- 1State Key Laboratory of Hybrid Rice, College of Life Sciences, Wuhan University, Wuhan, China
- 2School of Landscape and Ecological Engineering, Hebei University of Engineering, Handan, China
Osmotic stress severely affects plant growth and development, resulting in massive loss of crop quality and quantity worldwide. The 70-kDa heat shock proteins (HSP70s) are highly conserved molecular chaperones that play essential roles in cellular processes including abiotic stress responses. However, whether and how plastid-targeted heat shock cognate 70 kDa protein (cpHSC70-1) participates in plant osmotic stress response remain elusive. Here, we report that the expression of cpHSC70-1 is significantly induced upon osmotic stress treatment. Phenotypic analyses reveal that the plants with cpHSC70-1 deficiency are sensitive to osmotic stress and the plants overexpressing cpHSC70-1 exhibit enhanced tolerance to osmotic stress. Consistently, the expression of the stress-responsive genes is lower in cphsc70-1 mutant but higher in 35S:: cpHSC70-1 lines than that in wild-type plants when challenged with osmotic stress. Further, the cphsc70-1 plants have less APX and SOD activity, and thus more ROS accumulation than the wild type when treated with mannitol, but the opposite is observed in the overexpression lines. Overall, our data reveal that cpHSC70-1 is induced and functions positively in plant response to osmotic stress by promoting the expression of the stress-responsive genes and reducing ROS accumulation.
Introduction
Environmental stresses such as drought, cold, and salinity can alter water availability by changes in solute concentrations (i.e., inorganic cations, sugars, anions, and salts) and impose osmotic stress on plants, which affects cell membrane integrity, photosynthetic capacity, and osmotic regulation, leading to severe restrictions on plant growth and development (Zhao et al., 2020). Osmotic stress reduces the water uptake of plants, bringing about not only ionic stress but also oxidative damages caused by overaccumulated reactive oxygen species (ROS) including hydroperoxide (H2O2), superoxide anion , and hydroxyl radicals (•OH) (Wenjing et al., 2020; Zhao et al., 2020; Wang et al., 2021b; Zhang et al., 2021). These ROS can affect proteins, lipids, and nucleic acids if accumulated over a certain threshold, leading to cell damage and death (Yuan et al., 2017; Nadarajah, 2020). To overcome this issue, plants have employed complex antioxidant defense mechanisms to protect plants from osmotic stress-induced oxidative damage by scavenging ROS and maintaining the balance of ROS production (Hasanuzzaman et al., 2021).
The antioxidant defense system includes a series of enzymes such as catalase (CAT), superoxide dismutase (SOD), ascorbate peroxidase (APX), and non-enzymatic antioxidants (α-tocopherol, glutathione, β-carotene, ascorbate). They work in coordination to maintain ROS homeostasis by scavenging stress-induced excess ROS in plant cells (Waszczak et al., 2018; Sánchez-McSweeney et al., 2021). For instance, SOD can disproportionate O2- to H2O2, and then H2O2 is further detoxified into H2O by APX with the assistance of ascorbate in the chloroplast (Uzilday et al., 2014) and thus overexpressing SOD and APX can improve the plant’s tolerance under salt stress (Shafi et al., 2019). CATs, the enzymes directly degrading H2O2 without assistance of any reducing equivalent, are also necessary for scavenging ROS in plants under various stressed conditions (Hasanuzzaman et al., 2020). It is reported that the plants with CAT3 mutation accumulate higher H2O2 and are hypersensitive to water deprivation, but the plants overexpressing CAT3 have less H2O2 and are more tolerant to drought stress compared with the wild type (Zou et al., 2015). Similarly, the disruption of CAT2 causes excess H2O2 accumulation and reduces plant tolerance to high salinity (Bueso et al., 2007). Further, several factors have been indicated to regulate antioxidant enzymes in the stressed plants. For example, the zinc finger protein Zat12 involved in plant response to oxidative stress by promoting APX1 expression and Zat12-deficient plants are more sensitive to H2O2 application than wild-type plants (Rizhsky et al., 2004). Overexpression of AtbHLH112 increases salt and drought tolerance by promoting SOD activity to improve ROS scavenging ability (Liu et al., 2015). A recent report documented that leucine aminopeptidase 2 (LAP2), as a collaborator of CAT2, confers Arabidopsis plants increased osmotic stress tolerance possibly through maintaining CAT2 protein stability (Zhang et al., 2021). In addition, peroxisome-localized small heat shock protein Hsp17.6CII activates catalase by interacting with CAT2 and thus confers alkaline and salt stress tolerance in plants (Li et al., 2017). MeHSP90.9-silenced plants have repressed CAT1 expression, reduced CAT activity, and higher H2O2, resulting in more sensitivity to drought stress (Wei et al., 2020). These reports reveal that modulations of antioxidant enzymes play a vital role in regulating plant abiotic stress tolerance.
Heat shock proteins (HSPs) are a diverse group of multifamily proteins, functioning in adverse stimuli by preventing protein misfolding, reducing the aggregation of denatured proteins, and maintaining protein structural stability (Sable et al., 2018; Qi et al., 2019). Based on their apparent molecular weight, amino acid sequence homology, and functions, HSPs have been classified into HSP100s, HSP90s, HSP70s, HSP60s, and small heat shock protein (sHSP) families (Mayer and Bukau, 2005). Of all HSPs, HSP70 superfamily members are the most abundant, highly conserved, and well-characterized group of molecular chaperones in all organisms from prokaryotes to eukaryotes (Xu et al., 2020; Li et al., 2021). Structurally, HSP70s are identified by three distinct domains: a 45-kDa N-terminal ATPase, a 15-kDa β-sandwich domain, and a 10-kDa C-terminal α-helical domain (Zhu et al., 1996; Tang et al., 2016). Functionally, while HSP70s assist cellular machinery in regulating protein degradation and verifying proteins quality under normal conditions (Bukau et al., 2006; Su and Li, 2008; Hartl et al., 2011), they facilitate denatured protein refold, prevent denatured proteins from aggregating, and dissolve or degrade protein aggregates during stress (Wang et al., 2004; Lee and Tsai, 2005). In Arabidopsis, 18 HSP70s have been identified, and they are divided into four subclasses based on their subcellular localization: cytosol/nucleus, mitochondria, endoplasmic reticulum (ER), and plastids (Wu et al., 1994; Lin et al., 2001). The cytosolic/nuclear HSP70s mainly function in plant development, signaling pathways, abiotic stresses including drought, salinity, and high temperature, and biotic stresses such as virus infection (Jungkunz et al., 2011; Leng et al., 2017). The mitochondria-localized mtHSC70-1 and mtHSC70-2 are required for the mitochondrial Fe–S cluster assembly and aid in the translocation of precursor proteins to mitochondria as part of the translocon (Zhang and Glaser, 2002; Leaden et al., 2014). Early reports showed that overexpression of the rice (Oryza sativa) mtHSC70 inhibited heat- and H2O2-induced cell death in protoplasts through reducing reactive oxygen species (ROS) generation and sustaining mitochondrial membrane potential (Qi et al., 2011). A recent study showed that mtHSC70-1 mutation resulted in severe embryo defects (Li et al., 2021). As ER-localized HSP70s, immunoglobulin-binding proteins (BiPs) play an important role in male and female gametophyte development and unfolded protein responses (Srivastava et al., 2013; Maruyama et al., 2014). For plastid-localized HSP70s in Arabidopsis, two cpHSC70s (cpHSC70-1 and cpHSC70-2) are identified to be essential for maintaining chloroplast structure and functions (Sung et al., 2001; Latijnhouwers et al., 2010). While cphsc70-1 mutant plants exhibit variegated cotyledons, slow growth, deformed leaves, and impaired root growth under normal growth conditions, the stressed cphsc70-1 mutant plants are more sensitive to high temperature and drought stress (Su and Li, 2008; Latijnhouwers et al., 2010). However, whether and how cpHSC70-1 participates in plant response to osmotic stress remains unknown.
In this study, we report that cpHSC70-1 plays important roles in plant tolerance to osmotic stress. When challenged with osmotic stress, the expression of cpHSC70-1 is promoted in plants. Moreover, the knockout of cpHSC70-1 has enhanced sensitivity to osmotic stress with lower APX and SOD activities and increased ROS accumulation. Overexpression of cpHSC70-1 in wild type improves tolerance of transgenic Arabidopsis to osmotic stress, with a higher expression of genes encoding antioxidant enzymes and decreased ROS accumulation. Taken together, these results show that osmotic stress-induced cpHSC70-1 functions necessarily in the stress tolerance by modulating ROS scavenging capacity in Arabidopsis.
