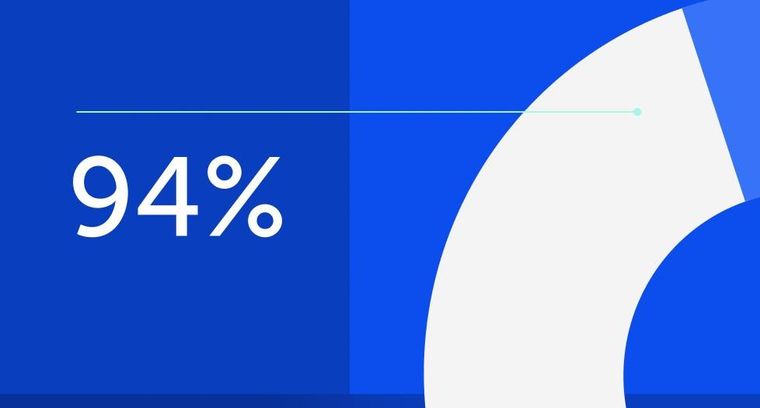
94% of researchers rate our articles as excellent or good
Learn more about the work of our research integrity team to safeguard the quality of each article we publish.
Find out more
ORIGINAL RESEARCH article
Front. Plant Sci., 12 January 2022
Sec. Plant Breeding
Volume 12 - 2021 | https://doi.org/10.3389/fpls.2021.785989
This article is part of the Research TopicAdvances in Breeding for Quantitative Disease ResistanceView all 28 articles
Plasmodiophora brassicae causes clubroot disease in brassica crops worldwide. Brassica rapa, a progenitor of Brassica napus (canola), possesses important sources for resistance to clubroot. A doubled haploid (DH) population consisting of 84 DH lines were developed from a Backcross2 (BC2) plant through an interspecific cross of B. rapa turnip cv. ECD01 (resistant, R) with canola line DH16516 (susceptible, S) and then backcrossed with DH16516 as the recurrent parent. The DH lines and their parental lines were tested for resistance to four major pathotypes (3A, 3D, 3H, and 5X) of P. brassicae identified from canola. The R:S segregation ratio for pathotype 3A was 1:3, and 3:1 for pathotypes 3D, 3H, and 5X. From genotyping by sequencing (GBS), a total of 355.3 M short reads were obtained from the 84 DH lines, ranging from 0.81 to 11.67 M sequences per line. The short reads were aligned into the A-genome of B. napus “Darmor-bzh” version 4.1 with a total of 260 non-redundant single-nucleotide polymorphism (SNP) sites. Two quantitative trait loci (QTLs), Rcr10ECD01 and Rcr9ECD01, were detected for the pathotypes in chromosomes A03 and A08, respectively. Rcr10ECD01 and Rcr9ECD01 were responsible for resistance to 3A, 3D, and 3H, while only one QTL, Rcr9ECD01, was responsible for resistance to pathotype 5X. The logarithm of the odds (LOD) values, phenotypic variation explained (PVE), additive (Add) values, and confidence interval (CI) from the estimated QTL position varied with QTL, with a range of 5.2–12.2 for LOD, 16.2–43.3% for PVE, 14.3–25.4 for Add, and 1.5–12.0 cM for CI. The presence of the QTLs on the chromosomes was confirmed through the identification of the percentage of polymorphic variants using bulked-segregant analysis. There was one gene encoding a disease resistance protein and 24 genes encoding proteins with function related to plant defense response in the Rcr10ECD01 target region. In the Rcr9ECD01 region, two genes encoded disease resistance proteins and 10 genes encoded with defense-related function. The target regions for Rcr10ECD01 and Rcr9ECD01 in B. napus were homologous to the 11.0–16.0 Mb interval of chromosome A03 and the 12.0–14.5 Mb interval of A08 in B. rapa “Chiifu” reference genome, respectively.
Brassica species are grown for the production of edible oil and vegetables. The genomic relationships among the main species of brassica crops were explained by the “triangle of U” (Morinaga, 1934; Nagaharu and Nagaharu, 1935); Brassica rapa (genome represented as AA; n = 10), Brassica nigra (BB; n = 8), and Brassica oleracea (CC; n = 9) are diploid species, and Brassica napus (AACC; n = 19), Brassica juncea (AABB; n = 18), and Brassica carinata (BBCC; n = 17) are amphidiploid species resulting from hybridization between pairs of the diploid species.
Clubroot, caused by the obligate soil-borne pathogen Plasmodiophora brassicae Woronin, is an important disease in brassica crops worldwide. The pathogen belongs to the infrakingdom Rhizaria, a diverse group of amoeboid microbes (Nikolaev et al., 2004). Root infection by P. brassicae results in the formation of characteristic clubs, also known as “galls,” on the roots of host plants. These abnormal growths restrict the flow of water and nutrients to the plant, resulting in above-ground symptoms that include stunting, yellowing, premature senescence, and reduction in both seed yield and quality (Pageau et al., 2006). B. napus (oilseed rape/canola) is an important crop for edible oil production worldwide. Clubroot was first identified in canola fields on the Canadian Prairies in 2003 but has spread rapidly to pose a serious threat to canola production in Canada.
Strains of P. brassicae collected in Canada have been classified into more than 30 pathotypes based on the reactions on the Canadian Clubroot Differential (CCD) set (Strelkov et al., 2018; Hollman et al., 2021). Among the pathotypes, 3H was the most prevalent original pathotype, 5X was the first new pathotype that was aggressive on the first generation of Canadian clubroot-resistant cultivars, and 3A and 3D are currently the predominate new pathotypes in Alberta (Dakouri et al., 2021). The pathogen can survive in soil as resting spores for a long period, so it is difficult to manage using cultural practices or chemical treatments (Voorrips, 1995). Genetic resistance can be an effective strategy for clubroot management, but the sources available for resistance to clubroot in B. napus are very limited. Strong resistance was identified in its progenitor species, B. rapa, especially in European turnip, B. rapa subsp. rapifera, which was reviewed by Hirai (2006). The resistance to clubroot available from European turnips has been transferred into Chinese cabbage (B. rapa) (Piao et al., 2009). Introgression of traits from turnip into B. napus is possible via interspecific crosses, so turnip has been a valuable source for resistance to clubroot in canola. Clubroot resistance (CR) from turnip cultivar “Debra” has been transferred into B. napus cultivars of swede (Lammerink, 1970) and from turnip cultivar “Waaslander” (also known as ECD04) into forage (Johnston, 1974) and oilseed lines of B. napus (Gowers, 1982).
