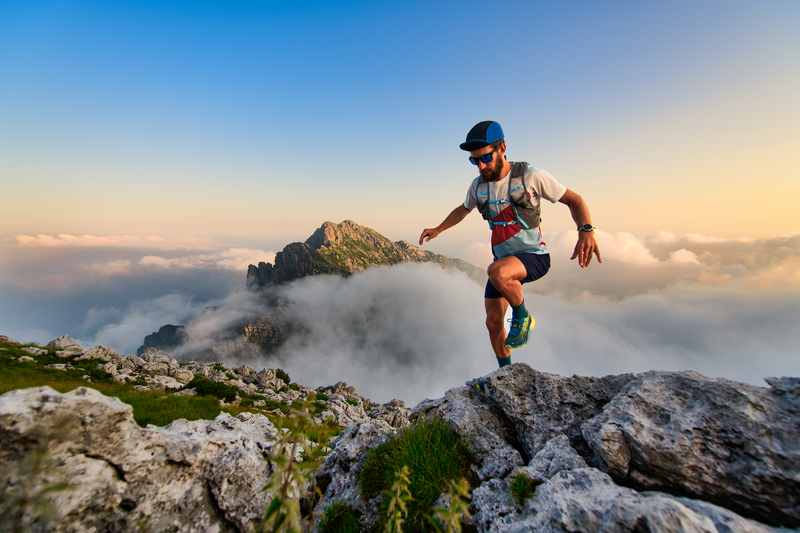
95% of researchers rate our articles as excellent or good
Learn more about the work of our research integrity team to safeguard the quality of each article we publish.
Find out more
ORIGINAL RESEARCH article
Front. Plant Sci. , 04 January 2022
Sec. Plant Systematics and Evolution
Volume 12 - 2021 | https://doi.org/10.3389/fpls.2021.790655
BRASSINAZOLE RESISTANT (BZR) are transcriptional factors that bind to the DNA of targeted genes to regulate several plant growth and physiological processes in response to abiotic and biotic stresses. However, information on such genes in Brassica napus is minimal. Furthermore, the new reference Brassica napus genome offers an excellent opportunity to systematically characterize this gene family in B. napus. In our study, 21 BnaBZR genes were distributed across 19 chromosomes of B. napus and clustered into four subgroups based on Arabidopsis thaliana orthologs. Functional divergence analysis among these groups evident the shifting of evolutionary rate after the duplication events. In terms of structural analysis, the BnaBZR genes within each subgroup are highly conserved but are distinctive within groups. Organ-specific expression analyses of BnaBZR genes using RNA-seq data and quantitative real-time polymerase chain reaction (qRT-PCR) revealed complex expression patterns in plant tissues during stress conditions. In which genes belonging to subgroups III and IV were identified to play central roles in plant tolerance to salt, drought, and Sclerotinia sclerotiorum stress. The insights from this study enrich our understanding of the B. napus BZR gene family and lay a foundation for future research in improving rape seed environmental adaptability.
Abiotic and biotic stresses are the two main environmental factors that influence plant development and quality throughout their life span. Transcriptional factors (TFs) play an indispensable role in mediating multiple hormonal signaling pathways to drive adaptive response against abiotic and biotic stresses. It is well known that a group of transcriptional factors, BRASSINAZOLE RESISTANT (BZR), bind to specific sequences to convey environmental stimuli in regulating plant tolerance to various stresses (Glazebrook, 2001). BZR, also named BRI1-EMS-SUPPRESSOR (BES), is a positive regulator of the brassinosteroid (BR) hormone. BR is the polyhydroxy steroid hormone required in various plant development processes, including photomorphogenesis, cell division, vascular differentiation, pollen tube formation, seed germination, reproduction, elongation, senescence, and exhibits response to various abiotic stresses (Zhu et al., 2013; Fridman et al., 2014; Wei and Li, 2016; Li et al., 2018). It is also noteworthy that the exogenous application of BR can act as an immunomodulator to improve plant tolerance against biotic stresses, including bacterial and pathogen infection (He et al., 2007; Chinchilla et al., 2009). BR mediates plant development through BRASSINOSTEROID-INSENTIVE 1 (BRI1), a membrane-localized receptor, which upon binding with BRI1-ASSOCIATED RECEPTOR KINASE (BAK1), directs the transcriptional activity of the BZR TFs (Li et al., 2001; He et al., 2002; Yin et al., 2002; Kim et al., 2011). BZR transcription family contains two homologous TFs, BRASSINAZOLE RESISTANT 1 (BZR1), and BRASSINOSTEROID INSENSTIVE 1-ETHYLE METHAESULFONATE SUPPRESSOR 1 (BES1/BZR2), which are known to be the central transcription factors in BR signaling. BZR TFs are mainly expressed in leaf, flower, root, and shoot (Li and Chory, 1999; Reise and Waller, 2009) and are localized in the nucleus to bind with the E-box (CANNTG) and BR-response (CGTGT/CG) cis-elements of one-quarter of BR-responsive genes (Yu et al., 2011; Zhang et al., 2014; Wu et al., 2016b). These interactions coordinate various aspects of molecular and cellular processes, including plant tolerance to abiotic stresses. For instance, BZR1 interacts with C-REPEAT/DRE BINDING FACTOR (CBF) to govern freezing tolerance in Arabidopsis thaliana (Li et al., 2017). At the same time, BZR2 directly binds with the promoter region of glutathione s-transferase-1 (GST1) to maintain plant growth under drought stress (Cui et al., 2019). Moreover, BZR1 also binds with FLOWERING LOCUS D (FLD) to regulate flowering (Zhang et al., 2013b). Furthermore, recent studies have also reported the interaction of BZR1/BZR2 with PHYTOCHROME INTERACTING FACTORS (PIFs), MAP KINASE 6 (MAPK6), WRKY TRANSCRIPTION FACTOR 54 (WRKY54), ATBS1-INTERACTING FACTOR 2 (AIF2), and transcription elongation factor IWS1 to mediate BR-related plant development and defense-related processes (Li et al., 2010; Oh et al., 2012; Kang et al., 2015; Chen et al., 2017; Kim et al., 2017; Jia et al., 2020). Subsequent studies indicate that BZR TFs also show higher affinity with transcriptional factors of multiple phytohormones. For instance, BZR1 and BZR2 interact with BRI1-like receptor gene 3 (BRL3) to suppress the transcriptional activity of the positive regulator of abscisic acid (ABA) to inhibit seed dormancy and increase flowering growth during the reproductive stage (Ryu et al., 2014; Yang et al., 2016). Additionally, BZR1 and BZR2 also bind with the negative regulator of gibberellins signaling to arbitrate plant growth in response to relentless stresses (Li et al., 2012). These molecular interactions between transcriptional factors of the different phytohormones evidenced the crosstalk between BR and multiple phytohormone signaling. However, the precise molecular mechanism of these synergies is mainly unknown.
In Arabidopsis, six members of the BZR gene family were identified, AtBZR1, AtBZR2, AtBEH1, AtBEH2, AtBEH3, and AtBEH4. The dominant mutants of bzr1-D and bes1-D displayed a delay in flowering with wider dark green leaves and showed upregulated expression of BR-responsive genes in A. thaliana (Wang et al., 2002; Yin et al., 2002). Additionally, a recent study also confirmed the function of BZR in light signaling pathways, which shows the interaction of BZR1 with light-regulated transcriptional factor HY5 in regulating plant photomorphogenesis (Li and He, 2016), whereas downregulation of wheat BZR2 displays sensitivity to drought stress (Cui et al., 2019). In contrast, BEH1-4 genes are thought to have a partially redundant function in BR signaling. For instance, in A. thaliana, the quadruple mutant (beh1, beh2, beh3, and beh4) did not exhibit any noticeable phenotype, while sextuple mutant (bzr1, bes1, beh1, beh2, beh3, and beh4) shows abnormal tapetum development, and male sterility (Chen et al., 2019a,b). However, a recent study listed the function of AtBEH4 in modulating embryonic stem development (Lachowiec et al., 2018), suggesting the redundant functions of the BZR gene family. However, there is insufficient progress in understanding the individual role of BEH1-4 genes, especially in abiotic and biotic stresses. Overall, significant progress has been made to systematically characterize the BZR gene family in Oryza sativa (Tong et al., 2012), Zea mays (Manoli et al., 2018), Brassica rapa (Saha et al., 2015), Glycine max (Zhang et al., 2016), Solanum lycopersicum (Liu et al., 2014), Petunia hybrida (Verhoef et al., 2013), Eucalyptus grandis (Fan et al., 2018), and other plant species (Table 1), which shows various functions of the BZR genes in regulating important agronomic traits including tillering, stress tolerance, and yield. However, no progress has been made to characterize the functional divergence of the BZR gene family and their regulatory mechanism in Brassica napus.
Brassica napus is one of the few dicot plants that have very valuable uses. Its oil-rich seed is utilized for industrial and nutritional purposes or as a protein-rich forage for animals (Friedt et al., 2018). However, B. napus growth is sensitive to a variety of biotic and abiotic stresses, including waterlogging, salt, drought, and Sclerotinia sclerotiorum infection (Xu et al., 2015; Sun et al., 2017; Hatzig et al., 2018; Sabagh et al., 2019), which significantly affects B. napus economic interest. Furthermore, its complex polyploid genome makes it challenging to understand the genetic variations underlying B. napus resistance to abiotic and biotic stresses.
This study comprehensively described the B. napus BZR gene family based upon their evolutionary relationship, structural features, and protein–protein interactions. Moreover, miRNA targets and promoter analyses were also performed. Additionally, expression patterns in response to salt and drought stress were also examined by qRT-PCR. The data present in our study showed that 21 BnaBZRs were scattered throughout 19 chromosomes of B. napus and organized into four subgroups. Functional divergence analysis among these groups evident the shifting of evolutionary rate after the duplication events. Organ-specific expression analysis of BnaBZR genes using RNA-seq data and qRT-PCR showed complex expression patterns in plant tissues (roots, mature siliqua, leaf, flower, flower-bud, stem, and seed) and during stress conditions. In which genes belonging to subgroups III and IV were identified to play central roles in plant tolerance to salt, drought, and S. sclerotiorum stress. In addition, we also explore some bna-miRNA, such as bna-miRNA395, bna-miR171, and bna-miR159, which are stimulated by the environmental cues, suggesting that different bna-miRNAs direct post-transcriptional regulation of BnaBZR gene family during biotic and abiotic stresses. Insights from these findings provide a framework for future studies in improving rape seed environmental adaptability.
The proteins sequences of the B. napus BZR genes were downloaded from the brassica genome browser GENOSCOPE1 (Chalhoub et al., 2014) and BnPIR2 (Song et al., 2020, 2021), respectively, by employing the six AtBZR genes from A. thaliana genome browser TAIR3 with corresponding Gene ID (At1G19350, At1G75080, At3G50750, At4G36780, At4G18890, and At1G78700). Furthermore, the amino acid sequences from B. oleracea and B. rapa were acquired from the Brassica database BRAD,4 using the AtBZRs as a query. The protein sequence with 80% sequence similarity was uploaded to ScanProsite5 (De Castro et al., 2006) and Pfam6 (Mistry et al., 2021) to search for the presence of the BZR1 domain in the sequence, protein sequences with lacking distinctive matching domains were eliminated. Moreover, multiple sequence alignment analysis was performed by aligning the 46 BZRs protein sequence from A. thaliana, B. oleracea, B. napus, and B. rapa to confirm the conserved domain. Physiochemical characteristics of the BnaBZRs proteins were investigated by the ProtParm7 tool (Gasteiger et al., 2005) and the cellular location was predicted by Plant-mPLoc8 (Chou and Shen, 2010).
