- 1State Key Laboratory of Tea Plant Biology and Utilization, Anhui Agricultural University, Hefei, China
- 2Biotechnology Center, Anhui Agricultural University, Hefei, China
- 3School of Biology, Food and Environment, Hefei University, Hefei, China
Theanine, a unique non-proteinogenic amino acid, is one of the most abundant secondary metabolites in tea. Its content largely determines green tea quality and price. However, its physiological roles in tea plants remain largely unknown. Here, we showed that salt stress significantly increased the accumulation of glutamate, glutamine, alanine, proline, and γ-aminobutyric acid, as well as theanine, in the new shoots of tea plants. We further found that salt stress induced the expression of theanine biosynthetic genes, including CsGOGATs, CsAlaDC, and CsTSI, suggested that salt stress induced theanine biosynthesis. Importantly, applying theanine to the new shoots significantly enhanced the salt stress tolerance. Similar effects were also found in a model plant Arabidopsis. Notably, exogenous theanine application increased the antioxidant activity of the shoots under salt stress, suggested by reduced the reactive oxygen species accumulation and lipid peroxidation, as well as by the increased SOD, CAT, and APX activities and expression of the corresponding genes. Finally, genetic evidence supported that catalase-mediated antioxidant scavenging pathway is required for theanine-induced salt stress tolerance. Taken together, this study suggested that salt stress induces theanine biosynthesize in tea plants to enhance the salt stress tolerance through a CAT-dependent redox homeostasis pathway.
Introduction
The tea plant (Camellia sinensis L.) is an important economic woody crop, and widely cultivated in the world (Chen et al., 2007). It contains numerous secondary metabolites, including theanine (γ-glutamylethylamide), flavonoids and caffeine. As a unique non-proteinogenic amino acid in tea plants, theanine is the component conferring the particular “umami” tastes, anti-depression and neuroprotection benefits of green tea infusion (Ashihara, 2015). Theanine is the most abundant free amino acid in tea plants, however, its physiological roles in tea plants remains unknown.
It is synthesized from glutamate and ethylamine via theanine synthatase (CsTS). In recent study, the TS gene (CsTSI) was identified in tea plants (Wei et al., 2018). CsTSI is highly homologous to glutamine synthetases (GSs) which also can catalyze theanine synthesis (Cheng et al., 2017; Wei et al., 2018). Glutamate Synthases (GOGATs) catalyze glutamate biosynthesis in tea plants, while ethylamine is synthesized by alanine decarboxylase (CsAlaDC; Takeo, 1979; Bai et al., 2019). Genes involved in theanine metabolism have been systemically identified along with the completion of the genome sequencing in tea plants (Xia et al., 2019). Theanine metabolism is regulated by many environment factors, especially high salinity, heat, drought, nutrient levels and light intensity (Deng et al., 2012; Wang et al., 2016; Li et al., 2018a; Yang et al., 2020, 2021). However, theanine, as a species-specific secondary metabolite, its roles in the adaption to these abiotic stresses for tea plants need to be explored.
Excessive irrigation and fertilization increase salt contents in soils, which imposes salt stress to crops, including tea plants (Causapé et al., 2004). Salt stress reduces the yield and quality of tea and greatly diminishes the widespread of tea plants (Upadhyaya and Panda, 2013). High soil salinity has become one of the major adverse environmental stresses in global agricultural production. Salt stress usually reduces efficiency of photosynthetic apparatus including PSII and the electron transport chain, and elicits the accumulation of reactive oxygen species (ROS), and ultimately leads to cell damage and oxidative stress (Wang et al., 2007; Considine and Foyer, 2021).
Plants have evolved a complex antioxidant defense system to balance the ROS levels in response to salt stress (Bose et al., 2013). Generally, the superoxide is converted into hydrogen peroxide (H2O2) through superoxide dismutase (SOD). Then, that H2O2 is converted into H2O and oxygen by ascorbate peroxidases (APXs), catalases (CATs), as well as other free radical scavengers (Bose et al., 2013). ROS imbalance is harmful to plants; however, H2O2 is also involved in the chloroplast-to-nucleus retrograde signaling which is critical for salt stress response in plants (Park and Seo, 2021; Zhuang et al., 2021).
