- 1Key Laboratory of Saline-Alkali Vegetation Ecology Restoration (Northeast Forestry University), Ministry of Education, Harbin, China
- 2College of Life Sciences and Food Engineering, Inner Mongolia Minzu University, Tongliao, China
- 3Zhejiang Provincial Key Laboratory for Water Environment and Marine Biological Resources Protection, College of Life and Environmental Science, Wenzhou University, Wenzhou, China
Glutathione (GSH) conjugation with intermediates is required for the biosynthesis of glucosinolate (GSL) by serving as a sulfur supply. Glutathione-S-transferases (GSTs) primarily work on GSH conjugation, suggesting their involvement in GSL metabolism. Although several GSTs, including GSTF11 and GSTU20, have been recently postulated to act in GSL biosynthesis, molecular evidence is lacking. Here, we demonstrated that GSTF11 and GSTU20 play non-redundant, although partially overlapping, roles in aliphatic GSL biosynthesis. In addition, GSTU20 plays a more important role than GSTF11, which is manifested by the greater loss of aliphatic GSLs associated with GSTU20 mutant and a greater number of differentially expressed genes in GSTU20 mutant compared to GSTF11 mutant. Moreover, a double mutation leads to a greater aggregate loss of aliphatic GSLs, suggesting that GSTU20 and GSTF11 may function in GSL biosynthesis in a dosage-dependent manner. Together, our results provide direct evidence that GSTU20 and GSTF11 are critically involved in aliphatic GSL biosynthesis, filling the knowledge gap that has been speculated in recent decades.
Introduction
Glucosinolates (GSLs) are sulfur-rich secondary metabolites primarily present in Brassicale plants and well-known as important defense compounds that are beneficial to human health (Petersen et al., 2018). GSLs share a common core structure with an S-β-D-glucopyranose connected to an O-sulfated (Z)- thiohydroximate ester via a sulfur atom and are originally derived from amino acids (Agerbirk and Olsen, 2012; Blažević et al., 2020). Depending on the precursor amino acid, GSLs are grouped into three categories, including aliphatic GSLs (derived from alanine, isoleucine, leucine, methionine, and valine), indolic GSLs (derived from tryptophan) and aromatic GSLs (derived from phenylalanine and tyrosine) (Fahey et al., 2001; Halkier and Gershenzon, 2006).
The GSL biosynthetic pathway has been almost completely elucidated in recent decades (Halkier and Gershenzon, 2006; Sønderby et al., 2010; Nguyen et al., 2020). In brief, the biosynthesis of GSLs involves three key steps: side chain elongation with precursor amino acids, construction of a GSL core structure including sulfate assimilation, and secondary modifications of the side chain (Grubb and Abel, 2006; Sønderby et al., 2010; Petersen et al., 2018). The elongation process initiates with a transamination reaction catalyzed by branched-chain amino acid aminotransferases (BCATs). The side chain is then subjected to condensation with acetyl-CoA by methylthioalkylmalate synthases (MAMs), followed by isomerization and oxidative decarboxylation by isopropylmate isomerases (IPMs) and isopropylmalate dehydrogenases (IMDHs) (Kliebenstein et al., 2001; Schuster et al., 2006; Textor et al., 2007; He et al., 2009, 2011; Kroymann, 2011). Later, several biochemical reactions facilitate the production of the GSL core structure: oxidation by cytochrome P450 monooxygenases (cytochrome P450) of the CYP79 family, oxidation with conjugation by the CYP83 family, C-S cleavage by C-S lyase SUR1, glucosylation by glucosyltransferases of the UGT74 family and sulfation by sulfotransferases (SOT) (Bak and Feyereisen, 2001; Grubb et al., 2004; Mikkelsen et al., 2004; Piotrowski et al., 2004; Sønderby et al., 2010; Harun et al., 2020). Ultimately, the secondary modification of side chains undergoes oxidation, elimination, alkylation or esterification according to the distinct categories of GSLs (Harun et al., 2020).
As multifunctional enzymes, glutathione-S-transferases (GSTs) are primarily involved in the conjugation of the tripeptide glutathione (GSH) to the electrophilic center of lipophilic compounds (Labrou et al., 2015). Based on the similarity of amino acid sequences, plant specific GSTs are classified into the tau (GSTU) and phi (GSTF) types (Wagner et al., 2002). GSH contributes to the core structure synthesis of GSLs as a sulfur donor, raising the probability that GSTs are involved in the biosynthesis of GSLs. Indeed, GSTF9, GSTF10, and GSTU13 have been recognized to participate in the indolic GSLs biosynthesis (Piślewska-Bednarek et al., 2018). In contrast, it remains unclear that which GSTs function in aliphatic GSL biosynthesis, although GSTF11 and GSTU20 (Supplementary Figure 1) have been identified through multifaceted gene co-expression network analysis (Hirai et al., 2005, 2007; Wentzell et al., 2007; Czerniawski and Bednarek, 2018). The assumption of GSTF11 and GSTU20 are involved in GSL biosynthesis relies completely on in silico prediction, and molecular evidence is lacking.
In this study, we created GSTF11 and GSTU20 mutants using the CRISPR/Cas9 approach to ascertain the biological roles of GSTF11 and GSTU20 in GSL biosynthesis. Our results demonstrate that GSTF11 and GSTU20 are involved in aliphatic GSL biosynthesis with partially overlapping but non-redundant functions. Moreover, the aggregate loss of aliphatic GSLs observed in the double mutant implies that GSTF11 and GSTU20 also work in a dose-dependent manner. However, a substantial amount of aliphatic GSLs remain presence in the double mutant, suggesting that other GST family proteins are involved in aliphatic GSL biosynthesis, which awaits further exploration.
Materials and Methods
Plant Materials and Growth Conditions
The Columbia accession of Arabidopsis thaliana was used as the wild-type plant. Seeds were surface-sterilized and germinated on 1/2 Murashige and Skoog medium containing 2% sucrose and 0.8% Phytagar and grown in a 22°C growth chamber with a 16-h light and 8-h dark photoperiod after vernalization at 4°C for 3 days. One-week-old seedlings were then transferred to soil and grown under the aforementioned conditions.
To generate knockout mutants of GSTF11 (AT3G03190) and GSTU20 (AT1G78370) based on the CRISPR-Cas9 system, the online website CRISPR-PLANT was used to design the gRNA spacer sequences (Xie et al., 2014), and the CRISPR/Cas9 vector was constructed as described previously (Wang et al., 2015). The full-length CDS of GSTF11/U20 was amplified and then integrated into pDONR222 (entry vector) and pGWB551 (destination vector) to generate overexpression lines. For plant transformation, all binary vectors were transformed into Agrobacteria strain GV3101 and subjected to the floral dipping method. Double mutants for GSTF11 and GSTU20 were created by crossing between gstf11-2 and gstu20-2 single mutants which use gstf11-2 as pollen supplier. The primers used for constructs cloning and genotyping are listed in Supplementary Table 1.
Co-expression Analysis
Glutathione-S-transferases and well-known aliphatic GSL synthesis genes (Supplementary Table 2) were retrieved from The Arabidopsis Information Resource (TAIR).1 Co-expression analysis was performed using ATTED 10.12 and STRING3 platforms. Gene co-expression networks were drawn using the online tool NetworkDrawer.4
Construction of β-Glucuronidase (GUS) Reporter and GUS Staining
Arabidopsis genomic DNA was extracted using the CTAB method and treated with RNase to remove RNA. For the generation of GUS reporter constructs, a genomic fragment of 668 and 1,454 bp upstream of translational start codon ATG of GSTF11 and GSTU20 was amplified, then cloned into pCAMBIA1305 using SalI and NcoI restriction sites. The primers used in these experiments are listed in Supplementary Table 1. Histochemical GUS assays were performed on T3 generation of GUS stable expression lines by GUS staining solution (Solarbio) according to the instruction. The sample were firstly fixed in the fixation buffer for 45 min and washed by diluted GUS Buffer A with three times. Then immersed the tissues in 500 mL GUS staining buffer and incubated at 37°C for 24 h. The pictures of GUS expression in different tissues were captured by optical microscope (Olympus SZX10).
Gene Expression Analysis
Total RNA was extracted from 100 mg leaf, stem and root of 3-week-old seedlings or flower and silique of mature plants with TRIzol reagent and then treated with gDNA Eraser to remove DNA contamination. cDNA was synthesized by Maxima H Minus reverse transcriptase (Thermo Scientific). SYBR Premix Ex TaqTM (Takara) was used for qRT-PCR analysis. The relative expression level of genes was calculated following the 2–ΔΔCt method and normalized to the expression level of ACTIN2. Three technical replications were conducted for each biological experiment. The primers used in the qRT-PCR analysis are listed in Supplementary Table 1.
Protoplast Isolation and Transfection
Two-week-old seedlings grown on 1/2 MS were used to isolate protoplasts as described previously with minor modifications (Jung et al., 2015), 10 g seedlings were used to generate more protoplast cells. The full-length coding sequences of GSTF11/U20 without stop codon was PCR amplified and cloned into pDONR222 vector by gateway BP Clonase (Invitrogen, 11789020), then inserted into the pSAT6-EGFP vector (Tzfira et al., 2005) by gateway LR Clonase (Invitrogen, 11791020). Approximately 10 μg DNA of recombined constructs (GSTF11/U20 fused with EGFP driven by 35S promoter) was transformed into protoplast cells as described by Yoo et al. (2007), incubated at room temperature for 16 h or longer, and observed for GFP signal under an Olympus BX53 microscope with a 40× objective to assess the subcellular localization of GSTF11/U20.
Transcriptome Analysis
Total RNA was extracted from 3-week-old plants with TRIzol (Invitrogen) and purified using a GeneJET plant RNA purification kit (Thermo Fisher Scientific). RNA integrity and concentration were assessed by gel electrophoresis and using the Qubit® 2.0 Fluorometer (Thermo Fisher Scientific). RNA (1.5 μg) was used for cDNA library preparation with the NEBNext® Ultra™ RNA Library Prep Kit for Illumina® (New England Biolabs, NEB) following the manufacturer’s protocol. Library quality was monitored using an Agilent Bioanalyzer 2100 (Agilent Technologies). The cDNA libraries were sequenced on an Illumina HiSeq 2500 platform, and 150-bp paired-end reads were generated.
