- 1INRAE, URP3F, F-86600 Lusignan, France
- 2Biotechnology Research Center (BTRC), Tripoli, Libya
- 3Univ. Angers, Institut Agro, INRAE, IRHS, SFR QUASAV, F-49000 Angers, France
Various adaptive mechanisms can ensure that seedlings are established at the most favourable time and place. These mechanisms include seed dormancy i.e., incapacity to germinate in any environment without a specific environmental trigger and inhibition i.e., incapacity to germinate in an unfavourable environment (water availability, temperature: thermoinhibition and light). The objective of this research was to study in the temperate range for germination of forage and turf grass species perennial ryegrass, if the thermal requirements for germination are under genetic controlled and could be selectively bred. Two divergent selections of three cycles were realized on a natural population: one to select for the capacity to germinate at 10°C vs. the impossibility to germinate at 10°C, and one to select for the capacity to germinate at 32°C vs. the impossibility to germinate at 32°C. Seeds of all the lots obtained from the two divergent selections were then germinated at constant temperatures from 5 to 35°C to evaluate their germination ability. Concerning the positive selection, the first cycle of positive selection at 10°C was highly efficient with a very strong increase in the germination percentage. However, afterward no selection effect was observed during the next two cycles of positive selection. By contrast, the positive selection at 32°C was efficient during all cycles with a linear increase of the percentage of germination at 32°C. Concerning the negative selection, we observed only a large positive effect of the first cycle of selection at 10°C. These findings demonstrate that seed thermoinhibition at 10 and 32°C observed in a natural population of perennial ryegrass has a genetic basis and a single recessive gene seems to be involved at 10°C.
Introduction
As the first stage of the plant life cycle, germination is particularly critical for successful establishment and growth of plants (Ooi, 2012; Nunes et al., 2016). Germination starts with water uptake by the dry seed (imbibition), continues with biochemical preparative processes and the elongation of the embryonic axis, and terminates with protrusion of the radicle out of the seed (Bewley and Black, 1994; Bewley et al., 2013). To be successful, germination has to be triggered in appropriate environmental conditions.
Seed germination ecology is a complex subject that aims to understand what controls the timing of seed germination in nature (Baskin and Baskin, 2014; Nunes et al., 2016). Various adaptive mechanisms ensure that seed germination occurs at the most advantageous time and place. These mechanisms could be classified as (i) “dormancy” that requires a special event or “trigger” before the embryo can resume growth e.g., stratification by cold temperatures and (ii) “inhibition” that requires just favourable environmental conditions for the embryo to grow (Hills and van Staden, 2003; Batlla and Benech-Arnold, 2015). Consequently, for some dormant seeds, special environmental conditions must be met to release this dormancy and then additional conditions must be met to allow germination of the seeds (Baskin and Baskin, 1988; Simpson, 1990; Bewley, 1997; Bewley et al., 2013). Important environmental factors influencing the inhibition of seed germination are water availability, temperature and light. In particular, the action of temperature on seed germination is related to enzyme activity and membrane stability and permeability affecting respiratory metabolism (Baskin and Baskin, 2014; Nunes et al., 2016).
The response of germination to temperature in different species has been largely studied (Bewley and Black, 1994; Brunel et al., 2009; Durr et al., 2015; Tribouillois et al., 2016; Ghaleb, 2019; Ghaleb et al., 2021). Major differences among species depend on the climatic conditions where the species originate. Furthermore, cultivated species germinate faster and under a wider range of temperatures than wild species, likely as a result of human selection (Durr et al., 2015; Ghaleb et al., 2021). The same tendency of various response of germination to temperature has been observed among populations of the same species (Butler et al., 2017; Ahmed and Escobar-Gutiérrez, 2022). In perennial ryegrass (Lolium perenne L.), it has been shown that the capacity of germination (after dormancy release) at different temperatures varies dramatically between natural populations of various geographical origins (Ghaleb, 2019). The variability within populations is also noticeable. For example, within the 3038 population, 95% of the seeds (different genotypes) do not germinate at a sub-optimal temperature (e.g., 5°C) whereas the remaining 5% germinate. Varieties of perennial ryegrass tend to germinate at a high rate at almost all temperatures (Ahmed et al., 2014, “accession identifier is ACVF60016”; Ghaleb, 2019, “accession identifier is 553”). These observations at different levels of genetic variability demonstrate that the response of germination to temperature is at least partly genetically controlled.
The heritability and the genetic determinism of the response of germination to temperature is still not completely understood (Baskin and Baskin, 2014; Yan and Chen, 2020; Ghaleb et al., 2021). It has been shown that it is possible to select for germination at a given temperature, for example in maize at low temperature (9.5°C) (Landi et al., 1992; Frascaroli and Landi, 2013). Several works, using mapping populations (e.g., Hayashi et al., 2008; Argyris et al., 2011; Dias et al., 2011; Jiang et al., 2017) and natural populations association studies (e.g., Chopra et al., 2017), have reported QTLs for the ability to germinate at specific temperatures. These results are encouraging for breeding for the ability or not to germinate at specific temperature.
Perennial ryegrass is the most sown grass forage species in temperate climates (Wilkins, 1991; Jones et al., 2002; Zhang et al., 2006). Natural populations are distributed in a wide range of environments across Europe and the Mediterranean regions (Wilkins, 1991; Blackmore et al., 2016; Blanco-Pastor et al., 2019). Consequently, vast natural genetic resources exist in this species (Blanco-Pastor et al., 2019) that can be used to produce genetic material with new traits adapted to new climatic constraints such as the inhibition of seed germination at extreme temperatures and meeting the needs of agriculture (Keep et al., 2020). Perennial ryegrass is an obligate outcrossing species for which varieties are synthetics (intercrosses of several selected individuals). As a consequence, genetic diversity exists in both natural populations and varieties (e.g., Kubik et al., 2001; Bolaric et al., 2005).
The objective of this research was to study if the ability of perennial ryegrass to germinate or not at a given temperature was genetically controlled and could be selected. For this purpose, two divergent selections (one at 10°C and one at 32°C) were realized on the 3,038 population of perennial ryegrass showing contrasting germination ability at different temperatures. Some seeds (genotypes) have the ability to germinate at 10°C or at 32°C whereas others do not, thus, revealing the variability within this population for this trait. Almost all seeds have the ability to germinate at 25°C after incubation at sub/supra-optimal temperatures, indicating that the absence of germination at low or high temperatures should be interpreted as an inhibition of germination and not as a dormancy, which has been released by a cold treatment (5°C during 7 days) (ISTA, 2015).
