- 1Natural and Medical Sciences Research Center, University of Nizwa, Nizwa, Oman
- 2Department of Biotechnology, Yeungnam University, Gyeongsan, South Korea
- 3Department of Applied Biology, School of Biological Science, University of Science and Technology, Ri-Bhoi, India
Since the beginning of space exploration, researchers have been exploring the role of microgravity, cosmic radiation, and other aspects of the space environment on plant growth and development. To create superior crop varieties and achieve noticeable success in the space environment, several types of research have been conducted thus far. Space-grown plants have been exposed to cosmic radiation and microgravity, which has led to the generation of crop varieties with diverse genotypes and phenotypes arising from different cellular, subcellular, genomic, chromosomal, and biochemical changes. DNA damage and chromosomal aberrations due to cosmic radiation are the major factors responsible for genetic polymorphism and the generation of crops with modified genetic combinations. These changes can be used to produce next-generation crop varieties capable of surviving diverse environmental conditions. This review aims to elucidate the detailed molecular mechanisms and genetic mutations found in plants used in recent space crop projects and how these can be applied in space breeding programmes in the future.
Introduction
Currently, space research has gained widespread attention and there have been considerable advances in in-depth space exploration (Frisbee, 2003; Anqi, 2021; Young and Sutton, 2021). Beyond the ozone layer, space gradually loses gravity, magnetic field, air, and environmental pressure (Eichinger et al., 2020). As a result, it receives large quantities of cosmic radiation, the effects of which are still not well understood (Bagshaw, 2008; Zhang et al., 2014). Additionally, space is a hyper-vacuum environment. Some scientists are working towards establishing life on Mars, and extensive laboratory and other researches have been conducted in order to do so (McKay, 1998, 2010; Simoneit et al., 1998; Westall et al., 2013). However, the absence of a magnetic field and other factors on Mars makes it difficult for plants to grow there (Acuña et al., 2001; Connerney et al., 2001). The International Space Station (ISS) an artificial habitable satellite that was launched and established in 1998 and is currently functioning very well (Thirsk et al., 2009). It has been used as a research centre for astrobiology studies in a microgravity environment (Figure 1; Penley et al., 2002; Jules et al., 2004; Rabbow et al., 2009, 2012, 2015). Space travel is not very quiet, as the noise generated by the spacecraft remains constantly high. Moreover, the higher sound frequency can be a deterrent to living cells. It was recently reported that China harvested the first batch of rice that it is calling “space rice” or “rice from heaven” (Anqi, 2021). During the 23days of China’s Chang’e-5 mission to lunar orbit, they had sent 40g of rice with it (Ghosh, 2021). Upon returning to Earth and completing the lunar mission, the rice grains were grown in the lab, and then in the field. Seeds were harvested at the South China Agriculture University, Guangzhou. The seeds were 1cm long and the best seeds were identified through genomic selection (Voss-Fels et al., 2019) and bred in laboratories for further investigation. During the journey in space, the rice seeds were exposed to cosmic radiation and may have undergone intense mutation that could potentially lead to higher yields. The space environment may influence plant growth and development and cause changes in the genetic makeup of the seeds, which will likely experience an increase in chromosomal aberrations when they are transported into space, and this could be very useful in terms of crop breeding (Resende et al., 2021). We discuss here some details about the phenotypic, genomic, and molecular events that plants incur on exposure to the space environment.
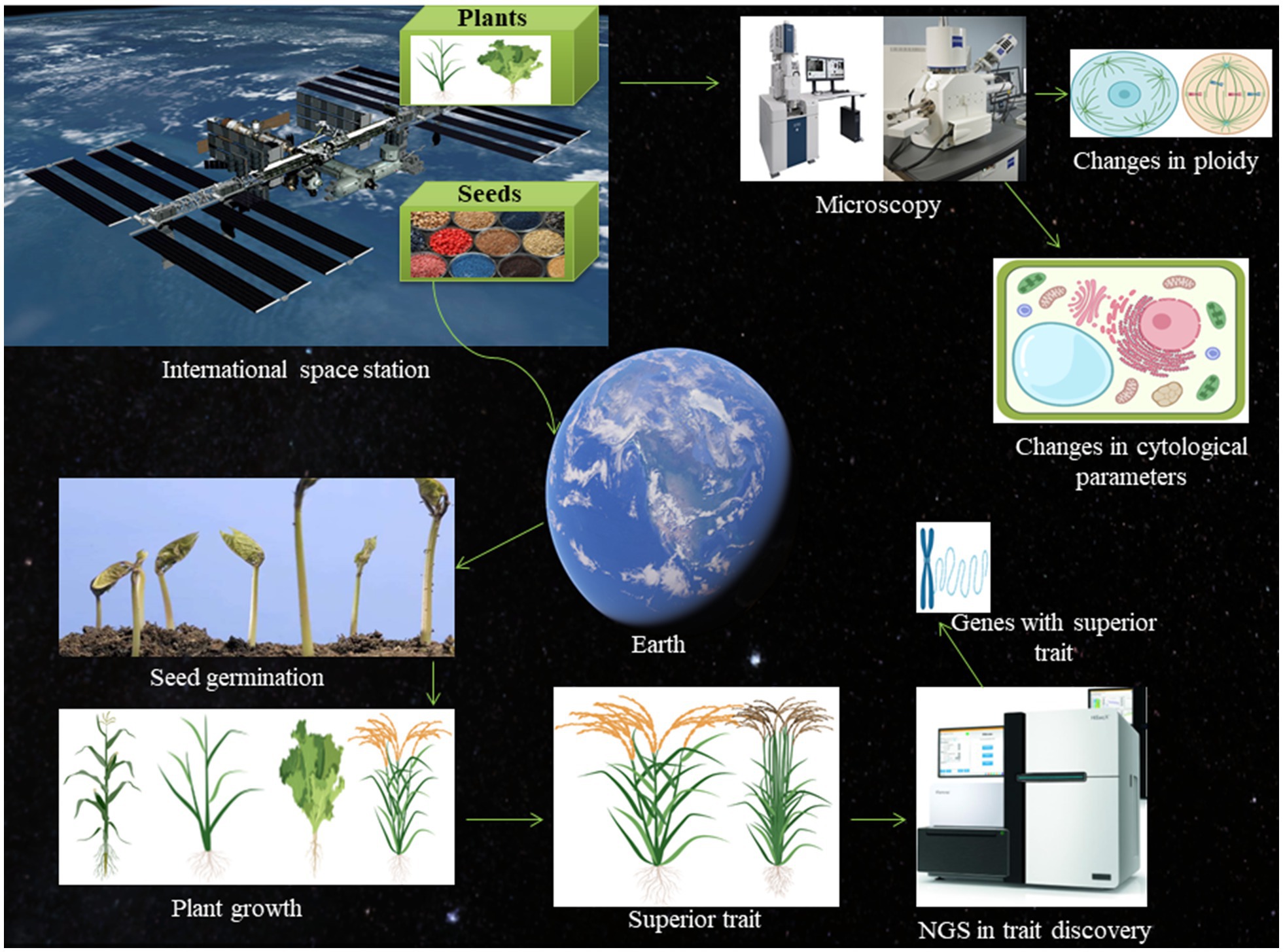
Figure 1. Schematic presentation of the space-breeding platform. The international space station is well-known for its role in space biology research. The plant seeds can be exposed to microgravity and cosmic radiation in space and brought back to the earth and sow for germination. The phenotypic and genotypic parameters can be measured to find the superior trait. The superior trait can be subjected to next-generation sequencing to find potential gene associated with the space environment. Once the gene will be identified, it can be used for molecular breeding to generate crops with the superior trait. The seeds can also be germinated in the space environment itself and grown plants can be brought back to the earth to check for chromosomal aberration, changes in cell shape and size, and alteration in the cell division.