Genetic mapping of CR genes is an important step toward breeding for resistance to clubroot. To date, more than 20 genes or quantitative trait locus (QTLs) have been mapped to six chromosomes of the A-genome in B. rapa through biparental mapping methods. Crr2 and PbBa1.1 were located on A01 (Suwabe et al., 2003; Chen et al., 2013); CRc and Rcr8 were located on A02 (Sakamoto et al., 2008; Yu et al., 2017); Bra.CR.a, Bra.CR.c, Crr3, CRa, CRb, CRbkato, CRd, CRk, PbBa3.1, PbBa3.2, PbBa3.3, Rcr1, Rcr2, Rcr4, and Rcr5 were located on A03 (Matsumoto et al., 1998; Hirai et al., 2004; Piao et al., 2004; Sakamoto et al., 2008; Chen et al., 2013; Chu et al., 2014; Pang et al., 2014; Yu et al., 2016, 2017; Huang et al., 2017, 2019; Hirani et al., 2018); Crr4 were located on A06 (Suwabe et al., 2003); qBrCR38-1 were located on A07 (Zhu et al., 2019); Crr1, CRs, PbBa8.1, Bra.CR.b, Rcr3, Rcr9/Rcr9wa, and qBrCR38-2 were located on A08 (Suwabe et al., 2006; Chen et al., 2013; Yu et al., 2017; Hirani et al., 2018; Laila et al., 2019; Zhu et al., 2019; Karim et al., 2020). Three genes, Crr1, CRa, and CRbkato, have been cloned, all of which encode toll-interleukin-1 receptor, nucleotide binding site, and leucine-rich repeat (TIR-NBS-LRR, TNL) proteins (Ueno et al., 2012; Hatakeyama et al., 2013, 2017). The identification of CR genes has been also carried out in B. oleracea (Lee et al., 2016; Dakouri et al., 2018; Peng et al., 2018), B. nigra (Chang et al., 2019), and B. napus (Manzanares-Dauleux et al., 2000; Werner et al., 2008; Fredua-Agyeman and Rahman, 2016; Hasan and Rahman, 2016; Botero-Ramírez et al., 2020).
Brassica rapa turnip cv. “Debra” was used as the donor for developing CR in swede cultivars (Lammerink, 1970). “Debra” was included in the European clubroot differential (ECD) set as differential line ECD01 (Buczacki et al., 1975; Diederichsen et al., 2009). CRb, a CR gene identified in a Chinese cabbage cv. “CR Shinki” and two CR genes in Chinese cabbage cv. “CR Kanko,” CRk and CRc, were derived from ECD01 (Piao et al., 2004) and “Debra” (Sakamoto et al., 2008), respectively. Two other CR genes, BraA.CR.a (A03) and BraA.CR.b (A08), were also identified from ECD01 (Hirani et al., 2018). Finally, ECD01 was resistant to all of the Canadian pathotypes of P. brassicae described by Strelkov et al. (2018) (Yu F, unpublished data), which makes it a valuable source of genes for CR canola in Canada.
In this study, an interspecific cross of ECD01 × B. napus line DH16516 was made, and the resulting F1 progeny were backcrossed with DH16516 to produce BC1. Continuing backcross was made by crossing the BC1 with DH16516 to produce Backcross2 (BC2). A doubled haploid (DH) population consisting of 84 DH lines from a single BC2 plant was developed. Genotyping by sequencing (GBS) analysis of the A-genome of B. napus was used to (1) characterize the genome-wide DNA variants in the DH lines, (2) detect QTLs associated with resistance to the most important pathotypes of P. brassicae on the Canadian Prairies, and (3) identify putative candidate genes for each QTL.
A seed of ECD01, a turnip (B. rapa) cultivar carrying genes for CR, was provided by Nutrien Ag Solutions (Saskatoon, SK, Canada). DH16516 is a spring-type, clubroot-susceptible, DH canola-quality line of B. napus developed by Dr. Séguin-Swartz at Saskatoon Research and Development Centre, Agriculture and Agri-Food Canada (SRDC, AAFC), Saskatoon, SK, Canada. ECD01 was crossed to DH16516 (pollen donor) to produce F1 progeny. Backcrosses with DH16516 (recurrent parent) were performed to produce the BC1 and BC2 populations. A BC2 plant with resistance to pathotype 5X was chosen as the donor for microspore culture. In total, 84 DH lines were developed by Haplotech Inc. (Winnipeg, Canada) through a fee-for-service contract. Seed from three plants of each DH line was increased in a greenhouse at SRDC for study.
Clubroot strains collected in canola fields in Alberta were characterized based on pathotype and provided by Dr. S. E. Strelkov at the University of Alberta, Canada. The method and experimental design used in this study were as described by Suwabe et al. (2003). Plants were tested for resistance to four pathotypes of P. brassicae (strain F.3-14 for pathotype 3A, F.1-14 for 3D, P. 41-14 for 3H, and LG02 for 5X). Fresh and clean clubbed roots harvested at 4–5 weeks after inoculation of each strain were cut into smaller pieces with scissors, macerated in distilled water for 1–2 h, and blended in a blender at high speed for 2 min. After filtering through eight layers of sterile cheesecloth, resting spores extracted from the clubbed roots were adjusted to a concentration of 1.0 × 107 resting spores/ml in distilled water for plant inoculation.
Seeds of the DH population were sown into Sunshine #3 soilless mix (Sun Gro Horticulture Canada Ltd., Seba Beach, AB, Canada) with Osmocote (Everris NA Inc., Dublin, OH, United States) in 32 pot inserts held by trays (The HC Companies, Twinsburg, OH, United States). Approximately 4 L of water was added to each tray to soak the soilless mix overnight. Seven days after planting, inoculation was performed by adding 15 ml of inoculum (1 × 107 spores/ml) into each pot with 6–9 seedlings of each line. The inoculated plants were grown in a growth chamber set at 22/18°C day/night temperature with a 16-h photoperiod. The canola cultivar “45H29” (resistant to pathotype 3H) and the parental lines (ECD01 and DH16516) were included as controls. Six weeks after inoculation, plants were pulled and the roots were examined for clubroot symptoms.
Clubroot severity was evaluated on a 0–3 scale, where 0 indicates no clubbing, 1 indicates a few small clubs, 2 indicates moderate clubbing, and 3 indicates severe clubbing. A disease severity index (DSI) was calculated for each line using the method of Horiuchi and Hori (1980):
Correlation coefficients of severity among the DH families to four pathotypes of P. brassicae were calculated in Microsoft Excel function “Correl” using the equation:
Significance was determined using t-tests (Iversen and Gergen, 1997). Each line with a resistance response (DSI ≤ 30%) in the initial study was reassessed two more times. Each of these repetitions provided a similar result in most cases. For those lines with inconsistent results, the highest DSI among the three repetitions of the assessment was considered to be the most accurate and was used to characterize the resistance response of the line. DH lines with DSI ≤ 30% were classified as R and those lines with DSI >30% as S lines.
The F1 plants were tested with pathotype 3H, and the BC2 donor plant was only tested with 5X for several reasons. First, the clubroot reaction of a single plant can be assessed for only one pathotype. Second, pathotype 3H was the predominant pathotype in Canada when we obtained the F1 progeny and performed the selection for CR. Similarly, at that time when the donor plant from BC2 was chosen for microspore culture, pathotype 5X was the only new pathotype that had been identified. Third, only a few seeds of F1 were obtained due to difficulties in the interspecific cross, so it was not possible to test multiple pathotypes in the F1 progeny.
