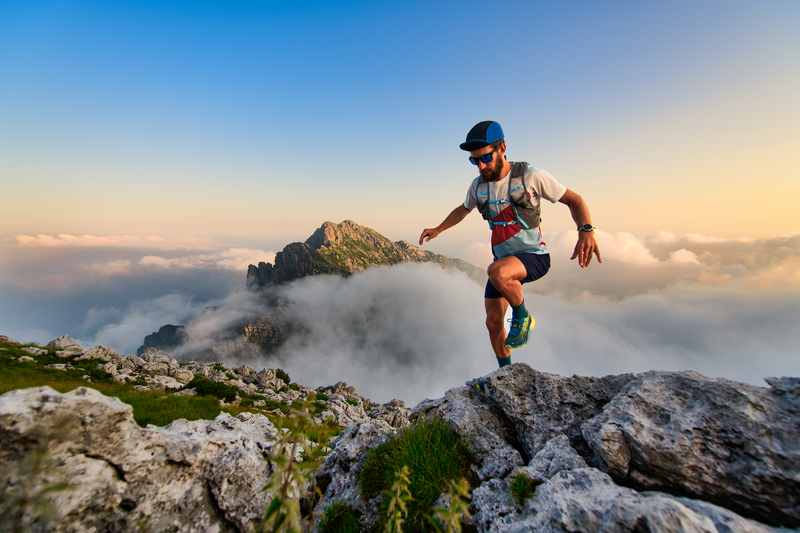
95% of researchers rate our articles as excellent or good
Learn more about the work of our research integrity team to safeguard the quality of each article we publish.
Find out more
ORIGINAL RESEARCH article
Front. Plant Sci. , 23 September 2022
Sec. Plant Bioinformatics
Volume 13 - 2022 | https://doi.org/10.3389/fpls.2022.1012277
This article is part of the Research Topic Multi-omics and Computational Biology in Horticultural Plants: From Genotype to Phenotype View all 32 articles
Quercus gilva is an ecologically and economically important species of Quercus section Cyclobalanopsis and is a dominant species in evergreen broad-leaved forests in subtropical regions of East Asia. In the present study, we reported a high-quality chromosome-scale genome assembly of Q. gilva, the first reference genome for section Cyclobalanopsis, using the combination of Illumina and PacBio sequencing with Hi-C technologies. The assembled genome size of Q. gilva was 889.71 Mb, with a contig number of 773 and a contig N50 of 28.32 Mb. Hi-C scaffolding anchored 859.07 Mb contigs (96.54% of the assembled genome) onto 12 pseudochromosomes, with a scaffold N50 of 70.35 Mb. A combination of de novo, homology-based, and transcript-based predictions predicted a final set of 36,442 protein-coding genes distributed on 12 pseudochromosomes, and 97.73% of them were functionally annotated. A total of 535.64 Mb (60.20%) of repetitive sequences were identified. Genome evolution analysis revealed that Q. gilva was most closely related to Q. suber and they diverged at 40.35 Ma, and Q. gilva did not experience species-specific whole-genome duplication in addition to the ancient gamma (γ) whole-genome triplication event shared by core eudicot plants. Q. gilva underwent considerable gene family expansion and contraction, with 598 expanded and 6,509 contracted gene families detected. The first chromosome-scale genome of Q. gilva will promote its germplasm conservation and genetic improvement and provide essential resources for better studying the evolution of Quercus section Cyclobalanopsis.
The genus Quercus, comprising approximately 450 species, is one of the most dominant woody genera throughout Asia, Europe, and America (Plomion et al., 2018; Zhou et al., 2022). Classifying Quercus genus has been historically challenging due to low interspecific differentiation and high intraspecific genetic variation partly caused by hybridization and introgression (Hipp, 2015). According to the recent molecular evidence provided by nuclear ribosomal internal transcribed spacer (ITS), restriction fragment length polymorphism (RFLP), and genomics datasets, the species in genus Quercus have been grouped into two subgenera Quercus and Cerris (Manos et al., 2001; Denk and Grimm, 2010; Denk et al., 2017; Deng et al., 2018; Hipp et al., 2020). Subgenus Quercus, which is primarily distributed in North America, includes five sections: Lobatae, Ponticae, Protobalanus, Quercus, and Virentes. Subgenus Cerris, of which the major distribution region is Eurasia, consists of Cerris, Cyclobalanopsis, and Ilex sections (Denk et al., 2017).
Quercus section Cyclobalanopsis is mainly distributed in subtropical and tropical regions of Asia (Denk and Grimm, 2010). Approximately 90 species have been recognized in section Cyclobalanopsis and are well-adapted to warm and humid climates. Due to their ecological importance, the phylogeny of Cyclobalanopsis species has been investigated using both phenotypic and molecular data over the past ten years (Denk and Grimm, 2010; Deng et al., 2013; Deng et al., 2014; Deng et al., 2018; Hipp et al., 2020), which helped us to better understand the evolutionary history of section Cyclobalanopsis. In the latest study, Deng et al. (2018) utilized restriction-site associated DNA sequencing (RAD-seq) data to resolve phylogenetic relationships of 34 Cyclobalanopsis species, inferring two major lineages that are compound trichome bases (CTB) lineage and single-celled trichome bases (STB) lineage. RAD-seq is a fractional genome sequencing strategy that usually only samples a small proportion of the genome (Davey and Blaxter, 2010; Lowry et al., 2017). Moreover, the RAD-seq approach relies on enzymes to isolate restriction site fragments; the polymorphic sites occurring at restriction sites consequently lead to missing information, resulting in potential bias in the phylogenetic estimation (Ai et al., 2022). The genome-wide sequencing data of white oaks (e.g., Q. robur, Q. lobata, Q. mongolica) have contributed significantly to resolving phylogenetic relationships within section Quercus (Plomion et al., 2018; Ai et al., 2022; Sork et al., 2022). Therefore, the availability of whole-genome sequencing data for Cyclobalanopsis species is essential to the phylogenetic inference of section Cyclobalanopsis. However, none of Cyclobalanopsis species have available genome-wide data yet.
Quercus gilva (2n=2x=24) is a representative species of section Cyclobalanopsis in East Asia, including southern and southeastern China, Japan, and Jeju Island of South Korea (Zeng et al., 2019; Han et al., 2020) (Figure 1A). Q. gilva is a native and dominant species in evergreen broad-leaved forests in subtropical areas of East Asia. In China, Q. gilva naturally distributes in mixed and secondary forests from 106°-122°E to 22°-29°N at altitudes of 300-1500 m (Zeng et al., 2019). It provides essential ecological services, including water conservation, soil protection, and carbon sequestration. Besides, its red, hard, and well-textured heartwood provides quality materials for high-end furniture and fine artware production (Zeng et al., 2019) (Figures 1B–D). Q. gilva has been therefore considered to be ecologically and economically valuable. However, the once widespread Q. gilva populations have greatly diminished as a consequence of human disturbance (e.g., large-scale logging and regional development) (Deng et al., 2018). Limited studies have been conducted on Q. gilva, which focused on its identification (Ohyama et al., 2001; Noshiro and Sasaki, 2011), marker development (Sugiura et al., 2014), genetic diversity (Sugiura et al., 2015), and potential distributions (Han et al., 2020). Although a chloroplast genome of Q. gilva was reported (Zeng et al., 2019), nuclear genome information is not available for Q. gilva.