Cell Size and Differentiation
The structure of a cell may change upon exposure to the external environment of space. For example, round cells were found in the cotyledon of pine, as well as in the root meristem of Crepis and maize (Il’ne and Parfenov, 1979). In microgravity, there is increased surface tension, which may result in rounded cells and reduced mechanical pressure on the attachment of cells (Il’ne and Parfenov, 1979). The moss Funaria hygrometrica grown in space for 96days contained more pear-shaped and dumbbell-shaped cells than the control moss grown on Earth. Furthermore, smaller cells, reduced volumes of starch granules, and altered chloroplast structures have been observed (Kordyum et al., 1981). Cells were found to be larger in the roots of Crepis, maize, pea, and wheat (Saunders, 1971; Merkys et al., 1975, 1976). An increase in cell elongation has been observed in wheat and maize seedlings (Merkys et al., 1976; Il’ne and Parfenov, 1979). On shuttle mission D-1, anise seeds were grown for somatic embryogenesis, and it was found that the somatic embryo cells differentiated more rapidly in space. Within 4days of growth in the space-grown culture, 90% of the cells displayed polarity and leaf or root primordia, whereas the control showed polarity after 6days. A larger differentiation zone was observed in carrot somatic embryos, resulting in longer roots (Il’ne and Parfenov, 1979). The space-grown plants received more sunlight than the ground-grown plants, which might explain why they generated chlorophyll earlier than ground-grown plants (Theimer et al., 1986). As the cells did not undergo normal elongation, they underwent early differentiation, leading to the formation of early root hairs near the tip of the roots (Merkys et al., 1983). Oat seedlings grown in the SL-2 spacecraft showed an increased number of lateral roots, which might be due to a reduced rate of cell division in the root tips (Halstead and Dutcher, 1987). The internode of pea seedlings grown in space was found to be extremely elongated compared to that of the control. The growth and development of orchid plants in space have been reported to be retarded. After the initiation of the space journey, the flowers suffered severe damage, cessation of growth, and death (Halstead and Dutcher, 1987). Despite the roots, stems, and leaves of orchid plants continuing to grow and develop, their flowers did not appear even after 6months (Gorkin et al., 1980). Arabidopsis plants grew very slowly, taking 28days to achieve the rosette stage and 44days to initiate flowering, while in the control plant they took 13 and 36days, respectively (Merkys and Laurinavichius, 1983).
Cell Division
For many years, induced mutations have been one of the most successful breeding strategies for plant breeders. Currently, there are a large number of mutant crop varieties worldwide, and their numbers are continuing to rise. Nevertheless, the space breeding approach of mutation through cosmic radiation is a recent development (Anqi, 2021). The space environment has microgravity, a hyper-vacuum, a weak geomagnetic field, and absence of microbes, which makes it an ideal environment for breeding plants (Paul et al., 2013; Kiss, 2014; Kordyum, 2014). Seed germination and plant growth have been found to be affected by the space environment in previous studies (Dutcher et al., 1994; Liu and Zheng, 1997). Compared to the control on Earth, mitotic cell division decreased with an increasing frequency of chromosomal aberrations. This spectrum of mutagenic effects has been reported to be universal among plants because of the mutagenic effects of space conditions (Liu et al., 2009). A reduction in cell division has been reported in plants grown in Biosatellite II (Thimann, 1968; Dubinin et al., 1977). Seedlings of Pisum sativum and Crepis capillaris grown during Soviet flights exhibit reduced cell division (Merkys et al., 1976; Tairbekov et al., 1979; Halstead and Dutcher, 1987). Oat seedlings grown on shuttle missions SL-2 and STS-3, and Helianthus annuus plants grown on STS-2 and STS-3 had 10% fewer dividing cells than the ground-control plants (Merkys et al., 1983; Sytnik et al., 1983). Reduced mitotic cell division of sunflowers, mung beans, and oats was found at the anaphase and telophase. A longer duration of space flight might have led to a greater reduction in cell division, in which the maximum number of cells was arrested at the metaphase of the cell cycle (Halstead and Dutcher, 1987). However, in lettuce, when cell division was reduced most cells were at the telophase (Merkys et al., 1983). The presence of multiple nuclei in Crepis and Pisum seedlings has been reported for flights conducted by the Soviet Union, while a sample of sunflowers grown on STS-2 revealed aneuploidy (2n−1=33) in root cells (Krikorian and O’Connor, 1984). Mitotic disturbances, including abnormal migration of the nucleus, were also found in the microspores of Tradescantia paludosa (Delone et al., 1964, 1966). Similarly, chromosomes were bunched at metaphase, as opposed to being located in the equatorial plate. A change in the spindle axis direction, unseparated chromatids, 3- and 4-pole mitoses, and non-disjunction of chromosomes were observed (Delone et al., 1965). It is possible that such incorrectly separated chromatids can produce the aneuploid genome that was observed in sunflowers. Fused embryo sacs have been identified in Tradescantia plants with clumped and scattered nuclei (Saunders, 1971). This could be caused by a malfunction in spindle organisation. Additionally, the root tips showed abnormal spindle organisation, multinucleated cells, and abnormal nuclei (Saunders, 1971).