Recent studies have reported that salt stress affects nitrogen (N) and amino acid metabolism in plants (Silveira et al., 2003; Dluzniewska et al., 2007). Ammonium is assimilated first into the glutamine by GSs, and then is assimilated into glutamate by GOGATs in plants (Groat and Vance, 1981; Miflin and Habash, 2002). Glutamate is further used to synthesize other amino acids like proline, γ-aminobutyric acid (GABA) and asparagine, etc. (Yang et al., 2020). Salt stress induces the accumulation of proline and GABA which act as compatible osmolyte or signaling molecule involved in mitigating high salinity stress (Szabados and Savouré, 2010; Hayat et al., 2012; Bao et al., 2015).
In animals, theanine is involved in various physiological functions, such as improvement of sleep quality, relaxation, neuroprotection, and Parkinson’s disease, etc. (Narukawa et al., 2008; Sharma et al., 2018; Deb et al., 2019). Impressively, theanine alleviates Cd-induced oxidative damage through reducing malondialdehyde (MDA) and ROS levels in the brain of mouse (Ben et al., 2016). It was reported that salt stress induces theanine biosynthesis in tea plants (Deng et al., 2012). In addition, exogenous supply of glutamate alleviated the salinity stress-induced inhibition of seed germination and radicle growth of buck wheat and cucumber (Chang et al., 2010; Yang, 2014). Given that theanine is a natural analog of glutamate, we hypothesize that theanine improves salt stress tolerance via modulating ROS homeostasis in tea plants.
In this study, we observed that salt stress induced theanine biosynthesis in the new shoots of tea plants, and exogenous theanine application enhanced the resistance to salt stress in the new shoots. We explored the underlying mechanism, with particular emphasis on the reestablishment of redox homeostasis by theanine. The study provided novel insights into the physiological roles of theanine in tea plants.
Materials and Methods
Plant Materials and Treatments
New shoots were collected from tea plants (Camellia sinensis var. sinensis cv. “Shuchazao”) grown in the tea plantation of Anhui Agricultural University in Hefei, China. For salt stress treatment, new shoots were exposed to nutrient solution containing 0, 100, 150, or 200 mM NaCl. To study the function of theanine, new shoots were exposed to nutrient solution containing 150 mM NaCl (NaCl), 10 mM theanine (Thea), 150 mM NaCl and 10 mM Thea (NaCl + Thea). Control samples (Con) were exposed to normal nutrient solution. The composition of the nutrient solution used was as follows: 0.54 mM (NH4)2SO4, 0.18 mM Ca(NO3)2, 0.1 mM, KH2PO4, 0.41 mM K2SO4, 0.39 mM CaCl2, 1.03 mM MgSO4, 6.27 μM C10H12FeN2NaO8, 9.25 μM H3BO3, 3.9 μM CuSO4, 18.2 μM MnSO4, 0.4 mM Al2(SO4)3⋅18H2O, 0.53 μM Na2MoO4, and 1.53 μM ZnSO4, and the pH of the nutrient solution was adjusted to 4.5–5.0 (Konishi et al., 1985).
Arabidopsis seeds of cat2cat3 and cat1cat2cat3 mutants were generous gifts from Professor Changle Ma in Shandong Normal University, China. Arabidopsis seeds were surface-sterilized by sodium hypochlorite and rinsed four times with sterile water, and then cultured on the solid Murashige and Skoog (MS) medium (pH 5.8) containing 1% (w/v) agar and 1% (w/v) sucrose. Seeds were stratified at 4°C for 2 days, and then transferred into the growth chamber with day/night cycle of 16 h/8 h, 150 μmol m–2 s–1 irradiance, 23/18°C, and 70% relative humidity. Arabidopsis wild-type (WT) and mutants were grown on MS medium containing 0 or 100 mM NaCl with or without 1 mM theanine. The time points of treatments were illustrated in the corresponding legends. After treatments, seedlings were imaged using a Canon IXUS 130 camera. Meanwhile, all tissues were sampled according to the demands of each experiment, and immediately frozen in liquid nitrogen and stored at −80°C until utilization.
Determination of Fv/Fm
After treatments, the new shoots of tea plants were dark adapted for 30 min before measurement of chlorophyll fluorescence. Maximum photochemical efficiency of photosystem II (Fv/Fm) was measured in the 2nd leaves by an imaging-PAM chlorophyll fluorimeter fitted with a computer-operated PAM-control unit (IMAG-MAXI; Heinz Walz, Effeltrich, Germany) as described previously (Li et al., 2018b).