Figure 1 Distribution and characteristics of Quercus gilva. (A) Distribution of Q. gilva based on specimen records, literature, and field survey, (B) trunk, (C) leaves, and (D) fruits of Q. gilva, (E) individual of the sampled 12-year-old Q. gilva.
We herein report the first chromosome-scale genome assembly and reveal the genome evolution of Q. gilva. This high-quality reference genome will promote germplasm conservation and genetic improvement of Q. gilva and provide essential resources for better understanding the phylogenetic relationships of Quercus section Cyclobalanopsis.
Fresh leaf tissues were sampled from a 12-year-old Q. gilva individual growing in Yuchi State-Owned Forest Farm (113.0697°E, 28.5965°N), Hunan Province, China (Figure 1E). Leaves were immediately stored in liquid nitrogen until being transported back to the laboratory and stored at -80°C. The genomic DNA extraction from leaf tissues was performed using DNeasy Plant Mini Kit (QIAGEN, Valencia, CA, USA). The quality and quantity of genomic DNA were assessed by NanoDrop 2000 spectrophotometer (Thermo Fisher Scientific, Wilmington, DE, USA), 0.8% agarose gel electrophoresis, and Qubit 3.0 fluorometer (Life Technologies, CA, USA).
Three different approaches were adopted to perform genomic DNA sequencing. Illumina libraries were constructed with ~350 bp inserts and sequenced on a NovaSeq 6000 platform (Illumina, San Diego, CA, USA) with paired-end reads of 150 bp (PE 150 bp). Paired-end adapters were removed from raw data using FastUniq v1.1 (Xu et al., 2012). Raw data were then filtered according to the following criteria: (a) duplicate read pairs; (b) reads with unknown bases ≥ 10%; (c) reads of which ≥ 50% bases with Phred quality score ≤ 5. BWA-MEM v0.7.12 (Li and Durbin, 2009) was used to filter out contamination reads. After quality control, Illumina clean reads were obtained and utilized to perform a genome survey.
PacBio library was prepared using the “Procedure & Checklist – Preparing HiFi SMRTbell® Libraries using the SMRTbell Express Template Prep Kit 2.0” protocol (Pacific Biosciences of California, Inc., CA, USA). HiFi sequencing was carried out on a PacBio Sequel II (Pacific Biosciences of California, Inc., CA, USA) with circular consensus sequencing (CCS) mode using Sequel II Binding Kit 2.2 and Sequel II Sequencing Kit 2.0. After removing adapters and low-quality reads using the PacBio SMRT Analysis module in SMRT Link v11.0, HiFi CCS clean data were obtained and used for subsequent analyses.
A Hi-C library was generated following the approach described by Lieberman-Aiden et al. (2009). Briefly, chromatin was fixed, extracted, and digested. Subsequently, DNA was purified from protein, then randomly sheared into fragment sizes of 100-500 bp, and sequenced using PE 150 bp mode on a NovaSeq 6000 platform. Data were cleaned and processed in the same manner as described in the genomic DNA sequencing for the genome survey.
Total RNA was extracted from root, leaf, and branch tissues of the same Q. gilva individual that was used for genome sequencing using RNAprep Pure Plant Kit (Tiangen, Beijing, China). RNA Nano 6000 Assay Kit of Agilent Bioanalyzer 2100 system (Agilent Technologies, CA, USA) and NanoDrop 2000 spectrophotometer (Thermo Fisher Scientific, Wilmington, DE, USA) were then used to examine RNA quality and quantity. A mixture of total RNA from three tissues was used for library construction, performed by Hieff NGS Ultima Dual-mode mRNA Library Prep Kit for Illumina (Yeasen Biotechnology, Shanghai, China) and sequenced using an Illumina NovaSeq 6000 platform with PE 150 bp mode.
Before de novo genome assembly, the genome size, repeated sequences, and heterozygosity were estimated using K-mer analysis with Illumina clean reads. The iterative selection of 19 bp base sequences (K-value=19) was used for K-mer analysis. K-mer frequency distribution was tallied and the K-mer depth distribution curve was calculated, which were then used to evaluate genome size, the percentage of repeated sequences, and heterozygosity ratio as described by Li et al. (2019).
HiFi CCS clean reads were initially assembled using Hifiasm v0.15.4 (Cheng et al., 2021) with parameters -z20 to trim both ends of reads by 20 bp. BWA-MEM v0.7.12 (Li and Durbin, 2009) was then used to align the Hi-C clean data onto the assembled genome. The read pairs were independently mapped to the genome assembly and only read pairs that were uniquely mapped were used in subsequent analyses. Valid interaction paired-end reads were used to cluster, sort, and correct the contigs into 12 pseudochromosomes using 3D-DNA (Dudchenko et al., 2017) and manual inspection was performed with Juicebox v1.22 (Robinson et al., 2018).
The quality of the assembled Q. gilva genome was assessed from four aspects. First, Illumina clean reads and CCS clean reads were re-aligned onto the final assembly by BWA-MEM v0.7.12 (Li and Durbin, 2009) and Minimap2 v2.24 (Li, 2018), respectively. Second, BUSCO v5.2.2 assessment (Simão et al., 2015) was performed using the embryophyta_odb10 dataset and default parameters. Third, the long terminal repeat (LTR) Assembly Index (LAI) was applied to assess the assembly continuity as described previously (Ou et al., 2018). Finally, the occurrence of telomeric tandem repeat ((TTAGGG/CCCTAA)n) was examined on both edges of 12 pseudochromosomes to assess the completeness and accuracy of our chromosomal assembly.
The repetitive sequences that include tandem repeats and transposable elements (TEs) in the Q. gilva genome were identified. Tandem repeats were annotated using MISA v2.1 (Thiel et al., 2003). TEs in the assembled genome were identified using de novo and homology-based approaches. A de novo repetitive sequence library of the Q. gilva genome was constructed using RepeatModeler v2.0 (http://www.repeatmasker.org/RepeatModeler/) and TEs were subsequently identified using RepeatMasker v4.0.5 (Tarailo-Graovac and Chen, 2009). For the homology-based approach, the assembled Q. gilva genome was aligned against the Repbase database v20.05 (Bao et al., 2015) using RepeatMasker v4.0.5 with default parameters for TEs identification. Results from these two approaches were merged to yield final TEs in the assembled Q. gilva genome.