Gene functional annotation was conducted by aligning reads to the Arabidopsis genome sequence (TAIR 10). Following alignment, the count of mapped reads from each sample was derived and normalized as RPKM (reads per kilobase of exon model per million mapped reads). Differentially expressed genes (DEGs) were identified using the DESeq R package (1.10.1). Genes with log2 fold change ≥ 1 and an FDR adjusted p value less than 0.05 were considered DEGs. GO term enrichment of DEGs was analyzed using tools in TAIR.
Ultra-Performance Liquid Chromatography (UPLC) Analysis of Glucosinolates
Total GSLs were extracted from 150 mg leaves of 3-week-old seedlings or 20 mg mature seeds according to previously reported protocols (Chen et al., 2003; Alvarez et al., 2008). The sample were ground after adding 1 mL preheated 70% MeOH and vortex for 1 min, further incubated at 80°C for 10 min and centrifuge at 4,000 g for 10 min, collect supernatant and repeat the extraction one more time. Loading 1 mL DEAE-Sephadex A-25 (Sigma-Aldrich) into chromatographic column and cover with some quartz sand, add the extracted GSL sample into column then wash the column successively by 70% MeOH, ddH2O and 20 mM acetate solution, incubated the sample overnight at RT after adding 0.5 mL sulfatase (Sigma-Aldrich), collect GSL extraction by 1.5 mL ddH2O washing for further analysis. Each component of GSLs was analyzed using ultra-performance liquid chromatography (Waters ACQUITY UPLC M-Class) with an Atlantis T3 C18 column (2.1 mm × 150 mm, 3 μm, Waters) based on UV detector. The flow rate was kept at 0.4 mL/min, the column temperature was maintained at 25°C and the injection volume was 5 μL. 0.1% Trifluoroacetic acid in water as eluent A and methanol as eluent B was set as the mobile phase. Gradient elution conditions were as follows: 0–7.6 min, 0–60% B; 7.6–8.2 min, 60–100% B; 8.2–8.8 min, 100% B; 8.8–9.6 min, 100–0% B). Ten μL of 5 mM desulfonated benzyl GSL were added in each sample as the internal standard, quantification was obtained according to integrative peak areas using known relative response factors at 229 nm. Data presented are the means of three biological repeats.
The abbreviations of each component of GSLs described as follows: 3MSOP, 3-methylsulfinylpropyl GSL; 3BOP, 3-benzoylpropyl GSL; 3OHP, 3-hydroxylpropyl GSL; 4MTP, 4-methylthiobutyl GSL; 4MSOB, 4-methylsulfinylbutyl GSL; 4BOB, 4-benzoylbuthyl GSL; 5MSOP, 5-methylsulphinylpentyl GSL; 5MTP, 5-methylthiopentyl GSL; 6MSOH, 6-methylsulphinylhexyl GSL; 6MTH, 6-methylthiohexyl GSL; 7MTH, 7-methylthiohepthyl GSL; 8MSOO, 8-methylsulphinyloctyl GSL; 8MTO, 8-methylthiooctyl GSL; I3M, indolyl-3-methyl GSL; 1MOI3M, 1-methoxyindol-3-ylmethyl GSL; 4MOI3M, 4-methoxyindol-3-ylmethyl GSL; 4OHI3M, 4-hydroxyindol-3-ylmethyl GSL.
Statistical Analysis
All claims of statistical significance (p < 0.05) were assessed by two-way ANOVA (p < 0.05) with Tukey’s HSD post hoc test.
Results
GSTF11 and GSTU20 Are Co-expressed With Numerous Genes Involved in Aliphatic Glucosinolate Biosynthesis
Many former gene co-expression analyses have identified GSTF11 and GSTU20 as candidate genes involved in the biosynthesis of aliphatic GSLs (Hirai et al., 2005, 2007; Wentzell et al., 2007; Hirai, 2009). To better interpret the co-expression, we constructed a gene regulatory network (GRN) of GSTF11 and GSTU20 with a total of 20 well-characterized genes involved in aliphatic GSL biosynthesis using the ATTED-II platform (Obayashi et al., 2018; Supplementary Table 2). As shown in Figure 1A, although all of the genes tested could be classified into a complex GRN module, a distinct but direct connection of genes with GSTF11 or GSTU20 was observed. In addition, the solid connections of GSTF11 and GSTU20 with a few known genes involved in aliphatic GSLs were further visualized after performing gene co-expression analysis using STRING co-expression viewers (Szklarczyk et al., 2019; Figure 1B). Moreover, GSTF11 and GSTU20 also displayed intimate correlations with aliphatic GSL biosynthetic genes in the protein-protein interaction network generated using the STRING program (Supplementary Figure 2). Overall, the integrative multiple in silico analyses support the putative involvement of GSTF11 and GSTU20 in aliphatic GSL biosynthesis.
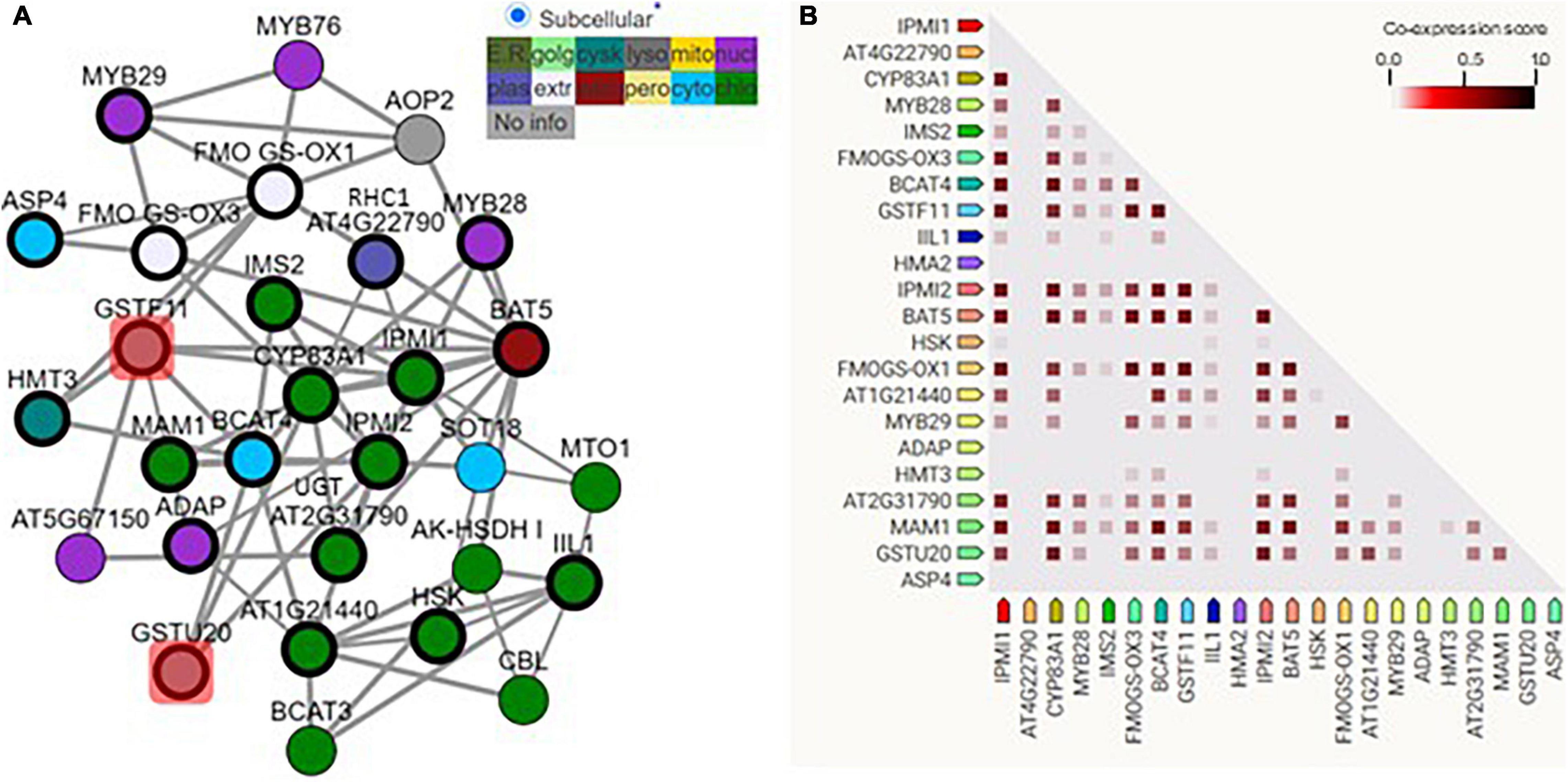
Figure 1. GSTF11/U20 correlated with glucosinolate (GSL) biosynthesis genes. (A) The regulatory network of GSTF11/U20 with genes involved in the biosynthesis of aliphatic GSLs. GSTF11/U20 are highlighted by red squares connected with several detected aliphatic GSL genes. The genes present in the network image are listed in Supplementary Table 1. Different color nodes represent the predicted or experimental subcellular information of each gene. (B) Co-expression strength predicts the functional association between GSTF11/U20 and aliphatic GSL genes. The intensity of the color in the triangle matrices indicates the level of confidence that two proteins are functionally associated, and more confidence was noted when the score approached 1.
GSTF11 and GSTU20 Localize to the Cytosol and Display Distinct Tissue-Specific Expression Patterns
To gain insights into the subcellular localization of GSTF11 and GSTU20, the full-length coding sequences of GSTF11 and GSTU20 fused to enhanced green fluorescent protein (EGFP) under the control of 35S promoter were transformed into Arabidopsis protoplast cells. Using fluorescence microscopy, both GSTF11 and GSTU20 were observed primarily in the cytoplasm (Figure 2A).
