- 1Division of Biological Science, Graduate School of Science, Nagoya University, Chikusa, Nagoya, Japan
- 2Institute of Transformative Bio-Molecules (WPI-ITbM), Nagoya University, Chikusa, Nagoya, Japan
Stomata open in response to several environmental stimuli, such as light and low CO2. Plasma membrane (PM) H+-ATPase in guard cells plays a pivotal role for light-induced stomatal opening. In contrast, stomata close in response to the dark or plant hormone abscisic acid (ABA). However, molecular mechanisms of stomatal movements remain unclear. To elucidate the molecular mechanism of stomatal movements, we performed a genetic screen based on stomatal aperture-dependent weight decrease of detached leaves from EMS-treated Arabidopsis thaliana and isolated a rapid transpiration in detached leaves 2 (rtl2). The rtl2 mutant showed constitutive open-stomata phenotype with lower leaf temperature. ABA had no effect on stomatal aperture in rtl2. The rtl2 mutant also showed increased stomatal density, severe dwarf phenotype with pale green leaves and dark veins. Map-based analysis of the RTL2 locus revealed that the rtl2 mutant possesses a single nucleotide substitution, which induces amino acid substitution Gly162 to Glu in the tryptophan synthase ß subunit 1 (TSB1). The TSB1 encodes an enzyme in tryptophan (Trp) biosynthetic pathway. Amount of TSB1 protein was drastically reduced in rtl2 mutant. A different allele of tsb1 mutant (tsb1-1) also showed constitutive open-stomata phenotype with reduced TSB1 protein as in rtl2. Analyses of test-crossed plants of rtl2 and tsb1-1 showed open-stomata and dwarf phenotypes. These results indicate that a responsible gene for rtl2 is TSB1. We further investigated stomatal phenotype in mutants from Trp biosynthetic pathway, such as wei2-1 wei7-1, trp3-1, and tsb2-1. The trp3-1 mutant showed significant wider stomatal aperture as well as tsb1-1. Trp biosynthetic pathway closely relates to auxin biosynthesis. Then, we investigated auxin responsible genes and found that an expression of AUR3 was up in rtl2. In contrast, auxin had no effect on stomatal aperture in Arabidopsis and the phosphorylation status of PM H+-ATPase in guard cell protoplasts from Vicia faba. In addition, auxin antagonist had no effect on stomatal aperture. Interestingly, tsb1-1 grown under hydroponic culture system showed normal stomatal aperture by exogenously application of Trp. These results suggest that open stomata phenotype in tsb1-1 is due to Trp deficiency but not auxin.
Introduction
Stomata in the plant epidermis, surrounded by a pair of guard cells, control gas exchange between the plants and the atmosphere. Opening of the stomata induces both transpiration and CO2 uptake for photosynthesis. Under drought condition, stomata close in response to the plant hormone abscisic acid (ABA) to prevent water loss (Schroeder et al., 2001; Shimazaki et al., 2007). Diverse external and internal stimuli, such as blue light (BL), red light (RL), the phytotoxin fusicoccin (FC), CO2, ABA, and microbial elicitors, regulate stomatal aperture (Munemasa et al., 2015; Inoue and Kinoshita, 2017; Ye et al., 2020). It has been demonstrated that stomatal opening contributes photosynthesis, plant growth, and yield (Wang et al., 2014; Toh et al., 2021; Zhang et al., 2021). In the BL-induced stomatal opening, BL photoreceptor phototropins activate plasma membrane (PM) H+-ATPase through the phosphorylation of C-terminal penultimate residue, threonine (Thr) (Kinoshita and Shimazaki, 1999; Kinoshita et al., 2001). BL-activated PM H+-ATPase creates an electrochemical gradient of H+ across the PM and an inside-negative electrical potential of PM that activate inward-rectifying voltage-gated K+ channels for K+ influx into guard cells (Schroeder et al., 1987). The K+ accumulation induces elevation of turgor pressure and opening of stomata (Schroeder et al., 2001; Shimazaki et al., 2007). Several signalling components, a protein kinase BLUE LIGHT SIGNALING1 (BLUS1) (Takemiya et al., 2013), a Raf-like protein kinase BLUE LIGHT-DEPENDENT H+-ATPASE PHOSPHORYLATION (BHP) (Hayashi et al., 2017), and a Type 1 protein phosphatase (PP1) (Takemiya et al., 2006), mediate the BL signalling pathway in guard cells. RL also induces stomatal opening through the phosphorylation of PM H+-ATPase penultimate residue, Thr, and activation of PM H+-ATPase in intact leaves (Ando and Kinoshita, 2018). However, the molecular mechanism of signalling pathway for light-induced stomatal opening remains incompletely understood (Inoue and Kinoshita, 2017).
Tryptophan (Trp) is an essential amino acid for all living organisms, but Trp is not synthesized in animals and some eubacteria. All catalytic enzymes and their encoding genes for Trp biosynthetic pathway have been identified in plants (Radwanski and Last, 1995; Tzin and Galili, 2010). The Trp biosynthesis includes six reaction steps from chorismate to Trp. In the first step, anthranilate synthase (AS) (CE 4.1.3.27) catalyzes a transfer of an amino group of glutamine to chorismate to generate anthranilate and pyruvate. In the second step, anthranilate phosphoribosylanthranilate transferase (PAT) (CE 2.4.2.1) catalyzes conversion of anthranilate and phosphoribosylpyrophosphate into phosphoribosylanthranilate and pyrophosphate. In the third step, phosphoribosylanthranilate isomerase (PAI) (CE 5.3.1.24) catalyzes conversion of phosphoribosylanthranilate into l-(O-carboxyphenylamino)-l-deoxyribulose-5-phosphate (CDRP). In the fourth step, indole-3-glycerol phosphate synthase (IGPS) (EC 4.1.1.48) catalyzes conversion of 1-(O-carboxyphenylamino)-1-deoxyribulose-5-phosphate to indole-3-glycerol phosphate. Trp synthase (TS) (CE 4.2.1.20), α (TSA) and ß (TSB) subunits, is involved in the last two steps. TSA cleaves indole-3-glycerol phosphate to indole and glyceraldehyde-3-phosphate. Finally, TSB catalyzes condensation of indole and serine to produce Trp. Trp biosynthetic pathway closely relates to auxin biosynthesis (Ouyang et al., 2000; Stepanova et al., 2008; Tao et al., 2008; Mashiguchi et al., 2011; Zhao, 2012; Tivendale et al., 2014).
Among them, Trp synthase ß subunit 1 (TSB1) is essential for Trp synthesis in plants; thus, it affects several developmental and physiological responses in plants (Last et al., 1991). Jing et al. (2009) reported that smo1/trp2-301, a mutant of TSB1, exhibits a reduction of the size of its aerial organs because of the retardation of growth by cell expansion, and that these phenotypes are rescued by addition of Trp. The trp2 mutants also showed higher endogenous IAA content and increased expression of auxin responsive genes, such as IAA1, IAA5 and IAA6. Ursache et al. (2014) reported that trp2-12 and trp2-13, mutants of TSB1, showed incomplete vascular tissue development, and found that the expression of most of the HD-ZIPIII genes, which play a crucial role in xylem specification, is down regulated in the trp2-1. Wang et al. (2015) showed that trp2-1, a mutant of TSB1, has higher level of IAA than the wild type probably through the Trp-independent auxin biosynthetic pathway. Liu et al. (2022) reported that TSB1 interacts with ABA metabolism enzyme, ß-glucosidase 1 and mediates regulation of plant growth and abiotic stress responses.
In the previous study, we performed a screen focused on stomatal aperture-dependent of weight decrease of the detached leaves from ethyl methanesulfonate (EMS)-treated Arabidopsis thaliana and identifed a rapid transpiration in detached leaves 1 (rtl1) mutant. The rtl1 mutant showed ABA-insensitive stomatal phenotype and possesses a novel missense mutantion in the Mg-chelatase H subunit (CHLH). It is worthy of note that CHLH affects ABA-induced stomatal closure and inhibition of stomatal opening, but does not act as an ABA receptor (Tsuzuki et al., 2011). In this study, we isolated rtl2 mutant, which shows open-stomata phenotype. Map-based analysis and genetic analysis strongly indicated that a responsible gene for rtl2 is TSB1 involved in Trp biosynthetic pathway. Based on phenotypic and genetic analyzes, we propose that the Trp synthesis pathway has a significant effect on stomatal phenotypes, control of stomatal aperture and density.
Materials and methods
Plant materials and growth conditions
For plant growth on soil, plants were grown under 16-h fluorescent light (50 µmol m-2 s-1)/8-h dark cycle at 24°C in 55-70% humidity in a growth room. For plant growth on plate followed by on soil, seeds were surface-sterilized and sown on Murashige and Skoog-agar plate supplied with 1% (w/v) sucrose under 16-h fluorescent light (50 µmol m-2 s-1)/8-h dark cycle. Four-week-old plants were transferred to soil and grown under 16-h fluorescent light (50 µmol m-2 s-1)/8-h dark cycle at 24°C in 55-70% humidity in a growth room. gl1 [Columbia (Col-0), carrying gl1 mutation] is the background ecotype of an rtl2 mutant and used as the wild type (WT). We backcrossed rtl2 with gl1 three times. The mutants used in this study, tsb1-1 (SAIL_886_A01), tsb2-1 (SAIL_598_H05), trp3-1 (CS8331; Radwanski et al., 1996), wei2-1 wei7-1 (CS16399; Stepanova et al., 2005) were obtained from the Arabidopsis Biological Resource Center (ABRC) (Ohio State University, Columbus, OH, USA). Col-0 is the background ecotype of these mutants. For plant growth in hydroponic culture, seeds were surface-sterilized and sown on Murashige and Skoog-agar plate as described above. Ten-day-old seedlings were transferred to hydroponic culture system with a nutrient solution (Gibeaut et al., 1997). The solution was constantly replaced every 1 week.