A combination strategy of de novo, homology-based, and transcript-based predictions was applied to predict protein-coding genes. De novo prediction was performed using AUGUSTUS v3.3.3 (Stanke et al., 2004) on the repeat-masked sequences. In homology-based prediction, the protein sequences of Quercus aquifolioides, Quercus lobata (Sork et al., 2022), Quercus mongolica (Ai et al., 2022), Quercus robur (Plomion et al., 2018), and Quercus suber (Ramos et al., 2018) were aligned against the Q. gilva assembly using TBLASTN v2.60 (Gertz et al., 2006). GeMoMa v1.8 (Keilwagen et al., 2016) was then employed to predict protein-coding genes based on homologous sequences. In the transcript-based approach, RNA sequencing clean data were mapped to the Q. gilva genome by HISAT2 v2.2.0 (Kim et al., 2019), and transcripts were then assembled using StringTie v2.1.3 (Pertea et al., 2015). PASA v2.4.1 (Haas et al., 2003) was utilized to predict gene models. MAKER v3.1.2 (Cantarel et al., 2008) was used to integrate the results from de novo, homology-based, and transcript-based approaches to generate a consensus gene set.
Functional annotation of the predicted genes was performed by searching for the best matches of alignments in non-redundant (NR) (https://ftp.ncbi.nlm.nih.gov/blast/db/FASTA/), Swiss-prot (Boeckmann et al., 2003), and Eukaryotic Orthologous Groups (KOG) (Tatusov et al., 2003) using BLASTP v.2.7.1 (Camacho et al., 2009) with e-value ≤ 1e–5. Gene Ontology (GO) (Ashburner et al., 2000) terms were assigned to the predicted genes based on eggNog-mapper v2.1.6 (Cantalapiedra et al., 2021) annotation. Putative gene pathways were inferred based on the Kyoto Encyclopedia of Genes and Genomes (KEGG) databases using the BlastKOALA webservice (http://www.kegg.jp/blastkoala/) (Kanehisa et al., 2016). Protein domains and motifs were characterized using InterProScan v5.42-78.0 (Jones et al., 2014) with Pfam (Finn et al., 2014) database.
Non-coding RNAs (ncRNAs), which include ribosomal RNAs (rRNAs), transfer RNAs (tRNAs), micro RNAs (miRNAs), and small nuclear RNAs (snRNAs), were identified through sequence alignment to the Rfam database (Griffiths-Jones et al., 2005). The rRNAs were identified using RNAmmer v1.2 (Lagesen et al., 2007). The tRNAs were predicted using tRNAscan-SE v1.3.1 (Schattner et al., 2005) with eukaryote parameters. Infernal v1.1 (Nawrocki and Eddy, 2013) was used to detect miRNAs and snRNAs.
Protein sequences of Arabidopsis thaliana, Betula platyphylla, Castanea dentata, Fagus sylvatica, Oryza sativa, Populus trichocarpa, Q. gilva, Q. lobata, Q. suber, and Ricinus communis were used to cluster the orthologous genes. For each gene, only the longest transcript was retained and protein sequences that are less than 50 amino acids in length or have internal stop codons were filtered. Gene family clustering analysis was then performed using OrthoFinder v2.5.2 (Emms and Kelly, 2019) with filtered protein sequences of the 10 species.
A phylogenetic tree on a basis of shared single-copy orthologous genes was generated for A. thaliana, B. platyphylla, C. dentata, F. sylvatica, O. sativa, P. trichocarpa, Q. gilva, Q. lobata, Q. suber, and R. communis. MAFFT v7.490 (Katoh and Standley, 2013) was used to independently perform the multiple sequence alignment for each gene, and Gblocks v0.91b (Talavera and Castresana, 2007) was then utilized to filter poorly aligned sequences. Protein sequences of all single-copy orthologous genes were concatenated, which was then used to construct a maximum-likelihood (ML) tree by RAxML v8.2.12 (Stamatakis, 2014) with PROTGAMMALGX model of sequence evolution.
Divergence times between Q. gilva and nine other species were estimated with MCMCTree in PAML v4.10.0 (Yang, 2007). Two calibration nodes were used in divergence time estimation. The first calibration was the divergence time between A. thaliana and O. sativa (152 Ma) obtained from the TimeTree database (http://www.timetree.org) (Kumar et al., 2017), which is widely used to estimate divergence times among plant species. The pollen fossil of a Quercus specimen (Hofmann et al., 2011) that has been commonly used to constrain the node of genus Quercus (Hipp et al., 2020; Zhou et al., 2022) was used to calibrate the stem node of Q. gilva, Q. suber, and Q. lobata with 56 (54-60) Ma.
To examine gene family expansion and contraction between the ancestor and each species, gene family clustering and phylogenetic analysis results were inputted into CAFÉ v3.1 (De Bie et al., 2006). Significant gene family expansion and contraction were determined with P-value ≤ 0.05. Functional enrichment analysis was conducted to identify expanded and contracted gene families in the Q. gilva genome. GO term assignment and KEGG pathway analysis were performed using eggNog-mapper v2.1.6 (Cantalapiedra et al., 2021) and BlastKOALA webservice (Kanehisa et al., 2016), respectively.
Colinear maps were generated by comparing genome sequences on 12 pseudochromosomes of Q. gilva with Q. lobata and Q mongolica genomes using MUMmer v4.0 (Marçais et al., 2018) to investigate the syntenic relationship between Q. gilva and these two oak genomes. Additionally, synteny analysis was performed between Q. gilva and Q. lobata genomes and between Q. gilva and Q. mongolica genomes using JCVI v1.1.19 (Tang et al., 2008) with following parameters: – cscore=.99, –minspan=30. The block comprising at least five sequential genes and with C-score≥0.99 was defined as the initial syntenic block and the syntenic blocks spanning more than 30 genes were displayed in the synteny map. According to the previous findings that Q. lobata does not undergo species-specific whole-genome duplication (WGD) events besides the ancient gamma whole-genome triplication (γ-WGT) event shared by core eudicot plants (Ai et al., 2022), Q. lobata and Q. suber were selected for the inference of WGD events in Q. gilva. Protein sequences of these species were compared with Q. gilva genome to identify syntenic blocks and syntenic genes using BLASTP v.2.7.1 (Camacho et al., 2009) (e-value ≤ 1e–5). Synonymous substitution rate (Ks) of the syntenic gene pairs within and among genomes was calculated using KaKs_Calculator 2.0 (Wang et al., 2010) and ParaAT v2.0 (Zhang et al., 2012), respectively. The probability density distribution curve of Ks was visualized using R software, and WGD events were inferred from the distribution peaks.
A genome survey was performed to predict the genome size, repeated sequences, and heterozygosity of Q. gilva using K-mer analysis based on ~55.66 Gb of Illumina clean data (Supplementary Table 1). With a K-mer number of 47,492,571,457 and K-mer depth of ~54.86, the genome size was estimated to be ~865.75 Mb. A high level of heterozygosity ratio of 1.16% and ~48.17% of repeated sequences were observed (Supplementary Figure 1; Supplementary Table 2).