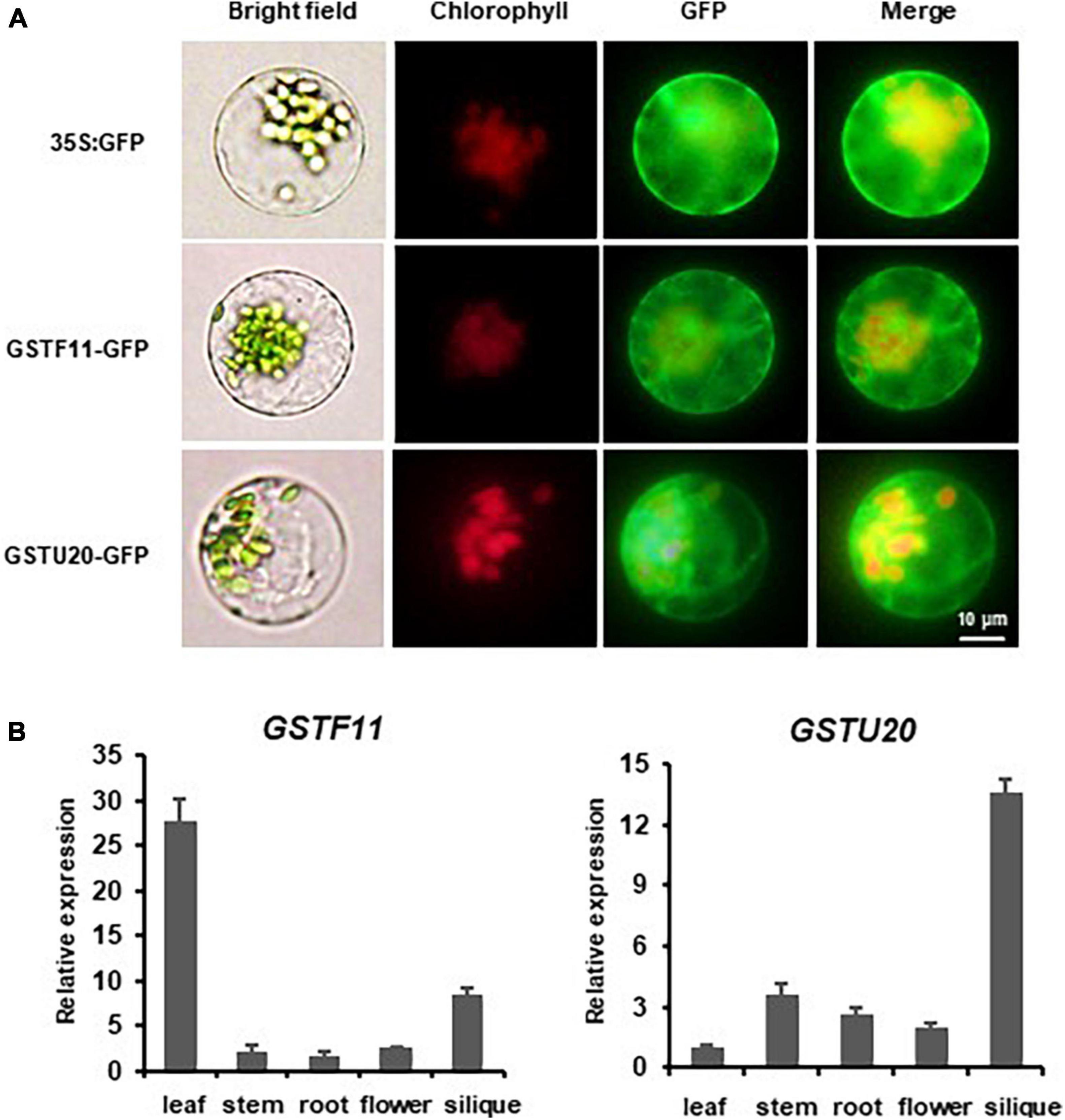
Figure 2. The expression pattern of GSTF11/U20 in Arabidopsis. (A) Subcellular localization analysis of GSTF11/U20. Fluorescent signals of GSTF11-GFP and GSTU20-GFP fusion protein expressed in protoplasts of Arabidopsis. Green denotes the GFP signal, and red indicates the chlorophyll signal. (B) GSTF11/U20 expression analysis by qPCR in different tissues. The tissue with the lowest expression was considered the standard and used to quantify the relative expression.
The spatiotemporal expression patterns of GSTF11 and GSTU20 were first investigated by performing quantitative RT-PCR analyses. The results showed that GSTF11 was highly expressed in rosette leaves, moderately expressed in siliques, and weakly expressed in stems, roots and flowers (Figure 2B). Compared with GSTF11, GSTU20 seemed to be expressed in a complementary pattern, which was highly expressed in siliques but weakly expressed in leaves (Figure 2B). The tissue-specific expression patterns of GSTF11 and GSTU20 were also analyzed by generating transgenic Arabidopsis plants carrying GUS (β-glucuronidase) as a reporter gene driven by the native promoter of GSTF11 and GSTU20 for histochemical analysis. Consistent with the RT-PCR results, intense GUS staining of the GSTF11 promoter was observed in leaves and siliques, but GSTU20 promoter activity was mainly detected in siliques (Supplementary Figure 3). Overall, these results indicate that although the subcellular localization is the same, GSTF11 and GSTU20 exhibit distinct tissue- and organ-specific expression patterns in Arabidopsis.
GSTF11 and GSTU20 Deficiencies Substantially Affect Aliphatic Glucosinolate Profiles
To ascertain the biological roles of GSTF11 and GSTU20 in GSL biosynthesis, we knocked out GSTF11 and GSTU20 using the CRISPR/Cas9 technique to obtain two mutant alleles for each gene. The gstf11-1 and gstf11-2 mutants contain an 18- or 41-bp deletion in the third exon of GSTF11, respectively. The deletion in gstf11-1 begin the 486th base result in a frameshift mutation. The mutation in gstf11-2 lead to the presence of a premature stop codon in the third exon (Figure 3A). Two GSTU20 mutations, gstu20-1 and gstu20-2, contain a 23- or 34-bp deletion in the first exon respectively, both of which lead to the presence of a premature stop codon in the first exon (Figure 3A). The gstf11 and gstu20 mutants generated in this study exhibited no obvious morphological phenotypes (Figure 3B), indicating that neither GSTF11 nor GSTU20 is critically required for plant growth and development.
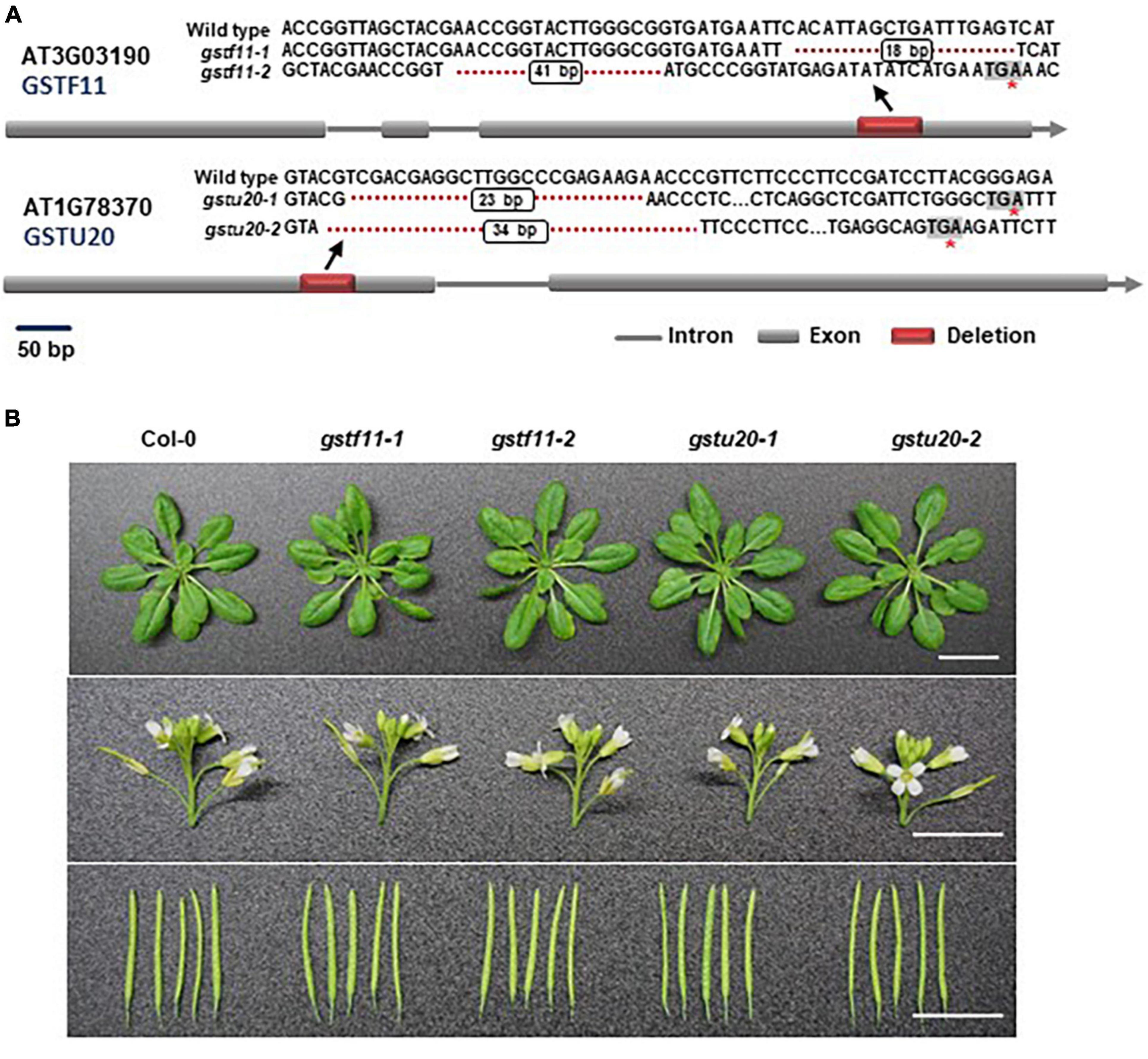
Figure 3. Morphological phenotypes of gstf11 and gstu20 mutants. (A) Diagram of mutations in GSTF11/U20 genes generated by CRISPR-Cas9. Exons are represented by filled boxes, and introns are noted by lines. The red box indicates the deletion region in GSTF11/U20, and the red asterisk indicates a premature stop codon. (B) Rosette leaves, inflorescences and siliques of gstf11 and gstu20 mutants compare to wild type Col-0. Scale bars = 1 cm.