Subcellular Changes
Weightlessness and microgravity play crucial roles in the subcellular organelles that make up a cell (Morrison, 1994; Bradbury et al., 2020). The cell walls of higher plants grown in a weightless condition were approximately one-third as thin as those of control plants (Dubinin, 1984; Laurinavichius et al., 1984). Plants grown in space for 24days had 54% lower cellulose content in their cell walls, while mung bean seedlings had 18% lower lignin content (Halstead and Dutcher, 1987). A reduction in protein and enzyme levels was also observed in space-grown seedlings (Cowles et al., 1984). We have already mentioned a reduction in mitosis cell division and chromosomal aberration in space-bound plants. The roots of Arabidopsis and pea plants grown in space had abnormal nuclei distribution and condensed chromatin in the statenchyma cells (Kordyum and Sytnik, 1983). The number of nucleoli was reduced in space-grown pine cells, whereas a significant increase in nucleus volume was observed in wheat seedlings. However, no changes were observed in the root caps of maize (Moore et al., 1987a). The location of the nucleus remained unaffected in cress, lentil, and lettuce, suggesting genetic programming of the nuclear organisation in the cell (Merkys et al., 1983; Perbal et al., 1986; Volkmann et al., 1986). Space-grown cells exhibited random distributions of amyloplasts in the root cap, whereas the 7-day-old seedlings showed a clearer stroma in the amyloplast and decreased starch grains and plastid reticulum. Most of the plastids post-18days contained a centrally located single starch granule, while others contained few or no starch grains (Sytnik et al., 1984). Maize seedlings at 4.8days had less starch in their columella cells than control plants. In a leaf sample of space-grown pea plants at 29days, disintegration of grana, separation of intergrana, shrinkage of the stack of grana, and formation of electron transport vesicles were observed (Halstead and Dutcher, 1987).
The electron density of the endoplasmic reticulum (ER) cisternae was reduced in the columella cells of space-grown Arabidopsis, cucumber, and pea seedlings (Halstead and Dutcher, 1987). In other cases, rounding and reduction of cisternae have been reported, whereas the disappearance of rough ER and ribosomes has been reported in Funaria protonema (Sytnik et al., 1984). While the maize seedlings germinated on Earth and grown in space had normal ER distribution in columella cells, those grown in space had spherical and ellipsoidal ER clustered at their periphery (Moore et al., 1987b). This reflects the effect of gravity on the distribution and integrity of the ER. The root caps of Arabidopsis, maize, pea, and seedlings with less-ordered membranes showed swollen mitochondria with electron-dense matrices (Il’ne and Parfenov, 1979; Kordyum and Sytnik, 1983). In the pea root meristem, dictyosomal cisternae underwent morphological changes as a result of weightlessness or microgravity, and there was a 50–90% reduction in dictyosome volume in the calyptrogen, columella, and peripheral cells of the root cap compared to the control (Moore et al., 1987a). In addition, mucilage secretion was considerably reduced in pea and maize seedlings (Tairbekov et al., 1986; Moore et al., 1987a).
Genetics and Polymorphism
Plant cultivated in space, the space environment can undergo changes during their development. In one experiment, Arabidopsis plants were carried into space at the cotyledon stage, and these plants succeeded in growth and flowering. However, the androecium and gynoecium of these plants were degenerated and sterile. Although the gross morphology of the reproductive organs was not affected, their sterility was caused by unsuitable illumination (Kordyum and Chernyaeva, 1982; Kordyum et al., 1983; Dubinin, 1984; Kostina et al., 1984). Afterwards, the plants were planted and sent back to Earth, where they completed flowering; however, their fertility was reduced, the frequency of recessive mutants increased, and germination success was reduced (Kostina et al., 1984, 1986). Eventually, the seeds from these Arabidopsis plants were grown in space to complete their life cycle. Nonetheless, the plants appeared shorter than normal, produced fewer leaves, and had smaller and fewer siliques (Halstead and Dutcher, 1987). When the F1 seeds obtained from the flight were planted in the ground, they showed reduced germination potential, with smaller hypocotyl and cotyledonary leaves. However, 42% of the seeds derived from the flight were biologically completed and produced fertile plants. Compared to the control plants, the F2 plants grown on Earth did not show any significant changes in developmental morphology (Parfenov and Abramova, 1981; Halstead and Dutcher, 1987).
The space environment induces mutations in crop genomes. A random amplified polymorphic DNA (RAPD) study of 201 rice germplasms developed from space-flown seeds revealed genomic polymorphisms. An estimated 30.2% genetic polymorphism was recorded from space-environment-induced mutations in rice, when compared to the ground control (Luo et al., 2007). However, the growth and fertility of all plants returned from the spaceships and the ground control plants were functionally normal (Luo et al., 2007). Studying 24 random primers led to 189 loci being detected, of which 4–15 loci were detected per primer. Fragments of the amplicon were between 400 and 2,000 base pairs (Luo et al., 2007). When rice seeds I-B11 DNA were amplified with OPS-19 as a primer, the sequencing report showed the presence of at least 12 single nucleotide polymorphisms (SNPs) in comparison with control samples (Luo et al., 2007). SNPs were also observed in OPN-9-B16 and OPB-13-A4 varieties (Luo et al., 2007). For successive generations, genetic polymorphisms were also examined for rice seeds I-B11 and I-B15. In total, 121 polymorphic loci were identified by polymorphism analysis using 29 random primers (Luo et al., 2007). Analysis revealed that polymorphism from individual plants and their progenies varies greatly compared to ground-based control (Luo et al., 2007). For I-B11, nine polymorphic loci were found in the first, whereas nine polymorphic loci were detected in third, fourth, and fifth generation (Luo et al., 2007). The field study revealed that plants with space-bound seeds grew shorter than their wild-type counterparts, QHZ. However, the progenies of I-B11 from the second generation headed 8–10days earlier than the control QHZ.
Oryza sativa lines R998, Gui99, and Hanghui No. 7 (restorer lines) were subjected to space mutation, and 104 pairs of SSR primers were used to check the polymorphisms; this revealed the presence of at least 2,538 polymorphic loci (Wu et al., 2011). The frequency of polymorphisms was 9.85%, which was caused by small fragments of DNA deletion or insertion mutations (Wu et al., 2011). The restoring and combining abilities of the mutants were evaluated through crossing with diverse sterile lines. The results showed that the space mutagenic effect has the potential to cause variations in the restoring ability of restorer lines in perpetuity (Wu et al., 2011). The pollen sterility in F1 hybrid combinations showed considerable variation compared to the control and some restorer lines mutated into the maintainer lines (Wu et al., 2011). The GCA values of Hanghui No. 7-4, Gui 99-4, Gui 99-2, R998-3, and R998-4 were higher. Genetic polymorphisms in maize male sterile mutants were also analysed following space exposure (Zhang et al., 2012). Analysis of amplified fragment length polymorphism using 56 primer pairs led to amplification of 32 primer combinations. Sequencing of the eluted fragments led to identification similarity with the expressed sequence tag gi33771201 of the maize sperm library (Zhang et al., 2012). The DNA sequence AC187265 was found to be located on the third chromosome (Zhang et al., 2012).