Materials and methods
Plant materials and growth conditions
The Columbia-0 (Col-0) Arabidopsis thaliana ecotype was employed in the present study. The mutants cphsc70-1 (Salk_140810) and cphsc70-2 (Salk_095715) were previously reported (Su and Li, 2008). Arabidopsis seeds were surface sterilized with 5% (w/v) bleach for 5 min, rinsed three times with sterile water, stored to 4°C for 3 days, and then grown on 1/2 strength MS (Murashige and Skoog) medium (pH 5.8) containing 1% (w/v) agar and 1% (w/v) sucrose, and plants were grown at 23°C with 16-h light (100 μmol m–2 s–1 illumination)/8-h dark conditions. For osmotic stress treatment, the corresponding seedlings were planted on 1/2 MS medium supplemented with or without 300 mM mannitol (Beijing Dingguo Changsheng Biotechnology Co., Ltd., DH190-2) for 5 days, and then the fresh weight and root length were determined and analyzed.
Plasmid construction and plant transformation
The full-length coding sequences (CDS) of cpHSC70-1 (AT4G24280), cpHSC70-2 (AT5G49910), and the promoter (2 kb) of cpHSC70-1 were amplified using PCR. The resulting fragments (cpHSC70-1 and cpHSC70-2) were cloned into the pCAMBIA1300S vector and verified by sequencing. cpHSC70-1 was also cloned into pCAMBIA1300 driven by the cpHSC70-1 promoter. The resultant plasmids were transformed into Col-0 or the homozygous cphsc70-1 mutant using Agrobacterium tumefaciens strain pGV3101 and the floral dip method. The primer sequences are listed in Supplemental Table 1.
RNA extraction and quantitative reverse transcription PCR
The RNA was extracted as previously described (Ding et al., 2022). Briefly, plants were thoroughly ground with liquid nitrogen, and TRIzol reagent (Invitrogen) was used to extract the total RNA by following the manufacturer’s instructions, and RQ1 RNase-free DNase I (Promega) was employed to remove the contaminated DNA. RNA reverse transcription was performed with ReverTra Ace kit (TOYOBO) according to the manufacturer’s instructions. qPCR was performed using a Bio-Rad CFX96 and SYBR Green I dye (Invitrogen) with the program of 95°C for 3 min, 35 cycles of 95°C for 10 s, and 60°C for 45 s, followed by 5 min incubation at 95°C. ACTIN2 (AT3G18780) was used as the reference gene, and all experiments included three independent biological replicates and three technical repetitions. Primer sequences are shown in Supplemental Table 1.
Seed germination and cotyledon expansion statistics
Germination was determined in the same way as before (Ding et al., 2022). Briefly, the seeds were planted on 1/2 MS medium with or without 300 mM mannitol. The radicle’s appearance served as a gauge for seed germination. The percentages of germinated seeds were counted at the specified times. For each seed germination experiment, at least 60 seeds of each genotype were used, and experiments were conducted three times. On the fifth day, the percentages of cotyledon expansion per plant was scored.
3,3-Diaminobenzidine and nitroblue tetrazolium staining
As detailed earlier (Yu et al., 2019; Luo et al., 2021), the 7-day-old seedlings grown on 1/2 MS medium were transferred to mannitol (300 mM) containing 1/2 MS medium for 48 h, then the seedlings were stained with 3,3-diaminobenzidine (DAB) or nitroblue tetrazolium (NBT) to determine H2O2 or superoxide anion accumulation. For DAB staining, seedlings were incubated for 8 h in freshly prepared DAB staining solution [1 mg/ml DAB (Beijing Dingguo Changsheng Biotechnology Co., Ltd., JD091) which was dissolved in 10 mM Na2HPO4 supplemented with 0.1% (v/v) Tween-20] and then washed with 70% ethanol to remove chlorophyll. The leaves were analyzed and photographed using a Nikon microscope (SMZ25; Nikon). The relative levels of DAB staining were quantitatively analyzed by using Photoshop CS6 software (Adobe). For superoxide anion labeling, seedlings were vacuum infiltrated with 0.1 mg/ml NBT (Sigma-Aldrich, N6876) in 25 mM HEPES buffer (pH 7.6) for 2 h in the dark. Seventy percent ethanol was used to remove chlorophyll from the leaves, then these leaves were imaged using a differential interference contrast (DIC) optical system (BX64; Olympus) and a charge-coupled device (CCD) camera (DP72; Olympus).
Detection of H2O2 and contents
The POD-coupled assay was utilized to quantify the H2O2 levels as previously reported (Yuan et al., 2017). First, 2 ml of HClO4 (1 M) containing insoluble polyvinylpyrrolidone (5%) was used to extract 0.2 g of the Arabidopsis seedlings. The homogenate was centrifuged at 12,000 g for 10 min, and the supernatant was neutralized to pH 5.6 with 5 M K2CO3 to pH 5.6 in the presence of 100 μl of 0.3 M phosphate buffer, pH 5.6. The solution was centrifuged for 1 min at 12,000 g, and the supernatant was then mixed with 1 unit of ascorbate oxidase and left to stand for 10 min at 25°C. 3-(Dimethylamino)benzoic acid (3.3 mM), 0.07 mM 3-methyl-2-benzothiazoline hydrazone, and 0.1 M phosphate buffer (pH 6.5) were added to 500 μl of the reaction mixture to start the final reaction. After standing for 30 min at 25°C, the 590-nm absorbance change in the solution was determined. A superoxide anion content detection kit (Beijing Solarbio Science and Technology Co., Ltd., BC1290) was used to measure the contents according to the provided instructions.
Determination of catalase, ascorbate peroxidase, and superoxide dismutase activities
Total CAT, APX, and SOD activities were analyzed using a Catalase Assay Kit (Beyotime Biotechnology), Ascorbate Peroxidase Assay Kit (Gelatins), and a Total Superoxide Dismutase Assay Kit with NBT (Beyotime Biotechnology), respectively, following supplied protocols. The CAT activity was assayed based on decreases in H2O2 accumulation. The APX activity was measured by calculating the AsA oxidation rate. The SOD activity was detected by NBT photoreduction inhibition.
Western blot analysis
Total proteins were extracted using the plant protein extracting buffer containing 375 mM NaCl, 2.5 mM EDTA, 1% β-mercaptoethanol, 125 mM Tris–HCl (pH8.0), and 1% SDS. Protein concentrations were assayed using a bicinchoninic acid assay (BCA) protein assay kit (Beijing Dingguo Changsheng Biotechnology Co., Ltd.). Afterward, the proteins were separated by electrophoresis using 12% SDS-PAGE gel. Immunoblotting was performed on PVDF membranes with anti-HSC70 (Agrisera, AS08348) and anti-ACTIN (Abmart, M20009M). The intensity of each immunodetection band was determined using an image-processing and analysis software package (ImageJ, version 1.52v). Relative protein levels were normalized against those in control, which were set to 1.
Results
Osmotic stress promotes the expression of cpHSC70-1
HSP70s play key roles in ensuring cellular homeostasis, whether cells are in normal or stressful environments (Leng et al., 2017). Although their family members, cpHSC70s, have been reported to be involved in some biotic stresses such as heat and drought (Su and Li, 2008; Latijnhouwers et al., 2010), the function of cpHSC70s in osmotic stress remains unknown. To investigate whether cpHSC70s are involved in plants osmotic stress response, we examined whether mannitol treatment affects the expression of cpHSC70-1 and cpHSC70-2. Our reverse transcription PCR (RT-qPCR) analyses showed that osmotic stress significantly induced the expression of cpHSC70-1, but not cpHSC70-2 (Figure 1A). Moreover, we measured cpHSC70 proteins in mannitol-treated wild-type plants; our Western blot analyses indicated that the mannitol-treated wild-type seedlings had higher cpHSC70 accumulation than untreated control (Figure 1B). Thus, our data suggest that osmotic stress promotes cpHSC70-1 expression in the stressed plant.
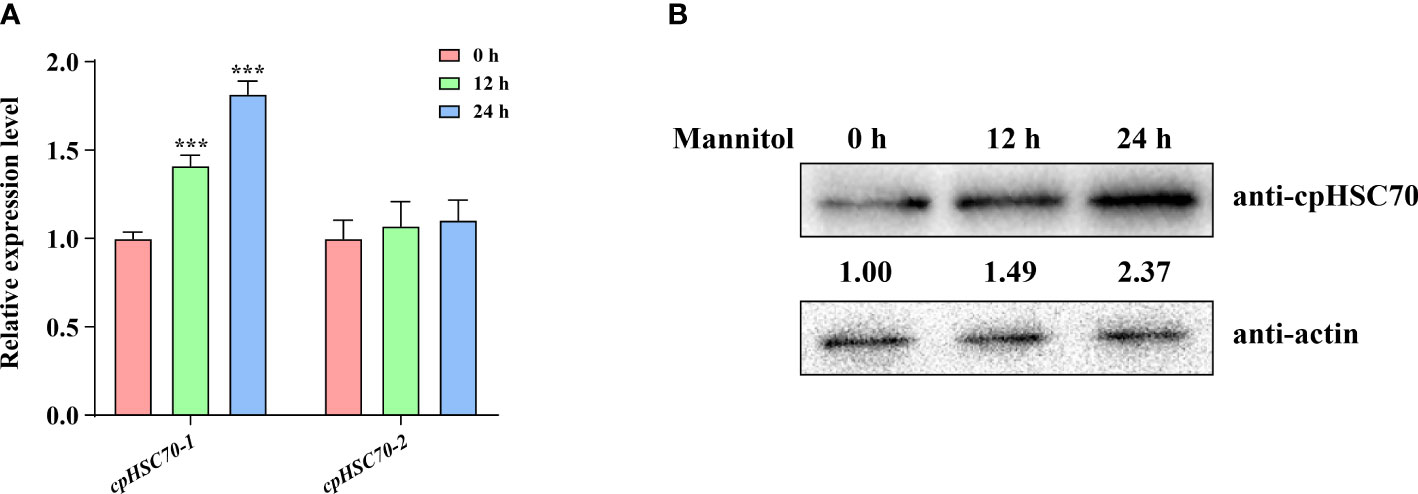
Figure 1 Osmotic stress induces cpHSC70-1 expression. (A) The cpHSC70-1 and cpHSC70-2 expression levels of 5-day-old wild-type seedlings subjected to osmotic stress (300 mM mannitol for 6, 12 h) and its control. The data are means ( ± SEM) from at least three independent experiments. Asterisks indicate significant differences revealed using a Student’s t-test (***p < 0.001). (B) cpHSC70 protein level of 5-day-old wild-type seedlings treated with 300 mM mannitol for 0, 12, and 24 h. The intensity of each immunodetection band was measured by using an image-processing and analysis software package (ImageJ). The protein levels of 0 h were set to 1. Actin was used as control.
