- State Key Laboratory of Pharmaceutical Biotechnology, School of Life Sciences, Nanjing University, Nanjing, China
The histone acetyltransferase (HAT) general control non-repressed protein 5 (GCN5) plays important roles in plant development via epigenetic regulation of its target genes. However, the role of GCN5 in tomato, especially in the regulation of tomato shoot meristem and flower development, has not been well-understood. In this study, we found that silencing of Solanum lycopersicum GCN5 (SlGCN5, Solyc10g045400.1.1) by virus-induced gene silencing (VIGS) and RNA interference (RNAi) resulted in the loss of shoot apical dominance, reduced shoot apical meristem (SAM) size, and dwarf and bushy plant phenotype. Besides, we occasionally observed extra carpelloid stamens and carpels fused with stamens at the late stages of flower development. Through gene expression analysis, we noticed that SlGCN5 could enhance SlWUS transcript levels in both SAM and floral meristem (FM). Similar to the known function of GCN5 in Arabidopsis, we demonstrated that SIGCN5 may form a HAT unit with S. lycopersicum alteration/deficiency in activation 2a (SlADA2a) and SlADA2b proteins in tomato. Therefore, our results provide insights in the SlGCN5-mediated regulation of SAM maintenance and floral development in tomato.
Introduction
Plants have a unique ability to give rise to new organs continuously due to the indeterminate production of undifferentiated stem cells located in specific regions of meristems. The shoot apical meristem (SAM) gives rise to the aerial organs, and the maintenance of SAM is key for the development of plants and adaptation to the changes of external environment (Pfeiffer et al., 2017). Unlike Arabidopsis, tomato is a typical sympodial plant. After the formation of 8–10 leaves, tomato SAM terminated and transforms into inflorescence meristem (IM) and sympodial meristem (SYM), which are formed at the leaf axils beneath the IM to sustain continuous growth. Thereafter, IM transforms to floral meristem (FM) and initiates a second IM in the meantime (Schmitz and Theres, 1999; Périlleux et al., 2014). Tomato FMs generate four whorls of floral organs, namely, sepals, petals, stamens, and carpels, sequentially in concentric whorls (Sekhar and Sawhney, 1984).
In Arabidopsis, the maintenance of the stem cell pool in the SAM is regulated by CLAVATA-WUSCHEL (CLV-WUS) feedback loop (Schoof et al., 2000). In this feedback loop, WUS could directly induce stem cell identity and the expression of the stem cell marker gene CLV3 (Yadav et al., 2011; Daum et al., 2014). The CLV genes including CLV1 and CLV3 repress WUS through signaling cascades (Shang et al., 2019; Han et al., 2020), therefore coordinating and balancing stem cell proliferation with differentiation. The CLV-WUS feedback loop appears to be highly conserved across different plant species (Somssich et al., 2016). In tomato, the mutation of SlCLV3 promotes stem cell overproliferation and results in extra floral organs and bigger fruits (Rodríguez-Leal et al., 2017). In SlWUS RNA interference (RNAi) lines, plants have reduced flower size and fruit locule numbers (Li et al., 2017). Changes in tomato meristem size have also been observed in fasciated (fas) and locule number (lc) mutants, both of which have misexpression of SlWUS and SlCLV3, respectively (Muños et al., 2011; Xu et al., 2015; Chu et al., 2019).
In various plant species, studies have discovered that the CLV-WUS regulatory loop could be modified by many additional factors, which can contribute to plant growth and productivity (Galli and Gallavotti, 2016). Among these factors, histone modifications including acetylation or methylation on several lysine residues of H3 are important for gene expression during plant development (Servet et al., 2010). Histone acetyltransferases (HATs) can catalyze acetylation of specific lysine residues on histone N-tails and leads to transcriptional regulation (Bannister and Kouzarides, 2011). It has been reported that in most cases, GCN5 acts as the catalytic core of the HAT complex, which also include vital adaptor proteins ADA2a and ADA2b (Shahbazian and Grunstein, 2007). GCN5 acetylates lysine 14 of histone H3 (H3K14ac) and influences H3K9ac and H3K27ac levels in promoter region of its targets (Benhamed et al., 2006; Servet et al., 2010; Ruggieri et al., 2020). In contrast, ADA2 proteins could help increase the HAT activity of GCN5 (Mao et al., 2006).
In Arabidopsis, both GCN5 and ADA2b are required for many developmental processes such as shoot apical dominance, root meristem activity, leaf development, IM or FM function, and flower fertility (Bertrand et al., 2003; Vlachonasios et al., 2003; Cohen et al., 2009; Kornet and Scheres, 2009; Anzola et al., 2010; Servet et al., 2010). In poplar trees, ABRE-motif binding protein PtrAREB1-2 binds to PtrNAC genes, recruits the HAT unit ADA2b-GCN5 by forming a AREB1-ADA2b-GCN5 protein complexes, and results in increased H3K9 acetylation levels on PtrNAC genes (Li et al., 2019). In rice, the homeodomain protein OsWOX11 recruits a HAT complex containing OsGCN5 to establish the programs of cell proliferation in crown root meristem (Zhou et al., 2017). One study implies that the SAGA (Spt-Ada-GCN5 acetyltransferase) complex is an evolutionarily conserved complex that has a critical role in various developmental processes (Spedale et al., 2012).
In this work, we identified SlGCN5, SIADA2a, and SlADA2b in tomato and found that SlGCN5 can form a HAT unit with SlADA2a and SlADA2b and influences H3K9ac, H3K14ac, and H3ac at the genomic level. Silencing of SlGCN5 resulted in dwarf plant phenotype, reduced SAM size, carpelloid stamens, and fusion of carpels with stamens in flowers. Furthermore, we proposed that SlGCN5 could enhance SlWUS expression, thereby maintaining stem cell homeostasis in tomato.
Materials and Methods
Plant Materials and Growth Conditions
Arabidopsis plants and wild-type (WT) tomato (Solanum lycopersicum) plants of Micro-Tom (MT) and transgenic Arabidopsis and tomato lines were grown in the greenhouse, under long-day condition (16-h light/8-h dark). For transformation, tomato cotyledons were cultivated in vitro in MS medium in a growth chamber (Panasonic, MLR-352H-PC) at 22°C/20°C under16-h light and 8-h dark conditions.
Construction of TRV-SlGCN5 and RNAi Vectors and Tomato Transformation
The tobacco rattle virus (TRV)-based vectors, i.e., pTRV1 and pTRV2, were used for virus-induced gene silencing (VIGS). To construct a pTRV2-SlGCN5 vector, according to the website1, a 400-bp DNA fragment of the SlGCN5 CDS was amplified from tomato cDNA using primers in Supplementary Table 1. The constructs were introduced into Agrobacterium tumefaciens GV301. Then, VIGS assays were carried out as previously described (Fu et al., 2005).
To generate amiRNA for silencing SlGCN5, the amiRNAs (21-nt) were designed by using the web MicroRNA Designer (WMD32). Pre-amiRNA was assembled by several rounds of PCR using primers listed in Supplementary Table 1. The final PCR fragments were driven under 35S promoters in pCHF3 vector. After SlGCN5-RNAi construct is transformed into Agrobacterium GV3101, the Agrobacterium-mediated transformation of tomato cotyledons was performed as described (Cortina and Culiáñez-Macià, 2004; Tripodi, 2020).
Phylogenetic Analysis
For phylogenetic analysis, the coding sequences of ADA2 orthologs were retrieved from JGI Genome Portal and Resources for Plant Comparative Genomics3 by BLAST using AtADA2a coding sequence as a query with default parameters. The phylogenetic tree of ADA2 orthologs in dicots was constructed by W-IQ-TREE (Nguyen et al., 2015), which identified the best evolutionary model as the general time reversible model (GTR + F + I + G4). The non-parametric UltraFast Bootstrap (UFBoot) method (Minh et al., 2013) was used to calculate the node support, and 1,000 bootstrap pseudo replicates were performed with bootstrap values indicated in branches.