The NJ (Neighbor-joining) method in MEGA X was utilized to plot the evolutionary tree. The tree’s validity was verified by performing 1,000 boot replications, and the Newick format of the phylogenetic tree was further annotated and visualized by the iTOL9 web server (Letunic and Bork, 2021). The genes structural features were recognized by uploading the coding and genomic sequences in the gene structure display server GSDS10 (Hu et al., 2015) and FGENESH (Solovyev et al., 2006). To further verify the intron/exon arrangement, we manually aligned the genomic and coding sequences of each BZR gene. Functional conserved motifs were examined by using the MEME11 program (Bailey and Elkan, 1994) with the minimum motif search configured to 20, and the rest of the settings left to default.
The genome feature file (GFF) of the B. napus genome was employed to inspect the chromosomal distribution of the 21 BnaBZRs, and the map was drawn using the TBtools program (Chen et al., 2020). Moreover, Gene duplication events in BnaBZR genes were performed using the MCScanX toolkit (Wang et al., 2012b). The collinear relationship between BnaBZR genes and BZR genes from B. oleracea, A. thaliana, and B. rapa were acquired by Dual Synteny Plotter function in TBtools. Moreover, positive and purifying selection of the BZR gene family was predicted by utilizing a Selecton Server12 (Stern et al., 2007). Besides this, the nonsynonymous (Ka) and synonymous mutation (Ks) of a single codon were also calculated by Ka/Ks Calculator 2.0 (Wang et al., 2010).
To determine the Type-I and Type-II functional divergence among clusters of the BnaBZRs, we used the DIVERGE 3.0 program (Gu et al., 2013), and Bayesian posterior probability Qk was set to 0.9 to predict the specific amino acid site. Protein 3D structure was generated by submitting the BnaA06BZR1 protein sequence into the I-TASSER server13 with default parameters (Yang et al., 2015), and specific amino acid sites were marked and visualized using the PyMOL software.14
Seeds of B. napus cultivar Zhongshuang 11 (ZS11) were obtained from the Oil Crops Research Institute of the Chinese Academy of Agricultural Sciences, Wuhan. The seeds were sprouted on moistened filter paper under the listed conditions: 70% relative humidity and 20 ± 5°C, 16 h light/8 h dark at a light intensity of 50 μmol/m2/s. To investigate the expression profile of BnaBZRs in B. napus, tissues from the adult plant, such as mature-siliqua, root, stem, seed, leaf, flower, flower-bud, and shoot-apex, were collected and stored at −80°C for further experiment. Furthermore, for salt treatment, the three-week-old seedlings were immersed into 400 mM sodium chloride (NaCl; received 100 mM NaCl per day). After reaching a final concentration (400 mM), the leaf samples were collected at 2, 8, and 16 h post-treatment, while for drought treatment, 25% PEG solution was applied to simulate a drought stress-like condition. The leaf tissues were harvested at 2, 8, and 16 h post-treatment. The collected leaves were instantly frozen into the LN2 (liquid nitrogen) and kept at −80°C. To extract RNA and synthesize cDNA, we employed the same procedure as described in our prior study (Sarwar et al., 2021). The synthesized cDNA of the individual sample was utilized as a template in the Thermo Fisher QuantStudio Real-Time PCR system with three separate biological replicates for qRT-PCR analysis. For internal control, the B. napus Actin gene (geneBank ID: XM013858992) was used. The expression patterns of BnaBZRs were measured by the 2−∆∆Ct method described by Livak and Schmittgen (2001), and a student t-test was performed to find the significant difference. The graph was generated using GraphPad Prism8.0 software (Swift, 1997). The qRT-PCR primers were designed according to the qPCR SYBR-Green Master Mix instruction and listed in Supplementary Table S12.
For protein–protein interaction analysis, the AtBZRs orthologs were searched in the STRING server15 (Szklarczyk et al., 2019), and the interaction map was drawn using the Cytoscape program. Moreover, to confirm the interaction of miRNA with BnaBZRs, we used the publicly available B. napus miRNAs from miRbase16 (Kozomara et al., 2019) and PNRD17 (Yi et al., 2015), and searched against the identified 21 coding sequences of BnaBZRs in psRNAtarget server18 (Dai and Zhao, 2011). Furthermore, cis-elements were investigated by using the 1.5 bp genomic promoter sequence of the individual BnaBZR gene and uploaded to the plantCARE database19 (Lescot et al., 2002). Additionally, the functional properties of the BnaBZRs were evaluated using the AtBZRs orthologs in DAVID (Huang et al., 2009a,b) and PANTHER (Mi et al., 2019) program, GO terms with significant value p < 0.05 were considered to be enriched in BZR genes.
The expression data of BnaBZRs under drought, salinity, cold, and S. sclerotiorum stress treatment were acquired from the publically available RNA-seq data sets (CRA001775; Lin et al., 2020) and (SRP311601; Walker et al., 2021). DSEeq2 package in R-studio was applied to measure the genes differential expression, the detected values were adjusted by log2 fold change method, and heatmap graph was created by the TBtools program. To locate the sequence polymorphism of the B. napus BZR gene family, the SNPs from the BnaBZRs of 159 accessions were extracted from the B. napus genomic browser BnPIR2 (Song et al., 2020, 2021), and the SNP distribution map was drawn using the TBtools.
The BZR gene family in A. thaliana and other plant species has been studied extensively. However, there are no studies available that have characterized the B. napus BZR genes in depth. Therefore, to examine the B. napus BZR genes, we obtained the peptide sequences of the AtBZR gene family (AtBZR1, AtBZR2, AtBEH1, AtBEH2, AtBEH3, and AtBEH4) from the A. thaliana genome browser and then used it as a query in BLASTP program in the B. napus genome browser GENOSCOPE (see footnote 1; Chalhoub et al., 2014). Moreover, the BnPIR (see footnote 2) B. napus genomic browser was also utilized to further verify the reliability of the BZR gene family, and redundant sequences were eliminated manually. A total of 21 BnaBZRs were recognized, including seven BnaBZRs and 14 BnaBEHs, which were named according to their loci (Supplementary Table S1). Simultaneously, seven and 12 BZRs were recognized in the genome of B. oleracea and B. rapa, respectively. This exhibits that the BnaBZR gene family might derive from their ancestor B. rapa and B. oleracea. Moreover, physicochemical properties of the BnaBZR proteins reveal that the instability index (II) was among 46.64–85.28, which shows that members of BnaBZRs were unstable. In addition, the isoelectric point (pI) values were between 5.14 and 9.81, indicating BnaBZRs were highly basic, and the negative GRAVY (grand average of hydrophobicity) value shows that these proteins are hydrophilic in nature. The amino acids encoded by the BnaBZR proteins are varied from 102aa to 400aa, in which the BnaA01BEH3 amino acid sequence was the shortest contained only 102 amino acids; however, the amino acid length of BnaC08BZR2-1 was the highest, around 400 amino acid residues. The molecular weight ranges from 10,990 KD to 44,156 KD, and the aliphatic index (AI) was between 48.12 and 73.3. Moreover, the subcellular localization signals of BnaBZRs were predicted by Plant-mPLoc. As transcriptional regulatory proteins, all BnaBZRs signals were detected in the nucleus (Supplementary Table S1).
To explain the phylogenetic relationship of the BnaBZRs, an evolutionary tree was generated by aligning the full-length coding sequence of six A. thaliana, 21 B. napus, 12 B. rapa, and seven B. oleracea BZR genes. As shown in Figure 1, all 47 genes were fell into four groups: group I carried BZR1 and BZR2 genes; group II contained BEH1; and group III contained BEH2 genes, while group IV contained BEH3 and BEH4 genes. Interestingly, the homologs of BEH3 and BEH4 were not detected in the B. oleracea related to those of A. thaliana, B. rapa, and B. napus indicating that the BZR gene family in B. oleracea might experience an evolutionary divergence in structural features. Additionally, the expansion of the BEH genes in A. thaliana, B. napus, and B. rapa take place in group II, III, and group IV due to whole-genome duplication. We also found that each BnaBZR gene shows higher homology with A. thaliana BZR genes. For instance, group I carried AtBZR1 and AtBZR2, along with BnaA06BZR1, BnaC05BZR1, and BnaC08BZR2-1, BnaC08BZR2-2, BnaC06BZR2, whereas BnaBEH genes were highly conserved with the A. thaliana BEH genes. Overall, in B. napus, group I, II, III, and IV had seven, two, four, and eight BZR family members, respectively. The genes cluster within the same subfamily displays a similar role in B. napus. Furthermore, to understand the diversity of BnaBZRs, Gene Structure Display (GSDS) analysis was performed using the coding and genomic sequence of the B. napus and A. thaliana. As shown in Figure 2, one to five exons were predicted in the BnaBZRs. Similar to the A. thaliana BZR gene family, most members in the BnaBZRs subfamily had the same number of exons and intron. For instance, most members of group I, group II, and group III have two to three exons and one to two introns, except for BnaA09BEH2, which contains four introns. Conversely, group IV contained one to three exons and only one intron, except for BnaA07BEH4, with no intron. Results from this analysis indicate that group I, II, and III might receive the original genes because, in the previous studies, it has been reported that the gain of introns is far slower than the intron loss during duplication (Nuruzzaman et al., 2010). Therefore, members in group IV might be derived from the original genes during evolutionary processes.
Figure 1. Phylogenetic relationship of the BZR gene family from Brassica napus (Bna), Arabidopsis thaliana (At), B. rapa (Bra), and B. oleracea (Bol). Neighbor-joining (NJ) tree was constructed using the MEGA X with 1,000 bootstrap replication and visualized using online tool iTOL. Members in the BZR gene family clustered into the four groups and specified with different colors.
Figure 2. Gene structure analysis of the B. napus and A. thaliana BZR gene family. Each BZR gene subfamily indicated as different color in Y-axis, while the X-axis represented the lengths of the exons and introns.
Our study observed that the B. napus BZR gene family was grouped into four groups. To determine the potential functional divergence between groups, we tested the Type I (ϴI) and Type II (ϴII) functional divergence utilizing the CodeML package in DIVERGE v2.0 (Gu and Vander Velden, 2002). For this analysis, we use group I, III, and IV members, while group II was excluded because it comprises only two members of the BnaBZRs. The results indicate that the all-divergence coefficients of Type I among group I/III, group I/IV, and group III/IV were statistically significant, with the ϴI and LRT values varying from 0.99 to 0.646 and 0.86 to 33.9, respectively, indicating that the amino acid sites among different groups of BnaBZR gene family have altered evolutionary constraints, which lead to group-specific functional evolution after divergence (Table 2). In contrast, the coefficients of Type II among group I/IV and group III/IV were also statistically significant with an ϴII value of 0.319 and 0.082, respectively, indicating the radical shift in amino acids properties of these pairs during evolution. However, the coefficient of ϴII among group I/III was not significant. We also found some crucial amino acid residues sites that may affect the functional divergence of the B. napus BZR gene family. To reduce false-positive results, posterior probability (Qk > 0.9) was selected to detect specific amino acid residues among the BnaBZR family (Table 2). The results predict 21 similar Type-I sites among group I/III and group I/IV. In comparison, only two sites were examined in group III/IV, implying that significant functional divergence might exist in group I/III and group I/IV as compared to group III/IV. Conversely, no Type-II functional divergence sites were predicted in group I/III and group I/IV. However, three sites occurred in group III/IV, of which 99T, 100R, and 155S were examined in both ϴI and ϴII, indicating the altered selective constraints may happen at these sites.
To predict the three-dimensional structure of the BnaBZR proteins, we utilized the representative BnaA06BZR1 protein sequence in the I-TASSER database (Zhang, 2008). The normalized C-score for the predicted model was > −3, suggesting the best template for the BnaBZR1 protein 3-D structure prediction. As shown in Figure 3, we found that the 3D structure of the BnaA06BZR1 contains nine α-helicases and one β-strands, in which the BZR1 domain has only four α-helicases. Furthermore, important amino acid sites, which take part in the functional divergence, were also displayed on the three-dimensional structure of the BnaA06BZR1. Out of 21 amino acid residues, only 14 were dispersed on the BZR1 domain, and the remaining eight sites were spread on the C-terminal region of the BnaA06BZR1, which indicates the BZR1 domain might be more vulnerable to positive selection during the evolution of the BnaBZR gene family. Additionally, amino acids residues 19T and 20R that are present in both ϴI and ϴII functional divergence were located on the N-terminal BZR domain, indicating the essential role of these sites in the evolution of the B. napus BZR gene family (Figure 3).