DNA was extracted from young leaves of each of the 84 DH lines and parental lines following the DNeasy Plant Mini Handbook from QIAGEN. GBS of the 84 DNA samples and two replications of the parental cultivar ECD01 were performed on an Illumina platform with pair-end sequencing at BGI Americas Corp (Cambridge, MA, United States). Two replications of cv. ECD01 were performed to increase the sequencing depth for this parental line to provide a more accurate call of the genotype at each single-nucleotide polymorphism (SNP) site in the DH population. DH16516 is an important B. napus canola recipient line for introgression of CR at AAFC, Saskatoon, so whole-genome sequencing of the line had already been performed at Plant Biotechnology Centre (Saskatoon, SK, Canada) as part of the generation of a new reference genome (unpublished data). The short reads from the whole-genome sequencing data were used for this study. The program SeqMan NGen 15 (DNASTAR, Madison, WI, United States) was used for short read assembly. ‘‘Whole genome DNA-Seq/Genotyping’’ assembly workflow, ‘‘Reference based assembly-normal workflows’’ assembly type, and ‘‘Automatic Mer size, Automatic Minimum match percentage, High Layout stringency and Medium SNP filtering stringency’’ assembly options were chosen. Short reads from each of the 84 DH samples, parental DH16516, and the combined two replicates of ECD01 were aligned to B. napus reference genome for cv. ‘‘Darmor-bzh’’ version 41.
Identification of variants (SNPs and InDels) in the DNA sequences of each BC2 DH sample relative to the reference genome of B. napus “Darmor-bzh” was performed using SeqMan Pro 15 (DNASTAR, Madison, WI, United States), but only SNPs were used for further study. Comparison of the variants among the 84 BC2 DH samples was carried out using Qseq 15 (DNASTAR).
Genotyping by sequencing-SNP sites were named based on the reference genome (DM: “Darmor-bzh”), the A-genome chromosome (A01–A10), and the position on the reference chromosome sequence. An SNP site was called in a given sample at following criteria: depth >5, Q > 30, and SNP percentage >50%. Since the recipient parent DH16516 and the 84 DH lines were DH lines, all SNP sites should theoretically be homozygous. After filtering, heterozygous genotypes in the parental line DH16516 and the DH lines and monomorphic phenotypes between the parents or among the 84 individuals were removed.
The remaining SNP sites after filtering were further analyzed using JoinMap 4.1 (Kyazma B.V.,Ln.v.A. Wageningen, Netherlands; Van Ooijen, 2011). SNP alleles from the resistant parent (ECD01) were scored as “B,” and those from the susceptible parent (DH16516) as “A.” Marker orders and positions in the genetic map were determined using maximum likelihood in the Kosambi’s model with a minimum logarithm of the odds (LOD) values of 10. Only SNP sites that could be assigned into the 10 chromosomes of the A-genome at LOD scores of 10.0 were kept. The set of filtered SNP sites obtained was used for binning of redundant markers, construction of linkage map, and mapping of QTLs for resistance to clubroot using the QTL IciMapping Inclusive Composite Interval Mapping (ICIM) method (Meng et al., 2015). A linkage map was drawn using MapChart 2.1 (Droevendaalsesteeg 4, Wageningen, Netherlands; Voorrips, 2002) based on the genetic location determined with QTL IciMapping. The LOD score threshold was set using a 1,000-permutation test with a type I error of 0.05 for QTL declaration. The QTL effects were estimated as phenotypic variation explained (PVE) and additive (Add) values by each QTL.
Gene annotation was analyzed using Blast2GO (Conesa et al., 2005) using coding sequences (CDS) of the genes in each of the QTL target regions from 1 Mb upstream to 1 Mb downstream of the SNP markers in the peak regions as determined by IciMapping. Genes related to disease resistance and defense responses were identified using Blast2GO information of the gene description and gene ontology. The most probable Arabidopsis homolog corresponding to each disease resistance gene and the class of disease resistance proteins were obtained using the CDS of the disease resistance gene in the B. napus by Blast search at www.arabidopsis.org.
Bulked segregant analysis (BSA) has been used to detect molecular markers linked to traits of interest, such as disease resistance (Michelmore et al., 1991). In BSA, bulks of plants with contrasting phenotypes are generated. Our previous studies showed that a gene could be genetically mapped by identifying the percentage of polymorphic variants (PPV) in a genome using BSA (Yu et al., 2016; Dakouri et al., 2018; Huang et al., 2019; Karim et al., 2020).
Doubled haploid lines were selected to form a R bulk and a S bulk based on their phenotypes using SNP marker-assisted selection. GBS data from the R and S bulks were aligned onto the B. napus reference genome separately using SeqMan NGen 15 (DNASTAR). Mapping of the QTLs was performed using the PPV method described by Yu et al. (2016) and Dakouri et al. (2018).
The B. rapa reference genome version 3.0 (Zhang et al., 2018) was downloaded from https://brassicadb.org/brad/downloadOverview.php. DNA sequences of the QTL target regions from the A-genome of B. napus were aligned into the B. rapa genome using MegAlign Pro 15 with MAUVE (DNASTAR).
The clubroot reaction of the parental lines (ECD01 and DH16516), controls, and the DH population was assessed against pathotypes 3A, 3D, 3H, and 5X (Table 1). As expected, ECD01 was highly resistant to all pathotypes (0% DSI), DH16516 was highly susceptible (100% DSI), and “45H29” was resistant to pathotype 3H only (Figure 1 and Table 1). The F1 plants from the interspecific crosses of DH16516 × ECD01 were highly resistant to pathotype 3H (0% DSI), which was the predominate pathotype in Canada before the emergence of the 3A, 3D, and 5X. Clubroot severity in response to inoculation with each pathotype in the DH population could be divided into two classes: resistant (R) lines with DSI ≤ 30% and susceptible (S) lines with DSI > 30% (Figure 2). The segregation ratio of R and S was calculated, and the goodness-of-fit was tested with a χ2 test using Microsoft Excel software. Of the four pathotypes, segregation of R and S best fit a 1:3 ratio for pathotype 3A and a 3:1 ratio for pathotypes 3D, 3H, and 5X. These results indicated that resistance to pathotype 3A was controlled by two genes in complementary action, and resistance to pathotypes 3D, 3H, and 5X was controlled by two genes in duplicate action.
Table 1. Genetic analysis of resistance of the parental lines (DH16516, ECD01), controls (cv. “45H29”), and the inoculation of doubled haploid (DH) population derived from BC2 with four pathotypes of Plasmodiophora brassicae based on the clubroot severity (disease severity index, DSI) of each line (Resistant, R, DSI ≤ 30; Susceptible, S, DSI > 30).
Figure 1. Plant phenotypes and clubroot response at 5 weeks after inoculation in the parental lines (Debra and DH16516) and a control cultivar (45H29) to inoculation with four pathotypes (3A, 3D, 3H, and 5X) of Plasmodiophora brassicae under controlled conditions. The bars represent 5 cm in length.
Figure 2. Distribution of clubroot severity (disease severity index, DSI) following inoculation with four pathotypes (3A, 3D, 3H, and 5X) of P. brassicae in a doubled haploid (DH) population derived from a BC2 plant of Brassica rapa ECD01 crossed with B. napus DH16516. Colors in each stacked column represent the proportion of the lines with a DSI value within that decile (= 10% range).