Subcellular Localization Analysis
DNA fragment of SlGCN5 was amplified by PCR (primers are listed in Supplementary Table 1) and inserted into pGreenII vector to generate the SlGCN5-GFP (green fluorescent protein) fusion protein. Then, pGreenII vector-based 35S:SlGCN5-GFP and the control vector pGreenII-based 35S:GFP were transformed into A. tumefaciens strain GV3101 and injected into 4-week-old tobacco leaves. GFP fluorescence was observed using Olympus (BX53) microscope after 72 h of infiltration.
RNA Extraction and Expression Analyses
RNA extraction and quantitative real-time (qRT)-PCR analysis were carried out as described previously (Sun et al., 2019). ACTIN2 and SlACTIN2 were served as the internal control in Arabidopsis and tomato, respectively. The sequences of all primers are listed in Supplementary Table 1.
In situ Hybridization
RNA in situ hybridization was performed as described previously (Sun et al., 2019). Briefly, SlGCN5 (Solyc10g045400.1.1) and SlWUS (Solyc02g083950) probes were synthesized from cDNA by using the primers listed in Supplementary Table 1, and the PCR products were cloned into pGEM-T Easy vector (TIANGEN, VT307). After linearization, the DIG RNA labeling kit (Roche, 11175025910) was used for in vitro transcription of probes. The experiments were performed twice using two different batches of plants. Photographs were taken by using an Olympus BX53 microscope.
Yeast Two-Hybrid Assay
To obtain yeast two-hybrid vectors, the full-length SlGCN5 was cloned into pGADT7 (Clontech). The full-length SlADA2a and SlADA2b were individually cloned into pGBKT7 (Clontech). The yeast two-hybrid assay was performed using the Yeastmaker Yeast Transformation System 2 (Clontech, T2001) according to the instruction of the manufacturer. Primer sequences are provided in Supplementary Table 1.
Bimolecular Fluorescence Complementation Assay
For bimolecular fluorescence complementation (BiFC) assay, SlGCN5 and SlADA2 were tagged with the C-terminal part of YFP (YFPC) and the N-terminal part of YFP (YFPN), respectively, as previously described (Kudla and Bock, 2016). Cloning primers are listed in Supplementary Table 1. After vectors were transformed into Agrobacterium, the Agrobacterium carrying different vectors were co-infiltrated into tobacco (Nicotiana benthamiana) leaves of 4-week-old plants as described previously (Sparkes et al., 2006). The infected tobacco leaves were cultured for 72 h before observation. Notably, 5 μg/ml DAPI was used to visualize the nuclei. The fluorescence was observed by using Olympus (BX53) microscope.
Statistical Analysis
The statistical analysis was conducted using two-tailed t-test. The statistically significant differences are indicated by *p < 0.05, **p < 0.01, or ***p < 0.001.
Results
Silencing of SlGCN5 Affects Tomato Plant Development
GCN5 was reported to participate in many biological processes in Arabidopsis, especially in plant development (Vlachonasios et al., 2003). In this study, we aimed to investigate the function of GCN5 in tomato development. For this purpose, we first searched for putative homologs of AtGCN5 in tomato genome sequence, and only one homologous gene with three isoforms was identified (Supplementary Figure 1). Among these three isoforms, we chose the one with the highest expression level in tomato inflorescences and the highest protein similarity with AtGCN5 for further study. To explore the effect of SlGCN5 silencing, tomato seedlings in two-cotyledon stage were infected with Agrobacterium carrying the TRV-based VIGS of SlGCN5 vector. TRV-SlGCN5 plants exhibited predominantly developmental defects, including reduced plant height, loss of shoot apical dominance, altered pattern of axillary shoot development, shortened internode, late flowering, and male sterility (Figure 1A), suggesting that SlGCN5 is required in various tomato plant developmental processes. To verify the phenotype of TRV-SlGCN5, we created SlGCN5-RNAi plants and found all three of the SlGCN5-RNAi lines exhibited similar phenotype with TRV-SlGCN5 plants (Figure 1B).
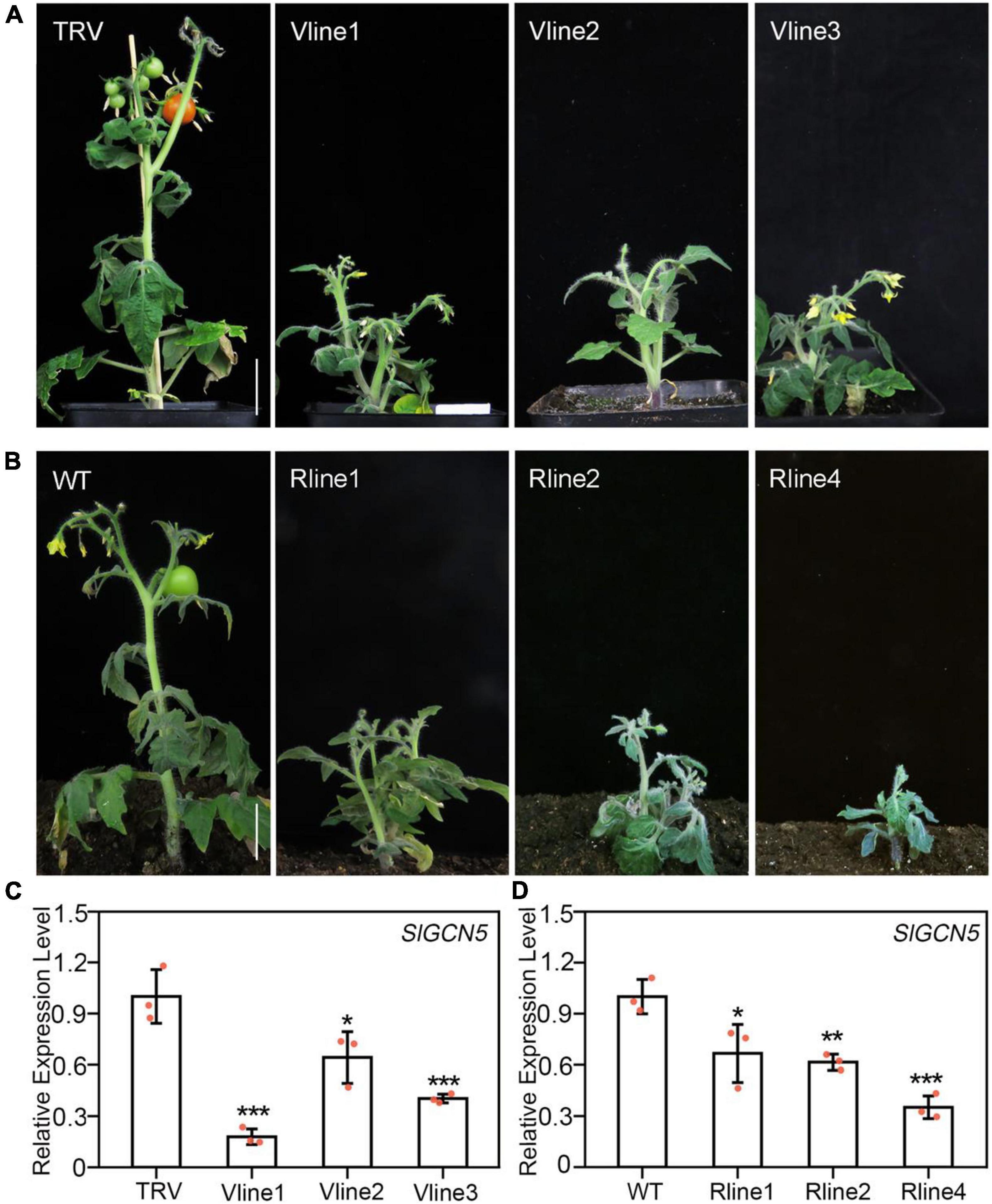
Figure 1. Silencing of SlGCN5 affects plant development. (A) Phenotype of three independent TRV-SlGCN5 lines of Micro-Tom tomato. V lines represent SlGCN5-VIGS lines. (B) Phenotype of three independent SlGCN5-RNAi lines. (C) qRT-PCR analysis of SlGCN5 transcripts in TRV control and TRV-SlGCN5 plants. (D) Transcript levels of SlGCN5 in SlGCN5-RNAi lines relative to wild type (WT). Error bars represent SD of three biological replicates. Asterisks indicate significant differences (*p < 0.05, **p < 0.01, and ***p < 0.001). Scale bars = 3 cm.