Figure 3. 3D model of the BnaA06BZR1 protein. The BZR1 domain was highlighted in pink, and the yellow color indicates the specific amino acid sites that are involved in functional divergence.
The full-length 42 coding sequences of the BZR proteins from B. oleracea, B. rapa, A. thaliana, and B. napus were employed to perform the multiple sequence alignment analysis to predict the highly conserved amino acids sites and examine the structural features of the BnaBZR gene family (Supplementary Figure S1). Based on the alignment, we found that six AtBZRs showed the highest percent amino acid homology with BnaBZRs (Supplementary Table S2). Furthermore, a highly conserved domain BRASSINAZOLE RESISTANT1 (BZR1) was identified in the N-terminal region of the BnaBZRs and other denoted plant species (Supplementary Figure S1). In the previous studies, it has been reported that the N-terminal BZR1 domain contains the serine-rich phosphorylation site and participates in modulating the expression of targeted genes to promote various plant physiological processes, such as reproduction, senescence, cell elongation, and cell division (Clouse et al., 1996; Ye et al., 2010). Overall, the domain distribution in B. napus and A. thaliana was similar (Figure 4). To further classify the diversification of the B. napus BZR gene family, we built a graph presenting 20 putative motifs and their distribution on BnaBZRs (Figure 4). Our results showed that all BnaBZRs contain five to 15 highly conserved motifs within a length range from eight to 50 amino acids. In which motif, 1, 3, 4, 5, and 11 encoded a BZR1 domain (Figure 4). The consensus sequences of these motifs were also shown in (Supplementary Figure S2). All BnaBZRs contain motif 5, except for BnaC03BEH3 and BnaC06BEH4, while other remaining motifs existed in some but not in all members (Figure 4). For instance, motif 8 and 10 were only present in group I, II, and III, whereas motif 7 and 18 are only found in group IV, except for BnaC03BEH3 and BnaA01BEH3 lacking motif 7. Furthermore, motif 19 was only detected in BnaC08BZR2-1 and BnaA09BZR2. In contrast, motif 20 was only found in AtBEH4, BnaA07BEH4, and BnaC06BEH4. Motif 12 and 16 were only present in group I, except for BnaA09BZR2 and BnaA07BZR2. However, the structure and motif distribution within the same group were similar, suggesting similar biological functions among the same group (Figure 4).
Figure 4. Schematic representation of conserved motifs distribution among B. napus and A. thaliana BZR gene family. The 20 conserved motifs are indicated by different colored boxes and numbers, the logo of each motif was presented in Supplementary Figure S2. The MEME score for the protein match to the motif model was equals to <1 × 10−20. The schematic illustration of BZR domain at the N-terminal regions of the BnaBZRs was a set of motifs.
To predict the distribution of BnaBZRs in B. napus chromosomes, we mapped the 21 BnaBZR members into the 19 B. napus chromosomes (Figure 5A). Based on their physical position, we found that members of the BnaBZR have distributed unevenly between B. napus chromosomes. In which chromosome, A07, A01, CO1, C06, and C08 contain two members of BnaBZR. Chromosomes A03 and C07 hold three members of the BnaBZR, while chromosomes A02, A06, A09, C03, and C05 have only one BnaBZR gene (Figure 5A). Furthermore, the MCScanX tool was also implemented to reveal the duplication of BnaBZRs in B. napus; 29 segmental duplication pairs were identified using this method. However, no tandem duplication pair was detected, implying that segmental duplication events played the central driving role in the functional divergence of BnaBZRs during B. napus genome duplication (Figure 5B). Moreover, genome duplication variation between four Brassicaceae species A. thaliana, B. napus, B. oleracea, and B. rapa was also investigated (Figure 6). The results show that B. napus contains both orthologous and paralogous copies of BZR genes. For instance, six AtBZR genes (AT1G19350, AT1G75080, AT3G50750, AT4G36780, AT4G18890, and AT1G78700) have two, five, two, four, five, and three orthologous copies in the B. napus genome, respectively (Supplementary Table S3). Moreover, the orthologous copies of BZR genes from the B. napus ancestor were also predicted. A total of 12 and 13 BZR genes from B. rapa and B. oleracea showed a syntenic relationship with BnaBZRs, respectively. These orthologous copies might be the reason for the distribution and functional diversification of the B. napus BZR gene family. In addition, we also measured the nonsynonymous (Ka) and synonymous (Ks) ratios for each pair of duplicated BnaBZR proteins (Supplementary Table S4). The results showed that all BnaBZR pairs contain the Ks/Ka ratio < 1, suggesting that BnaBZRs practiced a robust purifying selection (Supplementary Figure S3). However, there are some limitations in calculating the Ka/Ks ratio of the BnaBZRs, because the members of the BnaBZR have minute variations in their sequences, which may bring Ka/Ks value to lower than 1 (Nielsen and Yang, 1998; Nekrutenko et al., 2002). Therefore, we used the site models to predict the selection pressure on single amino acid codons (Yang, 2007; Supplementary Table S5). Model MO is one ratio model, which presumes the same ratio ɯ at all amino acid sites. In our study, we predicted the ratio value ɯ = 0.228 in M0, suggesting the strong purifying selection was the main reason for the evolution of the BnaBZRs. Furthermore, we also compared the model M0 and M3 to predict dN/dS ratio differentiation among codon sites. Interestingly, the log-likelihood 2∆InL was statistically significant (p < 0.01), indicating BnaBZR experienced immense selective pressure across different sites. Additionally, the models M7 (beta) and M8 (beta + ɯ > 1) were also compared, which considered a very stringent test of positive selection (Anisimova et al., 2001), to predict whether positive selection involves promoting divergence of BZR gene family in B. napus. However, no positive selection sites were identified. The results from this analysis indicate that the BnaBZR gene family underwent divergent selective pressure during duplication (Supplementary Table S5).
Figure 5. Chromosomal location and genome duplication of BnaBZRs. (A) Distribution of BnaBZRs on B. napus chromosomes. Genes from the same group are indicated by the same highlighted color. (B) Synteny analysis of BnaBZRs in B. napus. Lines with same color belong to the same BnaBZR gene subfamily indicate segmental duplication in B. napus chromosomes. Gray lines in the background indicated as B. napus genome synteny blocks. Number of each B. napus chromosome is represented as different colored box with the scale size is in Kb, and the outer circle represents the gene density profile of each chromosome.
Figure 6. Colinear gene pair analysis of BnaBZRs in B. napus in comparison with A. thaliana, B. rapa, and B. oleracea. The red lines indicate the collinear BZR gene pairs, and the gray lines indicate the collinear pairs within in the A. thaliana, B. napus, B. rapa, and B. oleracea genome.
Protein interaction study provides an essential insight to examine gene function in a systematic study (Pellegrini et al., 2004). Our analysis predicted that the BnaBZR proteins are highly homologous to the A. thaliana BZR proteins. Therefore, to further examine the interacting relationship of the BnaBZRs with its targeting partners, we constructed a network using the AtBZRs orthologs. As shown in Figure 7, we found that the members in the group I interact with more targeted proteins than others. BES1-INTERACTING MYC-LIKE1 (BIM1), BRI1 KINASE INHIBITOR 1 (BKI1), ARABIDOPSIS MYB-LIKE 2 (MYBL2), and PHYTOCHROME INTERACTING FACTOR 4 (PIF4) were predicted to bind with BnaBZR1, BnaBZR2, and BnaBEH1, while PHYTOCHROME B (PHYB) was only found to interact with BnaBZR2 and BnaBEH1 (Supplementary Table S6). However, the biological functions associated with their interaction are still unclear. In contrast, BRASSINOSTEROID-INSENSITIVE 2 (BIN2), BRASSINOSTEROID-INSENSITIVE 1 (BRI1), and BRI1 SUPPRESSOR 1 (BSU1) only interact with BnaBZR1 BnaBZR2, BnaBEH1, BnaBEH2, and BnaBEH4, whereas PHYTOCHROME INTERACTING FACTOR 3 (PIF3), REPRESSOR OF GA (RGA1), RGA-LIKE PROTEIN 3 (RGL3), and AUXIN RESPONSE FACTOR 6 (ARF6) are found to interacts with BnaBZR1 and BnaBZR2. In previous studies, it has been reported that the interaction of BZR1 with ARF6 and PIF mediates auxin-induced plant growth in response to environmental stresses. However, under constant stress conditions, gibberellin negative regulator RGA binds with the BZR1 to inhibit its transcriptional activity (Oh et al., 2014). Additionally, SLEEPY1 (SLY1) and TOPLESS (TPL) are only found to interact with BnaBZR2. Similarly, GA INSENSITIVE DWARF1B (GID1B) shows only interaction with BnaBEH2, which indicates the possible role of BnaBEH2 in regulating BR and GAs crosstalk in response to stresses. It is also worth noting that BnaBEH3 displays no interaction with other proteins (Figure 7). This analysis provides significant clues to understand the relation of the BnaBZRs with unknown proteins in different signaling pathways.
Figure 7. Protein interaction analysis of the BnaBZRs according to the Arabidopsis orthologs. Thicker lines represent the stronger interaction.
To further examine the individual role of BnaBZR members and how these genes respond to different stresses, we investigated the cis-regulatory elements in the promoter sequences of the BnaBZRs. It has been proposed that the homologs genes with similar roles might consist of the same cis-regulatory elements in their promoter regions. As shown in Supplementary Figure S4, a set of cis-elements belonging to the plant development, stress and defense, and hormonal response-related elements were detected (Supplementary Table S7), but their numbers and distribution were uneven in all BnaBZRs (Supplementary Figure S4A). For instance, BnaC01BEH3, BnaA03BEH2, and BnaC07BEH2 contained the highest number of development-related, hormone-responsive, and light-responsive cis-elements, respectively. In contrast, BnaA06BZR1 contains many stress and defense-related cis-regulatory elements (Supplementary Figure S4B). Nearly, all of the BnaBZRs hold the basic promoter elements including, CAAT-box and TATA-box. However, several core cis-elements were only being seen in a few BnaBZRs. For instance, Sp1 (light-responsive cis-element) and TGA-box (cis-element take part in ABA responsiveness) were only found in BnaA06BZR1 and BnaC05BZR1. A-Box (cis-element involved in the development process), CCGTCC-Box (cis-acting element activates the meristem development), and DRE-core (regulates drought and cold-responsive gene expression) were detected in BnaC05BZR2, BnaC08BZR2-1, and BnaC06BZR2. Furthermore, OCT (cis-element involved in cell proliferation), GCN4-motif (cis-element involved in nitrogen-related response), chs-CMA2a (light-responsive element), and DRE1 (regulates drought and cold-responsive gene expression) were detected in BnaC06BZR2, BnaC07BEH1, and BnaA01BEH2, respectively. GATT-motif, SARE (cis-element involved in salicylic acid response), 3-AF1 binding site (light-responsive cis-element), CARE (involved in ABA response), and LAMP-element were absent in all BnaBZRs except in BnaC08BZR2-2, BnaA07BZR2, BnaA03BEH1, BnaC07BEH2, and BnaC07BEH3, respectively. Similarly, AT1-motif, Box-III, motif-I, and CTAG-motif were only found in BnaC01BHE3. In addition, CAG-motif, ATC-motif, and dOCT (cis-element involved in cell cycle responses) were detected in BnaC01BEH2 and BnaC03BEH3. Overall, light-responsive cis-elements were the most abundant in all BnaBZRs promoters, which indicates the transcriptional activity of the BnaBZRs might be induced by light. Additionally, cis-elements related to hormone responses, such as auxin (AUX), gibberellins (GA), ABA, and salicylic acid (SA), were predicted, in which cis-element involved in ABA responses were the most common in all BnaBZRs. Moreover, stress and defense responsive cis-elements, including ARE (anaerobic regulatory element), WUN-motif (regulates wound-related response), LTR (associated with cold response), and STRE, which activated by the several stress condition, especially heat shock, nutrient deficiency, and osmotic stress were also predicted. Results from this analysis showed that the BnaBZRs contain various kinds of development and stress-related responsive elements, indicating that the BnaBZRs might control plant physiology in response to several phytohormones and environmental stresses.