Correlation coefficients among the DSI values for the pathotypes ranged from 0.55 to 0.81, but all were significant at P < 0.01 (Table 2). This indicated that the genes for resistance to the different pathotypes were likely controlled by the same genes or tightly linked genes.
Table 2. Correlation coefficients for clubroot severity after inoculation of DH population derived from BC2 of DH16516 × ECD01 for resistance to four pathotypes of P. brassicae.
Since CR in the DH population originated from the A-genome of B. rapa cv. ECD01, only A-genome DNA sequences in the reference genome B. napus “Darmor” version 4.1 were used for alignment of DNA short reads and discovery of DNA variants (SNPs and InDels). Approximately 219.9 million (M) short reads were obtained from whole-genome sequencing from DH16516, and 53.1% of the reads were assembled into the reference A-genome; 13.8 M sequences were obtained from GBS of ECD01, and 70.9% were assembled into the reference A-genome. A total of 355.3 M short reads from 84 DH lines were obtained, ranging from 0.81 to 11.67 M sequences per line (Supplementary Figure 1). The mean number of reads aligned into the reference genome from each line was 2.3 M (range 0.46–5.22 M, Supplementary Figure 1), and 54.7% were assembled into the reference A-genome.
After the initial filtering, 429 polymorphic SNP sites were left and were distributed to 9 of 10 chromosomes of the reference genome of “Darmor-bzh” (Supplementary Table 1). No polymorphic markers were identified from chromosome A06. There was no correlation between chromosome size and the number of SNP markers identified (r = −0.092) in the population. To remove redundant markers, the 429 SNP sites were further filtered using the binning function in IciMapping, which left only 260 non-redundant SNP sites (Table 3). A genetic map of the nine chromosomes of the A-genome was constructed from the distributed SNP sites (Supplementary Figure 2). The length of each chromosome ranged from 0 (chromosome A06) to 471.8 cM (A01), with an average length of 85.3 cM. Chromosome A01 was much longer than the other linkage groups. The number of SNP sites per chromosome ranged from 0 (A06) to 152 (A01), with a mean of 26 SNPs per chromosome. The SNP interval of each chromosome ranged from 0.8 to 4.8 cM, with a mean of 3.3 cM (Supplementary Table 1).
Table 3. QTL position, phenotypic variation explained (PVE), additive (Add), the logarithm of the odds (LOD), and confidence interval (CI) for the QTLs originating from Brassica rapa ECD01 for resistance to four pathotypes of P. brassicae (permutations = 1,000).
Mapping of the QTLs was performed using the linkage map (Supplementary Figure 1) and trait values for resistance to each pathotype (3A, 3D, 3H, and 5X). Two QTLs were identified: a QTL designated as Rcr10ECD01 on A03, with a peak at the SNP markers DM_A03_12570715 and DM_A03_10873502, and a QTL designated as Rcr9ECD01 (Figure 3), located near the previously identified genes Rcr9 and Rcr9wa (Yu et al., 2017; Karim et al., 2020) on A08, with a peak at DM_A08_10325589 and DM_A08_10529713 (Table 3). Resistance to pathotypes 3A, 3D, and 3H was associated with the two QTLs (Rcr10ECD01 and Rcr9ECD01), but resistance to 5X was only associated with Rcr9ECD01 (Table 3). LOD, PVE, Add values, and CI from the estimated QTL position varied between the QTLs, ranging from 5.2 to 12.2 for LOD, 16.2 to 43.3% for PVE, 14.6 to 25.4 for Add, and 1.5 to 12 cM for CI (Table 3). The values of Add for the two QTLs were positive, indicating that the resistant loci were derived from the resistant parent ECD01.
Searches for candidate genes for Rcr10ECD01 and Rcr9ECD01 that encoded disease resistance proteins and defense-related genes were performed using CDS of the reference genome in the target region including 1 Mb up- and downstream of the left and right markers (Table 3).
Rcr10ECD01, which was responsible for resistance to pathotypes 3A, 3D, and 3H, was mapped into chromosome A03, with a peak at SNP markers DM_A03_10873502 and DM_A03_12570715 (Table 3). There are 676 genes in this 3.7 Mb region (Supplementary Table 3). Among the genes, one gene (BnaA03g25330D) encoded a disease resistance protein (Table 4), and 24 genes encoded proteins with functions related to plant defense response (Supplementary Table 3). BnaA03g25330D is homologous to the Arabidopsis gene AT5G22690, which encoded a TNL protein (Table 4).
Table 4. A list of genes encoding proteins associated with plant disease resistance through BLAST2GO and Blast searches with CDS in the QTL target regions at https://www.arabidopsis.org/Blast/index.jsp.
Rcr9ECD01, which was responsible for resistance to all four pathotypes, was mapped into chromosome A08, with a peak at SNP markers DM_A08_10325589 and DM_A08_10529713. There were 338 genes in this 2.2 Mb region (Table 4 and Supplementary Table 3). Two genes (BnaA08g10100D and BnaA08g11840D) encoded disease resistance proteins, and BnaA08g10100D was homologous to the previously cloned resistance gene Crr1. BnaA08g10100D and BnaA08g11840D were homologous to the Arabidopsis genes AT5G11250 and AT4G33300, respectively. AT5G11250 encodes an atypical TNL protein and AT4G33300 encodes a member of the activated disease resistance 1 family nucleotide-binding leucine-rich repeat immune receptors (Table 4). Also, this region contained 10 genes that encoded proteins with defense-related functions (Supplementary Table 3).
Of the 84 DH lines, 19 lines were resistant to almost all the pathotypes. They all carried alleles from the resistant parent ECD01 (SNP genotype “B”) with Rcr10ECD01 (DM_A03_10873502 and DM_A03_12570715) and Rcr9ECD01 (DM_A08_10325589 and DM_A08_10529713). Also, 17 lines were susceptible to almost all the pathotypes and all of them carried alleles from the susceptible parent line DH16516 (SNP genotype “A”) for the two QTLs. As a result, the R bulk was formed from the 19 R DH lines, while the S bulk was formed from the 17 S DH lines for the BSA (Supplementary Table 4).
A total of 93.5 M short reads from the R bulk and 69.4 M short reads from the S bulk were aligned into the B. napus reference genome. A PPV peak (25–30%) occurred within the physical interval 9–14 Mb on chromosome A03 and the other peak (25–36%) within the physical interval 9–12 Mb on chromosome A08 (Figure 4), which indicated that Rcr10ECD01 and Rcr9ECD01 resided in the intervals of chromosomes A03 and A08, respectively. This result is consistent with that from the above QTL analysis.
Figure 4. Distribution of polymorphic variants (%): One peak for Rcr10ECD01 on chromosome A03 and the other for Rcr9ECD01 on A08 were identified through bulk segregant analysis with the mapping method of the percentage of polymorphic variants described by Yu et al. (2016) and Dakouri et al. (2018).
Most of the genes or QTLs for CR in Brassica species containing the A-genome that have been identified were from B. rapa. In this study, the DH population was developed with introgression of QTLs from B. rapa, so the QTL target regions of chromosome A03 and A08 of B. napus were compared with those of B. rapa.