The cpHSC70-1 functions positively in plant response to osmotic stress
The induction of cpHSC70-1 expression by osmotic stress as shown in our above results implies a possible role of this gene in plant response to osmotic stress. To verify this possibility, we obtained the mutant cphsc70-1 (SALK_140810), in which T-DNA was inserted in the second intron of cpHSC70-1 and cpHSC70-1 expression was significantly reduced in the mutant (Supplementary Figures 1A, B) and generated the complementation lines (cpHSC70-1::cpHSC70-1 cphsc70-1) by expressing the full-length coding sequence of cpHSC70-1 driven under its native promoter in cphsc70-1 mutant (Supplementary Figure 1B). Then, we examined the phenotypes of the mutant and its complementation lines under osmotic stress. We found that when grown under normal conditions, cphsc70-1 exhibited variegated cotyledons, malformed leaves, small size, and impaired root growth compared with the wild type as previously reported (Su and Li, 2008; Chu et al., 2020), but cphsc70-1 plants showed a similar germination rate and cotyledon expansion rate as the wild type had (Figures 2A-C). When challenged with osmotic stress, the cphsc70-1 seedlings had a reduced germination rate and cotyledon expansion rate than wild-type seedlings (Figures 2B, C). In addition, the fresh weight and primary root elongation were further repressed by osmotic stress in the mutant compared with the wild type (Figures 2D, H). Regarding the cpHSC70-1::cpHSC70-1 cphsc70-1 plants, they behaved as the wild type under either normal or stressed conditions (Figures 2A-H). Taken together, our results indicate that cpHSC70-1 functions positively in plant response to osmotic stress.
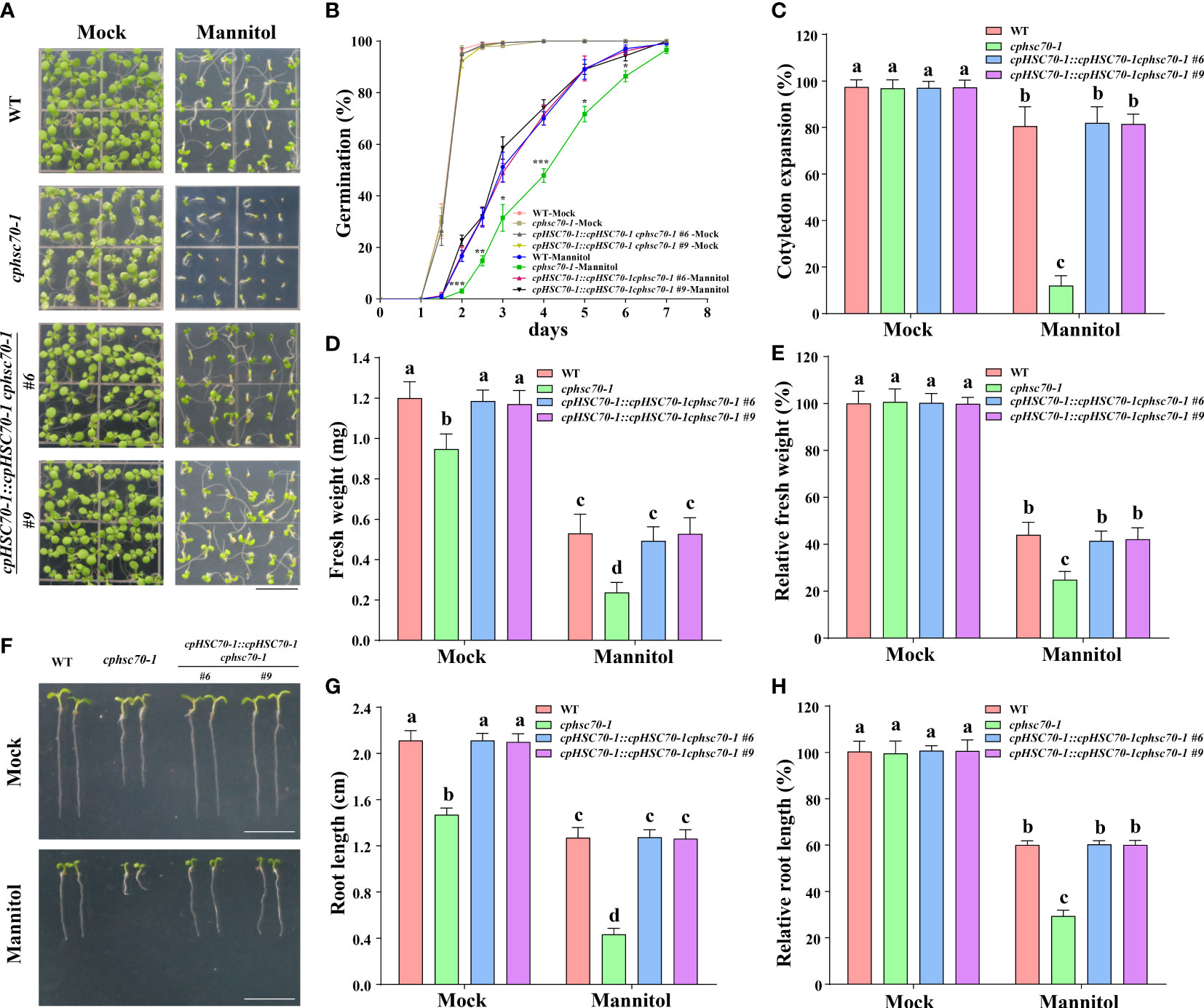
Figure 2 Loss-of-function mutation of cpHSC70-1 increases plant sensitivity to osmotic stress. (A) Images of 5-day-old wild-type, cphsc70-1, and cpHSC70-1::cpHSC70-1 cphsc70-1 seedlings grown on 1/2 MS medium with or without (Mock) 300 mM mannitol. Bars = 1 cm. (B) Germination rate of the wild-type, cphsc70-1, and cpHSC70-1::cpHSC70-1 cphsc70-1 plants in response to 300 mM mannitol. (C) Rate of cotyledon expansion, (D) fresh weight, and (E) relative fresh weight of the wild-type, cphsc70-1, and cpHSC70-1::cpHSC70-1 cphsc70-1 plants in panel (A). (F) Images of 5-day-old wild-type, cphsc70-1, and cpHSC70-1::cpHSC70-1 cphsc70-1 seedlings grown on 1/2 MS medium with or without (Mock) 300 mM mannitol. Bars = 1 cm. (G) Root length and (H) relative root length of the plants shown in (F). The data are means ( ± SD) from at least three independent experiments (n ≥60 for germination rate and n ≥30 for root length). Asterisks indicate significant differences revealed using a Student’s t-test (*p < 0.05; **p < 0.01; ***p< 0.001). Different letters indicate significant differences as determined using ANOVA followed by Tukey’s test (P < 0.05).
Overexpression of cpHSC70-1 enhances plant tolerance to osmotic stress
Further, we tested whether overexpression of this gene can confer plants more tolerance to osmotic stress. For this end, we generated transgenic lines 35S::cpHSC70-1 by overexpressing cpHSC70-1 under the control of the 35S promoter in the Col-0 background Arabidopsis. RT-qPCR results showed that the cpHSC70-1 expression in the overexpression lines was higher than that in the wild type (Supplementary Figure 1B). While 35S::cpHSC70-1 plants showed no aberrant phenotype compared to the wild type under normal growth conditions (Figure 3), these seedlings had a higher germination rate, promoted the cotyledon expansion rate, and increased fresh weight and longer primary root length than the wild-type seedlings (Figures 3A-F). These findings reveal that overexpression of cpHSC70-1 enhances osmotic stress tolerance in the transgenic plants.
