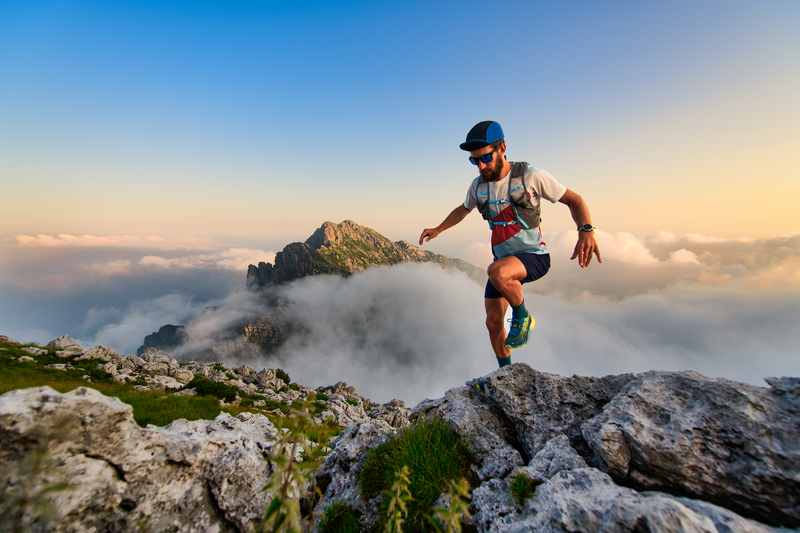
94% of researchers rate our articles as excellent or good
Learn more about the work of our research integrity team to safeguard the quality of each article we publish.
Find out more
ORIGINAL RESEARCH article
Front. Plant Sci. , 29 September 2022
Sec. Plant Biotechnology
Volume 13 - 2022 | https://doi.org/10.3389/fpls.2022.1011245
Eucalyptus, as an economically important species for wood and paper industries, still remains a challenge to genetic improvement by transgenic technology owing to the deficiency of a highly efficient and stable genetic transformation system, especially in cultivated superior clones. Eucalyptus urophylla × Eucalyptus grandis clone DH32-29 is most widely planted in southern China, but it is relatively recalcitrant to adventitious bud regeneration, which blocks the establishment of a genetic transformation system. Here, an efficient adventitious bud regeneration and transformation system of Eucalyptus was established using E. urophylla × E. grandis DH32-29 as material. The in vitro leaves from microshoots that were subcultured for 20–25 days were immersed into liquid Woody Plant Medium supplemented with 0.02 mg·L−1 of α-naphthaleneacetic acid (NAA) and 0.24 mg·L−1 of forchlorfenuron (callus-inducing medium (CIM)). After 15 days, explants were transferred to a medium containing 0.10 mg·L−1 of NAA and 0.50 mg·L−1 of 6-benzyladenine (shoot-inducing medium (SIM)) for adventitious bud induction. The highest regeneration efficiency of adventitious buds was 76.5%. Therefore, an Agrobacterium tumefaciens-mediated genetic transformation system was optimized. The leaves were precultured for 7 days and infected for 30 min with A. tumefaciens strain EHA105 grown to a bacterial density of 0.3 (OD600). After 72 h of cocultivation in the dark, leaves were transferred to CIM supplemented with 100 mg·L−1 of cefotaxime (Cef), 100 mg·L−1 of timentin, and 15 mg·L−1 of kanamycin (Kan) for 15 days to induce calluses. Then, the explants were transferred to SIM supplemented with the same concentration of antibiotics, and the fresh medium was replaced every 15 days until resistant adventitious buds appeared. After induction of roots in root-inducing medium supplemented with 200 mg·L−1 of Cef and 75 mg·L−1 of Kan, completely transgenic plants were obtained. With the use of the aforementioned method, the transformation frequency can reach 1.9%. This provides a powerful approach for genetic improvement of E. urophylla × E. grandis DH32-29 and gene function analysis in Eucalyptus.
Eucalyptus, an important multipurpose woody plant, originated in Australia and includes Eucalyptus, Corymbia, and Angophora, which are members of the Myrtaceae family (Hiwale, 2015). There are more than 700 species of Eucalyptus, and some are widely distributed in over 30 countries around the world and account for 16% of forest plantation areas (Teulieres and Marque, 2007). The Eucalyptus species is widely used in paper pulp, furniture, plywood, fuelwood, and essential oil due to its desirable characteristics. Due to its high economic reward, Eucalyptus has attracted the attention of forest workers, and the plantation area covers approximately 20 million hectares after many years of introduction and afforestation (Girijashankar, 2011). In China, for example, the area planted in Eucalyptus was 5.47 million hectares according to the ninth National Forest Inventory (2014–2018) (National Forestry and Grassland Administration, 2019).
With the increase in single clone plantations of Eucalyptus, an incidence of pests occurred (Ji et al., 2011), such as Leptocybe invasa (Luo et al., 2014), Ophelimus bipolaris (Chen et al., 2021), and Buzura suppressaria, and led to growth reduction and tree death and caused serious yield reduction. In addition, the use of herbicides to prevent weed growth during the planting of young Eucalyptus forests inevitably affected the growth of trees, time wasted in replanting, and increased input costs.
Transgenic technology is a powerful tool to accelerate the genetic improvement of trees and solve the problems that most forest trees are facing, such as improvement in productivity, wood quality, and stress resistance (Chang et al., 2018), especially pest and herbicide intolerance in Eucalyptus. Transgenic technology can introduce target genes of specific traits into an ideal genotype and pass through the barriers of species and genera, which is a large obstacle in traditional breeding methods. A stable and efficient genetic transformation system is a prerequisite for obtaining transgenic plants (Song et al., 2019). Many efforts have been made to establish genetic transformation systems in some forest trees (Chang et al., 2018), most frequently Populus, and including Eucalyptus (Prakash and Gurumurthi, 2009; Aggarwal et al., 2011; Silva et al., 2011; de Alcantara et al., 2011; Ahad et al., 2014; de França Bettencourt et al., 2020). Some attempts were made to introduce functional genes into Eucalyptus to obtain desirable phenotypes, which made it possible to cultivate new varieties by transgenic technology (Harcourt et al., 2000; Valério et al., 2003; Dibax et al., 2010; Navarro et al., 2011; Matsunaga et al., 2012; Ouyang et al., 2012; Yu et al., 2013; Aggarwal et al., 2015; Ouyang and Li, 2016; Thanananta et al., 2018).
Unfortunately, most protocols use cotyledons or hypocotyls derived from seedlings with characteristics different from those of explants, which are not suitable for further transgenic breeding and gene function analysis because of gene recombination (Pena and Seguin, 2001). Hence, it is necessary to develop an efficient genetic transformation system by using clonal materials as explants. Although genetic transformation systems were constructed for some Eucalyptus, there were still some superior clones that were widely cultivated that are recalcitrant to regeneration for unknown reasons.