Determination of Chlorophyll Content
Total chlorophyll in seedlings leaves was extracted using 95% (v/v) ethanol for 48 h in darkness, and the content was then calculated by examining the absorbance at 649, 665, and 470 nm as previously described (Lichtenthaler, 1987).
Measurement of Free Amino Acids
Amino acids in tea leaves were detected by the method previously described (Lu et al., 2019). Briefly, amino acids were extracted from 0.1 g samples with 2 ml of 4% (w/v) sulfosalicylic acid. After ultrasound extraction for 30 min at 50°C, and centrifuged at 12,000 g for 30 min, supernatants were filtered through a 0.22 μm filter for the amino acid content assay. Amino acids were separated by the High-Speed Amino Acid Analyzer system used a mobile phase involving lithium citrate and UV–Vis detection at 570 and 440 nm. The amounts of amino acids were determined according to the calibration curve of amino acid standard.
Determination of Theanine Content
Theanine was extracted with distilled water as previously described, with some modifications (Tsushida and Takeo, 1984). About 100 mg of freeze-dried sample powder were dissolved in 3 ml distilled water and heated in a water bath at 100°C for 30 min. After centrifugation at 13,000 rpm for 20 min, the supernatant was filtered with 0.22 μm filter for subsequent HPLC-based analysis of theanine content.
The detection conditions of HPLC analysis were as previously described (Dong et al., 2020). Theanine content was detected using the HPLC analysis (Waters e2695 system consisting a 2489 ultraviolet (UV)-visible detector, Waters Corporation, Milford, MA, United States), equipped with a C18 column (5 μm, 250 mm × 4.6 mm) at 28°C. The mobile phase comprised water (A), acetonitrile (B), and the gradient elution was performed as follows: B 0% (v/v) to 100% at 40 min, to 100% at 45 min and to 0% at 60 min. The amount of theanine was determined according to a calibration curve of theanine standard. L-Theanine standard and acetonitrile were purchased from Sigma-Aldrich (St Louis, MO, United States).
Determination of Reactive Oxygen Species
H2O2 and superoxide anion levels were detected by histochemical staining methods (Xie et al., 2012). For H2O2 detection, leaves were incubated in 1 mg ml–1 solution of 3, 3′-diaminobenzidine (DAB) in 50 mM Tris-acetate buffer (pH 6.5) for 24 h in darkness at room temperature. For detection, leaves were immersed in a 0.1% solution of nitroblue tetrazolium (NBT) in K-phosphate buffer (pH 6.5) in darkness for 12 h at room temperature. Afterward, the chlorophyll of leaves was removed with 95% ethanol. All samples were observed using a Canon IXUS 130 camera.
Content of thiobarbituric acid-reactive substance (TBARS; an indicator of lipid peroxidation) was measured by the method previously described (Buege and Aust, 1978). 0.5 g fresh tissue was ground with 3 ml 5% trichloroacetic acid (TCA) using a mortar and pestle, then added with 3 ml 0.5% 2-thiobarbituric acid (TBA) in 5% TCA. After incubation in 100°C water bath for 30 min, centrifuged at 12,000g for 15 min, then determined by examining the absorbance at 450, 532, and 600 nm. The concentration of lipid peroxides, together with oxidation modified proteins, was quantified in terms of TBARS amount using an extinction coefficient of 155 mM–1 cm–1 and expressed as nmol g–1 fresh weight (FW).
Determination of Antioxidant Enzyme
About 0.1 g the 2nd leaf sample was homogenized with 1 ml of 50 mM phosphate buffer (pH 7.8), or together with 1 mM ascorbic acid (ASC; in the case of ascorbate peroxidase activity determination), then centrifuged at 12,000g (4°C) for 15 min. Then, supernatants were used for determination of protein concentrations and the enzyme activity assays.