Plant Material
In this study, a wild population of perennial ryegrass (Lolium perenne L.) (accession 3038) was used. Seeds were obtained from INRAE’s Centre de Ressources Biologiques des Espèces Fourragères (CRB) at Lusignan, France. The coordinates of the site of collection are 49°15′29.98″N, 4°01′54.11″E. This population was chosen for its variable germination capacities in relation to temperature (Figure 1). Seeds were produced in 1991 and conserved at 5°C and 30% relative humidity until they were used.
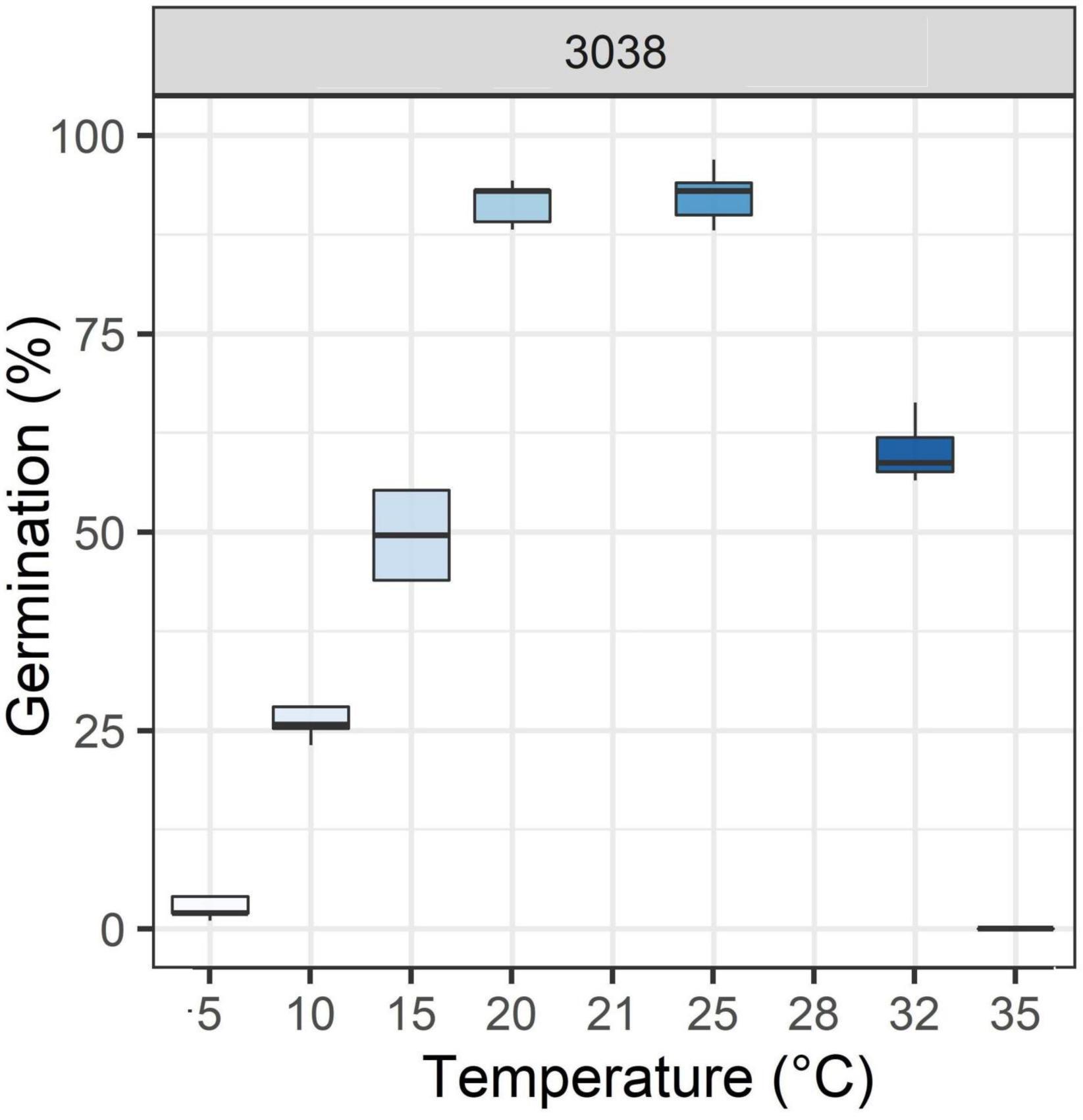
Figure 1. Boxplots of germination percentage in response to different temperatures of the 3038 wild population of perennial ryegrass (Ahmed et al., 2014; Ghaleb, 2019).
Divergent Selections
Regarding the response of germination capacity to temperature on the 3038 population, two divergent selections were performed: one to select for the capacity to germinate at 10°C vs. the impossibility to germinate at 10°C (inhibition of seed germination at 10°C), and one to select for the capacity to germinate at 32°C vs. the impossibility to germinate at 32°C (inhibition of seed germination at 32°C). The germination protocol is explained in the following part (Germination experiment).