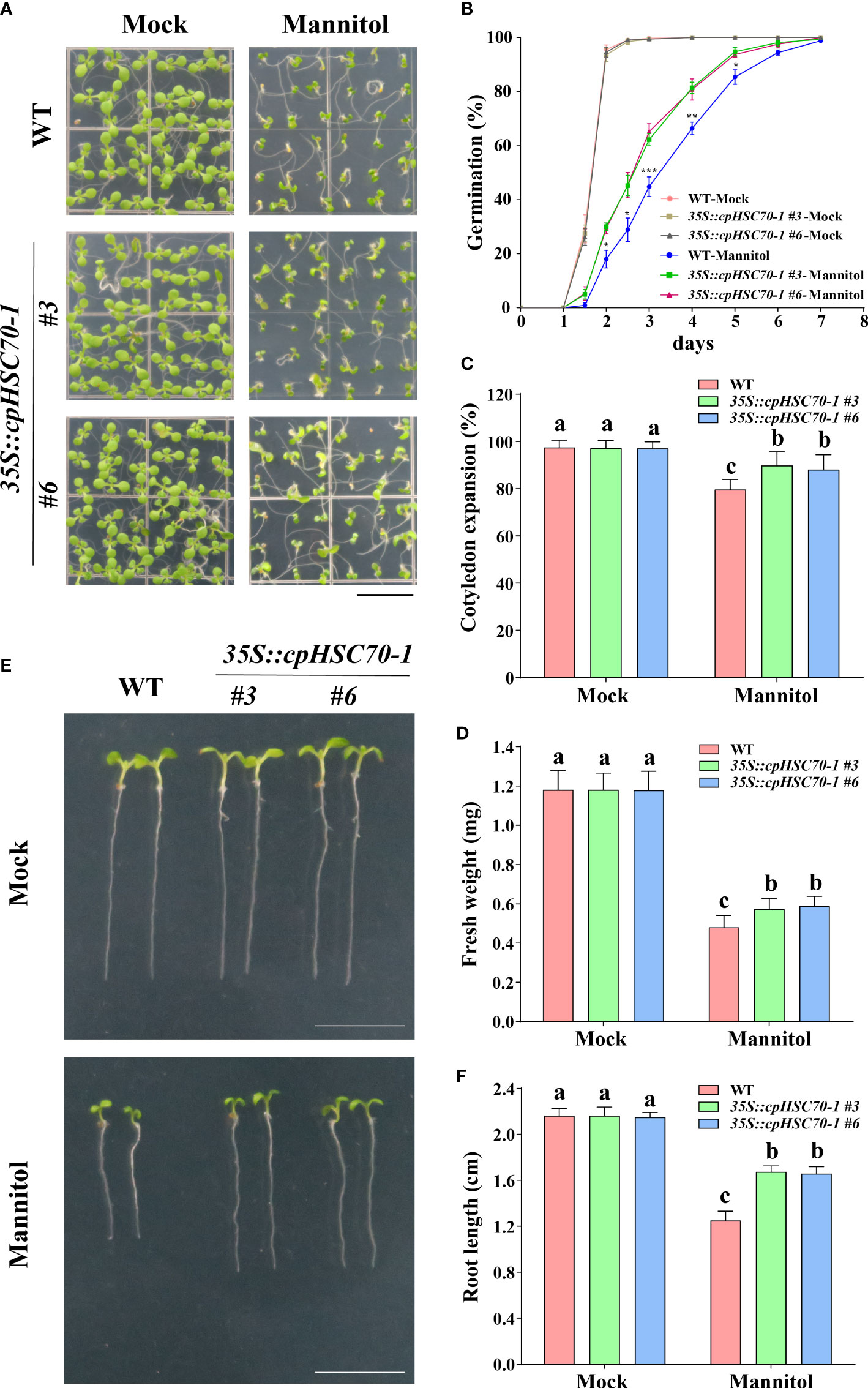
Figure 3 Overexpression of cpHSC70-1 enhanced osmotic stress tolerance. (A) Images of 5-day-old wild-type and 35S::cpHSC70-1 seedlings grown on 1/2 MS medium with or without (Mock) 300 mM mannitol. Bars = 1 cm. (B) Germination rate of the wild-type and 35S::cpHSC70-1 plants in response to 300 mM mannitol. (C) Rate of cotyledon expansion and (D) fresh weight of wild-type and 35S::cpHSC70-1 plants in panel (A). (E) Images of 5-day-old wild-type and 35S::cpHSC70-1 seedlings grown on 1/2 MS medium with or without (Mock) 300 mM mannitol. Bars = 1 cm. (F) Root length of the plants shown in (E). The data are means ( ± SD) from at least three independent experiments (n ≥60 for germination rate and n ≥ 30 for root length). Asterisks indicate significant differences revealed using a Student’s t-test (* p < 0.05; **p < 0.01; ***p < 0.001). Different letters indicate significant differences as determined using ANOVA followed by Tukey’s test (p < 0.05).
cpHSC70-1 regulates the expression of the genes involved in plant stress response
Previous studies reported that various abiotic stresses including high salinity and osmotic stress modulate the expression of stress-responsive genes (Wang et al., 2021a; Ding et al., 2022). To explore the impact of cpHSC70-1 on the molecular basis of osmotic stress response, we detected the transcripts of several key genes involved in osmotic stress (RD29A, KIN1, COR15A, and P5CS1) (Wang et al., 2021b). We found that the transcripts of all tested genes were similar in cphsc70-1, 35S::cpHSC70-1, cpHSC70-1::cpHSC70-1 cphsc70-1, and wild-type plants under normal conditions (Figures 4A-D). However, upon osmotic stress, phsc70-1 plants suppressed but 35S::cpHSC70-1 plants promoted the expression of these genes compared with both wild-type and cpHSC70-1::cpHSC70-1 cphsc70-1 plants (Figures 4A-D). These results suggest that the expression of the stress-responsive genes could be involved in cpHSC70-1-mediated plant osmotic stress response.
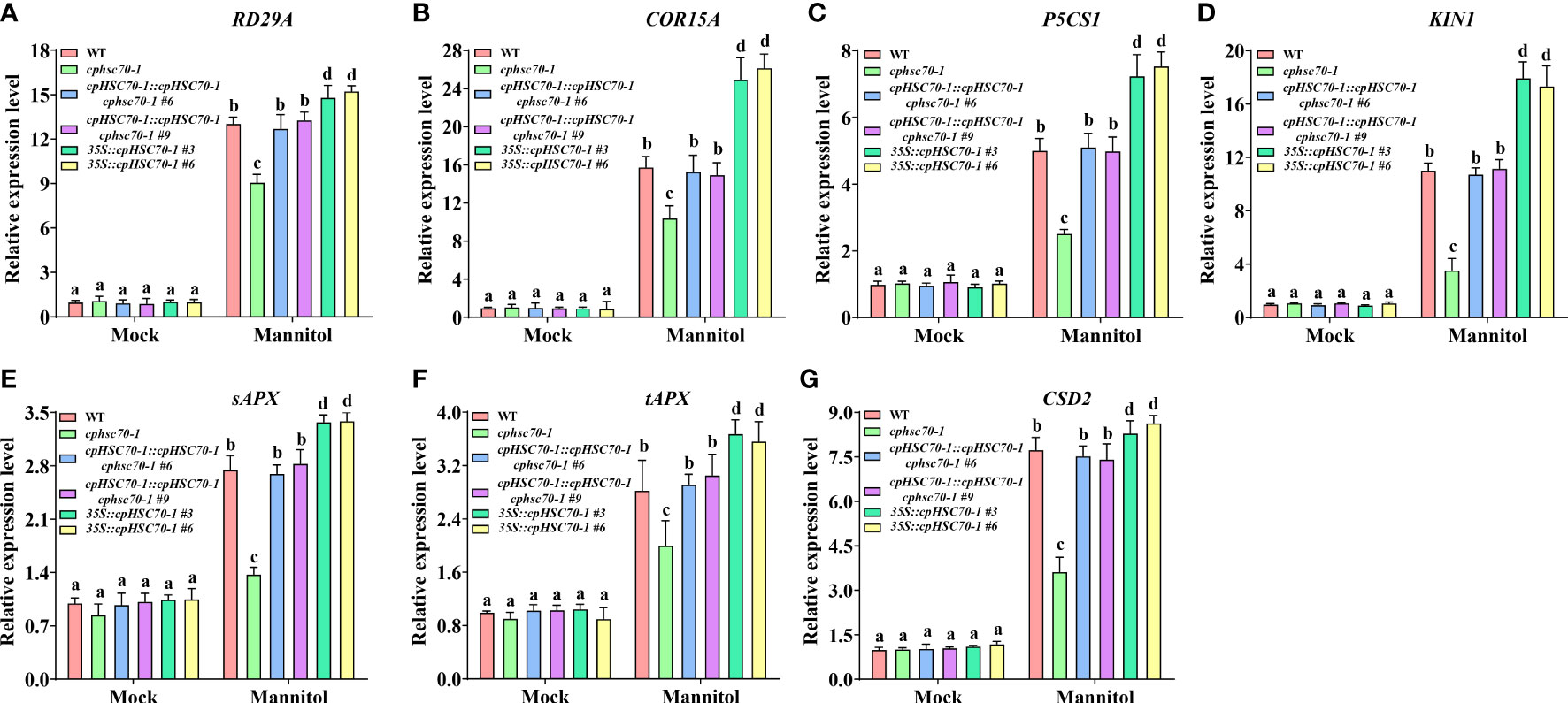
Figure 4 Effects of cpHSC70-1 on expression of genes involved in stress response and ROS-scavenging system. (A–G) The expression levels of (A) RD29A, (B) COR15A, (C) P5CS1, (D) KIN1, (E) sAPX, (F) tAPX, and (G) CSD2 of the 5-day-old wild-type, cphsc70-1, cpHSC70-1::cpHSC70-1 cphsc70-1, and 35S::cpHSC70-1 plants treated with or without (Mock) 300 mM mannitol for 12 h. The expression of these genes was determined by RT-qPCR and correlated with that of the WT, the value of which was set as 1. ACTIN2 was used as the reference gene. The data are means ( ± SEM) from at least three independent experiments. Different letters indicate significant differences as determined using ANOVA followed by Tukey’s test (p < 0.05).