Superoxide dismutase activity was determined by the capacity of inhibiting the photochemical reduction of NBT at A560 (Beauchamp and Fridovich, 1971). Catalase (CAT) activity was measured by the absorbance decrease at 240 nm due to the H2O2 decomposition (extinction coefficient of 40 M–1 cm–1) (Cui et al., 2013). Ascorbate peroxidase (APX) activity was measured by monitoring the decrease at A290 as reduced ascorbic acid was oxidized (extinction coefficient of 2.8 mM–1 cm–1) (Xie et al., 2008). Protein was determined by the method of Bradford (1976).
RNA Extraction and Quantitative Real-Time RT-PCR
Total RNA was extracted using an RNAprep Pure kit (Tiangen, Beijing, China), according to the manufacturer’s instructions. RNA concentration and quality were detected by the NanoDrop 2000 (Thermo Fisher Scientific, Wilmington, DE, United States). cDNAs were synthesized from 1 μg of total RNA using an oligo(dT) primer and an EasyScript All-in-One First-Strand cDNA Synthesis SuperMix (One-Step gDNA Removal) Synthesis (TransGen Biotech, Beijing, China). Real-time quantitative reverse-transcription (RT) PCR was conducted using a QuantStudio 6 Flex System (Thermo Lifetech, United States) with TransStart® Green qPCR SuperMix (TransGen Biotech, Beijing, China) by the gene-specific primers (Supplementary Table 1). The expression levels of genes were normalized to CsGAPDH transcript level, and presented as values relative to corresponding control samples.
Statistical Analysis
Statistical analysis was performed using SPSS 18.0 software. Data were means ± SE of at least three biological replicates. The asterisks indicate significant differences by Student’s t-test (∗P < 0.05). For statistical analysis, data was analyzed by one-way analysis of variance (ANOVA) followed by Duncan’s multiple range test, and P < 0.05 were considered statistically significant.
Results
New Shoots of Tea Plants Were Sensitive to Salt Stress
To examine the sensitivity of new shoots of tea plants to salt stress, the new shoots sampled from tea plantation were incubated in solutions containing 0, 100, 150, and 200 mM NaCl for 3 days (Figures 1A,B). We observed that 100, 150, and 200 mM NaCl severely damaged these new shoots. The effects of salt stress on the representative physiological characters, such as maximum photochemical efficiency of photosystem II (Fv/Fm) and loss of chlorophyll, were investigated. In our experimental conditions, salt stress significantly lowered the Fv/Fm in the 2nd leaves of the