- Division of Biological Science, Graduate School of Science and Technology, Nara Institute of Science and Technology, Ikoma, Japan
Idioblasts are defined by abnormal shapes, sizes, and contents that are different from neighboring cells. Myrosin cells are Brassicales-specific idioblasts and accumulate a large amount of thioglucoside glucohydrolases (TGGs, also known as myrosinases) in their vacuoles. Myrosinases convert their substrates, glucosinolates, into toxic compounds when herbivories and pests attack plants. In this review, we highlight the similarities and differences between myrosin cells and vascular cells/guard cells (GCs) because myrosin cells are distributed along vascular cells, especially the phloem parenchyma, and myrosin cells share the master transcription factor FAMA with GCs for their cell differentiation. In addition, we analyzed the overlap of cell type-specific genes between myrosin cells and GCs by using published single-cell transcriptomics (scRNA-seq) data, suggesting significant similarities in the gene expression patterns of these two specialized cells.
Physiological Roles of the Myrosinase–Glucosinolate System
Idioblasts are cells with abnormal shapes, sizes, and contents (proteins and metabolites) (Foster, 1956). Myrosin cells are Brassicales-specific idioblasts. In model plants, Arabidopsis thaliana, myrosin cells are distributed along veins in the aerial parts of the plants without hypocotyls. Myrosin cells are named after storage proteins, myrosinases, which are called “myrosin grain” and thioglucoside glucohydrolase (TGG) (Rask et al., 2000; Andreasson et al., 2001). The substrates of myrosinases are glucosinolates, which are sulfur-rich compounds in plants and accumulate at other types of specialized cells called S-cells (Koroleva et al., 2000; Shroff et al., 2008; Burow and Halkier, 2017; Nintemann et al., 2018). Under normal developmental conditions, enzymes and substrates never meet each other because they are separated into different cell types. After the cell collapses by the attack of herbivories, myrosinase cleaves the bond between sulfur and glucose in glucosinolates to produce the toxic compounds, isothiocyanates (Shirakawa and Hara-Nishimura, 2018). This defense strategy was named “mustard oil bomb” and is one of the critical factors for the habitat range of Brassicales plants (Prasad et al., 2012). In a model plant, A. thaliana, two kinds of myrosinases, TGG1 and TGG2, are stored in myrosin cells. Double knockout mutants of TGG1 and TGG2 have exhibited weak resistance against herbivories compared with the wild-type (Barth and Jander, 2006). Unlike TGG2, it is well known that TGG1 is also expressed in guard cells (GCs) (Zhao et al., 2008). However, the role of the myrosinase–glucosinolate system in GCs was unknown until recently. Salehin et al. (2019) showed that the myrosinase–glucosinolate system is required for the closure of stomata under drought conditions. These results suggested that the myrosinase–glucosinolate system has different functions in two different specialized cells.
Thioglucoside glucohydrolases are defined by the conserved glutamine that is required for binding to glucosinolates. The conserved residue is replaced by glutamic acid in the atypical myrosinases, PENETRATION2 (PEN2) and PYK10 (Matsushima et al., 2003; Bednarek et al., 2009). They prefer indole glucosinolates to aliphatic glucosinolates. For more detailed information about the function and evolution of atypical myrosinases, see Nakano et al. (2014); Pastorczyk and Bednarek (2016), and Nakano et al. (2017).
Anti-Myrosinase–Glucosinolate Strategies and Reuse of Glucosinolates
During evolution, herbivories evolved the resistance and secondary use of the myrosinase–glucosinolates system. Diamondback moth is a crucifer specialist insect and produces glucosinolate sulfatase (GSS) to detoxify glucosinolates (Ratzka et al., 2002). GSS hydrolases glucosinolates to produce desulfo-glucosinolates, which myrosinases cannot cleave. The evolution of GSS during the battle between insects and plants is an open question.
Other insects accumulate plant toxins, glucosinolates, for defense against predators. The specialist herbivorous insect Phyllotreta striolata (flea beetle) ingests glucosinolates and has myrosinases that may cleave glucosinolates from plants (Beran et al., 2014). Recently, it was reported that the horseradish flea beetle Phyllotreta armoraciae uses a sugar transporter as a glucosinolate transporter to transfer glucosinolates from the excretory system to the hemolymph (Yang et al., 2021). It is an interesting question how and why flea beetles start to use glucosinolates for their defense strategies.
