- 1State Key Laboratory Breeding Base of Dao-di Herbs, National Resource Center for Chinese Materia Medica, China Academy of Chinese Medical Sciences, Beijing, China
- 2Key Laboratory of Biology and Cultivation of Herb Medicine, Ministry of Agriculture and Rural Affairs, Beijing, China
- 3Kunming Xishan Forestry and Grassland Comprehensive Service Center, Kunming, China
- 4Laboratory of Systematic Evolution and Biogeography of Woody Plants, School of Ecology and Nature Conservation, Beijing Forestry University, Beijing, China
The genus Coptis belongs to the Ranunculaceae family, containing 15 recognized species highly diverse in morphology. It is a conspicuous taxon with special evolutionary position, distribution pattern and medicinal value, which makes it to be of great research and conservation significance. In order to better understand the evolutionary dynamics of Coptis and promote more practical conservation measures, we performed plastome sequencing and used the sequencing data in combination with worldwide occurrence data of Coptis to estimate genetic diversity and divergence times, rebuild biogeographic history and predict its potential suitable distribution area. The average nucleotide diversity of Coptis was 0.0067 and the hotspot regions with the highest hypermutation levels were located in the ycf1 gene. Coptis is most likely to have originated in North America and Japanese archipelago and has a typical Eastern Asian and North American disjunct distribution pattern, while the species diversity center is located in Mid-West China and Japan. The crown age of the genus is estimated at around 8.49 Mya. The most suitable climatic conditions for Coptis were as follows: precipitation of driest quarter > 25.5 mm, annual precipitation > 844.9 mm and annual mean temperature -3.1 to 19 °C. The global and China suitable area shows an upward trend in the future when emission of greenhouse gases is well controlled, but the area, especially in China, decreases significantly without greenhouse gas policy interventions. The results of this study provide a comprehensive insight into the Coptis evolutionary dynamics and will facilitate future conservation efforts.
Introduction
Understanding the evolutionary dynamics of living organisms is central to characterize biodiversity on Earth (Dong et al., 2022). The study of taxa with a significant phylogenetic position is a research hotspot in evolutionary biology. Ranunculaceae, located in the early-diverged of eudicot, has fascinated botanists for decades due to its fantastic features in diversification, evolution, and phylogeny (Zeng et al., 2014; Wei et al., 2019). In addition, intercontinental disjunction distribution is another research hotspot that has attracted considerable attention from botanists and biogeographers (Duan et al., 2020; Song et al., 2020; Nge et al., 2022). Understanding the past disjunct distribution pattern, timing, and the associated drivers is also a critical step in elucidating a clear evolutionary story. The Eastern Asian and North American (EA-NA) distribution is one of the most well-known disjunct distribution patterns, and the Bering land bridge probably provided opportunities for floristic exchange (Feng et al., 2021; Ye et al., 2022). Distribution of species was significantly shaped by environmental conditions, including temperature and precipitation. As a result, modeling species distribution has become popular in conservation, ecology, biogeography and evolution studies (Li et al., 2020; Huang et al., 2020).
Coptis, belongs to the Ranunculaceae family, which contains 15 recognized species that are highly diverse in floral morphology, but most species are extremely endangered, and disjunctly distributed from the warm-temperate to the boreal zone of East Asia and North America (Tamura, 1995). The species of this genus are also important medicinal plants worldwide. ‘Huanglian’, the rhizome of Coptis plants, has been clinically used as an antiviral, antimicrobial and anti-inflammatory agent for thousands of years (He et al., 2014). Currently, as the COVID-19 pandemic sweeps the world, ‘Huanglian’ shows the potential for fighting against the epidemic (Ma et al., 2020; Xu et al., 2022). In general, Coptis has a special evolutionary position, typical disjunct pattern and important medicinal value, which makes it to be of great research and conservation significance. To better characterize this special genus, an updated understanding of its evolutionary history, through a robust study on genetic variation, phylogeny, divergence time, and past disjunct distribution pattern is required. In addition, it is also necessary to develop more practical conservation measures, and predict its potential suitable distribution area from present to future.
As a well-known Chinese herbal medicine, the synthetic pathways of alkaloids derived from Coptis species, and their pharmacological and pharmacodynamic characteristics have been extensively studied (Minami et al., 2007; Yan et al., 2008; Chen et al., 2021; Liu et al., 2021; Yang et al., 2021). A few studies focused on phylogenetic reconstruction, distribution patterns assessment, divergence time estimation, and prediction of suitable distribution area (He et al., 2014; Xiang et al., 2016; Xiang et al., 2018; Li et al., 2020; Wang et al., 2020). Adequate sampling and sufficient variable molecular information are necessary for a reliable evolutionary process and prediction of the distribution. However, these studies only use a few DNA markers (rbcL, matK, trnL-F, trnH-psbA, ITS, etc.) which have limited variable information or the sample size of the studies was small that not all Coptis species from multiple distribution areas were included. Therefore, we expanded sampling to cover almost all the species of Coptis and used more informative markers to perform evolutionary research. In the meanwhile, we added more occurrence records to cover the worldwide distribution of Coptis for precise prediction of its suitable areas.
With the development of next-generation sequencing technologies, plastome genome sequences can be obtained more efficiently by directly sequencing total genomic DNA and de novo assembling whole plastid genomes (Sun et al., 2021). Due to its stable structure, rare recombination, moderate evolution rate, and largely uniparental inheritance, plastomes have been extensively used to reveal the evolutionary dynamics, such as phylogeny, phylogeography, demographic history and species diversity estimation (Aguirre Planter et al., 2020; Dong et al., 2021a; Dong et al., 2021b; Wang Y. et al., 2021; Wang Z. et al., 2021).
In this study, we newly sequenced, assembled eleven plastomes and analyzed twelve plastomes of Coptis (one of which was downloaded from GenBank). Combined with the geographical and climatic data of Coptis global distribution area, we aimed to (1) evaluate the plastome variation and identify the most variable regions, (2) reconstruct the phylogenetic relationship and estimate divergence times, (3) rebuild the biogeographic history and infer the formation of their disjunct pattern, and (4) predict its potential suitable distribution area. By combining these aspects, we aim to elucidate the evolutionary dynamics of this important genus and propose more reasonable protection measures.
Material and methods
Plant material and DNA extraction
Eleven specimens of genus Coptis were obtained from the herbarium of PE (Herbarium, Institute of Botany, CAS, Beijing, China) and CMMI (Institute of Chinese Materia Medica, China Academy of Chinese Medical Sciences, Beijing, China) (Table S1). The sequence of C. japonica was downloaded from GenBank (NC054329). Total genomic DNA was extracted from specimens using a modified cetyl trimethyl ammonium bromide (CTAB) method and purified with the Wizard DNA clean-up kit (Promega Corporation, Madison, WI, USA) (Li et al., 2013). DNA quality was assessed by spectrophotometry and stored at the -20°C.
Plastome sequencing, assembly, and annotation
After fragmenting into 300-350 bp fragments by sonication, a pair-end library was constructed using the NEBNext Ultra™ DNA library prep kit (New England Biolabs, Ipswich, MA, USA). Pair-end (PE150) sequencing of 11 accessions was performed on the Illumina HiSeq XTen platform at Novogene Co., Ltd (Beijing, China). The raw data of the PE150 sequencing were filtered using the Trimmomatic 0.39 software (with settings: ILLUMINACLIP : TruSeq3-PE. fa:2:30:10:1:true LEADING:20 TRAILING:20 SLIDINGWINDOW:4:15) (Bolger et al., 2014). De novo assembly of the high-quality reads was performed using the Getorganelle v1.7.5 software with the suggested settings: -R 15 and -k 85,105 (Jin et al., 2020). Ultimately, all reads were mapped to the assembled plastome sequence to verify the assembly accuracy in Geneious 8.1 software (Biomatters Ltd., Auckland, New Zealand) as a double-check process. Gene annotation was performed using the online platform CPGAVAS2 with default settings and manual checking in Sequin to avoid missing or incorrectly annotated genes (Shi et al., 2019). The circle maps of the plastomes were plotted using the online program Chloroplot (https://irscope.shinyapps.io/Chloroplot/).
Comparison of plastomes
Twelve plastomes were aligned using the multiple alignment software MAFFT online (https://mafft.cbrc.jp/alignment/server/) and manually adjusted using Se-al 2.0. Comparison of the whole plastomes of Coptis species was performed using the mVISTA program (http://genome.lbl.gov/vista/mvista/submit.shtml) in the Shuffle-LAGAN mode with C. aspleniifolia as a reference. Additionally, the nucleotide diversity (Pi, π) and Indels were calculated based on a 500-bp sliding window using the DnaSP v5.10 software (Librado and Rozas, 2009). Circos analysis was performed on the indel and nucleotide diversity data using the OmicStudio tools (https://www.omicstudio.cn/tool/) to visualize the hotspot region. A line graph was plotted to show the hyper-mutation region in detail (Gu et al., 2014).
Phylogeny, biogeography and divergence time estimation
A total of 19 plastome sequences were used to reconstruct the phylogeny, including the Coptis species and seven outgroup plastomes from GenBank (Table S1). All genome sequences were aligned using the MAFFT software and ambiguous regions were trimmed by the Gblocks 0.91b program (Castresana, 2000). The program ModelFinder was used to select the best-fit model according to the Bayesian information criterion (Kalyaanamoorthy et al., 2017). The maximum likelihood tree was inferred using IQ-TREE with the TVM+F+R3 model and 5,000 ultrafast bootstraps in PhyloSuite (Nguyen et al., 2015; Zhang et al., 2020). Bayesian Inference phylogenies were inferred using MrBayes 3.2.6 (Ronquist et al., 2012) under GTR+I+G+F model (12 parallel runs, 500,000 generations), in which the initial 25% of sampled data were discarded as burn-in. Trees were visualized in FigTree v1.3.1.