The B. rapa reference genome “Chiifu” version 3.0 is the most recent version available for the “Chiifu” reference genome (Zhang et al., 2018). The 3.7 Mb region from 9.8 to 13.5 Mb of B. napus chromosome A03, which included a fragment of the markers DM_A03_10873502 and DM_A03_12570715 for Rcr10ECD01, was homologous to the region 11.0–16 Mb of “Chiifu” A03 (Figure 5). Rcr9ECD01, located on the 2.2 Mb length from 9.3 to 11.5 Mb of B. napus chromosome A08, which included SNP markers DM_A08_10325589 and DM_A08_10529713, was homologous to the region 12.0–14.5 Mb of A08 in B. rapa “Chiifu” (Figure 5).
Figure 5. Maps of the Rcr10ECD01 and Rcr9ECD01 target regions in A03 and A08 of B. napus “Darmor” and the homologous regions of the B. rapa reference genome “Chiifu” version 3.0. Different colors represent the sequenced region of the B. rapa reference genome “Chiifu” version 3.0.
Clubroot has the potential to become an important constraint to canola production on the Canadian Prairies. Pathotype 3H was (and likely still is) the predominant pathotype in the Prairie region, pathotype 5X was the first new pathotype identified as virulent on resistant canola cultivars such as “45H29,” and 3A and 3D have become the most prevalent among the new virulent pathotypes (Hollman et al., 2021). Therefore, these four pathotypes were selected for this study.
Two QTLs for resistance to the four pathotypes of P. brassicae derived from B. rapa ECD01 were transferred to, identified, and mapped in a DH population of B. napus. The DH population was segregated in a 1:3 (R:S) ratio for resistance to pathotype 3A. This indicated that resistance to pathotype 3A was controlled by two genes in complementary action. The segregation ratio for resistance to pathotype 3H was 3:1, which was also the most likely fit for pathotypes 3D and 5X. This indicated that resistance to all three pathotypes in the DH population was controlled by two genes in duplicate action. Two QTLs, Rcr10ECD01 and Rcr9ECD01, for resistance to pathotypes 3A, 3D, and 3H were identified, which was consistent with the genetic analysis of phenotype ratios. However, only one QTL, Rcr9ECD01, was identified for resistance to 5X, although the segregation ratio was close to 3:1. This inconsistency merits further investigation.
In general, strong resistance to clubroot pathotypes is controlled by single dominant genes such as Rcr1–Rcr7 (Chu et al., 2014; Yu et al., 2016; Huang et al., 2017, 2019; Yu et al., 2017; Dakouri et al., 2018; Chang et al., 2019; Karim et al., 2020). Two genes in duplicate action (Rcr8 on chromosome A02 and Rcr9 on chromosome A08 from B. rapa line T19) that conferred resistance to pathotype 5X were reported previously (Yu et al., 2017). Similarly, a previous study indicated that neither Crr1 nor Crr2 on their own conferred resistance to Japanese strain “Wakayama-01” of P. brassica; resistance was only expressed when resistance alleles were present at both loci (Suwabe et al., 2003). In this study, the QTL for resistance to pathotype 3A derived from ECD01 may behave similarly to Crr1 and Crr2.
The number of SNP sites per chromosome is usually correlated with chromosome size in mapping populations (Yu et al., 2016) but was not correlated in this study. This unusual result likely occurred because the BC2 donor plant used for microspore culture carried a large fragment of chromosome A01 originating from ECD01 but smaller fragments of the other chromosomes from ECD01.
In this study, the target region for Rcr10ECD01 was defined as 9.8–13.5 Mb of B. napus chromosome A03 using QTL analysis. A similar interval (9–14 Mb) for Rcr10ECD01 was obtained using the identification of the PPV with BSA. The region for Rcr10ECD01 in B. napus was homologous to the 11.0–16.0 Mb region of A03 in the B. rapa “Chiifu” version 3.0. This was a distinct genetic region from Rcr1, Rcr2, Rcr4, and Rcr5 for resistance to pathotypes of P. brassicae (Figure 4). The genes Rcr1, Rcr2, and Rcr4, which confer resistance to pathotypes of P. brassica, have previously been mapped into chromosome A03 of B. rapa “Chiifu” version 3.0 at ∼25 Mb region (Chu et al., 2014; Yu et al., 2016; Huang et al., 2017), while Rcr5 was also mapped at ∼24 Mb region in that chromosome (Huang et al., 2019; Figure 4). Rcr1, Rcr2, and Rcr4 were subsequently co-localized with the cloned CR genes CRa/CRbkato (Ueno et al., 2012; Hatakeyama et al., 2017), while Rcr5 was located in a region close to CRa/CRbkato. In addition, resistance genes Rcr1, Rcr2, Rcr4, and Rcr5 were identified for resistance to pathotype 3H, not for 3A, 3D, or 5X. Several CR genes or QTLs, such as PbBa3.2 (Chen et al., 2013), CRd (Pang et al., 2018), Crr3 (Hirai et al., 2004), and CRk (Sakamoto et al., 2008) for resistance to clubroot strains collected from Japan and China, have been mapped into the regions different from CRa/CRbkato. Similarly, BraA.CR.c for resistance was mapped into chromosome A03 in turnip cvs. ECD01, ECD02, and ECD04 (Hirani et al., 2018). The relationship of Rcr10ECD01 to these previously identified genes needs to be determined. Also, CRb was identified in a Chinese cabbage cv. “CR Shinki” was originally derived from ECD01 for resistance to P. brassica strains collected in South Korea (Piao et al., 2004). It was located in a genetic region close to CRa/CRbkato. However, no QTL in the CRb region was identified in this study.
A QTL, identified and designated as Rcr9ECD01 (because it was mapped into the genetic region of Rcr9 and was originally derived from B. rapa cv. ECD01), conferred resistance to all four pathotypes (3A, 3D, 3H, and 5X) assessed in this study. Rcr9ECD01 was located on the 2.2 Mb length from 9.3 to 11.5 Mb of B. napus chromosome A08 using QTL analysis. The Rcr9ECD01 interval was confirmed through the identification of PPV with BSA, located in the physical interval 9–12 Mb. The region of Rcr9ECD01 in B. napus corresponded to 12.0–14.5 Mb of A08 in B. rapa “Chiifu” version 3.0 (Figure 4).