Isolation of the rtl2 mutant and identification of the RTL2 locus
Mutant screening based on stomatal aperture-dependent water loss was performed as previously described (Tsuzuki et al., 2011). Briefly, ethyl methanesulfonate (EMS)-treated gl1 M2 seeds were germinated and grown on soil. We measured the fresh weight of a detached rosette leaf at 0 and 90 min from each 4-week-old M2 plant and isolated some rapid transpiration in detached leaves (rtl) mutants (Tsuzuki et al., 2011). In this study, we investigated an rtl2 mutant, which shows rapid weight change compared to WT plants. Mapping populations were generated by crossing the rtl2 mutant with the Landsberg erecta (Ler) accession of Arabidopsis thaliana. RTL2 locus was identified by mapping using simple-sequence length polymorphism (SSLP) and cleaved amplified polymorphism (CAPS) markers from 910 F2 plants showing dwarf phenotype with pale green leaves and dark veins.
Measurement of stomatal aperture
Stomatal apertures in the isolated epidermis were measured according to the previous method (Inoue et al., 2008) with modifications. The epidermal fragments isolated from overnight dark-adapted 4- to 6-week-old plants or 4- to 6-week-old plants from illuminated growth condition at zeitgeber time (ZT) 4 to 7 were incubated in basal buffer (5 mM MES-BTP, pH 6.5, 50 mM KCl, and 0.1 mM CaCl2). For investigations of light-induced stomatal opening and effect of ABA or auxinole, the epidermal fragments were incubated under light [blue light (Stick-B-32; EYELA, Tokyo, Japan) at 10 µmol m-2 s-1 superimposed on background red light (LED-R; EYELA, Tokyo, Japan) at 50 µmol m-2 s-1] at 24°C in the presence or absence of 20 µM ABA or 10 µM auxinole for 2.5 hr or kept in the dark at 24°C for 2.5 hr. For investigation of the effect of auxin, the epidermal tissues were incubated in the basal buffer for 1.5 hr in darkness to deplete endogenous auxin. Then, the pre-incubated epidermal tissues were treated with 10 µM IAA in the dark for 3 hr. Stomatal apertures were measured microscopically by focusing on the inner lips of stomata in the abaxial epidermis.
For determination of stomatal aperture from test-crossed plants under illuminated growth condition, we used intact leaves for measurements according to the previous method (Toh et al., 2018). Rosette leaves were detached from the plants at ZT 4 to 7. We cut out central areas of the leaves without the midrib were cut out and mounted leaf disc on microscope slides with the abaxial side attached to the cover glass. We obtained Images using an optical microscope (BX43; Olympus) with a charge-coupled device (CCD) camera (DP27; Olympus) with a x20 objective lens (UPlanFL N; Olympus). For getting extended focus imaging, we used cellSens standard software (Olympus) to maximize the number of analyzable focused stomata within each image. Stomatal apertures in the abaxial side were measured on the inner lips of stomata using ImageJ software (http://imagej.nih.gov/ij/).
Determination of leaf temperature using the infrared thermography
Plants were grown on MS plate for 4 weeks and transferred to soil in each pot for 1 week. We measured leaf temperatures using a TVS-500EX infrared thermography instrument (NEC Avio Infrared Technologies Co., Ltd.) and analyzed images using the Avio Thermography Studio software.
Determination of stomatal size and density
Stomatal size and density were determined according to a previous method (Wang et al., 2011).
RT-PCR and quantitative RT-PCR
We purified total RNA from rosette leaves of 4- to 6-week-old plants using an RNeasy Plant Mini Kit (Qiagen). We synthesized 1st-strand cDNAs using a Takara PrimeScript II First Strand cDNA Synthesis Kit (Takara) with oligo(dT)12–18 primer. For RT-PCR, we amplified cDNA fragments by PCR using specific primers (Supplementary Table S2). Quantitative RT-PCR (qRT-PCR) was performed as previously described (Kinoshita et al., 2011) using specific primers (Supplementary Table S2). We used TUB2 (AT5G62690) as an internal standard for PCRs.
Anti-TSB1 antibody
Anti-TSB1 antibody was raised against the recombinant TSB1ΔN, TSB1 protein without chloroplast targeting signal peptide in its N-terminus, as an antigen in a rabbit (Medical & Biological Laboratories). We amplified the TSB1 DNA fragment from 1st-strand Arabidopsis cDNA by PCR using the specific primers 5′-CGGGATCCGACCCGGCCCTGTGGCAAC-3′ and 5′-CGGGATCCTCAAACATCAAGATATTTAGCCACTGTCTGAAC-3′. We cloned the TSB1 CDS of 202–1413 bp containing BamHI site at both ends into the BamHI site of the pGEX-2T vector (GE Healthcare) to express as a fusion protein with glutathione S-transferase (GST). The pGEX-2T-TSB1 was transformed into the E. coli BL21 strain. The fusion protein (GST-TSB1ΔN) was purified using the glutathione-Sepharose 4B (GE Healthcare). The TSB1ΔN protein was obtained by digestion with thrombin to cut off the GST and used for the immunization as antigen.
Immunoblots
Immunoblot analysis was performed according to the methods described in Hayashi et al. (2010) with modifications. We grinded leaves from 5- to 6-week-old plants using a mortar and pestle in extraction buffer (50 mM MOPS–KOH, pH 7.5, 2.5 mM EDTA, 100 mM NaCl, 1 mM phenylmethylsulfonyl fluoride, 20 µM leupeptin, and 2 mM DTT). Proteins (20 µg) were loaded and separated by SDS-PAGE. We detected TSB1 protein using the polyclonal antibody raised against recombinant TSB1ΔN protein (Anti-TSB1 antibody) in rabbits. We detected the 14-3-3 proteins with the anti-14-3-3 protein (GF14 ø) antibody (Kinoshita and Shimazaki, 1999) as a control. We used the antibodies at a 3,000-fold dilution.
Detections of PM H+-ATPase protein and phosphorylation status of the penultimate residue, Thr, of PM H+-ATPase in guard cell protoplasts (GCPs) from Vicia faba were performed using anti-H+-ATPase antibody and anti-pThr antibody, respectively (Hayashi et al., 2010).
Measurement of Tryptophan synthase ß subunit activity
TSB activity was determined according to Greenberg and Galston (1959) with modifications. For measurement in leaf extracts, leaves from 5- to 6-week-old plants were ground in phosphate buffer (0.1 M Potassium phosphate-KOH, pH 8.2, 30% [w/v] Insoluble-Polyvinylpolypyrrolidone [Sigma-Aldrich]) with 212-300 µm glass beads (Sigma-Aldrich) using a mortar and pestle. Then the extract was sonicated and centrifuged at 4 °C (12,000 g for 15 min). The supernatant was used as the enzyme source. The supernatant (150–250 µg of protein) was suspended in reaction buffer (50 µl; 80 mM Potassium phosphate-KOH, pH 8.2, 60 mM L-Serine, 200 µM indole, 10 µg/ml pyridoxal phosphate) and reacted at 30 °C for 90 min. The reaction was stopped by the addition of 5 µl of 0.2 M NaOH. To assay for residual unreacted indole, 200 µl of toluene was added to the reaction mixture and then shaken and centrifuged at 1,500 g for 15 min. The resulting toluene layer was transferred and mixed with 4 times the volume of Ethanol and twice the volume of Ehrlich’s reagent (36 mg/ml p-dimethylaminobenzaldehyde, 2.13 M HCl dissolved in Ethanol). After incubation at 25 °C for 30 min, the absorbance at 540 nm was measured and differences of absorbance between before and after the reaction was converted to the disappearance of indole using the standard curve generated with dilution series of indole. For expression of recombinant TSB1ΔN protein, the TSB1 DNA fragments derived from WT and rtl2 were cloned into the vector as described above. The recombinant TSB1ΔN proteins were purified using the glutathione-Sepharose 4B (GE Healthcare) and used for measurement of TSB activity.
Isolation of GCPs from Vicia faba and auxin treatments
We isolated guard cell protoplasts (GCPs) enzymatically from lower epidermis of leaves from 4- to 6-week-old Vicia faba according to a previous method (Hayashi et al., 2021). GCPs in suspension buffer (5mM MES-NaOH [pH 6.0], 10mM KCl, 0.4 M mannitol and 1mM CaCl2) were treated with auxins (IAA, 1-NAA, 2,4-D) and fusicoccin (FC) at 10 µM in the dark for 30 min. Proteins (20 µg) were loaded and separated by SDS-PAGE.
Accession number
ASA1; AT5G05730, ASB1; AT1G25220, AUR3; AT4G37390, IAA1; AT4G14560, IAA24; AT4G14560, SAUR9; AT4G36110, TSA1; AT3G54640, TSB1; AT5G54810, TSB2; AT4G27070, TUB2; AT5G62690.
Results
An rtl2 mutant exhibited widely opened stomatal phenotype
To elucidate the mechanism of stomatal movement, we have performed a mutant screening based on stomatal aperture-dependent weight loss of detached leaves via transpiration using a microbalance (Tsuzuki et al., 2011). In addition to a rapid transpiration in detached leaves 1 (rtl1) mutant (Tsuzuki et al., 2011), we isolated an rtl2 mutant. The rtl2 showed rapid weight loss of detached leaves under growth condition. The WT (Col-gl1; background plant of the screening) leaf weight decreased to 59% of initial weight for 90 min, whereas the rtl2 leaf weight decreased to 2% (Figure 1A). In addition, rtl2 mutant showed severe dwarf phenotype with pale green leaves and dark veins under soil grown condition (Figure 1B). It is worthy of note that stomatal size and index in rtl2 and tsb1-1 were almost comparable to those in background strains. In contrast, stomatal density in rtl2 and tsb1-1 were ~30-46% increase compared to that in background strains (Table 1). Furthermore, color of dry seeds from rtl2 and tsb1-1 showed a lighter color than background strain seeds (Supplementary Figure 1) (Last et al., 1991).