To ascertain the roles of GSTF11 and GSTU20 in GSL biosynthesis, GSL profiles were determined in the mutants described above (Supplementary Figure 4). Compared to wild-type plants, both gstf11 and gstu20 mutants exhibited substantial reduction in almost all categories of aliphatic GSLs with different side chain lengths in both 3-week-old leaves (Figures 4, 5). In contrast, no significant changes in the abundance of indolic GSLs were noted in gstf11 (Figures 4A,B) and gstu20 (Figures 5A,B) mutants, suggesting that GSTF11 and GSTU20 are not required for indolic GSL biosynthesis. For aliphatic GSLs, the formation of 3MSOP (3-methylsulfinylpentyl GSL), 4MTB (4-methylthiobutyl GSL), 7MTH (7-methylsulfinylheptyl GSL), and 8MTO (8-methylthiooctyl GSL) were significantly affected by GSTF11 deficiency (Figure 4C). In the leaves of gstu20 mutant plants, the accumulation of aliphatic GSLs exhibited a striking defect in both mutant alleles, which was more severe in gstu20-2 than gstu20-1 (Figure 5C). Except 5MSOP (5-methylsulphinylpentyl glucosinolate), the accumulation of all types aliphatic GSLs were reduced in gstu20 mutant, and gstu20-2 accumulated less these GSLs than gstu20-1 that is more likely a weak allele (Figure 5C). To sum up, the content of aliphatic GSLs with 3C, 4C, 7C, and 8C side chains were both affected by GSTF11 and GSTU20 mutation, and the accumulation of 6C aliphatic GSL only changed in gstu20 mutant (Supplementary Figure 5A). To test whether GSTF11 and GSTU20 also play synergistic functions in GSL biosynthesis, we constructed gstf11 and gstu20 double mutant (Supplementary Figure 6). Interestingly, compared to the two single mutants, the reduction in aliphatic GSLs was further exaggerated in the double mutant (Figures 6A,C). Like the measurement in single mutants, there is no significant change of indolic GSLs content in gstf11gstu20 double mutant (Figures 6A,B).
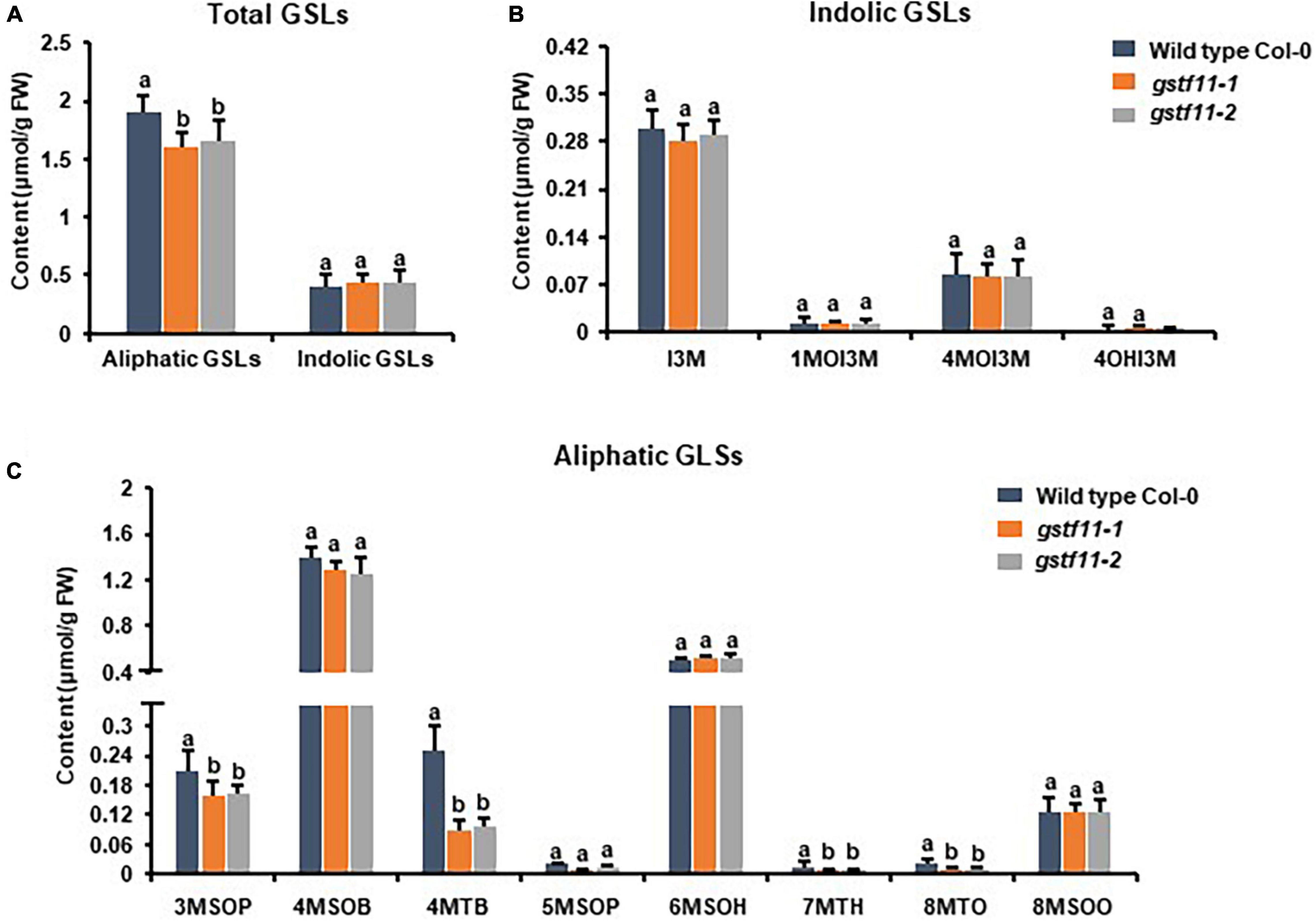
Figure 4. Glucosinolates content in the leaves of gstf11 mutant lines. (A) Total aliphatic and indolic GSLs concentration in leaves from Col-0 and gstf11 mutant lines. (B) Quantification of each component of aliphatic GSLs in gstf11 mutant. (C) Quantification of each component of indolic GSLs in gstf11 mutant. Values were obtained from three biological repeats. Letters indicate significant differences between wild type Col-0 and gstf11 mutant lines as determined by two-way ANOVA (p < 0.05) with Tukey HSD post hoc test.
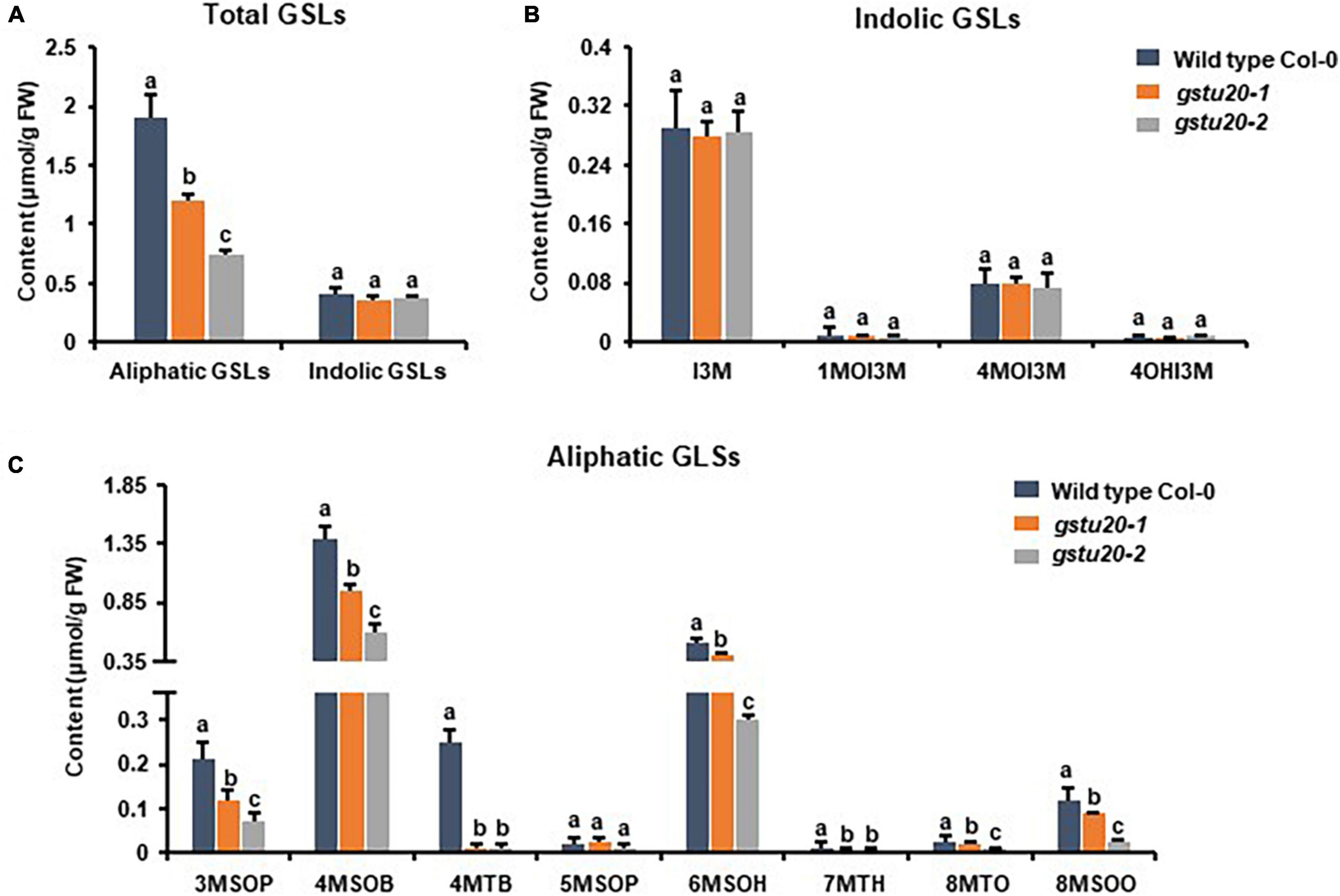
Figure 5. Glucosinolates content in the leaves of gstu20 mutant lines. (A) Total aliphatic and indolic GSLs concentration in leaves from Col-0 and gstu20 mutant lines. (B) Quantification of each component of aliphatic GSLs in gstu20 mutant. (C) Quantification of each component of indolic GSLs in gstu20 mutant. Values were obtained from three biological repeats. Letters indicate significant differences between wild type Col-0 and gstu20 mutant lines as determined by two-way ANOVA (p < 0.05) with Tukey HSD post hoc test.
