- 1Instituto de Bioingeniería, Universidad Miguel Hernández, Elche, Spain
- 2Área de Genética, Universidad Miguel Hernández, Alicante, Spain
- 3Centro Nacional de Biotecnología, CNB-CSIC, Madrid, Spain
- 4Umeå Plant Science Centre, Department of Forest Genetics and Plant Physiology, Swedish University of Agricultural Sciences, Umeå, Sweden
ATP-Binding Cassette E (ABCE) proteins dissociate cytoplasmic ribosomes after translation terminates, and contribute to ribosome recycling, thus linking translation termination to initiation. This function has been demonstrated to be essential in animals, fungi, and archaea, but remains unexplored in plants. In most species, ABCE is encoded by a single-copy gene; by contrast, Arabidopsis thaliana has two ABCE paralogs, of which ABCE2 seems to conserve the ancestral function. We isolated apiculata7-1 (api7-1), the first viable, hypomorphic allele of ABCE2, which has a pleiotropic morphological phenotype reminiscent of mutations affecting ribosome biogenesis factors and ribosomal proteins. We also studied api7-2, a null, recessive lethal allele of ABCE2. Co-immunoprecipitation experiments showed that ABCE2 physically interacts with components of the translation machinery. An RNA-seq study of the api7-1 mutant showed increased responses to iron and sulfur starvation. We also found increased transcript levels of genes related to auxin signaling and metabolism. Our results support for the first time a conserved role for ABCE proteins in translation in plants, as previously shown for the animal, fungal, and archaeal lineages. In Arabidopsis, the ABCE2 protein seems important for general growth and vascular development, likely due to an indirect effect through auxin metabolism.
Introduction
Messenger RNA (mRNA) molecules are decoded for protein synthesis by the complex and ancient translation machinery, formed by the ribosome and different sets of translation factors, which function at different translation phases. Translation initiation factors promote the formation of the 70S/80S initiation complex, and the recognition of the mRNA translation start site (Rodnina, 2018; Shirokikh and Preiss, 2018). Translation elongation factors participate in the binding of aminoacyl-tRNAs to the ribosome, the elongation of the peptides, and the ulterior release of the deacylated tRNA (Dever et al., 2018). Translation termination factors act when the ribosome reaches the translation stop codon and the newly synthesized peptide is released. In this latter phase, the ribosome is dissociated into its 50S/60S and 30S/40S subunits, which are recycled for a new cycle of translation initiation (Hellen, 2018). The ATP-Binding Cassette E (ABCE) proteins are soluble ABC proteins that participate in ribosome recycling and translation initiation, as have been demonstrated for archaea, fungi, and animals, but whose roles in plants remain unexplored (Kashima et al., 2014; Young et al., 2015; Nürenberg-Goloub et al., 2020; Simonetti et al., 2020). Human ABCE1 was first named RNASE L INHIBITOR (RLI) due to its ability to inhibit the activity of RNase L, an enzyme that is only present in mammals (Bisbal et al., 1995).
ABCE proteins contain an iron-sulfur cluster binding domain (FeSD), two nucleotide binding domains (NBD1 and NBD2), and two hinge motifs (Karcher et al., 2005; Barthelme et al., 2007; Karcher et al., 2008). The first hinge motif allows NBD movement to bind and hydrolyze ATP. The second hinge motif and a helix-loop-helix (HLH) mediate the interaction of the ABCE protein with the ribosome after occlusion of two ATP molecules. Once in the ribosome, the ABCE protein displaces its FeSD to split the ribosome, and remains bound to the 30S/40S subunit to prevent a premature recruitment of a 50S/60S subunit during translation initiation. Finally, ATP hydrolysis allows ABCE detachment from the 30S/40S subunit (Barthelme et al., 2011; Becker et al., 2012; Preis et al., 2014; Heuer et al., 2017; Nürenberg-Goloub et al., 2018; Gouridis et al., 2019; Kratzat et al., 2021).
In most genomes, the ABCE subfamily is represented by a single-copy gene, usually named ABCE1, whose null alleles are lethal, while hypomorphic alleles result in developmental defects and slow-growth phenotypes (Navarro-Quiles et al., 2018). Arabidopsis thaliana (hereafter referred to as Arabidopsis), however, has two ABCE paralogs named ABCE1 and ABCE2 (Sánchez-Fernández et al., 2001; Verrier et al., 2008). Arabidopsis ABCE2 has been studied for its RNA silencing suppression activity (Sarmiento et al., 2006; Mõttus et al., 2020). In Cardamine hirsuta, a close relative of Arabidopsis with compound leaves, only one ABCE gene has been identified, SIMPLE LEAF3 (SIL3), which is required for leaflet formation and leaf development. The leaves of homozygotes for the hypomorphic sil3 mutation are simple and have vascular defects, probably caused by an aberrant auxin transport and homeostasis (Kougioumoutzi et al., 2013).
Here, we report a functional analysis of the Arabidopsis ABCE2 gene. We studied two recessive alleles of ABCE2: the hypomorphic and viable apiculata7-1 (api7-1) allele, and the null and lethal api7-2 allele. The api7-1 mutant exhibits the typical morphological phenotype caused by mutations in genes encoding ribosome biogenesis factors and ribosomal proteins, which includes aberrant leaf venation patterns. We found by co-immunoprecipitation that ABCE2 physically interacts with components of the translation machinery, and by RNA-seq that its partial loss of function triggers iron and sulfur deficiency responses that might be related to FeS cluster biogenesis, as well as the upregulation of auxin biosynthesis genes. Our observations strongly suggest a conserved role for plant ABCE proteins in translation, probably through ribosome recycling as previously shown for the animal, fungal, and archaeal lineages.
Materials and methods
Plant materials, growth conditions, and crosses
The Arabidopsis thaliana (L.) Heynh. wild-type accessions Landsberg erecta (Ler) and Columbia-0 (Col-0), and the asymmetric leaves1-1 (as1-1; N3374; in the Col-1 genetic background) and as2-1 (N3117; in ER) mutants were initially obtained from the Nottingham Arabidopsis Stock Center (NASC; Nottingham, United Kingdom). We introgressed the as1-1 and as2-1 mutations into the Col-0 background by crossing to Col-0 three times. The NASC also provided seeds of the api7-2 (GABI_509C06; N448798) (Kleinboelting et al., 2012) and PIN1pro:PIN1:GFP DR5pro:3XVENUS:N7 (N67931) (Heisler et al., 2005) lines. The ATHB8pro:GUS line (N296) was kindly provided by Simona Baima (Baima et al., 1995). The api7-1 line was isolated in the Ler background after ethyl methanesulfonate (EMS) mutagenesis in our laboratory, and then backcrossed twice to Ler (Berná et al., 1999). Unless otherwise stated, all the mutants mentioned in this work are homozygous for the mutations indicated. Seed sterilization and sowing, plant culture, crosses, and allelism tests were performed as previously described (Ponce et al., 1998; Berná et al., 1999; Quesada et al., 2000).
Positional cloning and molecular characterization of api7 mutant alleles
Genomic DNA was extracted as previously described (Ponce et al., 2006). The ABCE2 gene was cloned as previously described (Mateo-Bonmatí et al., 2014). First, we mapped the api7-1 mutation to a 123.5-kb candidate interval containing 30 genes using a mapping population of 273 F2 plants derived from an api7-1 × Col-0 cross, and the primers listed in Supplementary Table S1, as previously described (Ponce et al., 1999; Ponce et al., 2006). Then, the whole api7-1 genome was sequenced by Fasteris (Geneva, Switzerland) using the Illumina HiSeq2000 platform. The bioinformatic analysis of the data was performed as previously described (Mateo-Bonmatí et al., 2014).
Discrimination between the wild-type ABCE2 and api7-1 mutant alleles was done by PCR with the api7-1_F/R primers (Supplementary Table S1), followed by restriction with Eco57I (Thermo Fisher Scientific), as the api7-1 mutation (CTCCAG→CTTCAG) creates an Eco57I restriction site. The presence and position of the api7-2 T-DNA insertion in the GABI_509C06 line was confirmed by PCR amplification and Sanger sequencing, respectively, using gene-specific primers and the o8409 primer for the GABI-Kat T-DNA (Supplementary Table S1).
Gene constructs and plant transformation
All inserts were PCR amplified from Col-0 genomic DNA using Phusion High Fidelity DNA Polymerase (Thermo Fisher Scientific) and primers that contained attB sites at their 5′ ends (Supplementary Table S1). PCR products were purified using an Illustra GFX PCR DNA and Gel Band Purification Kit (Cytiva), and then cloned into the pGEM-T Easy221 vector, transferred to Escherichia coli DH5α, and subcloned into the pEarleyGate 101, pMDC83, or pMDC107 destination vectors (Curtis and Grossniklaus, 2003; Earley et al., 2006) as previously described (Mateo-Bonmatí et al., 2018).
All constructs were transferred to electrocompetent Agrobacterium tumefaciens GV3101 (C58C1 RifR) cells, which were used to transform Ler or api7-1 plants by the floral dip method (Clough and Bent, 1998). Putative transgenic plants were selected on plates supplemented with 15 µg·ml−1 hygromycin B (Thermo Fisher Scientific, Invitrogen).
To obtain the GSRhino-TAP-tagged ABCE2 fusion, a pGEM-T Easy221 vector harboring the ABCE2 coding sequence, together with the pEN-L4-2-R1 and pEN-R2-GSRhinotag-L3 entry vectors were subcloned into the pKCTAP destination vector as previously described (Van Leene et al., 2015). Transformation of Arabidopsis cell cultures was performed as previously described (Van Leene et al., 2015).
Phenotypic analysis and morphometry
Photographs were taken with a Nikon SMZ1500 stereomicroscope equipped with a Nikon DXM1200F digital camera. For larger specimens, four to five partial images from the same plant were taken and merged using the Photomerge tool of Adobe Photoshop CS3 software. For rosette size, rosette silhouettes were drawn on the screen of a Cintiq 18SX Interactive Pen Display (Wacom) using Adobe Photoshop CS3, and their sizes were measured with the NIS Elements AR 3.1 image analysis package (Nikon). Root length was measured per triplicate from photographs with the Freehand line tool from Fiji software (https://imagej.net/ImageJ) (Schindelin et al., 2012). Shoot length was measured in vivo with a millimeter ruler, from the soil to the apex of the main shoot. Chlorophyll a and b and carotenoids were extracted and spectrophotometrically determined as previously described (Wellburn, 1994; Micol-Ponce et al., 2020), and normalized to the amount of collected tissue.
Differential interference contrast and bright-field microscopy, andGUS analyses
For differential interference contrast (DIC) and bright-field microscopy, all samples were cleared, mounted, and photographed as previously described (Candela et al., 1999). Micrographs of venation patterns, and leaf primordia expressing ATHB8pro:GUS were taken under bright field with a Nikon D-Eclipse C1 confocal microscope equipped with a Nikon DS-Ri1 camera, using the NIS-Elements AR 3.1 software (Nikon). Diagrams from leaf cells and venation patterns, and morphometric analysis of leaf cells were obtained as previously described (Pérez-Pérez et al., 2011; Mateo-Bonmatí et al., 2018). For venation pattern morphometry, the phenoVein (http://www.plant-image-analysis.org) (Bühler et al., 2015) software was used. Leaf lamina circularity was calculated as 4 · π · area/perimeter2. Lamina area and perimeter were measured on diagrams from the leaf lamina with the Fiji Wand tool. GUS assays were performed as previously described (Robles et al., 2010).
Confocal microscopy and fluorescence quantification
Confocal laser scanning microscopy images were obtained using a Nikon D-Eclipse C1 confocal microscope equipped with a Nikon DS-Ri1 camera and processed with the operator software EZ-C1 (Nikon). Visualization of the fluorescent proteins and dyes was performed on primary roots mounted with deionized water on glass slides. Fluorescent proteins, 4′,6-diamidino-2-phenylindole (DAPI), and propidium iodide were visualized as described in Supplementary Table S2. For fluorescence quantification of the PIN1pro:PIN1:GFP and DR5pro:3XVENUS:N7 protein products, wild-type and api7-1 seedlings homozygous for these transgenes were grown vertically on the same Petri dishes under identical conditions for 5 days. Image acquisition was performed using a 40× objective with a 0.75 numerical aperture. The dwell time was set at 2.16 and 1.68 µs for PIN1:GFP and 3XVENUS:N7, respectively. Four images were acquired and averaged per optical section. Five optical sections encompassing 4 µm from the innermost root layers were photographed. Acquired images (.ids files) were used to generate flat images (.tiff files) with Fiji, by stacking the optical sections from the fluorescent protein channel. Fluorescence quantification was performed using the Fiji Mean gray value measurement.
RNA isolation, cDNA synthesis, and quantitative PCR
Samples for RNA extraction were collected on ice and immediately frozen for storage at −80°C until use. RNA was isolated using TRIzol (Thermo Fisher Scientific, Invitrogen). Removal of contaminating DNA, cDNA synthesis, and quantitative PCR (qPCR) were performed as previously described (Mateo-Bonmatí et al., 2018). The qPCR was performed as follows: 2 min at 50°C, 10 min at 95°C, followed by 41 cycles of 15 s at 95°C and 1 min at 60°C, and a final step of 15 s at 95°C, and ACTIN2 (ACT2) was used as an internal control for relative expression analysis (Moschopoulos et al., 2012). Three biological replicates, each with three technical replicates, were analyzed per genotype. Relative quantification of gene expression data was performed using the comparative CT method (2−ΔΔCT) (Schmittgen and Livak, 2008).
RNA-seq analysis
Total RNA was isolated from 100 mg of Ler and api7-1 rosettes collected 14 days after stratification (das) using TRIzol. RNA concentration and quality were assessed using a 2100 Bioanalyzer (Agilent Genomics) with an RNA 600 Nano Kit (Agilent Technologies) as previously described (Mateo-Bonmatí et al., 2018). Three biological replicates per genotype, with more than 14 µg of total RNA per sample, and an RNA integrity number (RIN) higher than 7, were sent to Novogene (Cambridge, United Kingdom) for massive parallel sequencing. Sequencing libraries were generated using NEBNext Ultra RNA Library Prep Kit for Illumina (New England Biolabs) and fed into a NovaSeq 6000 Illumina platform with a S4 Flow Cell type, which produced paired-end reads of 150 bp (Supplementary Table S3). Read mapping to the Arabidopsis genome (TAIR10) using the 2.0.5 version of HISAT2 (Kim et al., 2019), with default parameters, and the identification of differentially expressed genes between Ler and api7-1 with the 1.22.2 version of DESeq2 R package (Love et al., 2014) were performed by Novogene. Genes with a P < 0.05 adjusted with the Benjamini and Hochberg’s method, and with a fold change > 1.5 were considered differentially expressed. The GO enrichment analysis of the differentially expressed genes was performed with the online tool DAVID (https://david.ncifcrf.gov/home.jsp) (Huang et al., 2009a; Huang et al., 2009b).