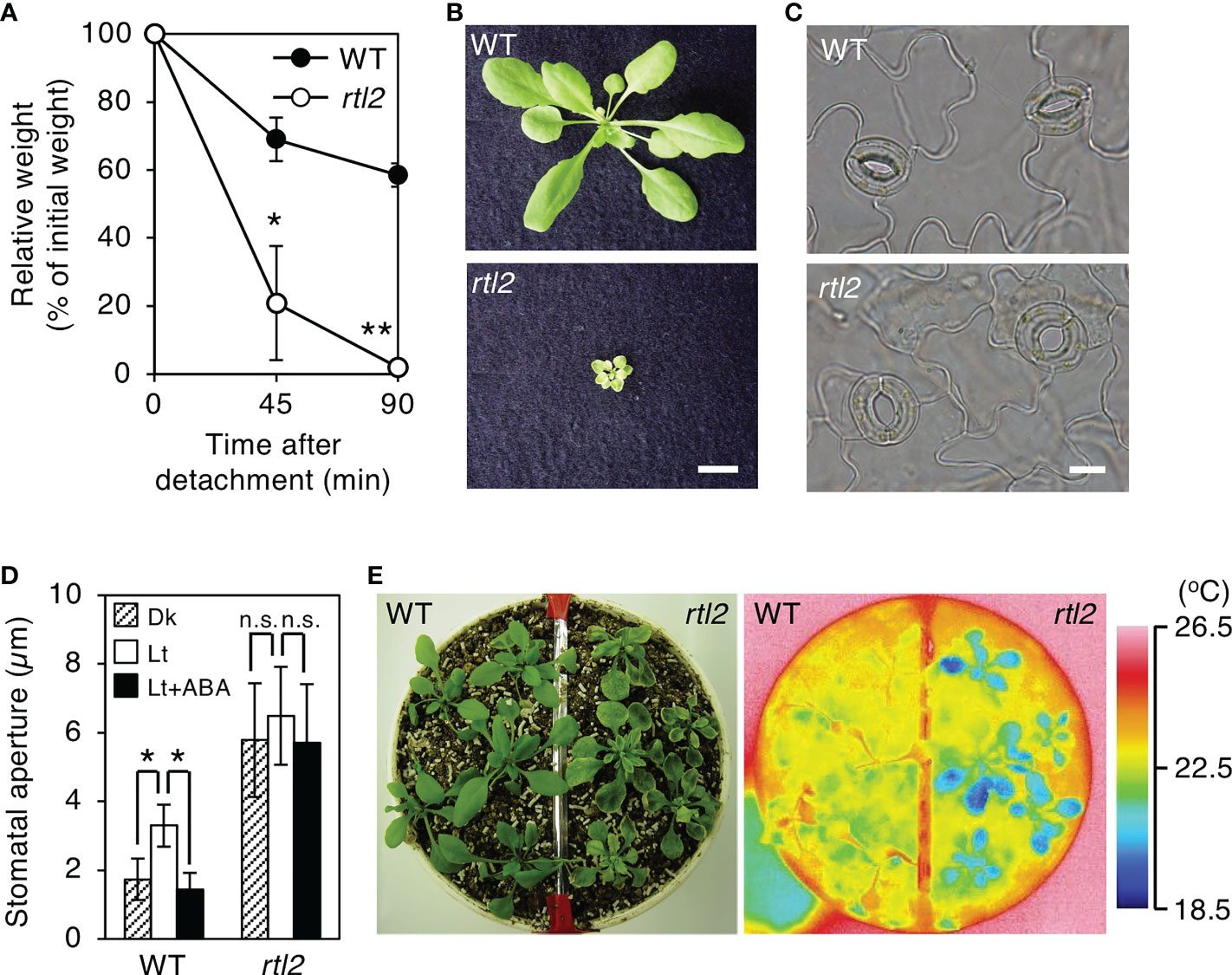
Figure 1 Characterization of the rtl2 mutant. (A) Kinetics of the fresh weight change in the detached rosette leaves from 4-week-old WT (closed circles) and rtl2 (open circles) plants. The relative weights of leaves are presented as a percentage of the initial weight, which was the weight of each rosette leaf immediately after detachment from the plants. Values are means of three independent experiments with SDs. Asterisks indicate a significant difference in leaf weight change relative to WT by Student t test; *P < 0.05, **P < 0.01. (B) Four-week-old WT and rtl2 plants grown on soil. Scale bar = 1 cm. (C) Typical stomata in the epidermis of WT and rtl2 plants under illuminated growth conditions. Scale bar = 10 µm. (D) Stomatal aperture of 5-week-old WT and rtl2 plants. Plants were grown on MS plate for 4 weeks and transferred to soil for 1 week. Epidermal tissues from dark-adapted plants were incubated under light (blue light at 10 µmol m-2 s-1 superimposed on red light at 50 µmol m-2 s-1) with (black; Lt+ABA) or without (white; Lt) 20 µM ABA or kept in the dark (hatched; Dk) for 2.5 hr. Values represent mean ± SD (n = 3); measurement of > 25 stomata in each experiment. Asterisks indicate a significant difference by Student t test; *P < 0.05. n.s., not significant. (E) Thermal image (right) and the corresponding bright-field image (left) of WT and rtl2 plants. The images of the 5-week-old plants were grown on MS plate for 4 weeks and transferred to soil for 1 week.
As shown in Figure 1C, stomata of rtl2 opened widely compared to WT under illuminated growth condition. We further examined stomatal responses of rtl2 in detail. The stomata in WT closed in the dark and opened in response to light, and ABA (20 µM) suppressed light-induced stomatal opening (Figure 1D). By contrast, the stomata in rtl2 opened widely even in the dark and 20 µM ABA had no effect on stomatal aperture. In accord with open-stomata phenotype in rlt2 mutant, rtl2 mutant exhibited clear low leaf temperature phenotype under illuminated growth condition (Figure 1E). Average leaf temperature of the rtl2 mutant was reduced over 3.0°C relative to WT.
Open stomata phenotype is caused by a missense mutation in TSB1
To identify the RTL2 locus, we performed a map-based analysis and found strong linkage the CAPS marker K5F14-1-1 and MBG8-1 in rtl2 (Figure 2A). According to the Arabidopsis Information Resource (TAIR) database, Tryptophan synthase beta-subunit 1 (TSB1; AT5G54810) is a candidate gene. Because there is a TSB1 locus between K5F14-1-1 and MBG8-1 and known TSB1 mutants showed dwarf phenotype with pale green leaves and dark veins same as in rtl2 (Figures 1B, 2B) (Jing et al., 2009). Sequence analysis of TSB1 cDNA from rtl2 revealed a single nucleotide substitution from G485 to A, which induces a novel missense mutation from Gly162 to Glu (Figure 2B). Next, we investigated theTSB1 transcript level and protein amount of TSB1 in rosette leaves. As shown in Figure 2C, 2D, the level of TSB1 transcript was similar to that of the WT, whereas the amount of TSB1 protein was significantly decreased in rtl2. We further determined the TSB activity in leaf extracts by measuring disappearance of indole. The results showed that rtl2 has ~35% TSB catalytic activity compared to that of WT (Figure 2E). Reduction of TSB activity in rtl2 may be caused by not only lower amount of TSB1 protein but also less activity of rtl2 mutation (Gly162 to Glu in TSB1), because the recombinant TSB1-G162E has only ~7.2% TSB activity compared to that of WT-TSB1 (Figure 2F).