The cpHSC70-1 regulates ROS homeostasis of plants under osmotic stress
It is reported that ROS is involved in plant response to osmotic stress (Foyer and Noctor, 2005); (Koussevitzky et al., 2008; Vishwakarma et al., 2015; Li et al., 2019; Wang et al., 2021a). Thus, we also tested the expression of genes involved in the ROS-scavenging system (CAT1, CAT2, CAT3; APX1, sAPX, tAPX; CSD1, CSD2, CSD3) in cphsc70-1 and 35S::cpHSC70-1 plants under osmotic stress. Our results showed that while cphsc70-1, 35S::cpHSC70-1, cpHSC70-1::cpHSC70-1 cphsc70-1, and wild-type plants had similar expressions of CAT1, CAT2, CAT3, APX1, CSD1, and CSD3, the cphsc70-1 mutant reduced but cpHSC70-1 overexpression lines increased the expression of sAPX, tAPX, and CSD2 compared with cpHSC70-1::cpHSC70-1 cphsc70-1 and wild-type plants when subjected with mannitol treatment (Figures 4E-G; Supplementary Figures 2A-F). Consistently, all tested plants had similar CAT activity when grown under both normal and stressful conditions; however, osmotic stress-promoted activities of APX and SOD in both cpHSC70-1::cpHSC70-1 cphsc70-1 and wild-type plants were repressed in cphsc70-1 mutant but enhanced in 35S::cpHSC70-1 plants (Figures 5A-C; Supplementary Figures 3A-C). These results suggest the possible involvement of ROS homeostasis in cpHSC70-1-mediated plant osmotic stress response. Therefore, we examined the contents of H2O2 in plant response to osmotic stress by using both 3,3-diaminobenzidine (DAB) staining experiments and the POD-coupled assay. Our results showed that while the stress elevated H2O2 levels in the stressed cphsc70-1, 35S::cpHSC70-1, cpHSC70-1::cpHSC70-1 cphsc70-1 and wild-type plants compared with their untreated control, respectively, H2O2 accumulation was higher in cphsc70-1 but lower in 35S::cpHSC70-1 than that in cpHSC70-1::cpHSC70-1 cphsc70-1 and the wild type upon osmotic stress treatment (Figures 5D, E; Supplementary Figures 3D, E). Similar results were found when we assayed the abundance of the superoxide anion using NBT staining and spectrophotometry (Figures 5F, G; Supplementary Figures 3F, G). These findings indicate that cpHSC70-1 regulates plant ROS homeostasis under osmotic stress.
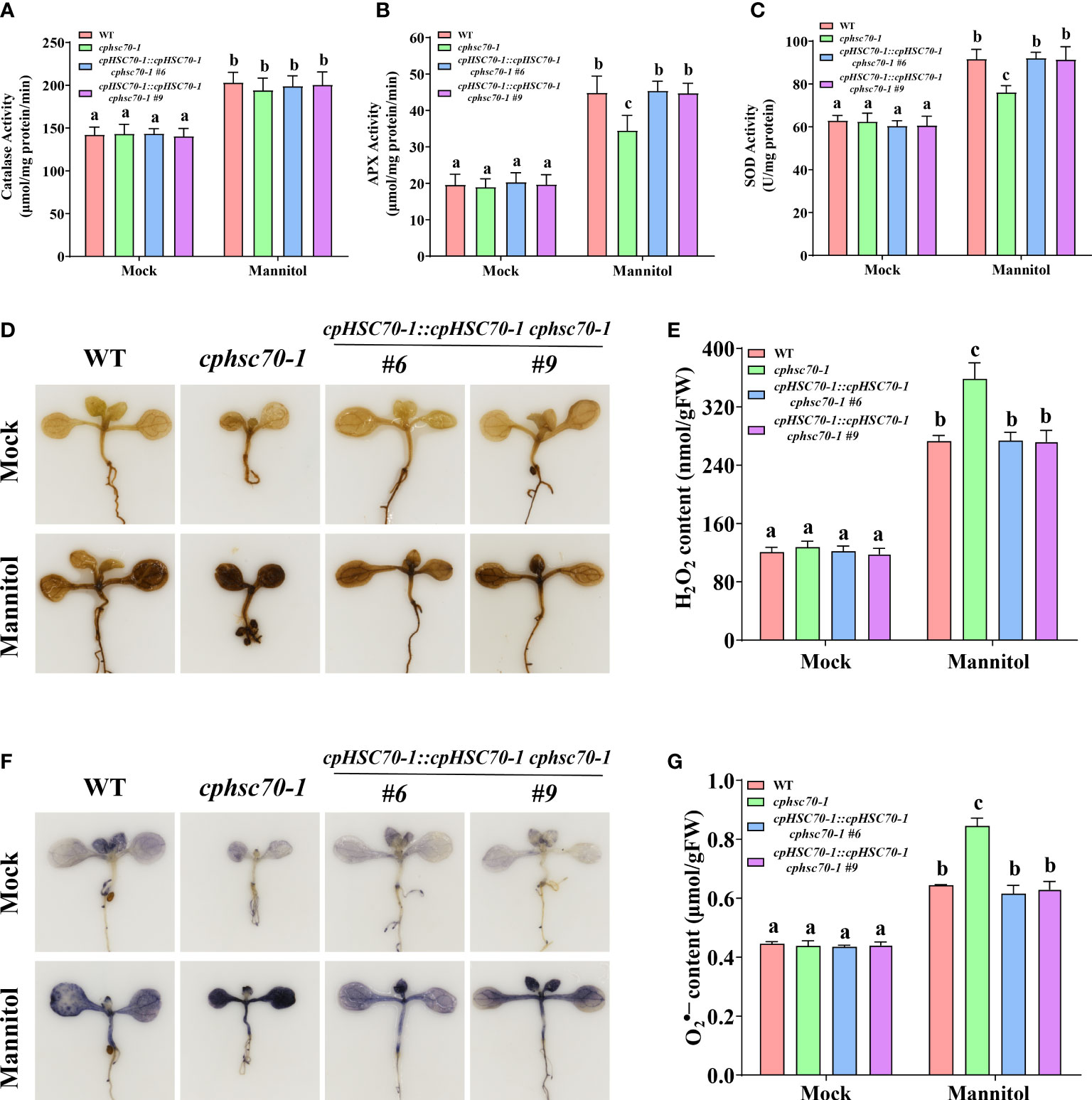
Figure 5 The cphsc70-1 mutant has higher ROS accumulation and reduced APX and SOD activity. (A) Catalase activity, (B) APX activity, and (C) SOD activity of the 5-day-old wild-type, cphsc70-1, and cpHSC70-1::cpHSC70-1 cphsc70-1 plants treated with or without (Mock) 300 mM mannitol for 2 days. (D) The DAB-staining images of leaves from the 5-day-old wild-type, cphsc70-1, and cpHSC70-1::cpHSC70-1 cphsc70-1 plants treated with or without (Mock) 300 mM mannitol for 2 days. (E) Measurements of H2O2 in the 5-day-old wild-type, cphsc70-1, and cpHSC70-1::cpHSC70-1 cphsc70-1 plants treated with or without (Mock) 300 mM mannitol for 2 days. (F) The NBT-staining images of leaves from the 5-day-old wild-type, cphsc70-1, and cpHSC70-1::cpHSC70-1 cphsc70-1 plants treated with or without (Mock) 300 mM mannitol for 2 days. (G) Measurements of in the 5-day-old wild-type, cphsc70-1, and cpHSC70-1::cpHSC70-1 cphsc70-1 plants treated with or without (Mock) 300 mM mannitol for 2 days. The data are means ( ± SD) from at least three independent experiments. Different letters indicate significant differences as determined using ANOVA followed by Tukey’s test (p < 0.05).