Myrosin Cells Versus Vascular Cells
Myrosin cells are distributed along veins, especially the phloem (Shirakawa and Hara-Nishimura, 2018; Shimada et al., 2018). Myrosin cells contact directly with phloem parenchyma. During development, myrosin cells never encounter veins, resulting in two networks, the network of veins and myrosin cells, which are wired coordinately (parallel organization and alignment). This observation provoked the question of whether myrosin cells differentiate from vascular precursor cells (procambium cells). Two groups compared the spatiotemporal expression patterns of a myrosin cell reporter and a procambium reporter and showed that myrosin cells do not differentiate from procambium cells and, rather, directly differentiate from ground meristem cells, which are stem cell-like cells in inner tissue (Li and Sack, 2014; Shirakawa et al., 2016b). Ground meristem cells are also mother cells of mesophyll cells and procambium cells. How plants coordinate the development of myrosin cells and vascular cells remains an open question. Polar auxin transport (PAT) is required for the proper development of both vascular cells and myrosin cells, suggesting that auxin may coordinate the development of both cell types. Interestingly, mutants of SYNTAXIN OF PLANTS 22 (SYP22) exhibited a less vascular network than wild-type and, in contrast, had more myrosin cells than the wild-type (Ueda et al., 2006; Shirakawa et al., 2009, 2010, 2014a). SYP22 is required for PAT in leaf primordia through the endocytosis of the auxin efflux carrier PIN-FORMED 1 (PIN1) (Shirakawa et al., 2014c). syp22 failed to canalize auxin resulting the abnormal distribution of auxin. Taken together, we hypothesized that high levels of auxin induce the vascular differentiation and low levels of auxin induce the differentiation of myrosin cells. Different dosages of auxin may regulate the development of two specialized cells.
Why do myrosin cells distribute along leaf veins? First, S-cells are distributed along the primary veins. Plants need to develop myrosin cells close to S-cells to efficiently produce toxic compounds when herbivories eat them. This may work as a costless defense system, protecting the lifeline of plants without the loss of photosynthetic organs. Second, myrosin cells may need to communicate with vascular cells, especially phloem cells, to exchange nutrients and metabolites. Consistent with this hypothesis, SUGAR TRANSPORTER PROTEIN 8 is specifically expressed in myrosin cells (Rottmann et al., 2018). Like the wiring of the vein network and myrosin cell network, two networks, the nervous system and blood vessel network, are wired in animals and are called “neurovascular links” (Walchli et al., 2015). The wiring of networks of vascular cells and myrosin cells (named myrovascular links) may be a good model for research on the coordination of the two networks. Future studies may identify new roles of myrosin cells in the context of communication with vascular cells, which may be independent of S-cells.
Myrosin Cells Versus Guard Cells
The basic helix–loop–helix transcription factor FAMA was identified as a master transcription factor for the differentiation of myrosin cells from ground meristem cells (Li and Sack, 2014; Shirakawa et al., 2014b, 2016a). FAMA is also known as the master TF for the transition from guard mother cells (GMCs) into GCs (Ohashi-Ito and Bergmann, 2006). Sister TFs, SPEECHLESS (SPCH) and MUTE, regulate the transition from protodermal cells into meristemoids and the transition from meristemoids into GMCs, respectively (MacAlister et al., 2007; Pillitteri et al., 2007; Lau and Bergmann, 2012; Han and Torii, 2016). Recently, it was shown that MUTE directly activates FAMA in stomatal lineage cells (Han et al., 2018). However, in inner tissues, MUTE is not required for the expression of FAMA, suggesting that other TF(s) activate the expression of FAMA in inner tissues (Shirakawa et al., 2014b). The distribution of FAMA-expressing cells in inner tissues was changed by treatment with PAT inhibitors and in mutants of PAT, including syp22 (Li and Sack, 2014; Shirakawa et al., 2014c). Auxin response factors (ARFs) may activate FAMA in inner tissues.
The downstream FAMA has been well studied in the stomatal lineage (Hachez et al., 2011; Weimer et al., 2018). In the stomatal lineage, FAMA inhibits the ectopic divisions of GMCs and promotes the differentiation of GCs (Ohashi-Ito and Bergmann, 2006). One of the D-type cyclins, CYCD7, is directly repressed by FAMA to inhibit ectopic divisions of GMCs (Figure 1A; Weimer et al., 2018). Although other downstream factors were identified by transcriptome analysis (Hachez et al., 2011), it was still unclear which direct targets of FAMA differentiate from GMCs to GCs. One of the candidates is DNA-binding with one finger (DOF) TF, STOMATAL CARPENTER 1. SCAP1 is upregulated in inducible FAMA-overexpression lines (Hachez et al., 2011). SCAP1 is expressed from young GCs to mature GCs, suggesting that the expression window of SCAP1 fits the later expression window of FAMA (Figure 1A; Negi et al., 2013; Lopez-Anido et al., 2021). In addition, half of GCs in scap1 mutants exhibited skewed morphologies (Negi et al., 2013). SCAP1 is a potential direct target of FAMA in young GCs. However, the stomatal phenotypes of scap1 were much weaker than those of fama. Other direct target(s) of FAMA must exist and cooperatively promote the differentiation of GCs with SCAP1.