In space-grown lucerne seedlings, peroxidase activity was increased by 18% compared to the ground control (Li et al., 2004). This might be due to the activation and accumulation of oxygen species induced by space flight factors. The gel electrophoresis band patterns of the peroxidase were also changed in the space-grown seedlings, resulting in a few new bands (Li et al., 2004).
Chromosomal Aberrations
The radiation encountered in space leads to various types of DNA damage in cells: single nucleotide damage, single-stranded breaks, and double-stranded breaks (Furukawa et al., 2020). Double-stranded DNA breakage is one of the most common and severe forms of DNA damage. This leads to the activation of several DNA damage repair pathways about genomic stability. If a double-stranded break is not repaired properly, it may lead to cellular senescence and cell death (Furukawa et al., 2020). Usually, space-mediated DNA damage is not repaired properly because of the heavy energy radiation of α-rays and others, leading to failure in the mechanism of non-homologous end joining for DNA damage repair (Mohanta et al., 2017a). In such a situation, the homologous recombination mechanism is activated, leading to damage repair. As previously discussed, space-grown plants often develop chromosomal aberrations, which can be stable or unstable. The unstable chromosomal aberrations are categorised as ring, rearranged acentric, and multicentric chromosomes (Furukawa et al., 2020) and always remain unrepaired. Unstable chromosomal aberrations are usually lost during cell division due to their impaired DNA replication fork, broken termini without telomeres, and loss of chromosomal segregation mechanisms. The dicentric chromosome, which is the most common type of unstable chromosomal aberration, can be easily identified because of the presence of two centromeres (MacKinnon and Campbell, 2011; Stimpson et al., 2012; Furukawa et al., 2020). Stable chromosomal aberrations contain monocentric chromosomes that can be transmitted to daughter cells and hence can be used as a biomarker of past exposure to cosmic radiation.
Chromosomal aberrations have been found in most cases in seeds that have been taken into space and subsequently germinated (Delone and Antipv, 1967; Delone et al., 1971). Root meristematic cells were more frequently found to exhibit chromosomal aberrations. In C. capillaris, the amount of chromosomal aberration was increased, and in Arabidopsis thaliana, the viability and germination of seeds were significantly decreased. When grown in space, Arabidopsis plants showed abnormal growth in their reproductive systems and seeds; the germination potential, survival, and fertility were also reduced (Halstead and Dutcher, 1987). All of these events were attributed to chromosomal aberrations in meristematic stem cells. As metabolising cells, these are more prone to cosmic radiation exposure in space flight than dormant seeds. After exposure to space radiation for 3–234days and germination in space, Crepis seeds showed more aberrations than their ground-germinated counterparts (Kostina et al., 1984). Seedlings of Pinus sylvestris, however, did not show such an increase in chromosomal aberrations (Il’in and Parfenov, 1979; Tairbekov et al., 1979). The main reason for chromosomal aberrations, however, is extremely unlikely to occur in a short flight where cosmic radiation is quite low (Aliyev et al., 1985). Radioprotectors, such as 5-methoxytryptamine, aminoethylisothiourea, and cysteine, have not been able to reduce space-induced chromosomal aberrations in barley (Nuzhdin and Dozotseva, 1972; Nuzdhin et al., 1975). An 827-day exposure resulted in a significant increase in chromosomal aberrations in Crepis seeds, although there was virtually no effect on Arabidopsis seed germination potential (Anikeva et al., 1983; Halstead and Dutcher, 1987).
Elemental Composition
The exposure of plants to space has resulted in several physiological, cellular, genomic, and molecular changes. As a result, the micro-and macro-elemental composition of the cell may change. For example, pea plants grown on the Salyut space station showed problems with mineral balance, resulting in an increase in phosphorus and potassium levels in the shoots, but a sharp decrease in calcium, iron, magnesium, manganese, and zinc levels (Dubinin, 1984). It is most likely that a reduction in elemental composition is related to a reduction in root growth. In calcium signalling events, calcium ions act as a second messenger, and its reduction in the cell explains the lower metabolic, cellular, and physiological events in the cell (Mohanta et al., 2012, 2015, 2017b, 2019; Mohanta and Sinha, 2016). The presence of manganese during photosynthesis contributes to the hydrolysis reaction, and reduced manganese in cells is directly related to a reduction in photosynthetic activity. Furthermore, in an analysis of 5-day-old pea seedlings, ATPase activity was inhibited by reduced Ca2+, Mg2+, and K+ levels, which suggests that this is a further effect of microgravity. The inhibition of ATPases leads to reduced protein content in plasmodesmata. It is most likely that the decreased ATPase activity was due to a considerable reduction in Ca2+-dependent ATPases. In the control group, Ca2+ was found in the plasmodesmata and plasmalemma, whereas in the treated group, Ca2+ disappeared from these organelles but was found in the plastid, ER, dictyosome, and nuclear membrane (Palladina et al., 1984). From these reports, it can be speculated that free cellular calcium is more likely to be membrane-bound under hypogravity. Changes in Ca2+ levels also trigger the activation of membrane phospholipases (Palladina et al., 1984). Consequently, active and passive Ca2+ transport is disrupted because of reduced Ca2+ ATPase activity.
Development of Mutant Varieties Through Space Breeding
Plants grown under zero gravity and cosmic radiation undergo physical, physiological, and genetic changes (Krikorian and Steward, 1978; Böhmer and Schleiff, 2019), and it is possible that cosmic radiation may be used as a method of genetic modification. Space crop projects have garnered enormous attention worldwide for a long time and have succeeded in revealing a considerable amount of biological information (Ferl et al., 2002; Wolverton and Kiss, 2009). It has been reported that China has been quite active in such a mission since 1987, and as of now, has developed approximately 200 plant varieties caused by space radiation (Zhihao, 2020). High-altitude balloons are also used by China to conduct breeding experiments in space. The launch of Shiijian-8, the first space breeding satellite, took place on 9 September 2006 (Chen, 2019). It contained approximately 2,000 plant accessions from 133 plant species. Seed germination of cotton, maize, sunflower, cucumber, tomato, wheat, barley, and soybean noticeably increased after space flight, but rice, millet, pea, sweet pepper, tobacco, and lettuce failed to show any differences (Liu et al., 2009). The seed germination potential of eggplant, radish, watermelon, and sorghum was reduced, whereas that of wheat, barley, and triticale was significantly higher than that of ground control and gamma-irradiated seeds (Liu et al., 2009). The activities of both esterase and peroxidase enzymes in these species were also increased during flight (Liu et al., 2009).