Discussion
HSP70 proteins are widespread and play key roles in organisms ranging from prokaryotes to land plants. They have been mainly reported in heat shock responses, protein folding and translocation, and prevention of protein aggregation (Miemyk, 1997). However, whether the chloroplast-located cpHSC70-1 participates in plant response to osmotic stress remains unknown. Here, we provide evidence that cpHSC70-1 is required for plant tolerance to osmotic stress because cphsc70-1 plants have higher sensitivity to osmotic stress and 35S::cpHSC70-1 plants exhibit higher osmotic stress tolerance.
It has been documented that there are two chloroplast-localized HSPs (cpHSC70-1 and cpHSC70-2) in Arabidopsis. These two proteins are structurally homologous based on their protein sequence alignment and play important roles in chloroplast development and functional integrity (Sung et al., 2001; Latijnhouwers et al., 2010). In this study, we found that osmotic stress induces the expression of cpHSC70-1, but the cpHSC70-2 expression is not affected by the stress (Figure 1). Thus, we also examined whether cpHSC70-2 is involved in plant response to osmotic stress. We obtained the mutant cphsc70-2 (SALK_095715), in which T-DNA was inserted in the second intron of cpHSC70-2 and cpHSC70-2 expression was significantly reduced in the mutant (Supplementary Figures 1C, D), and we assayed the stress response of this mutant under osmotic stress. We found that cphsc70-2 and wild-type plants had no substantial distinction in terms of germination rate, cotyledon expansion rate, fresh weight, and primary root elongation under either normal or osmotic-stressed conditions (Supplementary Figure 4). Further, the transgenic 35S::cpHSC70-2 lines, in which pHSC70-2 is overexpressed under the control of the 35S promoter (Supplementary Figure 1D), does not show higher stress tolerance compared with the wild type (Supplementary Figure 4). Thus, our data do not support the involvement of cpHSC70-2 in plant response to osmotic stress. Similar observations were also reported when the role of cpHSC70-1/2 was examined in plant response to drought and heat stresses (Su and Li, 2008; Latijnhouwers et al., 2010). These studies indicate that cpHSC70-1 acts positively in plant response to these stresses, but cpHSC70-2 is not required for plant tolerance to these stresses.
Many studies indicate that various abiotic stresses induce the expression of stress-responsive genes (Zhang et al., 2019; Fu et al., 2021; Ding et al., 2022). There have been various reports that hypersensitive mutants have increased the induction of stress-inducible genes. For example, the salt stress-induced expression of COR15A and ABCG6 is enhanced in salt stress-sensitive glyI2 mutants (Fu et al., 2021). Similarly, dpg1 mutants have higher expressions of RD29A and RD29B than the wild type under high salinity (Yi et al., 2019), and gcn20 mutants are more sensitive to salt stress with higher expressions of KIN1, KIN2, and COR15A (Ding et al., 2022). However, it is widely documented that hypersensitive mutants have a decreased induction of stress-inducible genes. For instance, cand2-1 mutants are hypersensitive to osmotic stress with decreased induction of RD29A, KIN1, P5CS1, and COR15A (Wang et al., 2021b). Also, mutation of DCD results in the inhibition of cadmium stress-induced PCR1 and PDR8 (Zhang et al., 2020). A recent report documents that expression levels of COR15A, COR47, and RD29A are significantly lower in rboh-D, rboh-F, and rboh-DF mutants than in the WT when treated with cold, and these mutants are less tolerant to cold stress (Liu et al., 2022a). In addition, salt and drought stress-induced RD29A and RD22 are significantly higher in the amiR-TSB1 mutants than in the wild type when the mutants show higher tolerances to drought and salt stress than the wild type (Liu et al., 2022b). Our experimental results indicate that osmotic stress promoted the expression of stress-responsive genes such as RD29A, COR15A, P5CS1, and KIN1 in wild-type plants which are repressed in cphsc70-1 mutant but enhanced in 35S::cpHSC70-1 plants. However, how cpHSC70-1 modulates the expression of these genes is unclear. ABA is a central phytohormone regulating plant responses to osmotic stress (Tivendale et al., 2014; Julkowska and Testerink, 2015). Many ABA-inducible genes such as RD29A, KIN1, and COR15A function in various abiotic stresses including osmotic stress (Ding et al., 2022). We speculate that cpHSC70-1 may be involved in regulating the expression of these stress-responsive genes by affecting ABA accumulation or ABA signaling, which is worthy of further exploration. Additionally, it has been documented that cpHSC70-1 can interact with GENOMES UNCOUPLED1 (GUN1), a protein that participates in multiple retrograde signaling pathways, regulating the expression of many nuclear-encoded genes (Wu et al., 2019). Thus, investigating whether GUN1 functions in cpHSC70-1-mediated plant osmotic stress response by modulating the expression of nuclear-encoded stress-responsive genes could be a future research direction.
Various environmental stresses including osmotic stress, result in oxidative damages caused by overaccumulated reactive oxygen species (ROS) (Wenjing et al., 2020; Zhao et al., 2020; Wang et al., 2021b; Zhang et al., 2021). These ROS can affect proteins, lipids, and nucleic acids if accumulated over a certain threshold, leading to cell damage and death (Yuan et al., 2017; Nadarajah, 2020). Thus, reducing stress-induced ROS overaccumulation is one of the most important and common protective mechanisms for plants under an adverse environment. Our study shows that the knockout of cpHSC70-1 has decreased APX and SOD activities and increased ROS accumulation, and overexpression of cpHSC70-1 in wild-type lines has higher ROS detoxification capacity and less ROS. It is known that a system of posttranslational protein transport into the chloroplast is absolutely essential for its functions such as photosynthesis, and cpHSC70-1 is a motor for protein import into the chloroplast and its mutation significantly reduces the efficiency of protein import (Su and Li, 2010; Li et al., 2020). In animals, HSP70 modulates SOD2 activity by promoting the import of SOD2 into the mitochondria (Zemanovic et al., 2018). We also notice that the mutation of cpHSC70-1 reduces activities of APX and SOD, but not CAT, under osmotic stress. It is possible that cpHSC70-1 may regulate the import of sAPX, tAPX, and CSD2 into the chloroplast, but not CAT1, CAT2, and CAT3, into the peroxisome, resulting in changes in the activities of APX and SOD under osmotic stress. Chloroplasts are thought to be the main source and target of ROS (Waszczak et al., 2018); thus, we speculate that when plants are subjected to osmotic stress, this impaired import of sAPX, tAPX, and CSD2 into the chloroplast in cphsc70-1 mutants, resulting in reduced activities of APX and SOD, which in turn causes ROS accumulation. Further experiments are needed to verify this hypothesis.
Data availability statement
The original contributions presented in the study are included in the article/Supplementary Material. Further inquiries can be directed to the corresponding author.
Author contributions
FD and BZ conceived and designed the project. FD and FL performed the experiments. FD and BZ analyzed the data and wrote the manuscript. All authors contributed to the article and approved the submitted version.
Acknowledgments
We thank Prof. Ying-Tang Lu (Wuhan University, China) for providing guidance and valuable advice.
Conflict of interest
The authors declare that the research was conducted in the absence of any commercial or financial relationships that could be construed as a potential conflict of interest.
Publisher’s note
All claims expressed in this article are solely those of the authors and do not necessarily represent those of their affiliated organizations, or those of the publisher, the editors and the reviewers. Any product that may be evaluated in this article, or claim that may be made by its manufacturer, is not guaranteed or endorsed by the publisher.