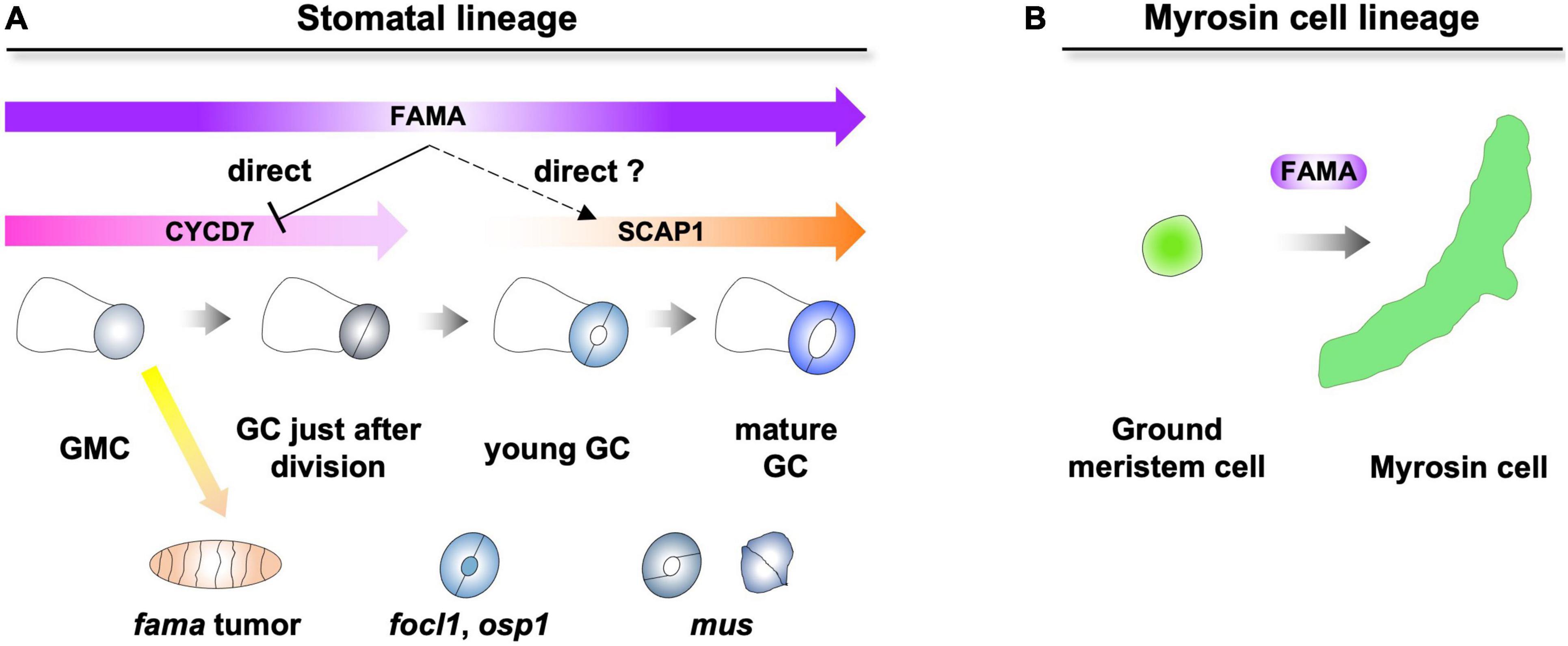
Figure 1. Dual role of FAMA in epidermis and inner tissues. (A) FAMA regulates the final division of guard mother cells (GMCs) and the differentiation of guard cells (GCs). FAMA directly represses the expression of CYCD7 and potentially regulates the expression of SCAP1. Loss of FAMA triggers the ectopic divisions of GMCs, resulting in the formation of fama tumors. In focl1 and osp1, the pores of stomata are covered by membranous cuticular material. mus exhibits skewed GC (left) and unopen GC (right). (B) FAMA regulates the differentiation of ground meristem cells into myrosin cells in inner tissues.
In addition to TFs, a leucine-rich repeat receptor-like kinase (MUSTACHES/MUS), a structural protein [FUSED OUTER CUTICULAR LEDGE1 (FOCL1)] and enzymes [POLYGALACTURONASE INVOLVED IN EXPANSION3 (PGX3) and OCCLUSION OF STOMATAL PORE 1 (OSP1)] that are involved in the formation of pores were identified over a decade (Keerthisinghe et al., 2015; Hunt et al., 2017; Rui et al., 2017; Tang et al., 2020). mus exhibited skewed GC and unopen GC, suggesting that MUS receives unknown ligands that coordinate the bilateral symmetry of GC. focl1 and osp1 showed similar phenotypes in that the pores of stomata were covered by membranous cuticular materials. FOCL1 is a secreted cell wall structural protein, and OSP1 is a GDSL lipase. It is an interesting question how FOCL1 and OSP1 interact genetically and biochemically. PGX3 is required for the formation of the proper pore size. It is still an open question whether the expression of these factors is regulated by FAMA.
Compared with stomata, key factor(s) of the differentiation of myrosin cells, which are downstream of FAMA, have not yet been identified (Figure 1B). Only one of the myrosinases, TGG1, has recently been reported to be a direct target of FAMA (Feng et al., 2021). Overall, key factor(s) that promote the differentiation of two specialized cells after FAMA remain enigmatic. In addition to downstream factors of FAMA, several interaction partners of FAMA have been identified (Mair et al., 2019). One of them, SCREAMs, is required for the differentiation of stomata and myrosin cells (Kanaoka et al., 2008; Shirakawa et al., 2014b). Other factors may have specific developmental/physiological functions in one of two specialized cells.