DH32-29, a hybrid of Eucalyptus urophylla and Eucalyptus grandis that was cultivated by the Dongmen forest farm in Guangxi, China, is the most widely planted superior clone in southern China because of its desirable traits, such as fast growth, high yield, short rotation period, straight trunk, high-quality wood, strong resistance, and wide adaptability. However, this clone has not overcome the difficulty of regeneration, which blocks the establishment of a genetic transformation system. In this research, the difficulties in regenerating E. urophylla × E. grandis DH32-29 were solved by adjusting various plant growth regulators and methods. Finally, a stable and high-frequency adventitious bud regeneration system of E. urophylla × E. grandis DH32-29 was established using leaves obtained from micropropagated plantlets as explants. An Agrobacterium-mediated genetic transformation system was optimized, and transgenic plants were obtained, which made foreign genes stably integrated into the plant genome. This study will pave the way for functional analysis of genes related to superior traits and the breeding of transgenic and gene-edited high-quality varieties in the foreseeable future.
Shoots were cut from E. urophylla × E. grandis clone DH32-29 and were transferred to modified Murashige and Skoog (Murashige and Skoog, 1962) medium supplemented with 0.10 mg·L−1 of α-naphthaleneacetic acid (NAA) and 0.50 mg·L−1 of 6-benzyladenine (6-BA) (shoot-inducing medium (SIM)) after sterilization to obtain tissue culture plantlets. The medium was replaced every 20 days to propagate the sterile plantlets.
Plantlets were cut and transferred to 1/2 MS medium supplemented with 0.10 mg·L−1 of NAA (root-inducing medium (RIM)) to induce adventitious roots. Then, plantlets with roots were transferred to SIM and cultured in darkness for 7 days and in weak light for 14 days to gain elongated stem internodes. Stem internodes from plantlets and leaves from micropropagation plantlets were used as explants.
All media contained 30 g·L−1 of sucrose and 7 g·L−1 of agar, and the pH of all media was adjusted to 5.8 before autoclaving. All plantlets were cultured under a 16-h photoperiod (100 μmol·m−2·s−1) at 25°C ± 2°C.
To obtain a higher frequency of adventitious bud induction, the combinations of plant growth regulators (PGRs) supplemented in callus-inducing medium (CIM; Woody Plant Medium (WPM) basal medium supplemented with different PGRs) were optimized. Stem internodes from rooted plantlets were cultured in CIM supplemented with NAA (0.02–0.60 mg·L−1), forchlorfenuron (CPPU; 0.06–0.72 mg·L−1), and thidiazuron (TDZ; 0.06–0.24 mg·L−1) (Table 1) for 15 days in darkness. Then, the stem internodes were transferred to SIM to induce adventitious buds. Based on the best combination of PGRs, leaves obtained from micropropagation plantlets were used as explants to explore the effects of callus induction time (5, 10, 15, and 20 days) on adventitious bud regeneration. The duration of subculture (15, 20, and 25 days) and different volumes of added coconut milk in CIM were explored to improve the frequency of adventitious bud induction. Then, individual buds of 3 cm were cut off and transferred to RIM to induce adventitious roots.
Table 1 Effects of plant growth regulators for regeneration of Eucalyptus urophylla × Eucalyptus grandis DH32-29.
The growth parameters were observed daily, and the frequencies of callus and adventitious bud induction were calculated to determine the best regeneration protocol after 2 months of in vitro culture. The size of the callus was also calculated. Each treatment involved 120 explants and was performed in at least three replicates.
Leaves were successively cultured in CIM and SIM supplemented with different concentrations and types of antibiotics, including cefotaxime (Cef) and timentin (Tmt). Buds were inoculated in RIM with the same treatments to effectively eliminate redundant Agrobacterium without affecting regeneration. Different concentrations of kanamycin (Kan; 0–75 mg·L−1) or hygromycin (Hyg; 0–5 mg·L−1) were supplemented in CIM, SIM, and RIM to determine the critical concentration of antibiotics and prevent untransformed plants from escaping. Fresh media were replaced every 15 days to avoid antibiotic failure. The growth parameters were observed daily. The frequencies of callus and adventitious bud induction and the size of callus were calculated after leaves were cultured for 8 weeks. The frequency of adventitious root induction and the length of roots were calculated after 4 weeks. Each treatment involved 120 explants and was performed in at least three replicates.
Agrobacterium tumefaciens strains GV3101, LBA4404, EHA105, and AGL1 were used for the genetic transformation. The binary vector pBI121 contains a neomycin phosphotransferase Ⅱ (nptⅡ) selection marker gene and a β-glucuronidase (uidA) reporter gene. A single colony of Agrobacterium strain that contained pBI121 was cultured in 5 ml of liquid Luria–Bertani (LB) medium with 50 mg·L−1 of Kan and 20 mg·L−1 of rifampicin (Rif) for 48 h at 28°C with shaking at 220 rpm. A 1-ml cloudy culture was cultivated in 30 ml of liquid LB medium with the same antibiotics at 28°C until the OD600 was approximately 0.5. The bacterial cells were collected by centrifugation for 10 min at 4,000 rpm and 4°C, and the precipitated cells were resuspended in 30 ml of CIM liquid medium without antibiotics.
Six factors affecting the efficiency of genetic transformation were successively optimized, including Agrobacterium strain, concentration, duration of infection, duration of cocultivation, duration of precultivation, and concentration of the additive acetosyringone (AS). Leaves were cut from micropropagation plantlets that were subcultured for 20–25 days, and the lower half of leaves with petioles were adopted as explants. After 0–9 days of preculture in CIM in darkness, leaves were immersed in cell suspensions of different densities (OD600 = 0.1, 0.3, 0.5, 0.7, and 0.9) and with different AS concentrations (0, 10, 50, 100, and 200 μM) for varying periods of time (15, 30, 60, 120, and 240 min). Subsequently, explants were placed in CIM without antibiotics and cocultured for different hours (24, 28, and 72 h) at 25°C ± 2°C in darkness. After cocultivation, leaves were washed with sterile water and blotted with sterile filter paper to decontaminate Agrobacteria. The inoculated leaves were then successively transferred to CIM supplemented with the aforementioned selected antibiotics. After 7 days, β-glucuronidase (GUS) staining was performed.
Based on the optimal genetic transformation system, leaves were transferred to CIM containing antibiotics and cultured for 15 days to induce callus formation. Then, the leaves were replaced by SIM supplemented with antibiotics to obtain resistant adventitious buds. The fresh SIM was replaced every 15 days until resistant adventitious buds regenerated. Regenerated adventitious buds with 2 cm were excised and transferred to RIM supplemented with 200 mg·L−1 of Cef and 75 mg·L−1 of Kan for adventitious root induction. Each treatment involved 120 explants and was performed in at least three replicates.