At least 66 mutant crop varieties, including lucerne, cotton, pepper, sesame, rapeseed, rice, tomato, and wheat, have been released in China through space breeding programmes (Liu et al., 2009). Although developing plant varieties that thrive in microgravity and resist cosmic radiation may be an important goal for the scientific community, an undesirable mutation in the genome could have deleterious effects on other crop varieties. It is necessary to consider the possibility of cross-contamination of deleterious traits between varieties. Therefore, the conduct of such research should be subject to strict international regulations to avoid the possibility of unexpected results.
Conclusion and Future Perspectives
Despite advances in conventional and molecular breeding in agricultural research, genetically modified crops are not widely accepted. Therefore, it remains uncertain how society will react to crops that have been genetically modified in space, specifically, the “space rice” released by China. Genome sequencing and transcriptome sequencing should be performed to better understand the genomic variations caused by cosmic radiation. It is important to perform further investigations to understand how the proteome and metabolome contents will be affected. In addition, SNPs and genome-wide association studies can aid in identifying potential genetic markers associated with space breeding programmes. Once a particular gene/locus is identified, its role can be expanded to other crop varieties. This information can be used in simulation models for further application in other crop varieties using mutations and chromosomal aberrations that occur in different crop species. Statistical software such as EnvRtype can be useful for analysing large-scale environmental data for quantitative genomics and environment-based ecophysiological interactions (Costa-Neto et al., 2021). Interconnecting genomic, high-throughput phenotyping, and environmental parameters (Crossa et al., 2021) can be very useful in addressing space breeding strategies. Modelling studies can be performed using genomic and space environment data for selection (Jarquín et al., 2014; Marcatti et al., 2017) and to identify better traits for crop production. The single-step genomic and pedigree×environment interaction model can extend the genomic relationship information on individual genotypes to pedigree information from multiple phenotyped species (Pérez-Rodríguez et al., 2017). The genomic relationship of individual species can be combined to obtain the desired traits for breeding programmes.
Author Contributions
TM conceived the idea, surveyed literature, and drafted and revised the manuscript. AM, YM, and AA-H revised the manuscript. All authors contributed to the article and approved the submitted version.
Conflict of Interest
The authors declare that the research was conducted in the absence of any commercial or financial relationships that could be construed as a potential conflict of interest.
Publisher’s Note
All claims expressed in this article are solely those of the authors and do not necessarily represent those of their affiliated organizations, or those of the publisher, the editors and the reviewers. Any product that may be evaluated in this article, or claim that may be made by its manufacturer, is not guaranteed or endorsed by the publisher.
Acknowledgments
The authors would like to extend their sincere thanks to the Natural and Medical Sciences Research Center, University of Nizwa, Oman for their support to conduct the work.
References
Acuña, M. H., Connerney, J. E. P., Wasilewski, P., Lin, R. P., Mitchell, D., Anderson, K. A., et al. (2001). Magnetic field of Mars: summary of results from the aerobraking and mapping orbits. J. Geophys. Res. Planets 106, 23403–23417. doi: 10.1029/2000JE001404
Aliyev, A., Abilov, Z., Manshinskiy, A., Gainiyeva, R., and Ragimova, G. (1985). Ultrastructural and some physiological features of photosynthetic apparatus of garden pea cultivated for 29 days in “Salyut-7” space station. Lzv. Akad. Nauk Az. SSR Ser. Bioi. Nauk 6, 18–23.
Anikeva, I., Kostina, L., and Vaulina, E. (1983). Experiments with airdried seeds of Arabidopsis thaliana (L.) Heynh. And Crepis capillaris (L.) Walll., aboard Salyut 6. Adv. Space Res. 3, 129–133. doi: 10.1016/0273-1177(83)90182-5
Anqi, F. (2021). China’s first “space rice” that made round trip to moon yields grain. Global Times. Available at: https://www.globaltimes.cn/page/202107/1228365.shtml#:~:text=China’s%20first%20batch%20of%20rice,to%20the%20moon%2C%20Mars%20and (Accessed July 11, 2021).
Bagshaw, M. (2008). Cosmic radiation in commercial aviation. Travel Med. Infect. Dis. 6, 125–127. doi: 10.1016/j.tmaid.2007.10.003
Böhmer, M., and Schleiff, E. (2019). Microgravity research in plants. EMBO Rep. 20:e48541. doi: 10.15252/embr.201948541
Bradbury, P., Wu, H., Choi, J. U., Rowan, A. E., Zhang, H., Poole, K., et al. (2020). Modeling the impact of microgravity at the cellular level: implications for human disease. Front. Cell Dev. Biol. 8:96. doi: 10.3389/fcell.2020.00096
Chen, S. (2019). Countdown starts for China’s big mutant crop space mission in race for food security. South China Morning Post, Available at: https://www.scmp.com/news/china/science/article/3035967/countdown-starts-chinas-big-mutant-crop-space-mission-race-food (Accessed November 7, 2019).
Connerney, J. E. P., Acuña, M. H., Wasilewski, P. J., Kletetschka, G., Ness, N. F., Rème, H., et al. (2001). The global magnetic field of Mars and implications for crustal evolution. Geophys. Res. Lett. 28, 4015–4018. doi: 10.1029/2001GL013619
Costa-Neto, G., Galli, G., Carvalho, H. F., Crossa, J., and Fritsche-Neto, R. (2021). EnvRtype: a software to interplay enviromics and quantitative genomics in agriculture. G3 11:jkab040. doi: 10.1093/g3journal/jkab040
Cowles, J. R., Scheld, H. W., Lemay, R., and Peterson, C. (1984). Growth and lignification in seedlings exposed to eight days of microgravity. Ann. Bot. 54, 33–48. doi: 10.1093/oxfordjournals.aob.a086865
Crossa, J., Fritsche-Neto, R., Montesinos-Lopez, O. A., Costa-Neto, G., Dreisigacker, S., Montesinos-Lopez, A., et al. (2021). The modern plant breeding triangle: optimizing the use of genomics, phenomics, and enviromics data. Front. Plant Sci. 12:651480. doi: 10.3389/fpls.2021.651480
Delone, N., and Antipv, V. (1967). Further investigation of the effect of space flight conditions on the chromosomes of radicles in the seeds of some higher plants. Cosm. Res. 5:274.
Delone, N., Bykovskii, V., Antipov, V., Parfenov, G., and Vysotskii, V. (1964). Effect of space-flight factors on Tradescantia paludosa microspores on board the satellite spaceships Vostok 5 and 6. Cosm. Res. 2, 268–727.
Delone, N., Egorov, B., and Antipov, V. (1966). Effect of the factors of cosmic flight of the satellite ship Voskhod on the microspore Tradescantia paludosa. Cosm. Res. 4, 139–143.