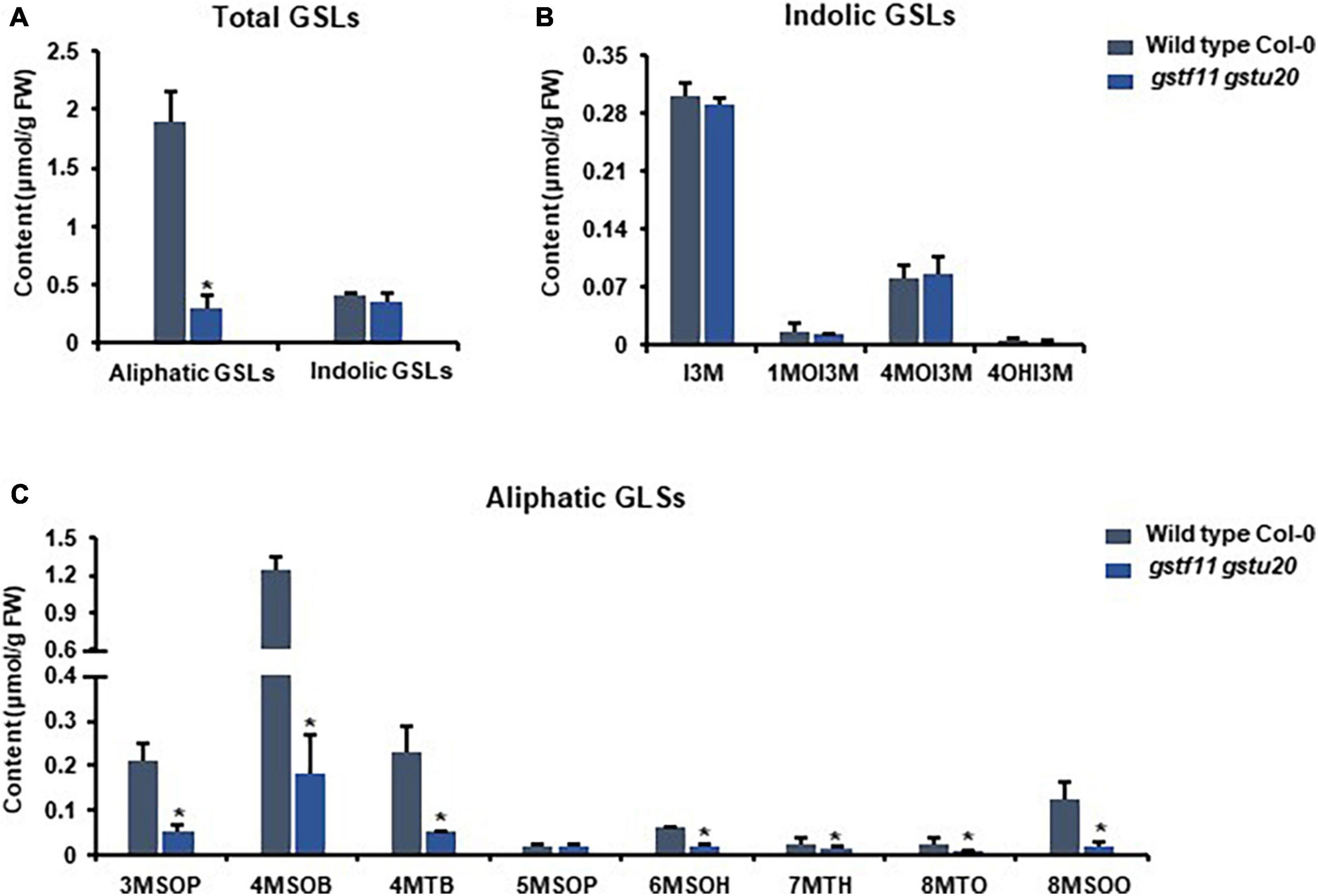
Figure 6. Glucosinolates accumulation in gstf11gstu20 double mutant. (A) Total aliphatic and indolic GSLs concentration in leaves from Col-0 and gstf11gstu20 mutant. (B) Quantification of each component of aliphatic GSLs in Col-0 and gst mutants. (C) Quantification of each component of indolic GSLs in Col-0 and gst mutants. Values were obtained from three biological repeats. Asterisks indicate significant differences between wild type Col-0 and gst mutants as determined by one-way ANOVA (p < 0.05) with Student’s t-test.
To further determine the correlation between the tissue specific-expression pattern of GSTs and GSL biosynthesis, we also detected the GSLs content in mature seeds of gstf11-2 and gstu20-2 mutants. As shown, the mutation of GSTF11 and GSTU20 significantly affected total content of aliphatic GSLs but not indolic GSLs in the seeds, and more aliphatic GSLs were lost in gstf20 (Figures 7A,B). Among aliphatic GSL components, the accumulation of 3OHP (3-hydroxylpropyl GSL), 3BOP (3-benzoylpropyl GSL), 4BOB (4-benzoylbuthyl GSL), 5MTP (5-methylthiopentyl GSL), and 6MTH (6-methylthiohexyl GSL) decreased in gstf11 and gstf20, and most of them were lost more in gstf20, but we found the content of 4MTB and 8MTO in seeds were only affected by GSTU20 mutation (Figure 7C). The results showed that GSTF11 and GSTU20 regulate the accumulation of aliphatic GSLs in both leaves and seeds, which is not directly correlated with the gene expression abundance in the particular tissues (Figure 2B), it is more likely GSTU20 play a primary role in the biosynthesis of aliphatic GSLs no matter the transcript level high or low in seed and leaf. Similar with the accumulation in leaves, the aliphatic GSLs content in gstf11 gstf20 was lower than single mutants (Figure 6C), also showed a synergistic effect between GSTF11 and GSTU20.
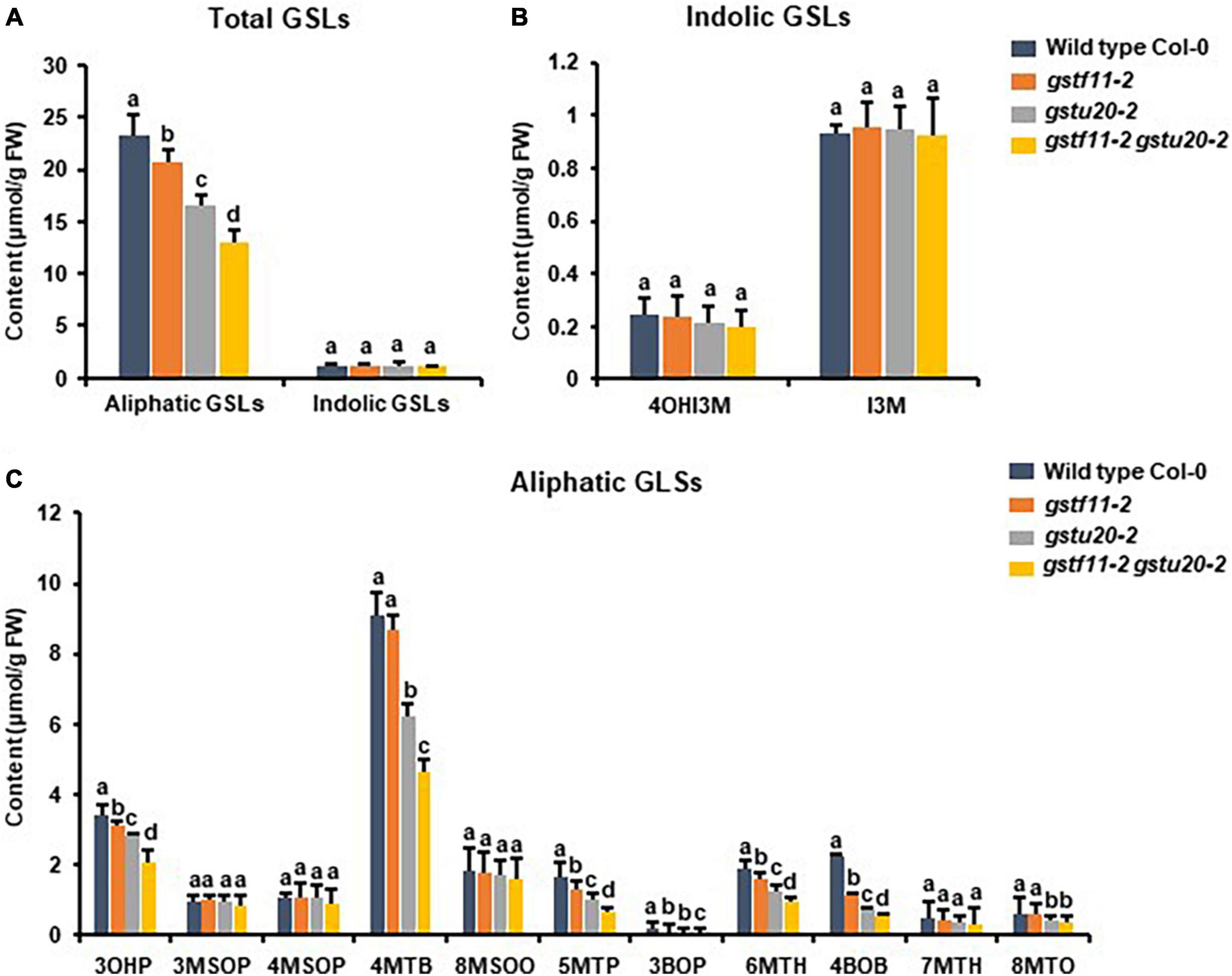
Figure 7. Glucosinolates content in the seeds of gst mutants. (A) Total aliphatic and indolic GSLs concentration in seeds from Col-0 and gst mutants. (B) Quantification of each component of aliphatic GSLs in gst mutants. (C) Quantification of each component of indolic GSLs in gst mutants. Values were obtained from three biological repeats. Letters indicate significant differences between wild type Col-0 and gst mutant lines as determined by two-way ANOVA (p < 0.05) with Tukey HSD post hoc test.