Leaves transformed with Agrobacterium and putatively transgenic plants were detected by GUS histochemical staining as previously described (Jefferson et al., 1987). The plant materials were immersed in a reagent with 2 mM of 5-bromo-4-chloro-3-indolyl-β-D-glucuronide (X-Gluc), 0.5 mM of potassium ferrocyanide, 0.5 mM of potassium ferricyanide, 0.1 M of Na-phosphate buffer (pH = 7.0), and 0.1% (v/v) Triton X-100 and were incubated at 37°C for 12 h. Then, the staining solution was replaced with 70% ethanol, and the plant materials were washed four to six times to completely remove the chlorophyll.
Genomic DNA was isolated from the leaves of wild-type and putatively transgenic plants using a modified cetyltrimethylammonium bromide (CTAB) method. nptⅡ gene and uidA gene were amplified by polymerase chain reaction (PCR) amplification using specific primers (nptⅡ-F: TTGAACAAGATGGATTGCACGCA, nptⅡ-R: GAGCGGCGATACCGTAAAGCA, uidA-F: ACTCAGCAAGCGCACTTACAGG, uidA-R: TCCATACCTGTTCACCGACGAC). The 2 × Taq PCR StarMix (Genstar, Fuzhou, Cangshan, China) was used according to the manufacturer’s instructions. The PCR program was as follows: initial denaturing at 94°C for 5 min, followed by 35 cycles at 94°C for 30 s, 58°C (nptII) or 56°C (uidA) for 30 s, 72°C for 45 s (nptII) or 30 s (uidA), and a final extension for 7 min at 72°C.
All experiments were performed in triplicate with 120 explants per treatment. The number of explants that regenerated calluses, adventitious buds, and stained blue were recorded to calculate the frequency of adventitious bud regeneration and genetic transformation. The size of the callus was calculated as follows: explant with no callus was denoted as 0, leaf with callus but little enlargement was denoted as 1, leaf with callus and enlargement 1.5 times the original volume was denoted as 2, leaf with callus and enlargement 2 times the original volume was denoted as 3, and leaf with callus and enlargement 3 times the original volume was denoted as 4. SPSS 26 was used for all data analyses. Data were transformed by the following formula and analyzed using one-way analysis of variance (ANOVA) followed by Duncan’s multiple range test. The different letters in the graphs indicate significant differences among treatments (p < 0.05).
To establish an efficient adventitious bud regeneration system of E. urophylla × E. grandis DH32-29, different hormone combinations containing PGRs (including NAA, CPPU, and TDZ) were adopted based on the preliminary experiments (data not shown) and previous studies (Table 1). However, the adventitious bud induction rate was low regardless of which hormone combination treatment with stem internodes as explants (Table 1). Among these adventitious bud induction rates, the highest adventitious bud regeneration rate was obtained when 0.02 mg·L−1 of NAA was combined with 0.24 mg·L−1 of CPPU (Table 1). The frequency of adventitious bud induction increased from 17.2% to 61.4% when stems were replaced by leaves and cultured in liquid CIM for 15 days (Figure 1), indicating that leaves were more suitable as explants for further genetic transformation. The induction of calli is very important for adventitious bud regeneration. A duration of callus induction that was too short (5–10 days) or too long (20 days) was not advantageous for adventitious bud induction (Figure 1). The highest frequency of adventitious bud induction (61.4%) was obtained when leaves were induced to form calluses for 15 days (Figure 1), and the number of adventitious buds regenerated from each explant was more than 5.
Figure 1 Effects of different durations of callus induction on adventitious bud induction of Eucalyptus urophylla × Eucalyptus grandis DH32-29. (A) Explants are induced to form calli for 5, 10, 15, and 20 days. Effects of different durations of callus induction on (B) the frequency of callus induction, (C) callus size, and (D) the frequency of adventitious bud induction. Scale bar: 1 cm. Different letters indicate significant differences among treatments using Duncan’s multiple range test at p < 0.05.
The effects of the condition of leaves on adventitious bud regeneration were tested. Subculture times (15, 20, and 25 days) of plantlets had no influence on adventitious bud regeneration (Supplementary Figure 1). Leaves from plantlets subcultured for 20–25 days were used as explants because of the proper size for operation. Furthermore, different proportions of coconut milk and WPM were used to improve the frequency of adventitious bud induction. However, the color of the explants changed to yellow-green with the increase in the volume of added coconut milk, and the frequency of adventitious bud induction gradually decreased (Supplementary Figure 2). When the WPM medium was replaced by 100% coconut milk, the adventitious bud induction rate decreased to 21.5% (Supplementary Figure 2D). Thus, coconut milk was not required in the medium to induce callus formation.
The complete regeneration process of E. urophylla × E. grandis DH32-29 is shown in Figure 2. Leaves from plantlets subcultured for 20–25 days were cut off, and the upper part was removed. Then, they were cultured in CIM (liquid WPM basal medium supplemented with 0.02 mg·L−1 of NAA and 0.24 mg·L−1 of CPPU) for 15 days to induce calluses (Figure 2A). After 3 days, the leaves began to swell and became discolored, and calluses were forming (Figure 2B). After 15 days, leaves were transferred to SIM and continued to expand, and calluses were further developing (Figure 2C). Green bud spots appeared on the petioles of leaves after 1 month of in vitro culture (Figure 2D). The regenerated adventitious buds gradually grew and elongated (Figures 2E–H), and they were cut into 1-cm pieces and transferred to RIM when the length of regenerated adventitious buds was more than 3 cm (Figure 2I). Taken together, the regeneration of E. urophylla × E. grandis DH32-29 from leaves to form a whole plantlet was accomplished in approximately 2 months. The highest adventitious bud rate was 76.5% (Supplementary Figure 2D) after optimization.
Figure 2 Adventitious bud regeneration from leaves of Eucalyptus urophylla × Eucalyptus grandis DH32-29. The explants were cultured in the medium for (A) 0, (B) 3, (C) 15, (D) 30, (E) 45, and (F) 60 days. Regenerated buds are propagated (G, H) and rooted (I). Scale bar: 1 cm.