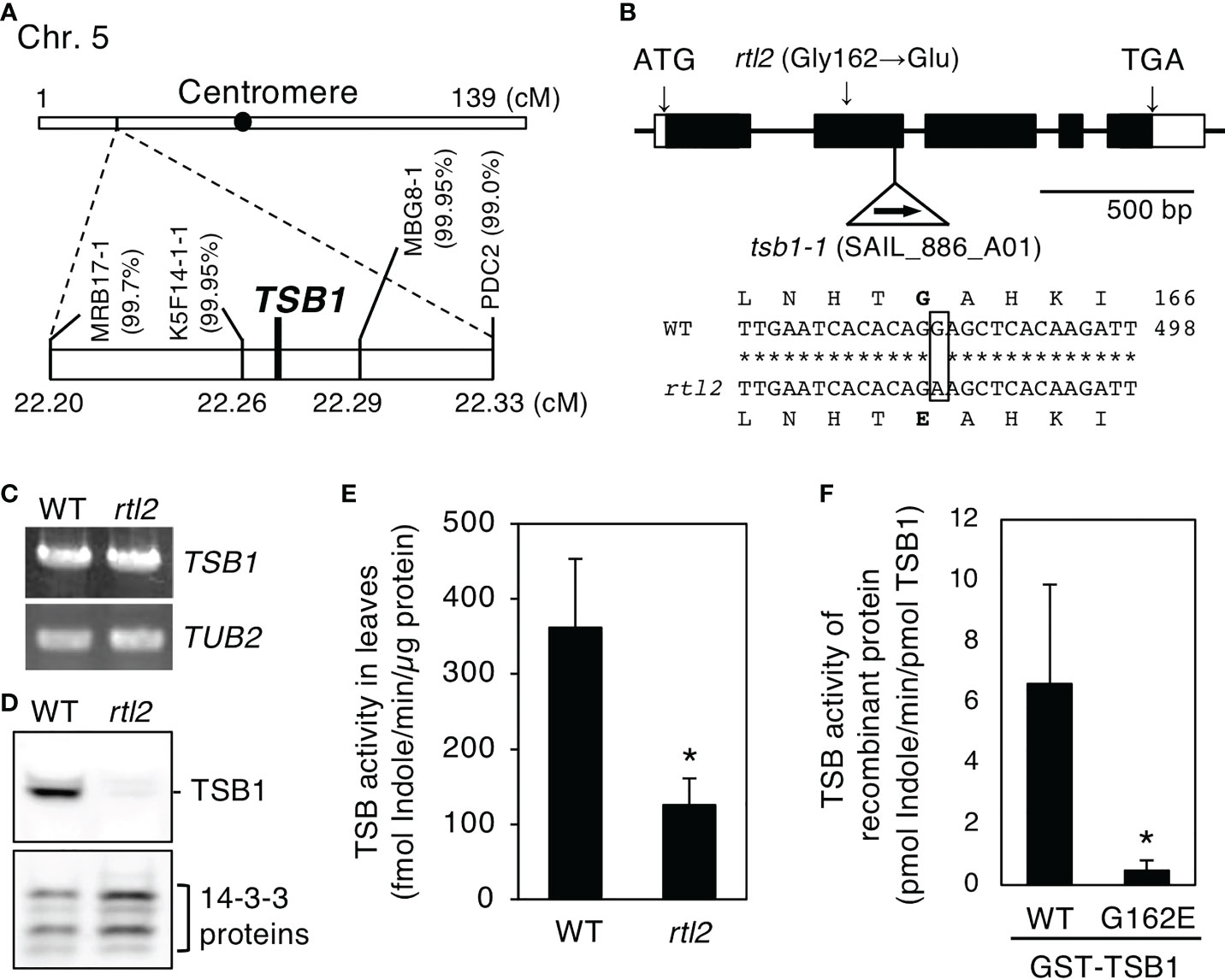
Figure 2 Determination of the mutation in the rtl2 mutant. (A) Mapping analysis of the RTL2 locus. Numbers in parentheses indicate percentages of no recombination in 1820 chromosomes. The RTL2 locus was close to CAPS marker K5F14-1-1, MBG8-1 and Tryptophan synthase ß subunit 1 (TSB1). (B) Schematic representation of the structure of the TSB1 gene and the position of T-DNA insertion in the tsb1-1 mutant (upper). Boxes and lines represent exons and introns respectively. The T-DNA insertion was located in 2nd exon of the TSB1 gene. The position of the amino acid substitution (Gly162 to Glu) in rtl2 is indicated. The partial sequences of TSB1 cDNA and the deduced amino acid in WT and rtl2 are shown (lower). A single nucleotide substitution (G485 to A) is shown by a box. Nucleotide and amino acid numbers are indicated in the right. Asterisks indicate the same nucleotide of the TSB1 gene in WT and rtl2. (C) TSB1 expression analyzed by RT-PCR in WT and rtl2. Total RNA was extracted from rosette leaves of 5-week-old plants grown on MS plate for 4 weeks and transferred to soil for 1 week. TUB2 was amplified as a control. PCRs were performed with 30 cycles for TSB1 and with 25 cycles for TUB2, respectively. (D) Immunoblot analysis of TSB1 protein in WT and rtl2. Twenty micrograms of protein extracted from rosette leaves of 5-week-old plants was loaded on each lane. The 14-3-3 proteins were detected using anti-14-3-3 antibody as a control. (E) TSB activity in rosette leaves of 5-week-old WT and rtl2. Values are means of three independent experiments with SDs. Asterisk indicates a significant difference in TSB activity relative to WT by Student t test (*P < 0.05). (F) TSB activity of recombinant TSB1 (WT) and TSB1-G162E. Values are means of three independent experiments with SDs. Asterisk indicates a significant difference in TSB activity relative to WT by Student t test (*P < 0.05).
To determine whether rtl2 is an allele of tsb1, a T-DNA insertion mutant tsb1-1 (SAIL_886_A01) was obtained from ABRC (Figure 2B). The tsb1-1 showed rtl2-like dwarf phenotype with pale green leaves and dark veins (Figure 3A). RT-PCR analysis revealed that tsb1-1 is a knockout mutant, which results in significant decrease of protein amount in rosette leaves of tsb1-1 (Figures 3B, C). Stomata of tsb1-1 also opened widely even in the dark and could not close in response to ABA, suggesting that TSB1 is responsible for the rtl2 phenotype (Figure 3D). To confirm this, we have tried to complement the WT TSB1 genome to rtl2, however, we could not obtain the transgenic rtl2 plants carrying the WT TSB1 gene under its own promoter, probably due to growth defect of rtl2 plants. Therefore, we performed test-cross of rtl2 and tsb1-1 to show that these are allelic gene. As shown in Figure 4A, F1-generation plants showed dwarf phenotype with pale green leaves and dark veins same as in rtl2 and tsb1-1, indicating that these are allelic gene. In addition, stomata of F1-generation plants widely opened under illuminated growth condition (Figure 4B). From these results, we concluded that TSB1 is responsible for the rtl2 phenotype.
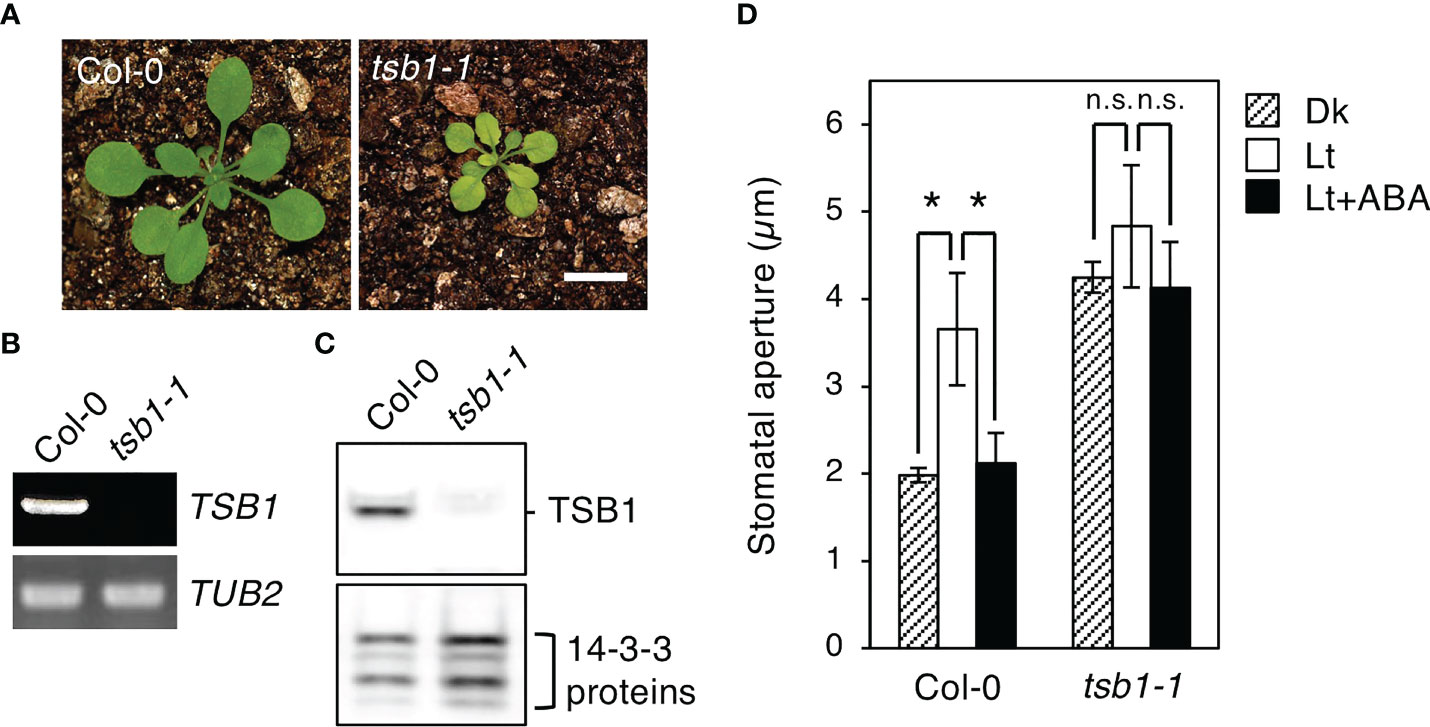
Figure 3 Phenotypic analysis of a TSB1 knockout mutant, tsb1-1. (A) Five-week-old Col-0 and tsb1-1 plants. Plants were grown on MS plate for 4 weeks and transferred to soil for 1 week. Scale bar = 1 cm. (B) TSB1 expression analyzed by RT-PCR in Col-0 and tsb1-1. Other details are the same as in Figure 2C. (C) Immunoblot analysis of TSB1 protein in Col-0 and tsb1-1. Other details are the same as in Figure 2D. (D) Stomatal aperture of Col-0 and tsb1-1 plants. Other details are the same as in Figure 1D. Values represent mean ± SD (n = 3); measurement of 35 stomata in each experiment. Asterisks indicate a significant difference by Student t test; *P < 0.05. n.s., not significant.