Indol-3-acetic acid metabolite profiling
Shoots, whole roots, and primary root tips (3 mm approximately) were collected 9 das from vertically grown seedlings. These samples were rapidly weighed and frozen in liquid nitrogen. Extraction and purification of the targeted compounds (anthranilate, Ant; tryptophan, Trp; indole-3-acetonitrile, IAN; indol-3-acetic acid, IAA; glycosylated IAA, IAA-glc; IAA conjugated to aspartate, IAA-Asp, and glutamate, IAA-Glu; 2-oxindole-3-acetic acid, oxIAA; oxIAA-glucoside, oxIAA-glc) were performed as previously described (Novák et al., 2012; Mateo-Bonmatí et al., 2021). Ultra-high performance liquid chromatography followed by MS/MS (UHPLC-MS/MS) analysis was performed as previously described (Pěnčík et al., 2018).
Co-immunoprecipitation assay
For protein extraction, 700 mg of whole api7-1 35Spro:ABCE2:YFP seedlings were collected 10 das per biological replicate. The tissue was crosslinked with 1× phosphate-saline buffer containing 1% (v/v) formaldehyde as previously described (Poza-Viejo et al., 2019). For protein extraction, the tissue was ground to a fine powder with liquid nitrogen and then resuspended in a lysis buffer (50 mM Tris-HCl, pH 7.5; 0.1% [v/v] IGEPAL CA-630 [Sigma-Aldrich]; 2 mM phenylmethylsulfonyl fluoride [PMSF; Sigma-Aldrich]; 150 mM NaCl; and a cOmplete protease inhibitor cocktail tablet [Sigma-Aldrich]) using a vortexer. After incubation on ice for 10 min, the samples were centrifuged at 4°C and the supernatants were used as protein extracts. Co-immunoprecipitation was performed with the µMACS GFP Isolation Kit (Milteny Biotec) using protein extracts from three biological replicates. The immunoprecipitation of the ABCE2:YFP fusion protein was checked by western blotting using an anti-GFP-HRP antibody (Milteny Biotec), and the WesternSure chemiluminiscent substrate on a C-DiGit Blot Scanner (LI·COR).
The co-immunoprecipitates were analyzed by liquid chromatography followed by electrospray ionization and tandem mass spectrometry (LC-ESI-MS/MS) at the Centro Nacional de Biotecnología (CNB) Proteomics facility (Madrid, Spain). Tandem mass spectra were searched against Araport11 using the MASCOT search engine (Matrix Science, http://www.matrixscience.com/). Peptide sequences identified with a false discovery rate (FDR) < 1% were considered statistically valid. Proteins identified with at least 2 peptides without overlapping sequences (unique peptides) in at least 2 biological replicates (namely, at least 4 peptides) were considered identified with high confidence. To search for potential ABCE2:YFP interactors, proteins whose subcellular localization was not predicted to be cytoplasmic by SUBA4 (https://suba.live/) (Hooper et al., 2014; Hooper et al., 2017) were discarded, with the exception of At2g20830, which is predicted to localize to mitochondria (see Results). To further discard potential false positive interactions, all the proteins identified in three other co-immunoprecipitations of GFP-fused proteins performed in our laboratory under identical conditions to that of ABCE2:YFP, but functionally unrelated, were used to create a subtract list. Proteins identified in ABCE2:YFP samples with at least twice the number of peptides assigned to the same protein in the subtract list were considered enriched. The rest of the proteins, which contained a more similar number of peptides between the ABCE2:YFP list and the subtract list, were considered false positives and discarded. In addition, there were few proteins that were solely identified in ABCE2:YFP samples.
Tandem affinity purification assay
TAP assay of the GSRhino-TAP-tagged ABCE2 fusion from Arabidopsis cell suspension cultures was performed as previously described (Van Leene et al., 2015; García-León et al., 2018). Proteins were identified by nano LC-MS/MS at the CNB. Tandem mass spectra were searched against Araport11 using the MASCOT search engine. Proteins identified with at least 1 unique peptide with a MASCOT score higher than 25 (P < 0.05) were considered to be valid. Proteins identified with at least 1 unique peptide in the 2 biological replicates or 2 unique peptides in 1 biological replicate were considered identified with high confidence. We discarded as putative ABCE2 interactors those proteins that were not predicted to be cytoplasmic by SUBA4.
Bioinformatic analyses
The identity and similarity values between conserved proteins were obtained from global pairwise sequence alignments performed with EMBOSS Needle (https://www.ebi.ac.uk/Tools/psa/emboss_needle/) (Madeira et al., 2019). The multiple sequence alignment of ABCE orthologs was obtained with Clustal Omega (https://www.ebi.ac.uk/Tools/msa/clustalo/) (Madeira et al., 2019).
A TBLASTN search was performed to identify ABCE genes within eudicots (taxid:71240) against the sequences contained in the Nucleotide collection database at the National Center for Biotechnology Information BLASTP server (NCBI; https://blast.ncbi.nlm.nih.gov/Blast.cgi) (Altschul et al., 1997) using Arabidopsis thaliana ABCE2 protein as the query (NP_193656). The phylogenetic analysis was performed using the NCBI accession numbers listed in Supplementary Table S4 with MEGA X software (Kumar et al., 2018): the multiple sequence alignment and the phylogenetic tree were obtained using codon recognition with Muscle (Edgar, 2004b; Edgar 2004a), and the Neighbor-Joining method (Saitou and Nei, 1987), respectively.
Accession numbers
Sequence data can be found at The Arabidopsis Information Resource (https://www.arabidopsis.org/) under the following accession numbers: ABCE1 (At3g13640), ABCE2 (At4g19210), ACT2 (At3g18780), ATHB8 (At4g32880), OTC (At1g75330), and PIN1 (At1g735
Results
The apiculata7-1 mutant exhibits a pleiotropic morphological phenotype
The apiculata7-1 (api7-1) mutant, which we initially named api7, was isolated in a previous large-scale screen for EMS-induced mutations affecting leaf development (Berná et al., 1999). Its pleiotropic morphological phenotype includes a small rosette, a short primary root, and a delay in main stem growth (Figures 1A–E; Supplementary Figure S1A). The api7-1 inflorescences and siliques are seemingly normal (Supplementary Figure S1B–G). The rosette leaves are pointed, indented, and pale, and contain a reduced amount of photosynthetic pigments, compared to its wild-type Ler (Figure 1; Supplementary Figure S1H).
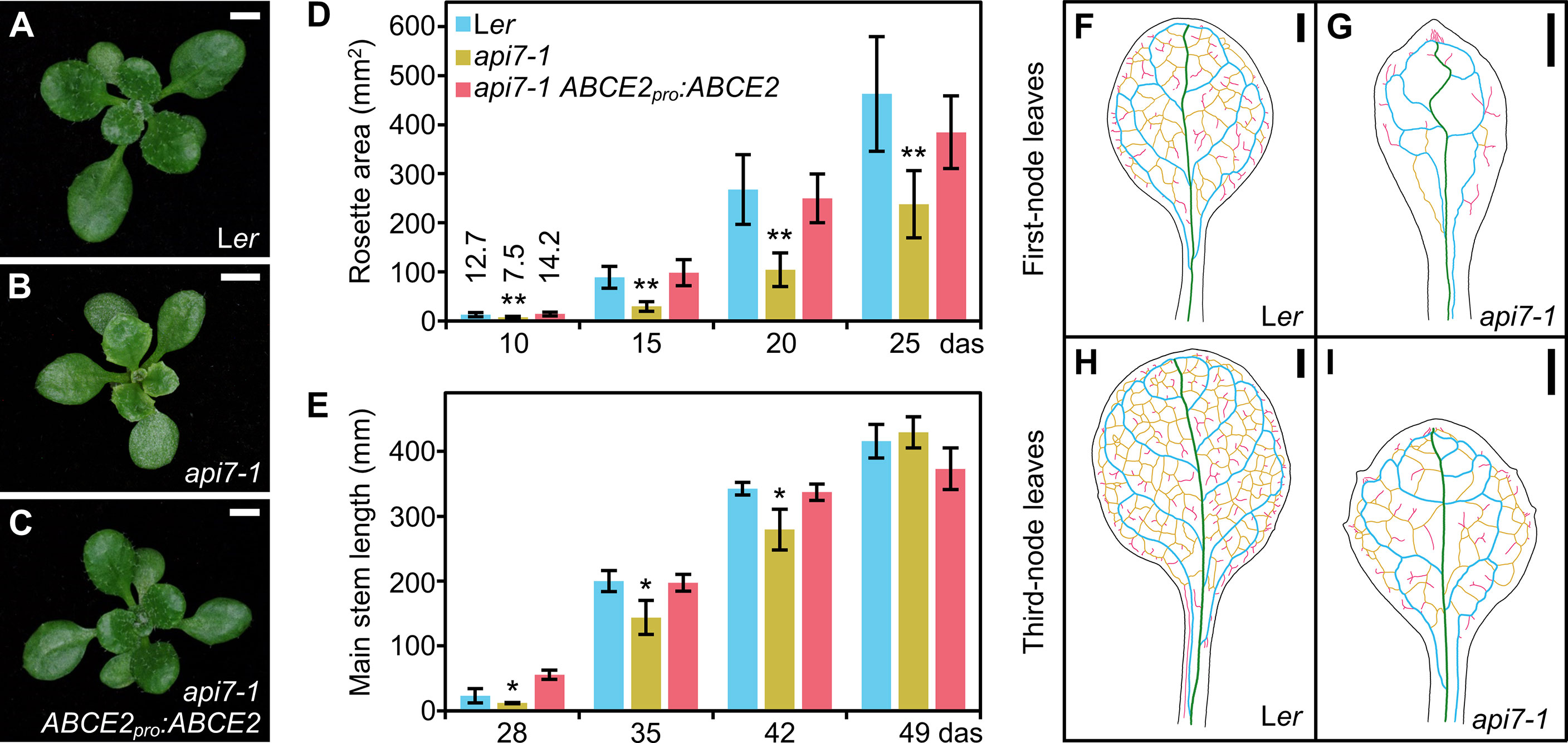
Figure 1 Morphological phenotype of the api7-1 mutant. (A–C) Rosettes from (A) the wild-type Ler, (B) the api7-1 mutant, and (C) an api7-1 ABCE2pro:ABCE2 mutant and transgenic plant. Pictures were taken 16 das. Scale bars indicate 2 mm. (D, E) Growth progression of (D) rosette area and (E) main stem length. (D) Bars indicate mean and (E) median values. Error bars represent (D) standard deviation and (E) median absolute deviation. Asterisks indicate a significant difference with Ler in (D) a Student’s t test (10 < n < 17) or (E) a Mann-Whitney U test (n = 8) (*P < 0.05, **P < 0.001). (F–I) Venation pattern of api7-1 first- and third-node leaves. Representative diagrams of mature (F, G) first- and (H, I) third-node leaves from (F, H) Ler and (G, I) api7-1 plants after visualization of 12 samples per organ and genotype. Margins were drawn in black, primary veins in green, secondary veins in blue, higher-order connected veins in yellow, and higher-order disconnected veins in pink. Organs were collected 21 das. Scale bars indicate 1 mm.
The pleiotropic phenotype of api7-1 plants is reminiscent of mutants carrying loss-of-function alleles of genes encoding ribosomal proteins or ribosome biogenesis factors (Byrne, 2009; Horiguchi et al., 2011; Rosado et al., 2012; Weis et al., 2015; Micol-Ponce et al., 2018). As these mutations usually alter leaf vascular development, we cleared api7-1 and Ler leaves with chloral hydrate, and observed their venation patterns. We confirmed that api7-1 fully expanded first-node and, to a lesser extent, third-node rosette leaves, contain fewer higher-order veins, and more prominent indentations and vascularized hydathodes, particularly in the leaf apex, than Ler leaves (Figures 1F–I; Supplementary Figure S2). In contrast, these phenotypic traits seemed to be unaffected on api7-1 cotyledons, cauline leaves, sepals, and petals (Supplementary Figure S3; Supplementary Table S5). The ARABIDOPSIS THALIANA HOMEOBOX GENE 8 (ATHB8) gene is expressed in pre-procambial cells that will differentiate into veins (Baima et al., 1995). To determine the stage at which api7-1 leaf venation pattern formation diverged from that of Ler, we crossed api7-1 plants to an ATHB8pro:GUS line, and studied the expression of the transgene in cleared first-node rosette leaf primordia of api7-1 ATHB8pro:GUS plants. Consistent with the slow growth phenotype of api7-1 plants, we observed a delay in the emergence of first-node leaves (Supplementary Figure S4). In addition, api7-1 primordia retained high GUS activity at their apical region even after the formation of the whole midvein (Supplementary Figure S4M, N), suggesting that an increased vascular differentiation in that region is responsible of the vascular phenotype of mature api7-1 leaves (Supplementary Figure S2).
Cleared api7-1 first-node leaves also showed a marked reduction in cell size in the abaxial and adaxial epidermal layers, but not in the palisade mesophyll (Figure 2). Indeed, the adaxial epidermis of api7-1 was more similar to its abaxial epidermis than to the adaxial epidermis of the wild-type. This observation suggests that api7-1 leaves are abaxialized, as has been reported for other mutants affected in the translation machinery (Pinon et al., 2008; Yao et al., 2008; Horiguchi et al., 2011; Moschopoulos et al., 2012; Casanova-Sáez et al., 2014; Mateo-Bonmatí et al., 2015; Matsumura et al., 2016). ASYMMETRIC LEAVES 1 (AS1) and AS2 encode transcription factors involved in leaf dorsoventral patterning. Double mutant combinations of as1 or as2 with mutations in genes encoding ribosomal proteins or other components of the translation machinery usually produce synergistic phenotypes. These phenotypes are easily distinguished by the presence of trumpet-shaped (peltate) or radial leaves originated by partial or complete loss of dorsoventrality, respectively (Pinon et al., 2008; Yao et al., 2008; Horiguchi et al., 2011; Moschopoulos et al., 2012; Casanova-Sáez et al., 2014; Mateo-Bonmatí et al., 2015; Matsumura et al., 2016). We obtained api7-1 as1-1 and api7-1 as2-1 double mutants in the Col-0 background; these double mutants exhibited additive and synergistic phenotypes, respectively (Figures 2M–S). The presence of radial leaves in api7-1 as2-1 plants further supports a role for API7 in translation (Figures 2R, S).