Cefotaxime and timentin are usually used to inhibit the excessive proliferation of Agrobacterium. The induction percentage of calli and adventitious buds was not significantly affected by supplementation with Cef and Tmt (Figures 3A–C). Calli were also found to decrease in size, which would be helpful for further antibiotic selection. The combination of 100 mg·L−1 of Cef and 100 mg·L−1 of Tmt (T6) was chosen for selecting transgenic adventitious buds because of the preferable inhibition effect. A total of 200 mg·L−1 of Cef (T3) was supplemented in RIM for the higher adventitious root induction rate (Figures 3D,E). Leaves were cultured in CIM and SIM supplemented with a series of concentrations of Kan or Hyg to determine the critical concentration for selection. The regeneration of adventitious buds was totally inhibited when Kan was applied at a concentration of 15 mg·L−1 (Figure 4). In contrast, the formation of adventitious roots was nearly inhibited when the concentration of Kan reached 75 mg·L−1 in RIM (Figures 4E, F). Hence, 15 mg·L−1 and 75 mg·L−1 of Kan were used to screen transgenic adventitious buds and roots, respectively. Interestingly, both adventitious bud regeneration and root formation were highly sensitive to Hyg. Hyg (5 mg·L−1) almost completely inhibited the regeneration of adventitious buds and root formation (Figure 5). Finally, 5 mg·L−1 of Hyg was determined for the selection of transgenic plantlets with the hygromycin resistance gene.
Figure 3 Effects of cefotaxime (Cef) and timentin (Tmt) on regeneration of Eucalyptus urophylla × Eucalyptus grandis DH32-29. Effects of Cef and Tmt on (A) the frequency of callus induction, (B) callus size, (C) frequency of adventitious bud induction, (D) frequency of adventitious root induction, and (E) length of roots. Combinations of antibiotics: A1, 0 mg·L−1 of Cef and 0 mg·L−1 of timentin (Tmt); A2, 100 mg·L−1 of Cef and 0 mg·L−1 of Tmt; A3, 200 mg·L−1 of Cef and 0 mg·L−1 of Tmt; A4, 300 mg·L−1 of Cef and 0 mg·L−1 of Tmt; A5, 400 mg·L−1 of Cef and 0 mg·L−1 of Tmt; A6, 100 mg·L−1 of Cef and 100 mg·L−1 of Tmt; A7, 100 mg·L−1 of Cef and 50 mg·L−1 of Tmt; A8, 200 mg·L−1 of Cef and 50 mg·L−1 of Tmt. Different letters indicate significant differences among treatments using Duncan’s multiple range test at p < 0.05.
Figure 4 Effects of kanamycin on regeneration of Eucalyptus urophylla × Eucalyptus grandis DH32-29. (A) Explants are induced to regenerate adventitious buds on medium supplemented with 0, 3, 6, 9, 12, and 15 mg·L−1 of kanamycin. Effects of kanamycin on (B) the frequency of callus induction, (C) callus size, (D) the frequency of adventitious bud induction, (E) the frequency of adventitious root induction, and (F) the length of roots. Scale bar: 1 cm. Different letters indicate significant differences among treatments using Duncan’s multiple range test p < 0.05.
Figure 5 Effects of hygromycin on regeneration of Eucalyptus urophylla × Eucalyptus grandis DH32-29. (A) Explants are induced to regenerate adventitious buds on medium supplemented with 0, 1, 2, 3, 4, and 5 mg·L−1 of hygromycin. Effects of hygromycin on (B) the frequency of callus induction, (C) callus size, (D) the frequency of adventitious bud induction, (E) the frequency of adventitious root induction, and (F) the length of root. Scale bar: 1 cm. Different letters indicate significant differences among treatments using Duncan’s multiple range test at p < 0.05.
The transformation efficiency showed no obvious difference in the GV3101, EHA105, LBA4404, and AGL1 strains (Figure 6A). EHA105 was chosen as the bacterial strain for the following optimization because most transgenic plants were infected with EHA105. The highest transformation rate of leaves reached 63.1% when they were infected with a bacterial suspension of 0.3 (Figure 6B). There was no significant difference in the transformation efficiency of leaves when they were infected for 30 or 120 min (Figure 6C). However, the longer infection time caused damage to explants, resulting in the browning of leaves in the subsequent selective culture. Thus, 30 min was chosen for subsequent experiments. Cocultivation time was also performed to promote the transformation. The result showed that cocultivation for 72 h reached 62.6% transformation efficiency, which benefitted the transfer and integration of T-DNA and avoided the overgrowth of Agrobacterium (Figure 6D). Moreover, the transformation efficiency was significantly increased when leaves were precultured for 7 days (Figure 6E). Additionally, AS supplemented in bacterial suspension was not observed to significantly improve the frequency of genetic transformation in this study (Figure 6F).
Figure 6 Optimization of Agrobacterium-mediated genetic transformation of Eucalyptus urophylla × Eucalyptus grandis DH32-29. Effect of (A) Agrobacterium strain, (B) Agrobacterium concentration, (C) duration of infection, (D) duration of cocultivation, (E) duration of precultivation, and (F) concentration of the additive acetosyringone on the transformation rate. Different letters indicate significant differences among treatments using Duncan’s multiple range test at p < 0.05.
The regenerated adventitious buds showing resistance to Kan were analyzed by GUS staining and PCR amplification to detect the integration of nptⅡ and uidA genes. Finally, seven regenerated plants were verified as positive transformants from 360 infected leaves in three experiments, with a 1.9% transformation rate (Figure 7). The negative control, in which adventitious buds were not infected, showed that GUS dyeing failed, and no target band was amplified in the negative control. Some resistant adventitious buds were chimeric blue, which might be caused by uneven staining or different expression levels of uidA gene at different locations.
Figure 7 Molecular detection and β-glucuronidase (GUS) expression in transgenic plants. (A) Negative control. (B, C) GUS staining was observed in transgenic plants. (D) PCR amplification of nptⅡ gene and uidA gene in pBI121: (M) marker, (+) plasmid, (WT) untransformed wild type, (H2O) water control, and (L1-L7) transgenic lines. Scale bar: 0.25 cm.
The whole workflow of genetic transformation in E. urophylla × E. grandis DH32-29 was drawn and is shown in Figure 8. Leaves were cut from plantlets that were subcultured for 20–25 days and laid on CIM supplemented with 0.02 mg·L−1 of NAA and 0.24 mg·L−1 of CPPU. After 7 days, the explants were transferred to an Agrobacterium EHA105 suspension of 0.3 (OD600) for infiltration for 30 min. Then, the explants were moved to coculture medium and cultivated for 72 h at 25°C to transfer exogenous genes and integrate them into plant cells. Next, explants were cultured in CIM supplemented with 100 mg·L−1 of Cef, 100 mg·L−1 of Tmt, and 15 mg·L−1 of Kan. Fifteen days later, explants were transferred to SIM supplemented with the same concentration of antibiotics as in CIM, and the same medium was replaced every 15 days until resistant adventitious buds gradually appeared. Putatively transgenic buds were cut and transferred to RIM supplemented with 200 mg·L−1 of Cef and 75 mg·L−1 of Kan to induce adventitious root formation. Finally, verified transgenic shoots were planted in the soil and grown in a greenhouse.