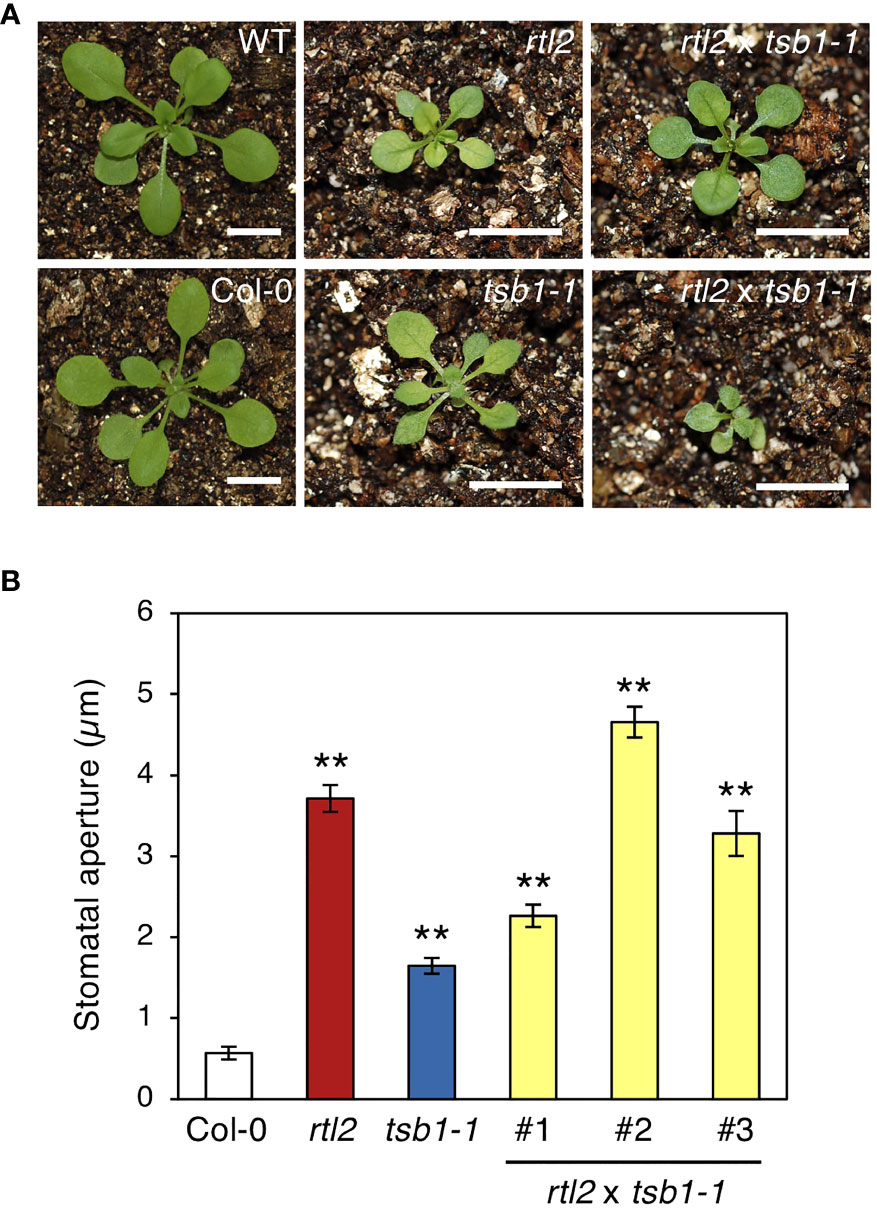
Figure 4 Phenotypes of test-crossed plants. (A) Five-week-old WT, Col-0, rtl2, tsb1-1 and test-crossed F1 plants (rtl2 x tsb1-1). Plants were grown on MS plate for 4 weeks and transferred to soil for 1 week. The scale bar represents 1 cm. (B) Stomatal aperture of 5-week-old Col-0, rtl2, tsb1-1 and test-crossed F1 plants (rtl2 x tsb1-1) under illuminated growth condition. Rosette leaves were detached from the plants at ZT4-7 and immediately subjected to measurement of stomatal aperture. Values are means of > 25 stomata with SDs. Asterisks indicate a significant statistical difference relative to Col-0 by Student t test (**P < 0.01).
Mutant of another tryptophan synthetic enzyme TSA1 also had open stomata phenotype
TSB1 encodes a predominantly expressed Trp synthase ß subunit in Trp biosynthetic pathway, in which Trp is synthesized from chorismate via six reaction steps as shown in Figure 5A (Radwanski and Last, 1995; Tzin and Galili, 2010). To investigate whether other Trp biosynthesis enzymes affect stomatal opening, we measured the stomatal aperture of the double mutant of AS, wei2-1 wei7-1, single substitution mutant of TSA1, trp3-1, and a T-DNA insertion knockout mutant of TSB1 paralog, TSB2 (tsb2-1; Supplementary Figure S2). Among the mutants, trp3-1 showed open stomata phenotype similar to tsb1-1, but not in wei2-1 wei7-1 and tsb2-1 (Figure 5B).
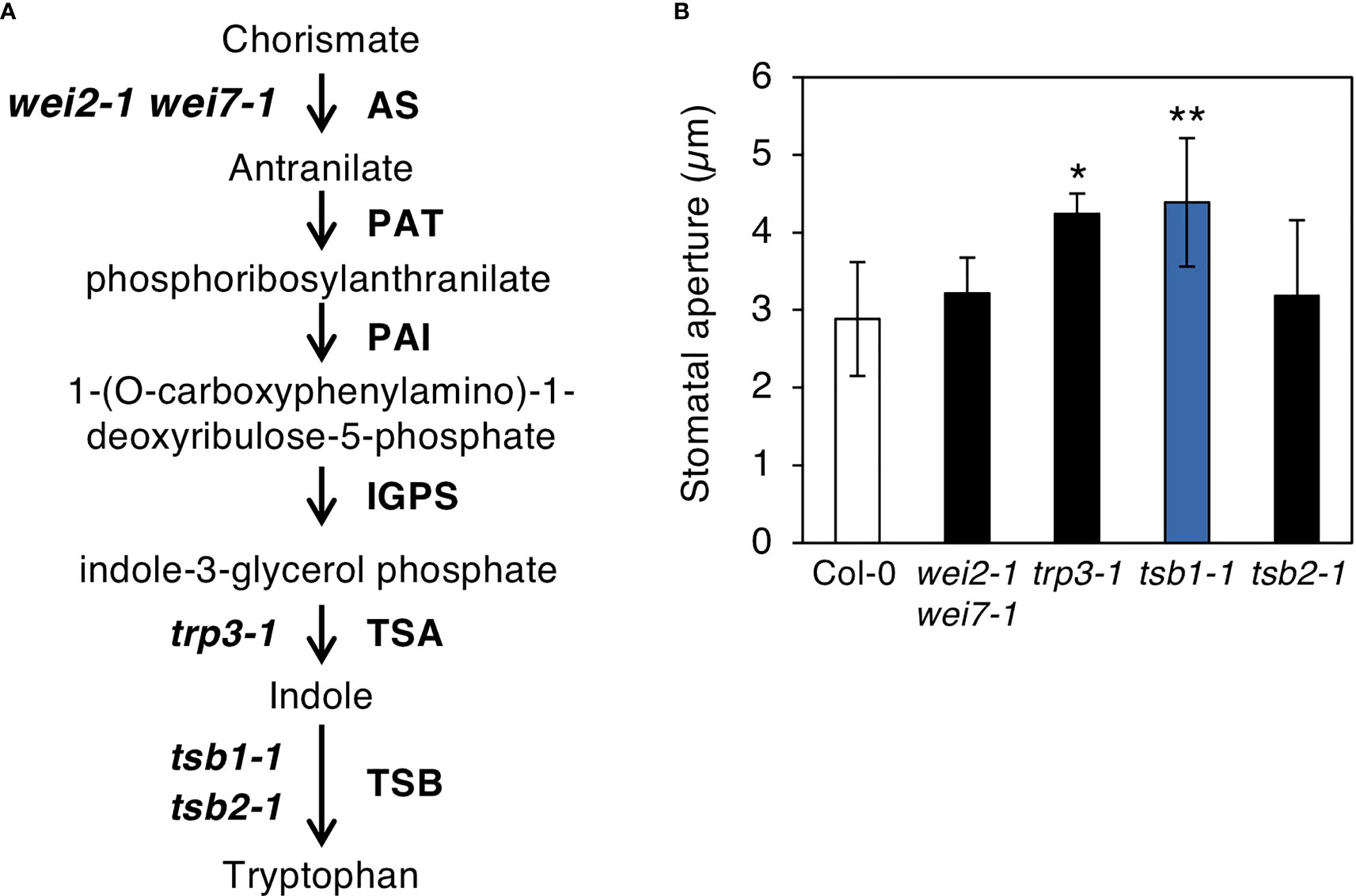
Figure 5 Characterization of the mutants related to Tryptophan biosynthetic pathway. (A) Tryptophan biosynthetic pathway in Arabidopsis thaliana. Tryptophan biosynthetic pathway starting from chorismate are shown with the enzymes of each step (right) and the mutants used in this study (left). AS, anthranilate synthase; PAT, anthranilate phosphoribosylanthranilate transferase; PAI, phosphorybosylanthranilate isomerase; IGPS, indole-3-glycerol phosphate synthase; TSA, tryptophan synthase α subunit; TSB, tryptophan synthase ß subunit. (B) Stomatal aperture of Tryptophan biosynthetic mutants under illuminated growth condition. Epidermal tissues were isolated from 5-week-old plants grown on MS plate for 4 weeks and transferred to soil for 1 week at ZT4-7 and immediately subjected to measurement of stomatal aperture. Values represent mean ± SD (n = 3); measurement of > 25 stomata in each experiment Asterisks indicate a significant statistical difference relative to Col-0 by Student t test (*P < 0.05, **P < 0.01).
Auxin treatment did not affect stomatal aperture
Indole-2-glycerol phosphate and Indole are substrates of TSA and TSB respectively. These are thought to be converted to indole-3-acetic-acid (IAA) via Trp-independent pathway (Ouyang et al., 2000). Jing et al. (2009) reported that free IAA levels in two tsb1 alleles, trp2-1 and trp2-8, were elevated and auxin responsive genes were up-regulated in these mutants. To clarify whether IAA contents in rtl2 is also elevated, we investigated the expression level of some auxin responsive genes, AUXIN UP REGULATED 3 (AUR3), SMALL AUXIN UPREGULATED RNA 9 (SAUR9), INDOLE-3-ACETIC ACID INDUCIBLE 1 (IAA1) and INDOLE-3-ACETIC ACID INDUCIBLE 24 (IAA24). As shown in Figure 6A, AUR3 was significantly up-regulated in both rtl2 and tsb1-1, though other auxin responsive genes were not. Given that up-regulation of AUR3 expression in tsb1 alleles, we speculated that open stomata phenotype in rtl2 is caused by elevated levels of auxin. To address the possibility, we first investigated the effect of auxin on stomatal aperture. Incubation of the epidermal tissues with 10 µM IAA in the dark for 3 hr had no effect on stomatal aperture (Figure 6B). Next, we investigated effect of auxins, IAA and synthetic auxins, 1-naphthylacetic acid (1-NAA) and 2,4-dichlorophenoxyacetic acid (2.4-D), on phosphorylation status of the penultimate residue, Thr, of PM H+-ATPase in guard cell protoplasts (GCPs) from Vicia faba, because auxin activates PM H+-ATPase via phosphorylation during auxin-induced hypocotyl elongation (Takahashi et al., 2012; Uchida et al., 2018). However, consistent with a recent report that IAA did not induce phosphorylation of PM H+-ATPase in guard cells of Arabidopsis thaliana (Akiyama et al., 2022), all auxins had no effect on phosphorylation status of PM H+-ATPase for 30 min, although a fungal toxin fusicoccin (FC) induced phosphorylation of PM H+-ATPase (Figure 6C). These results suggest that short term treatment of auxin (within 3 hr) has no effect on both stomatal aperture and phosphorylation status of PM H+-ATPase in guard cells. Furthermore, we found that auxin antagonist, auxinole (Hayashi et al., 2012), did not change the stomatal aperture in rtl2 and tsb1-1 (Figure 6D).