Divergence time estimation was performed using a relaxed log normal clock model in the BEAST v2.6.6 platform, using the GTR substitution model and a speciation Yule Process tree prior (Bouckaert et al., 2019). The Markov chain Monte Carlo chains (MCMC) were run for 900 million generations and sampled every 1,000 generations with a sampling frequency of 1,000 generations. Secondary calibration points for dating are listed in Table S2 according to previous studies (Xiang et al., 2018; Wei et al., 2019). The adequate effective sample size values (ESS > 200) were checked in Tracer 1.6. After a burn-in of 25%, a maximum clade credibility (MCC) tree with 95% highest posterior density intervals on each node was calculated using TreeAnnotator 2.1.3 and displayed in FigTree v1.3.1.
Based on the present distribution, we delimited four biogeographical areas: A, China mainland; B, Taiwan island; C, Japanese archipelago and a part of the Russian Far East; D, North America. We estimated ancestral distributions using the R package BioGeoBEARS implemented in Reconstruct Ancestral State in Phylogenies (RASP 4.0) (Yu Y. et al., 2020). The Dispersal Extinction Cladogenesis model with the jump dispersal parameter (DEC+J) was taken as the best model according to the model test in RASP (Table S3). In addition, we showed only the most likely status (MLS) for nodes where dispersal or vicariance had occurred.
Coptis occurrences and species diversity
The worldwide occurrence records of Coptis species were collected from the Global Biodiversity Information Facility (GBIF; occurrence download https://doi.org/10.15468/dl.zqzb7b) and the National Plant Specimen Resource Center (NSII; http://www.nsii.org.cn/). Two-step approach for distribution records quality control was carried out. First, checking the species name in The Plant List (http://www.theplantlist.org/), removed unresolved or incorrect species records and corrected synonyms species. Second, generating the respective distribution maps for each species in ArcGIS 10.8, manual check the points inconsistent with the flora description and verified the species credibility. After removing or revising distribution points, 9,182 distribution records of Coptis species were obtained (Table S4). The global land area was divided into 2° grid cell by ArcGIS and the number of species per grid were counted to determine the species diversity of Coptis. A density map was plotted to visualize the distribution pattern of Coptis species diversity.
Climatic variables and distribution modeling
In order to reduce sampling deviation in suitable habitats predicted by the MaxEnt model, we performed spatial rarefying of the data obtained in the previous step on a 10 km resolution using the SDM toolbox v2.5 and 2,898 records left for MaxEnt modeling (Brown et al., 2017).
A total of 19 climatic variables with a spatial resolution in 2.5’ for current climate data (average for 1970 - 2000) and four future periods data (BCC-CSM2-MR for 2021-2040, 2041-2060, 2061-2080, 2081-2100) used in the prediction of suitable species distributions were downloaded from WorldClim version 2.1 (https://www.worldclim.org/). We chose two scenarios, namely SSP126,and SSP585, to represent two extreme conditions in the future: a scenario with greenhouse gas well controlled at a low concentration (SSP126, 2.6 W/m2 in 2100) and a scenario with global warming trend without climate policy intervention (SSP585, 8.5 W/m2 in 2100), respectively (Zhao et al., 2021). All the climatic variables were converted to ASCII format using ArcGIS 10.8. To minimize overfitting of the MaxEnt model and ensure prediction accuracy, a Pearson correlation analysis was performed among the 19 climatic variables using the R package ggpairs (Emerson et al., 2013), and highly corelated ones were removed (> 0.75, Figure S1) (Dakhil et al., 2019). The correlation of climatic variables was tested all the species together, because one of our targets is to predict the whole genus potential suitable habitat and to raise the protection measures for Coptis. Ultimately, by using the software MaxEnt 3.4.1, eleven climatic variables were selected and entered along with the spatial rarefied occurrence data to predict potentially suitable distribution of Coptis species.
We set the cross-validation method to randomly selected 75% sites for model training, and the remaining for validation; the model was trained for ten replicate runs (Zhang Y. et al., 2021). Other parameters were kept as the default settings. The calculation result of the MaxEnt model was a grid layer in ASCII format and the value in each grid represented the potential suitable rate of species varying from 0 to 1. Then, we loaded the result into ArcGIS 10.8 to visualize the map of the potential species distribution and regrouped them into four levels as previous studies did: no suitability (0-0.2); low suitability (0.2-0.4); medium suitability (0.4-0.6); and high suitability (0.6-1) (Abolmaali et al., 2018; Zhang et al., 2018; Xu et al., 2019; Kong et al., 2021). Model calibrations and robustness validation were evaluated using the area under the curve (AUC) of the receiver operating characteristics (ROC) curve. The AUC value ranged from 0 to 1 with the following evaluation criteria: poor (0.6–0.7), fair (0.7–0.8), good (0.8–0.9), and excellent (0.9–1) (Zhao et al., 2021). In addition, the contribution rate and jackknife test generated by the MaxEnt model were used to measure the contribution weights of eleven climatic variables. The tool “Distribution changes between binary SDMs” in SDM toolbox v2.5 was used to calculate the changes in distribution area between adjacent time periods.
Result
Features and variation of plastomes genomes
Eleven Coptis plastomes were obtained by de novo assembly, and deposited in GenBank or National Genomics Data Center (NGDC) with the accession numbers listed in Table S1. The genomes ranged from 153,959 to 154,932 bp in size and contained 113 unique genes (80 protein coding genes, 29 tRNA genes, and four rRNA genes). The whole plastomes had a typical quadripartite structure including a pair of inverted-repeats (IR) regions (26,074 - 26,225 bp), large single copy (LSC) regions (84,112 - 85,178 bp) and small single copy (SSC) regions (17,215 - 17,606 bp). The average GC content ranged from 38.2 to 38.3%.
The results from the mVISTA program analysis showed that most mutation events occurring in the spacer regions and gene of the plastomes were highly conserved, especially in the exon regions (Figure S2). Total sequences had aligned length in 157,727 bp, which had 3,807 variable sites, 1,030 indels and the average nucleotide diversity was 0.0067. Based on the sliding window results, Pi and indels of Coptis species were visualized in a Circos map with a window size of each grid of 500 bp (Figure 1A). Most indels in the region were found in ndhF-rpl32. The Pi of a single window varied from 0 to 0.0359 and the indels of a single window varied from 0 to 40. Almost all mutations (92.5%) were located in the SSC and LSC regions, indicating highly conserved IR regions. Moreover, the most hypermutated region was located in the ycf1 gene. In order to reveal the detailed information of this region, a new sliding-window analysis was performed with settings of 50 bp window length and 25 step size. The most divergent region (Pi > 0.06) of the ycf1 gene was identified and limited to two tiny regions within 200 bp in size, considered as hotspots (Figure 1B). Compared to the three conventional plastid DNA barcodes (trnH-psbA, rbcL and matK), the two hotspots were nearly twice as high as the second highest barcode trnH-psbA and then followed by the complete ycf1 (Table 1).
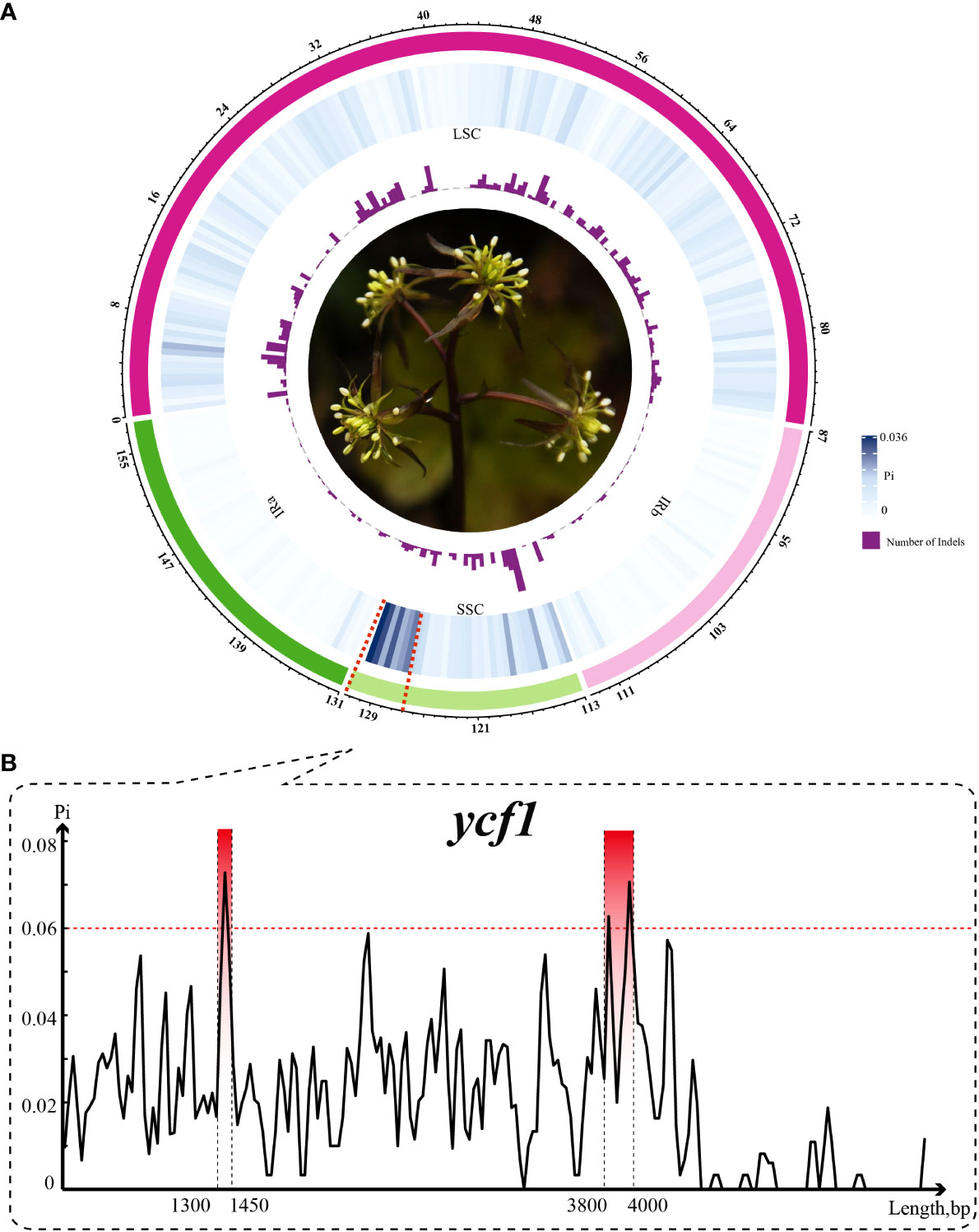
Figure 1 Variation of Coptis plastomes. (A) Circos plot showing the indel and nucleotide diversity of Coptis. Circles from the outer to inner area show the following: structure of plastomes indicated by different colors, nucleotide diversity shown by a heatmap and indel count shown by a histogram. Window size of each grid is 500 bp. (B) Sliding-window analysis of the ycf1 gene. The most divergent region (top three region with Pi > 0.06) is indicated.