A total of ~30.76 Gb of HiFi CCS clean reads were produced by the PacBio Sequel II and used for the subsequent genome assembly (Supplementary Table 1). The contigs were then polished with HiFi CCS clean data, generating a genome assembly of 889.71 Mb, with a number of contigs of 773 and a contig N50 of 28.32 Mb (Table 1). The contig N50 of the assembled Q. gilva genome is ~11-fold and ~405-fold compared with Q. mongolica and Q. robur, respectively. In total, ~121.70 Gb of Hi-C data were obtained and connected to 12 pseudochromosomes (Supplementary Table 1). Finally, 859.07 Mb of sequences (96.54% of the genome assembly) were anchored onto 12 pseudochromosomes, ranging in sizes of 40.26-104.15 Mb (Figure 2; Supplementary Figure 2; Supplementary Table 3). The chromosome-scale genome assembly of Q. gilva was characterized by a scaffold number of 515 and a scaffold N50 of 70.35 Mb. The scaffold N50 of Q. gilva is similar to that of Q. mongolica (66.74 Mb) and Q. lobata (75.00 Mb) while ~53-fold than Q. robur (1.34 Mb).
Figure 2 Features of the Quercus gilva genome. The outermost circle represents the 12 pseudochromosomes, with red dot at the end indicating telomeric repeat ((TTAGGG/CCCTAA)n) reached. From outer to inner circles: (A) sequence coverage by PacBio CSS clean reads; (B) LTR Assembly Index (LAI); (C) gene density; (D) GC content. (A–D) were drawn in 50 kb non-overlapping sliding windows. The intragenomic syntenic blocks were indicated by the innermost circle.
The quality of the Q. gilva genome assembly was assessed by four approaches. First, 100% of Illumina short reads and 99.85% of CCS clean reads were mapped to the assembled genome, which could cover 96.22% and 99.87% of the assembled genome sequence, respectively (Figure 2; Supplementary Table 4). Second, among 1,614 Benchmarking Universal Single-Copy Orthologs (BUSCO) genes, 98.6% of complete BUSCOs, including 93.5% of complete single-copy and 5.1% of complete duplicated, were detectable in our assembled genome (Supplementary Figure 3). Third, LAI of the Q. gilva genome assembly is 22.71 which is greater than the gold standard quality level of the assembly (LAI=20) (Ou et al., 2018) (Supplementary Figure 4). Additionally, our chromosomal assembly reached telomeric tandem repeats on both ends of six pseudochromosomes and on one end of six more (Figure 2). These results elucidated the high completeness and quality of our Q. gilva genome assembly.
In total, 929,678 tandem repeats, including 663,186 mono-, 199,396 di-, 53,203 tri-, 10,358 tetra-, 2,064 penta-, and 1,471 hexa-nucleotide repeats, were identified in the Q. gilva genome, accounting for ~2.63% of the assembled genome (23.38 Mb) (Supplementary Table 5). Approximately 512.26 Mb (~57.57% of the genome) of TEs were identified via the combination of de novo and homology-based predictions, with 0.20% of short interspersed nuclear elements (SINEs), 3.89% of long interspersed nuclear elements (LINEs), 18.67% of long terminal repeats (LTRs), and 2.41% of DNA transposons. The percentage of TEs in Q. gilva is comparable to that of Q. lobata (54.4%) and Q. robur (53.3%).
A final set of 36,442 protein-coding genes distributed on 12 pseudochromosomes was predicted through a combination of de novo, homology-based, and transcript-based approaches, with average exons per gene of 4.5 (Table 1; Supplementary Figure 5; Supplementary Table 6). The average gene and CDS length were 3,724 and 980 bp, respectively. In total, ~97.73% of the predicted protein-coding genes (35,615 genes) were functionally annotated in the databases described above (Supplementary Figure 6; Supplementary Table 7). The ncRNAs were identified in the Q. gilva genome, which included 709 tRNAs, 1,798 rRNAs, 38 miRNAs, and 142 snRNAs.
Gene family clustering analysis assigned 235,227 genes from Q. gilva and nine other species to 20,844 orthogroups. A total of 13,241 genes clustered into 9,259 gene families were revealed in Q. gilva genome through comparisons of protein sequences homologous between Q. gilva and nine other species (Supplementary Figure 7; Supplementary Table 8). In total, 1,244 single-copy orthologous genes were shared among Q. gilva and nine other species, which were used to construct a phylogenetic tree and to estimate species divergence time (Figure 3).
Figure 3 Phylogenetic tree based on shared single-copy gene families among Quercus gilva and nine other species. Inferred maximum-likelihood (ML) phylogenetic tree was generated on the basis of 1,244 single-copy orthologous genes across 10 species. The numerical value at the right of each node shows the estimated divergence time in millions of years. Red dots indicate calibrated nodes. Numbers in green (+) and red (−) show the number of expanded and contracted gene families, respectively. The green, red, and blue portions of the pie charts indicate the percentage of gene families undergoing expansion, contraction, and rapidly evolving event, respectively.
The maximum-likelihood phylogenetic tree indicated that Q. gilva (in section Cyclobalanopsis) was most closely related to Q. suber (in section Cerris), with a divergence time at ~40.35 (13.40-50.81) Ma. The estimated divergence time of Q. lobata (species of subgenus Quercus) from the common ancestor of Q. gilva and Q. suber (members of subgenus Cerris) was ~47.45 (41.97-53.63) Ma. The estimated split between C. dentata and three Quercus species was ~51.75 (47.98-55.88) Ma.
In total, 598 expanded and 6,509 contracted gene families have been observed in the Q. gilva genome (Figure 3). Among them, 41 and 207 gene families were significantly expanded and contracted (P<0.05), respectively. The expanded gene families in the Q. gilva were significantly enriched in 565 GO terms (Q<0.01), which were primarily enriched in cellular component of cell (GO:0005623, 59 genes) and molecular function of catalytic activity (GO:0003824, 52 genes) (Supplementary Table 9). The contracted gene families showed significant enrichment in 398 GO terms, with major enrichment in biological process of cellular process (GO:0009987, 37 genes) and cellular component of cell part (GO:0044464, 37 genes) (Supplementary Table 10). KEGG enrichment analysis of expanded gene families revealed only two significantly enriched pathways (Q<0.01) (Supplementary Table 11). While the contracted gene families were found to be significantly enriched in 15 KEGG pathways, with the chief enrichment in plant-pathogen interaction (ko04626) (Supplementary Table 12).
Colinear maps were generated by comparing Q. gilva genome with Q. lobata (Figure 4A) and Q. mongolica (Figure 4B) genomes. Both maps showed a small proportion (6.4% between Q. gilva and Q. mongolica genomes and 12.2% between Q. gilva and Q. lobata genomes) of blue dots showing the identical sequence in the opposite orientation, which elucidated high similarity between Q. gilva and Q. lobata genomes and between Q. gilva and Q. mongolica genomes. Moreover, syntenic blocks were generated for Q. gilva versus Q. lobata genomes and Q. gilva versus Q. mongolica genomes (Figure 4C). In total, 174 and 104 syntenic blocks have been obtained from the comparison of Q. gilva versus Q. lobata genomes and Q. gilva versus Q. mongolica genomes, respectively. A one-to-one corresponding relationship of the 12 chromosomes was observed between Q. gilva and Q. lobata genomes and between Q. gilva and Q. mongolica genomes.