Results of qRT-PCR showed that SlGCN5 transcription level in the TRV-SlGCN5-infected plants was significantly lower than plants infected with TRV control (Figure 1C), confirming that the abnormal phenotypes are caused by SlGCN5 gene silencing. Similarly, the expression level of SlGCN5 was significantly reduced in the RNAi lines compared with WT plants (Figure 1D). Due to the similar phenotypes of SlGCN5-RNAi and TRV-SlGCN5 plants, we used TRV-SlGCN5 plants for subsequent functional studies in tomato plant development.
SlGCN5 Is Located in the Nucleus and Highly Expresses in Tomato Early Floral Bud
To investigate the expression pattern of SlGCN5, we first analyzed subcellular localization of SlGCN5 protein. Results showed that SlGCN5-GFP fusion protein driven by constitutive cauliflower mosaic virus 35S promoter exclusively localized in the nucleus (Figure 2A), suggesting that SlGCN5 may have a putative role in histone modification. During tomato plant development, SlGCN5 transcripts expressed widely in roots, stems, leaves, flowers, and fruits (Figure 2B). Our in situ hybridization assays revealed that SlGCN5 is strongly expressed in the upper cell layers of SAM. Meanwhile, SlGCN5 was expressed throughout the entire floral transition meristem and FM of WT plants, which may overlap with the expression domain of SlWUS (Figure 2C), hinting at a potential role for SlGCN5 in regulation of meristematic activities.
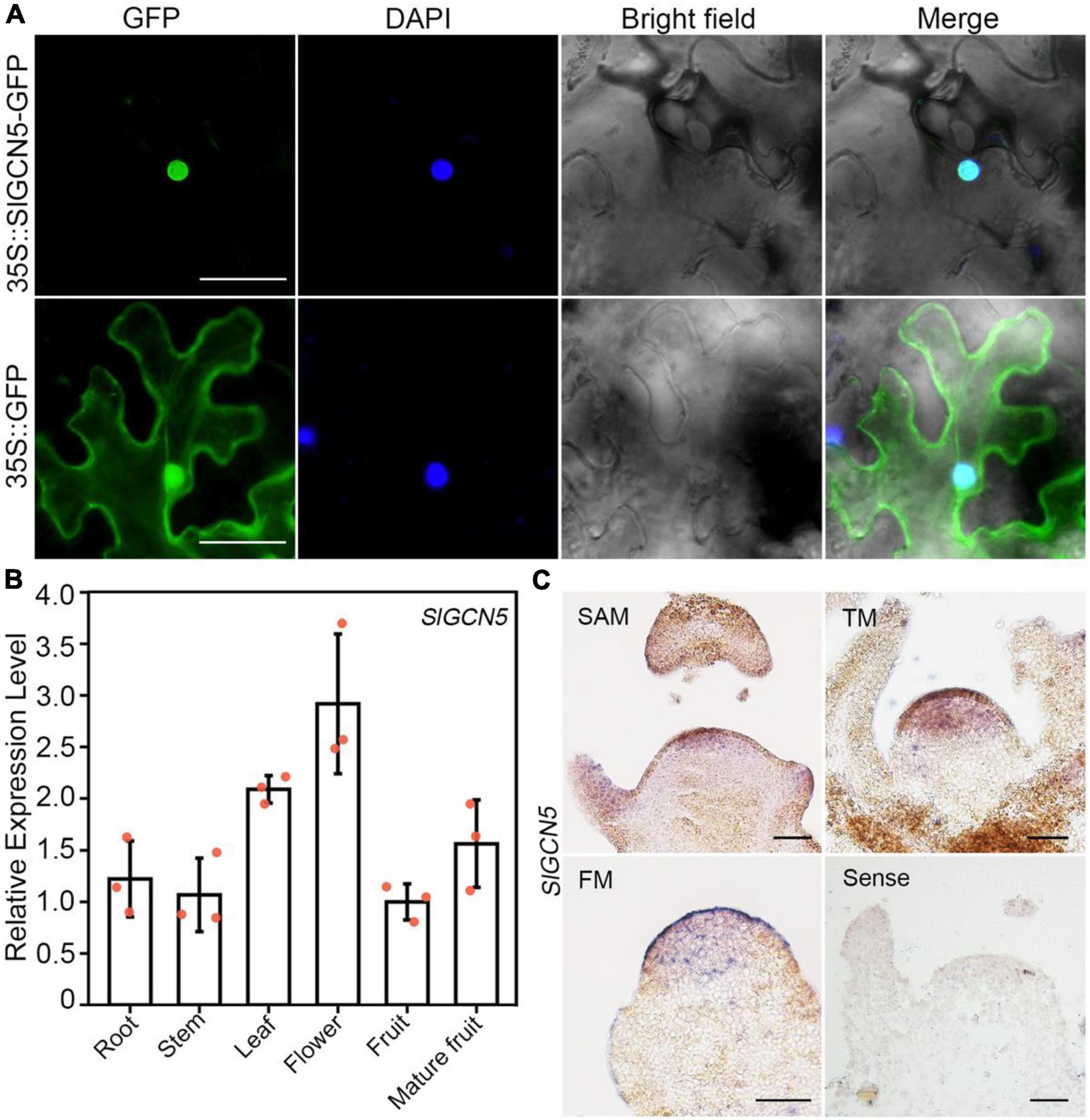
Figure 2. Subcellular localization and gene expression pattern of SlGCN5. (A) Subcellular localization of SlGCN5 in nuclei. 35S:SlGCN5-GFP represents SlGCN5-GFP fusion protein. 35S:GFP represents the control. Scale bars = 20 μm. (B) qRT-PCR analysis of SlGCN5 in different tomato organs. SlACTIN served as the internal control. Error bars represent SD of three biological replicates. (C) In situ hybridization of SlGCN5 in SAM, floral transition meristem (TM), and FM, respectively. Scale bars = 50 μm.