Species distribution and diversity pattern
All the 9,182 occurrence records consisted of 15 Coptis species that were mainly found in China, Japan, Canada, and United States of America, as well as additionally scattered in the Russian Far East, Korean Peninsula and Greenland (Figure 2A). This clearly indicated that Coptis has a typical Eastern Asian and North American (EA-NA) disjunct distribution pattern. The number of species per grid, a reflection of species diversity, varied from one to six. The grids contained more than three species all located in Eastern Asia, especially in Mid-West China and Japan, which could indicate that these two regions are diversity hotspots of Coptis. For North America, the regions along the Pacific Coast Range were slightly more diverse than other regions.
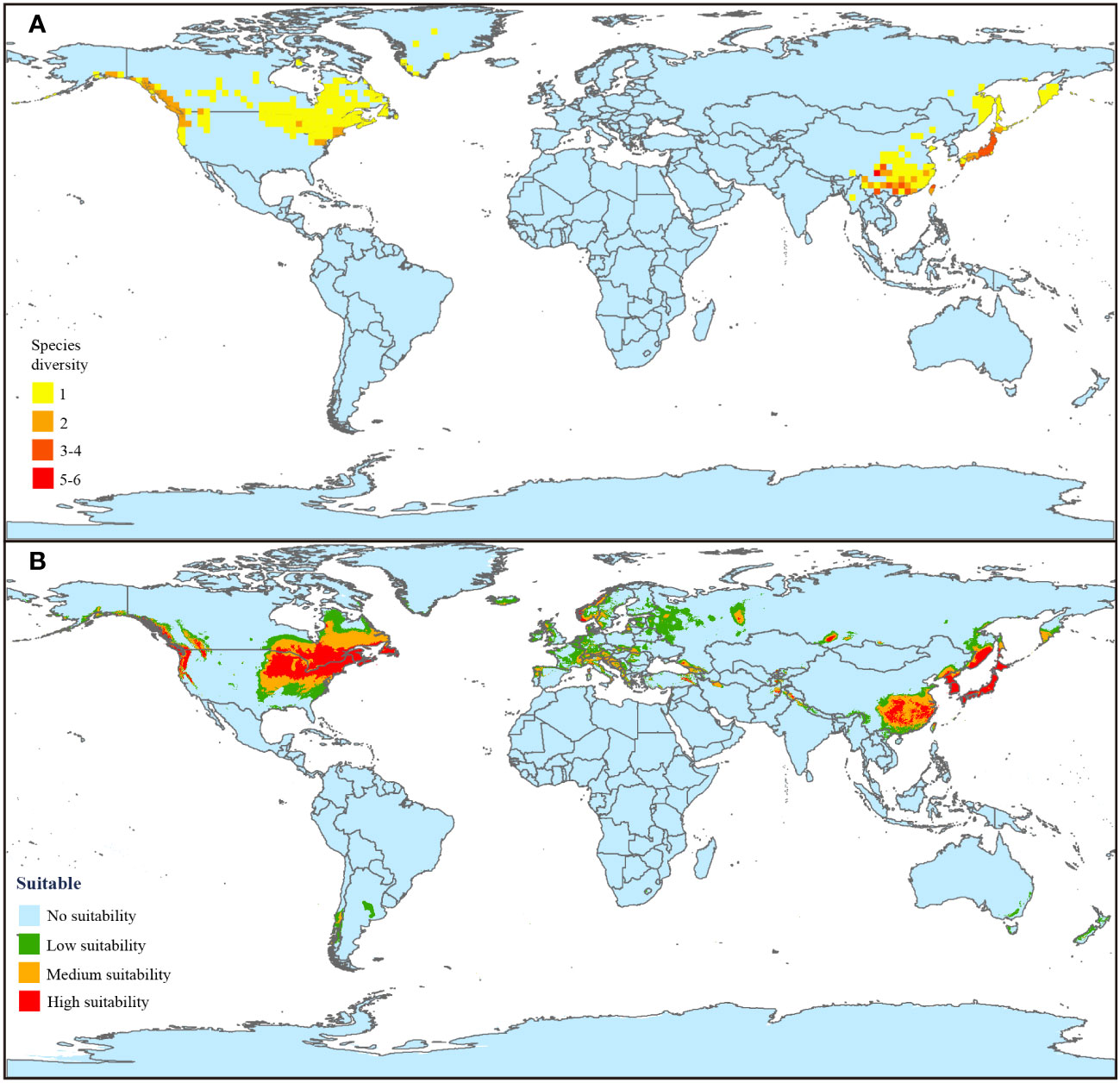
Figure 2 Current distribution and suitable habitat prediction of Coptis. (A) Current species distribution and diversity pattern. The global land area was divided into 2° grid cell by ArcGIS and the number of species per grid were counted to determine the species diversity of Coptis. The redder the grid, the higher the species diversity. (B) Current potential distribution of Coptis under 1970–2000 climate conditions. Four levels of suitability are shown in different colors as follows: no suitability (0-0.2, blue); low suitability (0.2-0.4, green); medium suitability (0.4-0.6, yellow); and high suitability (0.6-1, red).
Phylogeny, divergence time estimation and biogeographical history
The 19 aligned sequences for phylogenetic reconstruction were 191,086 bp in length and 152,783 bp left after trimming by Gblocks. All the Coptis species formed a monophyletic group with 100% support. Apparently, Coptis species were divided into two large clades, namely Clade I and Clade II (Figure 3 and Figure S3). Clade I (100% bootstrap value) comprised C. aspleniifolia, C. quinquesecta, C. japonica, C. teeta, C. omeiensis, C. deltoidei, C. chinensis var. chinensis and C. chinensis var. brevisepala. Species of Clade I were distributed disjunctively among three biogeographical areas (A, C and D). Clade II (100% bootstrap value) comprised C. trifolia, C. quinquefolia, C. ramosa and C. trifoliolata, and the latter two formed a monophyly. Additionally, Clade II also distributed disjunctively among three biogeographical areas (B, C and D).