Figure 4 Syntenic analysis through comparisons of the 12 pseudochromosomes of Q. gilva with Q. lobata and Q. mongolica. (A) Colinear map of the Q. gilva and Q. lobata genomes. (B) Colinear map of the Q. gilva and Q. mongolica genomes. Red and blue dots indicate the identical sequence in the same and opposite orientation, respectively. (C) Chromosome-level syntenic comparisons based on gene pairs between Q. gilva and Q. lobata and between Q. gilva and Q. mongolica. Syntenic blocks with more than 30 genes are connected by grey lines.
The distribution curves of Ks for Q. gilva and Q. lobata showed a peak at ~1.3 Ks units (Supplementary Figures 8, 9), indicating these two species shared a WGD event that is gamma whole-genome triplication (γ-WGT) event in the common ancestor of core eudicots. Another peak at low values of Ks (0.1-0.2) nearly fitted with exponential distribution was observed in three Quercus species, which indicated the recent burst of local gene duplications. The peak value of orthologous gene pairs of Q. gilva versus Q. lobata and Q. gilva versus Q. suber (Ks value of 1.1) was lower than the peak value of paralogous gene pairs of Q. gilva and Q. lobata, implying that the divergence between Q. gilva and two other Quercus species occurred later than the shared γ-WGT and Q. gilva did not experience species-specific WGD events.
In the present study, a high-quality chromosome-scale genome assembly of Q. gilva was generated by employing a combination strategy of Illumina NovaSeq 6000, PacBio Sequel II, and Hi-C sequencing technologies. The assembled genome size of Q. gilva was ~890 Mb, with contig and scaffold N50 values of 28.32 and 70.35 Mb, respectively. Greater than 96% of the Q. gilva genome sequences (~859 Mb) were anchored onto the 12 pseudochromosomes that ranged in a size of 40.26-104.15 Mb. Consistent with Q. lobata and Q. mongolica genomes, the greatest number of genes were observed on chromosome 2 (4,774) among the 12 chromosomes of Q. gilva. However, differing from Q. lobata and Q. mongolica genomes that chromosome 2 was found to be the longest, chromosome 4 is slightly longer (104.15 Mb) than chromosome 2 (101.11 Mb) in our Q. gilva genome assembly. The increase in the length of chromosome 4 may be caused by the high level of transposable elements (TEs) (~69 Mb) present on it. Genome assembly of woody plants has been generally challenged due to high levels of duplication and heterozygosity. The level of repetitive elements, specifically TEs, was found to be constantly high in genus Quercus, for instance, 51.78% in Q. mongolica (Ai et al., 2022), 53.3% in Q. robur (Plomion et al., 2018), 54.4% in Q. lobata (Sork et al., 2022), and 57.57% in Q. gilva. The heterozygosity of Q. gilva was estimated at 1.16%, which is comparable to Q. mongolica (1.09%) (Ai et al., 2022) and Q. lobata (1.25%) (Sork et al., 2016). Although high levels of repetitive sequences and heterozygosity are present in Q. gilva genome, our assembled genome displays a high assembly quality, with 98.6% of complete BUSCOs detected in the genome assembly, an LAI score of 22.71, and telomeric tandem repeats reaching at both ends of six pseudochromosomes and at one end of six more. This Q. gilva genome is the first reference genome for Quercus section Cyclobalanopsis. It will provide essential information to better understand the evolution of this dominant lineage in East Asia.
Phylogenetic analysis revealed that Q. gilva was most related to Q. suber (section Cerris), with an estimated divergence time of 40.35 (13.40-50.81) Ma, and the estimated split time between two subgenus Cerris species (Q. gilva and Q. suber) and Q. lobata (subgenus Quercus) was 47.45 (41.97-53.63) Ma. The result is consistent with the phylogenetic structure resolved by previous reports that the divergence of section Cyclobalanopsis from sections Cerris and Ilex and the divergence between subgenera Cerris and Quercus occurred in the early Eocene (Hipp et al., 2020; Zhou et al., 2022). While our study suggested earlier divergences between Quercus species than those proposed by Deng et al. (2018), which may be due to the different fossil calibrations of genus Quercus used in this previous study. Analysis of WGD event based on Ks distribution elucidated that Q. gilva and Q. lobata only underwent the gamma whole-genome triplication (γ-WGT) that was shared by core eudicot plants with a Ks peak value of 1.3. A deviated peak value (Ks of 1.1) was observed in Q. suber, which supports the previous finding that this deviation may be caused by the low-quality assembly of Q. suber using second-generation sequencing (Ai et al., 2022). Moreover, Ks distribution curve elucidated that the divergence between Q. gilva and the other two Quercus species occurred later than the shared γ-WGT and Q. gilva did not experience species-specific WGD events since Ks peak value (1.1) of orthologous gene pairs of Q. gilva versus Q. lobata and Q. gilva versus Q. suber was lower than the peak value (1.3) of paralogous gene pairs of Q. gilva and Q. lobata. The WGD event could also be inferred from the high level of synteny between Q. gilva and the other two Quercus genomes. A one-to-one corresponding relationship of the 12 chromosomes was found between Q. gilva and Q. lobata and between Q. gilva and Q. mongolica. At the same time, fewer inversions occurred between Q. gilva and Q. mongolica genomes, which may be due to the use of PacBio Sequel II sequencing technology with higher accuracy in Q. gilva and Q. mongolica. Based on the previous findings that Q. lobata and Q. mongolica did not experience lineage-specific WGD besides γ-WGT (Ai et al., 2022) and the high collinearity between Q. gilva and these two species, we could confirm that no lineage-specific WGD occurred in Q. gilva.
Compared with Q. suber and Q. lobata, the Q. gilva genome experienced considerable gene family contraction, with genes related to the plant-pathogen interaction pathway significantly contracted in the Q. gilva genome. The previous study indicated that as the key component of plant-pathogen interaction, disease-resistance (R) genes strongly expanded in Q. robur (Plomion et al., 2018). Our study may not support this point, however, it is consistent with the finding observed on Q. mongolica that the gene families in the plant-pathogen interaction pathway of this Asian oak exhibited significant contraction and the R gene number in Q. mongolica was considerably lower compared with other oak species (Ai et al., 2022). In the East Asian environment, the absence of some pathogens may result in a reduced number of corresponding resistance genes from the standpoint of fitness cost, leading to the contraction of related genes (Tian et al., 2003).
In conclusion, we herein report a high-quality chromosome-scale genome assembly of Q. gilva, the first reference genome for Quercus section Cyclobalanopsis, and elucidate the genome evolution of this ecologically and economically important species. Our study will promote germplasm conservation and genetic improvement of Q. gilva and provide valuable resources for a better understanding of the evolution of Quercus section Cyclobalanopsis.
The datasets presented in this study can be found in online repositories. The names of the repository/repositories and accession number(s) can be found below: https://www.ncbi.nlm.nih.gov/, PRJNA833760; https://figshare.com/, 10.6084/m9.figshare.20411082.