Supplementary material
The Supplementary Material for this article can be found online at: https://www.frontiersin.org/articles/10.3389/fpls.2022.1012145/full#supplementary-material
References
Bueso, E., Alejandro, S., Carbonell, P., Perez-Amador, M. A., Fayos, J., Bellés, J. M., et al. (2007). The lithium tolerance of the arabidopsis cat2 mutant reveals a cross-talk between oxidative stress and ethylene. Plant journal: Cell Mol. Biol. 52, 1052–1065. doi: 10.1111/j.1365-313X.2007.03305.x
Bukau, B., Weissman, J., Horwich, A. (2006). Molecular chaperones and protein quality control. Cell 125, 443–451. doi: 10.1016/j.cell.2006.04.014
Chu, C. C., Swamy, K., Li, H. M. (2020). Tissue-specific regulation of plastid protein import via transit-peptide motifs. Plant Cell 32, 1204–1217. doi: 10.1105/tpc.19.00702
Ding, F., Zhang, B.-L., Li, F., Li, Y.-R., Li, J.-H., Lu, Y.-T. (2022). General control non-repressible 20 functions in the salt stress response of arabidopsis seedling by modulating ABA accumulation. Environ. Exp. Bot. 198, 104856. doi: 10.1016/j.envexpbot.2022.104856
Foyer, C. H., Noctor, G. (2005). Redox homeostasis and antioxidant signaling: A metabolic interface between stress perception and physiological responses. Plant Cell 17, 1866–1875. doi: 10.1105/tpc.105.033589
Fu, Z. W., Li, J. H., Feng, Y. R., Yuan, X., Lu, Y. T. (2021). The metabolite methylglyoxal-mediated gene expression is associated with histone methylglyoxalation. Nucleic Acids Res. 49, 1886–1899. doi: 10.1093/nar/gkab014
Hartl, F. U., Bracher, A., Hayer-Hartl, M. (2011). Molecular chaperones in protein folding and proteostasis. Nature 475, 324–332. doi: 10.1038/nature10317
Hasanuzzaman, M., Bhuyan, M., Parvin, K., Bhuiyan, T. F., Anee, T. I., Nahar, K., et al. (2020). Regulation of ROS metabolism in plants under environmental stress: A review of recent experimental evidence. Int. J. Mol. Sci. 21:8695–8737. doi: 10.3390/ijms21228695
Hasanuzzaman, M., Raihan, M. R. H., Masud, A. A. C., Rahman, K., Nowroz, F., Rahman, M., et al. (2021). Regulation of reactive oxygen species and antioxidant defense in plants under salinity. Int. J. Mol. Sci. 22, 9326–9355. doi: 10.3390/ijms22179326
Julkowska, M. M., Testerink, C. (2015). Tuning plant signaling and growth to survive salt. Trends Plant Sci. 20, 586–594. doi: 10.1016/j.tplants.2015.06.008
Jungkunz, I., Link, K., Vogel, F., Voll, L. M., Sonnewald, S., Sonnewald, U. (2011). AtHsp70-15-deficient arabidopsis plants are characterized by reduced growth, a constitutive cytosolic protein response and enhanced resistance to TuMV. Plant journal: Cell Mol. Biol. 66, 983–995. doi: 10.1111/j.1365-313X.2011.04558.x
Koussevitzky, S., Suzuki, N., Huntington, S., Armijo, L., Sha, W., Cortes, D., et al. (2008). Ascorbate peroxidase 1 plays a key role in the response of arabidopsis thaliana to stress combination. J. Biol. Chem. 283, 34197–34203. doi: 10.1074/jbc.M806337200
Latijnhouwers, M., Xu, X. M., Møller, S. G. (2010). Arabidopsis stromal 70-kDa heat shock proteins are essential for chloroplast development. Planta 232, 567–578. doi: 10.1007/s00425-010-1192-z
Leaden, L., Busi, M. V., Gomez-Casati, D. F. (2014). The mitochondrial proteins AtHscB and AtIsu1 involved in fe-s cluster assembly interact with the Hsp70-type chaperon AtHscA2 and modulate its catalytic activity. Mitochondrion 19 Pt B, 375–381. doi: 10.1016/j.mito.2014.11.002
Lee, S., Tsai, F. T. (2005). Molecular chaperones in protein quality control. J. Biochem. Mol. Biol. 38, 259–265. doi: 10.5483/BMBRep.2005.38.3.259
Leng, L., Liang, Q., Jiang, J., Zhang, C., Hao, Y., Wang, X., et al. (2017). A subclass of HSP70s regulate development and abiotic stress responses in arabidopsis thaliana. J. Plant Res. 130, 349–363. doi: 10.1007/s10265-016-0900-6
Li, Z. Q., Li, J. T., Bing, J., Zhang, G. F. (2019). [The role analysis of APX gene family in the growth and developmental processes and in response to abiotic stresses in arabidopsis thaliana]. Yi Chuan = Hereditas 41, 534–547. doi: 10.16288/j.yczz.19-026
Li, G., Li, J., Hao, R., Guo, Y. (2017). Activation of catalase activity by a peroxisome-localized small heat shock protein Hsp17.6CII. J. Genet. Genomics = Yi Chuan xue bao 44, 395–404. doi: 10.1016/j.jgg.2017.03.009
Li, G., Li, Z., Yang, Z., Leshem, Y., Shen, Y., Men, S. (2021). Mitochondrial heat-shock cognate protein 70 contributes to auxin-mediated embryo development. Plant Physiol. 186, 1101–1121. doi: 10.1093/plphys/kiab138
Lin, B. L., Wang, J. S., Liu, H. C., Chen, R. W., Meyer, Y., Barakat, A., et al. (2001). Genomic analysis of the Hsp70 superfamily in arabidopsis thaliana. Cell Stress chaperones 6, 201–208. doi: 10.1379/1466-1268(2001)006<0201:GAOTHS>2.0.CO;2
Li, H. M., Schnell, D., Theg, S. M. (2020). Protein import motors in chloroplasts: On the role of chaperones. Plant Cell 32, 536–542. doi: 10.1105/tpc.19.00300
Liu, Y., Ji, X., Nie, X., Qu, M., Zheng, L., Tan, Z., et al. (2015). Arabidopsis AtbHLH112 regulates the expression of genes involved in abiotic stress tolerance by binding to their e-box and GCG-box motifs. New Phytol. 207, 692–709. doi: 10.1111/nph.13387
Liu, W. C., Song, R. F., Qiu, Y. M., Zheng, S. Q., Li, T. T., Wu, Y., et al. (2022a). Sulfenylation of ENOLASE2 facilitates H(2)O(2)-conferred freezing tolerance in arabidopsis. Dev. Cell 57, 1883–1898.e1885. doi: 10.1016/j.devcel.2022.06.012
Liu, W. C., Song, R. F., Zheng, S. Q., Li, T. T., Zhang, B. L., Gao, X., et al. (2022b). Coordination of plant growth and abiotic stress responses by tryptophan synthase β subunit 1 through modulation of tryptophan and ABA homeostasis in arabidopsis. Mol. Plant 15, 973–990. doi: 10.1016/j.molp.2022.04.009
Luo, X., Dai, Y., Zheng, C., Yang, Y., Chen, W., Wang, Q., et al. (2021). The ABI4-RbohD/VTC2 regulatory module promotes reactive oxygen species (ROS) accumulation to decrease seed germination under salinity stress. The New phytologist 229, 950–962. doi: 10.1111/nph.16921
Maruyama, D., Sugiyama, T., Endo, T., Nishikawa, S. (2014). Multiple BiP genes of arabidopsis thaliana are required for male gametogenesis and pollen competitiveness. Plant Cell Physiol. 55, 801–810. doi: 10.1093/pcp/pcu018
Mayer, M. P., Bukau, B. (2005). Hsp70 chaperones: Cellular functions and molecular mechanism. Cell. Mol. Life Sci. 62, 670–684. doi: 10.1007/s00018-004-4464-6
Miemyk, J. (1997). The 70 kDa stress-related proteins as molecular chaperones. Trends Plant Sci. 2, 180–187. doi: 10.1016/S1360-1385(97)85224-7
Nadarajah, K. K. (2020). ROS homeostasis in abiotic stress tolerance in plants. Int. J. Mol. Sci. 21, 5208–5236. doi: 10.3390/ijms21155208
Qi, C., Lin, X., Li, S., Liu, L., Wang, Z., Li, Y., et al. (2019). SoHSC70 positively regulates thermotolerance by alleviating cell membrane damage, reducing ROS accumulation, and improving activities of antioxidant enzymes. Plant Sci. 283, 385–395. doi: 10.1016/j.plantsci.2019.03.003
Qi, Y., Wang, H., Zou, Y., Liu, C., Liu, Y., Wang, Y., et al. (2011). Over-expression of mitochondrial heat shock protein 70 suppresses programmed cell death in rice. FEBS Lett. 585, 231–239. doi: 10.1016/j.febslet.2010.11.051
Rizhsky, L., Davletova, S., Liang, H., Mittler, R. (2004). The zinc finger protein Zat12 is required for cytosolic ascorbate peroxidase 1 expression during oxidative stress in arabidopsis. J. Biol. Chem. 279, 11736–11743. doi: 10.1074/jbc.M313350200
Sable, A., Rai, K. M., Choudhary, A., Yadav, V. K., Agarwal, S. K., Sawant, S. V. (2018). Inhibition of heat shock proteins HSP90 and HSP70 induce oxidative stress, suppressing cotton fiber development. Sci. Rep. 8, 3620. doi: 10.1038/s41598-018-21866-0
Sánchez-McSweeney, A., González-Gordo, S., Aranda-Sicilia, M. N., Rodríguez-Rosales, M. P., Venema, K., Palma, J. M., et al. (2021). Loss of function of the chloroplast membrane K(+)/H(+) antiporters AtKEA1 and AtKEA2 alters the ROS and NO metabolism but promotes drought stress resilience. Plant Physiol. Biochem. 160, 106–119. doi: 10.1016/j.plaphy.2021.01.010