SlGCN5 Catalyzes Histone Acetylation
The SAGA (Spt-Ada-Gcn5 acetyltransferase) complex is highly conserved for active regulation of gene transcription in yeast and plants (Carrozza et al., 2003; Vlachonasios et al., 2003; Zhou et al., 2017; Li et al., 2019). We also identified ADA2a- and ADA2b-like proteins in tomato (Supplementary Figure 2) and named them as SlADA2a and SlADA2b, respectively, which have the highest homology with AtADA2a and AtADA2b in Arabidopsis. SlADA2a and SlADA2b have 3 and 2 isoforms respectively. According to the transcript analysis results in tomato inflorescences, XP_004243566 and XP_004239816 were selected as representatives of SlADA2a and SlADA2b for further study (Supplementary Figure 3). To confirm the interactions between SlADA2a with SlGCN5 and SlADA2b with SlGCN5, we cloned the full-length cDNAs of SlADA2a, SlADA2b, and SlGCN5 and performed yeast two-hybrid assays. The results showed that SlGCN5 can interact with both SlADA2a and SlADA2b in yeast cells (Figure 3A). To verify the yeast two-hybrid results, we performed BiFC analysis in tobacco (Nicotiana tabacum) leaves. SlGCN5 was fused to the C-terminus of YFP and named as SlGCN5-cYFP. SlADA2a or SlADA2b was fused to the N-terminus of YFP and named as SlADA2a-nYFP or SlADA2b-nYFP, respectively. We noticed interactions between SlGCN5 and SlADA2a, as well as SlGCN5 and SlADA2b in the nucleus, both of which gave clear signals (Figure 3B). These results suggest that SlGCN5 can interact with both SlADA2a and SlADA2b and that the three proteins may form a protein complex.
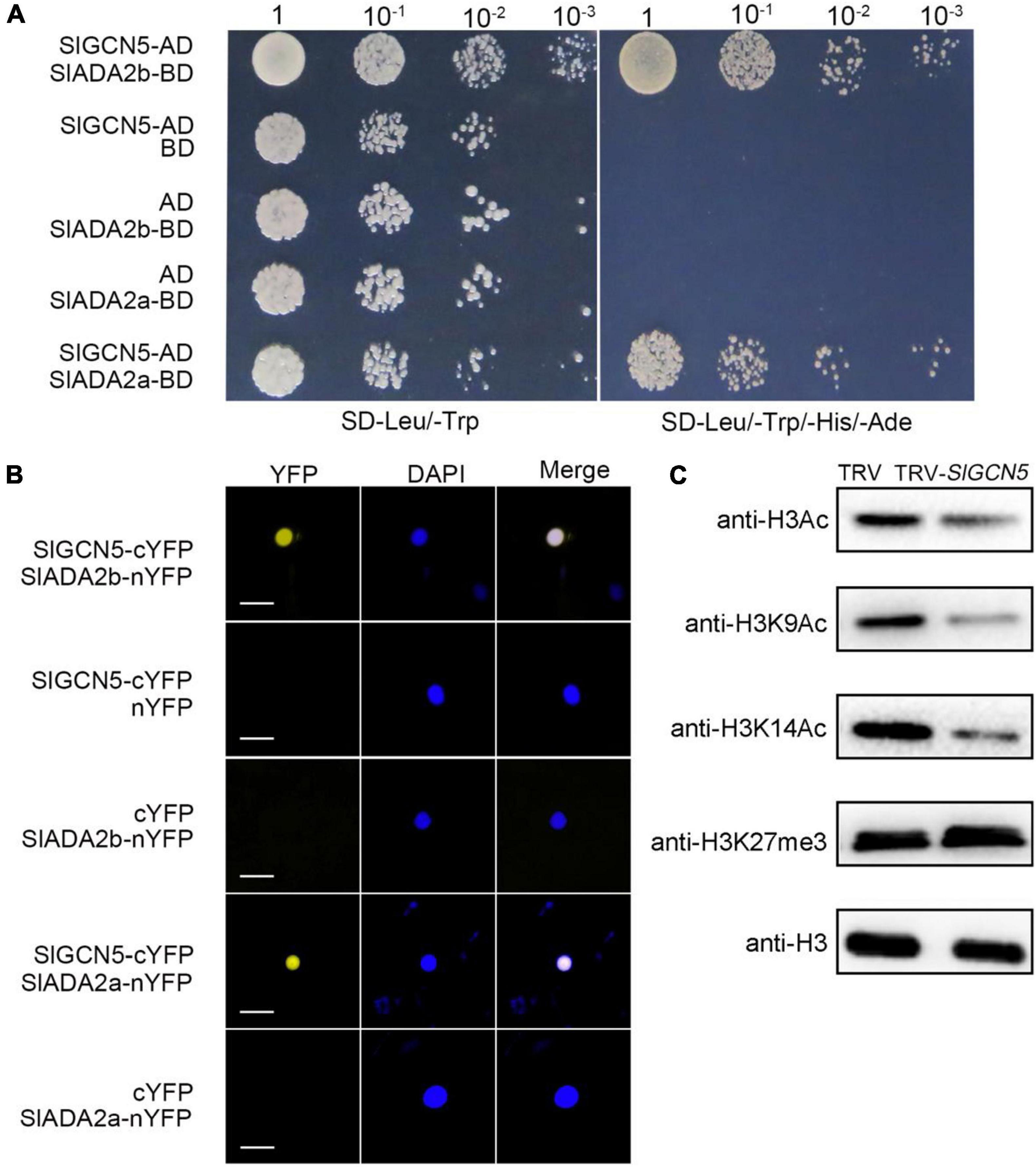
Figure 3. SlGCN5 functions as a histone H3 acetyltransferase. (A) Yeast two-hybrid assays of SlGCN5, SlADA2a, and SlADA2b. Full-length cDNAs of SlGCN5, SlADA2a, and SlADA2b were cloned into AD (the prey plasmid pGADT7) and BD (the bait plasmid pGBKT7), respectively. Yeast cells transformed with the indicated plasmids were grown on medium lacking leucine and tryptophan (SD/-Leu/-Trp) and selective medium lacking leucine, tryptophan, histidine, and adenine (SD/-Leu/-Trp/-His/-Ade). (B) Bimolecular fluorescent complementation analysis in tobacco leaves. Merge refers to merged images for yellow fluorescent protein (YFP) and DAPI fluorescence. SlGCN5 and SlADA2a/b were fused to cCFP and nYFP, respectively. Scale bars = 20 μm. (C) Histone acetyltransferase activity of SlGCN5 determined by in vivo histone acetyltransferase assay. Histone acetylation levels were detected by immunoblotting with antibodies of the indicated histone acetylation marks in TRV and TRV-SlGCN5 plants. Anti-H3 antibody was used as loading control.
To test the HAT activity of SlGCN5 in vivo, we compared histone acetylation levels in TRV-SlGCN5 plants with TRV control plants by immunoblotting, using anti-H3K9Ac, anti-H3K14Ac, anti-H3Ac, and anti-H3K27me3 antibodies. Our results revealed that obvious reduction of H3ac, H3K9ac, and H3K14ac levels in TRV-SlGCN5 compared with the TRV control plants (Figure 3C), suggesting that SlGCN5 can catalyze acetylation on histone H3, specifically at H3K9 and H3K14 residues. These results are consistent with the known function of AtGCN5, which was reported to catalyze H3K14ac, and additional histone residues, including H3K9, H3K18, H3K27, and H3K36, and other histones such as H4 and H2B in Arabidopsis (Kuo et al., 1996; Grant et al., 1997; Morris et al., 2007). To confirm the role of SlGCN5 in plant development, we generated transgenic Arabidopsis plants by transforming the null-mutant gcn5-7 with 35S:SlGCN5-GFP. 35S:SlGCN5-GFP gcn5-7 plants have noticeable gene and protein expressions of SlGCN5, which are examined by qRT-PCR and Western blot (Supplementary Figure 4A). Furthermore, 35S:SlGCN5-GFP gcn5-7 plants show almost fully rescued phenotype compared with gcn5-7 (Supplementary Figure 4B), indicating that SlGCN5 functions similarly as AtGCN5.