Commonality Between Myrosin Cells and Guard Cells
The FAMA-SCRM complex is a common master regulator of the differentiation of both myrosin cells and GCs. Therefore, it is expected that the two cell types may share gene expression patterns. Very recently, transcriptome analysis of Arabidopsis leaves was performed at single-cell resolution (scRNA-seq) (Berrío et al., 2021). Surprisingly, during the analysis, the unsupervised clustering of scRNA-seq data recognized myrosin cells and GCs as a single cluster. In addition, then, combined with the known markers (an epidermal marker was only expressed at GCs, not at myrosin cells), the authors divided them into two different clusters. Consistent with the previous experiment with reporter lines, TGG1 was expressed in both cell types, and TGG2 was exclusively expressed in myrosin cells in scRNA-seq, suggesting that scRNA-seq with manual clustering successfully separated the two cell types.
These results suggest that the gene expression patterns of the two types of specialized cells may be quite similar. Indeed, we compared cell type-specific genes of two specialized cells, and the analysis revealed that the commonly expressed genes were more than 50% of genes expressed in each cell type (myrosin cells 54%; GCs 64%) (Figure 2). This result indicates that unknown common function(s) may exist in two specialized cells. In addition, some myrosin cells expressed vascular markers, suggesting that myrosin cells can be segmentized into more small groups and that some of them may have features of vascular cells (Berrío et al., 2021). Taken together, scRNA-seq is a powerful tool for research on cell fate determination and differentiation.
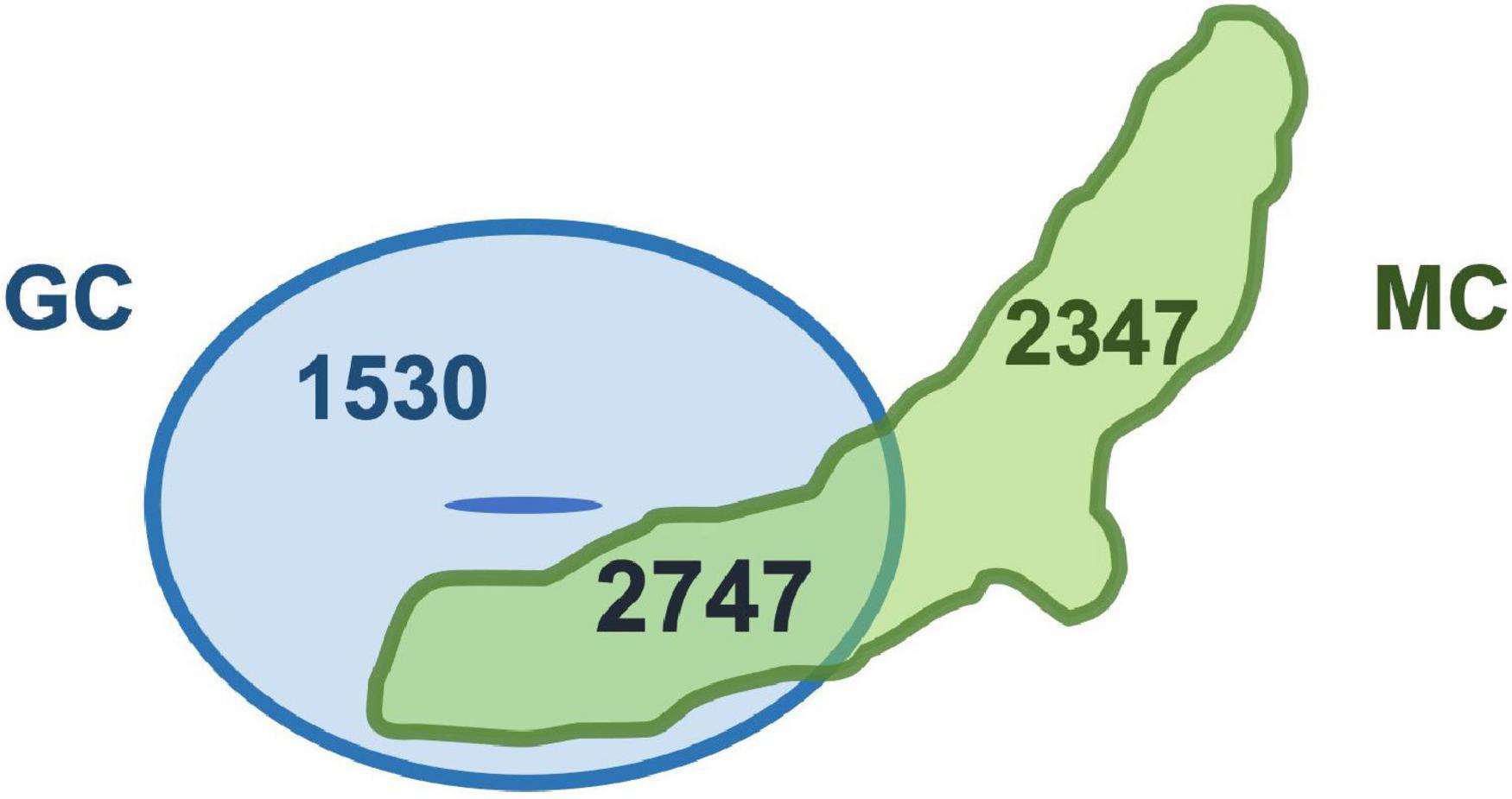
Figure 2. Two specialized cells share thousands of genes. Venn diagram of genes specifically expressed in guard cells (GC) and myrosin cells (MC). Original data were reported in Berrío et al. (2021).
