- 1The Key Laboratory of Plant Development and Environmental Adaptation Biology, Ministry of Education, College of Life Sciences, Shandong University, Qingdao, China
- 2Key Lab of Plant Biotechnology in Universities of Shandong Province, College of Life Science, Qingdao Agricultural University, Qingdao, China
The soluble form of aluminum (Al) is a major constraint to crop production in acidic soils. The Al exclusion correlated with the Al-induced organic acid is considered as an important mechanism of Al resistance. The regulation of organic acid exudation in response to Al stress mediated by the root organic acid transporters has been extensively studied. However, how plants respond to Al stress through the regulation of organic acid homeostasis is not well understood. In this study, we identified the functionally unknown Transition zone1 (TZ1) as an Al-inducible gene in the root transition zone, the most sensitive region to Al stress, in Arabidopsis. tz1 mutants showed enhanced Al resistance and displayed greatly reduced root growth inhibition. Furthermore, TZ1 was found to interact with the aconitases (ACOs) which can catalyze the conversion from citrate, one of the most important organic acids, into isocitrate. Consistently, in tz1 mutants, the citric acid content was highly increased. Collectively, this study provides evidence to show that TZ1 negatively regulates root growth response to Al stress through interacting with ACOs and regulating citric acid homeostasis.
Introduction
Aluminum (Al) is a prevalent kind of metal elements in the soil and most of the aluminum is in silicate or other solid forms, which are non-toxic to plants. However, in acidic soils (pH < 5.0), the trivalent aluminum ion (Al3+) are released from clay minerals and are quite toxic to crop plants (Kochian et al., 2004; Chauhan et al., 2021). Al toxicity is a major limiting factor that reduces crop yields worldwide and constrains the food security, especially in the developing countries in tropical and subtropical regions abundant with acidic soils (Kochian et al., 2015). Al toxicity can target the root apex and restrain the growth of the plant root and subsequently affect the uptake of water and nutrients, which finally results in a significant reduction in crop yields (Kochian et al., 2004; Delhaize et al., 2007; Motoda et al., 2007; Xu et al., 2017).
Based on the previous studies, plants have developed two main mechanisms to deal with the Al stress: (1) Al exclusion mechanism, in which Al is prevented from entering the plant root apex and (2) Al tolerance mechanism, which involves the detoxification and sequestration of the internal Al once it enters the roots. The most widely documented Al exclusion mechanism is Al-induced release of organic compounds, specially the diverse organic acid ions, such as malate, citrate, and oxalate, to chelate toxic Al3+ in rhizosphere by forming non-toxic compounds and thus prevent Al3+ from entering the root cell (Kochian et al., 2015). Until now, numerous studies in different monocot and dicot plant species demonstrated that the members of aluminum-activated malate transporter (ALMT) and multidrug and toxin extrusion (MATE) families facilitate the Al-activated efflux of malate and citrate, respectively (Sasaki et al., 2004; Hoekenga et al., 2006; Ligaba et al., 2006; Furukawa et al., 2007; Magalhaes et al., 2007; Liu et al., 2009, 2018; Maron et al., 2010; Yang et al., 2011; Tovkach et al., 2013; Zhou et al., 2017). Based on the studies in Arabidopsis, ALMT1-mediated malate exudation from roots under Al stress provides a major contribution to Al detoxification (Hoekenga et al., 2006). Citrate exudation facilitated by MATE plays a minor but an important role to detoxify Al (Liu et al., 2009). Additionally, previous studies have shown that the Cys2-His2-type zinc finger transcription factor STOP1 could stimulate ALMT1 and MATE1 expression under Al stress (Iuchi et al., 2007; Liu et al., 2009; Wu et al., 2019; Zhang et al., 2019; Huang, 2021; Xu et al., 2021).
The tricarboxylic acid (TCA) cycle which is called citric acid cycle or the Krebs cycle in the mitochondria provides a large number of organic acids, namely malic acid, citric acid, and oxalic acid (Millar et al., 2011). The citric acid content is strictly dependent on its biosynthesis and catabolism in the Krebs cycle. Overexpressing the enzymes involved in citric or malic acid synthesis, such as citrate synthase (Barone et al., 2008; Deng et al., 2009; Han et al., 2009), malate dehydrogenase (Tesfaye et al., 2001; Wang et al., 2010), and pyruvate phosphated kinase (Trejo-Téllez et al., 2009) could all enhance the citric or malic acid synthesis and improve Al tolerance of the plant. Aconitase (ACOs) and isocitrate dehydrogenase (IDH) of the TCA cycle involved in the citrate catabolism are responsible for the adjacent catalytic reactions to convert citrate into isocitrate and then into α-ketoglutaric acid, respectively, leading to the reduction of the citric acid content. The aco1 and idh12 mutants showed higher accumulation of citric acid and enhanced resistance to Al stress (Anoop et al., 2003).
The root tip is the major target site of Al toxicity (Ryan and Kochian, 1993; Baluska et al., 2010; Baluska and Mancuso, 2013; Alarcon and Salguero, 2017). The transition zone (TZ) in the root tip is the boundary located between the apical meristem and the basal elongation region where the cells are in a transitional stage of cyto-architectural rearrangement, preparing to perform rapid cell elongation (Baluska et al., 2010). In addition to its role in determining the cell fate and root growth, TZ perceives endogenous hormonal signal and exogenous environmental stimuli and translates them into differential growth responses (Baluska et al., 2010; Kong et al., 2018). Studies from maize (Zea mays), common bean, Arabidopsis and sorghum indicated that TZ is the most sensitive part of the root to Al stress (Sivaguru et al., 1999; Illés et al., 2006; Rangel et al., 2007; Sivaguru et al., 2013). For example, in maize, Al treatment to the root TZ but not the elongation zone (EZ) inhibits the root growth as the effect of the treatment to the entire root apex (Kollmeier et al., 2000). Al induces ethylene production in the root TZ and subsequently alters auxin distribution in roots by disrupting AUX1- and PIN2-mediated auxin polar transport and consequently results in the root growth inhibition (Sun et al., 2010). Furthermore, ethylene signaling can enhance the auxin responses in the TZ by locally upregulating the Trp aminotransferase (TAA1) expression and results in the auxin-regulated root growth inhibition through a number of auxin response factors (ARFs) under Al stress (Yang Z. B. et al., 2014). In addition, auxin synthesis genes YUCCAs (YUCs) can be transcriptionally regulated by ethylene signaling in the root TZ in response to Al stress. EIN3 and PIF4 transcriptionally regulate Al-induced YUC expression and thus involve in the Al-induced auxin accumulation in root TZ and inhibit the root growth (Liu et al., 2016). Al stress triggers a cytokinin response in the root TZ, which is dependent on the ethylene signaling and inhibit the root growth (Yang Z. B. et al., 2017). Additionally, cytokinin signaling regulates the root elongation synergistically with auxin signaling in response to Al stress, which is different from their antagonistic roles at the root TZ to control the root growth without Al (Dello Ioio et al., 2008; Di Mambro et al., 2017).
To further clarify the molecular mechanism involved in plant Al resistance, we transcriptomically analyzed the gene expression profile in root tips under Al stress and identified one unknown function gene Transition zone1 (TZ1), which was highly upregulated in the root TZ after Al treatment. tz1 mutants displayed increased citrate acid content and enhanced Al resistance. Further analyses showed that TZ1 could interact with ACOs and enhance their activity to catalyze the conversion of citric acid to isocitric acid and thus regulate the Al resistance of the plant.
Materials and Methods
Plant Materials and Growth Conditions
The Arabidopsis Columbia (Col-0) and T-DNA insertion lines, such as aco1 (GK_138A08), aco2 (SALK_090220), and aco3 (SALK_014661) were obtained from Arabidopsis Biological Resource Center (ABRC). For constructing the pYAO-based CRISPR/Cas9 (Yan et al., 2015) mutants tz1-1 and tz1-2, two targets 5′-ATGGGAAACTGTTTGATGGG-3′ and 5′-GGGAGAAGCGGCTGCAAAGG-3′ were designed on the website http://crispr.mit.edu/. Then, pYAO-based CRISPR/Cas9 constructs were transformed into Col-0 plants. The TZ1 full-length genomic sequence was constructed into pKGWFs7.1 driven by TZ1 native promoter and transformed into Col-0 to generate the TZ1p:TZ1-GFP-GUS transgenic plants. Seedlings were grown on 1/2 Murashige and Skoog (MS) medium or hydroponically 2% modified molecular genetics research laboratory (MGRL) solution (pH 5.0) under a 16-h photoperiod at 22°C.
Treatments and Experimental Conditions
In all the root growth analyses, the seeds were sown onto polypropylene mesh floating on 2% modified MGRL solution containing 0 or 6 μM AlCl3 (pH 5.0) for 7 days. For La3+ treatment, the seeds were sown onto polypropylene mesh floating on 2% modified MGRL solution containing 0 or 1 μM LaCl3 (pH 5.0) for 5 days. The primary root length was measured using image J software. Citric acid and Al content experiments were carried out with Al (AlCl3, 15 μM, pH 5.0) treatment for 24 h.
β-Glucuronidase Staining
The 7-day-old plants growing on 1/2 MS were used for histochemical β-glucuronidase (GUS) staining experiments and the GUS staining was conducted at 37°C overnight. The staining solution contains: 0.1 M potassium phosphate buffer (pH 7.0), 2 mM potassium ferri and ferrocyanide, 0.1% Triton X-100, and 2 mM X-glucuronide. The stained plants were observed with a differential interference contrast microscope (OLYMPUS BX53, Japan).
Citrate Content Analysis
After Al treatment, a proper amount of plant samples was weighed, added with 0.25 mol/L HCl at a ratio of 1:6 (W/V), and grounded in a centrifuge tube. The samples were bathed in water at 80°C for 20 min, with continuously shaking for several times. The supernatant was centrifuged at 12,000 g at 4°C for 20 min. The detection was conducted with the wavelength at 210 nm. The column temperature was 35°C with C18 column as the stationary phase. The mobile phase was 0.2% ammonium dihydrogen phosphate at pH 2.7, and the flow rate was 0.5.