To further explore the potential functions of the BnaBZR gene family, we study their expression patterns in cotyledon, leaf, silique, root, petal, bud, lower stem, sepal, vegetative rosette, pollen, upper stem, middle stem, seed, and filament of the B. napus variant Zhongshuang 11 (ZS11), by using the transcriptomic data available in BnTIR database http://yanglab.hzau.edu.cn/BnTIR. As shown in Supplementary Figure S5, 17 members of the BnaBZR gene family exhibited higher expression in sepal, petal, seed, lower stem, middle stem, upper stem, root, vegetative rosette, cotyledon, filament, and siliqua, while no or mild expression was observed in pollen and bud (Supplementary Table S8.1). In detail, pairs of homologs genes BnaC08BZR2-1/BnaC08BZR2-2, BnaA09BZR2, and BnaA07BZR2/BnaC06BZR2 showed higher expression in sepal, petal, and seed. In contrast, BnaA01BEH2, BnaA03BEH3, BnaC07BEH3, and BnaA02BEH4 higher expressions were observed in cotyledon and siliqua. Nevertheless, BnaA06BZR1 and BnaC05BZR1 display relatively lower expression in four tissues (root, sepal, upper stem, and vegetative rosette; Supplementary Figure S5). To further validate the organ-specific expression of the BnaBZRs, the relative expression pattern of the six putative BnaBZRs was determined in eight tissues of B. napus cultivar Zhongshuang 11 (ZS11) including mature-silique, shoot-apex, seed, leaf, flower-bud, flower, stem, and root by qRT-PCR. As shown in Figure 8, two of six BnaBZRs (BnaA06BZR1 and BnaC06BZR2) were consistently expressed in all selected tissues, except for BnaA06BZR1, which shows relatively lower expression in flower-bud. Additionally, the increased expression level of the BnaCO6BZR2, BnaC07BEH1, BnaA03BEH3, and BnaA02BEH4 was mainly observed in the mature siliqua (Figure 8, Supplementary Table S8.2). In contrast, BnaC07BEH2 was expressed highly in the leaf. Combined with transcriptomic data, the expression pattern of the BnaC07BEH1, BnaA03BEH3, and BnaA02BEH4 was also observed in the siliqua. However, the correlation test between the two datasets showed a non-significant association (r = 0.435, p = 0.713). The non-significant association between transcriptome data and qRT-PCR might be related to harvesting of siliqua at 38 and 44 days after flowering, respectively. Therefore, the fold change should not be expected to have same for both datasets. Overall, most members of the BnaBZRs expressed differently in the plant tissues, suggesting the key roles of BnaBZRs in various tissues during plant development.
Figure 8. Organ-specific expression pattern analysis of selected BnaBZRs in different tissues. The expression abundance of the specified BnaBZRs was adjusted with respect to the B. napus Actin gene. Tissues names are shown on the x-axis. The error bars on the y-axis represent the standard error of three independent biological replicates (listed in Supplementary Table S8.2).
To gain further insights into the BnaBZR gene family in response to the environmental stresses, we investigated their expression levels in drought, salinity, cold, and S. sclerotiorum stresses using publicly available RNA-seq data (CRA001775 and SRP311601). As shown in Supplementary Figure S6A, we found that the expression level of group I and group II was downregulated after 2 h of the drought treatment (Supplementary Table S9.1). Nevertheless, the expression pattern of the BnaA03BEH3 and BnaC06BEH4 were induced. In contrast, the expression pattern of the four BnaBZRs (BnaA07BZR2, BnaC06BZR2, BnaA03BEH3, and BnaC07BEH3) was peaked at 8 h of drought treatment. Under salinity treatment, the expression pattern of the BnaA03BEH3 and BnaC07BEH3 was first induced at 4 h but then reduced in 24 h of salinity treatment. Furthermore, under cold treatment, the RNA transcripts of the group IV members BnaC03BEH3, BnaA02BEH4, and BnaA07BEH4 were upregulated during 24 h of cold treatment. However, BnaA07BEH4 and BnaC06BEH4-induced expression was only observed in S. sclerotiorum infection (Supplementary Figure S6B). To elucidate the functions of the BnaBZRs in abiotic stresses, we monitored their expression changes by qRT-PCR in leaves of B. napus cultivar (ZS11) treated with different stresses, such as drought and salinity (Figure 9). On the whole, the expression patterns of the BnaBZRs were significantly changed during 8–16 h of drought and salt treatment (Supplementary Table S9.2). During salt treatment, BnaA06BZR1, BnaC06BZR2, and BnaA02BEH4 were dramatically induced at 8 h and reduced at 16 h, while BnaC07BEH1, BnaA03BEH3, and BnaC07BEH2 showed downregulation in their expression patterns, except for BnaC07BEH2, which showed upregulated expression at 16 h of drought treatment. Furthermore, expression patterns of the BnaC07BEH2 and BnaA02BEH4 were significantly increased at 8 h but then reduced at 16 h of NaCl treatment, indicating the expression patterns of the BnaC07BEH2 and BnaA02BEH4 were induced in more than one stress. In our, organ-specific expression analysis, we observed the different expression patterns of the BnaBZRs in various tissues (Supplementary Figure S5, Figure 8). Therefore, it is possible that the BnaBZR gene family in B. napus responded to drought, salinity, cold, and S. sclerotiorum stress might play a diverse regulatory function in various tissues.
Figure 9. The relative expression analysis of the BnaBZRs under different treatments. The expression abundance of the selected BnaBZRs was controlled with respect to the reference gene (Actin). The x-axis corresponds to different treatments, and values on the y-axis are denoted as the mean ± SE of three biological replicates (listed in Supplementary Table S9.2). Asterisk on vertical bar represent a significant difference at *p < 0.05 and **p < 0.01.
MicroRNAs regulate gene expression by cleaving the mRNA of the targeted genes in response to stresses (Song et al., 2019). Identifying the miRNA-targeted genes employing the computational approaches is vital for predicting the transcriptional regulatory role of the gene family. Therefore, to understand the transcription network and post-transcriptional regulation of the BnaBZRs, we examine the potential miRNA target sites using the psRNATarget database. Out of the 21 BnaBZRs, only nine genes were targeted by 12 novel bna-miRNA, with an average of one to two targets per bna-miRNA (Table 3). The two members of group I were predicted to be targeted by three novel bna-miRNA (bna-miR395, bna-miR171, and bna-miR170), whereas six members of group IV were targeted by the bna-miR158, bna-miR164, bna-miR159, bna-miRN273, and bna-miRN292. In addition, only one member of group II (BnaC07BEH1) was targeted by the bna-miRN291 and bna-miRN285. However, except for the bna-miRN285/BnaC07BEH1 and bna-miR164/BnaA02BEH4, the majority of the bna-miRNA-targeted genes was expected to be silenced by the cleavage inhibition. Furthermore, we have also predicted the UPE value, which is within 8.94–21.43, needed to unpair the secondary structure around the target site. Nevertheless, most of the bna-miRNA identified in this study, such as bna-miRNA395 (Huang et al., 2010; Zhang et al., 2013a), bna-miR171 (Jian et al., 2016), and bna-miR159, are stimulated by the environmental cues, suggesting that different bna-miRNAs direct post-transcriptional regulation of some of the members of the BnaBZR gene family during biotic and abiotic stresses.
It is well reported that a family of transcriptional factors BZR interacts with several sequences to regulate plant tolerance to environmental cues. To further examine the biological role of BnaBZRs, we conducted the gene ontology (GO) enrichment analysis. Our results showed that a total of 43 GO terms were categorized into three groups, BP (biological process), CC (cellular component), and MF (molecular function; Supplementary Table S10). Among BP category, all BnaBZRs were within the “metabolic processes (GO:1901360, GO:0006725, GO:0034641, GO:0046483, GO:0006807),” “biosynthetic processes (GO:1901576, GO:0034645, GO:0009059, GO:0009058),” “gene expression (GO:0010467),” and “cellular process (GO:0050794),” whereas BnaC05G0163000ZS, BnaA06G0135100ZS, BnaA02G0233100ZS, BnaA01G0099000ZS, and BnaC03G0707200ZS might be involved in plant tolerance to stresses (GO:0006950, GO:0006952, GO:0006955, GO:0009607, and GO:0009617). Under the CC category, all BnaBZRs were in the nucleus (GO:0005634), while some of the genes, such as BnaA09G0609500ZS, BnaC08G0463100ZS, BnaC05G0163000ZS, BnaA06G0135100ZS, BnaC06G0273700ZS, BnaA07G0248700ZS, BnaC08G0253000ZS, BnaA03G0416600ZS, and BnaC07G0389100ZS, were also predicted in the cytosol (GO:0005829) and cytoplasm (GO:0005737) category, respectively. Additionally, in the MF category, all BnaBZRs were significantly enriched in binding (GO:0003677, GO:0003700, and GO:1901363) together with “organic cyclic compound binding (GO:0097159)” (Supplementary Figure S7). Results from this analysis displayed the diverse roles of the BnaBZRs in various biological processes, mainly in cellular and metabolic processes and biological process regulation.
To locate the sequence polymorphism of the BnaBZRs, we used the sequencing data of 159 accessions of rape seed from the B. napus genomic browser [BnPIR (Song et al., 2020, 2021)]. On the whole, 55.5% of SNPs were predicted in the BnaBZR gene family (Supplementary Table S11). However, the SNP percentage frequency on each subfamily was dissimilar. For instance, group I, II, III, and IV hold an average of 49, 18, 39.2, and 64.8 SNPs, respectively. For BnaA07BEH4 and BnaC06BEH, no SNP was found. Meanwhile, we also observed that the SNPs density of the BnaBZRs on CC chromosomes was relatively higher than the AA chromosomes. Furthermore, a detailed SNPs distribution on the coding and non-coding regions of the BnaBZR gene family was also shown (Figure 10), which display that the distribution of SNPs in introns is varied between 9.5–52.8%, in which the highest number of SNPs (224) was found in the intron region of BnaC03BEH3. However, in exons, the distribution of SNPs was 8.5–20.14%, whereas the lowest number of SNPs (4) was found in the exon region of BnaA03BEH1. Results from this analysis indicate that the sequence variation in the members of the BnaBZRs might be associated with their differential expression pattern under different conditions.
Figure 10. SNPs distribution on coding and non-coding regions of the BnaBZRs. The yellow-colored block represents as the coding sequence, whereas the black lines indicate as the introns, and red-colored block represents as SNP.