Shafi, A., Gill, T., Zahoor, I., Ahuja, P. S., Sreenivasulu, Y., Kumar, S., et al. (2019). Ectopic expression of SOD and APX genes in arabidopsis alters metabolic pools and genes related to secondary cell wall cellulose biosynthesis and improve salt tolerance. Mol. Biol. Rep. 46, 1985–2002. doi: 10.1007/s11033-019-04648-3
Srivastava, R., Deng, Y., Shah, S., Rao, A. G., Howell, S. H. (2013). BINDING PROTEIN is a master regulator of the endoplasmic reticulum stress sensor/transducer bZIP28 in arabidopsis. Plant Cell 25, 1416–1429. doi: 10.1105/tpc.113.110684
Su, P. H., Li, H. M. (2008). Arabidopsis stromal 70-kD heat shock proteins are essential for plant development and important for thermotolerance of germinating seeds. Plant Physiol. 146, 1231–1241. doi: 10.1104/pp.107.114496
Su, P. H., Li, H. M. (2010). Stromal Hsp70 is important for protein translocation into pea and arabidopsis chloroplasts. Plant Cell 22, 1516–1531. doi: 10.1105/tpc.109.071415
Sung, D. Y., Vierling, E., Guy, C. L. (2001). Comprehensive expression profile analysis of the arabidopsis Hsp70 gene family. Plant Physiol. 126, 789–800. doi: 10.1104/pp.126.2.789
Tang, T., Yu, A., Li, P., Yang, H., Liu, G., Liu, L. (2016). Sequence analysis of the Hsp70 family in moss and evaluation of their functions in abiotic stress responses. Sci. Rep. 6, 33650. doi: 10.1038/srep33650
Tivendale, N. D., Ross, J. J., Cohen, J. D. (2014). The shifting paradigms of auxin biosynthesis. Trends Plant Sci. 19, 44–51. doi: 10.1016/j.tplants.2013.09.012
Uzilday, B., Turkan, I., Ozgur, R., Sekmen, A. H. (2014). Strategies of ROS regulation and antioxidant defense during transition from C3to C4 photosynthesis in the genus flaveria under PEG-induced osmotic stress. J. Plant Physiol. 171, 65–75. doi: 10.1016/j.jplph.2013.06.016
Vishwakarma, A., Tetali, S. D., Selinski, J., Scheibe, R., Padmasree, K. (2015). Importance of the alternative oxidase (AOX) pathway in regulating cellular redox and ROS homeostasis to optimize photosynthesis during restriction of the cytochrome oxidase pathway in arabidopsis thaliana. Ann. Bot. 116, 555–569. doi: 10.1093/aob/mcv122
Wang, L. F., Li, T. T., Zhang, Y., Guo, J. X., Lu, K. K., Liu, W. C. (2021a). CAND2/PMTR1 is required for melatonin-conferred osmotic stress tolerance in arabidopsis. Int. J. Mol. Sci. 22, 4014–4029. doi: 10.3390/ijms22084014
Wang, L. F., Lu, K. K., Li, T. T., Zhang, Y., Guo, J. X., Song, R. F., et al. (2021b). Maize PHYTOMELATONIN RECEPTOR1 functions in plant osmotic and drought stress tolerance. J. Exp. Bot. erab553. doi: 10.1093/jxb/erab553
Wang, W., Vinocur, B., Shoseyov, O., Altman, A. (2004). Role of plant heat-shock proteins and molecular chaperones in the abiotic stress response. Trends Plant Sci. 9, 244–252. doi: 10.1016/j.tplants.2004.03.006
Waszczak, C., Carmody, M., Kangasjärvi, J. (2018). Reactive oxygen species in plant signaling. Annu. Rev. Plant Biol. 69, 209–236. doi: 10.1146/annurev-arplant-042817-040322
Wei, Y., Liu, W., Hu, W., Yan, Y., Shi, H. (2020). The chaperone MeHSP90 recruits MeWRKY20 and MeCatalase1 to regulate drought stress resistance in cassava. New Phytol. 226, 476–491. doi: 10.1111/nph.16346
Wenjing, W., Chen, Q., Singh, P. K., Huang, Y., Pei, D. (2020). CRISPR/Cas9 edited HSFA6a and HSFA6b of arabidopsis thaliana offers ABA and osmotic stress insensitivity by modulation of ROS homeostasis. Plant Signaling Behav. 15, 1816321. doi: 10.1080/15592324.2020.1816321
Wu, G. Z., Meyer, E. H., Richter, A. S., Schuster, M., Ling, Q., Schöttler, M. A., et al. (2019). Control of retrograde signalling by protein import and cytosolic folding stress. Nat. Plants 5, 525–538. doi: 10.1038/s41477-019-0415-y
Wu, S. H., Wang, C., Chen, J., Lin, B. L. (1994). Isolation of a cDNA encoding a 70 kDa heat-shock cognate protein expressed in vegetative tissues of arabidopsis thaliana. Plant Mol. Biol. 25, 577–583. doi: 10.1007/BF00043887
Xu, L., Gao, J., Guo, L., Yu, H. (2020). Heat shock protein 70 (HmHsp70) from hypsizygus marmoreus confers thermotolerance to tobacco. AMB Express 10, 12. doi: 10.1186/s13568-020-0947-6
Yi, J., Zhao, D., Chu, J., Yan, J., Liu, J., Wu, M., et al. (2019). AtDPG1 is involved in the salt stress response of arabidopsis seedling through ABI4. Plant Sci. 287, 110180. doi: 10.1016/j.plantsci.2019.110180
Yu, Y., Wang, J., Li, S., Kakan, X., Zhou, Y., Miao, Y., et al. (2019). Ascorbic Acid Integrates the Antagonistic Modulation of Ethylene and Abscisic Acid in the Accumulation of Reactive Oxygen Species. Plant physiology 179, 1861–1875. doi: 10.1104/pp.18.0125
Yuan, H. M., Liu, W. C., Lu, Y. T. (2017). CATALASE2 coordinates SA-mediated repression of both auxin accumulation and JA biosynthesis in plant defenses. Cell Host Microbe 21, 143–155. doi: 10.1016/j.chom.2017.01.007
Zemanovic, S., Ivanov, M. V., Ivanova, L. V., Bhatnagar, A., Michalkiewicz, T., Teng, R. J., et al. (2018). Dynamic phosphorylation of the c terminus of Hsp70 regulates the mitochondrial import of SOD2 and redox balance. Cell Rep. 25, 2605–2616.e2607. doi: 10.1016/j.celrep.2018.11.015
Zhang, Q., Cai, W., Ji, T. T., Ye, L., Lu, Y. T., Yuan, T. T. (2020). WRKY13 enhances cadmium tolerance by promoting d-CYSTEINE DESULFHYDRASE and hydrogen sulfide production. Plant Physiol. 183, 345–357. doi: 10.1104/pp.19.01504
Zhang, X. P., Glaser, E. (2002). Interaction of plant mitochondrial and chloroplast signal peptides with the Hsp70 molecular chaperone. Trends Plant Sci. 7, 14–21. doi: 10.1016/S1360-1385(01)02180-X
Zhang, B.-L., Guo, C.-C., Ding, F., Lu, Y.-T., Fu, Z.-W. (2019). 14-3-3s function in plant cadmium response by changes of glutathione and glutathione synthesis in arabidopsis. Environ. Exp. Bot. 163, 69–77. doi: 10.1016/j.envexpbot.2019.04.009
Zhang, Y., Wang, L. F., Li, T. T., Liu, W. C. (2021). Mutual promotion of LAP2 and CAT2 synergistically regulates plant salt and osmotic stress tolerance. Front. Plant Sci. 12, 672672. doi: 10.3389/fpls.2021.672672
Zhao, M., Liu, Q., Zhang, Y., Yang, N., Wu, G., Li, Q., et al. (2020). Alleviation of osmotic stress by H(2)S is related to regulated PLDα1 and suppressed ROS in arabidopsis thaliana. J. Plant Res. 133, 393–407. doi: 10.1007/s10265-020-01182-3
Zhu, X., Zhao, X., Burkholder, W. F., Gragerov, A., Ogata, C. M., Gottesman, M. E., et al. (1996). Structural analysis of substrate binding by the molecular chaperone DnaK. Sci. (New York N.Y.) 272, 1606–1614. doi: 10.1126/science.272.5268.1606
Zou, J. J., Li, X. D., Ratnasekera, D., Wang, C., Liu, W. X., Song, L. F., et al. (2015). Arabidopsis CALCIUM-DEPENDENT PROTEIN KINASE8 and CATALASE3 function in abscisic acid-mediated signaling and H2O2 homeostasis in stomatal guard cells under drought stress. Plant Cell 27, 1445–1460. doi: 10.1105/tpc.15.00144
Keywords: osmotic stress, ROS accumulation, antioxidant enzymes, cpHSC70-1, Arabidopsis thaliana
Citation: Ding F, Li F and Zhang B (2022) A plastid-targeted heat shock cognate 70-kDa protein confers osmotic stress tolerance by enhancing ROS scavenging capability. Front. Plant Sci. 13:1012145. doi: 10.3389/fpls.2022.1012145
Received: 05 August 2022; Accepted: 12 September 2022;
Published: 05 October 2022.
Edited by:
Iftikhar Ali, State Key Laboratory of Molecular Developmental Biology, (CAS), ChinaReviewed by:
Jan Smalle, University of Kentucky, United StatesAimal Khan, University of Chinese Academy of Sciences, China
Copyright © 2022 Ding, Li and Zhang. This is an open-access article distributed under the terms of the Creative Commons Attribution License (CC BY). The use, distribution or reproduction in other forums is permitted, provided the original author(s) and the copyright owner(s) are credited and that the original publication in this journal is cited, in accordance with accepted academic practice. No use, distribution or reproduction is permitted which does not comply with these terms.
*Correspondence: Binglei Zhang, emhhbmdibEB3aHUuZWR1LmNu