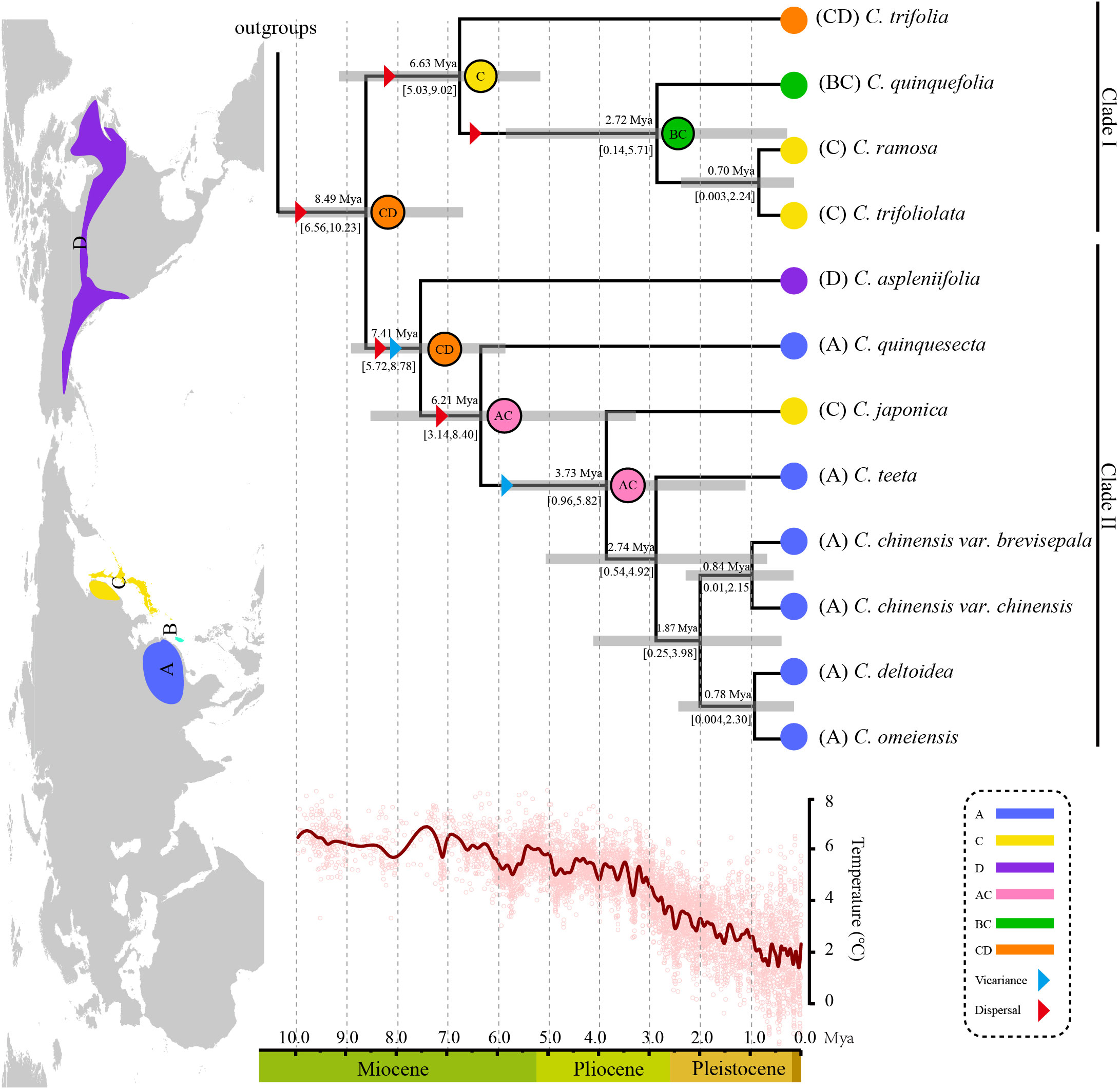
Figure 3 Combined dating analyses and ancestral habitat reconstruction of Coptis. The global temperature change in the past 10 Ma was obtained from Zachos et al. (Zachos et al., 2008). The insert Northern hemisphere map indicates the species distribution of Coptis used in the reconstruction with four defined biogeographical areas as follows: (A) mainland China; (B), Taiwan island; (C), Japanese archipelago and a part of the Russian Far East; (D), North America. Dated phylogeny of Coptis was derived from Figure S6. Numbers above and under the branches indicate the mean divergence times and 95% confidence interval of each node, respectively. Blue bars indicate the 95% highest posterior density intervals. Letters and colors in the legend represent extant ancestral areas and combination of them. Pie chart labeled with letters at each node indicates the most likely ancestral area.
According to the combined dating and RASP results, the Coptis genus was most likely to have originated in North America and the Japanese archipelago and the crown age was estimated to be at around 8.49 Mya (95% HPD: 6.56 – 10.23 Mya) in the late Miocene, when the two clades first diverged. In Clade I, C. trifolia first split off at around 6.63 Mya when a Bering Land Bridge dispersal (C & D) occurred. Then, the second dispersal (B & C) occurred at around 2.72 Mya when C. quinquefolia split off from C. ramose and C. trifoliolata. Species diversification in Clade II was gradual and successive. C. aspleniifolia diverged from Asian species to North American probably around 7.41 Mya. Moreover, C. quinquesecta and C. japonica successively separated at around 6.21 Mya and 3.73 Mya, corresponding respectively to a dispersal and a vicariance event that occurred between the Chinese mainland and Japanese archipelago with a part of the Russian Far East. This vicariance led to the colonization of Coptis in mainland China and the subsequent speciation into five species. In addition, these five species formed a subclade, in which C. teeta separated in 2.74 Mya, followed by a divergence of C. chinensis from its closest relatives (C. deltoidei and C. omeiensis) that occurred in 1.87 Mya.
Modeling validation and dominant climatic variables
The MaxEnt model was used to simulate the suitable habitats of Coptis. The final ROC curve (shown in Figure S4), revealed that the average test AUC for the replicate runs was 0.883, with a standard deviation of 0.005, which indicated that the MaxEnt model performed efficiently and reliably in the prediction of suitable habitats.
The eleven selected climatic variables involved in potential suitable habitat prediction are listed in Table 2. The contribution weight of selected climatic variables is shown by the contribution rate and jackknife test result. The variable with the highest contribution rate was precipitation of the driest quarter (Bio 17, contribution rate 38.1%), followed by annual precipitation (Bio 12, contribution rate 37.4%) and annual mean temperature (Bio 1, contribution rate 10.2%). The jackknife test results revealed that the highest gain when used in isolation was still precipitation of the driest quarter (Bio 17, training gain 0.83), followed by annual mean temperature (Bio 1, training gain 0.80) and annual precipitation (Bio 12, training gain 0.79). It is undisputable that these three factors were the most dominant climatic variables in influencing the potential suitable habitat of Coptis, while the Bio 1 and Bio12 rankings are different by two assessment methods.
The response curve of the three most dominant climatic variables shown in Figure S5 was generated by the MaxEnt model and illustrates the quantitative relationship between the species presence probability and climatic variables and clearly elucidated the suitability conditions of Coptis under different climatic variables. According to the levels of high suitability (> 0.6), the corresponding climatic conditions should be suitable for species growth. In terms of the three dominant climatic variables, the optimum ranges of precipitation of the driest quarter should be more than 25.5 mm, annual precipitation should be more than 844.9 mm and annual mean temperature should range from -3.1 to 19.9°C.
Current and future potential habitat prediction and dynamic changes
Based on the current climate condition (1970 - 2000) and occurrence records of Coptis, the global potential suitable habitat (suitable rate > 0.2) projected by the MaxEnt model was 1,315.0×104 km2, which was mostly located in the northern temperate zone, and rarely occurred in tiny regions of New Zealand, Australia, Argentina and Chile (Figure 2B). In China, the potential habitat was 239.6×104 km2, comprising 18% of global suitable area, whose area of the three suitable levels (suitable rate > 0.2) from high to low were 57.7×104, 112.2×104 and 69.7×104 km2, respectively. The global potential high, medium, and low suitable areas covered 328.9×104, 409.9×104 and 576.2×104 km2, respectively, and the high suitable area were restricted to the eastern border of the United States of America and Canada, the Pacific Coast Range, most of Northeast Asia, and Mid-West China.
Two climate change scenarios (SSP585 and SSP126) were analyzed to predict the suitable habitat in the future four periods from 2021 to 2100 (Figure 4). As mentioned above, SSP585 is a scenario with no policy intervention and the radiative forcing will rise to 8.5 W/m2 by 2100. In this scenario, the global potential suitable area (suitable rate > 0.2) has an upward trend followed by a downward trend with the peak occurring at 1,648.8×104 km2 (2061-2080). Meanwhile, the potential center noticeably shifts north. Correspondingly, the area in China declines continuously from 237.7×104 km2 (2021-2040) to 172.3×104 km2 (2081-2100). In particular, the high suitable area (suitable rate > 0.6) in China decreases most significantly, from 22.7×104 km2 (2021-2040) to merely 1.7×104 km2 (2081-2100). In the SSP126 scenario, the global warming trend may have been suppressed by 2100, the global suitable area shows a slight upward trend (Figure 5). The potential suitable area (suitable rate > 0.2) increases from 1,564.8×104 km2 (2021-2040) to 1,606.4×104 km2 (2081-2100) and the center of the suitable area is basically stable. In addition, in contrast to the SSP558 scenario, the suitable area in China shows a gradual upward trend, from 233.0×104 km2 (2021-2040) to 266.1×104 km2 (2081-2100). Also, the decline of the high suitable area (suitable rate > 0.6) is halted and rebounds to 18.6×104 km2 (2081-2100). The shifts of distribution areas between adjacent time periods under two scenarios were vividly presented in Figure S7.