Three cycles of divergent selection were performed (Figure 2):
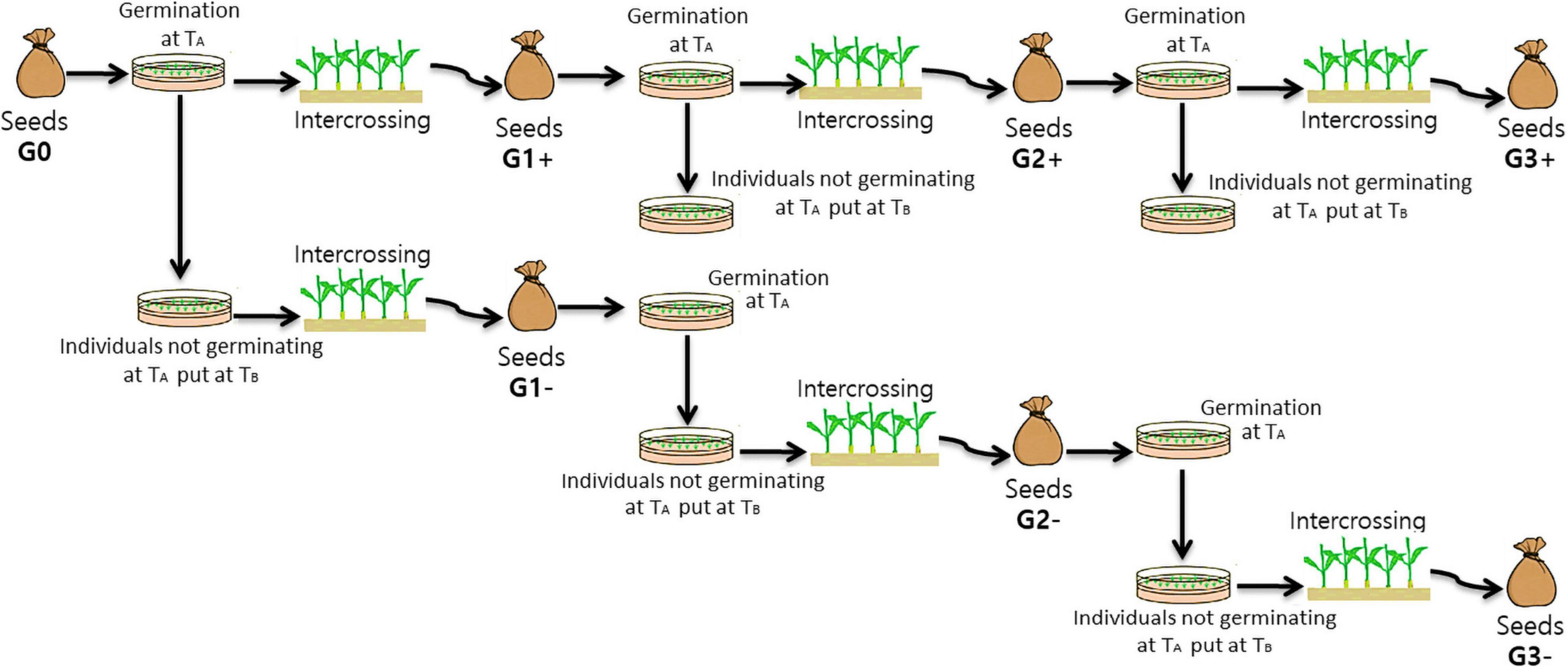
Figure 2. Diagram illustrating the steps of the divergent selections for the capacity to germinate or not at an unfavourable temperature. From G0 seeds (initial generation/generation 0) to G3 seeds (third generation of selection) (TA = Unfavourable temperature = 10°C or 32°C and TB = Optimum temperature = 25°C).
- In the first cycle about 1000 seeds from the initial population were placed at an sub/supra-optimal temperature TA (10 or 32°C) for germination and seedlings obtained from germinated seeds were selected. These grown-up plants were then intercrossed to form the positive selection (PS) of the next generation (G1+ obtained in 2015). In parallel, seeds that did not germinate at TA were placed at an optimal germination temperature TB (25°C), and seedlings obtained from germinated seeds were selected. These plants were then intercrossed to form the negative selection (NS) of the next generation (G1− obtained in 2015).
- In cycles two and three, 1000 seeds of PS were placed at TA and seedlings obtained from germinated seeds were selected. These grown-up plants were then intercrossed to form PS of the next generation (G2+ obtained in 2016 and G3+ obtained in 2017). The other seeds were placed at TB to evaluate the total germination capacity. In the other hand about 1000 seeds from NS were placed at TA and the seeds that did not germinate at TA were placed at an optimal germination temperature TB and seedlings that have germinated were selected. Grown-up plants were then intercrossed to form NS of the next generation (G2− obtained in 2016, no G3− were obtained due to the delay necessary to select the plants before intercrossing). Numbers of individuals intercrossed at each cycle of the divergent selection are indicated in Table 1.
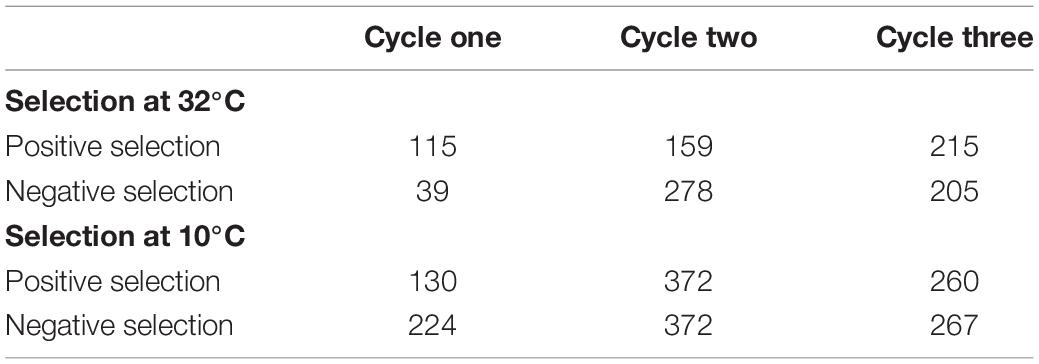
Table 1. Number of individuals (from 1000 seeds) intercrossed at each cycle of the divergent selections.
Germination Experiment
Seeds of all the lots obtained from the two divergent selections were germinated at constant temperatures from 5 to 35°C to evaluate their germination ability (in this assessment the initial lot of 1991 was replaced by a new lot obtained in 2014, for excluding the effect of seed age on seed germination). These large ranges of temperatures were conducted to evaluate the effect of divergent selection at one temperature on the ability to germinate at different temperatures. The germination test was performed between July and December 2019. Seeds of G1 were obtained in July 2016, the ones of G2 were obtained in July 2017, and the ones of G3 were obtained in July 2018.
For each temperature, every seed-lot was represented by five replicates of 100 seeds, which were arranged in a completely randomized design. Seeds were placed in 90 mm diameter Petri dishes containing a sheet of autoclave-sterilized Whatman paper (ref. 3645 Whatman, France) humidified with 5 ml of deionized and autoclave-sterilized water. Seeds were cold stratified in the dark for 7 days at 5°C and 30% relative humidity (0.61 kPa of Vapour-pressure deficit, VPD) in order to release any residual seed dormancy. After cold stratification, seeds were germinated in the dark at constant temperatures of 5, 10, 15, 20, 25, 32, and 35°C in 1.5 m3 useful-volume growth chambers (Froids et mesures, France). Temperature measurements from five to six thermocouples placed at different positions within each growth chamber, were logged every 20 s. Each growth chamber was regulated so that its temperature (average measurement from the thermocouples) did not exceed ± 0.2°C of the target temperature. The frequency of sampling for germination depended on temperature and the number of non-germinated seed within each Petri dish. Seeds were considered as germinated when either the radicle or the coleoptile had protrusion out of the seed and was at least 2 mm long. At each counting, germinated seeds were counted and removed from the Petri dishes. Deionized and autoclave-sterilized water was added as required to ensure moisture was non-limiting for germination.