Figure 8 Agrobacterium-mediated Eucalyptus urophylla × Eucalyptus grandis DH32-29 leaf transformation workflow. (A) Leaves were cut from plantlets that were subcultured for 20–25 days and precultured in callus-inducing medium for 7 days. (B) Agrobacterium EHA105 was cultured to 0.3 (OD600) and (C) infected leaves for 30 min. Leaves were cocultured for 72 h and transferred to callus-inducing medium to induce calluses. (D) Transgenic adventitious buds were generated when explants were cultured in shoot-inducing medium. (E) Transgenic buds were propagated, induced roots, and (F) planted in the soil.
Due to the huge cultivated area in China and its high economic value, the establishment of a regeneration and genetic transformation system of Eucalyptus has received increasing attention. Although many Eucalyptus species have been transformed successfully in the past two decades (Moralejo et al., 1998; Ho et al., 1998; Prakash and Gurumurthi, 2009; Aggarwal et al., 2011; de Alcantara et al., 2011; Silva et al., 2011; Ahad et al., 2014), sexual materials used as explants have resulted in differences between the mother tree and descendants, which has limited the application of transgenic plants. In addition, seedlings were also used as explants to establish a regeneration system in E. urophylla × E. grandis DH32-29 (Ouyang and Li, 2016). N-Phenyl-N′-[6-(2-chlorobenzothiazol)-yl] urea used in inducing calli of E. urophylla × E. grandis was not a commercial plant growth regulator (Ouyang and Li, 2016) and was not shared in different laboratories, which limited the application of this protocol in various research groups. However, this research provided a hint for our study. As the largest planted area tree species in southern China, the establishment of a stable and efficient genetic transformation system of Eucalyptus urophylla × E. grandis DH32-29 has practical value. The resulting transgenic lines with genotypes improved by genetic transformation can be used directly in afforestation and gene function analysis. Hence, it is necessary to establish an efficient adventitious bud regeneration and Agrobacterium-mediated genetic transformation system for E. urophylla × E. grandis DH32-29.
There are many factors affecting plant regeneration, including genotype, plant growth regulators, medium components, type of explants, and other factors. Plant growth regulators play a crucial role in plant development and regeneration. Thidiazuron, as an important cytokinin, has been widely used in Eucalyptus regeneration (Cid et al., 1999; Shabannejad Mamaghani et al., 2009; de França Bettencourt et al., 2020). Our research group successfully used TDZ to induce the regeneration of a variety of eucalyptus plants. However, TDZ did not work very well on E. urophylla × E. grandis DH32-29, which also indicated that this clone is difficult to regenerate. Other cytokinin hormones, such as 6-BA, kinetin, and zeatin, could also not promote adventitious bud regeneration in many preliminary experiments. CPPU, a cytokinin-like plant growth regulator widely used in promoting cell division and cell enlargement and preventing plant aging (Wu et al., 2020; Li et al., 2021), offered a breakthrough for our research. We found that the addition of CPPU observably enhanced the regeneration of adventitious buds. Although CPPU has also been applied to induce the regeneration of calli and adventitious buds (Mihaljević and Vršek, 2009; Sakr et al., 2018; Grzegorczyk-Karolak et al., 2021) and somatic embryogenesis (Murthy and Saxena, 1994; Fiore et al., 2002; Zhang et al., 2005; Zhang et al., 2006) in some plants, CPPU has rarely been used in woody plants. A previous study showed that the combination of CPPU and NAA was effective for the induction of nodule cultures in Eucalyptus that could regenerate shoots, and the effective concentration ranged from 0.2 to 0.5 mg·L−1 (Ito et al., 1996), consistent with our results that 0.24 mg·L−1 of CPPU promoted callus formation and adventitious bud regeneration. High contents of Ca, K, and Mg were reported to be detected in CPPU-containing medium, and CPPU enhanced regeneration and adventitious bud elongation of Jatropha curcas because of the high mineral uptake (Singh, 2017), which might explain the accelerative effect of CPPU.
The formation of calli is very important to regenerate adventitious buds. Calluses of different ages showed different growth capacities and regeneration abilities (Cheng et al., 2009; Carsono et al., 2021), which was also shown in our study. The highest frequency of callus and adventitious bud induction of E. urophylla × E. grandis DH32-29 was obtained when leaves were induced callus for 15 days. Young calluses were reported to contain more dividing cells, which were more sensitive to shoot initiation and development (Manimaran et al., 2013). In addition, liquid WPM media were used in our research instead of normal solid media, which promoted the absorption of nutrients by explants. In addition, the physiological status of explants affected their regeneration ability. Propagated plantlets with shorter days after subculture had a higher frequency of adventitious bud induction, and older aseptic plantlets had a lower regeneration rate due to the lack of nutrients or the accumulation of certain metabolites (Lv et al., 2005), which may be relevant to the expression of miR156, whose decreased expression level in old plants promotes the transcription of miR156-targeted Squamosa promoter binding protein-like (SPL) transcription factors, which affect the cytokinin signaling pathway (Zhang et al., 2015). However, plants with different durations of subculture had no significant difference in the frequency of adventitious bud induction in this research, which might be due to the comprehensive effect of multiple factors on explant status.
Suitable selection pressure allows the normal growth of transgenic plants and prevents untransformed plants from escaping. Therefore, it is essential to determine the critical concentration of antibiotics to obtain transgenic plants. Previous studies have shown that great differences exist in the kanamycin tolerance of different species, which varies from 12.5 to 110 mg·L−1 and varies with the type of explant (Prakash and Gurumurthi, 2009; Silva et al., 2010; Guo et al., 2012; de França Bettencourt et al., 2020; Wang et al., 2022). Eucalyptus was more sensitive to hygromycin, and a low concentration was enough to inhibit plant regeneration (Fernando et al., 2016; Rathinavel et al., 2020). In our research, no adventitious buds regenerated under 15 mg·L−1 Kan treatment or 5 mg·L−1 Hyg treatment. Vast differences existed in the response to antibiotics in adventitious buds and roots of E. urophylla × E. grandis DH32-29. Thus, different concentrations of antibiotics during genetic transformation are required for the induction of adventitious buds and roots.