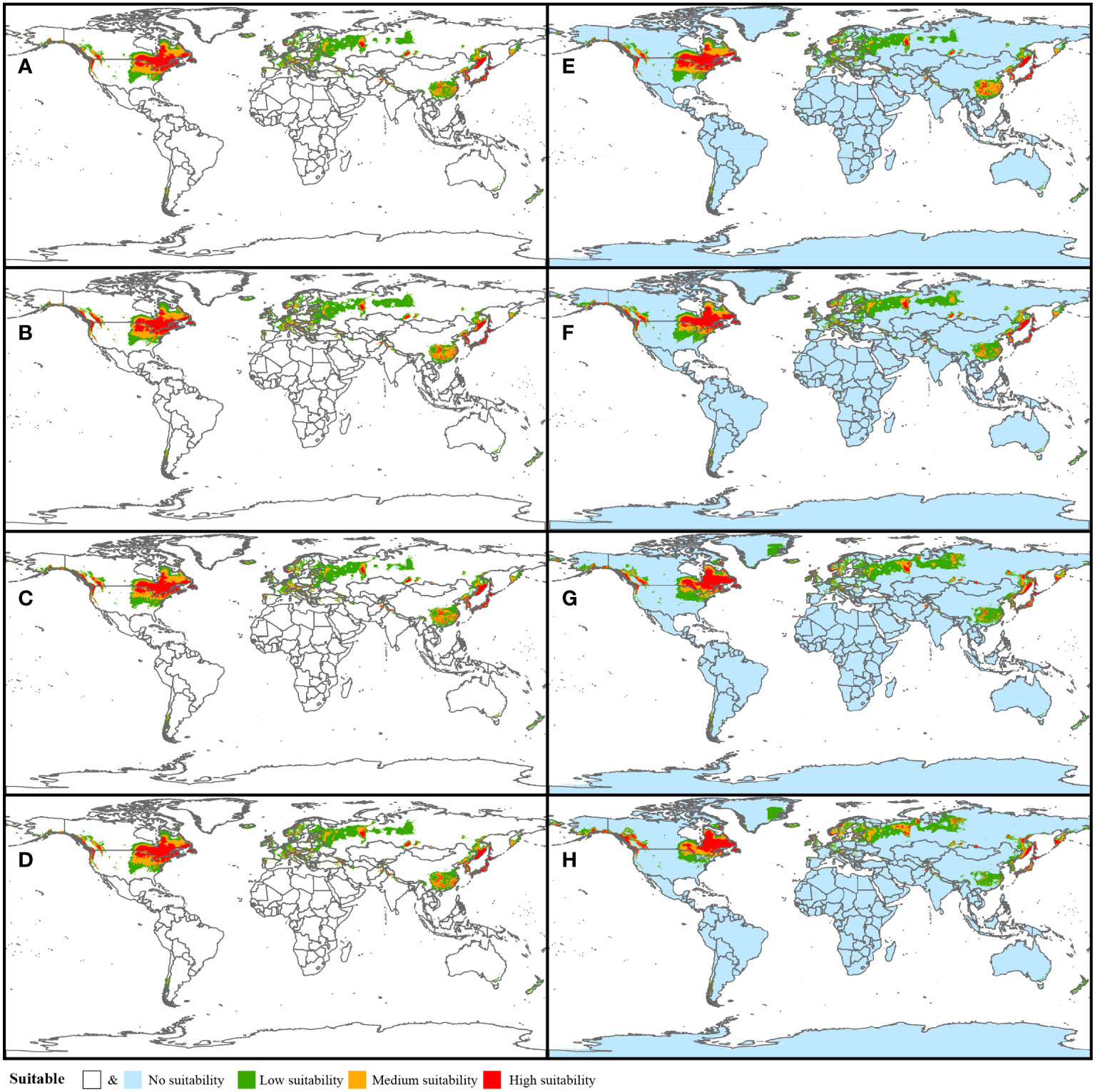
Figure 4 Potential habitat prediction of Coptis in the future periods: 2021-2040 (SSP126, A and SSP 585, E), 2041-2060 (SSP126, B and SSP 585, F), 2061-2080 (SSP126, C and SSP 585, G) and (SSP126, D and SSP 585, H).
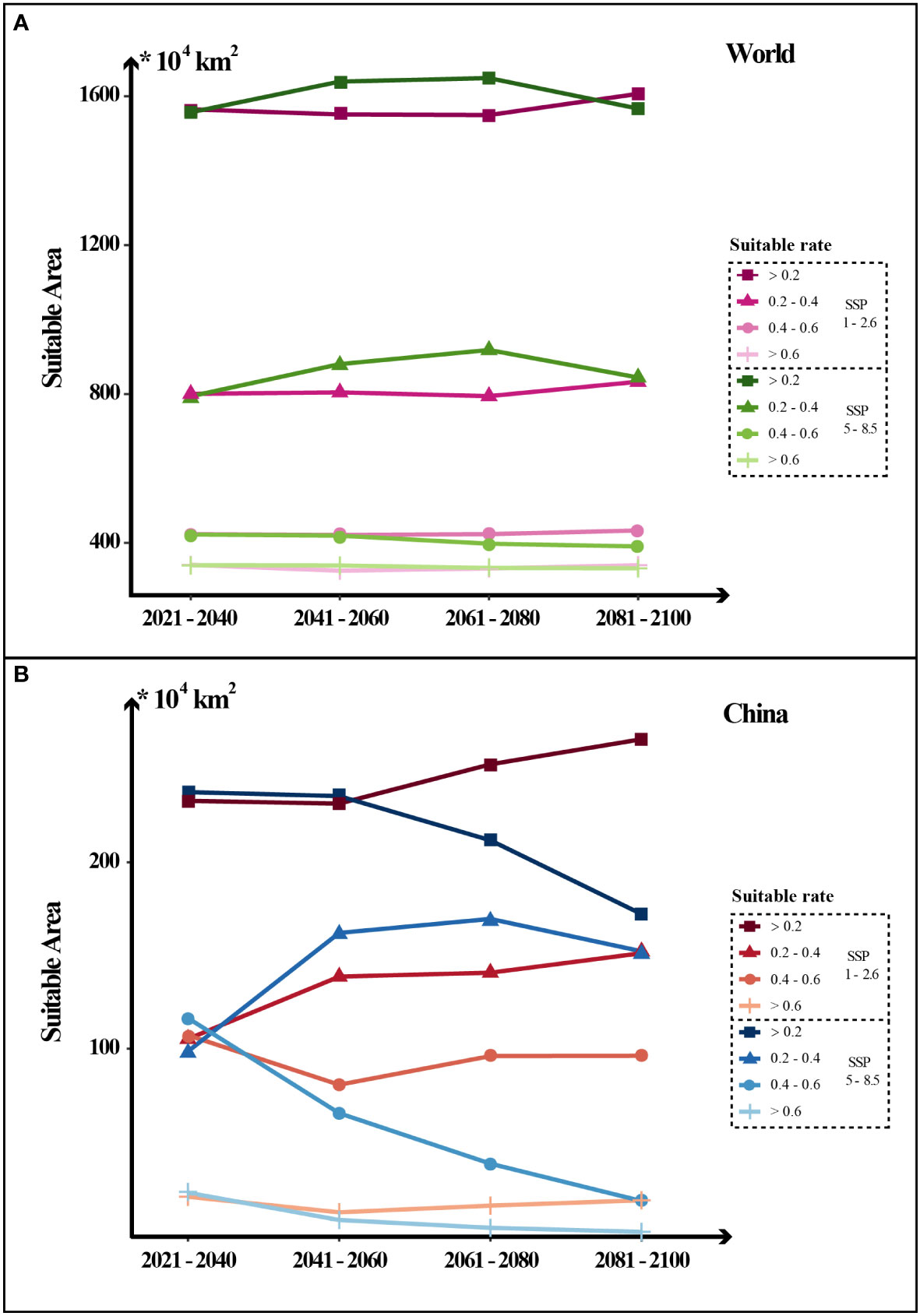
Figure 5 Dynamic changes in the area of potential Coptis suitable distribution in the world and China in the future four periods under two greenhouse gas emission scenarios (SSP126 and SSP585). The levels of suitability are shown in different line types: low suitability (0.2-0.4); medium suitability (0.4-0.6); high suitability (0.6-1) and total suitability (> 0.2, the sum of high, medium and low levels).
Discussion
Genetic divergence of Coptis species and candidate DNA barcodes
As mentioned above, Coptis is a genus with enormous value in research and development. Genetic diversity defines the evolutionary potential and resilience of species (Sun et al., 2021). Thus, it is crucially important to evaluate the genetic diversity of this genus. However, there is no comprehensive plastid genetic resource available. In this study, twelve Coptis plastomes were found to be highly conserved in genome structure and gene order, and no rearrangement occurred. Some variations were observed in the GC content and genome size. The GC content varied from 38.2 to 38.3% and the genome size varied from 153,959 to 154,932 bp, indicating the existence of genetic diversity. Compared to other medicinal plants, such as Artemisia (Pi = 0.0024), Atractylodes (Pi = 0.001), Crataegus (Pi = 0.00175) and Ligusticum (Pi = 0.002), Coptis (Pi = 0.0067) is a medicinal genus with relatively high genetic diversity (Kim et al., 2020; Wang Y. et al., 2021; Wu et al., 2022; Wei et al., 2022).
The mVISTA results revealed that, under evolutionary constrains and natural selection pressure, the noncoding region was more variable than the coding region (Zhang H. et al., 2021). According to the sliding-window analysis and Circos map, the LSC and SSC regions were more variable than the IR regions and may be possibly due to copy corrections between IR sequences by gene conversion (Zhao et al., 2018). In addition, it also revealed that the mutations were not uniformly or randomly distributed across all regions of the plastome. The aggregation of mutations in certain regions creates hotspots. Hotspots are potential DNA barcode development region that are usually efficient for interspecies discrimination and phylogeny reconstruction (Downie and Jansen, 2015; Song et al., 2017; Pang et al., 2019). Clearly, the hotspot regions of Coptis were located in the junction of SSC and IRs, which belonged to the ycf1 gene. As the second largest gene in the plastid genome, ycf1 is recognized for its variability in seed plants (Dong et al., 2015). Since it is very long (more than 5 kb) and quite variable in application, two highly mutant regions, namely ycf1a (800 bp) and ycf1b (1100 bp), of ycf1 have been developed and used as the most promising plastid DNA barcodes (Dong et al., 2012). Coincidentally, in this study, the precise locations of two hotspots in the ycf1 were identified, which corresponded to the ycf1a and ycf1b mutant regions, respectively. Furthermore, the size of these two hotspots were limited to 200 bp. Compared to the three conventional plastid DNA barcodes (trnH-psbA, rbcL and matK), these two regions are much shorter in size but more informative, which qualifies them as mini-barcodes. Notably, mini barcodes may facilitate discrimination of DNA degradation materials, such as herbarium specimens, processed medicinal plant, or even fossil material. Moreover, this strategy of developing taxon-specific barcodes by comparing plastid genome sequences has also been applied in other medicinal plant taxa, including Panax, Senna, and Paeonia (Dong et al., 2014; Yu X. et al., 2020; Yang et al., 2022). Collectively, these two hotspots should be promising mini-barcodes for Coptis species identification in future applications.