Under the external stimulus, plants modulate their responses to adapt to the complex environment. It has been reported that the BR regulates plant physiology by downstream components to stimulate environmental adaptation (Xie et al., 2011; Miyaji et al., 2014). These downstream components were transcriptional factors of BR signaling BZR1 and BZR2, which regulate plant growth and tolerance to stresses by integrating with a wide variety of BR-responsive genes. In recent years, due to the advancement in high-throughput sequencing, the BZR gene family in many economically important crops, such as Rice, Maize, Brassica rapa, Glycine max, Tomato, Eucalyptus grandis, and Petunia hybrid (Tong et al., 2012; Verhoef et al., 2013; Liu et al., 2014; Saha et al., 2015; Zhang et al., 2016; Fan et al., 2018; Manoli et al., 2018), have been isolated and examined (Table 1). These studies confirmed the roles of the BZR genes in plant biological processes and reported the possible function in stress conditions. For instance, SiBZR1 might regulate BR-signaling in response to salt stress in tomato (Jia et al., 2021). In contrast, the overaccumulation of the AtBZR1 in Glycine max induces the seed count per siliqua (Zhang et al., 2016). Furthermore, few studies have also reported the transcriptional response of BR signaling during environmental stresses in B. napus (Kagale et al., 2007; Sahni et al., 2016). For instance, overexpression of the A. thaliana BR biosynthetic gene in B. napus enhances drought tolerance phenotype, which is likely mediated by BZR transcriptional factors (Sahni et al., 2016). Moreover, there is only one study that helped to understand the cross-genome exploration of BZR origin between B. napus and other plant species (Song et al., 2018). However, there is a lack of studies regarding the structural features, functional divergence, and stress-resistant related response of the BZR members in the B. napus, which gives us an excellent opportunity to inspect the B. napus BZR gene family and analyze their features including evolutionary relationship, structure, protein evolution and functional divergence, protein–protein interaction, miRNA prediction, cis-elements, and expression profile in different organs and response to abiotic stresses. These findings provide an overview of the BZR genes structural features, evolution as well as important insights regarding the potential unknown functions of the BnaBZRs.
A total of 21 BnaBZRs were identified, which are separated into four groups based on A. thaliana BZR homologs (Figure 1). However, the numbers of BnaBZRs were higher than the B. oleracea, A. thaliana, and B. rapa. This variation in gene numbers might be due to the larger genome or genome duplication during the evolution of the B. napus. Group I and group II, III are the sister groups, while members in group IV are distantly associated with other groups. In terms of amino acid similarity, all members in the groups contain the highly conserved N-terminal BZR1 domain, but the gene structure and motif composition display discrepancies in family members. For instance, members in group IV contain only one intron, while most members in group I, II, and III contain two introns, although the exon distribution among family members is different (Figure 2). Moreover, group IV lacks motif 8, 10, and 14 (Figure 4), indicating that the genes from the same subfamily might function differently among other groups. The diversity among gene sub-families is mainly driven by the mutations in the specific amino acid site (Lynch and Conery, 2000; Ha et al., 2009). To identify the functional divergence between the BnaBZRs groups, ϴI and ϴII functional divergence analysis was performed. Results from this analysis showed that the total of 21 ϴI and three ϴII functional divergence sites were predicted in three subfamily (Table 2), suggesting that the ϴI functional divergence is dominant. Additionally, two amino acid sites, 19T and 20R, were predicted in both ϴI and ϴII. These sites might be responsible for diversification in evolutionary rates and physiochemical properties. Interestingly, we observed that ϴI and ϴII functional divergence sites were mainly distributed on the N-terminal BZR1 domain (Figure 3), suggesting that the BnaBZR gene family might experience varied selection pressures during duplication events. However, the insufficiency of significant variations in functional divergence among BnaBZR gene family pairs indicates the similar roles of their family members. Furthermore, synteny analysis predicts that the members in group IV have similar segmental duplication patterns in group I, II, and III, suggesting that the sub-functionalization or non-functionalization may include during the evolution of the B. napus BZR gene family. Nevertheless, segmental duplication appears to contribute more to BZR gene family expansion than tandem duplication during duplication events (Figure 5B). Brassica napus is an allopolyploid (AACC) crop, originated from the natural hybridization of the B. oleracea and B. rapa (Chalhoub et al., 2014), which were diverged approximately 20–40 years ago from the common ancestor A. thaliana. To study the genetic relationship among them, we performed the collinearity analysis, which indicates the 49, 62, and 62 colinear gene pairs between B. napus/A. thaliana, B. napus/B. rapa, and B. napus/B. oleracea, respectively (Figure 6; Supplementary Table S3).
Additionally, we investigate that BnaBZRs expressed ubiquitously in sepal, petal, seed, lower stem, middle stem, upper stem, root, vegetative rosette, cotyledon, filament, and siliqua wall (Supplementary Figure S5; Figure 8). According to the previous studies, the BR signaling pathway is needed to regulate the transition from vegetative to reproductive growth (Gallego-Bartolomé et al., 2012; Li et al., 2012; Oh et al., 2012; Zhiponova et al., 2013; Fridman et al., 2014; Vragović et al., 2015), indicating that the members of the BnaBZRs might mediate B. napus development by interacting with various TFs of different signaling pathways. In our protein interaction analysis, the members in the BnaBZR gene family show a strong association with hormonal and stress-induced transcriptional factors (Figure 7). For instance, BnaBZR1, BnaBZR2, and BnaBEH1 interact with MYBL2, PHYB, PIF3, PIF4, RGA1, RGL3, SLY1, and GID1B, which function in response to anthocyanin biosynthesis, light, gibberellins (GA), ABA, salt, and drought (Somers and Quail, 1995; Más et al., 2000; Mahmood et al., 2016). Based on these results, we speculate that the BnaBZRs might regulate plant physiology in response to stresses. However, the molecular mechanism underlying these interactions needs verification. To further examine the roles of the BnaBZRs during stresses, the different expression patterns of BnaBZRs were observed against salinity, drought, cold, and S. sclerotiorum stresses (Supplementary Figure S6; Figure 9). The results are consistent with the previous findings that reported BZR genes in other species. For instance, in B. rapa, the expression patterns of six BrBZRs display higher expression in response to salt stress, whereas 11 BrBZRs showed induced expression in response to drought stress (Saha et al., 2015). However, in maize, members of the BZR gene family exhibit reduced expression during heat stress (Manoli et al., 2018), while increased expression was observed during salinity stress. Besides this in RNA-seq analysis, we predicted the induced expression patterns of the BnaBEH3 and BnaBEH4 genes under S. sclerotiorum infection (Supplementary Figure S6B). Notably, the promoter regions of the BnaBZRs were predicted to contain the biotic stress-responsive cis-core elements (Supplementary Table S7), suggesting the possible role of the BnaBZR TFs in response to biotic stresses.
In addition, to examine the BnaBZRs regulatory pathways, we predicted the miRNAs targeted genes, which gives an important insight into the genes regulation role under stresses. In the B. napus, a total of 376 miRNAs had been identified (Zhang et al., 2019); however, only 88 novel miRNAs are predicted to show important roles in B. napus (Palatnik et al., 2007; Alonso-Peral et al., 2010; Shen et al., 2014). In this study, we observed that the nine members of the BnaBZRs were targeted by different miRNAs. In particular, BnaBEH3 and BnaBEH4, whose expression was significantly regulated under drought and salinity stress, show an oblivious interaction with bna-miR158, bna-miR164, bna-miR159, and bna-miRN273 (Table 3), which are reported to exhibit genes regulations at the post-transcriptional level in response to various stresses (Wang et al., 2012a; Zhao et al., 2012; Huang et al., 2013; Zhang et al., 2018). Furthermore, our GO analysis revealed that BP terms, such as response to biotic stimulus, immune response, response to the bacterium, defense response, and response to stresses, were present in BnaBEH3 and BnaBEH4 (Supplementary Figure S7). Therefore, our results suggest the prominent regulatory role of group III and group IV genes during abiotic and biotic stresses. However, the functional mechanism of these genes in B. napus still needs to be investigated, as this will help us understand the functional divergence of the BnaBZR gene family and enable further dissection of BR-signaling in B. napus.
In our study, a total of 21 BnaBZRs were recognized in B. napus genomic sequence, which was clustered into the four groups based upon the phylogenetic relationship among B. oleracea, B. rapa, and A. thaliana orthologs. Our protein sequence similarity analysis displayed that all members of the BnaBZRs are closely related to six A. thaliana BZR genes, which indicate similar structural features. Genome duplication and synteny analysis revealed that segmental duplication was the main reason for BZR genes expansion through purifying selection in B. napus. Furthermore, protein–protein interaction miRNA target prediction, cis-regulatory elements, Gene Ontology, and qRT-PCR analysis of BnaBZRs under salt and drought stress exhibit an obvious divergence between subgroups. Overall, results from this study provide valuable information on evolutionary characteristics and potential functions of the BnaBZRs in abiotic and biotic stresses, which lay a foundation for future work in modulating stress tolerance and B. napus development.
The datasets presented in this study can be found in online repositories. The names of the repository/repositories and accession number(s) can be found in the article/Supplementary Material.
RS conceived and planned the experiments and wrote the manuscript. RG, LL, and YS extracted the RNA and performed the qRT-PCR analysis. K-MZ and JW analyzed the data. X-LT revised and supervised the manuscript. All authors contributed to the article and approved the submitted version.
This study was funded by the Jiangsu Agriculture Science and Technology Innovation Fund (CX(21)2009).
The authors declare that the research was conducted in the absence of any commercial or financial relationships that could be construed as a potential conflict of interest.
All claims expressed in this article are solely those of the authors and do not necessarily represent those of their affiliated organizations, or those of the publisher, the editors and the reviewers. Any product that may be evaluated in this article, or claim that may be made by its manufacturer, is not guaranteed or endorsed by the publisher.
We like to thank the Oil Crop Research Institute, Chinese Academy of Agricultural Sciences, Wuhan, Hubei province, P.R. China for providing the Brassica napus cultivar Zhongshuang 11 (ZS11) seeds. We appreciate and thank the reviewers and editors for their rigorous reading and valuable comments on our manuscript.