Aluminum Content Analysis
After treatment, the roots were washed three times with double distilled water. Then, the roots were digested with 65% ultrapure HNO3, and the concentration of Al was determined by graphite furnace atomic absorption spectrometer (GF-AAS, SHIMADZU, Japan).
Hematoxylin Staining
The 7-day-old seedlings were exposed to 0 or 25 μM AlCl3 for 3 h and then, the roots were stained with hematoxylin according to the protocol described by Polle et al. (1978). The roots were rinsed in 5 ml distilled water for 20 min and the water was replaced with 5 ml 0.2% (w/v) hematoxylin (Merck) and 0.02% (w/v) potassium iodide solution for 15 min. Finally, the roots were re-rinsed in water. Root tips were then photographed using a digital camera (Olympus E-620).
Confocal Microscopy Analysis
Confocal micrographs were captured using an LSM-700 device (Zeiss, Germany). To visualize the Al stress-induced expression of TZ1p:TZ1-GFP-GUS transgene, the 7-day-old seedlings were treated with or without Al (AlCl3, 25 μM, pH 5.0) for 3 h and the roots were imaged in water supplemented with propidium iodide (PI, 10 mg/L). PI and green fluorescent protein (GFP) were detected at excitation wavelengths of 488 and 561 nm, respectively.
Subcellular Localization Assay
The 35S::TZ1-YFP construct was generated by introducing the coding sequence (CDS) of TZ1 into the binary vector pX-YFP (p35S::X-YFP), and then the construct was transformed into protoplasts of Arabidopsis mesophyll cells. The 35S::TZ1-YFP together with the cytosolic marker ADH-RFP or plasma membrane maker ZmCRN-mCherry was transiently expressed in Nicotiana benthamiana leaf mesophyll cells by the infiltration of the Agrobacterium tumefaciens strain GV3101 carrying the corresponding plasmid. The transfected tobacco grows in the greenhouse for at least 48 h at 28°C before GFP and RFP scanning. The fluorescent signals were visualized with the LSM-700 laser scanning confocal microscope (Zeiss). The ZmCRN-mCherry and ADH-RFP signals were detected with 561 nm laser excitation and 580–675 nm emission, and the TZ1-GFP signal was detected with 561 nm laser excitation and 500–530 nm emission.
Yeast Two-Hybrid Assay
Yeast two-hybrid (Y2H) assay was performed according to the match maker GAL4 Two-Hybrid System 3 manual of manufacturer (Clontech) (Liu et al., 2021). The CDS of TZ1 was cloned into pENTRY and then to the plasmid pGBKT7 by LR reaction (Takara, CA, United States). The prey TZ1-BD was used to screen a pre-transformed Arabidopsis root cDNA library. After culturing on synthetic medium plates (SD medium) lacking Trp, Leu, and His (SD-Trp-Leu-His) for 2 days, transformants were transferred onto SD-Trp-Leu-His-Ade. The aconitase1 (ACO1) (C-terminal) fragment was identified. Then, C-terminals of ACO genes were fused into pGADT7 and the interaction with TZ1 was reconfirmed by Y2H.
Luciferase Complementary Imaging Assay
The TZ1 and ACO (C-terminal) were fused into pCAMBIA1300-nLUC and pCAMBIA1300-cLUC, respectively. Four different combinations of A. tumefaciens were infiltrated into the same leaves of N. benthamiana and cultured for 48 h (Yu et al., 2019). The leaves were treated with 0.2 mM luciferin before being detected with CCD imaging apparatus.
Pull-Down and Western Blotting
The purified glutathione S-transferase (GST)-fused protein (ACO-GST) and maltose-binding protein (MBP)-fused protein (TZ1-MBP) were incubated with Glutathione Sepharose (GE Healthcare, catalog number 17-5132-01, IL, United States) or Amylose Resin (Biolabs, catalog number E8021S, MA, United States) overnight at 4°C. After being washed three times with buffer containing 25 mM HEPES pH 7.5, 150 mM NaCl, 1 mM DTT, the beads were boiled in sodium dodecyl sulfate–polyacrylamide gel electrophoresis (SDS-PAGE) gel loading buffer at 99°C for 10 min and subsequently western blotting was performed using anti-GST or anti-MBP antibody. Primers used for pull-down assay are listed in Supplementary Data 2. For western blotting, proteins from cell lysates were denatured and subjected to SDS-PAGE (Bio-Rad, CA, United States) and transferred to poly (vinylidene fluoride) (PVDF) membranes (Millipore, catalog number IPVH00010, MA, United States). The membranes were blocked in 1 × TBST with 5% milk for 2 h, immunoblotted with indicated antibodies at 4°C overnight, followed by incubating for 2 h with horseradish peroxidase-conjugated secondary antibody at room temperature. Blots were visualized by SuperSignal West Pico Luminol Enhancer Solution (Thermo Fisher Scientific, MA, United States).
Co-immunoprecipitation Assay
Cells were harvested and lysed in cell lysis buffer (10 mM Tris-HCl, pH 7.5, 150 mM NaCl, 0.5 mM EDTA, 0.5% NP-40, and 1 mM PMSF) on ice for 30 min with pipetting every 10 min. Cell lysate was centrifuged and the supernatant was incubated with MYC-Trap magnetic agarose beads (Chromotek, catalog number ytma-20, Germany) at 4°C for 2 h. The beads were washed three times with dilution buffer (10 mM Tris-HCl, pH 7.5, 150 mM NaCl, and 0.5 mM EDTA) and resuspended in SDS loading buffer. The resuspended beads were boiled for 10 min at 99°C and western blotting was followed using anti-MYC (Abclonal, catalog number AE010, MA, United States) or anti-GFP (TransGen Biotech, catalog number HT801-02, Beijing, China) antibody.
Statistical Analysis
The data between samples were compared by one way ANOVA with Student’s t-test. All values were presented as mean ± SD, and values of p < 0.05 were considered significant. * and** denote differences significant at p < 0.05 and p < 0.01, respectively.
Accession Number
Gene sequences described in this article are available in TAIR 10 under the accession numbers TZ1 (AT4G23870), ACO1 (AT4G35830), ACO2 (AT4G26970), and ACO3 (AT2G05710). The Gene Expression Omnibus (GEO) accession number for the RNA-seq data reported in this paper is GSE189946.
Results
Transition Zone1 Is Involved in Aluminum Resistance
To identify new factors involved in Al resistance, especially in the root TZ, we treated the 7-day-old wild type (WT) Arabidopsis seedlings with or without 25μM AlCl3 for 3 h and performed transcriptome analyses with root tissues (approximately 0.5 cm in length from root tips). In combination with the published zone-specific gene expression profile in primary root (Brady et al., 2007; Chaiwanon and Wang, 2015), we identified 13 function unknown genes which were transcriptionally upregulated after Al treatment in the root TZ (Supplementary Data 1) and one of them is TZ1. To explore the biological function of TZ1, especially in response to Al stress, we generated two independent mutant lines by CRISPR/Cas9 technology and named them as tz1-1 and tz1-2 (Supplementary Figure 1). According to the sequencing results, both tz1-1 and tz1-2 are frame shift mutations.
We then analyzed whether TZ1 is involved in Al stress response. Under normal conditions (2% MGRL solution), the two tz1 mutants showed slightly decreased root elongation, however, after 7 days of Al treatment, the tz1 mutants exhibited longer roots than the treated WT, displaying much less inhibited root growth (Figures 1A,B). All the results indicated that TZ1 negatively regulates Al resistance in Arabidopsis. To further confirm the specific function of TZ1 in Al resistance, we exposed WT and tz1 mutants to lanthanum (La3+) stress and found that there was no difference in the root growth between WT and tz1 mutants, indicating that TZ1 responds to Al stress specifically (Supplementary Figure 2). We then measured the Al content in tz1 mutants and WT, and the results showed less Al accumulated in the root of tz1 mutants (Figure 1C). Moreover, the intensity of hematoxylin staining was weaker in the root tip of tz1 mutants than that in WT, further indicating that TZ1 affects the Al accumulation in the cell wall of the root tip and thus regulates the Al resistance (Figure 1D).