Previously, our laboratory had identified Rcr9 for resistance to pathotype 5X in B. rapa breeding line T19, which originated from German turnip cv. “Pluto” (Yu et al., 2017). The proposed position of Rcr9 spanned a large interval (6.48 Mb) of chromosome A08, including the genome region of Rcr3 and Rcr9ECD01. However, several breeding lines that carried Rcr9 were resistant to 5X, but not to 3A, 3D (Yu, unpublished), and 3H (Yu et al., 2017). This difference in phenotype indicated that Rcr9 differed from Rcr9ECD01. Another resistance gene, designated as Rcr9wa, has also been identified from a turnip differential line in the ECD. It originated from cv. “Waaslander” (ECD04), provided resistance to pathotype 5X, and was mapped into the same region as Rcr9 (Karim et al., 2020). Rcr9wa was mapped based on flanking markers into 12.3–12.6 Mb of chromosome A08 (smaller interval than Rcr9). In addition, another resistance gene originated from cv. “Waaslander” and conferred resistance to pathotype 3H, designated as Rcr3, has been mapped into chromosome A08, flanked by SNP markers in position 11.3–11.6 Mb in the B. rapa “Chiifu” reference genome version 3.0 (Karim et al., 2020). The position of Rcr3 was separated from Rcr9ECD01 (Figure 4). Also, gene BraA.CR.b for resistance to pathotype 3H was previously identified from the turnip differentials ECD01, ECD02, ECD03, and ECD04 and mapped into chromosome A08 (Hirani et al., 2018), but no information on the genome region corresponding to the B. rapa “Chiifu” reference genome version 3.0 was provided. Several genes for resistance to collections of P. brassicae from Japan and China, including Crr1 (Suwabe et al., 2003), CRs (Laila et al., 2019), PbBa8.1 (Chen et al., 2013), and qBrCR38-2 (Zhu et al., 2019), have also been mapped into chromosome A08. The cloned CR gene Crr1 was highly homologous to Bra020861 in the B. rapa reference genome version 1.5 and to BraA08g014480 in the B. rapa reference genome version 3.0, which is located in the Rcr9ECD01 genomic region. However, breeding lines carrying Crr1 gene did not show resistance to the strains of P. brassica used in this study (Yu, unpublished). Therefore, Rcr9ECD01 is unlikely the same as Crr1. The relationship of Rcr9ECD01 with CRs (Laila et al., 2019), PbBa8.1 (Chen et al., 2013), and qBrCR38-2 needs to be determined.
CRc was identified in the Chinese cabbage cv. “CR Kanko” derived from “Debra,” which was located into chromosome A02 (Sakamoto et al., 2008). However, this gene was not found in the DH population used for this study.
Analysis of QTLs has been used for the identification of several major genes for resistance to clubroot (Yu et al., 2017). A QTL that can be consistently detected with a PVE of >10% of trait value can be designated as the main effect QTL or major QTL (Wang et al., 2019). In this study, QTLs Rcr10ECD01 and Rcr9ECD01 were identified with 16.2 to 43.3% PVE. Rcr10ECD01 was identified based on the response to inoculation with pathotypes 3A, 3D, and 3H. Rcr9ECD01 was identified based on the response to inoculation with all of the pathotypes used in this study. Therefore, both Rcr10ECD01 and Rcr9ECD01 appear to be major QTLs. The presence of the two major QTLs was also confirmed through BSA, which is consistent with the result obtained from the QTL analysis.
Clubroot severity in the DH lines in response to inoculation with the individual pathotypes was highly correlated, which indicated that resistance to these pathotypes was likely controlled by the same gene or tightly linked genes. However, the identification of QTLs in this study was based on relatively rough gene mapping, so it could not be determined if resistance to the pathotypes was controlled by a single gene or tightly linked genes. More detailed studies are in progress.
The original contributions presented in the study are included in the article/Supplementary Material, further inquiries can be directed to the corresponding author.
FY conceived the study, designed the experiments, performed the data analysis, and drafted the manuscript. YZ and JW developed the population, performed the data analysis, and collected phenotypic data. QC and MK performed the data analysis. BG and GP provided important materials. All authors reviewed the manuscript and approved the final draft.
This study was funded by the Saskatchewan Agriculture Development Fund, SaskCanola, and Western Grains Research Foundation.
The authors declare that the research was conducted in the absence of any commercial or financial relationships that could be construed as a potential conflict of interest.
All claims expressed in this article are solely those of the authors and do not necessarily represent those of their affiliated organizations, or those of the publisher, the editors and the reviewers. Any product that may be evaluated in this article, or claim that may be made by its manufacturer, is not guaranteed or endorsed by the publisher.
The authors thank S. E. Strelkov for providing the strains of P. brassicae, Nutrien for the B. rapa donor ECD01, G. Séguin-Swartz for the recipient B. napus line DH16516, and M. Kehler and L. Liu for technical assistance with plant phenotyping.
The Supplementary Material for this article can be found online at: https://www.frontiersin.org/articles/10.3389/fpls.2021.785989/full#supplementary-material
Supplementary Figure 1 | The total numbers of sequences obtained from genotype by sequencing and the number of sequence aligned into the A-genome of B. napus “Darmor-bzh” version 4.1 for each line in the DH population.
Supplementary Figure 2 | The linkage map of A-genome chromosomes of B. napus developed from 260 SNP sites. Note that the A06 chromosome did not contain any SNPs and so is not represented.
Botero-Ramírez, A., Laperche, A., Guichard, S., Jubault, M., Gravot, A., Strelkov, S. E., et al. (2020). Clubroot symptoms and resting spore production in a doubled haploid population of Oilseed Rape (Brassica napus) are controlled by four main QTLs. Front. Plant Sci. 11:1998. doi: 10.3389/fpls.2020.604527
Buczacki, S., Toxopeus, H., Mattusch, P., Johnston, T., Dixon, G., and Hobolth, L. (1975). Study of physiologic specialization in Plasmodiophora brassicae: proposals for attempted rationalization through an international approach. Trans. Br. Mycol. Soc. 65, 295–303.
Chang, A., Lamara, M., Wei, Y., Hu, H., Parkin, I. A., Gossen, B. D., et al. (2019). Clubroot resistance gene Rcr6 in Brassica nigra resides in a genomic region homologous to chromosome A08 in B. rapa. BMC Plant Biol. 19:224. doi: 10.1186/s12870-019-1844-5
Chen, J., Jing, J., Zhan, Z., Zhang, T., Zhang, C., and Piao, Z. (2013). Identification of novel QTLs for isolate-specific partial resistance to Plasmodiophora brassicae in Brassica rapa. PLoS One 8:e85307. doi: 10.1371/journal.pone.0085307
Chu, M., Song, T., Falk, K. C., Zhang, X., Liu, X., Chang, A., et al. (2014). Fine mapping of Rcr1 and analyses of its effect on transcriptome patterns during infection by Plasmodiophora brassicae. BMC Genomics 15:1166. doi: 10.1186/1471-2164-15-1166
Conesa, A., Götz, S., García-Gómez, J. M., Terol, J., Talón, M., and Robles, M. (2005). Blast2GO: a universal tool for annotation, visualization and analysis in functional genomics research. Bioinformatics 21, 3674–3676. doi: 10.1093/bioinformatics/bti610
Dakouri, A., Lamara, M., Karim, M. M., Wang, J., Chen, Q., Gossen, B. D., et al. (2021). Identification of resistance loci against new pathotypes of Plasmodiophora brassicae in Brassica napus based on genome-wide association mapping. Sci. Rep. 11:6599. doi: 10.1038/s41598-021-85836-9
Dakouri, A., Zhang, X., Peng, G., Falk, K. C., Gossen, B. D., Strelkov, S. E., et al. (2018). Analysis of genome-wide variants through bulked segregant RNA sequencing reveals a major gene for resistance to Plasmodiophora brassicae in Brassica oleracea. Sci. Rep. 8:17657. doi: 10.1038/s41598-018-36187-5
Diederichsen, E., Frauen, M., Linders, E. G., Hatakeyama, K., and Hirai, M. (2009). Status and perspectives of clubroot resistance breeding in crucifer crops. J.Plant Growth Regul. 28, 265–281. doi: 10.1007/s00344-009-9100-0
Fredua-Agyeman, R., and Rahman, H. (2016). Mapping of the clubroot disease resistance in spring Brassica napus canola introgressed from European winter canola cv.‘Mendel’. Euphytica 211, 201–213.