Delone, N., Morozova, E., and Antipov, V. (1971). Effect of conditions of space flight on station “Zond-5” on seeds, onions, and Tradescantia. Cosm. Res. 9, 146–148.
Delone, N., Rudneva, N., and Antipov, V. (1965). Effect of space-flight conditions in satellite spaceships “V ostok-5” and “Vostok-6” on chromosomes of radicies in seeds of some higher plants. Cosm. Res. 3, 371–378.
Dubinin, N. (1984). Biologicheskiye Issledovaniya na Orbital’nykh Stantsiyakh “Salyut”. Moscow: Nauka, 248.
Dubinin, N. P., Glembotsky, Y. L., Vaulina, E. N., Merkis, A. I., Laurinavichius, R. S., Palmbakh, L. R., et al. (1977). Biological experiments on the orbital station Salyut 4. Life Sci. Space Res. 15, 267–272.
Dutcher, F. R., Hess, E. L., and Halstead, T. W. (1994). Progress in plant research in space. Adv. Space Res. 14, 159–171. doi: 10.1016/0273-1177(94)90400-6
Eichinger, R., Garny, H., Šácha, P., Danker, J., Dietmüller, S., and Oberländer-Hayn, S. (2020). Effects of missing gravity waves on stratospheric dynamics; part 1: climatology. Clim. Dyn. 54, 3165–3183. doi: 10.1007/s00382-020-05166-w
Ferl, R., Wheeler, R., Levine, H. G., and Paul, A.-L. (2002). Plants in space. Curr. Opin. Plant Biol. 5, 258–263. doi: 10.1016/S1369-5266(02)00254-6
Frisbee, R. H. (2003). Advanced space propulsion for the 21st century. J. Propuls. Power 19, 1129–1154. doi: 10.2514/2.6948
Furukawa, S., Nagamatsu, A., Nenoi, M., Fujimori, A., Kakinuma, S., Katsube, T., et al. (2020). Space radiation biology for “living in space.” Biomed. Res. Int. 2020:4703286. doi: 10.1155/2020/4703286
Ghosh, P. (2021). What is Space rice? China harvests 1st batch of seeds that travelled around moon. Hindustan Times. Available at: https://www.hindustantimes.com/india-news/what-is-space-rice-china-harvests-1st-batch-of-seeds-that-travelled-around-moon-101626229101405.html (Accessed July 14, 2021).
Gorkin, Y., Mashinskiy, A., and Yazdovskiy, V. (1980). Birthday flowers: “Salyut-6”, our commentary. Pravda, p.3.
Halstead, T. W., and Dutcher, F. R. (1987). Plants in space. Annu. Rev. Plant Physiol. 38, 317–345. doi: 10.1146/annurev.pp.38.060187.001533
Il’in, Y., and Parfenov, G. (1979). Biologicheskiye Issledovaniya na Biosputnikakh “Kosmo”. Moscow: Nauka, 239.
Il’ne, Y., and Parfenov, G. (1979). Biologicheskiye Issledovaniya na Biosputnikakh “Kosmos.” Moscow: Nauka, 239.
Jarquín, D., Crossa, J., Lacaze, X., Du Cheyron, P., Daucourt, J., Lorgeou, J., et al. (2014). A reaction norm model for genomic selection using high-dimensional genomic and environmental data. Theor. Appl. Genet. 127, 595–607. doi: 10.1007/s00122-013-2243-1
Jules, K., McPherson, K., Hrovat, K., Kelly, E., and Reckart, T. (2004). A status report on the characterization of the microgravity environment of the international Space Station. Acta Astronaut. 55, 335–364. doi: 10.1016/j.actaastro.2004.05.057
Kiss, J. Z. (2014). Plant biology in reduced gravity on the moon and Mars. Plant Biol. 16, 12–17. doi: 10.1111/plb.12031
Kordyum, E. L. (2014). Plant cell gravisensitivity and adaptation to microgravity. Plant Biol. 16, 79–90. doi: 10.1111/plb.12047
Kordyum, E., and Chernyaeva, I. (1982). Peculiarities in formation of Arabidopsis thaliana (L.) Heynh. Generative organs under space flight cond. Dopov. Akad. Nauk Ukr. RSR, Ser B 8, 67–70.
Kordyum, E. L., Nedukha, E. M., Stynik, K. M., and Mashinsky, A. L. (1981). Optical and electron-microscopic studies of the Funaria hygrometrica protonema after cultivation for 96 days in space. Adv. Space Res. 1, 159–162. doi: 10.1016/0273-1177(81)90257-X
Kordyum, E., and Sytnik, K. (1983). Biological effects of weightlessness at cellular and subcellular levels. Physiologist 26, S141–S142.
Kordyum, E. L., Sytnik, K. M., and Chernyaeva, I. I. (1983). Peculiarities of genital organ formation in Arabidopsis thaliana (L) Heynh. Under spaceflight conditions. Adv. Space Res. 3, 247–250. doi: 10.1016/0273-1177(83)90064-9
Kostina, L., Anikeeva, I., and Vaulina, E. (1984). The influence of space flight factors on viability and mutability of plants. Adv. Space Res. 4, 65–70. doi: 10.1016/0273-1177(84)90225-4
Kostina, L., Anikeeva, I., and Vaulina, E. (1986). Experiments with developing plants aboard Salyut-5, Salyut6 and Salyut-7 orbital stations. Space Bioi. Aerosp. Med. 20, 73–78.
Krikorian, A., and O’Connor, S. (1984). Experiments on plants grown in space: Karyological observation. Ann. Bot. 54, 49–63. doi: 10.1093/oxfordjournals.aob.a086866
Krikorian, A. D., and Steward, F. C. (1978). Morphogenetic responses of cultured totipotent cells of carrot daucus carota var. carota at zero gravity. Science 200, 67–68. doi: 10.1126/science.200.4337.67
Laurinavichius, R., AV, Y., Marchyukaytis, A., Shvegzhdene, D. V., and Mashinskiy, A. (1984). Metabolism of Pea Plants Grown under Space Flight Conditions. Moscow: Nauka, 96–102.
Li, J., Ren, W.-B., Guo, H.-Q., Liu, Y.-X., Cheng, L.-B., W-J, Z., et al. (2004). Effect of space flight factors on the peroxidase polymorphism and its activity of alfalfa. Seed 04.
Liu, L., Guo, H., Zhao, L., Wang, J., Gu, Y., and Zhao, S. (2009). “Achievements and perspective of crop space breeding in China,” in Induced Plant Mutation in the Genomics Era. ed. Q. Y. Shu (Food and Agriculture Organization of the United Nations, Rome), 213–215.