SlGCN5 Regulates Tomato Shoot Meristem and Flower Development
SlGCN5-silenced plants exhibited reduced plant height. Thus, we measured SAM size in TRV-SlGCN5 and TRV control plants and observed reduced SAM size in TRV-SlGCN5 at different developmental stages compared with TRV control plants (Figures 4A,B). We also observed reduced FM width but relatively unchanged FM height (Supplementary Figure 5) in TRV-SlGCN5 young floral buds prior to the emergence of the carpel primordia (Figures 4C,D). Although FM size in TRV-SlGCN5 is reduced, floral organ number remains largely unaffected. However, in TRV-SlGCN5 flowers, we occasionally noticed some carpelloid stamens and carpels fused with stamens [2/15 (13.3%) independent transgenic lines show abnormal flowers] (Figure 4E). These results implied that silencing of SlGCN5 resulted in reduced SAM and FM sizes in tomato and may also influence reproductive floral organ development.
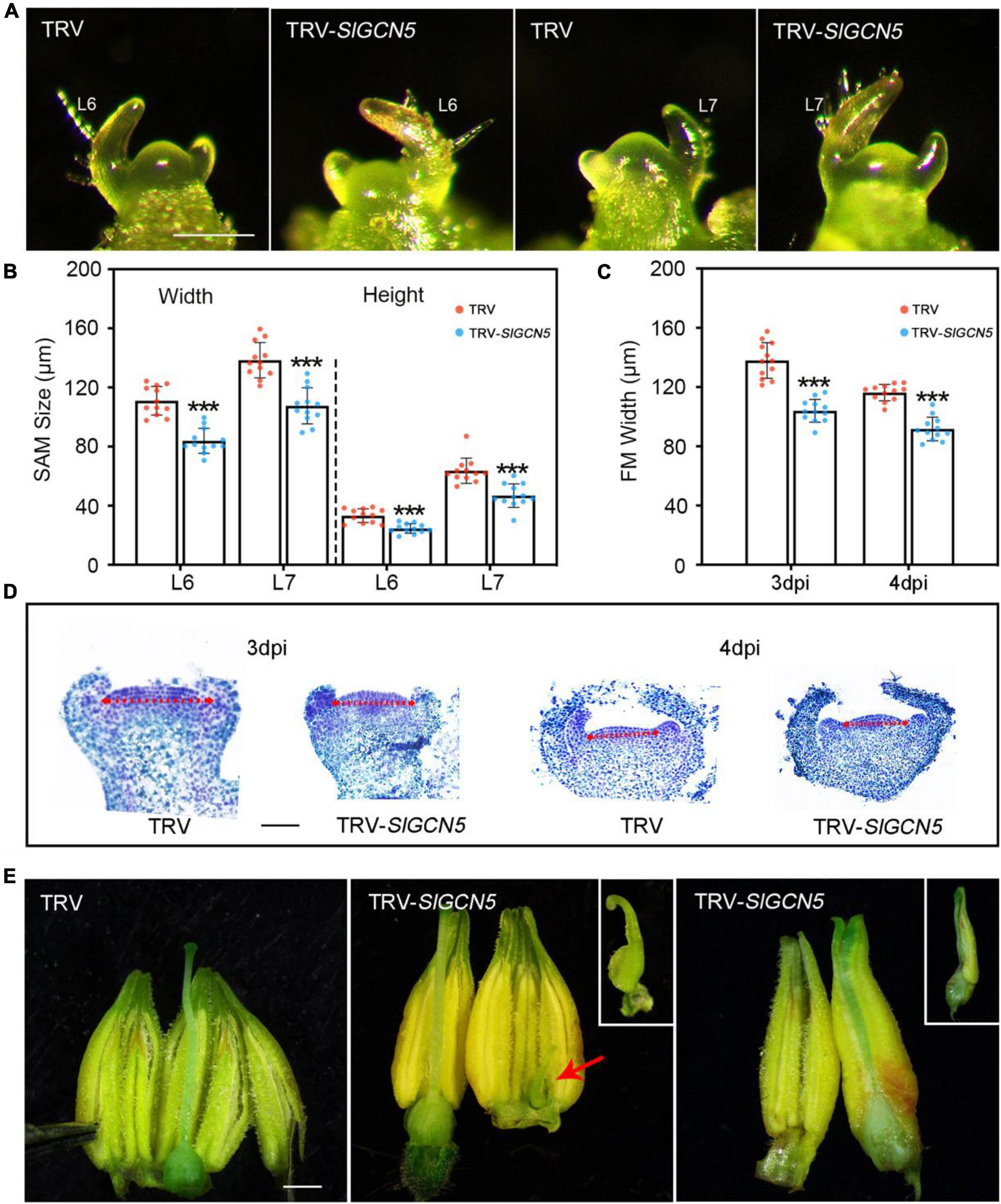
Figure 4. SAM and floral phenotype of silenced-SlGCN5 plants. (A) Images of the SAMs from TRV control and TRV-SlGCN5 plants. L6 and L7 indicate Leaf 6 and Leaf 7, respectively. Scale bars = 200 μm. (B) SAM size from TRV control and TRV-SlGCN5 plants. Error bar indicates SD of 12 biological replicates. (C) FM size from TRV control and TRV-SlGCN5 plants at 3 and 4 dpi (days post floral initiation). Error bar indicates SD of 12 biological replicates. (D) Longitudinal sections of floral meristem of TRV control and TRV-SlGCN5 plants. The red dash arrow marks the width of each floral meristem. Scale bars = 50 μm. (E) Flowers of TRV and TRV-SlGCN5. Scale bars = 1 mm. Asterisks indicate significant differences between TRV control and TRV-SlGCN5 (***p < 0.001).
SlGCN5 Positively Regulates SlWUS Expression
The reduced SAM and FM size leads us to examine expression changes of SlWUS in TRV-SlGCN5 plants. Expression analysis by qRT-PCR revealed that SlWUS transcript level was significantly reduced in TRV-SlGCN5 meristems (Figure 5A). To validate the qRT-PCR results, expression pattern of SlWUS was examined by in situ hybridization assays. We noticed obviously reduced expression of SlWUS mRNA in TRV-SlGCN5 SAMs and FMs (Figure 5B) compared with TRV control plants. These results suggested that SlGCN5 may positively regulate SlWUS expression in tomato shoot meristem and FM. Furthermore, we observed remarkable decrease in the transcript level of SlCLV1 and SlCLV3, the other two key factors in CLV-WUS feedback loop, in TRV-SlGCN5 meristems by qRT-PCR analysis (Supplementary Figure 6). These results indicate that SlGCN5 may potentially regulate multiple genes in meristem development of tomato.