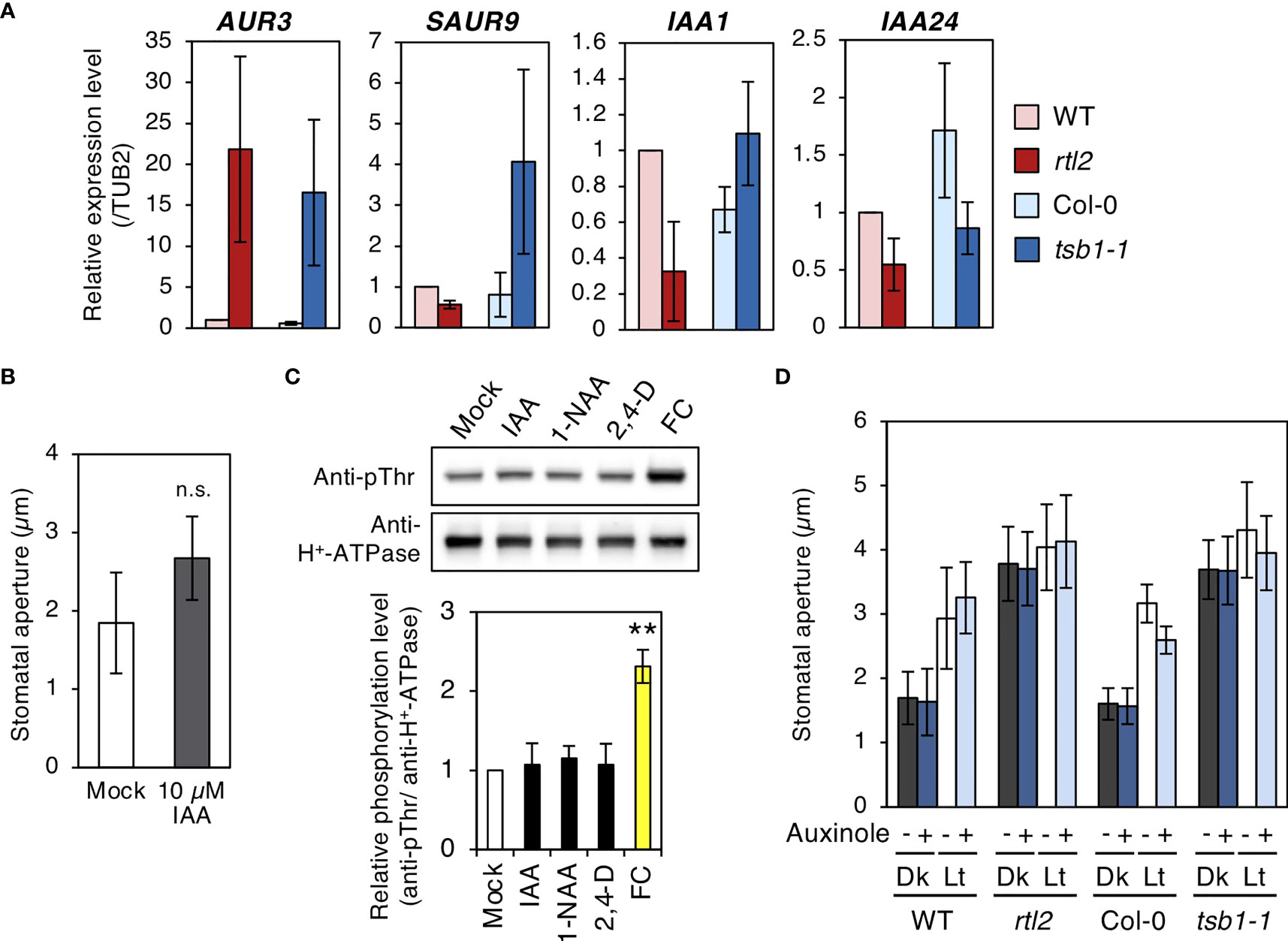
Figure 6 Characterizations of rtl2 and tsb1-1 mutants and auxin responses in stomata. (A) Expression of auxin responsive genes analyzed by qRT-PCR in WT, rtl2, Col-0 and tsb1-1. Total RNA was extracted from rosette leaves of 5-week-old plants grown on MS plate for 4 weeks and transferred to soil for 1 week. TUB2 was amplified as a control. (B) Effect of exogenous auxin on stomatal aperture in Col-0. Epidermal tissues from dark-adapted 4-week-old plants grown on soil were incubated with 0.25% (v/v) DMSO (Mock) and10 µM IAA in the dark for 3 hr. Values represent mean ± SD (n = 4); measurement of 45 stomata in each experiment n.s. not significant. (C) Effect of auxins to the phosphorylation status of the penultimate residue, Thr, of PM H+-ATPase in guard cell protoplasts (GCPs) from Vicia faba. GCPs were incubated with IAA and synthetic auxins (1-NAA, 2,4-D) and fusicoccin (FC) at 10 µM in the dark for 30 min. DMSO was used as a solvent control (Mock). Immunoblots of the phosphorylated PM H+-ATPase and total PM H+-ATPase were performed using anti-pThr antibody and anti-H+-ATPase antibody, respectively. The graph below the blot shows the relative phosphorylation level of PM H+-ATPase, defined as the ratio of the phosphorylated PM H+-ATPase to the total quantity of the protein, set as 1 for Mock. The bars represent the means ± SD for three independent experiments. Asterisks indicate a significant statistical difference relative to Col-0 by Student t test (**P < 0.01). (D) Effect of auxin antagonist, auxinole, on light-induced stomatal opening in WT, rtl2, Col-0 and tsb1-1. Plants were grown on MS plate for 4 weeks and transferred to soil for 1 week. Epidermal tissues from dark-adapted plants were incubated under light (Lt) or kept in the dark (Dk) in the presence or absence of auxinole for 2.5 hr. Other details are the same as in Figure 1D.
Exogenous Trp rescued the phenotypes of tsb1-1
To verify whether the open stomata phenotype in tsb1 mutants is caused by Trp deficiency, L-Tryptophan (L-Trp) was exogenously supplied to tsb1-1. We used only tsb1-1 mutant in this experiment due to less growth of rtl2 in hydroponic culture. Ten-day-old tsb1-1 seedlings grown on MS plate were transferred to hydroponic system supplemented with/without 0.25 mM L-Trp for 2-4 weeks. Similar to the previous report (Jing et al., 2009), dwarf and pale green phenotype in tsb1-1 were partially rescued by exogenous application of L-Trp (Figure 7A). Interestingly, tsb1-1 showed normal stomatal aperture by application of L-Trp (Figure 7B), suggesting that open stomata phenotype in tsb1-1 is due to Trp deficiency. On the other hand, under hydroponic culture condition, stomatal density in tsb1-1 did not show significant differences compared to that in Col-0 and L-Trp application did not change the stomatal density in both Col-0 and tsb1-1 (Supplementary Table S1). Therefore, Trp deficiency may have no effect on the stomatal density.
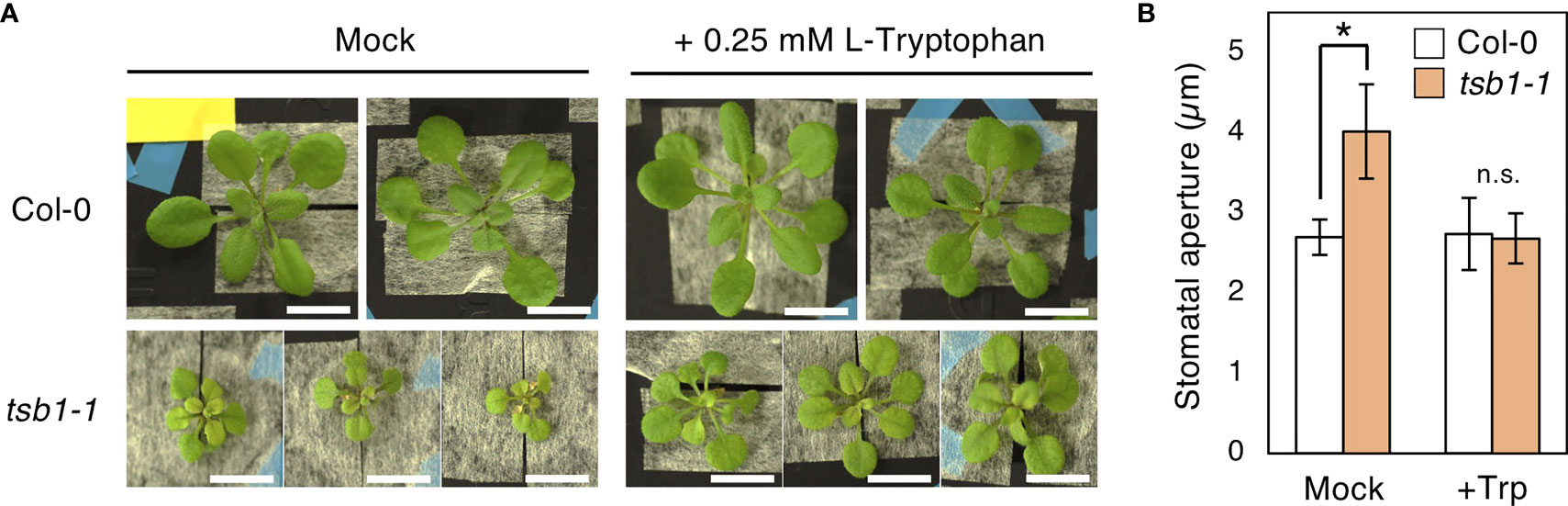
Figure 7 Effect of exogenous L-Trp on the phenotypes of tsb1-1. (A) Four-week-old Col-0 and tsb1-1 plants grown with or without L-Trp. Plants were grown on MS plate for 10days and transferred to hydroponic system with or without 0.25 mM L-Trp for 2 weeks. Scale bar = 1 cm. (B) Stomatal aperture of Col-0 and tsb1-1 plants grown with or without L-Trp under illuminated growth condition. 10-day-old seedlings germinated on MS plate were transferred to hydroponic system with or without 0.25 mM L-Trp, and were grown for a further 3 to 4 weeks. Epidermal tissues were isolated at ZT4-7 and immediately subjected to measurement of stomatal aperture. Values represent mean ± SD (n = 3); measurement of > 25 stomata in each experiment. Asterisk indicates a significant statistical difference relative to Col-0 by Student t test (*P < 0.05; n.s., not significant).