Liu, L., and Zheng, Q. (1997). Report no: CNIC01139/CSNAS-0111. Space-induced mutation for crop improvement. China Nuclear Science and Technology Report.
Luo, Y., Wang, X., Mei, M., Zhuang, C., Feng, Z., Zengquan, W., et al. (2007). Genomic polymorphism in consecutive generation rice plants from seeds on board a spaceship and their relationship with space HZE particles. Front. Biol. China 2, 297–302. doi: 10.1007/s11515-007-0043-1
MacKinnon, R. N., and Campbell, L. J. (2011). The role of dicentric chromosome formation and secondary centromere deletion in the evolution of myeloid malignancy. Genet. Res. Int. 2011:643628. doi: 10.4061/2011/643628
Marcatti, G. E., Resende, R. T., Resende, M. D. V., Ribeiro, C. A. A. S., dos Santos, A. R., da Cruz, J. P., et al. (2017). GIS-based approach applied to optimizing recommendations of eucalyptus genotypes. For. Ecol. Manag. 392, 144–153. doi: 10.1016/j.foreco.2017.03.006
McKay, C. P. (2010). An origin of life on Mars. Cold Spring Harb. Perspect. Biol. 2:a003509. doi: 10.1101/cshperspect.a003509
Merkys, A., and Laurinavichius, R. (1983). Complete cycle of individual development of Arabidopsis thaliana (L.) Heynh. Plants on board the “Salyut-T” orbital station. Dokl. Akad. Nauk SSSR 271, 509–512.
Merkys, A., Laurinavichius, R., Mashinskiy, A., Yaroshius, A., and Savichene, E. (1976). “Effect of weightlessness and its simulation on the growth and morphology of cells and tissues of pea and lettuce seedlings.” in Organizmy i Sila Tyazhesti. Materialy 1 Vsesoyuznoy Konferentsii “Gravitatsiya i Organism.” ed. A. J. Merkys, 238–246.
Merkys, A. J., Laurinavichius, R. S., Rupainene, O. J., Savichene, E. K., Jaroshius, A. V., Shvegzhdene, D. V., et al. (1983). The state of gravity sensors and peculiarities of plant growth during different gravitational loads. Adv. Space Res. 3, 211–219. doi: 10.1016/0273-1177(83)90059-5
Merkys, A. J., Mashinsky, A. L., Laurinavichius, R. S., Nechitailo, G. S., Yaroshius, A. V., and Izupak, E. A. (1975). The development of seedling shoots under space flight conditions. Life Sci. Space Res. 13, 53–57.
Mohanta, T. K., Bashir, T., Hashem, A., Abd Allah, E. F., and Bae, H. (2017a). Genome editing tools in plants. Gene 8:399. doi: 10.3390/genes8120399
Mohanta, T., Kumar, P., and Bae, H. (2017b). Genomics and evolutionary aspect of calcium signaling event in calmodulin and calmodulin-like proteins in plants. BMC Plant Biol. 17:38. doi: 10.1186/s12870-017-0989-3
Mohanta, T. K., Mohanta, N., Mohanta, Y. K., and Bae, H. (2015). Genome-wide identification of calcium dependent protein kinase gene family in plant lineage shows presence of novel D-x-D and D-E-L motifs in EF-hand domain. Front. Plant Sci. 6:1146. doi: 10.3389/fpls.2015.01146
Mohanta, T. K., Occhipinti, A., Atsbaha Zebelo, S., Foti, M., Fliegmann, J., Bossi, S., et al. (2012). Ginkgo biloba responds to herbivory by activating early signaling and direct defenses. PLoS One 7:e32822. doi: 10.1371/journal.pone.0032822
Mohanta, T. K., and Sinha, A. K. (2016). “Role of calcium-dependent protein kinases during abiotic stress tolerance,” in Abiotic Stress Response in Plants. eds. N. Tuteja and S. S. Gill, 185–206.
Mohanta, T. K., Yadav, D., Khan, A. L., Hashem, A., Abd_allah, E. F., and Al-Harrasi, A. (2019). Molecular players of EF-hand containing calcium signaling event in plants. Int. J. Mol. Sci. 20:1476. doi: 10.3390/ijms20061476
Moore, R., Fondren, W. M., McClelen, C. E., and Wang, C. L. (1987a). Influence of microgravity on cellular differentiation in root caps of Zea mays. Am. J. Bot. 74, 1006–1012.
Moore, R., McClelen, C., Fondren, W., and Wang, C. (1987b). The influence of microgravity on root-cap regeneration and the structure of columella cells in Zea mays. Am. J. Bot. 74, 216–221.
Morrison, D. R. (1994). Cellular changes in microgravity and the design of space radiation experiments. Adv. Space Res. 14, 1005–1019. doi: 10.1016/0273-1177(94)90567-3
Nuzdhin, N., Dozotseva, R., Samokhvalova, N., Nechaev, I., and Petrova, L. (1975). Genetic damages of seeds caused by their two-stage stay in space. Zh. Obshch. Bioi. 36, 332–340.
Nuzhdin, N., and Dozotseva, R. (1972). Genetic changes induced by space flight factors in barley seeds on “Soyuz-5” and “Soyuz-9” craft. Zh. Obshch. Bioi. 33, 336–346.
Palladina, T., Kordyum, E., and Bilyavs’ka, N. (1984). Activity and localization of transport adenosine triphosphatases in cells of pea seedling root cells in hypogravity conditions. Ukr. Bot. Zh. 41, 54–57.
Parfenov, G., and Abramova, V. (1981). Flowering and maturing of Arabidopsis seeds in weightlessness: experiment on the biosatellite “Kosmos-1 129.”. Dokl. Akad. Nauk SSSR 256, 254–256.