Major factors that affect Agrobacterium-mediated genetic transformation were optimized. Among these factors, the matching of suitable Agrobacterium strains and explants was important for successful transformation (Tzfira and Citovsky, 2006). Although the four strains showed no significant difference in the frequency of genetic transformation, large-scale selection experiments showed that it was easy to obtain transgenic plants from explants infected by EHA105. Hence, the EHA105 strain was chosen for further transformation. EHA105 was also reported to perform better in Populus (Wang et al., 2011). Furthermore, 7 days’ precultivation before the infection significantly increased the frequency of transformation in our research. Similar results were reported in other studies, and the duration of preculture depended on the species of Eucalyptus and the type of explants (Moralejo et al., 1998; de Alcantara et al., 2011; Ahad et al., 2014). This improvement may be related to the increased survival rate of explants by preculture (Maheshwari and Kovalchuk, 2016), which was also found in our research. Explants without precultivation were too weak and easily died after infection. In contrast, the precultured explants were more robust and could survive after coculture with bacteria, which made it more probable to obtain transgenic plants. Although the chance for untransformed plants to escape increases in the presence of precultivation, multiple methods can be used to detect the insertion of foreign genes effectively. Acetosyringone, which is often used to induce vir gene expression to improve the transfer of genes (Stachel et al., 1985; Stachel and Nester, 1986; Godwin et al., 1991), did not significantly improve genetic transformation in our research. The easy browning of explants indicates that abundant phenolic compounds are present in E. urophylla × E. grandis DH32-29 and that AS cannot play its role effectively.
In summary, we established and optimized an Agrobacterium-mediated genetic transformation system using leaves as explants in E. urophylla × E. grandis DH32-29. The frequency of genetic transformation was almost 1.9%, which made it possible to identify some key genes involved in their superior traits, such as fast growth and wide adaptability of Eucalyptus. Moreover, this system can be used for producing transgenic lines with modified traits, especially in improving the tolerance of insects and herbicides for further afforestation.
The original contributions presented in the study are included in the article/Supplementary Material. Further inquiries can be directed to the corresponding author.
CF conceived this study. XW, SC, HZ, PL, and FZ performed the experiments. XW analyzed the data. XW and CF wrote the manuscript. BZ and JX revised and gave advice on the manuscript. All authors contributed to the article and approved the submitted version.
This research was supported by the National Key R&D Program of China during the 14th Five-year Plan Period (2021YFD2200102) and Fundamental Research Funds for the Central Non-profit Research Institution of CAF (CAFYBB2020ZB004).
We would like to thank the AJE team for the English editing.
The authors declare that the research was conducted in the absence of any commercial or financial relationships that could be construed as a potential conflict of interest.
All claims expressed in this article are solely those of the authors and do not necessarily represent those of their affiliated organizations, or those of the publisher, the editors and the reviewers. Any product that may be evaluated in this article, or claim that may be made by its manufacturer, is not guaranteed or endorsed by the publisher.
The Supplementary Material for this article can be found online at: https://www.frontiersin.org/articles/10.3389/fpls.2022.1011245/full#supplementary-material
Aggarwal, D., Kumar, A., Reddy, M. S. (2015). Genetic transformation of endo-1,4-β-glucanase (Korrigan) for cellulose enhancement in Eucalyptus tereticornis. Plant Cell Tissue Organ Culture 122, 363–371. doi: 10.1007/s11240-015-0774-7
Aggarwal, D., Kumar, A., Sudhakara Reddy, M. (2011). Agrobacterium tumefaciens mediated genetic transformation of selected elite clone(s) of Eucalyptus tereticornis. Acta Physiologiae Plantarum 33, 1603–1611. doi: 10.1007/s11738-010-0695-3
Carsono, N., Juwendah, E., Liberty, Sari, S., Damayanti, F., Rachmadi, M. (2021). Optimize 2,4-d concentration and callus induction time enhance callus proliferation and plant regeneration of three rice genotypes. Biodiversitas J. Biol. Diversity 22, 2555–2560. doi: 10.13057/biodiv/d220702
Chang, S., Mahon, E. L., MacKay, H. A., Rottmann, W. H., Strauss, S. H., Pijut, P. M., et al. (2018). Genetic engineering of trees: Progress and new horizons. In Vitro Cell. Dev. Biol. - Plant 54, 341–376. doi: 10.1007/s11627-018-9914-1
Cheng, L., Wu, X., Hong, B. (2009). Establishment of plantlet regeneration system for Spiraea × bumalda ‘Gold mound’. J. Northeast Forestry Univ. 37, 32–34. doi: 10.3969/j.issn.1000-5382.2009.10.011
Chen, H., Yao, J., Huang, S., Pang, H. (2021). Ophelimus bipolaris sp. n. (Hymenoptera, eulophidae), a new invasive Eucalyptus pest and its host plants in China. Insects 12, 778. doi: 10.3390/insects12090778
Cid, L. P. B., Machado, A. C. M. G., Carvalheira, Í.B.R.C., Brasileiro, A. C. M. (1999). Plant regeneration from seedling explants of Eucalyptus grandis × E. urophylla. Plant Cell Tissue Organ Culture 56, 17–23. doi: 10.1023/A:1006283816625
de Alcantara, G. B., Filho, J. C. B., Quoirin, M. (2011). Organogenesis and transient genetic transformation of the hybrid Eucalyptus grandis × Eucalyptus urophylla. Scientia Agricola 68, 246–251. doi: 10.1590/S0103-90162011000200016
de França Bettencourt, G. M., Soccol, C. R., Giovanella, T. S., Franciscon, L., Kestring, D. R., Gerhardt, I. R., et al. (2020). Agrobacterium tumefaciens-mediated transformation of Eucalyptus urophylla clone BRS07-01. J. Forestry Res. 31, 507–519. doi: 10.1007/s11676-018-0777-4
Dibax, R., Deschamps, C., Filho, J. C. B., Vieira, L. G. E., Molinari, H. B. C., De Campos, M. K. F., et al. (2010). Organogenesis and Agrobacterium tumefaciens-mediated transformation of Eucalyptus saligna with P5CS gene. Biol. Plantarum 54, 6–12. doi: 10.1007/s10535-010-0002-6
Fernando, S. C., Goodger, J. Q. D., Gutierrez, S. S., Johnson, A. A. T., Woodrow, I. E. (2016). Plant regeneration through indirect organogenesis and genetic transformation of Eucalyptus polybractea R.T. baker. Ind. Crops Products 86, 73–78. doi: 10.1016/j.indcrop.2016.03.025
Fiore, S., De Pasquale, F., Carimi, F., Sajeva, M. (2002). Effect of 2,4-d and 4-CPPU on somatic embryogenesis from stigma and style transverse thin cell layers of Citrus. Plant Cell Tissue Organ Culture 68, 57–63. doi: 10.1023/A:1012944100210
Girijashankar, V. (2011). Genetic transformation of eucalyptus. Physiol. Mol. Biol. Plants 17, 9–23. doi: 10.1007/s12298-010-0048-0
Godwin, I., Todd, G., Ford-Lloyd, B., Newbury, H. J. (1991). The effects of acetosyringone and pH on Agrobacterium-mediated transformation vary according to plant species. Plant Cell Rep. 9, 671–675. doi: 10.1007/BF00235354
Grzegorczyk-Karolak, I., Hnatuszko-Konka, K., Krzemińska, M., Olszewska, M. A., Owczarek, A. (2021). Cytokinin-based tissue cultures for stable medicinal plant production: Regeneration and phytochemical profiling of Salvia bulleyana shoots. Biomolecules 11, 1513. doi: 10.3390/biom11101513
Guo, L., Zeng, B., Liu, Y., Li, X., Qiu, Z. (2012). Study on kanamycin and cefotaxime sensitivity of Eucalyptus grandis clone Eg5. J. Cent. South Univ. Forestry Technol. 32, 75–80. doi: 10.14067/j.cnki.1673-923x.2012.03.029
Harcourt, R. L., Kyozuka, J., Floyd, R. B., Bateman, K. S., Tanaka, H., Decroocq, V., et al. (2000). Insect- and herbicide-resistant transgenic eucalypts. Mol. Breed. 6, 307–315. doi: 10.1023/A:1009676214328
Hiwale, S. (2015). “Eucalyptus (Eucalyptus sp.),” in Sustainable horticulture in semiarid dry lands. Ed. Hiwale, S. (New Delhi, FL: Springer), 301–309.