Phylogenetic inferences and evolutionary history
Elucidation of the phylogenetic relationship of Coptis is crucial in understanding its evolutionary history. In previous studies, the phylogeny reconstruction of Coptis was performed using a small number of DNA loci, such as trnL-F, trnD-T, trnH-psbA, rpoB, accD, rbcL, or limited sampling, which resulted in inconsistent phylogenetic relationships, especially for the four late-diverging species: C. deltoidea, C. omeiensis, C. chinensis var. chinensis and C. chinensis var. brevisepala (He et al., 2014; Xiang et al., 2016; Xiang et al., 2018; Wang et al., 2020). Xiang et al. (2016) suggested that C. chinensis var. brevisepala formed a clade with C. deltoidea and C. omeiensis, while C. chinensis var. chinensis was separated in the early-diverged position based on the matrix combining trnL-F, trnH-psbA and ITS. Also, Wang et al. indicated that C. chinensis var. brevisepala was in the early-diverged part of these four species based on the dataset comprising trnH-psbA, rbcL and matK. Importantly, based on adequate sampling and whole plastid genomes with adequate genetic information, this study revealed the most comprehensive phylogeny for Coptis. The robust relationship was reconstructed and these controversial branches were fully resolved.
The evolutionary history was clearly shown by estimating the divergence time and biogeography of Coptis. The species-level age estimation suggested a crown group age of 8.49 Mya (95% HPD: 6.56 – 10.23 Mya) in the late Miocene, which was largely consistent with previous results with an age of 9.55 Mya (95% HPD: 6.66–12.92 Mya) determined by DNA barcodes (Xiang et al., 2018). In the middle to late Miocene, the most significant events influencing the evolutionary patterns of global plants were the uplift of the Qinghai-Tibetan Plateau in East Asia and the Rocky Mountains in western North America. These two significant tectonic events in the eastern and western hemispheres had effects on global atmospheric circulation, weathering rates, monsoon and even riverway trend, which might be the main factors underlying the origin and diversification of Coptis (Qiu et al., 2011; Pound et al., 2011). Coptis probably originated in the Japanese archipelago and a part of the Russian Far East (area C) and North America (area D), and the current distribution of Coptis seems to have been shaped by several dispersal and vicariance events. The Bering Land Bridge was the most likely dispersal corridor between the two continents, which has been considered as an explanation for the disjunct distribution of related extant floras in EA and NA. Furthermore, sea level fluctuations and climate cooling since the Pliocene have provided abundant opportunities for promoting dispersal and vicariance among the three Asian biogeographical areas and speciation of Coptis occurred rapidly since that time (Haq et al., 1987; Qian and Ricklefs, 2004; Qiu et al., 2011; Xiang, 2020).
It is well accepted that the climatic oscillations, sea-level fluctuations and land bridge configuration promoted speciation and extinction, shaped distribution and diversity pattern of species (Qian and Ricklefs, 2000; Qiu et al., 2009). The diversity center of Coptis is located in Japan and the mid-west of mainland China, which are the major parts of the widely recognized biodiversity hotspots “Sino-Japanese Floristic Region” (Hanson et al., 2010). Moreover, for disjunct distributed taxa, diversity is generally higher in East Asia than in North America. The main reason is that East Asia was less influenced by ice sheets during the Quaternary period thus having a lower species extinction rate, and older and more complex topographical features than North America, which may create this biodiversity hotspot in the world with much more species diversity than that in North America (Qian, 2002).
Conservation implications for Coptis
Temperature and precipitation are regarded as two of the main variables restricting the range of the majority of terrestrial plant species, as well as that of Coptis. As shown by the contribution weight of dominant climatic variables in the results, precipitation is one of the most important factors in future conservation. Understanding the suitable climatic ranges and trend of changes in potential suitable habitat for Coptis (precipitation of driest quarter > 25.5 mm, annual precipitation > 844.9 mm and annual mean temperature -3.1 to 19°C), may contribute to provide a basis for ex-situ conservation strategies and the establishment of ex-situ resource nursery for Coptis in the future and will also be useful for guiding cultivation and introduction of domestication.
Currently, global climate change greatly affects the distribution of various species and it is also a challenge for all of humanity. Under two greenhouse gas emission scenarios (SSP126 and SSP585), the suitable distribution area of Coptis varies largely. In the low-emission condition, the suitable area (suitable rate > 0.2) in world is stable or even shows a slight upward trend, and it is increasing significantly in China. However, without climate policy intervention, the global suitable (suitable rate > 0.2) distribution moves northward and the area in China precipitously declines. The results demonstrate the necessity of tight regulation of greenhouse gas emissions. Confronted by the challenge of climate change, it is important for the state parties and signatories to fulfill the Paris Agreement to reduce their carbon output. These actions should be beneficial not only for the Coptis conservation, particularly in China, but also for all lives in the world.
Data availability statement
The datasets presented in this study can be found in online repositories. The names of the repository/repositories and accession number(s) can be found in the article/Supplementary Material.
Author contributions
YW and JS wrote and revised the manuscript. PQ participated in the experiments. JW, YD, JL, QY, YW, MW and FX collected the materials and analyzed the data. WD, LH and LG conceived and designed the research. All authors contributed to the article and approved the submitted version.
Funding
This research was funded by CACMS innovation Fund (No.CI2021A03909), National Key Research and Development Program of China (2017YFC1703700: 2017YFC1703704), National Natural Science Foundation of China (No.81891014 & No.81874337), Innovation Team and Talents Cultivation Program of National Administration of Traditional Chinese Medicine (No. ZYYCXTD-D-202005) and Genetic Resources Management Project of State Forestry and Grassland Administration (KJZXSA202105).
Acknowledgments
The authors would like to thank professor Shiliang Zhou, Dr. Chao Xu, Kangjia Liu and Enze Li for providing suggestions, and thank the DNA Bank of China in Institute of Botany, Chinese Academy of Sciences for providing materials.
Conflict of interest
The authors declare that the research was conducted in the absence of any commercial or financial relationships that could be construed as a potential conflict of interest.
Publisher’s note
All claims expressed in this article are solely those of the authors and do not necessarily represent those of their affiliated organizations, or those of the publisher, the editors and the reviewers. Any product that may be evaluated in this article, or claim that may be made by its manufacturer, is not guaranteed or endorsed by the publisher.
Supplementary material
The Supplementary Material for this article can be found online at: https://www.frontiersin.org/articles/10.3389/fpls.2022.1003368/full#supplementary-material
References
Abolmaali, S., Tarkesh, M., Bashari, H. (2018). MaxEnt modeling for predicting suitable habitats and identifying the effects of climate change on a threatened species, Daphne mucronata, in central Iran. Ecol. Inform 43, 116–123. doi: 10.1016/j.ecoinf.2017.10.002
Aguirre Planter, E., Parra Leyva, J. G., Ramirez Barahona, S., Scheinvar, E., Lira Saade, R., Eguiarte, L. E. (2020). Phylogeography and genetic diversity in a southern north American desert: Agave kerchovei from the tehuacan cuicatlan valley, Mexico. Front. Plant Sci. 11. doi: 10.3389/fpls.2020.00863
Bolger, A. M., Marc, L., Bjoern, U. (2014). Trimmomatic: a flexible trimmer for illumina sequence data. Bioinformatics 15), 2114–2120. doi: 10.1093/bioinformatics/btu170
Bouckaert, R., Vaughan, T. G., Barido Sottani, J., Duchene, S., Fourment, M., Gavryushkina, A., et al. (2019). BEAST 2.5: an advanced software platform for Bayesian evolutionary analysis. PloS Comput. Biol. 15 (4), e1006650. doi: 10.1101/474296