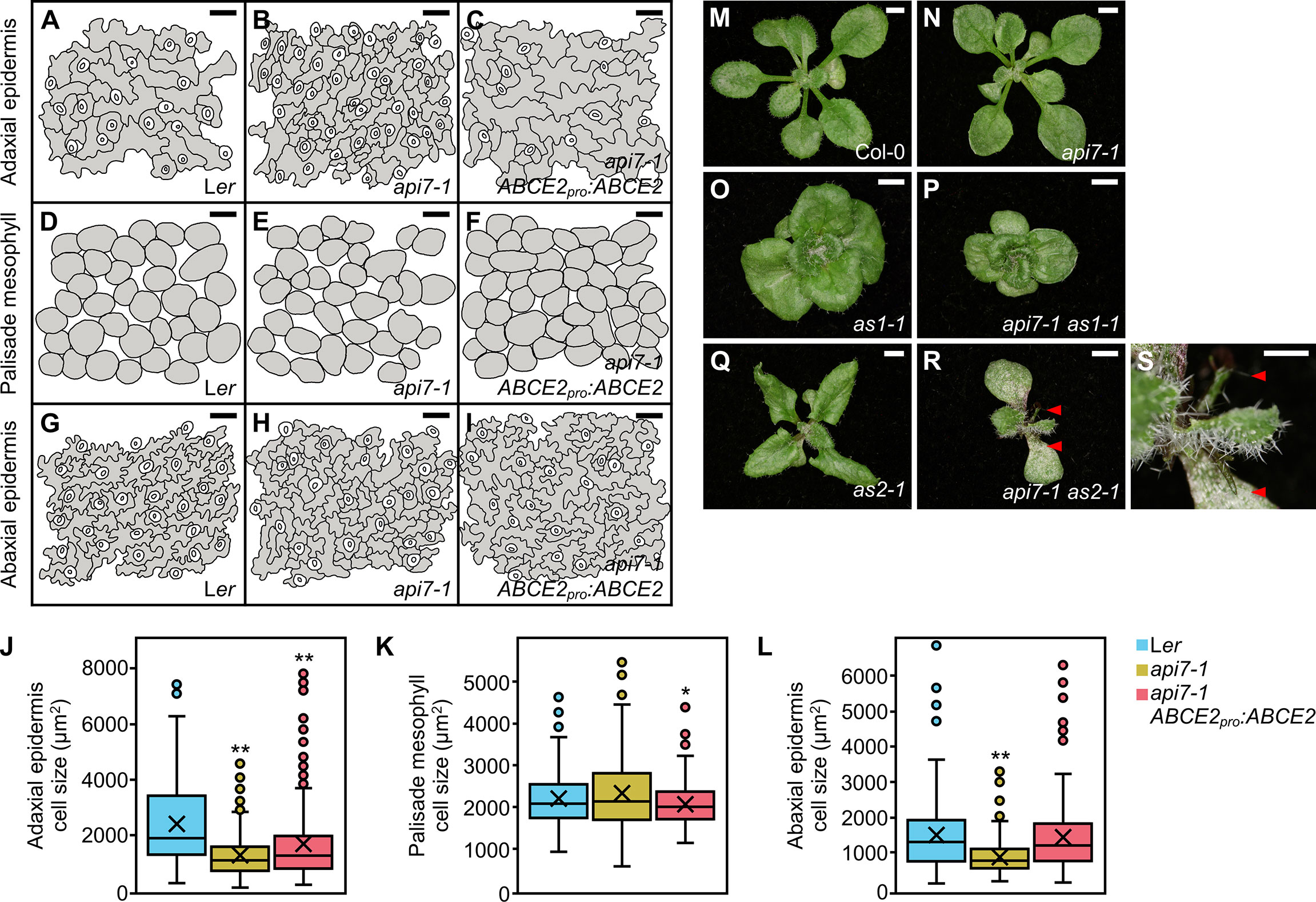
Figure 2 Dorsoventral patterning in api7-1 leaves. (A–L) Leaf cell phenotypes of Ler, api7-1, and api7-1 ABCE2pro:ABCE2 plants. (A–I) Representative diagrams of the (A–C) adaxial epidermis, (D–F) subepidermal layer of palisade mesophyll, and (G–I) abaxial epidermis, from (A, D, J, G) Ler, (B, E, H) api7-1, and (C, F, I) api7-1 ABCE2pro:ABCE2 plants. (J–L) Boxplot distributions of cell area in the (J) adaxial epidermis, (K) subepidermal layer of palisade mesophyll, and (L) abaxial epidermis, from first-node leaves collected 21 das. Other details as described in the legend of Supplementary Figure S1 for its (A) section. Between 144 and 351 cells were analyzed from at least 4 different samples. Asterisks indicate a significant difference with the Ler wild-type in a Student’s t test (*P < 0.05, **P < 0.001). (M–S) Genetic interactions of api7-1 with as1-1 and as2-1. Rosettes from the wild-type (M) Ler, the (N) api7-1, (O) as1-1, and (Q) as2-1 single mutants, and the (P) api7-1 as1-1 and (R) api7-1 as2-1 double mutants. (G) Close up of (F). Red arrowheads indicate radial leaves. Pictures were taken 16 das. Scale bars indicate (M–R) 2 and (S) 1 mm.
api7-1 is a viable mutant allele of the ABCE2 gene
The api7-1 mutation was previously mapped to chromosome 4 (Robles and Micol, 2001). To identify the mutated gene, we combined map-based cloning and next-generation sequencing, as previously described (Mateo-Bonmatí et al., 2014). First, we performed linkage analysis of an F2 mapping population, which allowed us to delimit a candidate interval encompassing 30 annotated genes (Supplementary Figure S5A). We then sequenced the whole api7-1 genome and identified 4 EMS-type nucleotide substitutions within the candidate interval (Supplementary Table S6). Only one of these, a C→T transition in At4g19210, was predicted to be a missense mutation causing a Pro138→Ser substitution (Supplementary Figure S5B). The At4g19210 gene encodes ABCE2, a protein of 605 amino acids (68.39 kDa). The Pro138 residue, at the beginning of the HLH motif located within NBD1, is conserved across all eukaryotic ABCE proteins tested, except in Caenorhabditis elegans (Supplementary Figure S6), in which it seems to have evolved more divergently (Chen et al., 2006). The conservation of this residue suggests that it is necessary for the proper function of ABCE proteins, probably for the interactions with the ribosome, which mainly occur through the HLH and hinge motifs (Heuer et al., 2017; Nürenberg-Goloub et al., 2020; Kratzat et al., 2021).
To confirm that the mutation found in At4g19210 causes the phenotype of the api7-1 mutant, we obtained the ABCE2pro:ABCE2 transgene, which was transferred into api7-1 plants. This transgene completely restored the wild-type rosette leaf shape and stem height (Figures 1C–E), as well as the photosynthetic pigment content (Supplementary Figure S1H). The ABCE2pro:ABCE2 transgene partially restored leaf epidermal cell sizes and root length (Figure 2; Supplementary Figure S1A). To provide further confirmation that api7-1 is an allele of ABCE2, we performed an allelism test using GABI_509C06 plants (Kleinboelting et al., 2012), which were heterozygous for a T-DNA insertion in the 10th exon of At4g19210 (Supplementary Figure S5B). We named api7-2 the insertional allele in GABI_509C06. In the F2 population of this cross, no api7-2/api7-2 plants were found, and api7-1/api7-2 and api7-1/api7-1 plants were phenotypically similar, confirming that these mutations are allelic and that loss of function of ABCE2 is responsible for the phenotype of the api7-1 mutant (Supplementary Figure S7A–C).
The absence of api7-2/api7-2 plants derived from GABI_509C06 seeds, and of ungerminated seeds in the F1 progeny of selfed heterozygous ABCE2/api7-2 plants, suggested an early lethality of this mutant allele. We dissected immature siliques from ABCE2/api7-2 plants and found 21.95% aborted seeds (n = 328), which fits a 1:3 Mendelian segregation ratio (χ2 = 1.63; P = 0.202; degrees of freedom = 1). Col-0 siliques showed 1.37% aborted ovules (n = 148; Supplementary Figure S7D, E). The lethality caused by api7-2 suggests that it is a null allele of ABCE2, while api7-1 is hypomorphic.
The Arabidopsis genome contains two partially redundant ABCE paralogs
To gather information about the origin of the two Arabidopsis ABCE paralogs, we performed a phylogenetic analysis of ABCE coding sequences from some Rosidae species (rosids; Supplementary Figure S8). Among them, we found that other Brassicaceae genomes also encode ABCE1 and ABCE2 proteins, but only ABCE2 was identified in Cardamine hirsuta. Consistent with the whole-genome triplication in Brassica rapa (Zhang et al., 2018), we found two and three Brassica rapa ABCE1 and ABCE2 sequences, respectively. All Brassicaceae ABCE1 genes grouped together in the phylogenetic tree, and separately from their ABCE2 paralogs, which formed other subclade. Although both ABCE1 and ABCE2 paralogs have been conserved, ABCE1 orthologs have evolved more rapidly than their ABCE2 paralogs, whose short evolutionary distances indicate that they are under strong evolutionary pressure, as expected for an essential gene.
As previously described (Braz et al., 2004; Sarmiento et al., 2006), we observed that ABCE2 is highly expressed throughout all Arabidopsis developmental stages. By contrast, the expression levels of its ABCE1 paralog are very low in all studied organs, in which first-node leaves and flowers show the lowest and highest expression levels, respectively (Supplementary Figure S9A, B). The expression level of ABCE1 in api7-1 rosettes was the same as in Ler, showing that ABCE1 cannot compensate for the partial loss of ABCE2 function in rosettes (Supplementary Figure S9C). However, api7-1 flowers, where we observed the highest ABCE1 expression levels, do not show apparent aberrations (Supplementary Figure S1C), suggesting that ABCE1 and ABCE2 might play similar roles during flower development.
The ABCE1 and ABCE2 proteins share 80.8% identity, suggesting that ABCE1 and ABCE2 might be functionally equivalent. To test this hypothesis, we performed a promoter swapping assay between the ABCE1 and ABCE2 genes (Figure 3). As expected from the lower expression levels driven by the ABCE1 promoter, api7-1 ABCE1pro:ABCE2 plants were indistinguishable from api7-1 mutants, highlighting that correct protein levels are as important as the correct sequence for normal ABCE2 function. In contrast, the ABCE2pro:ABCE1 transgene partially rescued the api7-1 phenotype, showing that the ABCE1 and ABCE2 proteins are functionally redundant. Further supporting equivalent functions for ABCE1 and ABCE2, the constitutive expression of ABCE1 with a 35Spro:ABCE1 transgene fully restored a wild-type phenotype in api7-1 rosettes (Supplementary Figure S10).
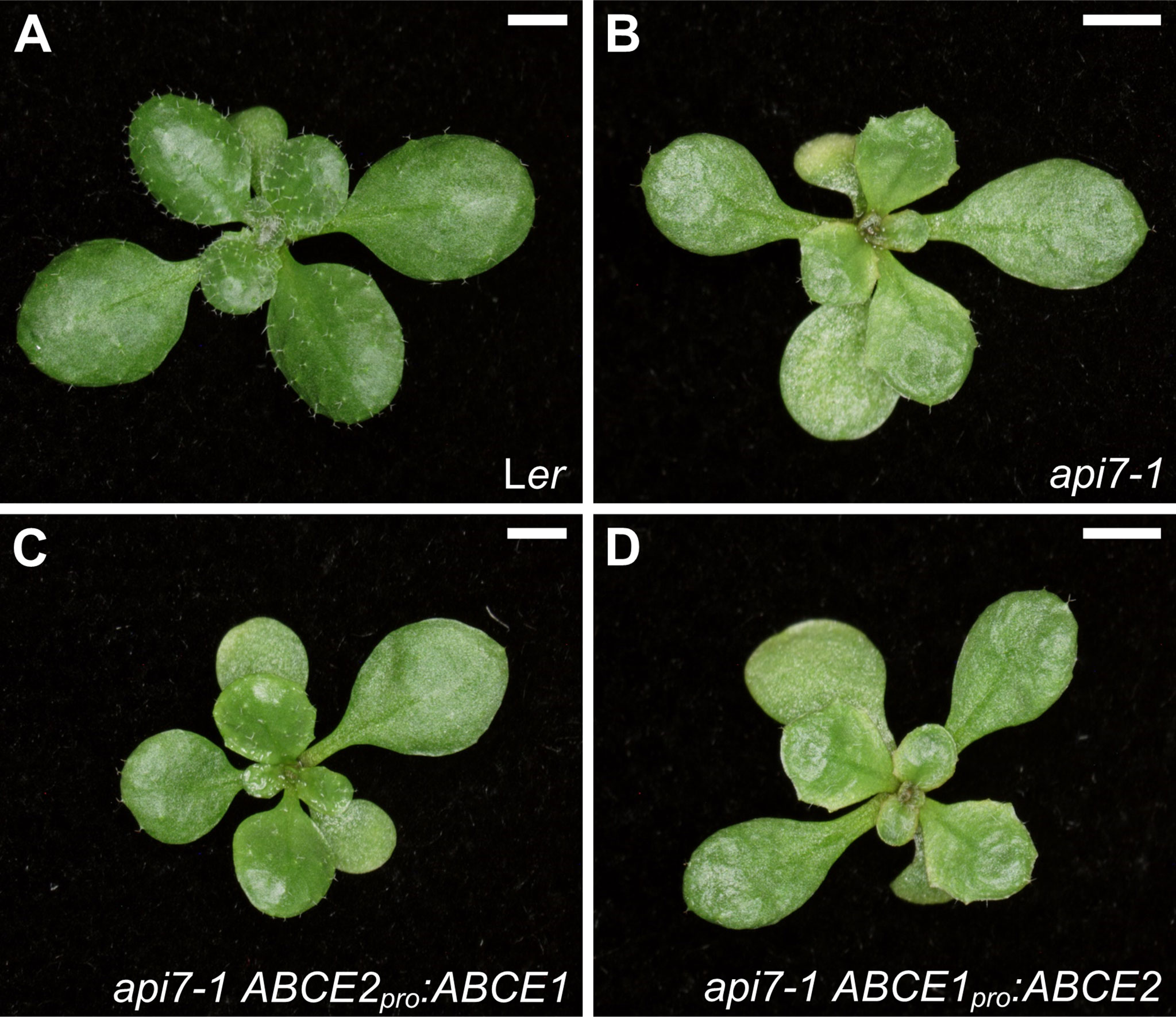
Figure 3 Effects of the ABCE2pro:ABCE1 and ABCE1pro:ABCE2 transgenes on the morphological phenotype of the api7-1 mutant. Rosettes from (A) Ler, (B) api7-1, (C) api7-1 ABCE2pro:ABCE1, and (D) api7-1 ABCE1pro:ABCE2 plants. Pictures were taken 14 das. Scale bars indicate 2 mm.