These results indicated that both GSTF11 and GSTU20 are non-redundantly involved in aliphatic GSL biosynthesis, GSTF11 and GSTU20 act on aliphatic GSL biosynthesis in a dosage-dependent manner. Moreover, the reduction in aliphatic GSLs was more severe in gstu20 compared to gstf11 mutant. Three components of aliphatic GSLs like 4MSOB (4-methylsulfinylbutyl glucosinolate), 6MSOH (6-methylsulphinylhexyl glucosinolate) and 8MSOO (8-methylsulphinyloctyl glucosinolate) reduced in gstu20 mutant but maintained wild type level in gsts11 leaves (Figures 4, 5), indicating that GSTU20 plays a greater role in GSL biosynthesis than GSTF11.
GSTF11 and GSTU20 Deficiencies Caused Partially Overlapping Transcriptome Alterations
To understand molecular changes in response to the perturbation of GSTF11 and GSTU20, RNA-seq analysis was performed to examine the transcript profiles in both gstf11-2 and gstu20-2 mutant leaves with three biological replicates (Supplementary Table 3). By applying a false discovery rate (FDR) ≤ 0.05 and fold change ≥ 2, a total of 463 differentially expressed genes (DEGs) were identified, including 298 up- and 165 downregulated genes in gstf11 compared to wild-type plants (Figure 8A and Supplementary Table 4). In gstu20, 1,232 genes were identified as DEGs, including 567 up- and 665 downregulated genes (Figure 8B and Supplementary Table 5). The larger number of DEGs observed in the gstu20 mutant compared with the gstf11 mutant suggests that defective GSTU20 causes a greater extent of cellular response relative to GSTF11. These DEGs were enriched in various biological terms shown in Supplementary Figures 7, 8, the metabolic processes related to plant growth and development, stress response were also enriched, suggesting that GST mutation results in perturbation of multiple metabolic processes. More importantly, a large proportion of DEGs overlapped in the gstf11 and gstu20 mutants, accounting for 62% (n = 298) and 63% (n = 165) of the up- and downregulated DEGs in gstf11, respectively (Figure 8C).
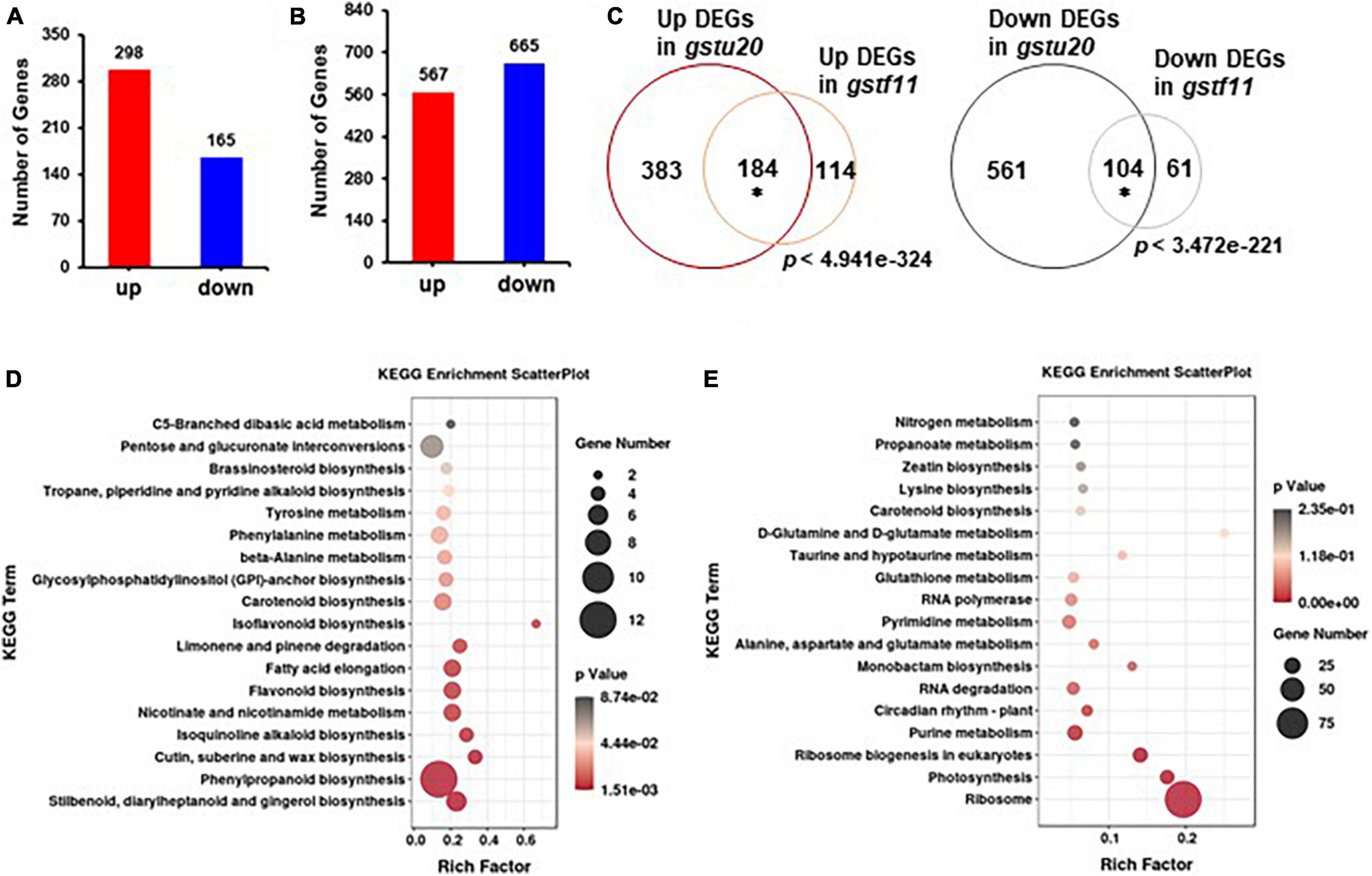
Figure 8. Gene expression profiles identified in gst mutant lines. The number of differentially expressed genes (DEGs) identified in gstf11 (A) and gstu20 (B) mutants. (C) Overlap of up- or downregulated genes between the gstf11 and gstu20 mutant. Venn diagrams were drawn in BioVenn (http://www.biovenn.nl/). Asterisks indicate the statistical significance (p < 0.01) of overlap, which was calculated using the online tool at http://nemates.org/MA/progs/overlap_stats.html. KEGG pathways were enriched for (D) up- and (E) downregulated DEGs expressed in the gstu20 mutant. A plot diagram was drawn by ggplot2 (https://rdocumentation.org/packages/ggplot2/versions/2.1.0). The Rich factor indicates the number of identified DEGs versus the total genes involved in the metabolic pathways and describes the significance of pathway enrichment. The dot size represents the number of identified DEGs in each pathway and p value was indicated by a color bar.
As the majority of DEGs in gstf11 were included in the gstu20 mutant, we performed Gene Ontology (GO) enrichment analysis (Mi et al., 2019) using DEGs in gstf20 as the representative mutant. The results showed that the upregulated DEGs were mainly enriched in response to stimulus, transcription factor activity, and metabolic processes (Supplementary Figure 7A and Supplementary Table 5). In contrast, the downregulated DEGs were primarily enriched in translation and rRNA processing (Supplementary Figure 7B and Supplementary Table 5). Moreover, KEGG pathway analysis (Kanehisa et al., 2016) revealed that upregulated DEGs were highly enriched in multiple primary and secondary metabolic processes (Figure 8D). Surprisingly, the downregulated DEGs were principally enriched in ribosome and ribosome biogenesis (Figure 8E). These results suggest that the perturbation of GSTF11 and GSTU20 caused a wide range of cellular alterations likely result from the disrupted GSL biosynthesis.
Discussion
The formation of the GSL core structure requires an intermediate with a GSH conjugate serving as a sulfur supply. The enzymatic activity of GST family proteins in conjugating GSH into substantial metabolic intermediates has enabled researchers to postulate that some GST proteins are involved in GSL biosynthesis. Indeed, GSTF9, GSTF10, and GSTU13 have been recently identified to participate in indolic GSL biosynthesis (Piślewska-Bednarek et al., 2018). In contrast, which GSTs undertake a function in aliphatic GSL biosynthesis is merely conceptual and simply based on in silico transcriptional co-expression analysis (Wentzell et al., 2007; Bednarek et al., 2009; Geu-Flores et al., 2011; Klein and Sattely, 2017). In this study, we provide evidence in planta demonstrating that both of GSTF11 and GSTU20 are involved in aliphatic GSL biosynthesis with two unanticipated but intriguing features.