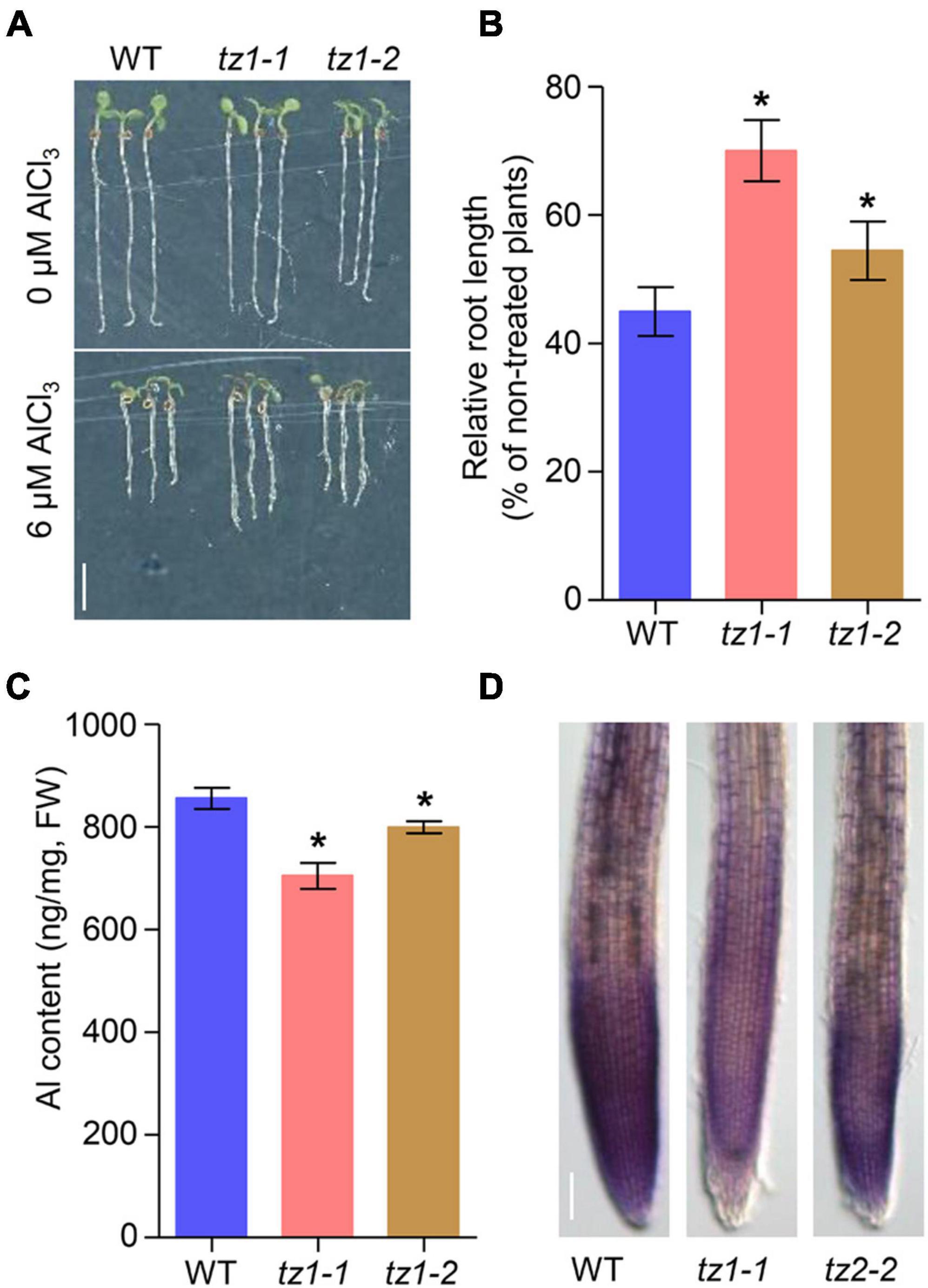
Figure 1. Transition zone1 (TZ1) mediates the Al stress-induced inhibition of root growth. (A,B) Root growth of wild type (WT) and TZ1 mutant seedlings after a 7-day exposure to 0 or 6 μM AlCl3. Three independent experiments were carried out, each with three replicates. Plants were grown at 22°C in long-day growth conditions. Scale bar, 0.5 cm. *p < 0.05 (Student’s t-test). (C) Al content in roots of WT seedlings and tz1 mutants. *p < 0.05 (Student’s t-test). (D) Hematoxylin staining of root tips exposed to 25 μM AlCl3 for 3 h. Scale bar, 100 μm.
Transition Zone1 Is Expressed in the Transition Zone and Localized in the Cytoplasm
Since TZ1 negatively regulates the Al resistance in Arabidopsis, we then generated TZ1p:TZ1-GFP-GUS transgenic plants to investigate the expression pattern of TZ1. GUS staining analyses showed TZ1 is expressed in the TZ, EZ, and mature zone (MZ) of the root, with the highest expression in the root TZ (Figure 2A). Without Al treatment, GFP signal was not detected in the root tips of TZ1p:TZ1-GFP-GUS plants, however, after 3-h exposure to Al stress, GFP signal was highly developed in the root TZ of the transgenic plants, indicating an upregulation of TZ1 in the root TZ (Figure 2B). Similarly, stronger GUS stain was found after Al treatment (Supplementary Figure 3). Quantitative real-time PCR (qRT-PCR) analyses of 7-day-old WT Arabidopsis roots with or without 25 μM AlCl3 treatment confirmed the increased expression of TZ1 at transcriptional level after Al treatment (Figure 2C). It is well known that the root TZ is the most sensitive site for Al toxicity, therefore, the high expression of TZ1 in the root TZ is consistent with the enhanced Al resistance of tz1 mutants. Additionally, we generated 35S::TZ1-YFP construct and transformed it into the protoplasts of Arabidopsis mesophyll cells to examine the subcellular localization of TZ1. The results showed that TZ1 was mainly located in the cytoplasm (Figure 3A). To further confirm the above results, we co-expressed 35S::TZ1-YFP with the cytosolic marker ADH-RFP (Yang Y. Z. et al., 2017) and the plasma membrane maker ZmCRN-mCherry (Je et al., 2018), respectively, and found that the YFP signal of the TZ1-YFP fusion protein was completely merged with ADH-RFP, but not ZmCRN-mCherry, implying the cytosolic localization of TZ1 protein (Figure 3B).
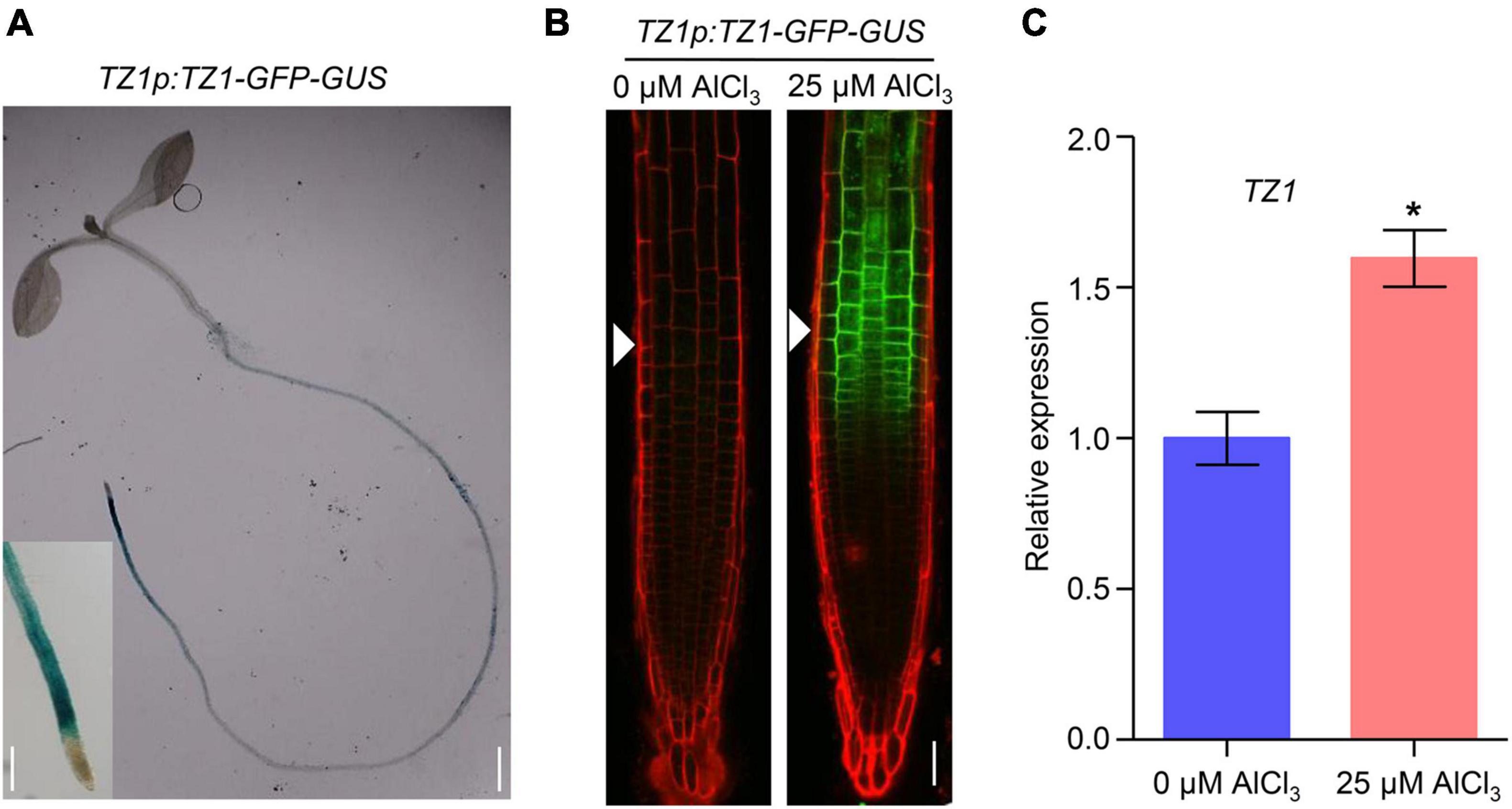
Figure 2. Aluminum stress-induced expression of TZ1 in the transition zone. (A) The β-glucuronidase (GUS) staining of TZ1p:TZ1-GFP-GUS showed that TZ1 is expressed in the Arabidopsis root, with the high expression in the transition zone (TZ), elongation zone (EZ), and mature zone (MZ). Left scale bar, 50 μm. Right scale bar, 1 cm. (B) 7-day-old TZ1p:TZ1-GFP-GUS seedlings were exposed to 0 or 25 μM AlCl3 for 3 h. Cell boundaries appear red following propidium iodide (PI) staining. The root TZ is marked with white arrowheads. Scale bar, 100 μm. (C) Relative expression of TZ1 by qRT-PCR assay in the 7-day-old WT Arabidopsis roots treated with 0 or 25 μM AlCl3 for 3 h. UBQ1 was used as the reference. Values are given as mean ± SD (n = 3). *p < 0.05 (Student’s t-test).