HL and FC conceived and designed this research. XZ, NL, XJ, and ZQ conducted the genome data analyses. XZ and NL wrote the manuscript. TF participated in the interpretation and discussion of results and revised the manuscript. All authors have reviewed and approved the final version of this manuscript.
This work was funded by National Natural Science Foundation of China (32201589), Natural Science Foundation of Hunan Province (2021JJ41069), and Forestry Scientific and Technological Innovation Project of Hunan Forestry Department (XLKY202218).
We would like to thank Changsha Luo and Tiantian Li for their technical support on genome data analysis.
The authors declare that the research was conducted in the absence of any commercial or financial relationships that could be construed as a potential conflict of interest.
All claims expressed in this article are solely those of the authors and do not necessarily represent those of their affiliated organizations, or those of the publisher, the editors and the reviewers. Any product that may be evaluated in this article, or claim that may be made by its manufacturer, is not guaranteed or endorsed by the publisher.
The Supplementary Material for this article can be found online at: https://www.frontiersin.org/articles/10.3389/fpls.2022.1012277/full#supplementary-material
Ai, W., Liu, Y., Mei, M., Zhang, X., Tan, E., Liu, H., et al. (2022). A chromosome-scale genome assembly of the Mongolian oak (Quercus mongolica). Mol. Ecol. Resour. 22 (6), 2396–2410. doi: 10.1111/1755-0998.13616
Ashburner, M., Ball, C. A., Blake, J. A., Botstein, D., Butler, H., Cherry, J. M., et al. (2000). Gene ontology: tool for the unification of biology. Nat. Genet. 25 (1), 25–29. doi: 10.1038/75556
Bao, W., Kojima, K. K., Kohany, O. (2015). Repbase update, a database of repetitive elements in eukaryotic genomes. Mobile DNA 6 (1), 11. doi: 10.1186/s13100-015-0041-9
Boeckmann, B., Bairoch, A., Apweiler, R., Blatter, M.-C., Estreicher, A., Gasteiger, E., et al. (2003). The SWISS-PROT protein knowledgebase and its supplement TrEMBL in 2003. Nucleic Acids Res. 31 (1), 365–370. doi: 10.1093/nar/gkg095
Camacho, C., Coulouris, G., Avagyan, V., Ma, N., Papadopoulos, J., Bealer, K., et al. (2009). BLAST+: architecture and applications. BMC Bioinf. 10 (1), 421. doi: 10.1186/1471-2105-10-421
Cantalapiedra, C. P., Hernández-Plaza, A., Letunic, I., Bork, P., Huerta-Cepas, J. (2021). eggNOG-mapper v2: Functional annotation, orthology assignments, and domain prediction at the metagenomic scale. Mol. Biol. Evol. 38 (12), 5825–5829. doi: 10.1093/molbev/msab293
Cantarel, B. L., Korf, I., Robb, S. M. C., Parra, G., Ross, E., Moore, B., et al. (2008). MAKER: An easy-to-use annotation pipeline designed for emerging model organism genomes. Genome Res. 18 (1), 188–196. doi: 10.1101/gr.6743907
Cheng, H., Concepcion, G. T., Feng, X., Zhang, H., Li, H. (2021). Haplotype-resolved de novo assembly using phased assembly graphs with hifiasm. Nat. Methods 18 (2), 170–175. doi: 10.1038/s41592-020-01056-5
Davey, J. W., Blaxter, M. L. (2010). RADSeq: next-generation population genetics. Briefings Funct. Genomics 9 (5-6), 416–423. doi: 10.1093/bfgp/elq031
De Bie, T., Cristianini, N., Demuth, J. P., Hahn, M. W. (2006). CAFE: a computational tool for the study of gene family evolution. Bioinformatics 22 (10), 1269–1271. doi: 10.1093/bioinformatics/btl097
Deng, M., Hipp, A. L., Song, Y.-G., Li, Q.-S., Coombes, A., Cotton, A. (2014). Leaf epidermal features of quercus subgenus cyclobalanopsis (Fagaceae) and their systematic significance. Botanical J. Linn. Soc. 176 (2), 224–259. doi: 10.1111/boj.12207
Deng, M., Jiang, X. L., Hipp, A. L., Manos, P. S., Hahn, M. (2018). Phylogeny and biogeography of East Asian evergreen oaks (Quercus section cyclobalanopsis; fagaceae): Insights into the Cenozoic history of evergreen broad-leaved forests in subtropical Asia. Mol. Phylogenet Evol. 119, 170–181. doi: 10.1016/j.ympev.2017.11.003
Deng, M., Zhou, Z. K., Li, Q. S. (2013). Taxonomy and systematics of quercus subgenus cyclobalanopsis. Int. Oaks 24, 48–60.
Denk, T., Grimm, G. W. (2010). The oaks of western Eurasia: Traditional classifications and evidence from two nuclear markers. TAXON 59 (2), 351–366. doi: 10.1002/tax.592002
Denk, T., Grimm, G. W., Manos, P. S., Deng, M., Hipp, A. L. (2017). “An updated infrageneric classification of the oaks: Review of previous taxonomic schemes and synthesis of evolutionary patterns,” in Oaks physiological ecology. exploring the functional diversity of genus quercus l. Eds. Gil-Pelegrín, E., Peguero-Pina, J. J., Sancho-Knapik, D. (Cham: Springer International Publishing), 13–38.
Dudchenko, O., Batra Sanjit, S., Omer Arina, D., Nyquist Sarah, K., Hoeger, M., Durand Neva, C., et al. (2017). De novo assembly of the aedes aegypti genome using Hi-c yields chromosome-length scaffolds. Science 356 (6333), 92–95. doi: 10.1126/science.aal3327
Emms, D. M., Kelly, S. (2019). OrthoFinder: phylogenetic orthology inference for comparative genomics. Genome Biol. 20 (1), 238. doi: 10.1186/s13059-019-1832-y
Finn, R. D., Bateman, A., Clements, J., Coggill, P., Eberhardt, R. Y., Eddy, S. R., et al. (2014). Pfam: the protein families database. Nucleic Acids Res. 42 (D1), D222–D230. doi: 10.1093/nar/gkt1223
Gertz, E. M., Yu, Y.-K., Agarwala, R., Schäffer, A. A., Altschul, S. F. (2006). Composition-based statistics and translated nucleotide searches: Improving the TBLASTN module of BLAST. BMC Biol. 4 (1), 41. doi: 10.1186/1741-7007-4-41
Griffiths-Jones, S., Moxon, S., Marshall, M., Khanna, A., Eddy, S. R., Bateman, A. (2005). Rfam: annotating non-coding RNAs in complete genomes. Nucleic Acids Res. 33 (suppl_1), D121–D124. doi: 10.1093/nar/gki081
Haas, B. J., Delcher, A. L., Mount, S. M., Wortman, J. R., Smith, R. K., Jr., Hannick, L. I., et al. (2003). Improving the arabidopsis genome annotation using maximal transcript alignment assemblies. Nucleic Acids Res. 31 (19), 5654–5666. doi: 10.1093/nar/gkg770
Han, E.-K., Cho, W.-B., Park, J.-S., Choi, I.-S., Kwak, M., Kim, B.-Y., et al. (2020). A disjunctive marginal edge of evergreen broad-leaved oak (Quercus gilva) in East Asia: The high genetic distinctiveness and unusual diversity of jeju island populations and insight into a massive, independent postglacial colonization. Genes 11 (10), 1114. doi: 10.3390/genes11101114
Hipp, A. L. (2015). Should hybridization make us skeptical of the oak phylogeny. Int. Oaks 26, 9–18.