Gowers, S. (1982). The transfer of characters from Brassica campestris L. to Brassica napus L.: production of clubroot-resistant oil-seed rape (B. napus ssp oleifera). Euphytica 31, 971–976. doi: 10.1007/bf00039237
Hasan, M. J., and Rahman, H. (2016). Genetics and molecular mapping of resistance to Plasmodiophora brassicae pathotypes 2, 3, 5, 6, and 8 in rutabaga (Brassica napus var. napobrassica). Genome 59, 805–815. doi: 10.1139/gen-2016-0034
Hatakeyama, K., Niwa, T., Kato, T., Ohara, T., Kakizaki, T., and Matsumoto, S. (2017). The tandem repeated organization of NB-LRR genes in the clubroot-resistant CRb locus in Brassica rapa L. Mol. Genet. Genom. 292, 397–405. doi: 10.1007/s00438-016-1281-1
Hatakeyama, K., Suwabe, K., Tomita, R. N., Kato, T., Nunome, T., Fukuoka, H., et al. (2013). Identification and characterization of Crr1a, a gene for resistance to clubroot disease (Plasmodiophora brassicae Woronin) in Brassica rapa L. PLoS One 8:e54745. doi: 10.1371/journal.pone.0054745
Hirai, M. (2006). Genetic analysis of clubroot resistance in Brassica crops. Breed. Sci. 56, 223–229. doi: 10.1270/jsbbs.56.223
Hirai, M., Harada, T., Kubo, N., Tsukada, M., Suwabe, K., and Matsumoto, S. (2004). A novel locus for clubroot resistance in Brassica rapa and its linkage markers. Theor. Appl. Genet. 108, 639–643. doi: 10.1007/s00122-003-1475-x
Hirani, A. H., Gao, F., Liu, J., Fu, G., Wu, C., Mcvetty, P. B., et al. (2018). Combinations of independent dominant loci conferring clubroot resistance in all four turnip accessions (Brassica rapa) from the European clubroot differential set. Front. Plant Sci. 9:1628. doi: 10.3389/fpls.2018.01628
Hollman, K., Hwang, S., Manolii, V., and Strelkov, S. (2021). Pathotypes of Plasmodiophora brassicae collected from clubroot resistant canola (Brassica napus L.) cultivars in western Canada in 2017-2018. Can. J. Plant Pathol. 43, 622–630.
Horiuchi, S., and Hori, M. (1980). A simple greenhouse technique for obtaining high levels of clubroot [disease] incidence [of Brassica vegetables]. Bull. Chugoku Natl. Agric. Exp. Stn. Ser E. Environ. Div. 17, 33–55.
Huang, Z., Peng, G., Gossen, B., and Yu, F. (2019). Fine mapping of a clubroot resistance gene from turnip using SNP markers identified from bulked segregant RNA-Seq. Mol. Breed. 39, 1–10. doi: 10.3389/fpls.2017.01448
Huang, Z., Peng, G., Liu, X., Deora, A., Falk, K. C., Gossen, B. D., et al. (2017). Fine mapping of a clubroot resistance gene in Chinese cabbage using SNP markers identified from bulked segregant RNA sequencing. Front. Plant Sci. 8:1448.
Iversen, G. R., and Gergen, M. (1997). Statistics: The Conceptual Approach. New York, NY: Springer Science & Business Media.
Johnston, T. (1974). Transfer of disease resistance from Brassica campestris L. to rape (B. napus L.). Euphytica 23, 681–683.
Karim, M., Dakouri, A., Zhang, Y., Chen, Q., Peng, G., Strelkov, S. E., et al. (2020). Two clubroot-resistance genes, Rcr3 and Rcr9wa, mapped in Brassica rapa using bulk segregant RNA sequencing. Int. J. Mol. Sci. 21:5033. doi: 10.3390/ijms21145033
Laila, R., Park, J.-I., Robin, A. H. K., Natarajan, S., Vijayakumar, H., Shirasawa, K., et al. (2019). Mapping of a novel clubroot resistance QTL using ddRAD-seq in Chinese cabbage (Brassica rapa L.). BMC Plant Biol. 19:13. doi: 10.1186/s12870-018-1615-8
Lammerink, J. (1970). Inter-specific transfer of clubroot resistance from Brassica campestris L. to B. napus L. N. Z. J. Agric. Res. 13, 105–110.
Lee, J., Izzah, N. K., Choi, B.-S., Joh, H. J., Lee, S.-C., Perumal, S., et al. (2016). Genotyping-by-sequencing map permits identification of clubroot resistance QTLs and revision of the reference genome assembly in cabbage (Brassica oleracea L.). DNA Res. 23, 29–41. doi: 10.1093/dnares/dsv034
Manzanares-Dauleux, M., Delourme, R., Baron, F., and Thomas, G. (2000). Mapping of one major gene and of QTLs involved in resistance to clubroot in Brassica napus. Theor. Appl. Genet. 101, 885–891. doi: 10.1007/s001220051557
Matsumoto, E., Yasui, C., Ohi, M., and Tsukada, M. (1998). Linkage analysis of RFLP markers for clubroot resistance and pigmentation in Chinese cabbage (Brassica rapa ssp. pekinensis). Euphytica 104, 79–86.
Meng, L., Li, H., Zhang, L., and Wang, J. (2015). QTL IciMapping: integrated software for genetic linkage map construction and quantitative trait locus mapping in biparental populations. Crop J. 3, 269–283. doi: 10.1016/j.cj.2015.01.001
Michelmore, R. W., Paran, I., and Kesseli, R. V. (1991). Identification of markers linked to disease-resistance genes by bulked segregant analysis: a rapid method to detect markers in specific genomic regions by using segregating populations. PNAS 88, 9828–9832. doi: 10.1073/pnas.88.21.9828
Morinaga, T. (1934). Interspecific hybridization in Brassica VI. The cytology of F 1 hybrids of B. juncea and B. nigra. Cytologia 6, 62–67. doi: 10.1508/cytologia.6.62
Nagaharu, U., and Nagaharu, N. (1935). Genome analysis in Brassica with special reference to the experimental formation of B. napus and peculiar mode of fertilization. Jpn. J. Bot. 7, 389–452.