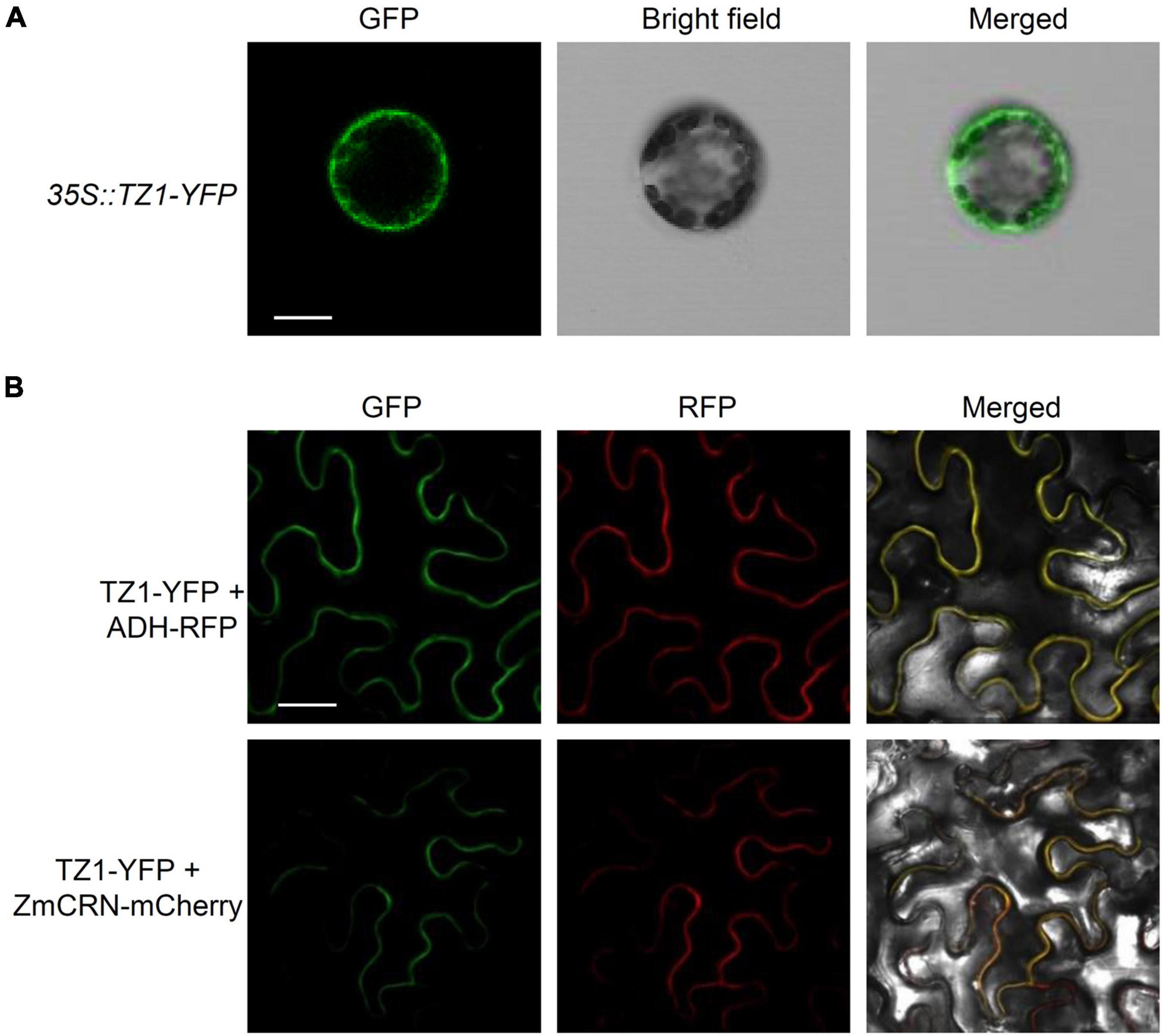
Figure 3. Subcellular localization of TZ1. (A) Plasmids containing genes encoding TZ1 protein and YFP protein were introduced into protoplasts of Arabidopsis mesophyll cells. Fluorescent and bright field images are shown. Scale bar, 50 μm. (B) Laser-scanning confocal images of TZ1-YFP fusion protein transiently expressed in Nicotiana. benthamiana leaf cells with ADH-RFP as a cytosolic maker or ZmCRN-mCherry as a plasma membrane maker. GFP: green fluorescent protein. RFP: red fluorescent protein. Scale bar, 50 μm.
Transition Zone1 Interacts With Aconitases
To investigate the underlying mechanisms of TZ1 in regulating the Al resistance, we carried out Y2H experiments with the Arabidopsis cDNA library to screen TZ1 interacting proteins and 10 fragments were identified to interact with TZ1 (Table 1). Finally, ACO1 was selected for further analysis since ACO1 is involved in the metabolism of citric acid which is an important Al-chelator in Al resistance. In addition to the interaction of TZ1 and ACO1 in the yeast (Figure 4A), we generated ACO1-cLUC and TZ1-nLUC constructs and confirmed their interaction by luciferase complementation assay (Figure 4B). The tobacco mesophyll cells co-transformed with ACO1-cLUC and TZ1-nLUC by A. tumefaciens-mediated transformation showed strong LUC signal, but not the control transformed with empty vectors (Figure 4B). Moreover, TZ1-YFP was successfully detected in the anti-MYC immunoprecipitates of proteins extracted from Arabidopsis mesophyll protoplasts transiently expressing ACO1-MYC and TZ1-YFP (Figure 4C). Furthermore, in vitro pull-down assays with GST-tagged ACO1 and MBP-tagged TZ1 confirmed TZ1 interacts with ACO1 (Figure 4D). Besides ACO1, there are two additional ACO homologous genes in the Arabidopsis genome, ACO2 and ACO3. Luciferase complementation and CoIP assays confirmed that TZ1 also interacts with ACO2 and ACO3 (Supplementary Figure 4).
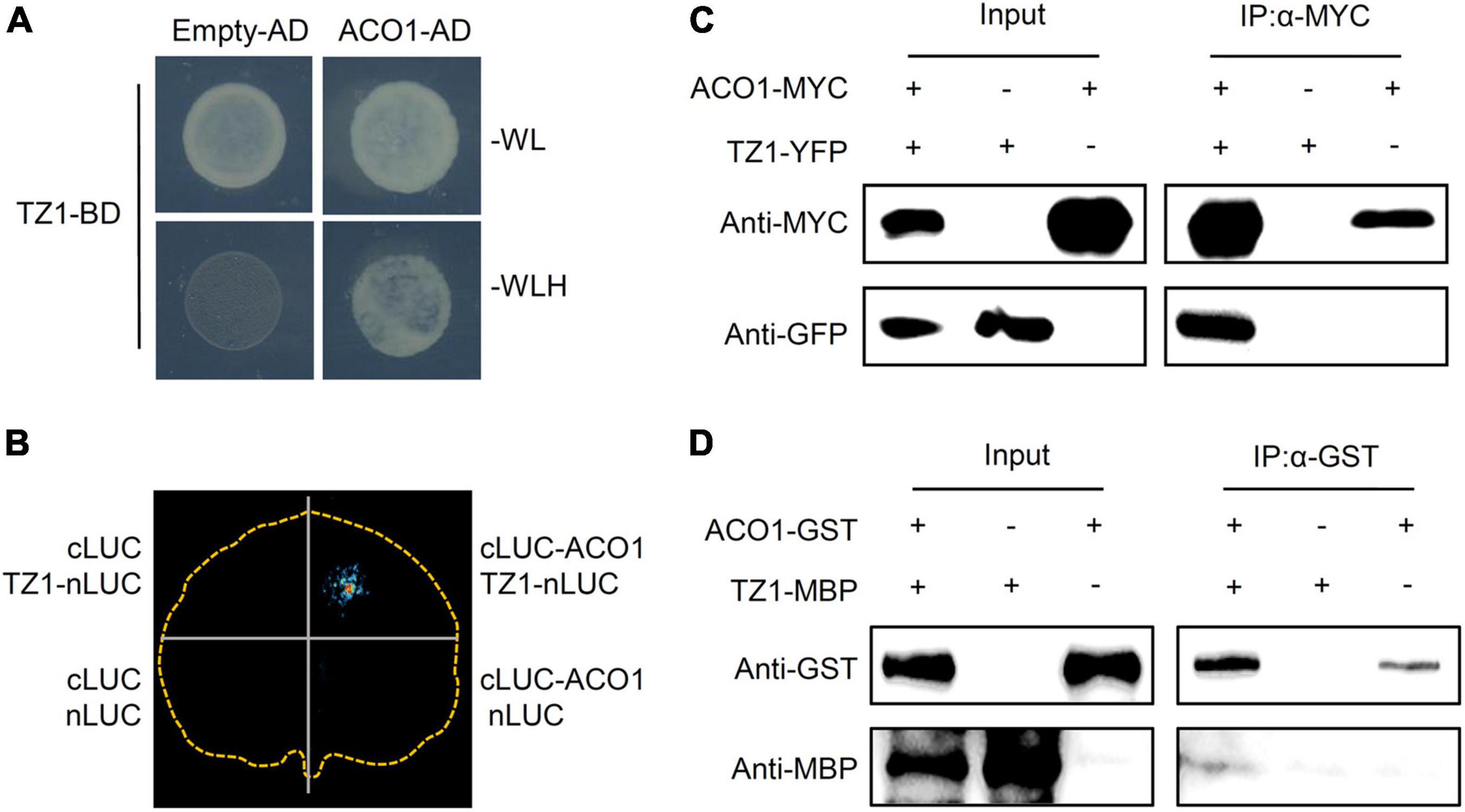
Figure 4. Transition zone1 interacts with ACO1. (A) Transition zone1 specifically interacts with aconitase1 (ACO1) in yeast. TZ1 was used as bait, the C-terminal of ACO1 was used as prey. Empty-AD was co-transformed as negative control. (B) Luciferase complementary imaging (LCI) analysis of interaction between TZ1 and ACO1. The N-terminal half of luciferase (nLUC) was fused to TZ1, and the C-terminal half of luciferase (cLUC) was fused to ACO1 (634–899 aa), and different construct combinations were co-expressed in N. benthamiana. LCI, luciferase complementation imaging. (C) In vivo Co-IP assay of TZ1 interaction with ACO1. ACO1-MYC was co-expressed with TZ1-YFP in Arabidopsis mesophyll cell protoplast. Protein extracts (Input) were immuno-precipitated with anti-MYC antibody (IP). Immunoblots were developed with anti-GFP antibody to detect TZ1 and with anti-MYC antibody to detect ACO1. (D) Western blotting assay of the GST pull down assay of ACO1-GST and TZ1-MBP.