Perspectives
In this review, we discuss the functional and developmental link between myrosin cells and vascular cells and GCs. The link between myrosin cells and vascular cells is hypothesized from anatomical research, and the unexpected link between myrosin cells and GCs is hypothesized from the discovery of master transcription factors. These hypotheses were partly supported by recent scRNA-seq data (Figure 2). These hypotheses may be connected with findings of the new physiological role of myrosin cells.
The downstream factors of FAMA must provide important information to answer this question. During this decade, some direct targets of FAMA were identified. However, the whole network of FAMA downstream is still largely unknown. By using inducible overexpression lines, RNA-seq analysis with sampling at multiple time points may be useful because FAMA is continuously expressed during the development of GCs (Lopez-Anido et al., 2021), and by using such methods, downstream targets of SPCH and MUTE have been identified (Lau et al., 2014; Han et al., 2018). In addition to classical transcriptome analysis, scRNA-seq is a useful tool not only for the identification of cell type-specific genes but also for the reconstruction of cell lineages (Lopez-Anido et al., 2021). Combined with stage-specific fluorescent reporters, fluorescence-activated cell sorting (FACS), and scRNA-seq, it may be possible to reveal the gradual change in gene expression patterns of myrosin cells from the beginning to the maturation of cell differentiation (lineage tracing).
Moreover, in the plant research field, small molecules with various biological functions have been recently identified (Nemhauser and Torii, 2016; Ziadi et al., 2017; Shirakawa et al., 2021). For example, the chemical compound bubblin increased the number of GCs (Sakai et al., 2017). By transient treatment and dosage control, small molecules can overcome the genetic redundancy and lethality of gene functions. It could be possible to identify the molecules that manipulate the number and distribution of myrosin cells and convert the identity of myrosin cells into GCs/vascular cells and vice versa.
The physiological function and developmental program of idioblast myrosin cells are largely unknown. Future works will shed light on the comprehensive molecular network of the function and development of myrosin cells. This information may be connected with the research field of applied plant science.
Author Contributions
MS and MT analyzed the scRNA-seq data and described the figures. MS and TI wrote the manuscript. All authors read and approved the final version of the manuscript.
Funding
This work was supported by a grant from the JSPS KAKENHI Grant-in-Aid for Scientific Research C (19K06722), the JSPS KAKENHI Grant-in-Aid for Scientific Research on Innovative Areas (20H05416), the Takeda Science Foundation, the Kato Memorial Bioscience Foundation, and the Ohsumi Frontier Science Foundation to MS, as well as a grant from the JSPS KAKENHI Grant-in-Aid for Scientific Research A (20H00470), the JSPS KAKENHI Grant-in-Aid for Scientific Research on Innovative Areas (20H04888), and the JSPS KAKENHI Grant-in-Aid for Scientific Research on Innovative Areas (19H04865) to TI.
Conflict of Interest
The authors declare that the research was conducted in the absence of any commercial or financial relationships that could be construed as a potential conflict of interest.
Publisher’s Note
All claims expressed in this article are solely those of the authors and do not necessarily represent those of their affiliated organizations, or those of the publisher, the editors and the reviewers. Any product that may be evaluated in this article, or claim that may be made by its manufacturer, is not guaranteed or endorsed by the publisher.
Acknowledgments
We thank lab members for helpful discussions.
References
Andreasson, E., Jorgensen, L. B., Hoglund, A. S., and Meijer, J. (2001). Different myrosinase and idioblast distribution in Arabidopsis and Brassica napus. Plant Physiol. 127, 1750–1763. doi: 10.1104/pp.010334
Barth, C., and Jander, G. (2006). Arabidopsis myrosinases TGG1 and TGG2 have redundant function in glucosinolate breakdown and insect defense. Plant J. 46, 549–562. doi: 10.1111/j.1365-313X.2006.02716.x
Bednarek, P., Pislewska-Bednarek, M., Svatos, A., Schneider, B., Doubsky, J., Mansurova, M., et al. (2009). A glucosinolate metabolism pathway in living plant cells mediates broad-spectrum antifungal defense. Science 323, 101–106. doi: 10.1126/science.1163732
Beran, F., Pauchet, Y., Kunert, G., Reichelt, M., Wielsch, N., Vogel, H., et al. (2014). Phyllotreta striolata flea beetles use host plant defense compounds to create their own glucosinolate-myrosinase system. Proc. Natl. Acad. Sci. U. S. A. 111, 7349–7354. doi: 10.1073/pnas.1321781111
Berrío, R. T., Verstaen, K., Vandamme, N., Pevernagie, J., Achon, I., Duyse, J. V., et al. (2021). Single-cell transcriptomics sheds light on the identity and metabolism of developing leaf cells. Plant Physiol. [Epub Online ahead of print]. doi: 10.1093/plphys/kiab489
Burow, M., and Halkier, B. A. (2017). How does a plant orchestrate defense in time and space? Using glucosinolates in Arabidopsis as case study. Curr. Opin. Plant Biol. 38, 142–147. doi: 10.1016/j.pbi.2017.04.009
Feng, Q., Li, L., Liu, Y., Shao, X., and Li, X. (2021). Jasmonate regulates the FAMA/mediator complex subunit 8-THIOGLUCOSIDE GLUCOHYDROLASE 1 cascade and myrosinase activity. Plant Physiol. 187, 963–980. doi: 10.1093/plphys/kiab283
Foster, A. S. (1956). Plant idioblasts: remarkable examples of cell specializaiton. Protoplasma 46, 184–193.