The Supplementary Material for this article can be found online at: https://www.frontiersin.org/articles/10.3389/fpls.2021.790655/full#supplementary-material
1. ^https://www.genoscope.cns.fr/brassicanapus
2. ^http://cbi.hzau.edu.cn/bnapus
3. ^http://www.arabidopsis.org
5. ^https://prosite.expasy.org
6. ^http://pfam.xfam.org/search/sequence
8. ^http://www.csbio.sjtu.edu.cn/bioinf/plant-multi
10. ^http://gsds.cbi.pku.edu.cn
11. ^http://meme-suite.org/tools/meme
12. ^http://selecton.tau.ac.il
13. ^https://zhanggroup.org//I-TASSER/
17. ^http://structuralbiology.cau.edu.cn/PNRD/index.php
18. ^https://www.zhaolab.org/psRNATarget/
19. ^http://bioinformatics.psb.ugent.be/webtools/plantcare/html
Alonso-Peral, M. M., Li, J., Li, Y., Allen, R. S., Schnippenkoetter, W., Ohms, S., et al. (2010). The microRNA159-regulated GAMYB-like genes inhibit growth and promote programmed cell death in Arabidopsis. Plant Physiol. 154, 757–771. doi: 10.1104/pp.110.160630
Anisimova, M., Bielawski, J. P., and Yang, Z. (2001). Accuracy and power of the likelihood ratio test in detecting adaptive molecular evolution. Mol. Biol. Evol. 18, 1585–1592. doi: 10.1093/oxfordjournals.molbev.a003945
Bailey, T. L., and Elkan, C. (1994). Fitting a mixture model by expectation maximization to discover motifs in bipolymers. Proc. Int. Conf. Intell. Syst. Mol. Biol. 2, 28–36. doi: 10.2139/ssrn.3705225
Cao, X., Khaliq, A., Lu, S., Xie, M., Ma, Z., Mao, J., et al. (2020). Genome-wide identification and characterization of the BES1 gene family in apple (Malus domestica). Plant Biol. 22, 723–733. doi: 10.1111/plb.13109
Chalhoub, B., Denoeud, F., Liu, S., Parkin, I. A., Tang, H., Wang, X., et al. (2014). Early allopolyploid evolution in the post-Neolithic Brassica napus oilseed genome. Science 345, 950–953. doi: 10.1126/science.1253435
Chen, C., Chen, H., Zhang, Y., Thomas, H. R., Frank, M. H., He, Y., et al. (2020). TBtools: an integrative toolkit developed for interactive analyses of big biological data. Mol. Plant 13, 1194–1202. doi: 10.1101/289660
Chen, J., Nolan, T. M., Ye, H., Zhang, M., Tong, H., Xin, P., et al. (2017). Arabidopsis WRKY46, WRKY54, and WRKY70 transcription factors are involved in brassinosteroid-regulated plant growth and drought responses. Plant Cell 29, 1425–1439. doi: 10.1105/tpc.17.00364
Chen, L.-G., Gao, Z., Zhao, Z., Liu, X., Li, Y., Zhang, Y., et al. (2019a). BZR1 family transcription factors function redundantly and indispensably in BR signaling but exhibit BRI1-independent function in regulating anther development in Arabidopsis. Mol. Plant 12, 1408–1415. doi: 10.1016/j.molp.2019.06.006
Chen, W., Lv, M., Wang, Y., Wang, P.-A., Cui, Y., Li, M., et al. (2019b). BES1 is activated by EMS1-TPD1-SERK1/2-mediated signaling to control tapetum development in Arabidopsis thaliana. Nat. Commun. 10:4164. doi: 10.1038/s41467-019-12118-4
Chen, X., Wu, X., Qiu, S., Zheng, H., Lu, Y., Peng, J., et al. (2021). Genome-wide identification and expression profiling of the BZR transcription factor gene family in Nicotiana benthamiana. Int. J. Mol. Sci. 22:10379. doi: 10.3390/ijms221910379
Chinchilla, D., Shan, L., He, P., de Vries, S., and Kemmerling, B. (2009). One for all: the receptor-associated kinase BAK1. Trends Plant Sci. 14, 535–541. doi: 10.1016/j.tplants.2009.08.002
Chou, K.-C., and Shen, H.-B. (2010). Plant-mPLoc: a top-down strategy to augment the power for predicting plant protein subcellular localization. PLoS One 5:e11335. doi: 10.1371/journal.pone.0011335
Clouse, S. D., Langford, M., and McMorris, T. C. (1996). A brassinosteroid-insensitive mutant in Arabidopsis thaliana exhibits multiple defects in growth and development. Plant Physiol. 111, 671–678. doi: 10.1104/pp.111.3.671
Cui, X.-Y., Gao, Y., Guo, J., Yu, T.-F., Zheng, W.-J., Liu, Y.-W., et al. (2019). BES/BZR transcription factor TaBZR2 positively regulates drought responses by activation of TaGST1. Plant Physiol. 180, 605–620. doi: 10.1104/pp.19.00100
Dai, X., and Zhao, P. X. (2011). psRNATarget: a plant small RNA target analysis server. Nucleic Acids Res. 39, W155–W159. doi: 10.1093/nar/gkr319
De Castro, E., Sigrist, C. J., Gattiker, A., Bulliard, V., Langendijk-Genevaux, P. S., Gasteiger, E., et al. (2006). ScanProsite: detection of PROSITE signature matches and ProRule-associated functional and structural residues in proteins. Nucleic Acids Res. 34, W362–W365. doi: 10.1093/nar/gkl124
Ding, L., Guo, X., Wang, K., Pang, H., Liu, Y., Yang, Q., et al. (2021). Genome-wide analysis of BES1/BZR1 transcription factors and their responses to osmotic stress in Ammopiptanthus nanus. J. For. Res. 26, 127–135. doi: 10.1080/13416979.2020.1867293
Fan, C., Guo, G., Yan, H., Qiu, Z., Liu, Q., and Zeng, B. (2018). Characterization of Brassinazole resistant (BZR) gene family and stress induced expression in Eucalyptus grandis. Physiol. Mol. Biol. Plants 24, 821–831. doi: 10.1007/s12298-018-0543-2
Fridman, Y., Elkouby, L., Holland, N., Vragović, K., Elbaum, R., and Savaldi-Goldstein, S. (2014). Root growth is modulated by differential hormonal sensitivity in neighboring cells. Genes Dev. 28, 912–920. doi: 10.1101/gad.239335.114
Friedt, W., Tu, J., and Fu, T. (2018). “Academic and economic importance of Brassica napus rapeseed,” in The Brassica napus Genome. eds. W. Friedt, S. Rod, and C. Boulos (Cham: Springer), 1–20.
Gallego-Bartolomé, J., Minguet, E. G., Grau-Enguix, F., Abbas, M., Locascio, A., Thomas, S. G., et al. (2012). Molecular mechanism for the interaction between gibberellin and brassinosteroid signaling pathways in Arabidopsis. Proc. Natl. Acad. Sci. U. S. A. 109, 13446–13451. doi: 10.1073/pnas.1119992109
Gasteiger, E., Hoogland, C., Gattiker, A., Wilkins, M. R., Appel, R. D., and Bairoch, A. (2005). “Protein identification and analysis tools on the ExPASy Server,” in The Proteomics Protocols Handbook. Springer Protocols Handbooks. ed. J. M. Walker (Humana Press), 571–607.
Glazebrook, J. (2001). Genes controlling expression of defense responses in Arabidopsis—2001 status. Curr. Opin. Plant Biol. 4, 301–308. doi: 10.1016/s1369-5266(00)00177-1
Gu, X., and Vander Velden, K. (2002). DIVERGE: phylogeny-based analysis for functional–structural divergence of a protein family. Bioinformatics 18, 500–501. doi: 10.1093/bioinformatics/18.3.500
Gu, X., Zou, Y., Su, Z., Huang, W., Zhou, Z., Arendsee, Z., et al. (2013). An update of DIVERGE software for functional divergence analysis of protein family. Mol. Biol. Evol. 30, 1713–1719. doi: 10.1093/molbev/mst069
Guo, X., Lu, P., Wang, Y., Cai, X., Wang, X., Zhou, Z., et al. (2017). Genome-wide identification and expression analysis of the gene family encoding Brassinazole resistant transcription factors in cotton. Cotton Sci. 29, 415–427.
Ha, M., Kim, E.-D., and Chen, Z. J. (2009). Duplicate genes increase expression diversity in closely related species and allopolyploids. Proc. Natl. Acad. Sci. U. S. A. 106, 2295–2300. doi: 10.1073/pnas.0807350106
Hatzig, S. V., Nuppenau, J.-N., Snowdon, R. J., and Schießl, S. V. (2018). Drought stress has transgenerational effects on seeds and seedlings in winter oilseed rape (Brassica napus L.). BMC Plant Biol. 18:297. doi: 10.1186/s12870-018-1531-y
He, J.-X., Gendron, J. M., Sun, Y., Gampala, S. S., Gendron, N., Sun, C. Q., et al. (2005). BZR1 is a transcriptional repressor with dual roles in brassinosteroid homeostasis and growth responses. Science 307, 1634–1638. doi: 10.1126/science.1107580
He, J.-X., Gendron, J. M., Yang, Y., Li, J., and Wang, Z.-Y. (2002). The GSK3-like kinase BIN2 phosphorylates and destabilizes BZR1, a positive regulator of the brassinosteroid signaling pathway in Arabidopsis. Proc. Natl. Acad. Sci. 99, 10185–10190. doi: 10.1073/pnas.152342599
He, K., Gou, X., Yuan, T., Lin, H., Asami, T., Yoshida, S., et al. (2007). BAK1 and BKK1 regulate brassinosteroid-dependent growth and brassinosteroid-independent cell-death pathways. Curr. Biol. 17, 1109–1115. doi: 10.1016/j.cub.2007.05.036
Hu, B., Jin, J., Guo, A.-Y., Zhang, H., Luo, J., and Gao, G. (2015). GSDS 2.0: an upgraded gene feature visualization server. Bioinformatics 31, 1296–1297. doi: 10.1093/bioinformatics/btu817
Huang, D., Koh, C., Feurtado, J. A., Tsang, E. W., and Cutler, A. J. (2013). MicroRNAs and their putative targets in Brassica napus seed maturation. BMC Genomics 14:140. doi: 10.1186/1471-2164-14-140
Huang, D. W., Sherman, B. T., and Lempicki, R. A. (2009a). Bioinformatics enrichment tools: paths toward the comprehensive functional analysis of large gene lists. Nucleic Acids Res. 37, 1–13. doi: 10.1093/nar/gkn923
Huang, D. W., Sherman, B. T., and Lempicki, R. A. (2009b). Systematic and integrative analysis of large gene lists using DAVID bioinformatics resources. Nat. Protoc. 4, 44–57. doi: 10.1038/nprot.2008.211
Huang, S. Q., Xiang, A. L., Che, L. L., Chen, S., Li, H., Song, J. B., et al. (2010). A set of miRNAs from Brassica napus in response to sulphate deficiency and cadmium stress. Plant Biotechnol. J. 8, 887–899. doi: 10.1111/j.1467-7652.2010.00517.x
Jia, C., Zhao, S., Bao, T., Zhao, P., Peng, K., Guo, Q., et al. (2021). Tomato BZR/BES transcription factor SlBZR1 positively regulates BR signaling and salt stress tolerance in tomato and Arabidopsis. Plant Sci. 302:110719. doi: 10.1016/j.plantsci.2020.110719
Jia, D., Chen, L. G., Yin, G., Yang, X., Gao, Z., Guo, Y., et al. (2020). Brassinosteroids regulate outer ovule integument growth in part via the control of INNER NO OUTER by BRASSINOZOLE-RESISTANT family transcription factors. J. Integr. Plant Biol. 62, 1093–1111. doi: 10.1111/jipb.12915
Jian, H., Wang, J., Wang, T., Wei, L., Li, J., and Liu, L. (2016). Identification of rapeseed microRNAs involved in early stage seed germination under salt and drought stresses. Front. Plant Sci. 7:658. doi: 10.3389/fpls.2016.00658
Jiang, S., Li, S., Liu, X., Wen, B., Wang, N., Zhang, R., et al. (2021). Genome-wide identification and characterization of the MdBZR1 gene family in apple and their roles in improvement of drought tolerance. Sci. Hortic. 288:110359. doi: 10.1016/j.scienta.2021.110359
Kagale, S., Divi, U. K., Krochko, J. E., Keller, W. A., and Krishna, P. (2007). Brassinosteroid confers tolerance in Arabidopsis thaliana and Brassica napus to a range of abiotic stresses. Planta 225, 353–364. doi: 10.1007/s00425-006-0361-6