The Arabidopsis Aconitase Mutants Display Enhanced Aluminum Resistance
Since TZ1 interacts with ACO proteins and tz1 mutants display Al resistance phenotype, we tried to investigate the role of Arabidopsis ACO genes in response to Al stress. Under normal conditions, the root length of aco1, aco2, and aco3 mutants exhibited slightly longer roots than WT (Figure 5A). When the plants were treated with 6 μM AlCl3 for 7 days, aco1, aco2, and aco3 mutants all showed less root growth inhibition when compared with the WT control (Figures 5A,B), which is similar to the tz1 mutants. Additionally, we detected the Al content in aco mutants and found that all three aco mutants displayed lower Al content than WT (Figure 5C). All these results implied that TZ1 may regulate the Al resistance by reducing the Al accumulation in the root through its interaction with ACO.
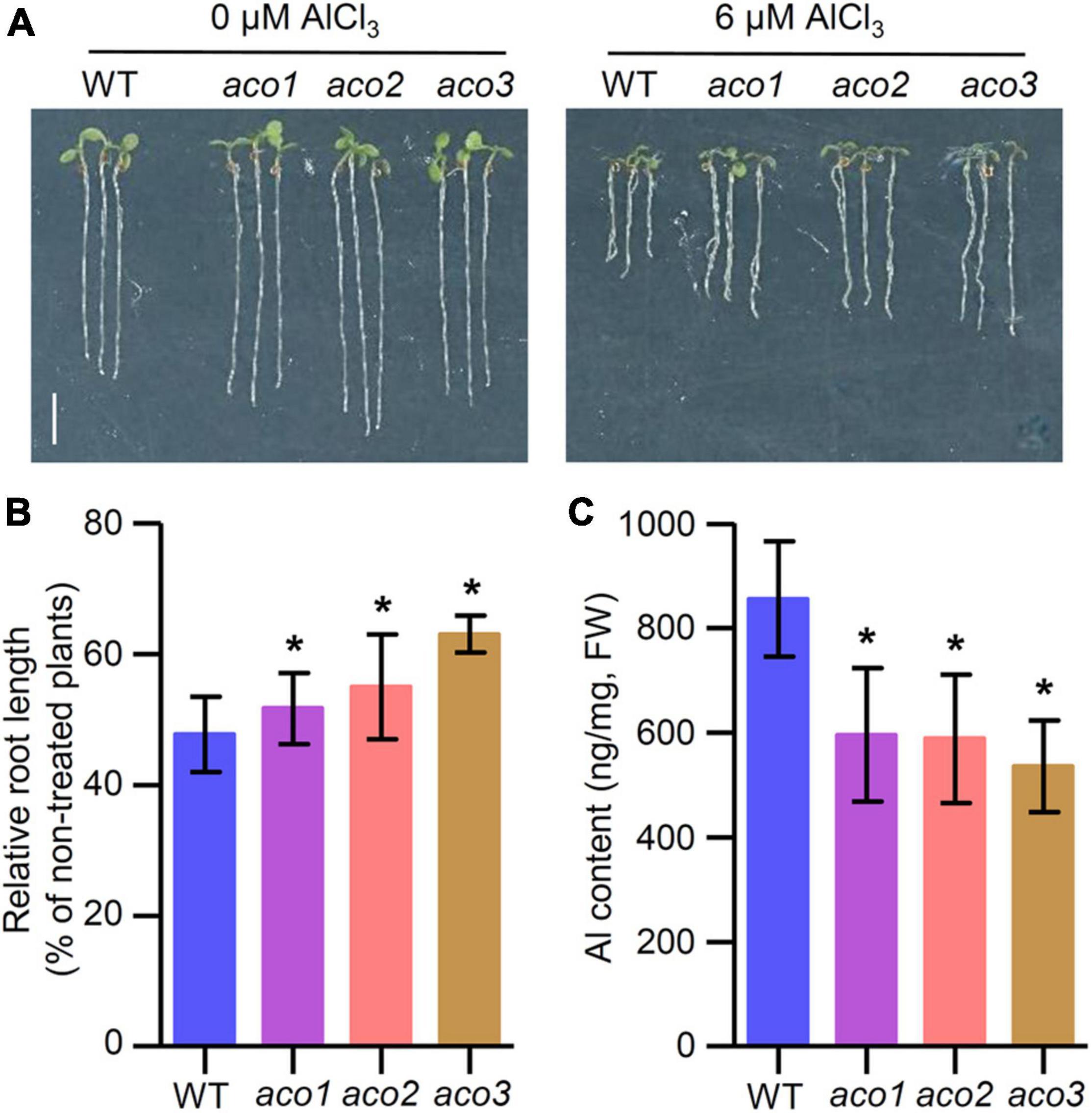
Figure 5. Aconitases (ACOs) mediate the Al stress-induced inhibition of root growth. (A,B) Root growth of WT and aco mutant seedlings after a 7-day exposure to 0 or 6 μM AlCl3. Three independent experiments were done, each with three replicates. Plants were grown at 22°C in long-day growth conditions. Scale bar, 0.5 cm. *p < 0.05 (Student’s t-test). (C) Al content in root of WT and aco mutants. *p < 0.05 (Student’s t-test).
Citric Acid Content Is Increased in Transition Zone1 Mutant
Aconitases play crucial roles in the Krebs cycle and catalyze the conversion of citric acid to isocitric acid. It is well known that citric acid can chelate toxic Al3+. Therefore, we measured the citric acid content of tz1 mutants through high performance liquid chromatography with WT plants as control. Results showed that the citric acid content of tz1-1 and tz1-2 mutants is higher than WT (Figures 6A,B). The higher content of citric acid is consistent with the decreased Al accumulation and increased Al resistance in tz1 mutants, implying TZ1 may function by influencing ACO activity and finally affect the citric acid homeostasis to regulate the Al stress response.
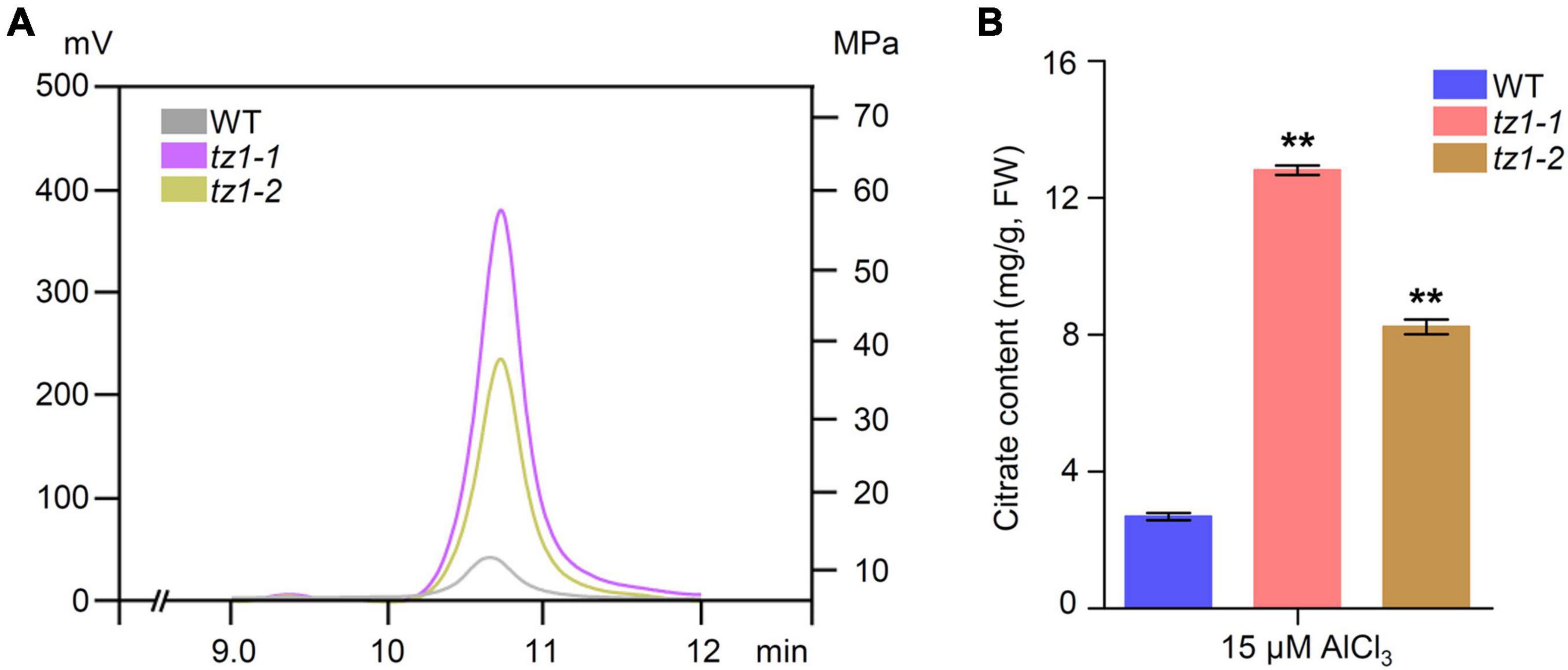
Figure 6. Citric acid content in tz1. (A) The citrate content in the roots were determined by high performance liquid chromatography (HPLC). The 7-day old seedings of WT and aco exposed to 15 μM AlCl3 for 24 h before measurement. (B) Quantification of the Al-induced citrate content in the WT and tz1 mutants. **p < 0.01 (Student’s t-test).