ABCE2 is a cytoplasmic protein that physically associates with components of the translation machinery
To determine the subcellular localization of Arabidopsis ABCE2, we obtained in-frame translational fusions of ABCE2 to GFP and YFP, driven by the 35S promoter: 35Spro:ABCE2:GFP and 35Spro:ABCE2:YFP. We visualized the ABCE2:GFP fusion protein in the cytoplasm of root cells treated with propidium iodide, which mainly stains cell walls, and the ABCE2:YFP fusion protein in roots stained with the nucleoplasm dye DAPI, and confirmed the nuclear exclusion of ABCE2 (Figure 4).
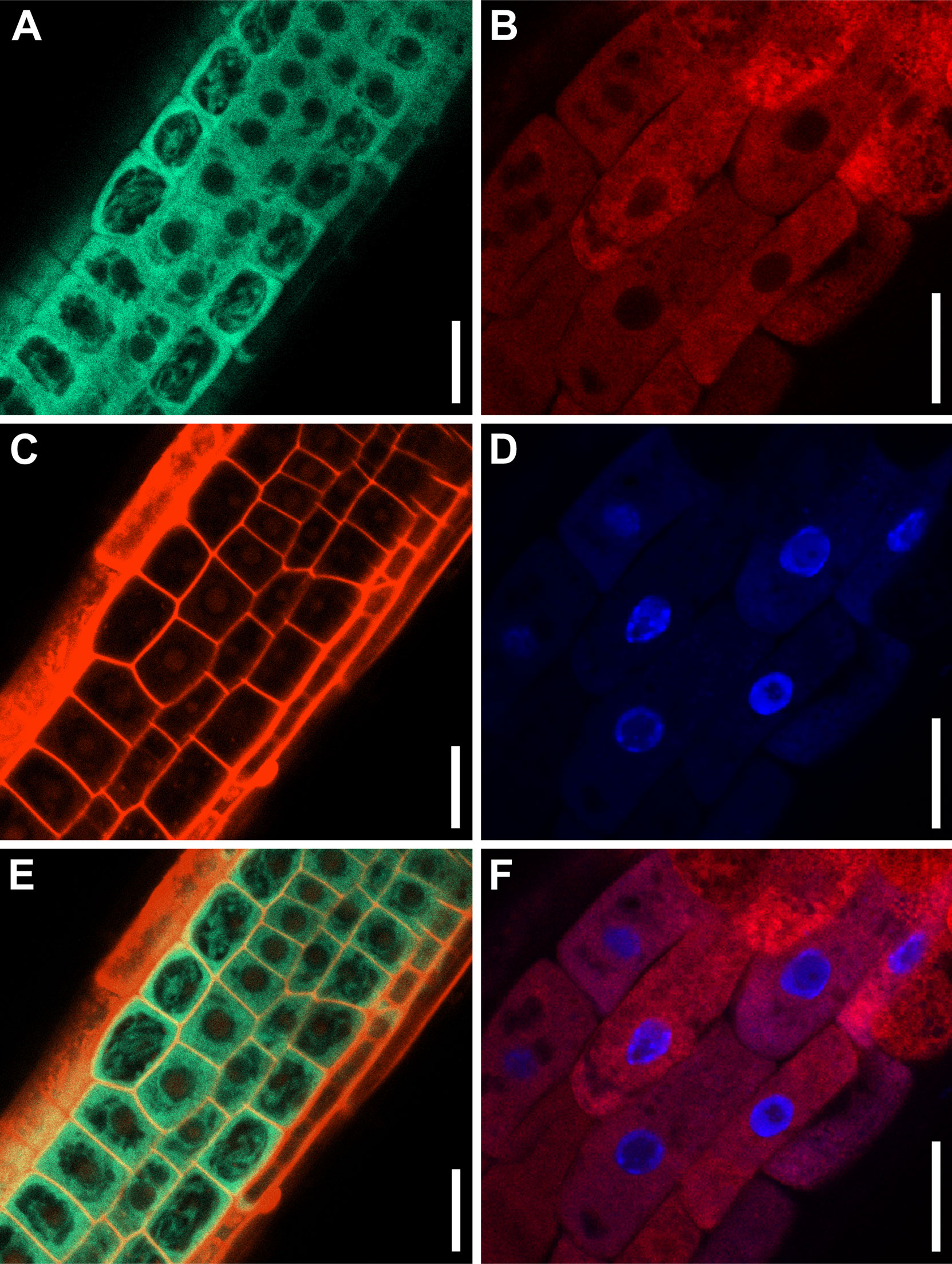
Figure 4 Subcellular localization of the ABCE2 protein in cells from the root elongation zone. Confocal laser scanning micrographs of (A, C, E) Ler 35Spro:ABCE2:GFP and (B, D, F) api7-1 35Spro:ABCE2:YFP transgenic plants. Fluorescent signals correspond to (A) GFP, (B) YFP, (C) propidium iodide, and (D) DAPI staining, and the overlay of (E) GFP and propidium iodide, and (F) YFP and DAPI. Pictures were taken (A, C, E) 14 and (B, D, F) 5 das. Scale bars indicate 20 μm.
To investigate the function of ABCE2, we performed a co-immunoprecipitation assay using the ABCE2:YFP protein from a homozygous T3 api7-1 35Spro:ABCE2:YFP line, which was phenotypically wild-type, confirming that the fusion protein is functional (Supplementary Figure S11A–C). We checked the purification of the fusion protein by western blotting using an anti-GFP antibody (Supplementary Figure S11D–F). Using LC-ESI-MS/MS, we identified 20 putative interactors of ABCE2, of which 13 participate in translation (6 subunits of the eIF3 complex, eIF5B, RPL3B, and ROTAMASE CYP 1 [ROC1]) or in its regulation (At5g58410, EVOLUTIONARILY CONSERVED C-TERMINAL REGION 2 [ECT2], ILITYHIA [ILA], and REGULATORY-ASSOCIATED PROTEIN OF TOR 1 [RAPTOR1] or RAPTOR2), and two others had previously been shown to interact with ABCE2 orthologs (At2g20830, and EXPORTIN 1A [XPO1A] or XPO1B). The functions of the remaining 5 proteins that co-immunoprecipitated with ABCE2 are unclear and these proteins were therefore set aside for future characterization (Figure 5; Supplementary Figure S12; Supplementary Tables S7, S8; Supplementary Data Set 1).
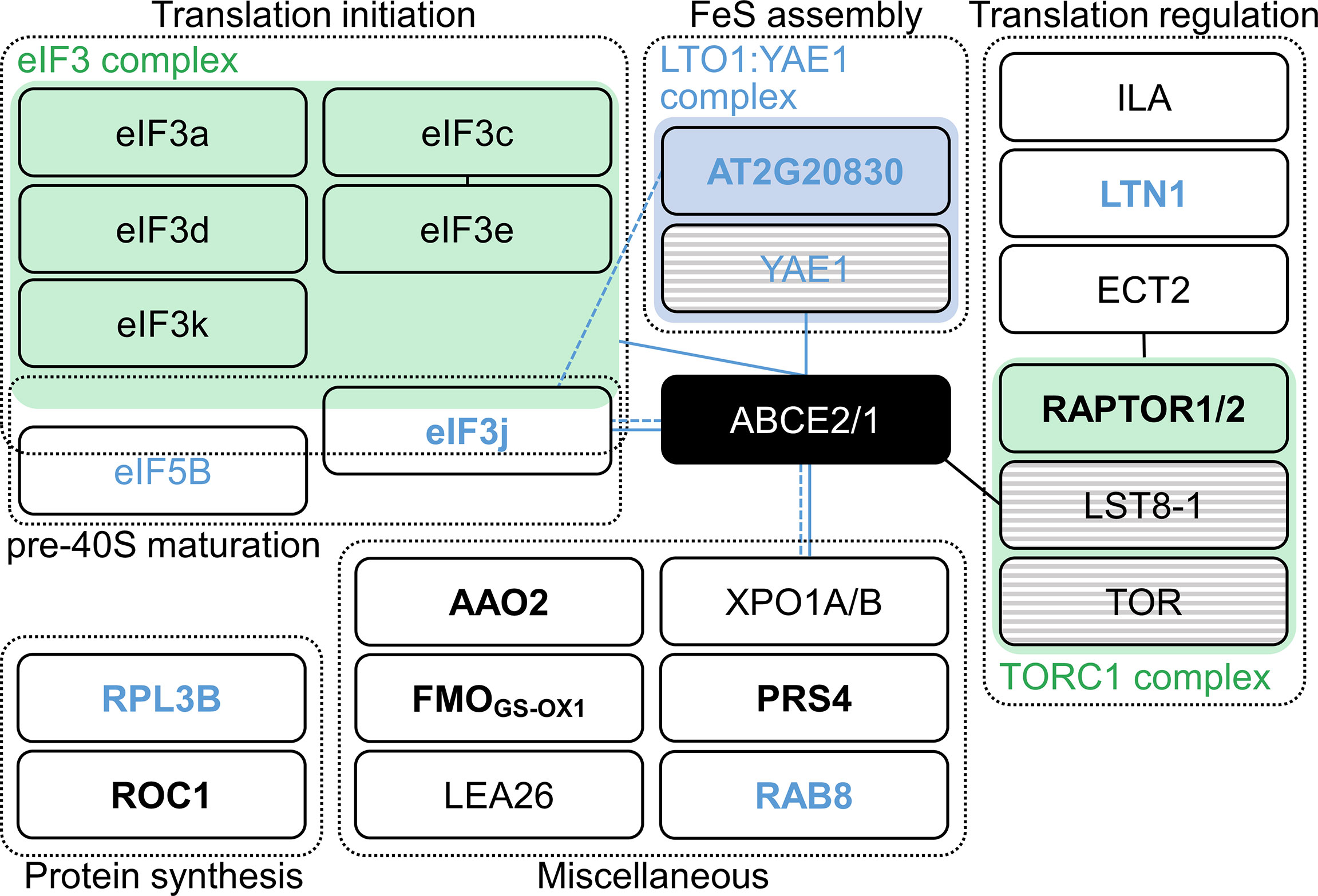
Figure 5 Proteins identified in an ABCE2:YFP co-immunoprecipitation assay. Proteins were grouped within dashed boxes according to their annotated functions for Arabidopsis (names in black letters) or orthologous (names in blue letters) proteins. Green and blue boxes represent complexes that have been described in Arabidopsis and other species, respectively. Proteins in striped boxes were not identified in our assay but have been included in this Figure because they are known to belong to a given complex. Continuous and dashed lines connecting boxes indicate physical and genetic interactions described elsewhere for Arabidopsis (black) or other species (blue), respectively. For references, see Supplementary Table S8. Names in bold and plain letters indicate proteins unique to or enriched in ABCE2:YFP samples, respectively.
At2g20830 encodes a folic acid binding/transferase that shares 30.2% and 26.7% identity with human and Saccharomyces cerevisiae Lto1 (named after “required for biogenesis of the large ribosomal subunit and initiation of translation in oxygen”), respectively (human and S. cerevisiae Lto1 proteins share 27.8% identity). Lto1, together with Yae1, constitute an essential complex for FeS cluster assembly on ABCE1 (Zhai et al., 2014; Paul et al., 2015; Zhu et al., 2020; Prusty et al., 2021). Despite the observation that At2g20830 protein was predicted to localize to mitochondria, the conservation level of this protein with its yeast and human Lto1 orthologs prompted us to consider At2g20830 an ABCE2 interactor. Indeed, At2g20830 may be necessary for FeS cluster assembly on ABCE2.
Our co-immunoprecipitation assay suggested that Arabidopsis ABCE2 interacts with 6 of the 13 eIF3 subunits: eIF3a, c, d, e, k, and j. In S. cerevisiae, those interactions have been related to the presence of the ABCE1 protein in the 40S subunit after ribosome dissociation, until late steps of initiation of a new cycle of translation (Heuer et al., 2017; Mancera-Martínez et al., 2017; Kratzat et al., 2021). Interestingly, the interaction between the non-stoichiometric subunit eIF3j and ABCE1 also occurs in humans and S. cerevisiae. In these species, eIF3j acts as an accessory factor for ABCE1-mediated ribosome dissociation (Young and Guydosh, 2019; Kratzat et al., 2021), a function that seems to be conserved in Arabidopsis.
To corroborate and extend the list of interactions between ABCE2 and components of the translation machinery, we performed a tandem affinity purification (TAP) assay of a GSRhino-TAP-tagged ABCE2 bait, obtained from cell suspension cultures, and identified its putative interactors by nano LC-MS/MS (Supplementary Data Set 2). We found that 81 proteins co-purified with ABCE2, of which 28 were ribosomal proteins (Supplementary Data Set 2E).
The api7-1 mutation perturbs auxin metabolism
To gain insight into the biological processes affected in the api7-1 mutant, we performed an RNA-seq analysis of Ler and api7-1 shoots collected 14 das. We identified 3218 downregulated and 2135 upregulated genes in the api7-1 mutant (Supplementary Data Set 3A). A gene ontology (GO) enrichment analysis performed separately for down- and upregulated genes showed that the downregulated genes were mainly related to responses to abiotic and biotic stresses and protein post-translational modifications. In contrast, upregulated genes grouped into more diverse Biological Process terms (Supplementary Data Set 3B, C). Among them, we found three terms related to auxin (response to auxin [GO:0009733], auxin-activated signaling pathway [GO:0009734], and auxin polar transport [GO:0009926]).