Discussion
In this study, we isolated rapid transpiration in detached leaves 2 (rtl2), that exhibited a higher rate of water loss via transpiration and clear low leaf temperature phenotype (Figures 1A, E). Detailed analysis of stomatal phenotypes of rtl2 revealed that constitutive open-stomata phenotype and higher stomatal density contribute rapid transpiration in rtl2 (Figure 1D, Table 1). Map-based analysis and test-cross with tsb1-1 revealed that Tryptophan synthase ß subunit 1 (TSB1) is responsible for RTL2 locus (Figures 2, 3, 4). The missense mutation of TSB1 Gly162 to Glu found in rtl2 resulted in significantly reduction of TSB activity (Figure 2F). Even though the transcript level of TSB1 in rtl2 were comparative to that in WT, amount of TSB1 protein in rtl2 was significantly reduced (Figures 2C, D). This could be due to active degradation of mutated TSB1 in rtl2. The residual TSB activity in rtl2, ~35% of WT, may come from TSB2, a paralog of TSB1 (Figure 2E).
Very recently, Liu et al. (2022) reported that amiR-TSB1 lines, which have reduced expression of TSB1 to 64-73% of wild-type plants, exhibited lower water loss rate from detached leaves and higher survival rate under salt and drought stress compared with wild-type plants. They also found that TSB1 interacts with and inhibits ß-glucosidase (BG1), catalyzing the conversion of glucose-conjugated ABA into active ABA. In consistent with this, the ABA contents in amiR-TSB1 lines were significantly higher than those in the wild-type. They concluded that TSB1 regulates stress tolerance and the accumulation of ABA via repression of BG1 activity. In contrast, we found that stomata in two tsb1 mutants, rtl2 and tsb1-1, significantly opened even in the dark condition and in the presence of ABA (Figures 1, 3). Thus, since the phenotype related to stomatal aperture is considered to be greatly different between amiR-TSB1 lines and rtl2 and tsb1-1, it is possible that amiR-TSB1 lines induce salt and drought resistance regardless of stomatal phenotype. Therefore, rtl2 and tsb1-1 may exhibit such salt and drought tolerance, it is necessary to investigate the tolerance in rtl2 and tsb1-1. As a point of concern, the amount of protein in amiR-TSB1 lines have decreased to 64-73%, while tsb1-1 used in this study is a complete knockout. In this study, we found that not only tsb1 mutants but also trp3-1, single substitution mutant of TSA1, exhibited open-stomata phenotype, whereas Liu et al. (2022) showed stress sensitivities in terms of chlorophyll content in trp3-1 was similar to those in wild type. In addition, TSA1 did not interact with BG1 in yeast cells. These results suggest that TSB1 may affect stomatal aperture as well as TSA1 without affecting BG1.
Why does the rtl2 mutant show an open-stomata phenotype compared to WT (Figure 1)? Jing et al. (2009) indicated that the trp2 mutants showed higher auxin contents in plants. Ouyang et al. (2000) found that IAA contents in TSA1 mutant, trp3-1, was also elevated and proposed Trp-independent IAA biosynthetic pathway, in which IAA is synthesized from IGP and indole. In this study, both trp3-1 and tsb1 mutants showed open-stomata phenotype (Figure 5). Stomatal phenotype in trp3-1 and tsb1 mutants showed correlation with IAA contents in these mutants. It has been demonstrated that auxin induces phosphorylation of the penultimate residue, Thr, of PM H+-ATPase and activation of PM H+-ATPase in the etiolated seedlings (Takahashi et al., 2012). To confirm the possibility that auxin mediates open-stomata phenotype in rtl2, we examined the effect of auxin on stomatal phenotypes. However, short-term treatment of auxin (within 3 hr) had no effect on stomatal aperture and phosphorylation status of PM H+-ATPase in guard cells (Figure 6B, C). Moreover, auxin antagonist auxinole did not affect the stomatal aperture in tsb1 mutants (Figure 6D). Further investigations will be needed to investigate effect of long-term treatment of auxin (e.g., for several days) on stomatal opening and the phosphorylation level of PM H+-ATPase in guard cells. Exogenous application of Trp to tsb1-1 resulted in suppression of open stomata phenotype in tsb1-1 (Figure 7B). Although molecular mechanisms are still unclear, but to our knowledge, this is the first report that Trp biosynthetic pathway has significant effect on stomatal aperture in plants.
Data availability statement
The original contributions presented in the study are included in the article/Supplementary Materials. Further inquiries can be directed to the corresponding author.
Author contributions
MNS, YH and TK designed the experiments. MNS, YH, KT and TK performed experiments. MNS, YH and TK wrote the manuscript. All authors contributed to the article and approved the submitted version.
Funding
This work was supported by Grants-in-Aid for Scientific Research from MEXT (nos. 20H05687 and 20H05910 to T.K.).
Acknowledgments
We are grateful to Mami Uchida (Nagoya University) for technical support.
Conflict of interest
The authors declare that the research was conducted in the absence of any commercial or financial relationships that could be construed as a potential conflict of interest.
Publisher’s note
All claims expressed in this article are solely those of the authors and do not necessarily represent those of their affiliated organizations, or those of the publisher, the editors and the reviewers. Any product that may be evaluated in this article, or claim that may be made by its manufacturer, is not guaranteed or endorsed by the publisher.
Supplementary material
The Supplementary Material for this article can be found online at: https://www.frontiersin.org/articles/10.3389/fpls.2022.1011360/full#supplementary-material
References
Akiyama, M., Sugimoto, H., Inoue, S. I., Takahashi, Y., Hayashi, M., Hayashi, Y., et al. (2022). Type 2C protein phosphatase clade D family members dephosphorylate guard cell plasma membrane H+-ATPase. Plant Physiol. 188, 2228–2240. doi: 10.1093/plphys/kiab571
Ando, E., Kinoshita, T. (2018). Red light-induced phosphorylation of plasma membrane H+-ATPase in stomatal guard cells. Plant Physiol. 178, 838–849. doi: 10.1104/pp.18.00544
Gibeaut, D. M., Hulett, J., Cramer, R., Seemann, J. R. (1997). Maximal biomass of arabidopsis fha/iana using a simple, low-maintenance hydroponic method and favorable environmental conditions. Plant Physiol. 115, 317–319. doi: 10.1104/pp.115.2.317
Greenberg, J. B., Galston, A. W. (1959). Tryptophan synthetase activity in pea seedling extracts. Plant Physiol. 34, 489–494. doi: 10.1104/pp.34.5.489
Hayashi, M., Inoue, S. I., Ueno, Y., Kinoshita, T. (2017). A raf-like protein kinase BHP mediates blue light-dependent stomatal opening. Sci. Rep. 7, 1–12. doi: 10.1038/srep45586
Hayashi, Y., Nakamura, S., Takemiya, A., Takahashi, Y., Shimazaki, K. I., Kinoshita, T. (2010). Biochemical characterization of in vitro phosphorylation and dephosphorylation of the plasma membrane H+-ATPase. Plant Cell Physiol. 51, 1186–1196. doi: 10.1093/pcp/pcq078
Hayashi, K. I., Neve, J., Hirose, M., Kuboki, A., Shimada, Y., Kepinski, S., et al. (2012). Rational design of an auxin antagonist of the SCF TIR1 auxin receptor complex. ACS Chem. Biol. 7, 590–598. doi: 10.1021/cb200404c
Hayashi, Y., Takahashi, Y., Fukatsu, K., Tada, Y., Takahashi, K., Kuwata, K., et al. (2021). Identification of abscisic acid-dependent phosphorylated basic helix-Loop-Helix transcription factors in guard cells of Vicia faba by mass spectrometry. Front. Plant Sci. 12. doi: 10.3389/fpls.2021.735271
Inoue, S. I., Kinoshita, T. (2017). Blue light regulation of stomatal opening and the plasma membrane H+-ATPase. Plant Physiol. 174, 531–538. doi: 10.1104/pp.17.00166
Inoue, S. I., Kinoshita, T., Matsumoto, M., Nakayama, K. I., Doi, M., Shimazaki, K. I. (2008). Blue light-induced autophosphorylation of phototropin is a primary step for signaling. Proc. Natl. Acad. Sci. U. S. A. 105, 5626–5631. doi: 10.1073/pnas.0709189105
Jing, Y., Cui, D., Bao, F., Hu, Z., Qin, Z., Hu, Y. (2009). Tryptophan deficiency affects organ growth by retarding cell expansion in arabidopsis. Plant J. 57, 511–521. doi: 10.1111/j.1365-313X.2008.03706.x