GSTU20 Plays a Greater Role in Aliphatic Glucosinolate Biosynthesis Than GSTF11
The greater loss of aliphatic GSLs in the loss-of-function of gstu20 mutants compared to gstf11 mutants in both leaves and seeds supports the notion that GSTU20 plays a more important role than GSTF11 in aliphatic GSL biosynthesis. This conclusion is further corroborated by the finding that more severe alteration of the transcriptome profile occurs in gstu20 mutant compared with gstf11 mutant. However, based on tissue-specific patterns, GSTFU20 was expressed at a lower level relative to GSTF11, which seems contradictory to its superior function in comparison to GSTF11. Three possibilities might explain this phenomenon. First, the deletion in gstf11 mutants happened at the last exon of GSTF11, which may generate a truncated protein and still remain function in GSL biosynthesis. Second, similar to other enzymes, the in vivo catalytic activity of GSTFU20 and GSTF11 is derived from proteins, the cellular content of which is determined by multiple steps of gene expression regulation, including transcription and posttranscription. In this context, the low level of GSTFU20 transcripts may be accompanied by a high extent of translational efficiency, leading to an increase in protein abundance. The main alternative possibility is that the enzymatic activity of distinct member of GSTs is variable and influenced by protein structure in planta. GSTU20 has been assayed for activity toward model xenobiotic substrate CDNB and BITC which are the typical GST substrates, revealing high GSH-conjugating activity (Gil and MacLeod, 1980; Edwards and Dixon, 2005; Dixon et al., 2009). The enzymatic activity of GSTF11 was undetectable because of the protein could not be isolated and purified in vitro due to the rare abundance. The metabolic engineering in tobacco and yeast indicated that the expression of GSTF11 is not essential for GSL production, even though it could increase GSL accumulation level (Mikkelsen et al., 2010, 2012). In this scenario, GSTU20 may have higher degree of GSH-conjugating activity than GSTF11. Regardless of which possibility is true, the levels of protein and its derived enzymatic activity should be validated in future studies.
GSTF11 and GSTU20 Function in Aliphatic Glucosinolates Biosynthesis in a Dosage-Dependent Manner
In both the gstf11 and gftu20 mutants, the total abundance of aliphatic GSLs decreased, indicating that GSTF11 and GSTU20 are not redundant to each other. In addition, the altered pattern in terms of GSLs with different side chains was similar between the gstf11 and gftu20 mutants, suggesting that GSTF11 and GSTU20 functionally overlap. This overlap is further reflected by the fact that a large number of DEGs also overlapped in the gstf11 and gftu20 mutants. These characteristics of non-redundance and overlap seem mutually contradictory. However, this finding could be simply explained if we consider that both GSTF11 and GSTU20 work in aliphatic GSL biosynthesis in a dosage-dependent manner, and the loss of GSTF11 or GSTU20 could result in reduced GST activity required for the GSH-conjugation step. This assumption is further corroborated by the aggregate loss of aliphatic GSLs in the gstf11 gftu20 double mutants. In addition, it is worth mentioning that the double mutation of GSTF11 and GSTU20 caused a dramatic decrease but did not completely abolish the formation of aliphatic GSLs, implying the existence of other isoforms like another unknown GST members that additionally functions in aliphatic GSL biosynthesis. Moreover, both GSTF11 and GSTU20 showed tissue-specific expression patterns but the content of GSLs was not intimately connected with the transcript abundance, enhancing the prospect that other GST family proteins also work on GSL biosynthesis in different tissues besides GSTF11 and GSTU20, maybe these GST members make distinct contribution on the accumulation of GSLs in the tissues including leaf, stem, root, flower and seed, which deserves further investigation in the future.
Data Availability Statement
The data presented in the study are deposited in the DRYAD repository, accession number https://doi.org/10.5061/dryad.nvx0k6dtk.
Author Contributions
QP and XY proposed the project and designed the experiments. AZ, RL, JL, RM, and HA performed the experiments and analyzed the data. AZ and QP prepared the manuscript with contributions from other authors. All authors contributed to the article and approved the submitted version.
Funding
This work was supported by the National Natural Science Foundation of China (32070350) to QP. AZ was supported by Fundamental Research Funds for the Central Universities (2572019BS01) and China Postdoctoral Science Foundation (2019M661235).
Conflict of Interest
The authors declare that the research was conducted in the absence of any commercial or financial relationships that could be construed as a potential conflict of interest.
Publisher’s Note
All claims expressed in this article are solely those of the authors and do not necessarily represent those of their affiliated organizations, or those of the publisher, the editors and the reviewers. Any product that may be evaluated in this article, or claim that may be made by its manufacturer, is not guaranteed or endorsed by the publisher.
Acknowledgments
We appreciate the assistance of all lab members in this study.
Supplementary Material
The Supplementary Material for this article can be found online at: https://www.frontiersin.org/articles/10.3389/fpls.2021.816233/full#supplementary-material
Footnotes
- ^ https://www.arabidopsis.org/index.jsp
- ^ https://atted.jp/
- ^ https://string-db.org
- ^ https://atted.jp/top_draw/#CoexViewer
References
Agerbirk, N., and Olsen, C. E. (2012). Glucosinolate structures in evolution. Phytochemistry 77, 16–45. doi: 10.1016/j.phytochem.2012.02.005
Alvarez, S., He, Y., and Chen, S. X. (2008). Comparative investigations of the glucosinolate-myrosinase system in Arabidopsis suspension cells and hypocotyls. Plant Cell Physiol. 49, 324–333. doi: 10.1093/pcp/pcn007
Bak, S., and Feyereisen, R. (2001). The involvement of two p450 enzymes, CYP83B1 and CYP83A1, in auxin homeostasis and glucosinolate biosynthesis. Plant Physiol. 127, 108–118. doi: 10.1104/pp.127.1.108
Bednarek, P., Pislewska-Bednarek, M., Svatos, A., Schneider, B., Doubsky, J., Mansurova, M., et al. (2009). A glucosinolate metabolism pathway in living plant cells mediates broad-spectrum antifungal defense. Science 323, 101–106. doi: 10.1126/science.1163732
Blažević, I., Montaut, S., Burčul, F., Olsen, C. E., Burow, M., Rollin, P., et al. (2020). Glucosinolate structural diversity, identification, chemical synthesis and metabolism in plants. Phytochemistry 169:112100. doi: 10.1016/j.phytochem.2019.112100
Chen, S. X., Glawischnig, E., Jørgensen, K., Naur, P., Jørgensen, B., Olsen, C. E., et al. (2003). CYP79F1 and CYP79F2 have distinct functions in the biosynthesis of aliphatic glucosinolates in Arabidopsis. Plant J. 33, 923–937. doi: 10.1046/j.1365-313x.2003.01679.x
Czerniawski, P., and Bednarek, P. (2018). Glutathione S-Transferases in the biosynthesis of sulfur-containing secondary metabolites in Brassicaceae Plants. Front. Plant Sci. 9:1639. doi: 10.3389/fpls.2018.01639
Dixon, D. P., Hawkins, T., Hussey, P. J., and Edwards, R. (2009). Enzyme activities and subcellular localization of members of the Arabidopsis glutathione transferase superfamily. J. Exp. Bot. 60, 1207–1218. doi: 10.1093/jxb/ern365
Edwards, R., and Dixon, D. P. (2005). Plant glutathione transferases. Methods Enzymol. 401, 169–186. doi: 10.1016/S0076-6879(05)01011-6
Fahey, J. W., Zalcmann, A. T., and Talalay, P. (2001). The chemical diversity and distribution of glucosinolates and isothiocyanates among plants. Phytochemistry 56, 5–51. doi: 10.1016/s0031-9422(00)00316-2
Geu-Flores, F., Møldrup, M. E., Böttcher, C., Olsen, C. E., Scheel, D., and Halkier, B. A. (2011). Cytosolic γ-glutamyl peptidases process glutathione conjugates in the biosynthesis of glucosinolates and camalexin in Arabidopsis. Plant Cell 23, 2456–2469. doi: 10.1105/tpc.111.083998
Gil, V., and MacLeod, A. J. (1980). Benzylglucosinolate degradation in Lepidium sativum: effects of plant age and time of autolysis. Phytochemistry 19, 1365–1368. doi: 10.1016/0031-9422(80)80175-0
Grubb, C. D., and Abel, S. (2006). Glucosinolate metabolism and its control. Trends Plant Sci. 11, 89–100. doi: 10.1016/j.tplants.2005.12.006
Grubb, C. D., Zipp, B. J., Ludwig-Müller, J., Masuno, M. N., Molinski, T. F., and Abel, S. (2004). Arabidopsis glucosyltransferase UGT74B1 functions in glucosinolate biosynthesis and auxin homeostasis. Plant J. 40, 893–908. doi: 10.1111/j.1365-313X.2004.02261.x
Halkier, B. A., and Gershenzon, J. (2006). Biology and biochemistry of glucosinolates. Annu. Rev. Plant Biol. 57, 303–333. doi: 10.1146/annurev.arplant.57.032905.105228
Harun, S., Abdullah-Zawawi, M. R., Goh, H. H., and Mohamed-Hussein, Z. A. (2020). A comprehensive gene inventory for glucosinolate biosynthetic pathway in Arabidopsis thaliana. J. Agric. Food Chem. 68, 7281–7297. doi: 10.1021/acs.jafc.0c0191
He, Y., Galant, A., Pang, Q. Y., Strul, J. M., Balogun, S. F., Jez, J. M., et al. (2011). Structural and functional evolution of isopropylmalate dehydrogenases in the leucine and glucosinolate pathways of Arabidopsis thaliana. J. Biol. Chem. 286, 28794–28801. doi: 10.1074/jbc.M111.262519
He, Y., Mawhinney, T. P., Preuss, M. L., Schroeder, A. C., Chen, B., Abraham, L., et al. (2009). A redox-active isopropylmalate dehydrogenase functions in the biosynthesis of glucosinolates and leucine in Arabidopsis. Plant J. 60, 679–690. doi: 10.1111/j.1365-313X.2009.03990.x
Hirai, M. Y. (2009). A robust omics-based approach for the identification of glucosinolate biosynthetic genes. Phytochem. Rev. 8, 15–23. doi: 10.1007/s11101-008-9114-4
Hirai, M. Y., Klein, M., Fujikawa, Y., Yano, M., Goodenowe, D. B., Yamazaki, Y., et al. (2005). Elucidation of gene-to-gene and metabolite-to-gene networks in Arabidopsis by integration of metabolomics and transcriptomics. J. Biol. Chem. 280, 25590–25595. doi: 10.1074/jbc.M502332200
Hirai, M. Y., Sugiyama, K., Sawada, Y., Tohge, T., Obayashi, T., Suzuki, A., et al. (2007). Omics-based identification of Arabidopsis Myb transcription factors regulating aliphatic glucosinolate biosynthesis. Proc. Natl. Acad. Sci. U.S.A. 104, 6478–6483. doi: 10.1073/pnas.0611629104
Jung, H. I., Yan, J., Zhai, Z., and Vatamaniuk, O. K. (2015). Gene functional analysis using protoplast transient assays. Methods Mol. Biol. 1284, 433–452. doi: 10.1007/978-1-4939-2444-8_22
Kanehisa, M., Sato, Y., Kawashima, M., Furumichi, M., and Tanabe, M. (2016). KEGG as a reference resource for gene and protein annotation. Nucleic Acids Res. 44, 457–462. doi: 10.1093/nar/gkv1070
Klein, A. P., and Sattely, E. S. (2017). Biosynthesis of cabbage phytoalexins from indole glucosinolate. Proc. Natl. Acad. Sci. U.S.A. 114, 1910–1915. doi: 10.1073/pnas.1615625114
Kliebenstein, D. J., Lambrix, V. M., Reichelt, M., Gershenzon, J., and Mitchell-Olds, T. (2001). Gene duplication in the diversification of secondary metabolism, tandem 2-oxoglutarate-dependent dioxygenases control glucosinolate biosynthesis in Arabidopsis. Plant Cell 13, 681–693. doi: 10.1105/tpc.13.3.681
Kroymann, J. (2011). Natural diversity and adaptation in plant secondary metabolism. Curr. Opin. Plant Biol. 14, 246–251. doi: 10.1016/j.pbi.2011.03.021
Kroymann, J., Textor, S., Tokuhisa, J. G., Falk, K. L., Bartram, S., Gershenzon, J., et al. (2001). A gene controlling variation in Arabidopsis glucosinolate composition is part of the methionine chain elongation pathway. Plant Physiol. 127, 1077–1088.