We observed that four out of the six genes that participate in the main auxin biosynthesis pathway in shoots were upregulated. They included two of the three genes encoding enzymes that convert tryptophan (Trp) into indole-3-pyruvic acid (IPyA), TRYPTOPHAN AMINOTRANSFERASE OF ARABIDOPSIS 1 (TAA1), and TAA1-RELATED 2 (TAR2), and two YUCCA genes (YUC2 and YUC6) encoding enzymes that turn IPyA into indole-3-acetic acid (IAA) (Cheng et al., 2007; Casanova-Sáez et al., 2021; Kneuper et al., 2021). However, the expression of two genes involved in a secondary pathway for IAA biosynthesis, CYTOCHROME P450, FAMILY 79, SUBFAMILY B, POLYPEPTIDE 2 (CYP79B2) and IAMHYDROLASE12 (IAMH2) were downregulated (Gao et al., 2020). We also found that four genes involved in auxin inactivation, IAA CARBOXYLMETHYLTRANSFERASE 1 (IAMT1), GRETCHEN HAGEN 3.17 (GH3.17), DIOXYGENASE FOR AUXIN OXIDATION 2 (DAO2), and UDP-GLYCOSYLTRANSFERASE 76E5 (UGT76E5) were upregulated, and that three genes involved in auxin reactivation, IAA-LEUCINE RESISTANT (ILR)-LIKE 2 (ILL2), ILL3, and ILL4 (Takubo et al., 2020; Casanova-Sáez et al., 2021; Hayashi et al., 2021; Mateo-Bonmatí et al., 2021), were downregulated, probably in response to high auxin levels (Figure 6A). In this manner, our transcriptional data point to an increase in auxin biosynthesis in api7-1 shoots, which might be partially or fully compensated by reducing the synthesis rate in secondary pathways, and by inactivating and preventing the reactivation of IAA.
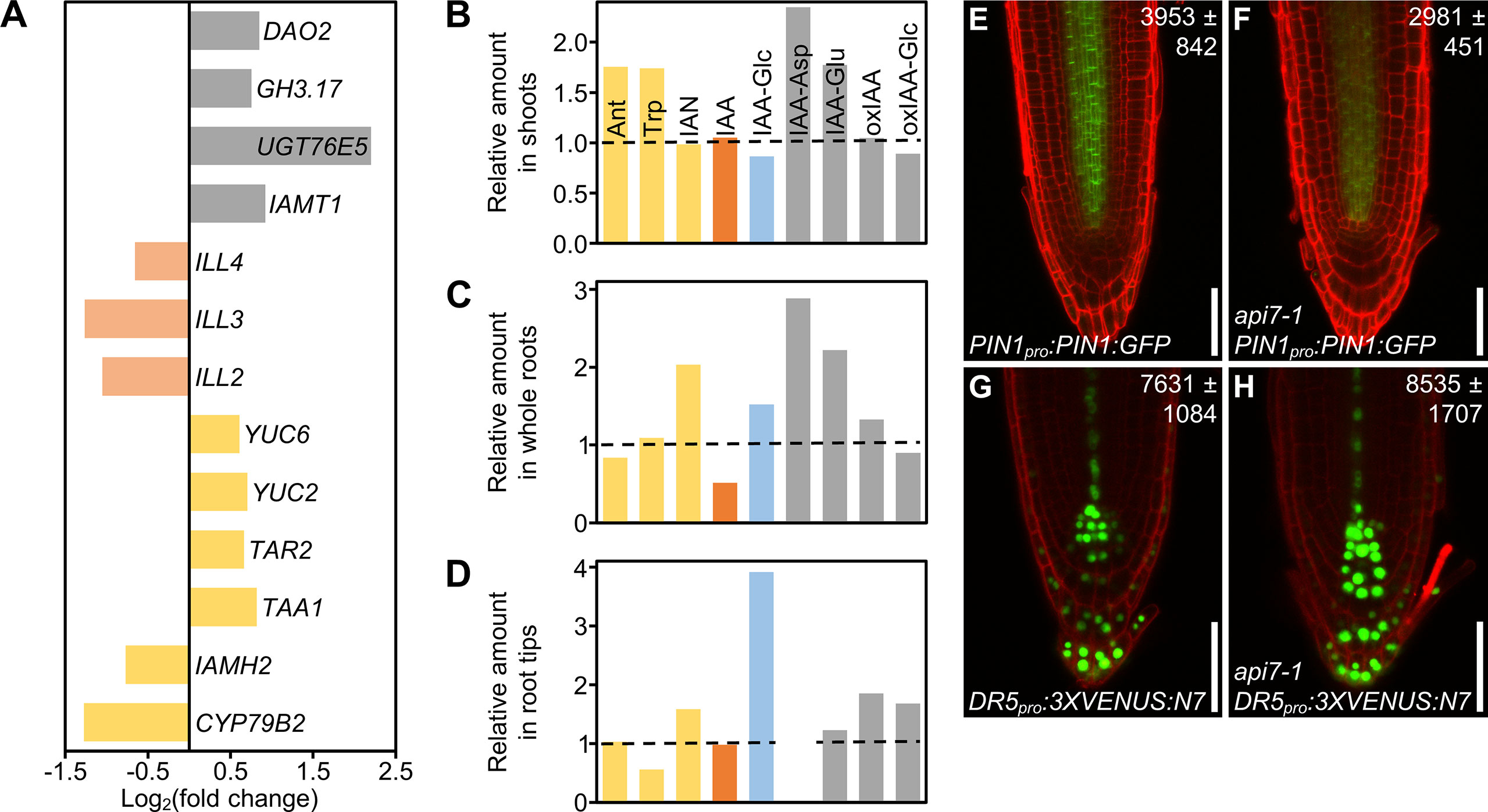
Figure 6 Auxin metabolism, transport and signaling are altered in api7-1 plants. (A) Expression levels of genes related to auxin metabolism (biosynthesis, yellow; activation, pale orange; storage and catabolism, grey) in api7-1 shoots 14 das. Values are shown as the binary logarithm of the fold change between api7-1 and Ler mean reads. Mean reads were calculated from three biological replicates. (B–D) Relative amounts of some IAA precursors (yellow; Ant, anthranilate; Trp, tryptophan; IAN, indole-3-acetonitrile), IAA (orange), the IAA storage molecule IAA-Glc (glycosylated IAA; blue), and IAA catabolites (grey; IAA-Asp and IAA-Glu, IAA conjugated to aspartate and glutamate, respectively; oxIAA, 2-oxindole-3-acetic acid; oxIAA-Glc, glycosylated 2-oxindole-3-acetic acid) in api7-1 (B) shoots, (C) whole roots, and (D) root tips 9 das. IAA-Asp was not detected in root tips. The mean amounts of each metabolite in Ler were used as the reference value (dashed lines; see Supplementary Figure S14). Mean amounts were calculated from four biological replicates. (E–H) Visualization of the expression of reporter transgenes for auxin (E, F) transport and (G, H) perception, in (E, G) wild-type and (F, H) api7-1 roots. Cell walls were stained with propidium iodide. Values indicate average fluorescence intensities ± standard deviation from (E, F) GFP and (G, H) VENUS, which are significantly different from the wild type in a Student’s t test [P < 0.001, (E, F) n = 25; P < 0.05, (G, H) n = 27]. Pictures were taken 5 das. Scale bars indicate 50 µm.
To directly assess our hypothesis, we checked the content of indol-3-acetic acid (IAA), the main auxin in most plants, as well as some of its precursors and inactive forms in api7-1 and Ler shoots, whole roots and root tips. We found a similar trend within the three tissues: an increase in IAA catabolism, as suggested by the RNA-seq results, and an accumulation of its precursors, when compared to Ler tissues (Figures 6B–D; Supplementary Figure S13). The inactivation of IAA in api7-1 shoots and whole roots mainly occurs through glutamate (IAA-Glu) and aspartate (IAA-Asp) conjugation, while in root tips occurs through IAA oxidation (oxIAA), and subsequent glycosylation (oxIAA-glc). api7-1 shoots accumulate Ant, a substrate for Trp biosynthesis, and Trp itself, the main precursor for IAA biosynthesis. Whole roots and root tips accumulate IAN, another IAA precursor, and store the inactive glycosylated IAA (IAA-glc). The IAA levels were normal in shoots and root tips, suggesting that auxin homeostasis is maintained in api7-1. However, the IAA levels in whole roots were decreased by almost 50%, maybe due to its high inactivation levels. Trp levels were low in root tips, suggesting that it might be converted to IAN, which is overaccumulated, or to IAA, which seems to be stored and catabolized to maintain its normal levels.
In agreement with the reduced levels of IAA in api7-1 whole roots, the levels of a fusion protein between the auxin exporter PIN-FORMED1 (PIN1) and GFP (PIN1:GFP) in api7-1 PIN1pro:PIN1:GFP roots were lower than in Ler roots (Figures 6E, F; Supplementary Figure S14A). In addition, we observed that the expression of the synthetic auxin-responsive promoter DR5 in api7-1 DR5pro:3XVENUS:N7 root tips, measured as the fluorescence intensity of 3XVENUS:N7, was slightly increased in comparison to Ler DR5pro:3XVENUS:N7 root tips, indicating that auxin signaling might be also altered in api7-1 (Figures 6G,H; Supplementary Figure S14B).
Genes related to iron homeostasis are deregulated in api7-1 plants
Interestingly, we also found in our RNA-seq assay that iron ion homeostasis and transport (GO:0055072 and GO:0006826), and response to iron and sulfur ion starvation (GO:0010106 and GO:0010438) terms were among the most enriched in the analysis of upregulated genes (Supplementary Data Set 3B). For instance, genes related to iron uptake, such as IRON-REGULATED TRANSPORTER 1 (IRT1) and FERRIC CHELATE REDUCTASE DEFECTIVE 1 (FRD1) (Eide et al., 1996; Robinson et al., 1999), or to iron mobility, such as NATURAL RESISTANCE-ASSOCIATED MACROPHAGE PROTEIN 3 (NRAMP3) and NRAMP6 (Lanquar et al., 2005; Li et al., 2019), and several genes encoding transcription factors induced by iron and sulfur deficiencies were upregulated in the api7-1 mutant (Supplementary Figure S15). These pathways might be activated in api7-1 plants to provide iron and sulfur for FeS cluster biogenesis, probably to compensate for the depletion in ABCE2 protein. Indeed, the gene that encodes the Arabidopsis NEET protein (termed after its conserved Asn-Glu-Glu-Thr sequence near its C-terminus) (Colca et al., 2004), which participates in FeS cluster transference during its biogenesis (Nechushtai et al., 2012; Zandalinas et al., 2020), was also upregulated (Supplementary Figure S15).
Consequently, the iron content in api7-1 cells might be higher than in the wild type, and might be inducing the formation of reactive oxygen species (ROS), as occurs in mutants affected in free iron storage (Briat et al., 2010). In agreement with this assumption, several terms related to oxidative stress responses were also enriched. Specifically, we found that FERRITIN 2 (FER2) and FER3, which encode iron storage proteins in response to high iron levels to avoid oxidative damage (Briat et al., 2010; Reyt et al., 2015), were upregulated (Supplementary Figure S15). In addition, previous studies have shown that ROS prevent FeS cluster assembly into ABCE proteins, which is necessary for their activity in ribosome recycling (Alhebshi et al., 2012; Sudmant et al., 2018; Zhu et al., 2020). In this manner, api7-1 plants might experience a positive feedback loop where a response to iron starvation due to reduced activity of ABCE2 increases iron levels, inducing the production of ROS which, in turn, further disturbs ABCE2 activity. Nevertheless, further studies are needed to ascertain a potential relation among ABCE2 activity, iron homeostasis, and oxidative stress, which were beyond the scope of this work.
Discussion
Plant ABCE proteins participate in translation in a cross-kingdom conserved manner
In this work, we studied Arabidopsis ABCE2, one of the most conserved proteins among archaea and eukaryotes (Hopfner, 2012). Archaea, fungi, and animal ABCE proteins dissociate cytoplasmic ribosomes into their 30S/40S and 50S/60S subunits at different translation-related events (Nürenberg-Goloub and Tampé, 2019). After ribosome dissociation, an ABCE escorts the 30S/40S subunit until the late steps of translation initiation, preventing premature joining of the 50S/60S subunit into the preinitiation complex (Heuer et al., 2017; Nürenberg-Goloub et al., 2020).
The crosslinking performed on the tissue used for the ABCE2 co-immunoprecipitation assay did not allow us to discern direct from indirect ABCE2 interactors. However, the interactions with XPO1A/B, eIF3j, and the protein encoded by At2g20830 are very likely to be direct, in agreement with previous studies in non-plant species (Kirli et al., 2015; Paul et al., 2015; Young and Guydosh, 2019; Kratzat et al., 2021). In contrast, the interactions observed with other eIF3 subunits, RPL3B, ROC1, ECT2, ILA, RAPTOR1/2, and the protein encoded by At5g58410, which is annotated as E3 ubiquitin-protein ligase listerin (LTN1; UniProt code: Q9FGI1) might occur indirectly as they are part of or interact with the translation machinery (Coaker et al., 2006; Shao et al., 2013; Kashima et al., 2014; Sesma et al., 2017; Wang et al., 2017; Arribas-Hernández et al., 2018; Faus et al., 2018; Izquierdo et al., 2018; Scutenaire et al., 2018; Wei et al., 2018). However, the interaction between ABCE2 and eIF5B, which does not seem to occur in S. cerevisiae and mammals (Heuer et al., 2017; Mancera-Martínez et al., 2017), will require further exploration.
Further supporting a role for ABCE2 in translation, we observed a synergistic interaction in the api7-1 as2-1 double mutant, which shows radial leaves, as previously described for double mutant combinations of loss-of-function alleles of AS1 or AS2 and other components of the translation machinery (Pinon et al., 2008; Yao et al., 2008; Horiguchi et al., 2011; Moschopoulos et al., 2012; Casanova-Sáez et al., 2014; Mateo-Bonmatí et al., 2015). In this manner, our results suggest that Arabidopsis and, by extension, all plant ABCEs, probably dissociate cytoplasmic ribosomes, as has been reported for species of other kingdoms (Nürenberg-Goloub and Tampé, 2019). In addition, previous works also support a conserved role for the Arabidopsis ABCE2 and human ABCE1 proteins as suppressors of RNA silencing (Braz et al., 2004; Sarmiento et al., 2006; Kärblane et al., 2015; Mõttus et al., 2020). However, we did not find any ABCE2 interactor potentially involved in this process, nor any enriched ontology term related to gene silencing in our RNA-seq assay. This might be due to the need for a cellular environment that triggers RNA silencing and exposes this novel function of ABCE proteins. Further research will help to assess a potential relationship between ribosome recycling and RNA silencing.