Brown, J., Bennett, J., French, C. (2017). SDMtoolbox 2.0: the next generation Python-based GIS toolkit for landscape genetic, biogeographic and species distribution model analyses. PeerJ 5, e4095. doi: 10.7717/peerj.4095
Castresana, J. (2000). GBLOCLKS: selection of conserved blocks from multiple alignments for their use in phylogenetic analysis. Mol. Biol. Evol. 17 (4), 540–552. doi: 10.1093/oxfordjournals.molbev.a026334
Chen, D., Pan, Y., Wang, Y., Cui, Y., Zhang, Y., Mo, R., et al. (2021). The chromosome-level reference genome of coptis chinensis provides insights into genomic evolution and berberine biosynthesis. Hortic. Res. 8, 121. doi: 10.1038/s41438-021-00559-2
Dakhil, M. A., Xiong, Q., Farahat, E. A., Zhang, L., Pan, K., Pandey, B., et al. (2019). Past and future climatic indicators for distribution patterns and conservation planning of temperate coniferous forests in southwestern China. Ecol. Indic 107, 105559. doi: 10.1016/j.ecolind.2019.105559
Dong, W., Li, E., Liu, Y., Xu, C., Wang, Y., Liu, K., et al. (2022). Phylogenomic approaches untangle early divergences and complex diversifications of the olive plant family. BMC Biol. 20 (1), 1–25. doi: 10.1186/s12915-022-01297-0
Dong, W., Liu, J., Jing, Y., Wang, L., Zhou, S. (2012). Highly variable chloroplast markers for evaluating plant phylogeny at low taxonomic levels and for DNA barcoding. PloS One 7 (4), e35071. doi: 10.1371/journal.pone.0035071
Dong, W., Liu, Y., Li, E., Xu, C., Sun, J., Li, W., et al. (2021a). Phylogenomics and biogeography of catalpa (Bignoniaceae) reveal incomplete lineage sorting and three dispersal events. Mol. Phylogenet Evol. 166, 107330. doi: 10.1016/j.ympev.2021.107330
Dong, W., Liu, H., Xu, C., Zuo, Y., Chen, Z., Zhou, S. (2014). A chloroplast genomic strategy for designing taxon specific DNA mini-barcodes: a case study on ginsengs. BMC Genet. 15, 138. doi: 10.1186/s12863-014-0138-z
Dong, W., Sun, J., Liu, Y., Xu, C., Wang, Y., Suo, Z., et al. (2021b). Phylogenomic relationships and species identification of the olive genus olea (Oleaceae). J. Syst. Evol., 60(6), 1–18. doi: 10.1111/jse.12802
Dong, W., Xu, C., Li, C., Sun, J., Zuo, Y., Shi, S., et al. (2015). Ycf1, the most promising plastid DNA barcode of land plants. Sci. Rep. 5, 8348. doi: 10.1038/srep08348
Downie, S. R., Jansen, R. K. (2015). A comparative analysis of whole plastid genomes from the apiales: expansion and contraction of the inverted repeat, mitochondrial to plastid transfer of DNA, and identification of highly divergent noncoding regions. Syst. Bot. 40 (1), 336–351. doi: 10.1600/036364415X686620
Duan, L., Harris, A., Su, C., Ye, W., Deng, S., Fu, L., et al. (2020). A fossil-calibrated phylogeny reveals the biogeographic history of the cladrastis clade, an amphi-pacific early-branching group in papilionoid legumes. Mol. Phylogenet Evol. 143, 106673. doi: 10.1016/j.ympev.2019.106673
Emerson, J. W., Green, W. A., Schloerke, B., Crowley, J., Cook, D., Hofmann, H., et al. (2013). The generalized pairs plot. J. Comput. Graph Stat. 22 (1), 79–91. doi: 10.1080/10618600.2012.694762
Feng, Y., Shen, T., Shao, C., Du, H., Ran, J., Wang, X. (2021). Phylotranscriptomics reveals the complex evolutionary and biogeographic history of the genus tsuga with an East Asian-north American disjunct distribution. Mol. Phylogenet Evol. 157, 107066. doi: 10.1016/j.ympev.2020.107066
Gu, Z., Gu, L., Eils, R., Schlesner, M., Brors, B. (2014). Circlize implements and enhances circular visualization in r. Bioinformatics 30 (19), 2811–2812. doi: 10.1093/bioinformatics/btu393
Hanson, T., Brooks, T. M., Fonseca, G., Hoffmann, M., Lamoreux, J. F., Machlis, G., et al. (2010). Warfare in biodiversity hotspots. Conserv. Biol. 23 (3), 578–587. doi: 10.1111/j.1523-1739.2009.01166.x
Haq, B. U., Hardenbol, J., Vail, P. R. (1987). Chronology of fluctuating sea levels since the Triassic. Science 235 (4793), 1156–1167. doi: 10.1126/science.235.4793.1156
He, Y., Hou, P., Fan, G., Arain, S., Peng, C. (2014). Comprehensive analyses of molecular phylogeny and main alkaloids for coptis (Ranunculaceae) species identification. Biochem. Syst. Ecol. 56, 88–94. doi: 10.1016/j.bse.2014.05.002
Huang, X., Ma, L., Chen, C., Zhou, H., Yao, B., Ma, Z. (2020). Predicting the suitable geographical distribution of sinadoxa corydalifolia under different climate change scenarios in the three-river region using the MaxEnt model. Plants (Basel) 9 (8), 1015-1029. doi: 10.3390/plants9081015
Jin, J., Yu, W., Yang, J., Song, Y., dePamphilis, C. W., Yi, T., et al. (2020). GetOrganelle: a fast and versatile toolkit for accurate de novo assembly of organelle genomes. Genome Biol. 21 (1), 241. doi: 10.1186/s13059-020-02154-5
Kalyaanamoorthy, S., Minh, B. Q., Wong, T. K. F., Von Haeseler, A., Jermiin, L. S. (2017). ModelFinder: fast model selection for accurate phylogenetic estimates. Nat. Methods 14 (6), 587–589. doi: 10.1038/nmeth.4285
Kim, G., Lim, C., Kim, J., Kim, K., Lee, J., Yu, H., et al. (2020). Comparative chloroplast genome analysis of artemisia (Asteraceae) in East Asia: insights into evolutionary divergence and phylogenomic implications. BMC Genomics 21 (1), 415. doi: 10.1186/s12864-020-06812-7
Kong, F., Tang, L., He, H., Yang, F., Tao, J., Wang, W. (2021). Assessing the impact of climate change on the distribution of osmanthus fragrans using maxent. Environ. Sci. pollut. Res. Int. 28 (26), 34655–34663. doi: 10.1007/s11356-021-13121-3
Librado, P., Rozas, J. (2009). DnaSP v5: a software for comprehensive analysis of DNA polymorphism data. Bioinformatics 25 (11), 1451–1452. doi: 10.1093/bioinformatics/btp187
Li, J., Fan, G., He, Y. (2020). Predicting the current and future distribution of three coptis herbs in China under climate change conditions, using the MaxEnt model and chemical analysis. Sci. Total Environ. 698, 134141. doi: 10.1016/j.scitotenv.2019.134141
Liu, Y., Wang, B., Shu, S., Li, Z., Song, C., Liu, D., et al. (2021). Analysis of the coptis chinensis genome reveals the diversification of protoberberine-type alkaloids. Nat. Commun. 12 (1), 3276. doi: 10.1038/s41467-021-23611-0
Li, J., Wang, S., Yu, J., Wang, L., Zhou, S. (2013). A modified CTAB protocol for plant DNA extraction. Chin. Bull. Bot. 48 (1), 72–78. doi: 10.3724/SP.J.1259.2013.00072
Ma, K., Wang, X., Feng, S., Xia, X., Zhang, H., Rahaman, A., et al. (2020). From the perspective of traditional Chinese medicine: treatment of mental disorders in COVID-19 survivors. BioMed. Pharmacother. 132, 110810. doi: 10.1016/j.biopha.2020.110810
Minami, H., Dubouzet, E., Iwasa, K., Sato, F. (2007). Functional analysis of norcoclaurine synthase in coptis japonica. J. Biol. Chem. 282 (9), 6274–6282. doi: 10.1074/jbc.M608933200
Nge, F. J., Biffin, E., Waycott, M., Thiele, K. R. (2022). Phylogenomics and continental biogeographic disjunctions: insight from the Australian starflowers (Calytrix). Am. J. Bot. 109 (2), 291–308. doi: 10.1002/ajb2.1790
Nguyen, L. T., Schmidt, H. A., von Haeseler, A., Minh, B. Q. (2015). IQ-TREE: a fast and effective stochastic algorithm for estimating maximum-likelihood phylogenies. Mol. Biol. Evol. 32 (1), 268–274. doi: 10.1093/molbev/msu300
Pang, X., Liu, H., Wu, S., Yuan, Y., Li, H., Dong, J., et al. (2019). Species identification of oaks (Quercus l., fagaceae) from gene to genome. Int. J. Mol. Sci. 20, (23). doi: 10.3390/ijms20235940
Pound, M. J., Haywood, A. M., Salzmann, U., Riding, J. B., Lunt, D. J., Hunter, S. J. (2011). A tortonian (late Miocene, 11.61–7.25 ma) global vegetation reconstruction. Palaeogeogr Palaeocl 300 (1-4), 29–45. doi: 10.1016/j.palaeo.2010.11.029
Qian, H. (2002). A comparison of the taxonomic richness of temperate plants in East Asia and north America. Am. J. Bot. 89 (11), 1818–1825. doi: 10.3732/ajb.89.11.1818
Qian, H., Ricklefs, R. E. (2000). Large-Scale processes and the Asian bias in species diversity of temperate plants. Nature 407 (6801), 180–182. doi: 10.1038/35025052
Qian, H., Ricklefs, R. E. (2004). Geographical distribution and ecological conservatism of disjunct genera of vascular plants in eastern Asia and eastern north America. J. Ecol. 92 (2), 253–265. doi: 10.1111/j.0022-0477.2004.00868.x
Qiu, Y., Fu, C., Comes, H. (2011). Plant molecular phylogeography in China and adjacent regions: tracing the genetic imprints of quaternary climate and environmental change in the world's most diverse temperate flora. Mol. Phylogenet Evol. 59 (1), 225–244. doi: 10.1016/j.ympev.2011.01.012