Statistical Analysis
The data of germination percentages were analysed by two-way analysis of variance (ANOVA) and a post hoc Tukey’s test with significance level set at P < 0.05 was carried out to examine the effects of generation (i.e., cycle of selection), temperature and interaction of both factors on the divergent selection. The germination percentage of a Petri dish containing 100 seeds was considered as the basic datum. In addition, a model with population as random effect was performed to estimate genetic and residual variances at 10°C for the divergent selection at 10°C and at 32°C for the divergent selection at 32°C. The variances were used to calculate heritability as genetic variance divided by total variance. All calculations and statistical tests were carried out using R software (version 4.0.3; R Core Team, 2020).
Results
Two divergent selections were performed at two targeted temperatures: TA = 10°C and TA = 32°C and using the same optimum temperature TB = 25°C. The effect of the divergent selections at each generation are shown in Figures 3, 4 for the selection at TA = 32°C and the selection at TA = 10°C, respectively.
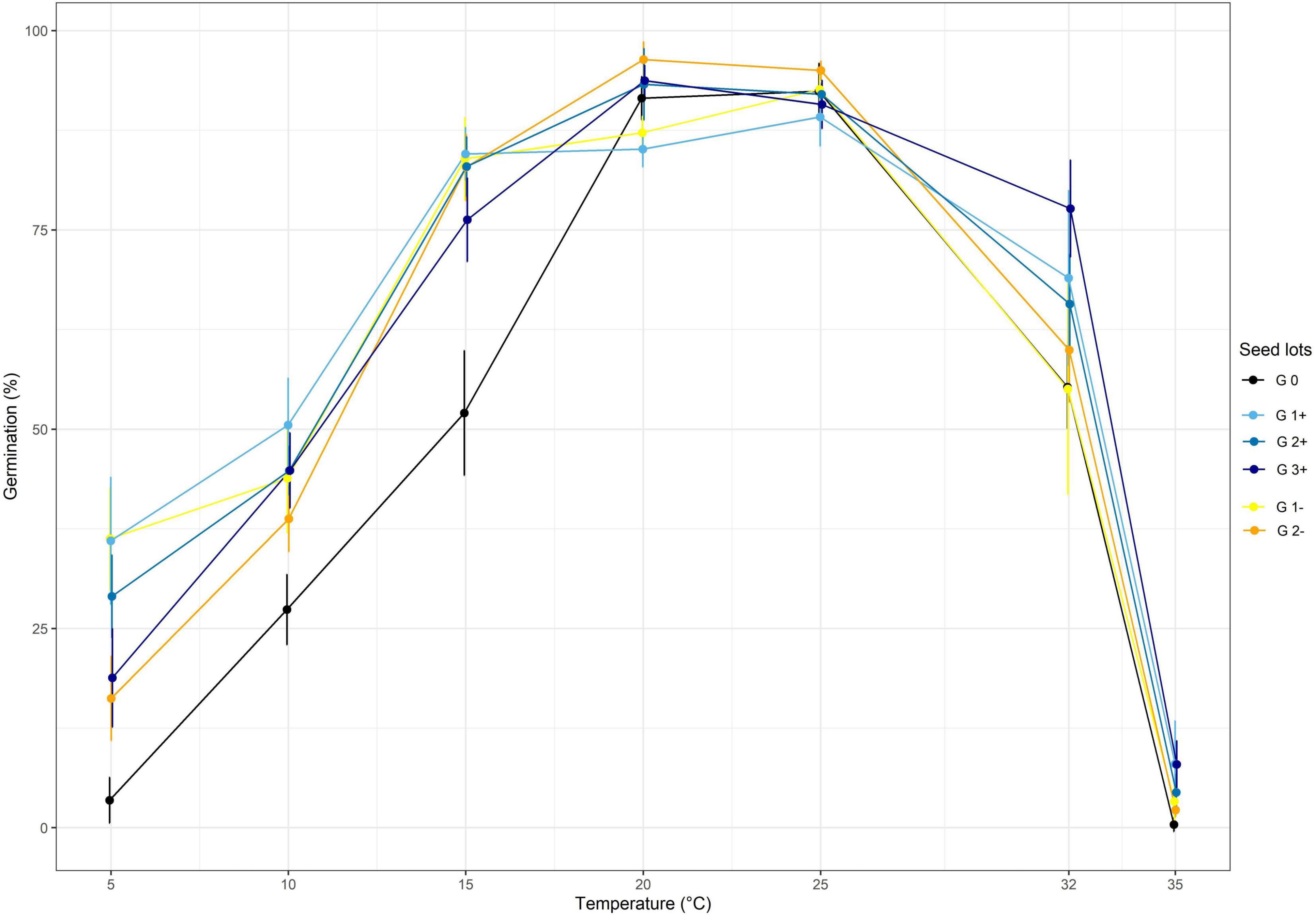
Figure 3. The effect of divergent selection at 32°C on the seed germination percentage of all the populations obtained from positive selection (G1+, G2+, G3+) and negative selection (G1-, G2-). G0 corresponds to the initial population.