The developmental defects of the api7-1 mutant have different causes
The essential function of ABCEs has been confirmed in several species: null alleles of ABCE genes in all studied organisms are lethal, while hypomorphic alleles cause severe growth aberrations (Navarro-Quiles et al., 2018). In this work, we describe the first hypomorphic and null alleles of the Arabidopsis ABCE2 gene, api7-1 and api7-2, respectively. We showed that the api7-2 mutation is lethal and that api7-1 plants share developmental defects with other mutants affected in genes encoding ribosomal proteins or ribosome biogenesis factors. These phenotypic traits include an aberrant leaf venation pattern (Horiguchi et al., 2011), as is the case for the api7-1 mutant. Indeed, a mutant allele of SIL3, the Cardamine hirsuta ABCE ortholog, also causes venation pattern defects which may be related to an aberrant auxin transport and signaling (Kougioumoutzi et al., 2013).
In agreement with the involvement of local auxin biosynthesis, polar transport and signalling in vascular development (Verna et al., 2019; Kneuper et al., 2021), we observed that auxin metabolism and auxin-induced genes were upregulated in the api7-1 mutant. In addition, a previous study found that the IAA content in api7-1 seedlings was slightly reduced when compared to Ler (Pěnčík et al., 2018). In our experimental conditions, IAA levels in api7-1 shoots and root tips were normal, but reduced in whole roots. However, the general accumulation of IAA precursors and catabolites in api7-1 seedlings suggests that, despite auxin metabolism in api7-1 is perturbed, auxin homeostasis is maintained through different compensation mechanisms, like occurs in other mutants affected in IAA metabolism (Mellor et al., 2016; Porco et al., 2016; Zhang et al., 2016). In this sense, the altered levels of IAA precursors and catabolites, and the deregulation of auxin signalling might contribute to the aberrant phenotype of api7-1 plants. Our transcriptomic results also point to the deregulation of additional biological pathways as potential contributors to the api7-1 phenotype: one of them might be an increased production of ROS, caused by a potential deregulation of iron and sulfur homeostasis.
The Arabidopsis ABCE1 and ABCE2 proteins are functionally redundant
Arabidopsis has two ABCE paralogs, ABCE1 and ABCE2 (Sánchez-Fernández et al., 2001; Verrier et al., 2008). In agreement with previous literature (Braz et al., 2004; Sarmiento et al., 2006), we observed that ABCE1 expression levels are low in all studied organs and throughout development, in contrast to the high expression of ABCE2. We also showed that ABCE1 is unable to complement ABCE2 dysfunction in api7-1 rosettes per se.
However, the wild-type phenotype of api7-1 flowers, where we found the highest expression levels of ABCE1, and the ability of ABCE2pro:ABCE1 and 35Spro:ABCE1 to complement the api7-1 mutant phenotype, indicate that the ABCE1 protein is functional and that it may contribute to translation in the reproductive tissues of wild-type plants. In addition, our phylogenetic analysis showed that the ABCE duplication event occurred early during the evolution of Brassicaceae, and that at least five species from this clade conserved an ABCE1 gene that evolved more rapidly than its ABCE2 paralog, suggesting that ABCE2 conserved the ancestral function, whereas ABCE1 underwent hypofunctionalization (Veitia, 2017).
ABCE proteins are encoded by a single gene in most species, and they are essential for archaea and eukaryotes (Navarro-Quiles et al., 2018). Due to their importance, the molecular mechanisms by which they participate in ribosome recycling have been deeply studied, and remain a subject of intense research (Heuer et al., 2017; Mancera-Martínez et al., 2017; Nürenberg-Goloub et al., 2018; Nürenberg-Goloub et al., 2020; Kratzat et al., 2021). Nevertheless, the biological consequences of ABCE depletion or disruption are poorly understood in all organisms. In this sense, future research linking the molecular function of ABCEs with the phenotypic output of their dysfunction will contribute to determining the pathways through which translation modulates development, as we show here with the isolation and study of the hypomorphic and viable api7-1 allele of the Arabidopsis ABCE2 gene.
Data availability statement
The raw data from genome resequencing and RNA-seq were deposited in the Sequence Read Archive (https://www.ncbi.nlm.nih.gov/sra/) database under accession numbers SRP065876 and PRJNA719000, respectively. The mass spectrometry proteomics data from the co-immunoprecipitation and TAP assays have been deposited to the ProteomeXchange Consortium via the PRIDE (Perez-Riverol et al., 2022) partner repository with the dataset identifiers PXD036412 and PXD036626, respectively.
Author contributions
JLM conceived, designed, and supervised the research, provided resources, and obtained funding. Several experiments were codesigned by CN-Q, EM-B, and JLM, CN-Q performed most of the experiments. EM-B obtained the ABCE2pro:ABCE2 and 35Spro:ABCE2:GFP transgenes, and contributed to the phenotypic analysis of api7-1. EM-B and HC obtained the api7-1 as double mutants. CN-Q and HC performed the phylogenetic analysis. HC and AM-L screened the Micol collection of leaf mutants for abnormal leaf venation patterns. PR performed preliminary morphometric analysis of cells and venation from api7-1 leaves. JŠ and KL performed the IAA metabolite profiling. YF and VR performed the TAP assay. MRP, HC, and EM-B performed the mapping and cloning of the api7-1 mutation. CN-Q and JLM wrote the manuscript. All authors revised and approved the manuscript.
Funding
This work was supported by the Ministerio de Ciencia e Innovación of Spain [PID2019-105495GB-I00 (MCI/AEI/FEDER, UE), to VR; PGC2018-093445-B-I00 (MCI/AEI/FEDER, UE), to JLM]; and the Generalitat Valenciana [PROMETEO/2019/117, to JLM and MRP]. CN-Q and EM-B held predoctoral fellowships from the Universidad Miguel Hernández [401PREDO] and the Ministerio de Educación, Cultura y Deporte of Spain [FPU13/00371], respectively. KL and JŠ were funded by the Knut and Alice Wallenberg Foundation (KAW 2016.0341 and KAW 2016.0352) and the Swedish Governmental Agency for Innovation Systems (VINNOVA 2016-00504). Funding for open access charge: Universidad Miguel Hernández.
Acknowledgments
We thank J. Castelló, J.M. Serrano, and M.J. Ñíguez for their excellent technical assistance, and M. Sendra-Ortolà and I.C. Pomares-Bri for helping in the phenotypic analysis of api7-1 and some gene constructs. This manuscript was previously published as a preprint at: https://www.biorxiv.org/content/10.1101/2022.05.30.493987v1.
Conflict of interest
The authors declare that the research was conducted in the absence of any commercial or financial relationships that could be construed as a potential conflict of interest.
Publisher’s note
All claims expressed in this article are solely those of the authors and do not necessarily represent those of their affiliated organizations, or those of the publisher, the editors and the reviewers. Any product that may be evaluated in this article, or claim that may be made by its manufacturer, is not guaranteed or endorsed by the publisher.
Supplementary material
The Supplementary Material for this article can be found online at: https://www.frontiersin.org/articles/10.3389/fpls.2022.1009895/full#supplementary-material
References
Alhebshi, A., Sideri, T. C., Holland, S. L., Avery, S. V. (2012). The essential iron-sulfur protein Rli1 is an important target accounting for inhibition of cell growth by reactive oxygen species. Mol. Biol. Cell 23 (18), 3582–3590. doi: 10.1091/mbc.E12-05-0413
Altschul, S. F., Madden, T. L., Schäffer, A. A., Zhang, J., Zhang, Z., Miller, W., et al. (1997). Gapped BLAST and PSI-BLAST: A new generation of protein database search programs. Nucleic Acids Res. 25 (17), 3389–3402. doi: 10.1093/nar/25.17.3389
Arribas-Hernández, L., Bressendorff, S., Hansen, M. H., Poulsen, C., Erdmann, S., Brodersen, P. (2018). An m6A-YTH module controls developmental timing and morphogenesis in arabidopsis. Plant Cell 30 (5), 952–967. doi: 10.1105/tpc.17.00833
Baima, S., Nobili, F., Sessa, G., Lucchetti, S., Ruberti, I., Morelli, G. (1995). The expression of the Athb-8 homeobox gene is restricted to provascular cells in Arabidopsis thaliana. Development 121 (12), 4171–4182. doi: 10.1242/dev.121.12.4171
Barthelme, D., Dinkelaker, S., Albers, S. V., Londei, P., Ermler, U., Tampé, R. (2011). Ribosome recycling depends on a mechanistic link between the FeS cluster domain and a conformational switch of the twin-ATPase ABCE1. Proc. Natl. Acad. Sci. U.S.A. 108 (8), 3228–3233. doi: 10.1073/pnas.1015953108
Barthelme, D., Scheele, U., Dinkelaker, S., Janoschka, A., MacMillan, F., Albers, S. V., et al. (2007). Structural organization of essential iron-sulfur clusters in the evolutionarily highly conserved ATP-binding cassette protein ABCE1. J. Biol. Chem. 282 (19), 14598–14607. doi: 10.1074/jbc.M700825200
Becker, T., Franckenberg, S., Wickles, S., Shoemaker, C. J., Anger, A. M., Armache, J. P., et al. (2012). Structural basis of highly conserved ribosome recycling in eukaryotes and archaea. Nature 482 (7386), 501–506. doi: 10.1038/nature10829
Berná, G., Robles, P., Micol, J. L. (1999). A mutational analysis of leaf morphogenesis in Arabidopsis thaliana. Genetics 152 (2), 729–742. doi: 10.1093/genetics/152.2.729
Bisbal, C., Martinand, C., Silhol, M., Lebleu, B., Salehzada, T. (1995). Cloning and characterization of a RNase l inhibitor. a new component of the interferon-regulated 2-5A pathway. J. Biol. Chem. 270 (22), 13308–13317. doi: 10.1074/jbc.270.22.13308
Braz, A. S., Finnegan, J., Waterhouse, P., Margis, R. (2004). A plant orthologue of RNase l inhibitor (RLI) is induced in plants showing RNA interference. J. Mol. Evol. 59 (1), 20–30. doi: 10.1007/s00239-004-2600-4
Briat, J. F., Duc, C., Ravet, K., Gaymard, F. (2010). Ferritins and iron storage in plants. Biochim. Biophys. Acta 1800 (8), 806–814. doi: 10.1016/j.bbagen.2009.12.003
Bühler, J., Rishmawi, L., Pflugfelder, D., Huber, G., Scharr, H., Hülskamp, M., et al. (2015). phenoVein−A tool for leaf vein segmentation and analysis. Plant Physiol. 169 (4), 2359–2370. doi: 10.1104/pp.15.00974
Byrne, M. E. (2009). A role for the ribosome in development. Trends Plant Sci. 14 (9), 512–519. doi: 10.1016/j.tplants.2009.06.009
Candela, H., Martínez-Laborda, A., Micol, J. L. (1999). Venation pattern formation in Arabidopsis thaliana vegetative leaves. Dev. Biol. 205 (1), 205–216. doi: 10.1006/dbio.1998.9111
Casanova-Sáez, R., Candela, H., Micol, J. L. (2014). Combined haploinsufficiency and purifying selection drive retention of RPL36a paralogs in arabidopsis. Sci. Rep. 4, 4122. doi: 10.1038/srep04122
Casanova-Sáez, R., Mateo-Bonmatí, E., Ljung, K. (2021). Auxin metabolism in plants. Cold Spring Harbor Perspect. Biol. 13, a039867. doi: 10.1101/cshperspect.a039867
Chen, Z. Q., Dong, J., Ishimura, A., Daar, I., Hinnebusch, A. G., Dean, M. (2006). The essential vertebrate ABCE1 protein interacts with eukaryotic initiation factors. J. Biol. Chem. 281 (11), 7452–7457. doi: 10.1074/jbc.M510603200
Cheng, Y., Dai, X., Zhao, Y. (2007). Auxin synthesized by the YUCCA flavin monooxygenases is essential for embryogenesis and leaf formation in Arabidopsis. Plant Cell 19 (8), 2430–2439. doi: 10.1105/tpc.107.053009
Clough, S. J., Bent, A. F. (1998). Floral dip: a simplified method for Agrobacterium-mediated transformation of Arabidopsis thaliana. Plant J. 16 (6), 735–743. doi: 10.1046/j.1365-313x.1998.00343.x
Coaker, G., Zhu, G., Ding, Z., Van Doren, S. R., Staskawicz, B. (2006). Eukaryotic cyclophilin as a molecular switch for effector activation. Mol. Microbiol. 61 (6), 1485–1496. doi: 10.1111/j.1365-2958.2006.05335.x