Discussion
Aluminum toxicity is one of the major factors that limits the crop production in acid soils (Liu et al., 2014; Sade et al., 2016). The exudation of organic acids, such as malate, citrate, and oxalate to chelate toxic Al3+ plays an important role in preventing Al3+ from entering the root cell (Kochian et al., 2015). In the current study, we characterized TZ1 which was upregulated by Al stress in the TZ to be a negative regulator of Al resistance in Arabidopsis (Figures 1, 2). Furthermore, TZ1 interacts with ACOs (Figure 4 and Supplementary Figure 4) and promotes the conversion of citric acid to isocitric acid, leading to a decrease in the citric acid content and the corresponding increase in Al deposition in the root cell wall and the attenuation of root growth in Al stress response.
Due to the crucial role of citric acid in Al resistance, its secretion and accumulation in response to the Al stress have been extensively studied (Liu et al., 2018; Wang et al., 2018; Qiu et al., 2019; Matonyei et al., 2020). The Al-activated root citrate exudation is facilitated by the citrate transporter MATE (Sasaki et al., 2004; Hoekenga et al., 2006; Ligaba et al., 2006; Furukawa et al., 2007; Magalhaes et al., 2007; Liu et al., 2009, 2018; Maron et al., 2010; Yang et al., 2011; Tovkach et al., 2013). The transcription factor STOP1 is well known to upregulate the MATE expression and thus controls the release of citrate (Iuchi et al., 2007; Sawaki et al., 2009; Fang et al., 2020). The Al resistance of plants could be achieved by increasing the in vivo citrate content. Citrate is an intermediate of tricarboxylic acid cycle and glyoxylate cycle and its content was controlled by the level of the relevant enzymes involved in citrate metabolism. The aco1- and idh12 mutants with more citrate displayed increased levels of Al resistance (Anoop et al., 2003). Overexpressing CS in tobacco, papaya, and Arabidopsis all lead to higher resistance to Al toxicity (De la Fuente et al., 1997; Koyama et al., 2000; Deng et al., 2009; Han et al., 2009). Our study first found an functionally unknown protein TZ1 interacts with ACOs (Figure 4 and Supplementary Figure 4) to negatively regulate the Al resistance by interfering with the citrate accumulation (Figure 6).
The activities and level of the citrate metabolism related enzymes are regulated by Al stress. It was reported that the activity of citrate synthases (CS) could be enhanced by Al stress in rye and Yuzu (Citrus Junos Sieb. ex Tanaka) (Li et al., 2000; Deng et al., 2009). Al stress affects the gene expression of citrate metabolism related enzymes including the upregulation of CS and ACO1 that catalyze the conversion of citric acid to isocitric acid in the TCA cycle and the downregulation of isocitrate dehydrogenase1 (IDH1) (Anoop et al., 2003). In our study, TZ1, an ACOs-interacting protein, is induced by Al stress at transcriptional level (Figure 2), presumably enhancing the activity of ACOs through the directly interaction with ACOs. Under Al stress, the interaction with TZ1 enhances the activity of ACO and thus balances the overproduced citrate by Al-activated citrate synthase. It is interesting to clarify how the activity of ACOs is affected by the interaction with TZ1. Our present results showed that TZ1 interacts with the C-terminal of ACOs (Figure 4 and Supplementary Figure 4). Whether the interaction with TZ1 influences the binding of ACOs to the substrate citrate, or the conformation and activity of ACOs, requires more research in the future.
Previous studies have shown that the root TZ is the most sensitive site in response to Al stress. Multiple phytohormones, such as auxin, cytokinin, and ethylene interact with each other in the root TZ and are involved in the regulation of root growth under Al stress (Dello Ioio et al., 2008; Sun et al., 2010; Yang Z. B. et al., 2014; Liu et al., 2016; Di Mambro et al., 2017; Yang Z. B. et al., 2017). However, little is known about whether the root TZ is an important site for other factors, such as organic acids in response to Al toxicity. In this study, TZ1 is highly upregulated in the root TZ after Al treatment and regulates plant Al resistance via ACO-regulated citrate catabolism (Figure 7). In Al toxicity responses, with the increased citrate production by CS, TZ1 is induced and interacts with ACOs to enhance the activity of ACO and finally promotes the conversion of citrate to isocitrate, and slow-down the plant growth. Under Al resistance condition, the decreased TZ1 releases ACO and results in the reduced activity of ACO, which finally results in the high content of citrate and increased Al resistance and plant growth. However, whether this proposed model of TZ1-ACOs module is widespread in nature requires further investigations in the future.
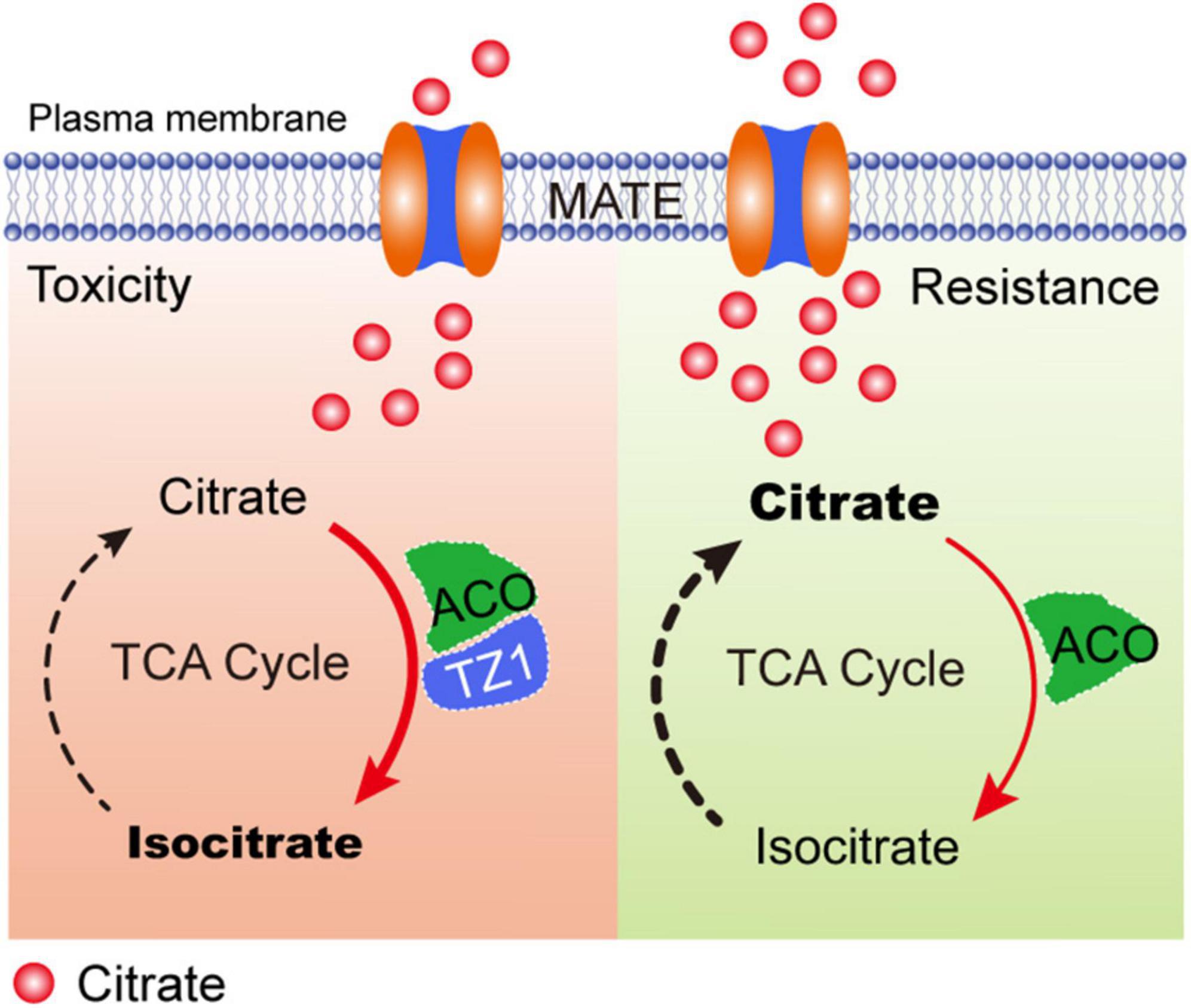
Figure 7. A proposed model of TZ1 and ACO mediated Al-stress response to control root growth. In toxicity responses, TZ1 is induced by Al stress and the interaction with ACO enhances the conversion of citrate to isocitrate and slows down the plant growth. Under Al resistance condition, the decreased TZ1 releases ACO and thus reduces the activity of ACO, which finally results in the high citrate content and enhanced Al resistance and plant growth.
Data Availability Statement
The datasets presented in this study can be found in online repositories. The names of the repository/repositories and accession number(s) can be found in the article/Supplementary Material.
Author Contributions
HT, ZD, and JL planned and designed the experiments. JL, BS, MZ, and GL performed the major experiments. HT and JL wrote the manuscript. All authors contributed to the article and approved the submitted version.
Funding
This work was supported by the National Natural Science Foundation of China (32070319 and 31770305) and the Youth Interdisciplinary Science and Innovative Research Groups of Shandong University (2020QNQT014).
Conflict of Interest
The authors declare that the research was conducted in the absence of any commercial or financial relationships that could be construed as a potential conflict of interest.
Publisher’s Note
All claims expressed in this article are solely those of the authors and do not necessarily represent those of their affiliated organizations, or those of the publisher, the editors and the reviewers. Any product that may be evaluated in this article, or claim that may be made by its manufacturer, is not guaranteed or endorsed by the publisher.
Acknowledgments
We thank Mingyi Bai (Shandong University) for the p35S::X-YFP vector and Qianhua Shen (Chinese Academy of Sciences) for the pCAMBIA1300-nLUC and pCAMBIA1300-cLUC vectors. We also thank Xiangpei Kong, Jiameng Xu, and Zipeng Yu for the constructive suggestions during the manuscript preparation.