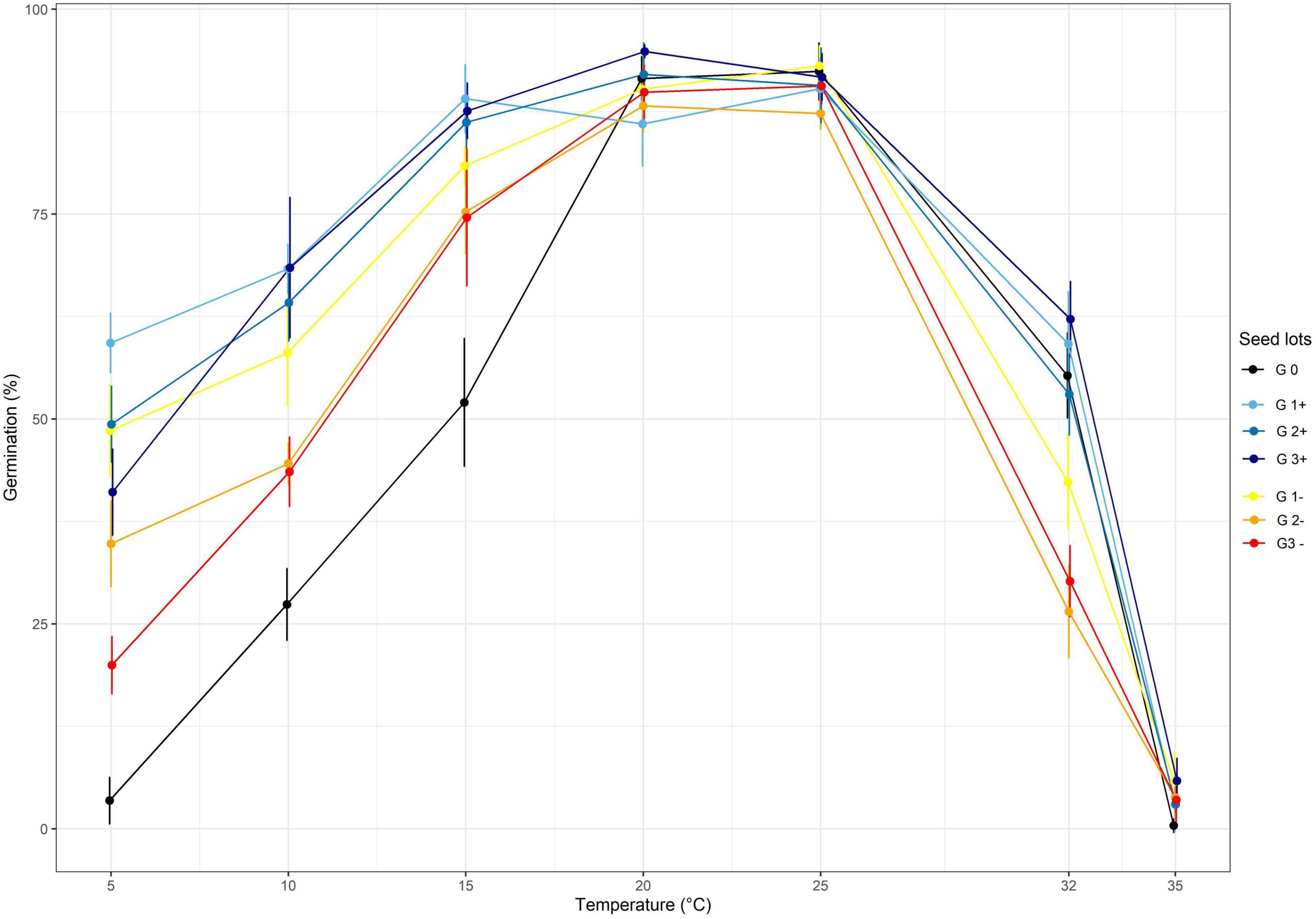
Figure 4. The effect of divergent selection at 10°C on the seed germination percentage of all the populations obtained from positive selection (G1+, G2+, G3+) and negative selection (G1-, G2-). G0 corresponds to the initial population.
In order to better assess the effect of selection, the germination capacity of the different populations resulting from the selection and the initial population, was evaluated in the range of temperatures from 5 to 35°C. The heritability were 89.7 and 87.7% for the divergent selection at 10 and 32°C, respectively.
Regarding the divergent selection at 32°C, significant effects of generations, temperature and the interaction of both factors on the germination percentage were established using a two-way ANOVA (Table 2 and Figure 3). A significant increase of germination percentage at 32°C was observed during the three cycle of PS going from 55.3% for G0 to 77.7% for G3+, showing a clear effect of selection (Supplementary Table 1). By contrast, the effect of the NS was not significant at 32°C. The first cycle of PS and NS at 32°C significantly increased the percentage of germination at temperatures below 20°C.
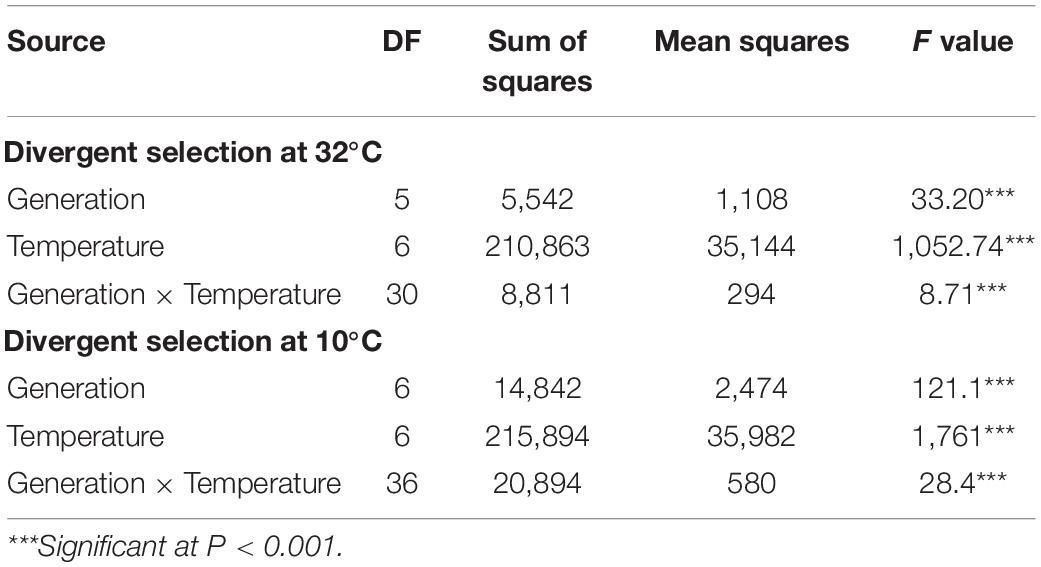
Table 2. Two-way ANOVA of the germination percentage used to evaluate the effect of divergent selection at 32 and 10°C.
Regarding the divergent selection at 10°C, significant effects of generations, temperature and the interaction of both factors on the germination percentage were established using a two-way ANOVA (Table 2 and Figure 4). An important significant increase of germination percentage at 10°C was observed during the first cycle of PS going from 27.4 to 68.3%, showing a clear effect of selection (Supplementary Table 2). Afterward, no significant increase of germination percentage at 10°C was observed during the two following cycles of selection. Surprisingly, we observed an increase of germination percentage at 10°C after the first cycle of NS. Then, as expected, the percentage of germination at 10°C decreased after the second cycle of NS but no significant effect of selection was observed after the third cycle of NS. The first cycle of PS and NS at 10°C significantly increased the percentage of germination at low temperatures: 5, 10 and 15°C. No effect of selection was observed at 20 and 25°C for which the percentage of germination was high (>85%) for all seed lots showing their good quality.
Discussion
This experimental study showed clearly that the ability to germinate at specific unfavourable temperatures could be selectively bred within a natural population of perennial ryegrass. This implies the existence of a genetic variability for the temperature at which the inhibition of seed germination is released. This variability could be essential for the adaptation of the population to inter-annual climatic variation e.g., some years it could be beneficial to germinate at 10°C even if in the majority of the years it is detrimental due to late frost. Moreover, in a longer term, this variability could allow adaptation to climate change. Few examples of within species genetic variability of the ability to germinate at different temperatures have been reported (Hampton et al., 1987; Palazzo and Brar, 1997; Lonati et al., 2009; Zhang et al., 2013; Nori et al., 2014; Butler et al., 2017; Ahmed et al., 2019; Ghaleb, 2019; Ahmed and Escobar-Gutiérrez, 2022; Ghaleb et al., 2021). In some cases, the genetic nature of this variability has been demonstrated by divergent selections like in alfalfa and maize for the ability to germinate at low temperatures (McConnell and Gardner, 1979; Landi et al., 1992; Klos and Brummer, 2000; Frascaroli and Landi, 2013).