Hachez, C., Ohashi-Ito, K., Dong, J., and Bergmann, D. C. (2011). Differentiation of Arabidopsis guard cells: analysis of the networks incorporating the basic helix-loop-helix transcription factor, FAMA. Plant Physiol. 155, 1458–1472. doi: 10.1104/pp.110.167718
Han, S. K., Qi, X., Sugihara, K., Dang, J. H., Endo, T. A., Miller, K. L., et al. (2018). MUTE directly orchestrates cell-stare switch and the single symmetric division to create stomata. Dev. Cell. 45, 303–315. doi: 10.1016/j.devcel.2018.04.010
Han, S. K., and Torii, K. U. (2016). Lineage-specific stem cells, signals and asymmetries during stomatal development. Development 143, 1259–1270. doi: 10.1242/dev.127712
Hunt, L., Amsbury, S., Baillie, A., Movahedi, M., Mitchell, A., Afsharinafar, M., et al. (2017). Formation of the stomatal outer cuticular ledge requires a guard cell wall proline-rich protein. Plant Physiol. 174, 689–699. doi: 10.1104/pp.16.01715
Kanaoka, M. M., Pillitteri, L. J., Fujii, H., Yoshida, Y., Bogenschutz, N. L., Takabayashi, J., et al. (2008). SCREAM/ICE1 and SCREAM2 specify three cell-state transitional steps leading to Arabidopsis stomatal differentiation. Plant Cell 20, 1775–1785. doi: 10.1105/tpc.108.060848
Keerthisinghe, S., Nadeau, J. A., Lucas, J. R., Nakagawa, T., and Sack, F. D. (2015). The Arabidopsis leucine-rich repeat receptor-like kinase MUSTACHES enforces stomatal bilateral symmetry in Arabidopsis. Plant J. 81, 684–694. doi: 10.1111/tpj.12757
Koroleva, O. A., Davies, A., Deeken, R., Thorpe, M. R., Tomos, A. D., Hedrich, R., et al. (2000). Identification of a new glucosinolate-rich cell type in Arabidopsis flower stalk. Plant Physiol. 124, 599–608. doi: 10.1104/pp.124.2.599
Lau, O. S., and Bergmann, D. C. (2012). Stomatal development: a plant’s perspective on cell polarity, cell fate transitions and intercellular communication. Development 139, 3683–3692. doi: 10.1242/dev.080523
Lau, O. S., Davies, K. A., Chang, J., Adrian, J., Rowe, M. H., Ballenger, C. E., et al. (2014). Direct roles of SPEECHLESS in the specification of stomatal self-renewing cells. Science 345, 1605–1609. doi: 10.1126/science.1256888
Li, M., and Sack, F. D. (2014). Myrosin idioblast cell fate and development are regulated by the Arabidopsis transcription factor FAMA, the Auxin pathway, and vesicular trafficking. Plant Cell 26, 4053–4066. doi: 10.1105/tpc.114.129726
Lopez-Anido, C. B., Vaten, A., Smoot, N. K., Sharma, N., Guo, V., Gong, Y., et al. (2021). Single-cell resolution of lineage trajectories in the Arabidopsis stomatal lineage and developing leaf. Dev. Cell 56, 1043–1055. doi: 10.1016/j.devcel.2021.03.014
MacAlister, C. A., Ohashi-Ito, K., and Bergmann, D. C. (2007). Transcription factor control of asymmetric cell divisions that establish the stomatal lineage. Nature 445, 537–540. doi: 10.1038/nature05491
Mair, A., Xu, S. L., Branon, T. C., Ting, A. Y., and Bergmann, D. C. (2019). Proximity labeling of protein complexes and cell-type-specific organellar proteomes in Arabidopsis enabled by TurboID. Elife 8:e47864.