Colca, J. R., McDonald, W. G., Waldon, D. J., Leone, J. W., Lull, J. M., Bannow, C. A., et al. (2004). Identification of a novel mitochondrial protein (“mitoNEET”) cross-linked specifically by a thiazolidinedione photoprobe. Am. J. Physiol.: Endocrinol. Metab. 286 (2), 252–260. doi: 10.1152/ajpendo.00424.2003
Curtis, M. D., Grossniklaus, U. (2003). A gateway cloning vector set for high-throughput functional analysis of genes in planta. Plant Physiol. 133 (2), 462–469. doi: 10.1104/pp.103.027979
Dever, T. E., Dinman, J. D., Green, R. (2018). Translation elongation and recoding in eukaryotes. Cold Spring Harbor Perspect. Biol. 10 (8), a032649. doi: 10.1101/cshperspect.a032649
Earley, K. W., Haag, J. R., Pontes, O., Opper, K., Juehne, T., Song, K., et al. (2006). Gateway-compatible vectors for plant functional genomics and proteomics. Plant J. 45 (4), 616–629. doi: 10.1111/j.1365-313X.2005.02617.x
Edgar, R. C. (2004a). MUSCLE: A multiple sequence alignment method with reduced time and space complexity. BMC Bioinf. 5, 113. doi: 10.1186/1471-2105-5-113
Edgar, R. C. (2004b). MUSCLE: Multiple sequence alignment with high accuracy and high throughput. Nucleic Acids Res. 32 (5), 1792–1797. doi: 10.1093/nar/gkh340
Eide, D., Broderius, M., Fett, J., Guerinot, M. L. (1996). A novel iron-regulated metal transporter from plants identified by functional expression in yeast. Proc. Natl. Acad. Sci. U.S.A. 93 (11), 5624–5628. doi: 10.1073/pnas.93.11.5624
Faus, I., Niñoles, R., Kesari, V., Llabata, P., Tam, E., Nebauer, S. G., et al. (2018). Arabidopsis ILITHYIA protein is necessary for proper chloroplast biogenesis and root development independent of eIF2α phosphorylation. J. Plant. Physiol. 224–225, 173–182. doi: 10.1016/j.jplph.2018.04.003
Gao, Y., Dai, X., Aoi, Y., Takebayashi, Y., Yang, L., Guo, X., et al. (2020). Two homologous INDOLE-3-ACETAMIDE (IAM) HYDROLASE genes are required for the auxin effects of IAM in Arabidopsis. J. Genet. Genomics 47 (3), 157–165. doi: 10.1016/j.jgg.2020.02.009
García-León, M., Iniesto, E., Rubio, V. (2018). Tandem affinity purification of protein complexes from arabidopsis cell cultures. Methods Mol. Biol. 1794, 297–309. doi: 10.1007/978-1-4939-7871-7_21
Gouridis, G., Hetzert, B., Kiosze-Becker, K., de Boer, M., Heinemann, H., Nürenberg-Goloub, E., et al. (2019). ABCE1 controls ribosome recycling by an asymmetric dynamic conformational equilibrium. Cell Rep. 28 (3), 723–734. doi: 10.1016/j.celrep.2019.06.052
Hayashi, K. I., Arai, K., Aoi, Y., Tanaka, Y., Hira, H., Guo, R., et al. (2021). The main oxidative inactivation pathway of the plant hormone auxin. Nat. Commun. 12 (1), 6752. doi: 10.1038/s41467-021-27020-1
Heisler, M. G., Ohno, C., Das, P., Sieber, P., Reddy, G. V., Long, J. A., et al. (2005). Patterns of auxin transport and gene expression during primordium development revealed by live imaging of the arabidopsis inflorescence meristem. Curr. Biol. 15 (21), 1899–1911. doi: 10.1016/j.cub.2005.09.052
Hellen, C. U. T. (2018). Translation termination and ribosome recycling in eukaryotes. Cold Spring Harbor Perspect. Biol. 10 (10), a032656. doi: 10.1101/cshperspect.a032656
Heuer, A., Gerovac, M., Schmidt, C., Trowitzsch, S., Preis, A., Kötter, P., et al. (2017). Structure of the 40S-ABCE1 post-splitting complex in ribosome recycling and translation initiation. Nat. Struct. Mol. Biol. 24 (5), 453–460. doi: 10.1038/nsmb.3396
Hooper, C. M., Castleden, I. R., Tanz, S. K., Aryamanesh, N., Millar, A. H. (2017). SUBA4: The interactive data analysis centre for arabidopsis subcellular protein locations. Nucleic Acids Res. 45 (D1), 1064–1074. doi: 10.1093/nar/gkw1041
Hooper, C. M., Tanz, S. K., Castleden, I. R., Vacher, M. A., Small, I. D., Millar, A. H. (2014). SUBAcon: A consensus algorithm for unifying the subcellular localization data of the Arabidopsis proteome. Bioinformatics 30 (23), 3356–3364. doi: 10.1093/bioinformatics/btu550
Hopfner, K. P. (2012). Rustless translation. Biol. Chem. 393 (10), 1079–1088. doi: 10.1515/hsz-2012-0196
Horiguchi, G., Mollá-Morales, A., Pérez-Pérez, J. M., Kojima, K., Robles, P., Ponce, M. R., et al. (2011). Differential contributions of ribosomal protein genes to Arabidopsis thaliana leaf development. Plant J. 65 (5), 724–736. doi: 10.1111/j.1365-313X.2010.04457.x
Huang, D. W., Sherman, B. T., Lempicki, R. A. (2009a). Bioinformatics enrichment tools: paths toward the comprehensive functional analysis of large gene lists. Nucleic Acids Res. 37 (1), 1–13. doi: 10.1093/nar/gkn923
Huang, D. W., Sherman, B. T., Lempicki, R. A. (2009b). Systematic and integrative analysis of large gene lists using DAVID bioinformatics resources. Nat. Protoc. 4 (1), 44–57. doi: 10.1038/nprot.2008.211
Izquierdo, Y., Kulasekaran, S., Benito, P., López, B., Marcos, R., Cascón, T., et al. (2018). Arabidopsis nonresponding to oxylipins locus NOXY7 encodes a yeast GCN1 homolog that mediates noncanonical translation regulation and stress adaptation. Plant. Cell Environ. 41 (6), 1438–1452. doi: 10.1111/pce.13182
Kärblane, K., Gerassimenko, J., Nigul, L., Piirsoo, A., Smialowska, A., Vinkel, K., et al. (2015). ABCE1 is a highly conserved RNA silencing suppressor. PloS One 10 (2), e0116702. doi: 10.1371/journal.pone.0116702
Karcher, A., Büttner, K., Märtens, B., Jansen, R. P., Hopfner, K. P. (2005). X-Ray structure of RLI, an essential twin cassette ABC ATPase involved in ribosome biogenesis and HIV capsid assembly. Structure 13 (4), 649–659. doi: 10.1016/j.str.2005.02.008
Karcher, A., Schele, A., Hopfner, K. P. (2008). X-Ray structure of the complete ABC enzyme ABCE1 from Pyrococcus abyssi. J. Biol. Chem. 283 (12), 7962–7971. doi: 10.1074/jbc.M707347200
Kashima, I., Takahashi, M., Hashimoto, Y., Sakota, E., Nakamura, Y., Inada, T. (2014). A functional involvement of ABCE1, eukaryotic ribosome recycling factor, in nonstop mRNA decay in Drosophila melanogaster cells. Biochimie 106, 10–16. doi: 10.1016/j.biochi.2014.08.001
Kim, D., Paggi, J. M., Park, C., Bennett, C., Salzberg, S. L. (2019). Graph-based genome alignment and genotyping with HISAT2 and HISAT-genotype. Nat. Biotechnol. 37 (8), 907–915. doi: 10.1038/s41587-019-0201-4
Kirli, K., Karaca, S., Dehne, H. J., Samwer, M., Pan, K. T., Lenz, C., et al. (2015). A deep proteomics perspective on CRM1-mediated nuclear export and nucleocytoplasmic partitioning. eLIFE 4, e11466. doi: 10.7554/eLife.11466
Kleinboelting, N., Huep, G., Kloetgen, A., Viehoever, P., Weisshaar, B. (2012). GABI-kat SimpleSearch: New features of the Arabidopsis thaliana T-DNA mutant database. Nucleic Acids Res. 40 (D1), D1211–D1215. doi: 10.1093/nar/gkr1047
Kneuper, I., Teale, W., Dawson, J. E., Tsugeki, R., Katifori, E., Palme, K., et al. (2021). Auxin biosynthesis and cellular efflux act together to regulate leaf vein patterning. J. Exp. Bot. 72, 1151–1165. doi: 10.1093/jxb/eraa501
Kougioumoutzi, E., Cartolano, M., Canales, C., Dupré, M., Bramsiepe, J., Vlad, D., et al. (2013). SIMPLE LEAF3 encodes a ribosome-associated protein required for leaflet development in Cardamine hirsuta. Plant J. 73 (4), 533–545. doi: 10.1111/tpj.12072
Kratzat, H., Mackens-Kiani, T., Ameismeier, M., Potocnjak, M., Cheng, J., Dacheux, E., et al. (2021). A structural inventory of native ribosomal ABCE1-43S pre-initiation complexes. EMBO J. 40 (1), e105179. doi: 10.15252/embj.2020105179
Kumar, S., Stecher, G., Li, M., Knyaz, C., Tamura, K. (2018). MEGA X: Molecular evolutionary genetics analysis across computing platforms. Mol. Biol. Evol. 35 (6), 1547–1549. doi: 10.1093/molbev/msy096
Lanquar, V., Lelièvre, F., Bolte, S., Hamès, C., Alcon, C., Neumann, D., et al. (2005). Mobilization of vacuolar iron by AtNRAMP3 and AtNRAMP4 is essential for seed germination on low iron. EMBO J. 24 (23), 4041–4051. doi: 10.1038/sj.emboj.7600864
Li, J., Wang, Y., Zheng, L., Li, Y., Zhou, X., Li, J., et al. (2019). The intracellular transporter AtNRAMP6 is involved in fe homeostasis in Arabidopsis. Front. Plant Sci. 10. doi: 10.3389/fpls.2019.01124
Love, M. I., Huber, W., Anders, S. (2014). Moderated estimation of fold change and dispersion for RNA-seq data with DESeq2. Genome Biol. 15 (12), 550. doi: 10.1186/s13059-014-0550-8
Madeira, F., Park, Y. M., Lee, J., Buso, N., Gur, T., Madhusoodanan, N., et al. (2019). The EMBL-EBI search and sequence analysis tools APIs in 2019. Nucleic Acids Res. 47 (W1), 636–641. doi: 10.1093/nar/gkz268
Mancera-Martínez, E., Brito Querido, J., Valasek, L. S., Simonetti, A., Hashem, Y. (2017). ABCE1: A special factor that orchestrates translation at the crossroad between recycling and initiation. RNA Biol. 14, 1279–1285. doi: 10.1080/15476286.2016.1269993
Mateo-Bonmatí, E., Casanova-Sáez, R., Candela, H., Micol, J. L. (2014). Rapid identification of angulata leaf mutations using next-generation sequencing. Planta 240 (5), 1113–1122. doi: 10.1007/s00425-014-2137-8
Mateo-Bonmatí, E., Casanova-Sáez, R., Quesada, V., Hricová, A., Candela, H., Micol, J. L. (2015). Plastid control of abaxial-adaxial patterning. Sci. Rep. 5, 15975. doi: 10.1038/srep15975
Mateo-Bonmatí, E., Casanova-Sáez, R., Šimura, J., Ljung, K. (2021). Broadening the roles of UDP-glycosyltransferases in auxin homeostasis and plant development. New Phytol. 232 (2), 642–654. doi: 10.1111/nph.17633
Mateo-Bonmatí, E., Esteve-Bruna, D., Juan-Vicente, L., Nadi, R., Candela, H., Lozano, F. M., et al. (2018). INCURVATA11 and CUPULIFORMIS2 are redundant genes that encode epigenetic machinery components in arabidopsis. Plant Cell 30 (7), 1596–1616. doi: 10.1105/tpc.18.00300
Matsumura, Y., Ohbayashi, I., Takahashi, H., Kojima, S., Ishibashi, N., Keta, S., et al. (2016). A genetic link between epigenetic repressor AS1-AS2 and a putative small subunit processome in leaf polarity establishment of Arabidopsis. Biol. Open 5 (7), 942–954. doi: 10.1242/bio.019109
Mellor, N., Band, L. R., Pěnčík, A., Novák, O., Rashed, A., Holman, T., et al. (2016). Dynamic regulation of auxin oxidase and conjugating enzymes AtDAO1 and GH3 modulates auxin homeostasis. Proc. Natl. Acad. Sci. U.S.A. 113 (39), 11022–11027. doi: 10.1073/pnas.1604458113
Micol-Ponce, R., Sarmiento-Mañús, R., Fontcuberta-Cervera, S., Cabezas-Fuster, A., de Bures, A., Sáez-Vásquez, J., et al. (2020). SMALL ORGAN4 is a ribosome biogenesis factor involved in 5.8S ribosomal RNA maturation. Plant Physiol. 184 (4), 2022–2039. doi: 10.1104/pp.19.01540
Micol-Ponce, R., Sarmiento-Mañús, R., Ruiz-Bayón, A., Montacié, C., Sáez-Vasquez, J., Ponce, M. R. (2018). Arabidopsis RIBOSOMAL RNA PROCESSING7 is required for 18S rRNA maturation. Plant Cell 30 (11), 2855–2872. doi: 10.1105/tpc.18.00245
Moschopoulos, A., Derbyshire, P., Byrne, M. E. (2012). The Arabidopsis organelle-localized glycyl-tRNA synthetase encoded by EMBRYO DEFECTIVE DEVELOPMENT1 is required for organ patterning. J. Exp. Bot. 63 (14), 5233–5243. doi: 10.1093/jxb/ers184
Mõttus, J., Maiste, S., Eek, P., Truve, E., Sarmiento, C. (2020). Mutational analysis of Arabidopsis thaliana ABCE2 identifies important motifs for its RNA silencing suppressor function. Plant Biol. 23, 21–31. doi: 10.1111/plb.13193
Navarro-Quiles, C., Mateo-Bonmatí, E., Micol, J. L. (2018). ABCE proteins: From molecules to development. Front. Plant Sci. 9. doi: 10.3389/fpls.2018.01125
Nechushtai, R., Conlan, A. R., Harir, Y., Song, L., Yogev, O., Eisenberg-Domovich, Y., et al. (2012). Characterization of Arabidopsis NEET reveals an ancient role for NEET proteins in iron metabolism. Plant Cell 24 (5), 2139–2154. doi: 10.1105/tpc.112.097634
Novák, O., Hényková, E., Sairanen, I., Kowalczyk, M., Pospíšil, T., Ljung, K. (2012). Tissue-specific profiling of the Arabidopsis thaliana auxin metabolome. Plant J. 72 (3), 523–536. doi: 10.1111/j.1365-313X.2012.05085.x
Nürenberg-Goloub, E., Heinemann, H., Gerovac, M., Tampé, R. (2018). Ribosome recycling is coordinated by processive events in two asymmetric ATP sites of ABCE1. Life Sci. Alliance. 1 (3), e201800095. doi: 10.26508/lsa.201800095
Nürenberg-Goloub, E., Kratzat, H., Heinemann, H., Heuer, A., Kötter, P., Berninghausen, O., et al. (2020). Molecular analysis of the ribosome recycling factor ABCE1 bound to the 30S post-splitting complex. EMBO J. 39 (9), e103788. doi: 10.15252/embj.2019103788