Our results showed that the genetic determinism of the regulation of the release of seed germination inhibition involved at least one “major” gene at 10°C and several “minor” genes at 32°C. The first cycle of PS at 10°C was highly efficient with a very strong increase in the germination percentage (from 27.4 to 68.3%). However, afterward no selection effect was observed during the following two cycles of positive selection. These results could be explained by the presence of a dominant gene (D) inhibiting the germination of seeds at low temperatures. The allele frequency of the recessive allele (d) would be about 52% in the G0 population (square root of 27.4%: the percentage of individuals without any thermoinhibition of seed germination). The absence of response after the first cycle of selection could be explained by the fixation of the recessive (thermoinhibition of seed germination) allele. This “major” gene would explain only a part of the variability since 22–26% of the genotypes from G2+ and G3+ showed a thermoinhibition of seed germination despite the fact that they should be fixed at this “major” gene (dd). “Minor” genes and the environment could be involved in order to explain the thermoinhibition of seed germination of these genotypes. A “major” recessive gene could explain the fact that the varieties have generally lost this thermoinhibition of seed germination (only one generation of selection is needed). By contrast, the PS at 32°C showed a linear increase of the percentage of germination at 32°C (R2 = 0.79) despite a relatively stronger effect of the first cycle of selection. This result could be explained by the progressive accumulation of no thermoinhibition alleles at several loci.
The control of the temperature requirements for germination (i.e., thermoinhibition) at low temperatures by a “major” gene has been observed in different species. For example, the ability to germinate at 10°C of Lycopersicon esculentum seeds is controlled by a single recessive gene (Baskin and Baskin, 2014). In studies on the germination requirements of Avena fatua, A. ludoviciana and their hybrids, hypotheses were made regarding the number of genes involved in the temperature requirement for dormancy breaking. Failure of A. fatua to germinate at 5°C is probably controlled by three recessive loci, and failure of A ludoviciana to germinate at 18°C seems to be controlled by a single recessive gene (Whittington et al., 1970). In other studies, a major QTL has been identified for thermotolerance in lettuce and cucumber seeds (O’Brien, 2007; Argyris et al., 2008; Yoong et al., 2016; Yagcioglu et al., 2019). In maize, Hu et al. (2016) identified three QTL for optimum temperature germination rate and six QTL for low temperature germination rate.
The results on the NS were surprising. No consequent effect was observed except a large positive effect of the first cycle of selection at 10°C i.e., the percentage of germination at 10°C went from 27.4 to 58% whereas we selected for seeds unable to germinate at 10°C (Figure 4). This might be due to the fact that we selected the first seeds which germinated after their transfer from 10 to 25°C. Some of these individuals could be lagged not inhibited individuals which should have germinated at 10°C but for some reason were delayed. These individuals would carry the dd alleles.
In conclusion, we have demonstrated that thermoinhibition of seed germination at 10 and 32°C in a natural population of perennial ryegrass has a genetic basis and a single recessive gene seems to be involved at 10°C. It would be interesting to confirm this result in other natural populations showing a similar thermoinhibition at low temperatures. In addition, human selection has been efficient to suppress the thermoinhibition of seed germination so that seeds germinate in a wide range of temperature conditions. Nevertheless, it should be possible to select for the thermoinhibition of seed germination to avoid germination at unfavourable temperatures. For example, it could be interesting to select for varieties unable to germinate at high temperature so that these varieties could be sown at the end of the summer when the temperatures are still unfavourable for germination and that seeds germinate only when the temperatures decrease in autumn. At 10°C it would be helpful to develop a molecular marker in order to differentiate rapidly the Dd and DD genotypes showing both a phenotype with thermoinhibition of seed germination.
Data Availability Statement
The datasets presented in this study can be found in online repositories. The names of the repository/repositories and accession number(s) can be found below: https://data.inrae.fr/dataset.xhtml?persistentId=doi:10.15454/MO7RP9, 10.15454/MO7RP9.
Author Contributions
AE-G and PB conceived the study. AE-G, PB, and WG designed the experiment. WG collected the data with contributions of PB, AE-G, and LQA. WG and PB carried out the data analysis. PB, AE-G, and LQA contributed to write the manuscript. All authors contributed to the article and approved the submitted version.
Funding
This project has received funding from the CASDAR project REGATE from the French ministry of agriculture. WG was the recipient of a France-Libya Ph. D. Fellowship.
Conflict of Interest
The authors declare that the research was conducted in the absence of any commercial or financial relationships that could be construed as a potential conflict of interest.
Publisher’s Note
All claims expressed in this article are solely those of the authors and do not necessarily represent those of their affiliated organizations, or those of the publisher, the editors and the reviewers. Any product that may be evaluated in this article, or claim that may be made by its manufacturer, is not guaranteed or endorsed by the publisher.
Acknowledgments
We would like to thank the French ministry of Agriculture for the funding of this study included in the CASDAR project: REGATE involving the French Association of Forage Breeders (ACVF). We would also like to thank the technical staff of URP3F, in particular Cedric Perot, Magali Caillaud, Mélanie Despeigne, and Rémi Dupuis. WG was the recipient of a France-Libya Ph.D. Fellowship. We thank Julia Buitink for her expert comments on the manuscript.
Supplementary Material
The Supplementary Material for this article can be found online at: https://www.frontiersin.org/articles/10.3389/fpls.2021.794488/full#supplementary-material
References
Ahmed, L. Q., Durand, J. L., and Escobar-Gutiérrez, A. J. (2019). Genetic diversity of alfalfa (Medicago sativa) in response to temperature during germination. Seed Sci. Technol. 47, 351–356. doi: 10.15258/sst.2019.47.3.10
Ahmed, L. Q., Durand, J.-L., Louarn, G., Fourtie, S., Sampoux, J.-P., and Escobar-Gutiérrez, A. J. (2014). “Grassland science in Europe, Vol. 19–EGF at 50: the future of European grasslands,” in Proceedings of the 25th General Meeting of the European Grassland Federation, Aberystwyth.