Matsushima, R., Kondo, M., Nishimura, M., and Hara-Nishimura, I. (2003). A novel ER-derived compartment, the ER body, selectively accumulates a b-glucosidase with an ER-retention signal in Arabidopsis. Plant Cell Physiol. 33, 493–502. doi: 10.1046/j.1365-313x.2003.01636.x
Nakano, R. T., Yamada, K., Bednarek, P., Nishimura, M., and Hara-Nishimura, I. (2014). ER bodies in plants of the Brassicales order: biogenesis and association with innate immunity. Front. Plant Sci. 5:73. doi: 10.3389/fpls.2014.00073
Nakano, R. T., Yamada, K., Bednarek, P., Nishimura, M., and Hara-Nishimura, I. (2017). PYK10 myrosinase reveals a functional coordination between endoplasmic reticulum bodies and glucosinolates in Arabidopsis thaliana. Plant J. 89, 204–220. doi: 10.1111/tpj.13377
Negi, J., Moriwaki, K., Konishi, M., Yokoyama, R., Nakano, T., Kusumi, K., et al. (2013). A Dof transcription factor, SCAP1, is essential for the development of functional stomata in Arabidopsis. Curr. Biol. 23, 479–484. doi: 10.1016/j.cub.2013.02.001
Nemhauser, J. L., and Torii, K. U. (2016). Plant synthetic biology for molecular engineering of signalling and development. Nat. Plants 2:16010. doi: 10.1038/nplants.2016.10
Nintemann, S. J., Hunziker, P., Andersen, T. G., Schulz, A., Burow, M., and Halkier, B. A. (2018). Localization of the glucosinolate biosynthetic enzymes reveals distinct spatial patterns for the biosynthesis of indole and aliphatic glucosinolates. Physiol. Plant 163, 138–154. doi: 10.1111/ppl.12672
Ohashi-Ito, K., and Bergmann, D. C. (2006). Arabidopsis FAMA controls the final proliferation/differentiation switch during stomatal development. Plant Cell 18, 2493–2505. doi: 10.1105/tpc.106.046136
Pastorczyk, M., and Bednarek, P. (2016). The function of glucosinolates and related metabolites in plant innate immunity. Adv. Bot. Res. 80, 171–198.
Pillitteri, L. J., Sloan, D. B., Bogenschutz, N. L., and Torii, K. U. (2007). Termination of asymmetric cell division and differentiation of stomata. Nature 445, 501–505. doi: 10.1038/nature05467
Prasad, K. V., Song, B. H., Olson-Manning, C., Anderson, J. T., Lee, C. R., Schranz, M. E., et al. (2012). A gain-of-function polymorphism controlling complex traits and fitness in nature. Science 337, 1081–1084. doi: 10.1126/science.1221636
Rask, L., Andreasson, E., Ekbom, B., Eriksson, S., Pontoppidan, B., and Meijer, J. (2000). Myrosinase: gene family evolution and herbivore defense in Brassicaceae. Plant Mol. Biol. 42, 93–113. doi: 10.1007/978-94-011-4221-2_5
Ratzka, A., Vogel, H., Kliebenstein, D. J., Mitchell-Olds, T., and Kroymann, J. (2002). Disarming the mustard oil bomb. Proc. Natl. Acad. Sci. U. S. A. 99, 11223–11228. doi: 10.1073/pnas.172112899
Rottmann, T., Klebl, F., Schneider, S., Kischka, D., Ruscher, D., Sauer, N., et al. (2018). Sugar Transporter STP7 Specificity for L-Arabinose and D-Xylose Contrasts with the Typical Hexose Transporters STP8 and STP12. Plant Physiol. 176, 2330–2350. doi: 10.1104/pp.17.01493
Rui, Y., Xian, C., Yi, H., Kandemir, B., Wang, J. Z., Puri, V. M., et al. (2017). POLYGALACTURONASE INVOLVED IN EXPANSION3 Functions in Seedling Development, Rosette Growth, and Stomatal Dynamics in Arabidopsis thaliana. Plant Cell 29, 2413–2432. doi: 10.1105/tpc.17.00568
Sakai, Y., Sugano, S. S., Kawase, T., Shirakawa, M., Imai, Y., Kawamoto, Y., et al. (2017). The chemical compound bubblin induces stomatal mispatterning in Arabidopsis by disrupting the intrinsic polarity of stomatal lineage cells. Development 144, 499–506. doi: 10.1242/dev.145458
Salehin, M., Li, B., Tang, M., Katz, E., Song, L., Ecker, J. R., et al. (2019). Auxin-sensitive Aux/IAA proteins mediate drought tolerance in Arabidopsis by regulating glucosinolate levels. Nat. Commun. 10:4021. doi: 10.1038/s41467-019-12002-1
Shimada, T., Takagi, J., Ichino, T., Shirakawa, M., and Hara-Nishimura, I. (2018). Plant Vacuoles. Annu. Rev. Plant Biol. 69, 123–145.