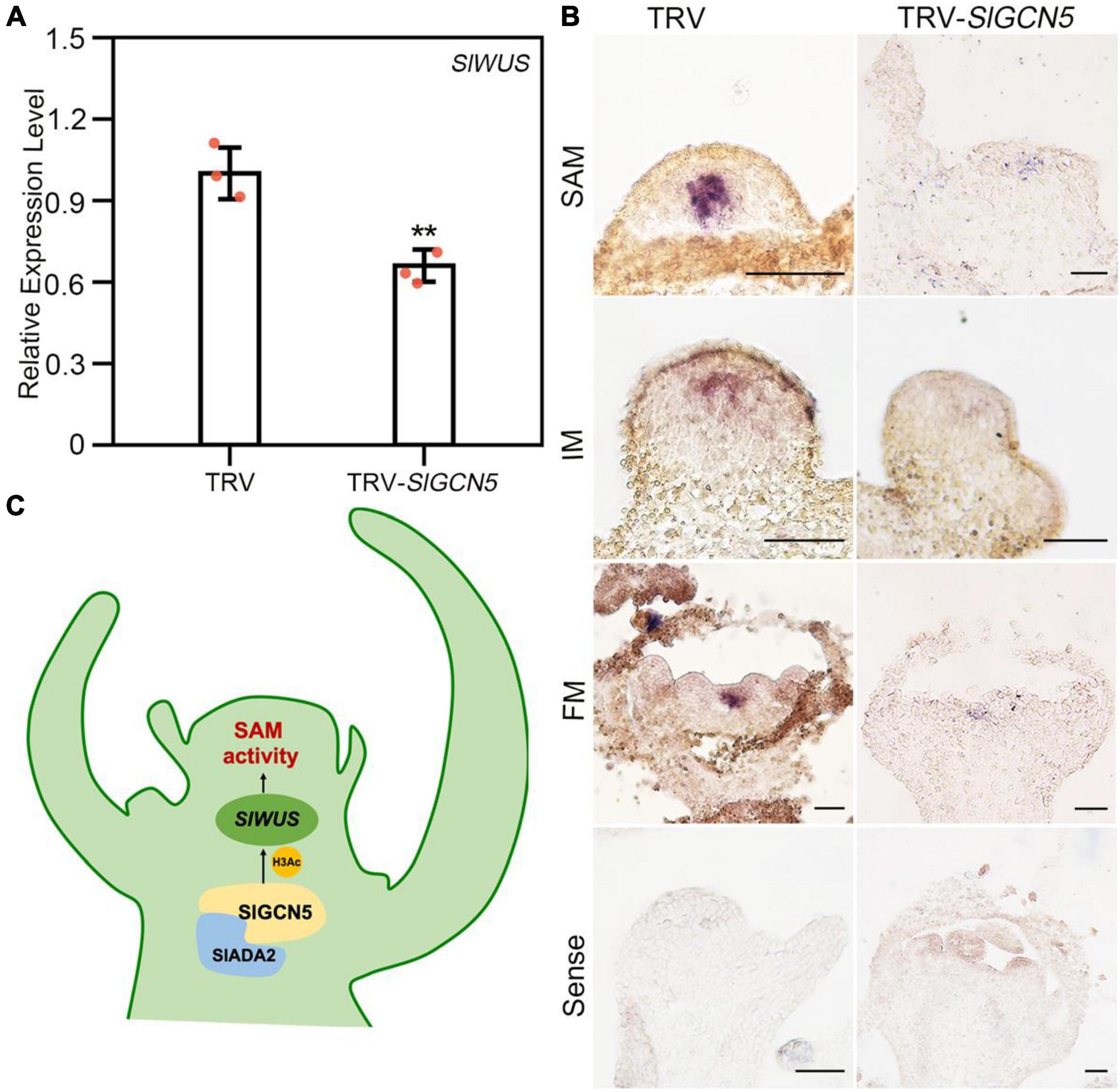
Figure 5. SlGCN5 positively regulates SlWUS in tomato. (A) qRT-PCR analysis of SlWUS expression level in SAM. The error bar represents SD of three biological replicates. The asterisks indicate significant differences between TRV and TRV-SlGCN5 (**p < 0.01). (B) Expression of SlWUS mRNA in TRV control and TRV-SlGCN5 plants by in situ hybridization. Scale bars = 50 μm. (C) Model: SlGCN5 together with SlADA2a and SlADA2b could form HAT complex, which positively regulate SlWUS to ensure the proper development of SAM.
Discussion
Histone lysine acetylation is an essential chromatin modification for epigenetic regulation of gene expression in plant development and plant response to environmental stress. AtGCN5 was identified as the first transcription-linked HAT (Brownell et al., 1996), with specificity for histone H3K14ac (Kuo et al., 1996). In addition, GCN5 could also acetylate histone lysine residues such as H3K9, H3K18, H3K23, H3K27, and H3K36 and other histones such as H4 and H2B (Grant et al., 1997; Morris et al., 2007). The SAGA complex is an evolutionarily conserved HAT complex (Spedale et al., 2012), which catalyzes histone acetylation for modulating gene expression and participates in various developmental processes in eukaryotes. In this study, we showed that SlGCN5 can acetylate histones H3K9 and H3K14 at the genomic level in tomato, and SlGCN5 also interacts with SlADA2a and SlADA2b to form HAT unit.
Shoot apical meristem is an organized structure and responds to different development signals. The stem cell pool is maintained within the central zone of the SAM (Fletcher, 2018). Compromised SAM activity leads to premature plant growth stagnation before forming full organs (Laux et al., 1996; Kieffer et al., 2006), whereas plants with overproliferated stem cells in SAM can produce many extra organs (Clark et al., 1993; Taguchi-Shiobara et al., 2001; Yuste-Lisbona et al., 2020). Therefore, the maintenance of SAM homeostasis is key for plant development. It is well-understood that conserved CLV-WUS feedback signaling is important for the maintenance of SAM activity (Somssich et al., 2016), but it is not well-known how this feedback loop is modified in various plant species. In this study, we characterized the function of SlGCN5 and studied its role in SAM maintenance. Our data indicate that SlGCN5 is important to maintain SAM activity in tomato. Weakened SlGCN5 activity affects SAM development and resulted in reduced SAM and FM size (Figure 4). Consistent with the phenotype, we also observed reduced SlWUS expression (Figure 5B) in SAM and FM in the plants with compromised SlGCN5 activity. However, we did not observe obvious changes in floral organ numbers. Instead, we occasionally observed some carpelloid stamens and carpels fused with stamens (Figure 4E). These phenotypes resemble the S. lycopersicum GT11 (SlGT11) mutant, in which the function of floral B-class genes was affected (Yang et al., 2020). Therefore, we suspect that the transformation of floral homeotic genes may also exist in TRV-SlGCN5 plants and that SlGCN5 could participate in the maintenance of floral organ identity.
Modulation of CLV-WUS pathway is one important approach to increase crop yield (Fletcher, 2018). In tomato, several transcription factors that could influence the CLV-WUS loop also have been discovered. DEFECTIVE TOMATO MERISTEM (DTM) forms a negative feedback loop with the class III homeodomain-leucine zipper (HD-ZIP III) transcription factors to confine SlCLV3 and SlWUS expression to specific domains in the shoot meristem of tomato (Xu et al., 2019). APETALA2/ethylene responsive factor (AP2/ERF) superfamily transcription factor excessive number of floral organs (ENO) regulates SlWUS expression to restrict stem cell proliferation, thereby maintaining floral stem cell homeostasis (Yuste-Lisbona et al., 2020). In addition to transcription factors, SlWUS expression can also be regulated by chromatin remodeling factors such as histone deacetylase 19 in tomato (Bollier et al., 2018).
In this study, we identified and investigated the function of SlGCN5 in tomato meristem development and found that SlGCN5 acts as an acetyltransferase to activate the expression of SlWUS, thus maintaining SAM activity (Figure 5C). We also noticed SlGCN5 may play a role in floral organ development. These findings could potentially shed light on genetic enhancement of tomato plants.
Data Availability Statement
The original contributions presented in the study are included in the article/Supplementary Material, further inquiries can be directed to the corresponding author/s.
Author Contributions
BS conceived and designed research, wrote the manuscript, and revised the manuscript. AH and SX conducted experiments. ZY performed data analysis. All authors have read and approved the manuscript.
Funding
This work was supported by the Fundamental Research Funds for the Central Universities (0208/14380167) to BS.
Conflict of Interest
The authors declare that the research was conducted in the absence of any commercial or financial relationships that could be construed as a potential conflict of interest.
Publisher’s Note
All claims expressed in this article are solely those of the authors and do not necessarily represent those of their affiliated organizations, or those of the publisher, the editors and the reviewers. Any product that may be evaluated in this article, or claim that may be made by its manufacturer, is not guaranteed or endorsed by the publisher.
Acknowledgments
We would like to thank Zhuqing Shao (Nanjing University) for providing tomato seeds and Bote Luo from Xiaofeng Wang lab (Northwest A&F University) for providing pTRV1 and pTRV2 vectors.