Kang, S., Yang, F., Li, L., Chen, H., Chen, S., and Zhang, J. (2015). The Arabidopsis transcription factor BRASSINOSTEROID INSENSITIVE1-ETHYL METHANESULFONATE-SUPPRESSOR1 is a direct substrate of MITOGEN-ACTIVATED PROTEIN KINASE6 and regulates immunity. Plant Physiol. 167, 1076–1086. doi: 10.1104/pp.114.250985
Kesawat, M. S., Kherawat, B. S., Singh, A., Dey, P., Kabi, M., Debnath, D., et al. (2021). Genome-wide identification and characterization of the Brassinazole-resistant (BZR) gene family and its expression in the various developmental stage and stress conditions in wheat (Triticum aestivum L.). Int. J. Mol. Sci. 22:8743. doi: 10.3390/ijms22168743
Kim, T.-W., Guan, S., Burlingame, A. L., and Wang, Z.-Y. (2011). The CDG1 kinase mediates brassinosteroid signal transduction from BRI1 receptor kinase to BSU1 phosphatase and GSK3-like kinase BIN2. Mol. Cell 43, 561–571. doi: 10.1016/j.molcel.2011.05.037
Kim, Y., Song, J.-H., Park, S.-U., Jeong, Y.-S., and Kim, S.-H. (2017). Brassinosteroid-induced transcriptional repression and dephosphorylation-dependent protein degradation negatively regulate BIN2-interacting AIF2 (a BR signaling-negative regulator) bHLH transcription factor. Plant Cell Physiol. 58, 227–239. doi: 10.1093/pcp/pcw223
Kozomara, A., Birgaoanu, M., and Griffiths-Jones, S. (2019). miRBase: from microRNA sequences to function. Nucleic Acids Res. 47, D155–D162. doi: 10.1093/nar/gky1141
Lachowiec, J., Mason, G. A., Schultz, K., and Queitsch, C. (2018). Redundancy, feedback, and robustness in the Arabidopsis thaliana BZR/BEH gene family. Front. Genet. 9:523. doi: 10.3389/fgene.2018.00523
Lescot, M., Déhais, P., Thijs, G., Marchal, K., Moreau, Y., Van de Peer, Y., et al. (2002). PlantCARE, a database of plant cis-acting regulatory elements and a portal to tools for in silico analysis of promoter sequences. Nucleic Acids Res. 30, 325–327. doi: 10.1093/nar/30.1.325
Letunic, I., and Bork, P. (2021). Interactive Tree Of Life (iTOL) v5: an online tool for phylogenetic tree display and annotation. Nucleic Acids Res. 49, W293–W296. doi: 10.1093/nar/gkab301
Li, H., Ye, K., Shi, Y., Cheng, J., Zhang, X., and Yang, S. (2017). BZR1 positively regulates freezing tolerance via CBF-dependent and CBF-independent pathways in Arabidopsis. Mol. Plant 10, 545–559. doi: 10.1016/j.molp.2017.01.004
Li, J., and Chory, J. (1999). Brassinosteroid actions in plants. J. Exp. Bot. 50, 275–282. doi: 10.1093/jexbot/50.332.275
Li, J., Nam, K. H., Vafeados, D., and Chory, J. (2001). BIN2, a new brassinosteroid-insensitive locus in Arabidopsis. Plant Physiol. 127, 14–22. doi: 10.1104/pp.127.1.14
Li, L., Ye, H., Guo, H., and Yin, Y. (2010). Arabidopsis IWS1 interacts with transcription factor BES1 and is involved in plant steroid hormone brassinosteroid regulated gene expression. Proc. Natl. Acad. Sci. U. S. A. 107, 3918–3923. doi: 10.1073/pnas.0909198107
Li, Q.-F., and He, J.-X. (2016). BZR1 interacts with HY5 to mediate brassinosteroid-and light-regulated cotyledon opening in Arabidopsis in darkness. Mol. Plant 9, 113–125. doi: 10.1016/j.molp.2015.08.014
Li, Q.-F., Lu, J., Yu, J.-W., Zhang, C.-Q., He, J.-X., and Liu, Q.-Q. (2018). The brassinosteroid-regulated transcription factors BZR1/BES1 function as a coordinator in multisignal-regulated plant growth. Biochim. Biophys. Acta 1861, 561–571. doi: 10.1016/j.bbagrm.2018.04.003
Li, Q.-F., Wang, C., Jiang, L., Li, S., Sun, S. S., and He, J.-X. (2012). An interaction between BZR1 and DELLAs mediates direct signaling crosstalk between brassinosteroids and gibberellins in Arabidopsis. Sci. Signal. 5:ra72. doi: 10.1126/scisignal.2002908
Lin, E., Zhuang, H., Yu, J., Liu, X., Huang, H., Zhu, M., et al. (2020). Genome survey of Chinese fir (Cunninghamia lanceolata): identification of genomic SSRs and demonstration of their utility in genetic diversity analysis. Sci. Rep. 10, 4698–4612. doi: 10.1038/s41598-020-61611-0
Liu, D., Cui, Y., Zhao, Z., Li, S., Liang, D., Wang, C., et al. (2021). Genome-wide identification and characterization of the BES/BZR gene family in wheat and foxtail millet. BMC Genomics 22:682. doi: 10.1186/s12864-021-08002-5
Liu, L., Jia, C., Zhang, M., Chen, D., Chen, S., Guo, R., et al. (2014). Ectopic expression of a BZR1-1D transcription factor in brassinosteroid signalling enhances carotenoid accumulation and fruit quality attributes in tomato. Plant Biotechnol. J. 12, 105–115. doi: 10.1111/pbi.12121
Livak, K. J., and Schmittgen, T. D. (2001). Analysis of relative gene expression data using real-time quantitative PCR and the 2−ΔΔCT method. Methods 25, 402–408. doi: 10.1006/meth.2001.1262
Lynch, M., and Conery, J. S. (2000). The evolutionary fate and consequences of duplicate genes. Science 290, 1151–1155. doi: 10.1126/science.290.5494.1151
Mahmood, K., Xu, Z., El-Kereamy, A., Casaretto, J. A., and Rothstein, S. J. (2016). The Arabidopsis transcription factor ANAC032 represses anthocyanin biosynthesis in response to high sucrose and oxidative and abiotic stresses. Front. Plant Sci. 7:1548. doi: 10.3389/fpls.2016.01548
Manoli, A., Trevisan, S., Quaggiotti, S., and Varotto, S. (2018). Identification and characterization of the BZR transcription factor family and its expression in response to abiotic stresses in Zea mays L. Plant Growth Regul. 84, 423–436. doi: 10.1007/s10725-017-0350-8
Más, P., Devlin, P. F., Panda, S., and Kay, S. A. (2000). Functional interaction of phytochrome B and cryptochrome 2. Nature 408, 207–211. doi: 10.1046/j.1365-313x.1999.t01-1-00599.x
Mi, H., Muruganujan, A., Ebert, D., Huang, X., and Thomas, P. D. (2019). PANTHER version 14: more genomes, a new PANTHER GO-slim and improvements in enrichment analysis tools. Nucleic Acids Res. 47, D419–D426. doi: 10.1093/nar/gky1038
Mistry, J., Chuguransky, S., Williams, L., Qureshi, M., Salazar, G. A., Sonnhammer, E. L., et al. (2021). Pfam: the protein families database in 2021. Nucleic Acids Res. 49, D412–D419. doi: 10.6019/tol.pfam_fams-t.2018.00001.1
Miyaji, T., Yamagami, A., Kume, N., Sakuta, M., Osada, H., Asami, T., et al. (2014). Brassinosteroid-related transcription factor BIL1/BZR1 increases plant resistance to insect feeding. Biosci. Biotechnol. Biochem. 78, 960–968. doi: 10.1080/09168451.2014.910093
Nekrutenko, A., Makova, K. D., and Li, W.-H. (2002). The KA/KS ratio test for assessing the protein-coding potential of genomic regions: an empirical and simulation study. Genome Res. 12, 198–202. doi: 10.1101/gr.200901
Nielsen, R., and Yang, Z. (1998). Likelihood models for detecting positively selected amino acid sites and applications to the HIV-1 envelope gene. Genetics 148, 929–936. doi: 10.1093/genetics/148.3.929
Nuruzzaman, M., Manimekalai, R., Sharoni, A. M., Satoh, K., Kondoh, H., Ooka, H., et al. (2010). Genome-wide analysis of NAC transcription factor family in rice. Gene 465, 30–44. doi: 10.1016/j.gene.2010.06.008
Oh, E., Zhu, J.-Y., Bai, M.-Y., Arenhart, R. A., Sun, Y., and Wang, Z.-Y. (2014). Cell elongation is regulated through a central circuit of interacting transcription factors in the Arabidopsis hypocotyl. elife 3:e03031. doi: 10.7554/elife.03031
Oh, E., Zhu, J.-Y., and Wang, Z.-Y. (2012). Interaction between BZR1 and PIF4 integrates brassinosteroid and environmental responses. Nat. Cell Biol. 14, 802–809. doi: 10.1038/ncb2545
Palatnik, J. F., Wollmann, H., Schommer, C., Schwab, R., Boisbouvier, J., Rodriguez, R., et al. (2007). Sequence and expression differences underlie functional specialization of Arabidopsis microRNAs miR159 and miR319. Dev. Cell 13, 115–125. doi: 10.1016/j.devcel.2019.09.016
Pellegrini, M., Haynor, D., and Johnson, J. M. (2004). Protein interaction networks. Expert Rev. Proteomics 1, 239–249. doi: 10.1586/14789450.1.2.239
Reise, S. P., and Waller, N. G. (2009). Item response theory and clinical measurement. Annu. Rev. Clin. Psychol. 5, 27–48. doi: 10.1146/annurev.clinpsy.032408.153553
Ryu, H., Cho, H., Bae, W., and Hwang, I. (2014). Control of early seedling development by BES1/TPL/HDA19-mediated epigenetic regulation of ABI3. Nat. Commun. 5, 4138–4111. doi: 10.1038/ncomms5138
Sabagh, A. E., Hossain, A., Barutçular, C., Islam, M. S., Ratnasekera, D., Kumar, N., et al. (2019). Drought and salinity stress management for higher and sustainable canola (‘Brassica napus’ L.) production: a critical review. Aust. J. Crop. Sci. 13, 88–97. doi: 10.21475/ajcs.19.13.01.p1284
Saha, G., Park, J.-I., Jung, H.-J., Ahmed, N. U., Kayum, M. A., Kang, J.-G., et al. (2015). Molecular characterization of BZR transcription factor family and abiotic stress induced expression profiling in Brassica rapa. Plant Physiol. Biochem. 92, 92–104. doi: 10.1016/j.plaphy.2015.04.013
Sahni, S., Prasad, B. D., Liu, Q., Grbic, V., Sharpe, A., Singh, S. P., et al. (2016). Overexpression of the brassinosteroid biosynthetic gene DWF4 in Brassica napus simultaneously increases seed yield and stress tolerance. Sci. Rep. 6, 1–14. doi: 10.1038/srep28298
Sarwar, R., Jiang, T., Ding, P., Gao, Y., Tan, X., and Zhu, K. (2021). Genome-wide analysis and functional characterization of the DELLA gene family associated with stress tolerance in B. napus. BMC Plant Biol. 21:286. doi: 10.1186/s12870-021-03054-x
Shen, Y., Zhao, Q., Zou, J., Wang, W., Gao, Y., Meng, J., et al. (2014). Characterization and expression patterns of small RNAs in synthesized Brassica hexaploids. Plant Mol. Biol. 85, 287–299. doi: 10.1007/s11103-014-0185-x
Solovyev, V., Kosarev, P., Seledsov, I., and Vorobyev, D. (2006). Automatic annotation of eukaryotic genes, pseudogenes and promoters. Genome Biol. 7(Suppl 1), S10.1–S10.12. doi: 10.1186/gb-2006-7-s1-s10
Somers, D. E., and Quail, P. H. (1995). Temporal and spatial expression patterns of PHYA and PHYB genes in Arabidopsis. Plant J. 7, 413–427. doi: 10.1046/j.1365-313x.1995.7030413.x
Song, J.-M., Guan, Z., Hu, J., Guo, C., Yang, Z., Wang, S., et al. (2020). Eight high-quality genomes reveal pan-genome architecture and ecotype differentiation of Brassica napus. Nat. Plants 6, 34–45. doi: 10.1038/s41477-019-0577-7
Song, J. M., Liu, D. X., Xie, W. Z., Yang, Z., Guo, L., Liu, K., et al. (2021). BnPIR: Brassica napus pan-genome information resource for 1689 accessions. Plant Biotechnol. J. 19, 412–414. doi: 10.1111/pbi.13491
Song, X., Li, Y., Cao, X., and Qi, Y. (2019). MicroRNAs and their regulatory roles in plant–environment interactions. Annu. Rev. Plant Biol. 70, 489–525. doi: 10.1146/annurev-arplant-050718-100334
Song, X., Ma, X., Li, C., Hu, J., Yang, Q., Wang, T., et al. (2018). Comprehensive analyses of the BES1 gene family in Brassica napus and examination of their evolutionary pattern in representative species. BMC Genomics 19:346. doi: 10.1186/s12864-018-4744-4