Hipp, A. L., Manos, P. S., Hahn, M., Avishai, M., Bodénès, C., Cavender-Bares, J., et al. (2020). Genomic landscape of the global oak phylogeny. New Phytol. 226 (4), 1198–1212. doi: 10.1111/nph.16162
Hofmann, C.-C., Mohamed, O., Egger, H. (2011). A new terrestrial palynoflora from the Palaeocene/Eocene boundary in the northwestern tethyan realm (St. pankraz, Austria). Rev. Palaeobotany Palynol. 166 (3), 295–310. doi: 10.1016/j.revpalbo.2011.06.003
Jones, P., Binns, D., Chang, H.-Y., Fraser, M., Li, W., McAnulla, C., et al. (2014). InterProScan 5: genome-scale protein function classification. Bioinformatics 30 (9), 1236–1240. doi: 10.1093/bioinformatics/btu031
Kanehisa, M., Sato, Y., Morishima, K. (2016). BlastKOALA and GhostKOALA: KEGG tools for functional characterization of genome and metagenome sequences. J. Mol. Biol. 428 (4), 726–731. doi: 10.1016/j.jmb.2015.11.006
Katoh, K., Standley, D. M. (2013). MAFFT multiple sequence alignment software version 7: Improvements in performance and usability. Mol. Biol. Evol. 30 (4), 772–780. doi: 10.1093/molbev/mst010
Keilwagen, J., Wenk, M., Erickson, J. L., Schattat, M. H., Grau, J., Hartung, F. (2016). Using intron position conservation for homology-based gene prediction. Nucleic Acids Res. 44 (9), e89–e89. doi: 10.1093/nar/gkw092
Kim, D., Paggi, J. M., Park, C., Bennett, C., Salzberg, S. L. (2019). Graph-based genome alignment and genotyping with HISAT2 and HISAT-genotype. Nat. Biotechnol. 37 (8), 907–915. doi: 10.1038/s41587-019-0201-4
Kumar, S., Stecher, G., Suleski, M., Hedges, S. B. (2017). TimeTree: A resource for timelines, timetrees, and divergence times. Mol. Biol. Evol. 34 (7), 1812–1819. doi: 10.1093/molbev/msx116
Lagesen, K., Hallin, P., Rødland, E. A., Stærfeldt, H.-H., Rognes, T., Ussery, D. W. (2007). RNAmmer: consistent and rapid annotation of ribosomal RNA genes. Nucleic Acids Res. 35 (9), 3100–3108. doi: 10.1093/nar/gkm160
Li, H. (2018). Minimap2: pairwise alignment for nucleotide sequences. Bioinformatics 34 (18), 3094–3100. doi: 10.1093/bioinformatics/bty191
Li, H., Durbin, R. (2009). Fast and accurate short read alignment with burrows–wheeler transform. Bioinformatics 25 (14), 1754–1760. doi: 10.1093/bioinformatics/btp324
Li, G., Song, L., Jin, C., Li, M., Gong, S., Wang, Y. (2019). Genome survey and SSR analysis of apocynum venetum. Biosci. Rep. 39 (6), BSR20190146. doi: 10.1042/BSR20190146
Lieberman-Aiden, E., van Berkum, N. L., Williams, L., Imakaev, M., Ragoczy, T., Telling, A., et al. (2009). Comprehensive mapping of long-range interactions reveals folding principles of the human genome. Science 326 (5950), 289–293. doi: 10.1126/science.1181369
Lowry, D. B., Hoban, S., Kelley, J. L., Lotterhos, K. E., Reed, L. K., Antolin, M. F., et al. (2017). Breaking RAD: an evaluation of the utility of restriction site-associated DNA sequencing for genome scans of adaptation. Mol. Ecol. Resour. 17 (2), 142–152. doi: 10.1111/1755-0998.12635
Manos, P. S., Zhou, Z.-K., Cannon, C. (2001). Systematics of fagaceae: phylogenetic tests of reproductive trait evolution. Int. J. Plant Sci. 162 (6), 1361–1379. doi: 10.1086/322949
Marçais, G., Delcher, A. L., Phillippy, A. M., Coston, R., Salzberg, S. L., Zimin, A. (2018). MUMmer4: A fast and versatile genome alignment system. PloS Comput. Biol. 14 (1), e1005944. doi: 10.1371/journal.pcbi.1005944
Nawrocki, E. P., Eddy, S. R. (2013). Infernal 1.1: 100-fold faster RNA homology searches. Bioinformatics 29 (22), 2933–2935. doi: 10.1093/bioinformatics/btt509
Noshiro, S., Sasaki, Y. (2011). Identification of Japanese species of evergreen quercus and lithocarpus (Fagaceae). IAWA J. 32 (3), 383–393. doi: 10.1163/22941932-90000066
Ohyama, M., Baba, K.i., Itoh, T. (2001). Wood identification of JapaneseCyclobalanopsis species (Fagaceae) based on DNA polymorphism of the intergenic spacer betweentrnT andtrnL 5′ exon. J. Wood Sci. 47 (2), 81–86. doi: 10.1007/bf00780554
Ou, S., Chen, J., Jiang, N. (2018). Assessing genome assembly quality using the LTR assembly index (LAI). Nucleic Acids Res. 46 (21), e126–e126. doi: 10.1093/nar/gky730
Pertea, M., Pertea, G. M., Antonescu, C. M., Chang, T.-C., Mendell, J. T., Salzberg, S. L. (2015). StringTie enables improved reconstruction of a transcriptome from RNA-seq reads. Nat. Biotechnol. 33 (3), 290–295. doi: 10.1038/nbt.3122
Plomion, C., Aury, J.-M., Amselem, J., Leroy, T., Murat, F., Duplessis, S., et al. (2018). Oak genome reveals facets of long lifespan. Nat. Plants 4 (7), 440–452. doi: 10.1038/s41477-018-0172-3
Ramos, A. M., Usié, A., Barbosa, P., Barros, P. M., Capote, T., Chaves, I., et al. (2018). The draft genome sequence of cork oak. Sci. Data 5 (1), 180069. doi: 10.1038/sdata.2018.69
Robinson, J. T., Turner, D., Durand, N. C., Thorvaldsdóttir, H., Mesirov, J. P., Aiden, E. L. (2018). Juicebox.js provides a cloud-based visualization system for Hi-c data. Cell Syst. 6 (2), 256–258.e251. doi: 10.1016/j.cels.2018.01.001
Schattner, P., Brooks, A. N., Lowe, T. M. (2005). The tRNAscan-SE, snoscan and snoGPS web servers for the detection of tRNAs and snoRNAs. Nucleic Acids Res. 33 (suppl_2), W686–W689. doi: 10.1093/nar/gki366
Simão, F. A., Waterhouse, R. M., Ioannidis, P., Kriventseva, E. V., Zdobnov, E. M. (2015). BUSCO: assessing genome assembly and annotation completeness with single-copy orthologs. Bioinformatics 31 (19), 3210–3212. doi: 10.1093/bioinformatics/btv351