Nürenberg-Goloub, E., Tampé, R. (2019). Ribosome recycling in mRNA translation, quality control, and homeostasis. Biol. Chem. 401 (1), 47–61. doi: 10.1515/hsz-2019-0279
Paul, V. D., Mühlenhoff, U., Stümpfig, M., Seebacher, J., Kugler, K. G., Renicke, C., et al. (2015). The deca-GX3 proteins Yae1-Lto1 function as adaptors recruiting the ABC protein Rli1 for iron-sulfur cluster insertion. eLIFE 4, e08231. doi: 10.7554/eLife.08231
Pěnčík, A., Casanova-Sáez, R., Pilařová, V., Žukauskaitė, A., Pinto, R., Micol, J. L., et al. (2018). Ultra-rapid auxin metabolite profiling for high-throughput mutant screening in arabidopsis. J. Exp. Bot. 69 (10), 2569–2579. doi: 10.1093/jxb/ery084
Pérez-Pérez, J. M., Rubio-Díaz, S., Dhondt, S., Hernández-Romero, D., Sánchez-Soriano, J., Beemster, G. T., et al. (2011). Whole organ, venation and epidermal cell morphological variations are correlated in the leaves of Arabidopsis mutants. Plant. Cell Environ. 34 (12), 2200–2211. doi: 10.1111/j.1365-3040.2011.02415.x
Perez-Riverol, Y., Bai, J., Bandla, C., García-Seisdedos, D., Hewapathirana, S., Kamatchinathan, S., et al. (2022). The PRIDE database resources in 2022: a hub for mass spectrometry-based proteomics evidences. Nucleic Acids Res. 50 (D1), D543–D552. doi: 10.1093/nar/gkab1038
Pinon, V., Etchells, J. P., Rossignol, P., Collier, S. A., Arroyo, J. M., Martienssen, R. A., et al. (2008). Three PIGGYBACK genes that specifically influence leaf patterning encode ribosomal proteins. Development 135 (7), 1315–1324. doi: 10.1242/dev.016469
Ponce, M. R., Quesada, V., Micol, J. L. (1998). Rapid discrimination of sequences flanking and within T-DNA insertions in the Arabidopsis genome. Plant J. 14 (4), 497–501. doi: 10.1046/j.1365-313x.1998.00146.x
Ponce, M. R., Robles, P., Lozano, F. M., Brotóns, M. A., Micol, J. L. (2006). Low-resolution mapping of untagged mutations. Methods Mol. Biol. 323, 105–113. doi: 10.1385/1-59745-003-0:105
Ponce, M. R., Robles, P., Micol, J. L. (1999). High-throughput genetic mapping in Arabidopsis thaliana. Mol. Gen. Genet. 261 (2), 408–415. doi: 10.1007/s004380050982
Porco, S., Pěnčík, A., Rashed, A., Voβ, U., Casanova-Sáez, R., Bishopp, A., et al. (2016). Dioxygenase-encoding AtDAO1 gene controls IAA oxidation and homeostasis in Arabidopsis. Proc. Natl. Acad. Sci. U.S.A. 113 (39), 11016–11021. doi: 10.1073/pnas.1604375113
Poza-Viejo, L., del Olmo, I., Crevillén, P. (2019). Plant chromatin immunoprecipitation v.2. protocols.io. Berkely, CA 94704, USA 25468. doi: 10.17504/protocols.io.444gyyw
Preis, A., Heuer, A., Barrio-Garcia, C., Hauser, A., Eyler, D. E., Berninghausen, O., et al. (2014). Cryoelectron microscopic structures of eukaryotic translation termination complexes containing eRF1-eRF3 or eRF1-ABCE1. Cell Rep. 8 (1), 59–65. doi: 10.1016/j.celrep.2014.04.058
Prusty, N. R., Camponeschi, F., Ciofi-Baffoni, S., Banci, L. (2021). The human YAE1-ORAOV1 complex of the cytosolic iron-sulfur protein assembly machinery binds a [4Fe-4S] cluster. Inorganica. Chim. Acta 518, 120252. doi: 10.1016/j.ica.2021.120252
Quesada, V., Ponce, M. R., Micol, J. L. (2000). Genetic analysis of salt-tolerant mutants in Arabidopsis thaliana. Genetics 154 (1), 421–436. doi: 10.1093/genetics/154.1.421
Reyt, G., Boudouf, S., Boucherez, J., Gaymard, F., Briat, J. F. (2015). Iron- and ferritin-dependent reactive oxygen species distribution: Impact on Arabidopsis root system architecture. Mol. Plant 8 (3), 439–453. doi: 10.1016/j.molp.2014.11.014
Robinson, N. J., Procter, C. M., Connolly, E. L., Guerinot, M. L. (1999). A ferric-chelate reductase for iron uptake from soils. Nature 397 (6721), 694–697. doi: 10.1038/17800
Robles, P., Fleury, D., Candela, H., Cnops, G., Alonso-Peral, M. M., Anami, S., et al. (2010). The RON1/FRY1/SAL1 gene is required for leaf morphogenesis and venation patterning in arabidopsis. Plant Physiol. 152 (3), 1357–1372. doi: 10.1104/pp.109.149369
Robles, P., Micol, J. L. (2001). Genome-wide linkage analysis of Arabidopsis genes required for leaf development. Mol. Genet. Genomics 266 (1), 12–19. doi: 10.1007/s004380100535
Rodnina, M. V. (2018). Translation in prokaryotes. Cold Spring Harbor Perspect. Biol. 10 (9), a032664. doi: 10.1101/cshperspect.a032664
Rosado, A., Li, R., van de Ven, W., Hsu, E., Raikhel, N. V. (2012). Arabidopsis ribosomal proteins control developmental programs through translational regulation of auxin response factors. Proc. Natl. Acad. Sci. U.S.A. 109 (48), 19537–19544. doi: 10.1073/pnas.1214774109
Saitou, N., Nei, M. (1987). The neighbor-joining method: A new method for reconstructing phylogenetic trees. Mol. Biol. Evol. 4 (4), 406–425. doi: 10.1093/oxfordjournals.molbev.a040454
Sánchez-Fernández, R., Davies, T. G., Coleman, J. O., Rea, P. A. (2001). The Arabidopsis thaliana ABC protein superfamily, a complete inventory. J. Biol. Chem. 276 (32), 30231–30244. doi: 10.1074/jbc.M103104200
Sarmiento, C., Nigul, L., Kazantseva, J., Buschmann, M., Truve, E. (2006). AtRLI2 is an endogenous suppressor of RNA silencing. Plant Mol. Biol. 61 (1-2), 153–163. doi: 10.1007/s11103-005-0001-8
Schindelin, J., Arganda-Carreras, I., Frise, E., Kaynig, V., Longair, M., Pietzsch, T., et al. (2012). Fiji: An open-source platform for biological-image analysis. Nat. Methods 9 (7), 676–682. doi: 10.1038/nmeth.2019
Schmittgen, T. D., Livak, K. J. (2008). Analyzing real-time PCR data by the comparative CT method. Nat. Protoc. 3 (6), 1101–1108. doi: 10.1038/nprot.2008.73
Scutenaire, J., Deragon, J. M., Jean, V., Benhamed, M., Raynaud, C., Favory, J. J., et al. (2018). The YTH domain protein ECT2 is an m6A reader required for normal trichome branching in arabidopsis. Plant Cell 30 (5), 986–1005. doi: 10.1105/tpc.17.00854
Sesma, A., Castresana, C., Castellano, M. M. (2017). Regulation of translation by TOR, eIF4E and eIF2α in plants: Current knowledge, challenges and future perspectives. Front. Plant Sci. 8. doi: 10.3389/fpls.2017.00644
Shao, S., von der Malsburg, K., Hegde, R. S. (2013). Listerin-dependent nascent protein ubiquitination relies on ribosome subunit dissociation. Mol. Cell 50 (5), 637–648. doi: 10.1016/j.molcel.2013.04.015
Shirokikh, N. E., Preiss, T. (2018). Translation initiation by cap-dependent ribosome recruitment: Recent insights and open questions. Wiley. Interdiscip. Rev.: RNA 9 (4), e1473. doi: 10.1002/wrna.1473
Simonetti, A., Guca, E., Bochler, A., Kuhn, L., Hashem, Y. (2020). Structural insights into the mammalian late-stage initiation complexes. Cell Rep. 31 (1), 107497. doi: 10.1016/j.celrep.2020.03.061
Sudmant, P. H., Lee, H., Dominguez, D., Heiman, M., Burge, C. B. (2018). Widespread accumulation of ribosome-associated isolated 3’ UTRs in neuronal cell populations of the aging brain. Cell Rep. 25 (9), 2447–2456. doi: 10.1016/j.celrep.2018.10.094
Takubo, E., Kobayashi, M., Hirai, S., Aoi, Y., Ge, C., Dai, X., et al. (2020). Role of arabidopsis INDOLE-3-ACETIC ACID CARBOXYL METHYLTRANSFERASE 1 in auxin metabolism. Biochem. Biophys. Res. Commun. 527 (4), 1033–1038. doi: 10.1016/j.bbrc.2020.05.031
Van Leene, J., Eeckhout, D., Cannoot, B., De Winne, N., Persiau, G., Van De Slijke, E., et al. (2015). An improved toolbox to unravel the plant cellular machinery by tandem affinity purification of Arabidopsis protein complexes. Nat. Protoc. 10 (1), 169–187. doi: 10.1038/nprot.2014.199
Veitia, R. A. (2017). Gene duplicates: Agents of robustness or fragility? Trends Genet. 33 (6), 377–379. doi: 10.1016/j.tig.2017.03.006
Verna, C., Ravichandran, S. J., Sawchuk, M. G., Linh, N. M., Scarpella, E. (2019). Coordination of tissue cell polarity by auxin transport and signaling. eLIFE 8, e51061. doi: 10.7554/eLife.51061
Verrier, P. J., Bird, D., Burla, B., Dassa, E., Forestier, C., Geisler, M., et al. (2008). Plant ABC proteins − a unified nomenclature and updated inventory. Trends Plant Sci. 13 (4), 151–159. doi: 10.1016/j.tplants.2008.02.001
Wang, L., Li, H., Zhao, C., Li, S., Kong, L., Wu, W., et al. (2017). The inhibition of protein translation mediated by AtGCN1 is essential for cold tolerance in Arabidopsis thaliana. Plant. Cell Environ. 40 (1), 56–68. doi: 10.1111/pce.12826
Weis, B. L., Kovacevic, J., Missbach, S., Schleiff, E. (2015). Plant-specific features of ribosome biogenesis. Trends Plant Sci. 20 (11), 729–740. doi: 10.1016/j.tplants.2015.07.003
Wei, L. H., Song, P., Wang, Y., Lu, Z., Tang, Q., Yu, Q., et al. (2018). The m6A reader ECT2 controls trichome morphology by affecting mRNA stability in arabidopsis. Plant Cell 30 (5), 968–985. doi: 10.1105/tpc.17.00934
Wellburn, A. R. (1994). The spectral determination of chlorophylls a and b, as well as total carotenoids, using various solvents with spectrophotometers of different resolution. J. Plant Physiol. 144 (3), 307–313. doi: 10.1016/S0176-1617(11)81192-2
Yao, Y., Ling, Q., Wang, H., Huang, H. (2008). Ribosomal proteins promote leaf adaxial identity. Development 135 (7), 1325–1334. doi: 10.1242/dev.017913
Young, D. J., Guydosh, N. R. (2019). Hcr1/eIF3j is a 60S ribosomal subunit recycling accessory factor in vivo. Cell Rep. 28 (1), 39–50. doi: 10.1016/j.celrep.2019.05.111
Young, D. J., Guydosh, N. R., Zhang, F., Hinnebusch, A. G., Green, R. (2015). Rli1/ABCE1 recycles terminating ribosomes and controls translation reinitiation in 3’UTRs in vivo. Cell 162 (4), 872–884. doi: 10.1016/j.cell.2015.07.041
Zandalinas, S. I., Song, L., Sengupta, S., McInturf, S. A., Grant, D. G., Marjault, H. B., et al. (2020). Expression of a dominant-negative AtNEET-H89C protein disrupts iron−sulfur metabolism and iron homeostasis in arabidopsis. Plant J. 101 (5), 1152–1169. doi: 10.1111/tpj.14581
Zhai, C., Li, Y., Mascarenhas, C., Lin, Q., Li, K., Vyrides, I., et al. (2014). The function of ORAOV1/LTO1, a gene that is overexpressed frequently in cancer: Essential roles in the function and biogenesis of the ribosome. Oncogene 33 (4), 484–494. doi: 10.1038/onc.2012.604
Zhang, L., Cai, X., Wu, J., Liu, M., Grob, S., Cheng, F., et al. (2018). Improved Brassica rapa reference genome by single-molecule sequencing and chromosome conformation capture technologies. Horticult. Res. 5, 50. doi: 10.1038/s41438-018-0071-9
Zhang, J., Lin, J. E., Harris, C., Campos Mastrotti Pereira, F., Wu, F., Blakeslee, J. J., et al. (2016). DAO1 catalyzes temporal and tissue-specific oxidative inactivation of auxin in Arabidopsis thaliana. Proc. Natl. Acad. Sci. U.S.A. 113 (39), 11010–11015. doi: 10.1073/pnas.1604769113
Keywords: Arabidopsis ABCE2, ribosome recycling, translation machinery, venation pattern, auxin metabolism
Citation: Navarro-Quiles C, Mateo-Bonmatí E, Candela H, Robles P, Martínez-Laborda A, Fernández Y, Šimura J, Ljung K, Rubio V, Ponce MR and Micol JL (2022) The Arabidopsis ATP-Binding Cassette E protein ABCE2 is a conserved component of the translation machinery. Front. Plant Sci. 13:1009895. doi: 10.3389/fpls.2022.1009895
Received: 02 August 2022; Accepted: 14 September 2022;
Published: 17 October 2022.
Edited by:
Marcos Egea-Cortines, Universidad Politécnica de Cartagena, SpainReviewed by:
Catharina Merchante, University of Malaga, SpainPengcheng Li, Shandong Academy of Agricultural Sciences, China
Paola Londei, Sapienza University of Rome, Italy
Copyright © 2022 Navarro-Quiles, Mateo-Bonmatí, Candela, Robles, Martínez-Laborda, Fernández, Šimura, Ljung, Rubio, Ponce and Micol. This is an open-access article distributed under the terms of the Creative Commons Attribution License (CC BY). The use, distribution or reproduction in other forums is permitted, provided the original author(s) and the copyright owner(s) are credited and that the original publication in this journal is cited, in accordance with accepted academic practice. No use, distribution or reproduction is permitted which does not comply with these terms.
*Correspondence: José Luis Micol, amxtaWNvbEB1bWguZXM=
†Present address: Eduardo Mateo-Bonmatí, John Innes Centre, Norwich, United Kingdom