Stern, A., Doron-Faigenboim, A., Erez, E., Martz, E., Bacharach, E., and Pupko, T. (2007). Selecton 2007: advanced models for detecting positive and purifying selection using a Bayesian inference approach. Nucleic Acids Res. 35, W506–W511. doi: 10.1093/nar/gkm382
Su, D., Xiang, W., Wen, L., Lu, W., Shi, Y., Liu, Y., et al. (2021). Genome-wide identification, characterization and expression analysis of BES1 gene family in tomato. BMC Plant Biol. 21:161. doi: 10.1186/s12870-021-02933-7
Sun, G., Yao, T., Feng, C., Chen, L., Li, J., and Wang, L. (2017). Identification and biocontrol potential of antagonistic bacteria strains against Sclerotinia sclerotiorum and their growth-promoting effects on Brassica napus. Biol. Control 104, 35–43. doi: 10.1016/j.biocontrol.2016.10.008
Swift, M. L. (1997). GraphPad prism, data analysis, and scientific graphing. J. Chem. Inf. Comput. Sci. 37, 411–412. doi: 10.1021/ci960402j
Szklarczyk, D., Gable, A. L., Lyon, D., Junge, A., Wyder, S., Huerta-Cepas, J., et al. (2019). STRING v11: protein–protein association networks with increased coverage, supporting functional discovery in genome-wide experimental datasets. Nucleic Acids Res. 47, D607–D613. doi: 10.1093/nar/gky1131
Tong, H., Liu, L., Jin, Y., Du, L., Yin, Y., Qian, Q., et al. (2012). DWARF AND LOW-TILLERING acts as a direct downstream target of a GSK3/SHAGGY-like kinase to mediate brassinosteroid responses in rice. Plant Cell 24, 2562–2577. doi: 10.1105/tpc.112.097394
Verhoef, N., Yokota, T., Shibata, K., de Boer, G.-J., Gerats, T., Vandenbussche, M., et al. (2013). Brassinosteroid biosynthesis and signalling in Petunia hybrida. J. Exp. Bot. 64, 2435–2448. doi: 10.1093/jxb/ert102
Vragović, K., Sela, A., Friedlander-Shani, L., Fridman, Y., Hacham, Y., Holland, N., et al. (2015). Translatome analyses capture of opposing tissue-specific brassinosteroid signals orchestrating root meristem differentiation. Proc. Natl. Acad. Sci. U. S. A. 112, 923–928. doi: 10.1073/pnas.1417947112
Walker, P., Girard, I., Giesbrecht, S., Whyard, S., Fernando, D., de Kievit, T., et al. (2021). Tissue-specific mRNA profiling of the Brassica napus-Sclerotinia sclerotiorum interaction uncovers novel regulators of plant immunity. bioRxiv. doi: 10.1101/2021.03.27.437327
Wang, B., Zhu, X., and Wei, X. (2021). Genome-wide identification, structural analysis, and expression profiles of the BZR gene family in tomato. J. Plant Biochem. Biotechnol. 1–12. doi: 10.1007/s13562-021-00711-y
Wang, D., Zhang, Y., Zhang, Z., Zhu, J., and Yu, J. (2010). KaKs_Calculator 2.0: a toolkit incorporating gamma-series methods and sliding window strategies. Genomics Proteomics Bioinformatics 8, 77–80. doi: 10.1016/s1672-0229(10)60008-3
Wang, W., Sun, Y.-Q., Li, G.-L., and Zhang, S.-Y. (2019). Genome-wide identification, characterization, and expression patterns of the BZR transcription factor family in sugar beet (Beta vulgaris L.). BMC Plant Biol. 19:191. doi: 10.1186/s12870-019-1783-1
Wang, Y., Sun, F., Cao, H., Peng, H., Ni, Z., Sun, Q., et al. (2012a). TamiR159 directed wheat TaGAMYB cleavage and its involvement in anther development and heat response. PLoS One 7:e48445. doi: 10.1371/journal.pone.0048445
Wang, Y., Tang, H., DeBarry, J. D., Tan, X., Li, J., Wang, X., et al. (2012b). MCScanX: a toolkit for detection and evolutionary analysis of gene synteny and collinearity. Nucleic Acids Res. 40:e49. doi: 10.1093/nar/gkr1293
Wang, Z.-Y., Nakano, T., Gendron, J., He, J., Chen, M., Vafeados, D., et al. (2002). Nuclear-localized BZR1 mediates brassinosteroid-induced growth and feedback suppression of brassinosteroid biosynthesis. Dev. Cell 2, 505–513. doi: 10.1016/s1534-5807(02)00153-3
Wei, Z., and Li, J. (2016). Brassinosteroids regulate root growth, development, and symbiosis. Mol. Plant 9, 86–100. doi: 10.1016/j.molp.2015.12.003
Wu, P., Song, X., Wang, Z., Duan, W., Hu, R., Wang, W., et al. (2016a). Genome-wide analysis of the BES1 transcription factor family in Chinese cabbage (Brassica rapa ssp. pekinensis). Plant Growth Regul. 80, 291–301. doi: 10.1007/s10725-016-0166-y
Wu, X., Chen, J., Xu, S., Zhu, Z., and Zha, D. (2016b). Exogenous 24-epibrassinolide alleviates zinc-induced toxicity in eggplant (Solanum melongena L.) seedlings by regulating the glutathione-ascorbate-dependent detoxification pathway. J. Hortic. Sci. Biotechnol. 91, 412–420. doi: 10.1080/14620316.2016.1162030
Xie, L., Yang, C., and Wang, X. (2011). Brassinosteroids can regulate cellulose biosynthesis by controlling the expression of CESA genes in Arabidopsis. J. Exp. Bot. 62, 4495–4506. doi: 10.1093/jxb/err164
Xu, M., Ma, H., Zeng, L., Cheng, Y., Lu, G., Xu, J., et al. (2015). The effect of waterlogging on yield and seed quality at the early flowering stage in Brassica napus L. Field Crop Res. 180, 238–245. doi: 10.1016/j.fcr.2015.06.007
Yang, J., Yan, R., Roy, A., Xu, D., Poisson, J., and Zhang, Y. (2015). The I-TASSER suite: protein structure and function prediction. Nat. Methods 12, 7–8. doi: 10.1038/nmeth.3213
Yang, X., Bai, Y., Shang, J., Xin, R., and Tang, W. (2016). The antagonistic regulation of abscisic acid-inhibited root growth by brassinosteroids is partially mediated via direct suppression of ABSCISIC ACID INSENSITIVE 5 expression by BRASSINAZOLE RESISTANT 1. Plant Cell Environ. 39, 1994–2003. doi: 10.1111/pce.12763
Yang, Z. (2007). PAML 4: phylogenetic analysis by maximum likelihood. Mol. Biol. Evol. 24, 1586–1591. doi: 10.1093/molbev/msm088
Ye, Q., Zhu, W., Li, L., Zhang, S., Yin, Y., Ma, H., et al. (2010). Brassinosteroids control male fertility by regulating the expression of key genes involved in Arabidopsis anther and pollen development. Proc. Natl. Acad. Sci. U. S. A. 107, 6100–6105. doi: 10.1073/pnas.0912333107
Yi, X., Zhang, Z., Ling, Y., Xu, W., and Su, Z. (2015). PNRD: a plant non-coding RNA database. Nucleic Acids Res. 43, D982–D989. doi: 10.1093/nar/gku1162
Yin, Y., Wang, Z.-Y., Mora-Garcia, S., Li, J., Yoshida, S., Asami, T., et al. (2002). BES1 accumulates in the nucleus in response to brassinosteroids to regulate gene expression and promote stem elongation. Cell 109, 181–191. doi: 10.1016/s0092-8674(02)00721-3
Yu, H., Feng, W., Sun, F., Zhang, Y., Qu, J., Liu, B., et al. (2018). Cloning and characterization of BES1/BZR1 transcription factor genes in maize. Plant Growth Regul. 86, 235–249. doi: 10.1007/s10725-018-0424-2
Yu, X., Li, L., Zola, J., Aluru, M., Ye, H., Foudree, A., et al. (2011). A brassinosteroid transcriptional network revealed by genome-wide identification of BESI target genes in Arabidopsis thaliana. Plant J. 65, 634–646. doi: 10.1111/j.1365-313X.2010.04449.x
Zhang, C., Bai, M.-Y., and Chong, K. (2014). Brassinosteroid-mediated regulation of agronomic traits in rice. Plant Cell Rep. 33, 683–696. doi: 10.1007/s00299-014-1578-7
Zhang, L., Zou, J., Li, S., Wang, B., Raboanatahiry, N., and Li, M. (2019). Characterization and expression profiles of miRNAs in the triploid hybrids of Brassica napus and Brassica rapa. BMC Genomics 20:649. doi: 10.1186/s12864-019-6001-x
Zhang, L. W., Song, J. B., Shu, X. X., Zhang, Y., and Yang, Z. M. (2013a). miR395 is involved in detoxification of cadmium in Brassica napus. J. Hazard. Mater. 250, 204–211. doi: 10.1016/j.jhazmat.2013.01.053
Zhang, X. D., Sun, J. Y., You, Y. Y., Song, J. B., and Yang, Z. M. (2018). Identification of Cd-responsive RNA helicase genes and expression of a putative BnRH 24 mediated by miR158 in canola (Brassica napus). Ecotoxicol. Environ. Saf. 157, 159–168. doi: 10.1016/j.ecoenv.2018.03.081
Zhang, Y. (2008). I-TASSER server for protein 3D structure prediction. BMC Bioinformatics 9:40. doi: 10.1186/1471-2105-9-40
Zhang, Y., Li, B., Xu, Y., Li, H., Li, S., Zhang, D., et al. (2013b). The cyclophilin CYP20-2 modulates the conformation of BRASSINAZOLE-RESISTANT1, which binds the promoter of FLOWERING LOCUS D to regulate flowering in Arabidopsis. Plant Cell 25, 2504–2521. doi: 10.1105/tpc.113.110296
Zhang, Y., Zhang, Y.-J., Yang, B.-J., Yu, X.-X., Wang, D., Zu, S.-H., et al. (2016). Functional characterization of GmBZL2 (AtBZR1 like gene) reveals the conserved BR signaling regulation in Glycine max. Sci. Rep. 6:31134. doi: 10.1038/srep31134
Zhao, Y.-T., Wang, M., Fu, S.-X., Yang, W.-C., Qi, C.-K., and Wang, X.-J. (2012). Small RNA profiling in two Brassica napus cultivars identifies microRNAs with oil production-and development-correlated expression and new small RNA classes. Plant Physiol. 158, 813–823. doi: 10.1104/pp.111.187666
Zhiponova, M. K., Vanhoutte, I., Boudolf, V., Betti, C., Dhondt, S., Coppens, F., et al. (2013). Brassinosteroid production and signaling differentially control cell division and expansion in the leaf. New Phytol. 197, 490–502. doi: 10.1111/nph.12036
Keywords: Brassica napus, brassinosteroid, miRNAs, abiotic stress, gene expression
Citation: Sarwar R, Geng R, Li L, Shan Y, Zhu K-M, Wang J and Tan X-L (2022) Genome-Wide Prediction, Functional Divergence, and Characterization of Stress-Responsive BZR Transcription Factors in B. napus. Front. Plant Sci. 12:790655. doi: 10.3389/fpls.2021.790655
Received: 07 October 2021; Accepted: 01 December 2021;
Published: 04 January 2022.
Edited by:
Sangram Keshari Lenka, TERI Deakin Nanobiotechnology Centre, IndiaReviewed by:
Vishal Sharma, Institute of Himalayan Bioresource Technology (CSIR), IndiaCopyright © 2022 Sarwar, Geng, Li, Shan, Zhu, Wang and Tan. This is an open-access article distributed under the terms of the Creative Commons Attribution License (CC BY). The use, distribution or reproduction in other forums is permitted, provided the original author(s) and the copyright owner(s) are credited and that the original publication in this journal is cited, in accordance with accepted academic practice. No use, distribution or reproduction is permitted which does not comply with these terms.
*Correspondence: Xiao-Li Tan, eGx0YW5AdWpzLmVkdS5jbg==
Disclaimer: All claims expressed in this article are solely those of the authors and do not necessarily represent those of their affiliated organizations, or those of the publisher, the editors and the reviewers. Any product that may be evaluated in this article or claim that may be made by its manufacturer is not guaranteed or endorsed by the publisher.
Research integrity at Frontiers
Learn more about the work of our research integrity team to safeguard the quality of each article we publish.