Labrou, N. E., Papageorgiou, A. C., Pavli, O., and Flemetakis, E. (2015). Plant GSTome, structure and functional role in xenome network and plant stress response. Curr. Opin. Biotechnol. 32, 186–194. doi: 10.1016/j.copbio.2014.12.024
Mi, H., Muruganujan, A., Ebert, D., Huang, X., and Thomas, P. D. (2019). PANTHER version 14: more genomes, a new PANTHER GO-slim and improvements in enrichment analysis tools. Nucleic Acids Res. 47, 419–426. doi: 10.1093/nar/gky1038
Mikkelsen, M. D., Buron, L. D., Salomonsen, B., Olsen, C. E., Hansen, B. G., Mortensen, U. H., et al. (2012). Microbial production of indolylglucosinolate through engineering of a multi-gene pathway in a versatile yeast expression platform. Metab. Eng. 14, 104–111. doi: 10.1016/j.ymben.2012.01.006
Mikkelsen, M. D., Naur, P., and Halkier, B. A. (2004). Arabidopsis mutants in the C-S lyase of glucosinolate biosynthesis establish a critical role for indole-3-acetaldoxime in auxin homeostasis. Plant J. 37, 770–777. doi: 10.1111/j.1365-313x.2004.02002.x
Mikkelsen, M. D., Olsen, C. E., and Halkier, B. A. (2010). Production of the cancer-preventive glucoraphanin in tobacco. Mol. Plant 3, 751–759. doi: 10.1093/mp/ssq020
Nguyen, V. P. T., Stewart, J., Lopez, M., Ioannou, I., and Allais, F. (2020). Glucosinolates: natural occurrence, biosynthesis, accessibility, isolation, structures, and biological activities. Molecules 25:4537. doi: 10.3390/molecules25194537
Obayashi, T., Aoki, Y., Tadaka, S., Kagaya, Y., and Kinoshita, K. (2018). ATTED-II in 2018: a plant coexpression database based on investigation of the statistical property of the mutual rank index. Plant Cell Physiol. 59:e3. doi: 10.1093/pcp/pcx191
Petersen, A., Wang, C., Crocoll, C., and Halkier, B. A. (2018). Biotechnological approaches in glucosinolate production. J. Integr. Plant Biol. 60, 1231–1248. doi: 10.1111/jipb.12705
Piotrowski, M., Schemenewitz, A., Lopukhina, A., Müller, A., Janowitz, T., Weiler, E. W., et al. (2004). Desulfoglucosinolate sulfotransferases from Arabidopsis thaliana catalyze the final step in the biosynthesis of the glucosinolate core structure. J. Biol. Chem. 279, 50717–50725. doi: 10.1074/jbc.M407681200
Piślewska-Bednarek, M., Nakano, R. T., Hiruma, K., Pastorczyk, M., Sanchez-Vallet, A., Singkaravanit-Ogawa, S., et al. (2018). Glutathione Transferase U13 functions in pathogen-triggered glucosinolate metabolism. Plant Physiol. 176, 538–551. doi: 10.1104/pp.17.01455
Schuster, J., Knill, T., Reichelt, M., Gershenzon, J., and Binder, S. (2006). Branched-chain aminotransferase4 is part of the chain elongation pathway in the biosynthesis of methionine-derived glucosinolates in Arabidopsis. Plant Cell 18, 2664–2679. doi: 10.1105/tpc.105.039339
Sønderby, I. E., Geu-Flores, F., and Halkier, B. A. (2010). Biosynthesis of glucosinolates–gene discovery and beyond. Trends Plant Sci. 15, 283–290. doi: 10.1016/j.tplants.2010.02.005
Szklarczyk, D., Gable, A. L., Lyon, D., Junge, A., Wyder, S., Huerta-Cepas, J., et al. (2019). STRING v11: protein-protein association networks with increased coverage, supporting functional discovery in genome-wide experimental datasets. Nucleic Acids Res. 47, 607–613. doi: 10.1093/nar/gky1131
Textor, S., de Kraker, J. W., Hause, B., Gershenzon, J., and Tokuhisa, J. G. (2007). MAM3 catalyzes the formation of all aliphatic glucosinolate chain lengths in Arabidopsis. Plant Physiol. 144, 60–71. doi: 10.1104/pp.106.091579
Tzfira, T., Tian, G. W., Lacroix, B., Vyas, S., Li, J., Leitner-Dagan, Y., et al. (2005). pSAT vectors, a modular series of plasmids for autofluorescent protein tagging and expression of multiple genes in plants. Plant Mol. Biol. 57, 503–516. doi: 10.1007/s11103-005-0340-5
Wagner, U., Edwards, R., Dixon, D. P., and Mauch, F. (2002). Probing the diversity of the Arabidopsis glutathione S-transferase gene family. Plant Mol. Biol. 49, 515–532. doi: 10.1023/a:1015557300450
Wang, Z. P., Xing, H. L., Dong, L., Zhang, H. Y., Han, C. Y., Wang, X. C., et al. (2015). Egg cell-specific promoter-controlled CRISPR/Cas9 efficiently generates homozygous mutants for multiple target genes in Arabidopsis in a single generation. Genome Biol. 16:144. doi: 10.1186/s13059-015-0715-0
Wentzell, A. M., Rowe, H. C., Hansen, B. G., Ticconi, C., Halkier, B. A., and Kliebenstein, D. J. (2007). Linking metabolic QTLs with network and cis-eQTLs controlling biosynthetic pathways. PLoS Genet. 3:e0030162. doi: 10.1371/journal.pgen.0030162
Xie, K., Zhang, J., and Yang, Y. (2014). Genome-wide prediction of highly specific guide RNA spacers for CRISPR-Cas9-mediated genome editing in model plants and major crops. Mol. Plant 7, 923–926. doi: 10.1093/mp/ssu009
Keywords: Arabidopsis, aliphatic glucosinolate, glutathione S-transferase, GSTF11, GSTU20
Citation: Zhang A, Luo R, Li J, Miao R, An H, Yan X and Pang Q (2022) Arabidopsis Glutathione-S-Transferases GSTF11 and GSTU20 Function in Aliphatic Glucosinolate Biosynthesis. Front. Plant Sci. 12:816233. doi: 10.3389/fpls.2021.816233
Received: 16 November 2021; Accepted: 22 December 2021;
Published: 25 January 2022.
Edited by:
Pan Liao, Purdue University, United StatesReviewed by:
Jeongim Kim, University of Florida, United StatesSarma Rajeev Kumar, String Bio Pvt Ltd., India
Copyright © 2022 Zhang, Luo, Li, Miao, An, Yan and Pang. This is an open-access article distributed under the terms of the Creative Commons Attribution License (CC BY). The use, distribution or reproduction in other forums is permitted, provided the original author(s) and the copyright owner(s) are credited and that the original publication in this journal is cited, in accordance with accepted academic practice. No use, distribution or reproduction is permitted which does not comply with these terms.
*Correspondence: Qiuying Pang, cWl1eWluZ0BuZWZ1LmVkdS5jbg==; Xiufeng Yan, eWFueGl1ZmVuZ0B3enUuZWR1LmNu
†These authors have contributed equally to this work