Ho, C. K., Chang, S. H., Tsay, J. Y., Tsai, C. J., Chiang, V. L., Chen, Z. Z. (1998). Agrobacterium tumefaciens-mediated transformation of Eucalyptus camaldulensis and production of transgenic plants. Plant Cell Rep. 17, 675–680. doi: 10.1007/s002990050464
Ito, K., Doi, K., Tatemichi, Y., Shibata, M. (1996). Plant regeneration of eucalypts from rotating nodule cultures. Plant Cell Rep. 16, 42–45. doi: 10.1007/BF01275446
Jefferson, R. A., Kavanagh, T. A., Bevan, M. W. (1987). GUS fusions: Beta-glucuronidase as a sensitive and versatile gene fusion marker in higher plants. EMBO J. 6, 3901–3907. doi: 10.1002/j.1460-2075.1987.tb02730.x
Ji, L., Wang, Z., Wang, X., An, L. (2011). Forest insect pest management and forest management in China: An overview. Environ. Manage. 48, 1107–1121. doi: 10.1007/s00267-011-9697-1
Li, F., Huang, H., Ding, X., Liu, J., He, M., Shan, Y., et al. (2021). Effect of CPPU on postharvest attributes of Chinese flowering cabbage during storage. Postharvest Biol. Technol. 174, 111438. doi: 10.1016/j.postharvbio.2020.111438
Luo, J., Arnold, R., Lu, W., Lin, Y. (2014). Genetic variation in Eucalyptus camaldulensis and E. tereticornis for early growth and susceptibility to the gall wasp Leptocybe invasa in China. Euphytica 196, 397–411. doi: 10.1007/s10681-013-1042-8
Lv, J., Wu, Y., Sun, L., Gao, Y., Zhang, Q. (2005). Establishment of shoot regeneration system from leaves of Dendranthema × grandiflora. J. Beijing Forestry Univ. 27, 97–100. doi: 10.13332/j.1000-1522.2005.04.020
Maheshwari, P., Kovalchuk, I. (2016). Agrobacterium-mediated stable genetic transformation of Populus angustifolia and Populus balsamifera. Front. Plant Sci. 7. doi: 10.3389/fpls.2016.00296
Manimaran, P., Kumar, G. R., Reddy, M. R., Jain, S., Rao, T. B., Mangrauthia, S. K., et al. (2013). Infection of early and young callus tissues of indica rice BPT 5204 enhances regeneration and transformation efficiency. Rice Sci. 20, 415–426. doi: 10.1016/S1672-6308(13)60153-5
Matsunaga, E., Nanto, K., Oishi, M., Ebinuma, H., Morishita, Y., Sakurai, N., et al. (2012). Agrobacterium-mediated transformation of Eucalyptus globulus using explants with shoot apex with introduction of bacterial choline oxidase gene toenhance salt tolerance. Plant Cell Rep. 31, 225–235. doi: 10.1007/s00299-011-1159-y
Mihaljević, S., Vršek, I. (2009). In vitro shoot regeneration from immature seeds of Epimedium alpinum induced by thidiazuron and CPPU. Scientia Hortic. 120, 406–410. doi: 10.1016/j.scienta.2008.11.019
Moralejo, M. F., Rochange, A. M. B., Teulières, C. (1998). Generation of transgenic eucalyptus globulus plantlets through Agrobacterium tumefaciens mediated transformation. Aust. J. Plant Physiol. 25, 207–212. doi: 10.1071/PP97041
Murashige, T., Skoog, F. (1962). A revised medium for rapid growth and bio assays with tobacco tissue cultures. Physiologia Plantarum 15, 473–497. doi: 10.1111/j.1399-3054.1962.tb08052.x
Murthy, B. N. S., Saxena, P. K. (1994). Somatic embryogenesis in peanut (Arachis hypogaea l.): Stimulation of direct differentiation of somatic embryos by forchlorfenuron (CPPU). Plant Cell Rep. 14, 145–150. doi: 10.1007/BF00233779
National Forestry and Grassland Administration (2019). China forest resources report (2014-2018) BeiJing: China: Forestry Publishing House.