Nikolaev, S. I., Berney, C., Fahrni, J. F., Bolivar, I., Polet, S., Mylnikov, A. P., et al. (2004). The twilight of Heliozoa and rise of Rhizaria, an emerging supergroup of amoeboid eukaryotes. Proc. Natl. Acad. Sci. 101, 8066–8071. doi: 10.1073/pnas.0308602101
Pageau, D., Lajeunesse, J., and Lafond, J. (2006). Impact de l’hernie des crucifères [Plasmodiophora brassicae] sur la productivité et la qualité du canola. Can. J. Plant Pathol. 28, 137–143. doi: 10.1080/07060660609507280
Pang, W., Fu, P., Li, X., Zhan, Z., Yu, S., and Piao, Z. (2018). Identification and mapping of the clubroot resistance gene CRd in Chinese cabbage (Brassica rapa ssp. pekinensis). Front. Plant Sci. 9:653. doi: 10.3389/fpls.2018.00653
Pang, W., Liang, S., Li, X., Li, P., Yu, S., Lim, Y. P., et al. (2014). Genetic detection of clubroot resistance loci in a new population of Brassica rapa. Hortic. Environ. Biotechnol. 55, 540–547.
Peng, L., Zhou, L., Li, Q., Wei, D., Ren, X., Song, H., et al. (2018). Identification of quantitative trait loci for clubroot resistance in Brassica oleracea with the use of Brassica SNP microarray. Front. Plant Sci. 9:822. doi: 10.3389/fpls.2018.00822
Piao, Z., Deng, Y., Choi, S., Park, Y., and Lim, Y. (2004). SCAR and CAPS mapping of CRb, a gene conferring resistance to Plasmodiophora brassicae in Chinese cabbage (Brassica rapa ssp. pekinensis). Theor. Appl. Genet. 108, 1458–1465. doi: 10.1007/s00122-003-1577-5
Piao, Z., Ramchiary, N., and Lim, Y. P. (2009). Genetics of clubroot resistance in Brassica species. J. Plant Growth Regul. 28, 252–264.
Sakamoto, K., Saito, A., Hayashida, N., Taguchi, G., and Matsumoto, E. (2008). Mapping of isolate-specific QTLs for clubroot resistance in Chinese cabbage (Brassica rapa L. ssp. pekinensis). Theor. Appl. Genet. 117, 759–767. doi: 10.1007/s00122-008-0817-0
Strelkov, S. E., Hwang, S.-F., Manolii, V. P., Cao, T., Fredua-Agyeman, R., Harding, M. W., et al. (2018). Virulence and pathotype classification of Plasmodiophora brassicae populations collected from clubroot resistant canola (Brassica napus) in Canada. Can. J. Plant Pathol. 40, 284–298.
Suwabe, K., Tsukazaki, H., Iketani, H., Hatakeyama, K., Fujimura, M., Nunome, T., et al. (2003). Identification of two loci for resistance to clubroot (Plasmodiophora brassicae Woronin) in Brassica rapa L. Theor. Appl. Genet. 107, 997–1002. doi: 10.1007/s00122-003-1309-x
Suwabe, K., Tsukazaki, H., Iketani, H., Hatakeyama, K., Kondo, M., Fujimura, M., et al. (2006). Simple sequence repeat-based comparative genomics between Brassica rapa and Arabidopsis thaliana: the genetic origin of clubroot resistance. Genetics 173, 309–319. doi: 10.1534/genetics.104.038968
Ueno, H., Matsumoto, E., Aruga, D., Kitagawa, S., Matsumura, H., and Hayashida, N. (2012). Molecular characterization of the CRa gene conferring clubroot resistance in Brassica rapa. Plant Mol. Biol. 80, 621–629. doi: 10.1007/s11103-012-9971-5
Van Ooijen, J. (2011). Multipoint maximum likelihood mapping in a full-sib family of an outbreeding species. Genetics Res. 93, 343–349. doi: 10.1017/S0016672311000279
Voorrips, R. (2002). MapChart: software for the graphical presentation of linkage maps and QTLs. J. Hered. 93, 77–78. doi: 10.1093/jhered/93.1.77
Voorrips, R. E. (1995). Plasmodiophora brassicae: aspects of pathogenesis and resistance in Brassica oleracea. Euphytica 83, 139–146.
Wang, L., Yang, X., Cui, S., Mu, G., Sun, X., Liu, L., et al. (2019). QTL mapping and QTL× environment interaction analysis of multi-seed pod in cultivated peanut (Arachis hypogaea L.). Crop J. 7, 249–260. doi: 10.1016/j.cj.2018.11.007
Werner, S., Diederichsen, E., Frauen, M., Schondelmaier, J., and Jung, C. (2008). Genetic mapping of clubroot resistance genes in oilseed rape. Theor. Appl. Genet. 116, 363–372. doi: 10.1007/s00122-007-0674-2
Yu, F., Zhang, X., Huang, Z., Chu, M., Song, T., Falk, K. C., et al. (2016). Identification of genome-wide variants and discovery of variants associated with Brassica rapa clubroot resistance gene Rcr1 through bulked segregant RNA sequencing. PLoS One 11:e0153218. doi: 10.1371/journal.pone.0153218
Yu, F., Zhang, X., Peng, G., Falk, K. C., Strelkov, S. E., and Gossen, B. D. (2017). Genotyping-by-sequencing reveals three QTL for clubroot resistance to six pathotypes of Plasmodiophora brassicae in Brassica rapa. Sci. Rep. 7:4516. doi: 10.1038/s41598-017-04903-2
Zhang, L., Cai, X., Wu, J., Liu, M., Grob, S., Cheng, F., et al. (2018). Improved Brassica rapa reference genome by single-molecule sequencing and chromosome conformation capture technologies. Hortic. Res. 5, 1–11.
Keywords: Brassica napus, Brassica rapa, Plasmodiophora brassicae, clubroot, genotyping by sequencing, ECD01, resistance, pathotype
Citation: Yu F, Zhang Y, Wang J, Chen Q, Karim MM, Gossen BD and Peng G (2022) Identification of Two Major QTLs in Brassica napus Lines With Introgressed Clubroot Resistance From Turnip Cultivar ECD01. Front. Plant Sci. 12:785989. doi: 10.3389/fpls.2021.785989
Received: 29 September 2021; Accepted: 02 December 2021;
Published: 12 January 2022.
Edited by:
Harsh Raman, New South Wales Department of Primary Industries, AustraliaReviewed by:
Chuchuan Fan, Huazhong Agricultural University, ChinaCopyright © 2022 Yu, Zhang, Wang, Chen, Karim, Gossen and Peng. This is an open-access article distributed under the terms of the Creative Commons Attribution License (CC BY). The use, distribution or reproduction in other forums is permitted, provided the original author(s) and the copyright owner(s) are credited and that the original publication in this journal is cited, in accordance with accepted academic practice. No use, distribution or reproduction is permitted which does not comply with these terms.
*Correspondence: Fengqun Yu, ZmVuZ3F1bi55dUBhZ3IuZ2MuY2E=
Disclaimer: All claims expressed in this article are solely those of the authors and do not necessarily represent those of their affiliated organizations, or those of the publisher, the editors and the reviewers. Any product that may be evaluated in this article or claim that may be made by its manufacturer is not guaranteed or endorsed by the publisher.
Research integrity at Frontiers
Learn more about the work of our research integrity team to safeguard the quality of each article we publish.