Supplementary Material
The Supplementary Material for this article can be found online at: https://www.frontiersin.org/articles/10.3389/fpls.2021.805879/full#supplementary-material
Footnotes
- ^ https://solgenomics.net
- ^ http://wmd3.weigelworld.org./cgi-bin/webapp.cgi
- ^ https://phytozome-next.jgi.doe.gov/
References
Anzola, J. M., Sieberer, T., Ortbauer, M., Butt, H., Korbei, B., Weinhofer, I., et al. (2010). putative arabidopsis transcriptional adaptor protein (PROPORZ1) is required to modulate histone acetylation in response to auxin. Proc. Natl. Acad. Sci. U.S.A. 107, 10308–10313. doi: 10.1073/pnas.0913918107
Bannister, A. J., and Kouzarides, T. (2011). Regulation of chromatin by histone modifications. Cell Res. 21, 381–395. doi: 10.1038/cr.2011.22
Benhamed, M., Bertrand, C., Servet, C., and Zhou, D.-X. (2006). Arabidopsis GCN5, HD1, and TAF1/HAF2 interact to regulate histone acetylation required for light-responsive gene expression. Plant Cell 18, 2893–2903. doi: 10.1105/tpc.106.043489
Bertrand, C., Bergounioux, C., Domenichini, S., Delarue, M., and Zhou, D.-X. (2003). Arabidopsis histone acetyltransferase AtGCN5 regulates the floral meristem activity through the WUSCHEL/AGAMOUS pathway. J. Biol. Chem. 278, 28246–28251. doi: 10.1074/jbc.M302787200
Bollier, N., Sicard, A., Leblond, J., Latrasse, D., Gonzalez, N., Gevaudant, F., et al. (2018). At-MINI ZINC FINGER2 and Sl-INHIBITOR OF MERISTEM ACTIVITY, a conserved missing link in the regulation of floral meristem termination in Arabidopsis and tomato. Plant Cell 30, 83–100. doi: 10.1105/tpc.17.00653
Brownell, J. E., Zhou, J., Ranalli, T., Kobayashi, R., Edmondson, D. G., Roth, S. Y., et al. (1996). Tetrahymena histone acetyltransferase A: a homolog to Yeast Gcn5p linking histone acetylation to gene activation. Cell 84, 843–851. doi: 10.1016/S0092-8674(00)81063-6
Carrozza, M. J., Utley, R. T., Workman, J. L., and Côté, J. (2003). The diverse functions of histone acetyltransferase complexes. Trends Genet. 19, 321–329. doi: 10.1016/S0168-9525(03)00115-X
Chu, Y. H., Jang, J. C., Huang, Z., and van der Knaap, E. (2019). Tomato locule number and fruit size controlled by natural alleles of lc and fas. Plant Direct 3:e00142. doi: 10.1002/pld3.142
Clark, S. E., Running, M. P., and Meyerowitz, E. M. (1993). Clavata1, a regulator of meristem and flower development in Arabidopsis. Development 119, 397–418.
Cohen, R., Schocken, J., Kaldis, A., Vlachonasios, K. E., Hark, A. T., and McCain, E. R. (2009). The histone acetyltransferase GCN5 affects the inflorescence meristem and stamen development in Arabidopsis. Planta 230, 1207–1221. doi: 10.1007/s00425-009-1012-5
Cortina, C., and Culiáñez-Macià, F. A. (2004). Tomato transformation and transgenic plant production. Plant Cell Tissue Organ Cult. 76, 269–275. doi: 10.1023/B:TICU.0000009249.14051.77
Daum, G., Medzihradszky, A., Suzaki, T., and Lohmann, J. U. (2014). A mechanistic framework for noncell autonomous stem cell induction in Arabidopsis. Proc. Natl. Acad. Sci. U.S.A. 111:14619.
Fletcher, J. C. (2018). The CLV-WUS stem cell signaling pathway: a roadmap to crop yield optimization. Plants 7:87. doi: 10.3390/plants7040087
Fu, D.-Q., Zhu, B.-Z., Zhu, H.-L., Jiang, W.-B., and Luo, Y.-B. (2005). Virus-induced gene silencing in tomato fruit: VIGS in tomato fruit. Plant J. 43, 299–308. doi: 10.1111/j.1365-313X.2005.02441.x
Galli, M., and Gallavotti, A. (2016). Expanding the regulatory network for meristem size in plants. Trends Genet. 32, 372–383. doi: 10.1016/j.tig.2016.04.001
Grant, P. A., Duggan, L., Cote, J., Roberts, S. M., Brownell, J. E., Candau, R., et al. (1997). Yeast Gcn5 functions in two multisubunit complexes to acetylate nucleosomal histones: characterization of an Ada complex and the SAGA (Spt/Ada) complex. Genes Dev. 11, 1640–1650. doi: 10.1101/gad.11.13.1640
Han, H., Liu, X., and Zhou, Y. (2020). Transcriptional circuits in control of shoot stem cell homeostasis. Curr. Opin. Plant Biol. 53, 50–56.
Kieffer, M., Stern, Y., Cook, H., Clerici, E., Maulbetsch, C., Laux, T., et al. (2006). Analysis of the transcription factor WUSCHEL and its functional homologue in antirrhinum reveals a potential mechanism for their roles in meristem maintenance. Plant Cell 18, 560–573. doi: 10.1105/tpc.105.039107
Kornet, N., and Scheres, B. (2009). Members of the GCN5 histone acetyltransferase complex regulate PLETHORA-mediated root stem cell niche maintenance and transit amplifying cell proliferation in Arabidopsis. Plant Cell 21, 1070–1079. doi: 10.1105/tpc.108.065300
Kudla, J., and Bock, R. (2016). Lighting the way to protein-protein interactions: recommendations on best practices for bimolecular fluorescence complementation analyses[OPEN]. Plant Cell 28, 1002–1008. doi: 10.1105/tpc.16.00043
Kuo, M.-H., Brownell, J. E., Sobel, R. E., Ranalli, T. A., Cook, R. G., Edmondson, D. G., et al. (1996). Transcription-linked acetylation by Gcn5p of histones H3 and H4 at specific lysines. Nature 383, 269–272. doi: 10.1038/383269a0
Laux, T., Mayer, K. F. X., Berger, J., and Jurgens, G. (1996). The WUSCHEL gene is required for shoot and floral meristem integrity in Arabidopsis. Development 122, 87–96.
Li, H., Qi, M., Sun, M., Liu, Y., Liu, Y., Xu, T., et al. (2017). Tomato Transcription factor SlWUS plays an important role in tomato flower and locule development. Front. Plant Sci. 8:457. doi: 10.3389/fpls.2017.00457
Li, S., Lin, Y.-C. J., Wang, P., Zhang, B., Li, M., Chen, S., et al. (2019). The AREB1 transcription factor influences histone acetylation to regulate drought responses and tolerance in populus trichocarpa. Plant Cell 31, 663–686. doi: 10.1105/tpc.18.00437
Mao, Y., Pavangadkar, K. A., Thomashow, M. F., and Triezenberg, S. J. (2006). Physical and functional interactions of Arabidopsis ADA2 transcriptional coactivator proteins with the acetyltransferase GCN5 and with the cold-induced transcription factor CBF1. Biochim. Biophys. Acta 1759, 69–79.