Sork, V. L., Cokus, S. J., Fitz-Gibbon, S. T., Zimin, A. V., Puiu, D., Garcia, J. A., et al. (2022). High-quality genome and methylomes illustrate features underlying evolutionary success of oaks. Nat. Commun. 13 (1), 2047. doi: 10.1038/s41467-022-29584-y
Sork, V. L., Fitz-Gibbon, S. T., Puiu, D., Crepeau, M., Gugger, P. F., Sherman, R., et al. (2016). First draft assembly and annotation of the genome of a California endemic oak quercus lobata née (Fagaceae). G3 Genes|Genomes|Genetics 6 (11), 3485–3495. doi: 10.1534/g3.116.030411
Stamatakis, A. (2014). RAxML version 8: a tool for phylogenetic analysis and post-analysis of large phylogenies. Bioinformatics 30 (9), 1312–1313. doi: 10.1093/bioinformatics/btu033
Stanke, M., Steinkamp, R., Waack, S., Morgenstern, B. (2004). AUGUSTUS: a web server for gene finding in eukaryotes. Nucleic Acids Res. 32 (suppl_2), W309–W312. doi: 10.1093/nar/gkh379
Sugiura, N., Kurokochi, H., Tan, E., Asakawa, S., Sato, N., Saito, Y., et al. (2014). Development of 13 polymorphic chloroplast DNA markers in quercus gilva, a regionally endemic species in Japan. Conserv. Genet. Resour. 6 (4), 961–965. doi: 10.1007/s12686-014-0256-y
Sugiura, N., Tang, D., Kurokochi, H., Saito, Y., Ide, Y. (2015). Genetic structure of quercus gilva blume in Japan as revealed by chloroplast DNA sequences. Botany 93 (12), 873–880. doi: 10.1139/cjb-2015-0025
Talavera, G., Castresana, J. (2007). Improvement of phylogenies after removing divergent and ambiguously aligned blocks from protein sequence alignments. Systematic Biol. 56 (4), 564–577. doi: 10.1080/10635150701472164
Tang, H., Bowers John, E., Wang, X., Ming, R., Alam, M., Paterson Andrew, H. (2008). Synteny and collinearity in plant genomes. Science 320 (5875), 486–488. doi: 10.1126/science.1153917
Tarailo-Graovac, M., Chen, N. (2009). Using RepeatMasker to identify repetitive elements in genomic sequences. Curr. Protoc. Bioinf. 25 (1), 4.10.11–14.10.14. doi: 10.1002/0471250953.bi0410s25
Tatusov, R. L., Fedorova, N. D., Jackson, J. D., Jacobs, A. R., Kiryutin, B., Koonin, E. V., et al. (2003). The COG database: an updated version includes eukaryotes. BMC Bioinf. 4 (1), 41. doi: 10.1186/1471-2105-4-41
Thiel, T., Michalek, W., Varshney, R., Graner, A. (2003). Exploiting EST databases for the development and characterization of gene-derived SSR-markers in barley (Hordeum vulgare l.). Theor. Appl. Genet. 106 (3), 411–422. doi: 10.1007/s00122-002-1031-0
Tian, D., Traw, M. B., Chen, J. Q., Kreitman, M., Bergelson, J. (2003). Fitness costs of r-gene-mediated resistance in arabidopsis thaliana. Nature 423 (6935), 74–77. doi: 10.1038/nature01588
Wang, D., Zhang, Y., Zhang, Z., Zhu, J., Yu, J. (2010). KaKs_Calculator 2.0: A toolkit incorporating gamma-series methods and sliding window strategies. Genomics Proteomics Bioinf. 8 (1), 77–80. doi: 10.1016/S1672-0229(10)60008-3
Xu, H., Luo, X., Qian, J., Pang, X., Song, J., Qian, G., et al. (2012). FastUniq: a fast de novo duplicates removal tool for paired short reads. PloS One 7 (12), e52249. doi: 10.1371/journal.pone.0052249
Yang, Z. (2007). PAML 4: Phylogenetic analysis by maximum likelihood. Mol. Biol. Evol. 24 (8), 1586–1591. doi: 10.1093/molbev/msm088
Zeng, Q. M., Liu, B., Lin, R. Q., Jiang, Y. T., Liu, Z. J., Chen, S. P. (2019). The complete chloroplast genome sequence of quercus gilvaFagaceae. Mitochondrial DNA B Resour 4 (2), 2493–2494. doi: 10.1080/23802359.2019.1637299
Zhang, Z., Xiao, J., Wu, J., Zhang, H., Liu, G., Wang, X., et al. (2012). ParaAT: A parallel tool for constructing multiple protein-coding DNA alignments. Biochem. Biophys. Res. Commun. 419 (4), 779–781. doi: 10.1016/j.bbrc.2012.02.101
Keywords: Quercus gilva, PacBio sequencing, chromosome-scale genome assembly, phylogeny, evolution
Citation: Zhou X, Liu N, Jiang X, Qin Z, Farooq TH, Cao F and Li H (2022) A chromosome-scale genome assembly of Quercus gilva: Insights into the evolution of Quercus section Cyclobalanopsis (Fagaceae). Front. Plant Sci. 13:1012277. doi: 10.3389/fpls.2022.1012277
Received: 05 August 2022; Accepted: 13 September 2022;
Published: 23 September 2022.
Edited by:
Yunpeng Cao, Chinese Academy of Sciences (CAS), ChinaCopyright © 2022 Zhou, Liu, Jiang, Qin, Farooq, Cao and Li. This is an open-access article distributed under the terms of the Creative Commons Attribution License (CC BY). The use, distribution or reproduction in other forums is permitted, provided the original author(s) and the copyright owner(s) are credited and that the original publication in this journal is cited, in accordance with accepted academic practice. No use, distribution or reproduction is permitted which does not comply with these terms.
*Correspondence: He Li, bGloZTkwQGNzdWZ0LmVkdS5jbg==
Disclaimer: All claims expressed in this article are solely those of the authors and do not necessarily represent those of their affiliated organizations, or those of the publisher, the editors and the reviewers. Any product that may be evaluated in this article or claim that may be made by its manufacturer is not guaranteed or endorsed by the publisher.
Research integrity at Frontiers
Learn more about the work of our research integrity team to safeguard the quality of each article we publish.