Kinoshita, T., Doi, M., Suetsugu, N., Kagawa, T., Wada, M., Shimazaki, K. (2001). phot1 and phot2 mediate blue light regulation of stomatal opening. Nature 414, 656–660. doi: 10.1038/414656a
Kinoshita, T., Ono, N., Hayashi, Y., Morimoto, S., Nakamura, S., Soda, M., et al. (2011). FLOWERING LOCUS T regulates stomatal opening. Curr. Biol. 21, 1232–1238. doi: 10.1016/j.cub.2011.06.025
Kinoshita, T., Shimazaki, K. I. (1999). Blue light activates the plasma membrane H+-ATPase by phosphorylation of the C-terminus in stomatal guard cells. EMBO J. 18, 5548–5558. doi: 10.1093/emboj/18.20.5548
Last, R. L., Bissinger, P. H., Mahoney, D. J., Radwanski, E. R., Fink, G. R. (1991). Tryptophan mutants in arabidopsis: The consequences of duplicated tryptophan synthase β genes. Plant Cell 3, 345–358. doi: 10.1105/tpc.3.4.345
Liu, W.-C., Song, R.-F., Zheng, S.-Q., Li, T.-T., Zhang, B.-L., Gao, X., et al. (2022). Coordination of plant growth and abiotic stress responses by tryptophan synthase β subunit 1 through modulation of tryptophan and ABA homeostasis in arabidopsis. Mol. Plant 15, 973–990. doi: 10.1016/j.molp.2022.04.009
Mashiguchi, K., Tanaka, K., Sakai, T., Sugawara, S., Kawaide, H., Natsume, M., et al. (2011). The main auxin biosynthesis pathway in arabidopsis. Proc. Natl. Acad. Sci. U. S. A. 108, 18512–18517. doi: 10.1073/pnas.1108434108
Munemasa, S., Hauser, F., Park, J., Waadt, R., Brandt, B., Schroeder, J. I. (2015). Mechanisms of abscisic acid-mediated control of stomatal aperture. Curr. Opin. Plant Biol. 28, 154–162. doi: 10.1016/j.pbi.2015.10.010
Ouyang, J., Shao, X., Li, J. (2000). Indole-3-glycerol phosphate, a branchpoint of indole-3-acetic acid biosynthesis from the tryptophan biosynthetic pathway in Arabidopsis thaliana. Plant J. 24, 327–333. doi: 10.1046/j.1365-313X.2000.00883.x
Radwanski, E. R., Barczak, A. J., Last, R. L. (1996). Characterization of tryptophan synthase alpha subunit mutants of Arabidopsis thaliana. Mol. Gen. Genet. 253, 353–361. doi: 10.1007/s004380050331
Radwanski, E. R., Last, R. L. (1995). Tryptophan biosynthesis and metabolism: Biochemical and molecular genetics. Plant Cell 7, 921–934. doi: 10.2307/3870047
Schroeder, J. I., Allen, G. J., Hugouvieux, V., Kwak, J. M., Waner, D. (2001). Guard cell signal transduction. Annu. Rev. Plant Biol. 52, 627–658. doi: 10.1146/annurev.arplant.52.1.627
Schroeder, J. I., Raschke, K., Neher, E. (1987). Voltage dependence of k+ channels in guard-cell protoplasts. Proc. Natl. Acad. Sci. U. S. A. 84, 4108–4112. doi: 10.1073/pnas.84.12.4108
Shimazaki, K. I., Doi, M., Assmann, S. M., Kinoshita, T. (2007). Light regulation of stomatal movement. Annu. Rev. Plant Biol. 58, 219–247. doi: 10.1146/annurev.arplant.57.032905.105434
Stepanova, A. N., Hoyt, J. M., Hamilton, A. A., Alonso, J. M. (2005). A link between ethylene and auxin uncovered by the characterization of two root-specific ethylene-insensitive mutants in arabidopsis. Plant Cell 17, 2230–2242. doi: 10.1105/tpc.105.033365
Stepanova, A. N., Robertson-Hoyt, J., Yun, J., Benavente, L. M., Xie, D. Y., Doležal, K., et al. (2008). TAA1-mediated auxin biosynthesis is essential for hormone crosstalk and plant development. Cell 133, 177–191. doi: 10.1016/j.cell.2008.01.047
Takahashi, K., Hayashi, K. I., Kinoshita, T. (2012). Auxin activates the plasma membrane H+-ATPase by phosphorylation during hypocotyl elongation in arabidopsis. Plant Physiol. 159, 632–641. doi: 10.1104/pp.112.196428
Takemiya, A., Kinoshita, T., Asanuma, M., Shimazaki, K. I. (2006). Protein phosphatase 1 positively regulates stomatal opening in response to blue light in Vicia faba. Proc. Natl. Acad. Sci. U. S. A. 103, 13549–13554. doi: 10.1073/pnas.0602503103
Takemiya, A., Sugiyama, N., Fujimoto, H., Tsutsumi, T., Yamauchi, S., Hiyama, A., et al. (2013). Phosphorylation of BLUS1 kinase by phototropins is a primary step in stomatal opening. Nat. Commun. 4, 2094. doi: 10.1038/ncomms3094
Tao, Y., Ferrer, J.-L., Ljung, K., Pojer, F., Hong, F., Long, J. A., et al. (2008). Rapid synthesis of auxin via a new tryptophan-dependent pathway is required for shade avoidance in plants. Cell 133, 164–176. doi: 10.1016/j.cell.2008.01.049
Tivendale, N. D., Ross, J. J., Cohen, J. D. (2014). The shifting paradigms of auxin biosynthesis. Trends Plant Sci. 19, 44–51. doi: 10.1016/j.tplants.2013.09.012
Toh, S., Inoue, S., Toda, Y., Yuki, T., Suzuki, K., Hamamoto, S., et al. (2018). Identification and characterization of compounds that affect stomatal movements. Plant Cell Physiol. 59, 1568–1580. doi: 10.1093/pcp/pcy061
Toh, S., Takata, N., Ando, E., Toda, Y., Wang, Y., Hayashi, Y., et al. (2021). Overexpression of plasma membrane H+-ATPase in guard cells enhances light-induced stomatal opening, photosynthesis, and plant growth in hybrid aspen. Front. Plant Sci. 12. doi: 10.3389/fpls.2021.766037
Tsuzuki, T., Takahashi, K., Inoue, S.i., Okigaki, Y., Tomiyama, M., Hossain, M. A., et al. (2011). Mg-chelatase H subunit affects ABA signaling in stomatal guard cells, but is not an ABA receptor in Arabidopsis thaliana. J. Plant Res. 124, 527–538. doi: 10.1007/s10265-011-0426-x
Tzin, V., Galili, G. (2010). The biosynthetic pathways for shikimate and aromatic amino acids in Arabidopsis thaliana. Arab. B. 8, e0132. doi: 10.1199/tab.0132
Uchida, N., Takahashi, K., Iwasaki, R., Yamada, R., Yoshimura, M., Endo, T. A., et al. (2018). Chemical hijacking of auxin signaling with an engineered auxin-TIR1 pair. Nat. Chem. Biol. 14, 299–305. doi: 10.1038/nchembio.2555
Ursache, R., Miyashima, S., Chen, Q., Vatén, A., Nakajima, K., Carlsbecker, A., et al. (2014). Tryptophan-dependent auxin biosynthesis is required for HD-ZIP III-mediated xylem patterning. Dev. 141, 1250–1259. doi: 10.1242/dev.103473
Wang, B., Chu, J., Yu, T., Xu, Q., Sun, X., Yuan, J., et al. (2015). Tryptophan-independent auxin biosynthesis contributes to early embryogenesis in arabidopsis. Proc. Natl. Acad. Sci. U. S. A. 112, 4821–4826. doi: 10.1073/pnas.1503998112
Wang, Y., Noguchi, K., Ono, N., Inoue, S. I., Terashima, I., Kinoshita, T. (2014). Overexpression of plasma membrane H+-ATPase in guard cells promotes light-induced stomatal opening and enhances plant growth. Proc. Natl. Acad. Sci. U. S. A. 111, 533–538. doi: 10.1073/pnas.1305438111
Wang, Y., Noguchi, K., Terashima, I. (2011). Photosynthesis-dependent and -independent responses of stomata to blue, red and green monochromatic light: Differences between the normally oriented and inverted leaves of sunflower. Plant Cell Physiol. 52, 479–489. doi: 10.1093/pcp/pcr005
Ye, W., Munemasa, S., Shinya, T., Wu, W., Ma, T., Lu, J., et al. (2020). Stomatal immunity against fungal invasion comprises not only chitin-induced stomatal closure but also chitosan-induced guard cell death. Proc. Natl. Acad. Sci. U. S. A. 117, 20932–20942. doi: 10.1073/pnas.1922319117
Zhang, M., Wang, Y., Chen, X., Xu, F., Ding, M., Ye, W., et al. (2021). Plasma membrane H+-ATPase overexpression increases rice yield via simultaneous enhancement of nutrient uptake and photosynthesis. Nat. Commun. 12. doi: 10.1038/s41467-021-20964-4
Keywords: stomata, guard cell, water loss, tryptophan biosynthetic pathway, tryptophan synthase ß subunit 1, PM H+-ATPase
Citation: Soda MN, Hayashi Y, Takahashi K and Kinoshita T (2022) Tryptophan synthase ß subunit 1 affects stomatal phenotypes in Arabidopsis thaliana. Front. Plant Sci. 13:1011360. doi: 10.3389/fpls.2022.1011360
Received: 04 August 2022; Accepted: 11 November 2022;
Published: 28 November 2022.
Edited by:
Valya N. Vassileva, Bulgarian Academy of Sciences, BulgariaReviewed by:
Denise Scuffi, Consejo Nacional de Investigaciones Científicas y Técnicas, ArgentinaTakayuki Shimizu, The University of Tokyo, Japan
Copyright © 2022 Soda, Hayashi, Takahashi and Kinoshita. This is an open-access article distributed under the terms of the Creative Commons Attribution License (CC BY). The use, distribution or reproduction in other forums is permitted, provided the original author(s) and the copyright owner(s) are credited and that the original publication in this journal is cited, in accordance with accepted academic practice. No use, distribution or reproduction is permitted which does not comply with these terms.
*Correspondence: Toshinori Kinoshita, a2lub3NoaXRhQGJpby5uYWdveWEtdS5hYy5qcA==
†These authors share first authorship