Paul, A.-L., Wheeler, R. M., Levine, H. G., and Ferl, R. J. (2013). Fundamental plant biology enabled by the space shuttle. Am. J. Bot. 100, 226–234. doi: 10.3732/ajb.1200338
Penley, N. J., Schafer, C. P., and Bartoe, J.-D. F. (2002). The international space station as a microgravity research platform. Acta Astronaut. 50, 691–696. doi: 10.1016/S0094-5765(02)00003-6
Perbal, G., Driss-Ecole, D., Salle, G., and Raffin, J. (1986). Perception of gravity in the lentil root. Naturwissenschaften 73, 444–446. doi: 10.1007/BF00367293
Pérez-Rodríguez, P., Crossa, J., Rutkoski, J., Poland, J., Singh, R., Legarra, A., et al. (2017). Single-step genomic and pedigree genotype × environment interaction models for predicting wheat lines in international environments. Plant Genome 10. doi: 10.3835/plantgenome2016.09.0089
Rabbow, E., Horneck, G., Rettberg, P., Schott, J.-U., Panitz, C., L’Afflitto, A., et al. (2009). EXPOSE, an astrobiological exposure facility on the international space station—from proposal to flight. Orig. Life Evol. Biosph. 39, 581–598. doi: 10.1007/s11084-009-9173-6
Rabbow, E., Rettberg, P., Barczyk, S., Bohmeier, M., Parpart, A., Panitz, C., et al. (2012). EXPOSE-E: An ESA astrobiology Mission 1.5 years in space. Astrobiology 12, 374–386. doi: 10.1089/ast.2011.0760
Rabbow, E., Rettberg, P., Barczyk, S., Bohmeier, M., Parpart, A., Panitz, C., et al. (2015). The astrobiological mission EXPOSE-R on board of the international Space Station. Int. J. Astrobiol. 14, 3–16. doi: 10.1017/S1473550414000202
Resende, R. T., Piepho, H.-P., Rosa, G. J. M., Silva-Junior, O. B., E Silva, F. F., de Resende, M. D. V., et al. (2021). Enviromics in breeding: applications and perspectives on envirotypic-assisted selection. Theor. Appl. Genet. 134, 95–112. doi: 10.1007/s00122-020-03684-z
Simoneit, B. R., Summons, R. E., and Jahnke, L. L. (1998). Biomarkers as tracers for life on early earth and Mars. Orig. Life Evol. Biosph. 28, 475–483. doi: 10.1023/A:1006508012904
Stimpson, K. M., Matheny, J. E., and Sullivan, B. A. (2012). Dicentric chromosomes: unique models to study centromere function and inactivation. Chromosome Res. 20, 595–605. doi: 10.1007/s10577-012-9302-3
Sytnik, K. M., Kordyum, E. L., Belyavskaya, N. A., Nedukha, E. M., and Tarasenko, V. A. (1983). Biological effects of weightlessness and clinostatic conditions registered in cells of root meristem and cap of higher plants. Adv. Space Res. 3, 251–255. doi: 10.1016/0273-1177(83)90065-0
Sytnik, K., Kordyum, E., Nedukha, E., Sidorenko, P., and Fomicheva, V. (1984). Rastitel’naya Kletka pri lzmenenii Geofizicheskikh Faktorov. Kiev: Naukova Dumk, 135.
Tairbekov, M., Grif, V., Barmicheva, E., and Valovich, E. (1986). Cytomorphology and ultrastructure of the root of the maize meristem in weightlessness. lzv. Akad. Nauk SSSR, Ser. Biol. 5, 680–687.
Tairbekov, M., Parfenov, G., Platonova, R., and Zhvalikovskaya, V. (1979). Study of Plant Cells Using the BiofIksator-L. Instrument, 161–169.
Theimer, R. R., Kudielka, R. A., and Rosch, I. (1986). Induction of somatic embryogenesis in anise in microgravity. Naturwissenschaften 73, 442–443. doi: 10.1007/BF00367292
Thimann, K. V. (1968). Biosatellite II experiments: preliminary results. Proc. Natl. Acad. Sci. U. S. A. 60, 347–361. doi: 10.1073/pnas.60.2.347
Thirsk, R., Kuipers, A., Mukai, C., and Williams, D. (2009). The space-flight environment: the international Space Station and beyond. Can. Med. Assoc. J. 180, 1216–1220. doi: 10.1503/cmaj.081125
Volkmann, D., Behrens, H. M., and Sievers, A. (1986). Development and gravity sensing of cress roots under microgravity. Naturwissenschaften 73, 438–441. doi: 10.1007/BF00367291
Voss-Fels, K. P., Cooper, M., and Hayes, B. J. (2019). Accelerating crop genetic gains with genomic selection. Theor. Appl. Genet. 132, 669–686. doi: 10.1007/s00122-018-3270-8
Westall, F., Loizeau, D., Foucher, F., Bost, N., Betrand, M., Vago, J., et al. (2013). Habitability on Mars from a microbial point of view. Astrobiology 13, 887–897. doi: 10.1089/ast.2013.1000
Wolverton, C., and Kiss, J. Z. (2009). An update on plant space biology. Gravit. Space Biol. Bull. 22, 13–20.
Wu, D., Liu, Y., Guo, T., Zhang, J., Chen, Z., and Wang, H. (2011). Variation of restoring ability and analysis of genetic polymorphism in posterity of restorer lines by space mutagenesis. J. South China Agric. Univ. 32, 1–5.
Zhang, S. N., Adriani, O., Albergo, S., Ambrosi, G., An, Q., Bao, T. W., et al. (2014). “The high energy cosmic-radiation detection (HERD) facility onboard China’s Space Station.” in InSpace Telescopes and Instrumentation 2014: Ultraviolet to Gamma Ray, 91440X.
Zhang, L., Niu, X., Dai, Y., and Cao, M. (2012). Amplified fragment length polymorphism (AFLP) analysis of male sterile mutant induced by space flight in maize. J. Maize Sci. 20, 50–53.
Zhihao, Z. (2020). Crops bred in space produce heavenly results. CHINA DAILY. Available at: https://www.chinadaily.com.cn/a/202011/13/WS5faddbf8a31024ad0ba93d27_2.html (Accessed November 13, 2020).
Keywords: space, breeding, crop, microgravity, cosmic radiation
Citation: Mohanta TK, Mishra AK, Mohanta YK and Al-Harrasi A (2021) Space Breeding: The Next-Generation Crops. Front. Plant Sci. 12:771985. doi: 10.3389/fpls.2021.771985
Edited by:
Rafael Tassinari Resende, Universidade Federal de Goiás, BrazilReviewed by:
Ailton Crispim-Filho, Universidade Federal de Goiás, BrazilIasmine Zaidan, Universidade Federal do Espírito Santo, Brazil
Copyright © 2021 Mohanta, Mishra, Mohanta and Al-Harrasi. This is an open-access article distributed under the terms of the Creative Commons Attribution License (CC BY). The use, distribution or reproduction in other forums is permitted, provided the original author(s) and the copyright owner(s) are credited and that the original publication in this journal is cited, in accordance with accepted academic practice. No use, distribution or reproduction is permitted which does not comply with these terms.
*Correspondence: Tapan Kumar Mohanta, bm9zdG9jLnRhcGFuQGdtYWlsLmNvbQ==; dGFwYW4ubW9oYW50YUB1bml6d2EuZWR1Lm9t; Ahmed Al-Harrasi, YWhhcnJhc2lAdW5pendhLmVkdS5vbQ==
†These authors have contributed equally to this work