Shirakawa, M., and Hara-Nishimura, I. (2018). Specialized vacuoles of myrosin cells: chemical defense strategy in brassicales plants. Plant Cell Physiol. 59, 1309–1316. doi: 10.1093/pcp/pcy082
Shirakawa, M., Morisaki, Y., Gan, E. S., Sato, A., and Ito, T. (2021). Identification of a devernalization inducer by chemical screening approaches in Arabidopsis thaliana. Front. Plant Sci. 12:634068. doi: 10.3389/fpls.2021.634068
Shirakawa, M., Ueda, H., Koumoto, Y., Fuji, K., Nishiyama, C., Kohchi, T., et al. (2014a). CONTINUOUS VASCULAR RING (COV1) is a trans-golgi network-localized membrane protein required for golgi morphology and vacuolar protein sorting. Plant Cell Physiol. 55, 764–772. doi: 10.1093/pcp/pct195
Shirakawa, M., Ueda, H., Shimada, T., Kohchi, T., and Hara-Nishimura, I. (2014c). Myrosin Cell Development Is Regulated by Endocytosis Machinery and PIN1 Polarity in Leaf Primordia of Arabidopsis thaliana. Plant Cell 26, 4448–4461. doi: 10.1105/tpc.114.131441
Shirakawa, M., Ueda, H., Nagano, A. J., Shimada, T., Kohchi, T., and Hara-Nishimura, I. (2014b). FAMA is an essential component for the differentiation of two distinct cell types, myrosin cells and guard cells, in Arabidopsis. Plant Cell 26, 4039–4052. doi: 10.1105/tpc.114.129874
Shirakawa, M., Ueda, H., Shimada, T., and Hara-Nishimura, I. (2016a). FAMA: a molecular link between stomata and myrosin cells. Trends Plant Sci. 21, 861–871. doi: 10.1016/j.tplants.2016.07.003
Shirakawa, M., Ueda, H., Shimada, T., and Hara-Nishimura, I. (2016b). Myrosin cells are differentiated directly from ground meristem cells and are developmentally independent of the vasculature in Arabidopsis leaves. Plant Signal. Behav. 11:e1150403. doi: 10.1080/15592324.2016.1150403
Shirakawa, M., Ueda, H., Shimada, T., Koumoto, Y., Shimada, T. L., Kondo, M., et al. (2010). Arabidopsis Qa-SNARE SYP2 proteins localized to different subcellular regions function redundantly in vacuolar protein sorting and plant development. Plant J. 64, 924–935. doi: 10.1111/j.1365-313X.2010.04394.x
Shirakawa, M., Ueda, H., Shimada, T., Nishiyama, C., and Hara-Nishimura, I. (2009). Vacuolar SNAREs function in the formation of the leaf vascular network by regulating auxin distribution. Plant Cell Physiol. 50, 1319–1328. doi: 10.1093/pcp/pcp076
Shroff, R., Vergara, F., Muck, A., Svatos, A., and Gershenzon, J. (2008). Nonuniform distribution of glucosinolates in Arabidopsis thaliana leaves has important consequences for plant defense. Proc. Natl. Acad. Sci. U. S. A. 105, 6196–6201. doi: 10.1073/pnas.0711730105
Tang, J., Yang, X., Xiao, C., Li, J., Chem, Y., Li, R., et al. (2020). GDSL lipase occluded stomatal pore 1 is required for wax biosynthesis and stomatal cuticular ledge formation. New Phytol. 228, 1880–1896. doi: 10.1111/nph.16741
Ueda, H., Nishiyama, C., Shimada, T., Koumoto, Y., Hayashi, Y., Kondo, M., et al. (2006). AtVAM3 is required for normal specification of idioblasts, myrosin cells. Plant Cell Physiol. 47, 164–175. doi: 10.1093/pcp/pci232
Walchli, T., Wacker, A., Frei, K., Regli, L., Schwab, M. E., Hoerstrup, S. P., et al. (2015). Wiring the vascular network with neural cues: a CNS perspective. Neuron 87, 271–296. doi: 10.1016/j.neuron.2015.06.038
Weimer, A. K., Matos, J. L., Sharma, N., Patell, F., Murray, J. A. H., Dewitte, W., et al. (2018). Lineage- and stage-specific expressed CYCD7;1 coordinates the single symmetric division that creates stomatal guard cells. Development 145:dev160671. doi: 10.1242/dev.160671
Yang, Z. L., Nour-Eldin, H. H., Hanniger, S., Reichelt, M., Crocoll, C., Seitz, F., et al. (2021). Sugar transporters enable a leaf beetle to accumulate plant defense compounds. Nat. Commun. 12:658. doi: 10.1038/s41467-021-22982-8
Zhao, Z., Zhang, W., Stanley, B. A., and Assmann, S. M. (2008). Functional proteomics of Arabidopsis thaliana guard cells uncovers new stomatal signaling pathways. Plant Cell 20, 3210–3226. doi: 10.1105/tpc.108.063263
Keywords: cell differentiation, FAMA, glucosinolate, guard cell, myrosin cell, myrosinase, vascular cell
Citation: Shirakawa M, Tanida M and Ito T (2022) The Cell Differentiation of Idioblast Myrosin Cells: Similarities With Vascular and Guard Cells. Front. Plant Sci. 12:829541. doi: 10.3389/fpls.2021.829541
Received: 05 December 2021; Accepted: 17 December 2021;
Published: 10 January 2022.
Edited by:
Takumi Higaki, Kumamoto University, JapanReviewed by:
Yuki Kondo, Kobe University, JapanCopyright © 2022 Shirakawa, Tanida and Ito. This is an open-access article distributed under the terms of the Creative Commons Attribution License (CC BY). The use, distribution or reproduction in other forums is permitted, provided the original author(s) and the copyright owner(s) are credited and that the original publication in this journal is cited, in accordance with accepted academic practice. No use, distribution or reproduction is permitted which does not comply with these terms.
*Correspondence: Makoto Shirakawa, c2hpcmFrYXdhQGJzLm5haXN0Lmpw; Toshiro Ito, aXRvdEBicy5uYWlzdC5qcA==