Ahmed, L. Q., and Escobar-Gutiérrez, A. (2022). Analysis of intra-specific variability of cocksfoot (Dactylis glomerata L.) in response to temperature during germination. Acta Physiol. Plant. 43.
Argyris, J., Dahal, P., Hayashi, E., Still, D. W., and Bradford, K. J. (2008). Genetic variation for lettuce seed thermoinhibition is associated with temperature-sensitive expression of abscisic acid, gibberellin, and ethylene biosynthesis, metabolism, and response genes. Plant Physiol. 148, 926–947. doi: 10.1104/pp.108.125807
Argyris, J., Truco, M. J., Ochoa, O., McHale, L., Dahal, P., Van Deynze, A., et al. (2011). A gene encoding an abscisic acid biosynthetic enzyme (LsNCED4) collocates with the high temperature germination locus Htg6.1 in lettuce (Lactuca sp.). Theor. Appl. Genet. 122, 95–108. doi: 10.1007/s00122-010-1425-3
Baskin, C. C., and Baskin, J. M. (1988). Germination ecophysiology of herbaceous plant species in a temperate region. Am. J. Bot. 75, 286–305. doi: 10.1002/j.1537-2197.1988.tb13441.x
Baskin, C. C., and Baskin, J. M. (2014). Seeds: Ecology, Biogeography, and Evolution of Dormancy and Germination, 2nd Edn. San Diego, CA: Elsevier / Academic Press.
Batlla, D., and Benech-Arnold, R. L. (2015). A framework for the interpretation of temperature effects on dormancy and germination in seed populations showing dormancy. Seed Sci. Res. 25, 147–158.
Bewley, J. D. (1997). Seed germination and dormancy. Plant Cell 9, 1055–1066. doi: 10.1105/tpc.9.7.1055
Bewley, J. D., and Black, M. (1994). Seeds Physiology of Development and Germination, 3rd Edn. New York, NY: Plenum Press, 445.
Bewley, J. D., Bradford, K., Hilhorst, H., and Nonogaki, H. (2013). Seeds: Physiology of Development, Germination and Dormancy, 3rd Edn. New York, NY: Springer. doi: 10.1007/978-1-4614-4693-4
Blackmore, T., Thorogood, D., Skot, L., McMahon, R., and Powell, W. (2016). Germplasm dynamics: the role of ecotypic diversity in shaping the patterns of genetic variation in Lolium perenne. Sci. Rep. 6:22603. doi: 10.1038/srep22603
Blanco-Pastor, J. L., Manel, S., Barre, P., Roschanski, A., Willner, E., Dehmer, K. J., et al. (2019). Pleistocene climate changes, and not agricultural spread, accounts for range expansion and admixture in the dominant grassland species Lolium perenne L. J. Biogeogr. 46, 1451–1465. doi: 10.1111/jbi.13587
Bolaric, S., Barth, S., Melchinger, A. E., and Posselt, U. K. (2005). Genetic diversity in European perennial ryegrass cultivars investigated with RAPD markers. Plant Breed. 124, 161–166. doi: 10.1111/j.1439-0523.2004.01032.x
Brunel, S., Teulat-Merah, B., Wagner, M. H., Huguet, T., Prospéri, J. M., and Dürr, C. (2009). Using a model-based framework for analysing genetic diversity during germination and heterotrophic growth of Medicago truncatula. Ann. Bot. 103, 1103–1117. doi: 10.1093/aob/mcp040
Butler, T. J., Celen, A. E., Webb, S. L., Krstic, D. B., and Interrante, S. M. (2017). Germination in cool-season forage grasses under a range of temperatures. Crop Sci. 57, 1725–1731. doi: 10.2135/cropsci2015.10.0647
Chopra, R., Burow, G., Burke, J. J., Gladman, N., and Xin, Z. (2017). Genome-wide association analysis of seedling traits in diverse Sorghum germplasm under thermal stress. BMC Plant Biol. 17:12. doi: 10.1186/s12870-016-0966-2
Dias, P. M., Brunel-Muguet, S., Dürr, C., Huguet, T., Demilly, D., Wagner, M. H., et al. (2011). QTL analysis of seed germination and pre-emergence growth at extreme temperatures in Medicago truncatula. Theor. Appl. Genet. 122, 429–444. doi: 10.1007/s00122-010-1458-7
Durr, C., Dickie, J. B., Yang, X.-Y., and Pritchard, H. W. (2015). Ranges of critical temperature and water potential values for the germination of species worldwide: contribution to a seed trait database. Agric. For. Meteorol. 200, 222–232. doi: 10.1016/j.agrformet.2014.09.024
Frascaroli, E., and Landi, P. (2013). Divergent selection in a maize population for germination at low temperature in controlled environment: study of the direct response, of the trait inheritance and of correlated responses in the field. Theor. Appl. Genet. 126, 733–746. doi: 10.1007/s00122-012-2014-4
Ghaleb, W. (2019). Analyse de la Diversité Génétique de la Réponse Germinative à la Température de Populations de Lolium perenne L., Festuca arundinacea Schreb et Dactylis glomerata L. Ph.D. thesis. France: University of Poitiers.