Supplementary Material
The Supplementary Material for this article can be found online at: https://www.frontiersin.org/articles/10.3389/fpls.2021.827797/full#supplementary-material
References
Alarcon, M. V., and Salguero, J. (2017). Transition zone cells reach G2 phase before initiating elongation in maize root apex. Biol. Open 6, 909–913. doi: 10.1242/bio.025015
Anoop, V. M., Basu, U., Mccammon, M. T., Mcalister-Henn, L., and Taylor, G. J. (2003). Modulation of citrate metabolism alters aluminum tolerance in yeast and transgenic canola overexpressing a mitochondrial citrate synthase. Plant Physiol. 132, 2205–2217. doi: 10.1104/pp.103.023903
Baluska, F., and Mancuso, S. (2013). Root apex transition zone as oscillatory zone. Front. Plant Sci. 4:354. doi: 10.3389/fpls.2013.00354
Baluska, F., Mancuso, S., Volkmann, D., and Barlow, P. W. (2010). Root apex transition zone: a signalling-response nexus in the root. Trends Plant Sci. 15, 402–408. doi: 10.1016/j.tplants.2010.04.007
Barone, P., Rosellini, D., Lafayette, P., Bouton, J., Veronesi, F., and Parrott, W. (2008). Bacterial citrate synthase expression and soil aluminum tolerance in transgenic alfalfa. Plant Cell Rep. 27, 893–901. doi: 10.1007/s00299-008-0517-x
Brady, S. M., Orlando, D. A., Lee, J. Y., Wang, J. Y., Koch, J., Dinneny, J. R., et al. (2007). A high-resolution root spatiotemporal map reveals dominant expression patterns. Science 318, 801–806. doi: 10.1126/science.1146265
Chaiwanon, J., and Wang, Z. Y. (2015). Spatiotemporal brassinosteroid signaling and antagonism with auxin pattern stem cell dynamics in Arabidopsis roots. Curr. Biol. 25, 1031–1042. doi: 10.1016/j.cub.2015.02.046
Chauhan, D. K., Yadav, V., Vaculik, M., Gassmann, W., Pike, S., Arif, N., et al. (2021). Aluminum toxicity and aluminum stress-induced physiological tolerance responses in higher plants. Crit. Rev. Biotechnol. 41, 715–730. doi: 10.1080/07388551.2021.1874282
De la Fuente, J. M., RamıìRez-RodrıìGuez, V., Cabrera-Ponce, J. L., and Herrera-Estrella, L. (1997). Aluminum tolerance in transgenic plants by alteration of citrate synthesis. Science 276, 1566–1568. doi: 10.1126/science.276.5318.1566
Delhaize, E., Gruber, B. D., and Ryan, P. R. (2007). The roles of organic anion permeases in aluminium resistance and mineral nutrition. FEBS Lett. 581, 2255–2262. doi: 10.1016/j.febslet.2007.03.057
Dello Ioio, R., Nakamura, K., Moubayidin, L., Perilli, S., Taniguchi, M., Morita, M. T., et al. (2008). A genetic framework for the control of cell division and differentiation in the root meristem. Science 322, 1380–1384. doi: 10.1126/science.1164147
Deng, W., Luo, K., Li, Z., Yang, Y., Hu, N., and Wu, Y. (2009). Overexpression of Citrus junos mitochondrial citrate synthase gene in Nicotiana benthamiana confers aluminum tolerance. Planta 230, 355–365. doi: 10.1007/s00425-009-0945-z
Di Mambro, R., De Ruvo, M., Pacifici, E., Salvi, E., Sozzani, R., Benfey, P. N., et al. (2017). Auxin minimum triggers the developmental switch from cell division to cell differentiation in the Arabidopsis root. Proc. Natl. Acad. Sci. U.S.A. 114, E7641–E7649. doi: 10.1073/pnas.1705833114
Fang, Q., Zhang, J., Zhang, Y., Fan, N., Van Den Burg, H. A., and Huang, C. F. (2020). Regulation of aluminum resistance in Arabidopsis involves the SUMOylation of the Zinc finger transcription factor STOP1. Plant Cell 32, 3921–3938. doi: 10.1105/tpc.20.00687
Furukawa, J., Yamaji, N., Wang, H., Mitani, N., Murata, Y., Sato, K., et al. (2007). An aluminum-activated citrate transporter in barley. Plant Cell Physiol. 48, 1081–1091. doi: 10.1093/pcp/pcm091
Han, Y., Zhang, W., Zhang, B., Zhang, S., Wang, W., and Ming, F. (2009). One novel mitochondrial citrate synthase from Oryza sativa L. can enhance aluminum tolerance in transgenic tobacco. Mol. Biotechnol. 42, 299–305. doi: 10.1007/s12033-009-9162-z
Hoekenga, O. A., Maron, L. G., Pineros, M. A., Cancado, G. M., Shaff, J., Kobayashi, Y., et al. (2006). AtALMT1, which encodes a malate transporter, is identified as one of several genes critical for aluminum tolerance in Arabidopsis. Proc. Natl. Acad. Sci. U.S.A. 103, 9738–9743. doi: 10.1073/pnas.0602868103
Huang, C. F. (2021). Activation and activity of STOP1 in aluminium resistance. J. Exp. Bot. 72, 2269–2272. doi: 10.1093/jxb/erab015
Illés, P., Schlicht, M., Pavlovkin, J., Lichtscheidl, I., Baluska, F., and Ovecka, M. (2006). Aluminium toxicity in plants: internalization of aluminium into cells of the transition zone in Arabidopsis root apices related to changes in plasma membrane potential, endosomal behaviour, and nitric oxide production. J. Exp. Bot. 57, 4201–4213. doi: 10.1093/jxb/erl197
Iuchi, S., Koyama, H., Iuchi, A., Kobayashi, Y., Kitabayashi, S., Kobayashi, Y., et al. (2007). Zinc finger protein STOP1 is critical for proton tolerance in Arabidopsis and coregulates a key gene in aluminum tolerance. Proc. Natl. Acad. Sci. U.S.A. 104, 9900–9905. doi: 10.1073/pnas.0700117104
Je, B. I., Xu, F., Wu, Q., Liu, L., Meeley, R., Gallagher, J. P., et al. (2018). The CLAVATA receptor FASCIATED EAR2 responds to distinct CLE peptides by signaling through two downstream effectors. eLife 7:e35673. doi: 10.7554/eLife.35673
Kochian, L. V., Hoekenga, O. A., and Pineros, M. A. (2004). How do crop plants tolerate acid soils? Mechanisms of aluminum tolerance and phosphorous efficiency. Annu. Rev. Plant Biol. 55, 459–493. doi: 10.1146/annurev.arplant.55.031903.141655
Kochian, L. V., Pineros, M. A., Liu, J., and Magalhaes, J. V. (2015). Plant adaptation to acid soils: the molecular basis for crop aluminum resistance. Annu. Rev. Plant Biol. 66, 571–598. doi: 10.1146/annurev-arplant-043014-114822
Kollmeier, M., Felle, H. H., and Horst, W. J. (2000). Genotypical differences in aluminum resistance of maize are expressed in the distal part of the transition zone. Is reduced basipetal auxin flow involved in inhibition of root elongation by aluminum? Plant Physiol. 122, 945–956. doi: 10.1104/pp.122.3.945
Kong, X., Liu, G., Liu, J., and Ding, Z. (2018). The root transition zone: a hot spot for signal crosstalk. Trends Plant Sci. 23, 403–409. doi: 10.1016/j.tplants.2018.02.004
Koyama, H., Kawamura, A., Kihara, T., Hara, T., Takita, E., and Shibata, D. (2000). Overexpression of mitochondrial citrate synthase in Arabidopsis thaliana improved growth on a phosphorus-limited soil. Plant Cell Physiol. 41, 1030–1037. doi: 10.1093/pcp/pcd029
Li, X. F., Ma, J. F., and Matsumoto, H. (2000). Pattern of aluminum-induced secretion of organic acids differs between rye and wheat. Plant Physiol. 123, 1537–1544. doi: 10.1104/pp.123.4.1537
Ligaba, A., Katsuhara, M., Ryan, P. R., Shibasaka, M., and Matsumoto, H. (2006). The BnALMT1 and BnALMT2 genes from rape encode aluminum-activated malate transporters that enhance the aluminum resistance of plant cells. Plant Physiol. 142, 1294–1303. doi: 10.1104/pp.106.085233
Liu, G., Gao, S., Tian, H., Wu, W., Robert, H. S., and Ding, Z. (2016). Local transcriptional control of YUCCA regulates auxin promoted root-growth inhibition in response to aluminium stress in Arabidopsis. PLoS Genet. 12:e1006360. doi: 10.1371/journal.pgen.1006360
Liu, J., Magalhaes, J. V., Shaff, J., and Kochian, L. V. (2009). Aluminum-activated citrate and malate transporters from the MATE and ALMT families function independently to confer Arabidopsis aluminum tolerance. Plant J 57, 389–399. doi: 10.1111/j.1365-313X.2008.03696.x
Liu, J., Pineros, M. A., and Kochian, L. V. (2014). The role of aluminum sensing and signaling in plant aluminum resistance. J. Integr. Plant Biol. 56, 221–230. doi: 10.1111/jipb.12162
Liu, L., Yu, Z. P., Xu, Y., Guo, C., Zhang, L., Wu, C. G., et al. (2021). Function identification of MdTIR1 in apple root growth benefited from the predicted MdPPI network. J. Integr. Plant Biol. 63, 723–736.