Navarro, M., Ayax, C., Martinez, Y., Laur, J., El Kayal, W., Marque, C., et al. (2011). Two EguCBF1 genes overexpressed in Eucalyptus display a different impact on stress tolerance and plant development. Plant Biotechnol. J. 9, 50–63. doi: 10.1111/j.1467-7652.2010.00530.x
Ouyang, L., He, W., Huang, Z., Zhao, L., Peng, S., Sha, Y., et al. (2012). Introduction of the Rs-AFP2 gene into Eucalyptus urophylla for resistance to Phytophthora capsici. J. Trop. For. Sci. 24, 198–208. doi: jstor.org/stable/23617076
Ouyang, L., Li, L. (2016). Effects of an inducible aiiA gene on disease resistance in Eucalyptus urophylla × Eucalyptus grandis. Transgenic Res. 25, 441–452. doi: 10.1007/s11248-016-9940-x
Pena, L., Seguin, A. (2001). Recent advances in the genetic transformation of trees. Trends Biotechnol. 19, 500–506. doi: 10.1016/s0167-7799(01)01815-7
Prakash, M. G., Gurumurthi, K. (2009). Genetic transformation and regeneration of transgenic plants from precultured cotyledon and hypocotyl explants of Eucalyptus tereticornis sm. using Agrobacterium tumefaciens. In Vitro Cell. Dev. Biol. - Plant 45, 429–434. doi: 10.1007/s11627-008-9179-1
Shabannejad Mamaghani, M., Assareh, M. H., Omidi, M., Matinizadeh, M., Ghamari-Zare, A., Shahrzad, S., et al. (2009). The effect of thidiazuron level on in vitro regeneration type and peroxidase profile in Eucalyptus microtheca f. muell. Plant Growth Regul. 59, 199–205. doi: 10.1007/s10725-009-9404-x
Silva, A. L. L. D., Oliveira, Y. D., Costa, J. D. L., Masetto, E., Mudry, C. D. S., Erasmo, E. A. L., et al. (2010). Shoot tip and cotyledon explants of Eucalyptus saligna sm. cultivated on different kanamycin levels. J. Biotechnol. Biodiversity 1, 1–5. doi: 10.20873/jbb.uft.cemaf.v1n1.silva
Silva, A. L. L. D., Oliveira, Y., Costa, J. D. L., Mudry, C. D. S., Scheidt, G. N., Brondani, G. E. (2011). Preliminary results for genetic transformation of shoot tip of Eucalyptus saligna sm. Via agrobacterium tumefaciens. J. Biotechnol. Biodiversity 2, 1–6. doi: 10.20873/jbb.uft.cemaf.v2n1.silva
Singh, A. (2017). N-(2-chloro-4-pyridyl)-N-phenylurea enhanced regeneration of Jatropha curcas leaf explants by high mineral acquisition. Biologia 72, 300–304. doi: 10.1515/biolog-2017-0030
Song, C., Lu, L., Guo, Y., Xu, H., Li, R. (2019). Efficient Agrobacterium-mediated transformation of the commercial hybrid poplar Populus alba × Populus glandulosa uyeki. Int. J. Mol. Sci. 20, 2594. doi: 10.3390/ijms20102594
Stachel, S. E., Messens, E., Van Montagu, M., Zambryski, P. (1985). Identification of the signal molecules produced by wounded plant cells that activate T-DNA transfer in Agrobacterium tumefaciens. Nature 318, 624–629. doi: 10.1038/318624a0
Stachel, S. E., Nester, E. W. (1986). The genetic and transcriptional organization of the vir region of the A6 Ti plasmid of Agrobacterium tumefaciens. EMBO J. 5, 1445–1454. doi: 10.1002/j.1460-2075.1986.tb04381.x
Teulieres, C., Marque, C. (2007). “Eucalyptus,” in Transgenic crops Ⅴ. Eds. Pua, E.-C., Davey Michael, R. (Berlin, FL: Springer), 387–406.
Thanananta, N., Vuttipongchaikij, S., Apisitwanich, S. (2018). Agrobacterium-mediated transformation of a Eucalyptus camaldulensis × E. tereticornis hybrid using peeled nodal-stem segments with yeast HAL2 for improving salt tolerance. New Forests 49, 311–327. doi: 10.1007/s11056-017-9621-5
Tzfira, T., Citovsky, V. (2006). Agrobacterium-mediated genetic transformation of plants: Biology and biotechnology. Curr. Opin. Biotechnol. 17, 147–154. doi: 10.1016/j.copbio.2006.01.009
Valério, L., Carter, D., Rodrigues, J. C., Tournier, V., Gominho, J., Marque, C., et al. (2003). Down regulation of cinnamyl alcohol dehydrogenase, a lignification enzyme, in Eucalyptus camaldulensis. Mol. Breed. 12, 157–167. doi: 10.1023/A:1026070725107
Wang, X., Luo, P., Qiu, Z., Li, X., Zeng, B., Fan, C. (2022). Adventitious bud regeneration and Agrobacterium tumefaciens-mediated genetic transformation of Eucalyptus urophylla × E. tereticornis interspecific hybrid. In Vitro Cell. Dev. Biol. - Plant 58, 416–426. doi: 10.1007/s11627-021-10240-x
Wang, H., Wang, C., Liu, H., Tang, R., Zhang, H. (2011). An efficient Agrobacterium-mediated transformation and regeneration system for leaf explants of two elite aspen hybrid clones Populus alba × P. berolinensis and Populus davidiana × P. bolleana. Plant Cell Rep. 30, 2037–2044. doi: 10.1007/s00299-011-1111-1
Wu, L., Lan, J., Xiang, X., Xiang, H., Jin, Z., Khan, S., et al. (2020). Transcriptome sequencing and endogenous phytohormone analysis reveal new insights in CPPU controlling fruit development in kiwifruit (Actinidia chinensis). PloS One 15, e240355. doi: 10.1371/journal.pone.0240355
Yu, X., Kikuchi, A., Matsunaga, E., Morishita, Y., Nanto, K., Sakurai, N., et al. (2013). The choline oxidase gene codA confers salt tolerance to transgenic Eucalyptus globulus in a semi-confined condition. Mol. Biotechnol. 54, 320–330. doi: 10.1007/s12033-012-9575-y
Zhang, Q., Chen, J., Henny, R. J. (2005). Direct somatic embryogenesis and plant regeneration from leaf, petiole, and stem explants of golden pothos. Plant Cell Rep. 23, 587–595. doi: 10.1007/s00299-004-0882-z
Zhang, Q., Chen, J., Henny, R. J. (2006). Regeneration of Syngonium podophyllum ‘Variegatum’ through direct somatic embryogenesis. Plant Cell Tissue Organ Culture 84, 181–188. doi: 10.1007/s11240-005-9019-5
Keywords: Eucalyptus, regeneration, adventitious bud, Agrobacterium tumefaciens, genetic transformation
Citation: Wang X, Chen S, Zhang H, Luo P, Zhou F, Zeng B, Xu J and Fan C (2022) Agrobacterium-mediated genetic transformation of the most widely cultivated superior clone Eucalyptus urophylla × Eucalyptus grandis DH32-29 in Southern China. Front. Plant Sci. 13:1011245. doi: 10.3389/fpls.2022.1011245
Received: 04 August 2022; Accepted: 12 September 2022;
Published: 29 September 2022.
Edited by:
Qingzhang Du, Beijing Forestry University, ChinaReviewed by:
Kunxi Ouyang, South China Agricultural University, ChinaCopyright © 2022 Wang, Chen, Zhang, Luo, Zhou, Zeng, Xu and Fan. This is an open-access article distributed under the terms of the Creative Commons Attribution License (CC BY). The use, distribution or reproduction in other forums is permitted, provided the original author(s) and the copyright owner(s) are credited and that the original publication in this journal is cited, in accordance with accepted academic practice. No use, distribution or reproduction is permitted which does not comply with these terms.
*Correspondence: Chunjie Fan, ZmFuY2h1bmppZUBjYWYuYWMuY24=
Disclaimer: All claims expressed in this article are solely those of the authors and do not necessarily represent those of their affiliated organizations, or those of the publisher, the editors and the reviewers. Any product that may be evaluated in this article or claim that may be made by its manufacturer is not guaranteed or endorsed by the publisher.
Research integrity at Frontiers
Learn more about the work of our research integrity team to safeguard the quality of each article we publish.