes and establishment of a comprehensive evaluation method for grapevines (V. vinifera). Front. Plant Sci. 13:1014330. doi: 10.3389/fpls.2022.1014330
Received: 08 August 2022; Accepted: 02 September 2022;
Published: 24 November 2022.
Edited by:
Supratim Basu, New Mexico Consortium, United StatesReviewed by:
Yanlun Ju, Northwest A&F University, ChinaYuejin Wang, Northwest A&F University, China
Lingfei Shangguan, Nanjing Agricultural University, China
Jianfu Jiang, Zhengzhou Fruit Research Institute (CAAS), China
Copyright © 2022 Wang, Wu, Hui, Wang, Han, Yao, Cao, Li, Li and Wang. This is an open-access article distributed under the terms of the Creative Commons Attribution License (CC BY). The use, distribution or reproduction in other forums is permitted, provided the original author(s) and the copyright owner(s) are credited and that the original publication in this journal is cited, in accordance with accepted academic practice. No use, distribution or reproduction is permitted which does not comply with these terms.
*Correspondence: Hua Li, bGlodWF3aW5lQG53YWZ1LmVkdS5lZHU=; Hua Wang, d2FuZ2h1YUBud2FmdS5lZHUuZWR1
†These authors have contributed equally to this work