Liu, M. Y., Lou, H. Q., Chen, W. W., Pineros, M. A., Xu, J. M., Fan, W., et al. (2018). Two citrate transporters coordinately regulate citrate secretion from rice bean root tip under aluminum stress. Plant Cell Environ. 41, 809–822. doi: 10.1111/pce.13150
Magalhaes, J. V., Liu, J., Guimaraes, C. T., Lana, U. G., Alves, V. M., Wang, Y. H., et al. (2007). A gene in the multidrug and toxic compound extrusion (MATE) family confers aluminum tolerance in sorghum. Nat. Genet. 39, 1156–1161. doi: 10.1038/ng2074
Maron, L. G., Pineros, M. A., Guimaraes, C. T., Magalhaes, J. V., Pleiman, J. K., Mao, C., et al. (2010). Two functionally distinct members of the MATE (multi-drug and toxic compound extrusion) family of transporters potentially underlie two major aluminum tolerance QTLs in maize. Plant J. 61, 728–740. doi: 10.1111/j.1365-313X.2009.04103.x
Matonyei, T. K., Barros, B. A., Guimaraes, R. G. N., Ouma, E. O., Cheprot, R. K., Apolinario, L. C., et al. (2020). Aluminum tolerance mechanisms in Kenyan maize germplasm are independent from the citrate transporter ZmMATE1. Sci. Rep. 10:7320. doi: 10.1038/s41598-020-64107-z
Millar, A. H., Whelan, J., Soole, K. L., and Day, D. A. (2011). Organization and regulation of mitochondrial respiration in plants. Annu. Rev. Plant Biol. 62, 79–104. doi: 10.1146/annurev-arplant-042110-103857
Motoda, H., Sasaki, T., Kano, Y., Ryan, P. R., Delhaize, E., Matsumoto, H., et al. (2007). The membrane topology of ALMT1, an aluminum-activated malate transport protein in wheat (Triticum aestivum). Plant Signal. Behav. 2, 467–472. doi: 10.4161/psb.2.6.4801
Polle, E., Konzak, C. F., and Kattrick, J. A. (1978). Visual detection of aluminum tolerance levels in wheat by hematoxylin staining of seedling roots 1. Crop Sci. 18, 823–827.
Qiu, W., Wang, N., Dai, J., Wang, T., Kochian, L. V., Liu, J., et al. (2019). AhFRDL1-mediated citrate secretion contributes to adaptation to iron deficiency and aluminum stress in peanuts. J. Exp. Bot. 70, 2873–2886. doi: 10.1093/jxb/erz089
Rangel, A. F., Rao, I. M., and Horst, W. J. (2007). Spatial aluminium sensitivity of root apices of two common bean (Phaseolus vulgaris L.) genotypes with contrasting aluminium resistance. J. Exp. Bot. 58, 3895–3904. doi: 10.1093/jxb/erm241
Ryan, P. R., and Kochian, L. V. (1993). Interaction between aluminum toxicity and calcium uptake at the root apex in near-isogenic lines of wheat (Triticum aestivum L.) differing in aluminum tolerance. Plant Physiol. 102, 975–982. doi: 10.1104/pp.102.3.975
Sade, H., Meriga, B., Surapu, V., Gadi, J., Sunita, M. S., Suravajhala, P., et al. (2016). Toxicity and tolerance of aluminum in plants: tailoring plants to suit to acid soils. Biometals 29, 187–210. doi: 10.1007/s10534-016-9910-z
Sasaki, T., Yamamoto, Y., Ezaki, B., Katsuhara, M., Ahn, S. J., Ryan, P. R., et al. (2004). A wheat gene encoding an aluminum-activated malate transporter. Plant J. 37, 645–653. doi: 10.1111/j.1365-313x.2003.01991.x
Sawaki, Y., Iuchi, S., Kobayashi, Y., Kobayashi, Y., Ikka, T., Sakurai, N., et al. (2009). STOP1 regulates multiple genes that protect Arabidopsis from proton and aluminum toxicities. Plant Physiol. 150, 281–294. doi: 10.1104/pp.108.134700
Sivaguru, M., Baluska, F., Volkmann, D., Felle, H. H., and Horst, W. J. (1999). Impacts of aluminum on the cytoskeleton of the maize root apex. short-term effects on the distal part of the transition zone. Plant Physiol. 119, 1073–1082. doi: 10.1104/pp.119.3.1073
Sivaguru, M., Liu, J., and Kochian, L. V. (2013). Targeted expression of SbMATE in the root distal transition zone is responsible for sorghum aluminum resistance. Plant J. 76, 297–307. doi: 10.1111/tpj.12290
Sun, P., Tian, Q. Y., Chen, J., and Zhang, W. H. (2010). Aluminium-induced inhibition of root elongation in Arabidopsis is mediated by ethylene and auxin. J. Exp. Bot. 61, 347–356. doi: 10.1093/jxb/erp306
Tesfaye, M., Temple, S. J., Allan, D. L., Vance, C. P., and Samac, D. A. (2001). Overexpression of malate dehydrogenase in transgenic alfalfa enhances organic acid synthesis and confers tolerance to aluminum. Plant Physiol. 127, 1836–1844.
Tovkach, A., Ryan, P. R., Richardson, A. E., Lewis, D. C., Rathjen, T. M., Ramesh, S., et al. (2013). Transposon-mediated alteration of TaMATE1B expression in wheat confers constitutive citrate efflux from root apices. Plant Physiol. 161, 880–892. doi: 10.1104/pp.112.207142
Trejo-Téllez, L. I., Stenzel, R., Gómez-Merino, F. C., and Schmitt, J. M. (2009). Transgenic tobacco plants overexpressing pyruvate phosphate dikinase increase exudation of organic acids and decrease accumulation of aluminum in the roots. Plant Soil 326, 187–198.
Wang, Q., Yu, L., and Yu, C. A. (2010). Cross-talk between mitochondrial malate dehydrogenase and the cytochrome bc1 complex. J. Biol. Chem. 285, 10408–10414. doi: 10.1074/jbc.M109.085787
Wang, Y., Cai, Y., Cao, Y., and Liu, J. (2018). Aluminum-activated root malate and citrate exudation is independent of NIP1;2-facilitated root-cell-wall aluminum removal in Arabidopsis. Plant Signal. Behav. 13:e1422469. doi: 10.1080/15592324.2017.1422469
Wu, L., Sadhukhan, A., Kobayashi, Y., Ogo, N., Tokizawa, M., Agrahari, R. K., et al. (2019). Involvement of phosphatidylinositol metabolism in aluminum-induced malate secretion in Arabidopsis. J. Exp. Bot. 70, 3329–3342. doi: 10.1093/jxb/erz179
Xu, J., Zhu, J., Liu, J., Wang, J., Ding, Z., and Tian, H. (2021). SIZ1 negatively regulates aluminum resistance by mediating the STOP1-ALMT1 pathway in Arabidopsis. J. Integr. Plant Biol. 63, 1147–1160. doi: 10.1111/jipb.13091
Xu, J. M., Fan, W., Jin, J. F., Lou, H. Q., Chen, W. W., Yang, J. L., et al. (2017). Transcriptome analysis of al-induced genes in buckwheat (Fagopyrum esculentum Moench) root apex: new insight into al toxicity and resistance mechanisms in an Al accumulating species. Front. Plant Sci. 8:1141. doi: 10.3389/fpls.2017.01141
Yan, L., Wei, S., Wu, Y., Hu, R., Li, H., Yang, W., et al. (2015). High-efficiency genome editing in Arabidopsis using YAO promoter-driven CRISPR/Cas9 System. Mol. Plant 8, 1820–1823. doi: 10.1016/j.molp.2015.10.004
Yang, X. Y., Yang, J. L., Zhou, Y., Pineros, M. A., Kochian, L. V., Li, G. X., et al. (2011). A de novo synthesis citrate transporter, Vigna umbellata multidrug and toxic compound extrusion, implicates in Al-activated citrate efflux in rice bean (Vigna umbellata) root apex. Plant Cell Environ. 34, 2138–2148. doi: 10.1111/j.1365-3040.2011.02410.x
Yang, Y. Z., Ding, S., Wang, Y., Li, C. L., Shen, Y., Meeley, R., et al. (2017). Small kernel2 encodes a glutaminase in vitamin B(6) biosynthesis essential for maize seed development. Plant Physiol. 174, 1127–1138. doi: 10.1104/pp.16.01295
Yang, Z. B., Geng, X., He, C., Zhang, F., Wang, R., Horst, W. J., et al. (2014). TAA1-regulated local auxin biosynthesis in the root-apex transition zone mediates the aluminum-induced inhibition of root growth in Arabidopsis. Plant Cell 26, 2889–2904. doi: 10.1105/tpc.114.127993
Yang, Z. B., Liu, G., Liu, J., Zhang, B., Meng, W., Muller, B., et al. (2017). Synergistic action of auxin and cytokinin mediates aluminum-induced root growth inhibition in Arabidopsis. EMBO Rep. 18, 1213–1230. doi: 10.15252/embr.201643806
Yu, Z., Zhang, D., Xu, Y., Jin, S., Zhang, L., Zhang, S., et al. (2019). CEPR2 phosphorylates and accelerates the degradation of PYR/PYLs in Arabidopsis. J. Exp. Bot. 70, 5457–5469. doi: 10.1093/jxb/erz302
Zhang, Y., Zhang, J., Guo, J., Zhou, F., Singh, S., Xu, X., et al. (2019). F-box protein RAE1 regulates the stability of the aluminum-resistance transcription factor STOP1 in Arabidopsis. Proc. Natl. Acad. Sci. U.S.A. 116, 319–327. doi: 10.1073/pnas.1814426116
Keywords: aluminum stress, root growth, TZ1, ACO, citrate
Citation: Liu J, Shi B, Zhang M, Liu G, Ding Z and Tian H (2022) Transition Zone1 Negatively Regulates Arabidopsis Aluminum Resistance Through Interaction With Aconitases. Front. Plant Sci. 12:827797. doi: 10.3389/fpls.2021.827797
Received: 02 December 2021; Accepted: 27 December 2021;
Published: 27 January 2022.
Edited by:
Jian Li Yang, Zhejiang University, ChinaCopyright © 2022 Liu, Shi, Zhang, Liu, Ding and Tian. This is an open-access article distributed under the terms of the Creative Commons Attribution License (CC BY). The use, distribution or reproduction in other forums is permitted, provided the original author(s) and the copyright owner(s) are credited and that the original publication in this journal is cited, in accordance with accepted academic practice. No use, distribution or reproduction is permitted which does not comply with these terms.
*Correspondence: Huiyu Tian, dGlhbmh1aXl1QHNkdS5lZHUuY24=