Minh, B. Q., Nguyen, M. A., and von Haeseler, A. (2013). Ultrafast approximation for phylogenetic bootstrap. Mol. Biol. Evol. 30, 1188–1195. doi: 10.1093/molbev/mst024
Morris, S. A., Rao, B., Garcia, B. A., Hake, S. B., Diaz, R. L., Shabanowitz, J., et al. (2007). Identification of histone H3 lysine 36 acetylation as a highly conserved histone modification. J. Biol. Chem. 282, 7632–7640. doi: 10.1074/jbc.M607909200
Muños, S., Ranc, N., Botton, E., Bérard, A., Rolland, S., Duffé, P., et al. (2011). Increase in tomato locule number is controlled by two single-nucleotide polymorphisms located near WUSCHEL1[C][W]. Plant Physiol. 156, 2244–2254. doi: 10.1104/pp.111.173997
Nguyen, L.-T., Schmidt, H. A., von Haeseler, A., and Minh, B. Q. (2015). IQ-TREE: a fast and effective stochastic algorithm for estimating maximum-likelihood phylogenies. Mol. Biol. Evol. 32, 268–274. doi: 10.1093/molbev/msu300
Périlleux, C., Lobet, G., and Tocquin, P. (2014). Inflorescence development in tomato: gene functions within a zigzag model. Front. Plant Sci. 5:121. doi: 10.3389/fpls.2014.00121
Pfeiffer, A., Wenzl, C., and Lohmann, J. U. (2017). Beyond flexibility: controlling stem cells in an ever changing environment. Curr. Opin. Plant Biol. 35, 117–123. doi: 10.1016/j.pbi.2016.11.014
Rodríguez-Leal, D., Lemmon, Z. H., Man, J., Bartlett, M. E., and Lippman, Z. B. (2017). Engineering quantitative trait variation for crop improvement by genome editing. Cell 171, 470–480.e8. doi: 10.1016/j.cell.2017.08.030
Ruggieri, V., Alexiou, K., Morata, J., Argyris, J., and Benhamed, M. (2020). GCN5 modulates salicylic acid homeostasis by regulating H3K14ac levels at the 5′ and 3′ ends of its target genes. Nucleic Acids Res. 48, 5953–5966.
Schmitz, G., and Theres, K. (1999). Genetic control of branching in Arabidopsis and tomato. Curr. Opin. Plant Biol. 2, 51–55. doi: 10.1016/S1369-5266(99)80010-7
Schoof, H., Lenhard, M., Haecker, A., Mayer, K. F. X., Jürgens, G., and Laux, T. (2000). The stem cell population of arabidopsis shoot meristems is maintained by a regulatory loop between the CLAVATA and WUSCHEL genes. Cell 100, 635–644. doi: 10.1016/S0092-8674(00)80700-X
Sekhar, K., and Sawhney, V. K. (1984). A scanning electron microscope study of the development and surface features of floral organs of tomato (Lycopersicon esculentum). Can. J. Bot. 62, 2403–2413.
Servet, C., Conde e Silva, N., and Zhou, D.-X. (2010). Histone acetyltransferase AtGCN5/HAG1 is a versatile regulator of developmental and inducible gene expression in Arabidopsis. Mol. Plant 3, 670–677. doi: 10.1093/mp/ssq018
Shahbazian, M. D., and Grunstein, M. (2007). Functions of site-specific histone acetylation and deacetylation. Annu. Rev. Biochem. 76, 75–100. doi: 10.1146/annurev.biochem.76.052705.162114
Shang, E., Ito, T., and Sun, B. (2019). Control of floral stem cell activity in Arabidopsis. Plant Signal. Behav. 14:1659706. doi: 10.1080/15592324.2019.1659706
Somssich, M., Je, B. I., Simon, R., and Jackson, D. (2016). CLAVATA-WUSCHEL signaling in the shoot meristem. Development 143, 3238–3248. doi: 10.1242/dev.133645
Sparkes, I. A., Runions, J., Kearns, A., and Hawes, C. (2006). Rapid, transient expression of fluorescent fusion proteins in tobacco plants and generation of stably transformed plants. Nat. Protoc. 1, 2019–2025. doi: 10.1038/nprot.2006.286
Spedale, G., Timmers, H. T. M., and Pijnappel, W. W. M. P. (2012). ATAC-king the complexity of SAGA during evolution. Genes Dev. 26, 527–541. doi: 10.1101/gad.184705.111
Sun, B., Zhou, Y., Cai, J., Shang, E., Yamaguchi, N., Xiao, J., et al. (2019). Integration of transcriptional repression and polycomb-mediated silencing of WUSCHEL in floral meristems. Plant Cell 31, 1488–1505. doi: 10.1105/tpc.18.00450
Taguchi-Shiobara, F., Yuan, Z., Hake, S., and Jackson, D. (2001). The fasciated ear2 gene encodes a leucine-rich repeat receptor-like protein that regulates shoot meristem proliferation in maize. Genes Dev 15, 2755–2766. doi: 10.1101/gad.208501
Tripodi, P. (2020). Crop Breeding: Genetic Improvement Methods. Methods in Molecular Biology. New York, NY: Humana.
Vlachonasios, K. E., Thomashow, M. F., and Triezenberg, S. J. (2003). Disruption mutations of ADA2b and GCN5 transcriptional adaptor genes dramatically affect arabidopsis growth, development, and gene expression. Plant Cell 15, 626–638. doi: 10.1105/tpc.007922
Xu, C., Liberatore, K. L., MacAlister, C. A., Huang, Z., Chu, Y.-H., Jiang, K., et al. (2015). A cascade of arabinosyltransferases controls shoot meristem size in tomato. Nat. Genet. 47, 784–792. doi: 10.1038/ng.3309
Xu, Q., Li, R., Weng, L., Sun, Y., Li, M., and Xiao, H. (2019). Domain-specific expression of meristematic genes is defined by the LITTLE ZIPPER protein DTM in tomato. Commun. Biol. 2:134. doi: 10.1038/s42003-019-0368-8
Yadav, R. K., Perales, M., Gruel, J., Girke, T., Jnsson, H., and Reddy, G. V. (2011). WUSCHEL protein movement mediates stem cell homeostasis in the Arabidopsis shoot apex. Genes Dev. 25, 2025–2030.
Yang, L., Qi, S., Touqeer, A., Li, H., Zhang, X., Liu, X., et al. (2020). SlGT11 controls floral organ patterning and floral determinacy in tomato. BMC Plant Biol. 20:562. doi: 10.1186/s12870-020-02760-2
Yuste-Lisbona, F. J., Fernández-Lozano, A., Pineda, B., Bretones, S., Ortíz-Atienza, A., García-Sogo, B., et al. (2020). ENO regulates tomato fruit size through the floral meristem development network. Proc. Natl. Acad. Sci. U.S.A. 117, 8187–8195. doi: 10.1073/pnas.1913688117
Keywords: tomato, SAM, SlGCN5, SlWUS, SlADA2
Citation: Hawar A, Xiong S, Yang Z and Sun B (2022) Histone Acetyltransferase SlGCN5 Regulates Shoot Meristem and Flower Development in Solanum lycopersicum. Front. Plant Sci. 12:805879. doi: 10.3389/fpls.2021.805879
Received: 31 October 2021; Accepted: 21 December 2021;
Published: 21 January 2022.
Edited by:
Xigang Liu, Hebei Normal University, ChinaReviewed by:
Weibing Yang, Institute of Plant Physiology and Ecology, Shanghai Institutes for Biological Sciences, Chinese Academy of Sciences (CAS), ChinaYun Zhou, Purdue University, United States
Copyright © 2022 Hawar, Xiong, Yang and Sun. This is an open-access article distributed under the terms of the Creative Commons Attribution License (CC BY). The use, distribution or reproduction in other forums is permitted, provided the original author(s) and the copyright owner(s) are credited and that the original publication in this journal is cited, in accordance with accepted academic practice. No use, distribution or reproduction is permitted which does not comply with these terms.
*Correspondence: Bo Sun, c3VuYm9Abmp1LmVkdS5jbg==