Qiu, Y., Sun, Y., Zhang, X., Lee, J., Fu, C., Comes, H. (2009). Molecular phylogeography of East Asian kirengeshoma (Hydrangeaceae) in relation to quaternary climate change and landbridge configurations. New Phytol. 183 (2), 480–495. doi: 10.1111/j.1469-8137.2009.02876.x
Ronquist, F., Teslenko, M., van der Mark, P., Ayres, D. L., Darling, A., Hohna, S., et al. (2012). MrBayes 3.2: Efficient Bayesian phylogenetic inference and model choice across a Large model space. Syst. Biol. 61 (3), 539–542. doi: 10.1093/sysbio/sys029
Shi, L., Chen, H., Jiang, M., Wang, L., Wu, X., Huang, L., et al. (2019). CPGAVAS2, an integrated plastome sequence annotator and analyzer. Nucleic Acids Res. 47 (W1), W65–W73. doi: 10.1093/nar/gkz345
Song, Y., Li, Y., Meng, H., Fragniere, Y., Ge, B., Sakio, H., et al. (2020). Phylogeny, taxonomy, and biogeography of pterocarya (Juglandaceae). Plants (Basel) 9, (11). doi: 10.3390/plants9111524
Song, Y., Wang, S., Ding, Y., Xu, J., Li, M. F., Zhu, S., et al. (2017). Chloroplast genomic resource of paris for species discrimination. Sci. Rep. 7 (1), 3427. doi: 10.1038/s41598-017-02083-7
Sun, J., Wang, Y., Garran, T. A., Qiao, P., Wang, M., Yuan, Q., et al. (2021). Heterogeneous genetic diversity estimation of a promising domestication medicinal motherwort leonurus cardiaca based on chloroplast genome resources. Front. Genet. 12. doi: 10.3389/fgene.2021.721022
Wang, X., Liu, X., Ko, Y., Jin, X., Sun, J., Zhao, Z., et al. (2020). Genetic diversity and phylogeography of the important medical herb, cultivated Huang-lian populations, and the wild relatives coptis species in China. Front. Genet. 11. doi: 10.3389/fgene.2020.00708
Wang, Y., Wang, S., Liu, Y., Yuan, Q., Sun, J., Guo, L. (2021). Chloroplast genome variation and phylogenetic relationships of atractylodes species. BMC Genomics 22 (1), 103. doi: 10.1186/s12864-021-07394-8
Wang, Z., Zhong, C., Li, D., Yan, C., Yao, X., Li, Z. (2021). Cytotype distribution and chloroplast phylogeography of the actinidia chinensis complex. BMC Plant Biol. 21 (1), 325. doi: 10.1186/s12870-021-03099-y
Wei, Z., Xiaoshan, D., Rui, Z., Chunce, G., Lin, L., Guixia, X., et al. (2019). Chloroplast genomic data provide new and robust insights into the phylogeny and evolution of the Ranunculaceae. Mol. Phylogenet Evol. 135, 12-21. doi: 10.1016/j.ympev.2019.02.024
Wei, X., Zhang, X., Dong, Y., Cheng, J., Bai, Y., Liu, J., et al. (2022). Molecular structure and phylogenetic analyses of the complete chloroplast genomes of three medicinal plants conioselinum vaginatum, ligusticum sinense, and ligusticum jeholense. Front. Plant Sci. 13. doi: 10.3389/fpls.2022.878263
Wu, X., Luo, D., Zhang, Y., Yang, C., Crabbe., M. J. C., Zhang, T., et al. (2022). Comparative genomic and phylogenetic analysis of chloroplast genomes of hawthorn (Crataegus spp.) in southwest China. Front. Genet. 13, 900357. doi: 10.3389/fgene.2022.900357
Xiang, K. (2020). “Phylogeny and diversification of ranunculaceae,” in PhD Dissertation (University of Chinese Academy of Sciences).
Xiang, K., Erst, A., Xiang, X., Jabbour, F., Wang, W. (2018). Biogeography of coptis salisb. (Ranunculales, ranunculaceae, coptidoideae), an Eastern Asian and north American genus. BMC Evol. Biol. 18 (1), 74. doi: 10.1186/s12862-018-1195-0
Xiang, K., Wu, S., Yu, S., Liu, Y., Jabbour, F., Erst, A., et al. (2016). The first comprehensive phylogeny of coptis (Ranunculaceae) and its implications for character evolution and classification. PloS One 11 (4), e0153127. doi: 10.1371/journal.pone.0153127
Xu, X., Liu, L., Cao, X., Long, X., Peng, S., Zhang, G. (2022). Network pharmacology and molecular docking analysis on molecular targets and mechanism prediction of huanglian jiedu decoction in the treatment of COVID-19. Digital Chin. Med. 5 (1), 18–32. doi: 10.1016/j.compbiomed.2022.105389
Xu, D., Zhuo, Z., Wang, R., Ye, M., Pu, B. (2019). Modeling the distribution of zanthoxylum armatum in China with MaxEnt modeling. Glob Ecol. Conserv. 19, e00691. doi: 10.1016/j.gecco.2019.e00691
Yang, Y., Vong, C., Zeng, S., Gao, C., Chen, Z., Fu, C., et al. (2021). Tracking evidences of coptis chinensis for the treatment of inflammatory bowel disease from pharmacological, pharmacokinetic to clinical studies. J. Ethnopharmacology 268, 113573. doi: 10.1016/j.jep.2020.113573
Yang, X., Yu, X., Zhang, X., Guo, H., Xing, Z., Xu, L., et al. (2022). Development of mini-barcode based on chloroplast genome and its application in metabarcoding molecular identification of chinese medicinal material radix paeoniae rubra (Chishao). Front. Plant Sci. 13. doi: 10.3389/fpls.2022.819822
Yan, D., Jin, C., Xiao, X., Dong, X. (2008). Antimicrobial properties of berberines alkaloids in coptis chinensis franch by microcalorimetry. J. Biochem. 70 (6), 845–849. doi: 10.1016/j.jbbm.2007.07.009
Ye, W., Zhu, S., Comes, H., Yang, T., Lian, L., Wang, W., et al. (2022). Phylogenomics and diversification drivers of the Eastern Asian-Eastern north American disjunct podophylloideae. Mol. Phylogenet Evol. 169, 107427. doi: 10.1016/j.ympev.2022.107427
Yu, Y., Blair, C., He, X. (2020). RASP 4: Ancestral state reconstruction tool for multiple genes and characters. Mol. Biol. Evol. 37 (2), 604–606. doi: 10.1093/molbev/msz257
Yu, X., Tan, W., Gao, H., Miao, L., Tian, X. (2020). Development of a specific mini-barcode from plastome and its application for qualitative and quantitative identification of processed herbal products using DNA metabarcoding technique: a case study on senna. Front. Pharmacol. 11. doi: 10.3389/fphar.2020.585687
Zachos, J. C., Dickens, G. R., Zeebe, R. E. (2008). An early Cenozoic perspective on greenhouse warming and carbon-cycle dynamics. Nature 451 (7176), 279–283. doi: 10.1038/nature06588
Zeng, L., Qiang, Z., Sun, R., Kong, H., Ning, Z., Hong, M. (2014). Resolution of deep angiosperm phylogeny using conserved nuclear genes and estimates of early divergence times. Nat. Commun. 5, 4956. doi: 10.1038/ncomms5956
Zhang, D., Gao, F., Jakovlic, I., Zou, H., Zhang, J., Li, W., et al. (2020). PhyloSuite: an integrated and scalable desktop platform for streamlined molecular sequence data management and evolutionary phylogenetics studies. Mol. Ecol. Resour 20 (1), 348–355. doi: 10.1111/1755-0998.13096
Zhang, X., Landis, J., Wang, H., Zhu, Z., Wang, H. (2021). Comparative analysis of chloroplast genome structure and molecular dating in myrtales. BMC Plant Biol. 21 (1), 219. doi: 10.1186/s12870-021-02985-9
Zhang, Y., Tang, J., Ren, G., Zhao, K., Wang, X. (2021). Global potential distribution prediction of xanthium italicum based on maxent model. Sci. Rep. 11 (1), 16545. doi: 10.1038/s41598-021-96041-z
Zhang, K., Yao, L., Meng, J., Tao, J. (2018). Maxent modeling for predicting the potential geographical distribution of two peony species under climate change. Sci. Total Environ. 634, 1326–1334. doi: 10.1016/j.scitotenv.2018.04.112
Zhao, Y., Deng, X., Xiang, W., Chen, L., Ouyang, S. (2021). Predicting potential suitable habitats of Chinese fir under current and future climatic scenarios based on maxent model. Ecol. Indic 64, 101393. doi: 10.1016/j.ecoinf.2021.101393
Keywords: Coptis, plastid genetic diversity, divergence time, biogeographic history, potential suitable distribution, phylogenetic relationship
Citation: Wang Y, Sun J, Qiao P, Wang J, Wang M, Du Y, Xiong F, Luo J, Yuan Q, Dong W, Huang L and Guo L (2022) Evolutionary history of genus Coptis and its dynamic changes in the potential suitable distribution area. Front. Plant Sci. 13:1003368. doi: 10.3389/fpls.2022.1003368
Received: 22 August 2022; Accepted: 07 November 2022;
Published: 23 November 2022.
Edited by:
JianJun Jin, Columbia University, United StatesReviewed by:
Kunli Xiang, Institute of Botany (CAS), ChinaIsabel Marques, University of Lisbon, Portugal
Haidong Yan, University of Georgia, United States
Copyright © 2022 Wang, Sun, Qiao, Wang, Wang, Du, Xiong, Luo, Yuan, Dong, Huang and Guo. This is an open-access article distributed under the terms of the Creative Commons Attribution License (CC BY). The use, distribution or reproduction in other forums is permitted, provided the original author(s) and the copyright owner(s) are credited and that the original publication in this journal is cited, in accordance with accepted academic practice. No use, distribution or reproduction is permitted which does not comply with these terms.
*Correspondence: Wenpan Dong, d3Bkb25nQGJqZnUuZWR1LmNu; Luqi Huang, aHVhbmdsdXFpMDFAMTI2LmNvbQ==; Lanping Guo, Z2xwMDFAMTI2LmNvbQ==
†These authors have contributed equally to this work