Ghaleb, W., Ahmed, L. Q., Escobar-Gutiérrez, A. J., and Julier, B. (2021). The history of domestication and selection of lucerne: a new perspective from the genetic diversity for seed germination in response to temperature and scarification. Front. Plant Sci. 11:578121. doi: 10.3389/fpls.2020.578121
Hampton, J. G., Charlton, J. F. L., Bell, D. D., and Scott, D. J. (1987). Temperature effects on the germination of herbage legumes in New Zealand. Proc. N. Z. Grassl. Assoc. 48, 177–183. doi: 10.33584/jnzg.1987.48.1772
Hayashi, E., Aoyama, N., and Still, D. W. (2008). Quantitative trait loci associated with lettuce seed germination under different temperature and light environments. Genome 51, 928–947. doi: 10.1139/G08-077
Hills, P. N., and van Staden, J. (2003). Thermoinhibition of seed germination. South Afr. J. Bot. 69, 455–461. doi: 10.1016/S0254-6299(15)30281-7
Hu, S., Lübberstedt, T., Zhao, G., and Lee, M. (2016). QTL mapping of low-temperature germination ability in the maize IBM Syn4 RIL population. PLoS One 11:e0152795. doi: 10.1371/journal.pone.0152795
ISTA (2015). “Chapter 5- The germination test,” in International Rules for Seed Testing 2015, Vol. 2015, (Bassersdorf: International Seed Testing Association), i–5–56. doi: 10.15258/istarules.2015.05
Jiang, N., Shi, S., Shi, H., Khanzada, H., Wassan, G. M., Zhu, C., et al. (2017). Mapping QTL for seed germinability under low temperature using a new high-density genetic map of rice. Front. Plant Sci. 8:1223. doi: 10.3389/fpls.2017.01223
Jones, E. S., Mahoney, N. L., Hayward, M. D., Armstead, I. P., Jones, J. G., Humphreys, M. O., et al. (2002). An enhanced molecular marker based genetic map of perennial ryegrass (Lolium perenne) reveals comparative relationships with other Poaceae genomes. Genome 45, 282–295. doi: 10.1139/g01-144
Keep, T., Sampoux, J. P., Blanco-Pastor, J. L., Dehmer, K. J., Hegarty, M. J., Ledauphin, T., et al. (2020). High-Throughput genome-wide genotyping to optimize the use of natural genetic resources in the grassland species perennial ryegrass (Lolium perenne L.). G3 10, 3347–3364. doi: 10.1534/g3.120.401491
Klos, K. L., and Brummer, E. C. (2000). Response of six alfalfa populations to selection under laboratory conditions for germination and seedling vigor at low temperature. Crop Sci. 40, 959–964. doi: 10.2135/cropsci2000.404959x
Kubik, C., Sawkins, M., Meyer, W. A., and Gaut, B. S. (2001). Genetic diversity in seven perennial ryegrass (Lolium perenne L.) cultivars based on SSR markers. Crop Sci. 41, 1565–1572. doi: 10.2135/cropsci2001.4151565x
Landi, P., Frascaroli, E., and Lovato, A. (1992). Divergent full-sib recurrent selection for germination at low temperature in a maize population. Euphytica 64, 21–29. doi: 10.1007/BF00023534
Lonati, M., Moot, D. J., Aceto, P., Cavallero, A., and Lucas, R. J. (2009). Thermal time requirements for germination, emergence and seedling development of adventive legume and grass species. N. Z. J. Agric. Res. 52, 17–29. doi: 10.1080/00288230909510485
McConnell, R. L., and Gardner, C. O. (1979). Selection for cold germination in two corn populations. Crop Sci. 19, 765–768.
Nori, H., Moot, D., and Black, A. (2014). Thermal time requirements for germination of four annual clover species. N. Z. J. Agric. Res. 57, 30–37. doi: 10.1080/00288233.2013.863786
Nunes, F. P., Dayrell, R. L. C., Silveira, F. A. O., Negreiros, D., de Santana, D. G., Carvalho, F. J., et al. (2016). “Seed germination ecology in rupestrian grasslands,” in Ecology and Conservation of Mountaintop Grasslands in Brazil, ed. G. Fernandes (Cham: Springer). doi: 10.1007/978-3-319-29808-5_10
O’Brien, L. (2007). Lettuce (Lactuca sativa) Seed and Seedling Traits and Sclerotinia Disease Resistance: A Quantitative Trait Loci Analysis of a Recombinant Inbred Line Population. Master’s thesis. Davis, CA: University of California.
Ooi, M. (2012). Seed bank persistence and climate change. Seed Sci. Res. 22, S53–S60. doi: 10.1017/S0960258511000407
Palazzo, A. J., and Brar, G. S. (1997). The Effects of Temperature on Germination of Eleven Festuca Cultivars. Special Report 97-19. Hanover, NH: US Army Corps of Engineers. Cold Regions Research and Engineering Laboratory.
R Core Team (2020). A Language and Environment for Statistical Computing. Vienna: R Foundation for Statistical Computing.
Simpson, G. (1990). Seed Dormancy in Grasses. Cambridge: Cambridge University Press. doi: 10.1017/CBO9780511721816
Tribouillois, H., Dürr, C., Demilly, D., Wagner, M. H., and Justes, E. (2016). Determination of germination response to temperature and water potential for a wide range of cover crop species and related functional groups. PLoS One 11:e161185. doi: 10.1371/journal.pone.0161185
Whittington, W., Hillman, J., Gatenby, S., and Hooper, B. (1970). Light and temperature effects on the germination of wild oats. Heredity 25, 641–650. doi: 10.1038/hdy.1970.69
Wilkins, P. W. (1991). Breeding perennial ryegrass for agriculture. Euphytica 52, 201–214. doi: 10.1007/BF00029397
Yagcioglu, M., Jiang, B., Wang, P., Wang, Y., Ellialtioglu, S. S., and Weng, Y. (2019). QTL mapping of low temperature germination ability in cucumber. Euphytica 215:84. doi: 10.1007/s10681-019-2408-3
Yan, A., and Chen, Z. (2020). The control of seed dormancy and germination by temperature, light and nitrate. Bot. Rev. 86, 39–75. doi: 10.1007/s12229-020-09220-4
Yoong, F. Y., O’Brien, L. K., Truco, M. J., Huo, H., Sideman, R., Hayes, R., et al. (2016). Genetic variation for thermotolerance in lettuce seed germination is associated with temperature-sensitive regulation of ETHYLENE RESPONSE FACTOR1 (ERF1). Plant Physiol. 170, 472–488. doi: 10.1104/pp.15.01251
Zhang, H., McGill, C. R., Irving, L. J., Kemp, P. D., and Zhou, D. (2013). A modified thermal time model to predict germination rate of ryegrass and tall fescue at constant temperatures. Crop Sci 53, 240–249. doi: 10.2135/cropsci2012.02.0085
Keywords: divergent selection, germination, temperature, perennial ryegrass, Lolium perenne L., thermoinhibition
Citation: Ghaleb W, Barre P, Teulat B, Ahmed LQ and Escobar-Gutiérrez AJ (2022) Divergent Selection for Seed Ability to Germinate at Extreme Temperatures in Perennial Ryegrass (Lolium perenne L.). Front. Plant Sci. 12:794488. doi: 10.3389/fpls.2021.794488
Received: 13 October 2021; Accepted: 14 December 2021;
Published: 31 January 2022.
Edited by:
Petr Smýkal, Palacký University, CzechiaReviewed by:
Birte Boelt, Aarhus University, DenmarkJuan Pablo Renzi, Instituto Nacional de Tecnología Agropecuaria, Argentina
Copyright © 2022 Ghaleb, Barre, Teulat, Ahmed and Escobar-Gutiérrez. This is an open-access article distributed under the terms of the Creative Commons Attribution License (CC BY). The use, distribution or reproduction in other forums is permitted, provided the original author(s) and the copyright owner(s) are credited and that the original publication in this journal is cited, in accordance with accepted academic practice. No use, distribution or reproduction is permitted which does not comply with these terms.
*Correspondence: Philippe Barre, cGhpbGlwcGUuYmFycmVAaW5yYWUuZnI=