new shoots, in proportion to the NaCl concentrations (Figures 1C,D). Compared to the control, chlorophyll contents were also significantly decreased in high salinity-stressed leaves (Figure 1E). When subjected to 150 and 200 mM NaCl, chlorophyll contents were reduced by 15.5% and 24.9%, respectively. These results indicated high salinity imposed significant damage to the new shoots of tea plants.
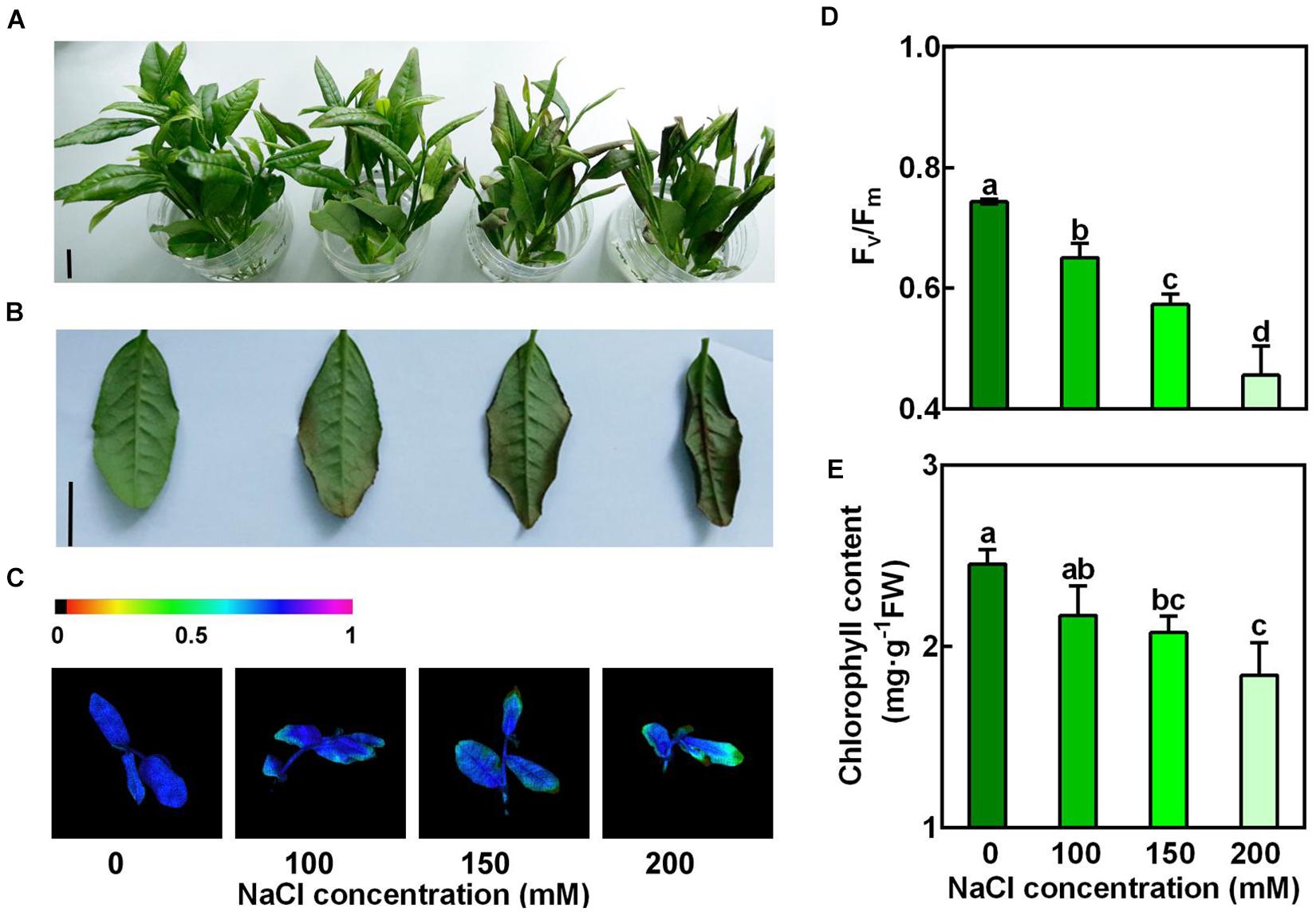
Figure 1. Concentration-dependent effects of NaCl on the new shoots of tea plants. Tea new shoots of tea plants were treated in nutrient solution containing 0, 100, 150, or 200 mM NaCl. (A,B) Phenotypes of the new shoots and the 2nd leaves exposed to 0, 100, 150, or 200 mM NaCl treatments. Photographs were taken 3 days after treatment. Bar = 2 cm. (C,D) Pseudo-color image and statistical analysis of Fv/Fm of the 2nd leaves 2 days after treatment. The Pseudo-color gradient shows the degree of damage: 1, no damage; 0, strong damage. (E) Chlorophyll contents of the 2nd leaves. The contents were determined 3 days after treatment. Data are means ± SE of at least three biological replicates. Bars with different letters are significantly different at P < 0.05 according to Duncan’s multiple range test.
Salt Stress Increased the Accumulation of Glutamate, Glutamine, Proline, γ-Aminobutyric Acid, Alanine and Theanine in the New Shoots
To examine the changes of amino acid accumulation in the new shoots in response to salt stress, the 2nd leaves were sampled 3 days after treatment and were used for amino acid measurement by High-Speed Amino Acid Analyzer system. The glutamate pathway amino acids (glutamine, proline, GABA, arginine, and theanine) were then analyzed (Figure 2A). Alanine, which provides ethylamine for theanine synthesis, was also analyzed. As shown in Figure 2B, NaCl treatments significant increased the accumulation of theanine in the new shoots. For example, it was increased by 0.84-fold after 3 days exposure to 150 mM NaCl compared to the control (0 mM NaCl). Besides, it was evident that NaCl treatments markedly increased the accumulation of alanine, glutamate, glutamine, GABA and proline (Figures 2C–G). Glutamate and alanine were increased 0.87- and 4.73-fold by 150 mM NaCl, respectively. In contrast, arginine content was not significantly changed by salt stress (Figure 2H). These results indicated that glutamate pathway amino acids were induced by salt stress, and implied a role of these amino acids in tolerance to salt stress.
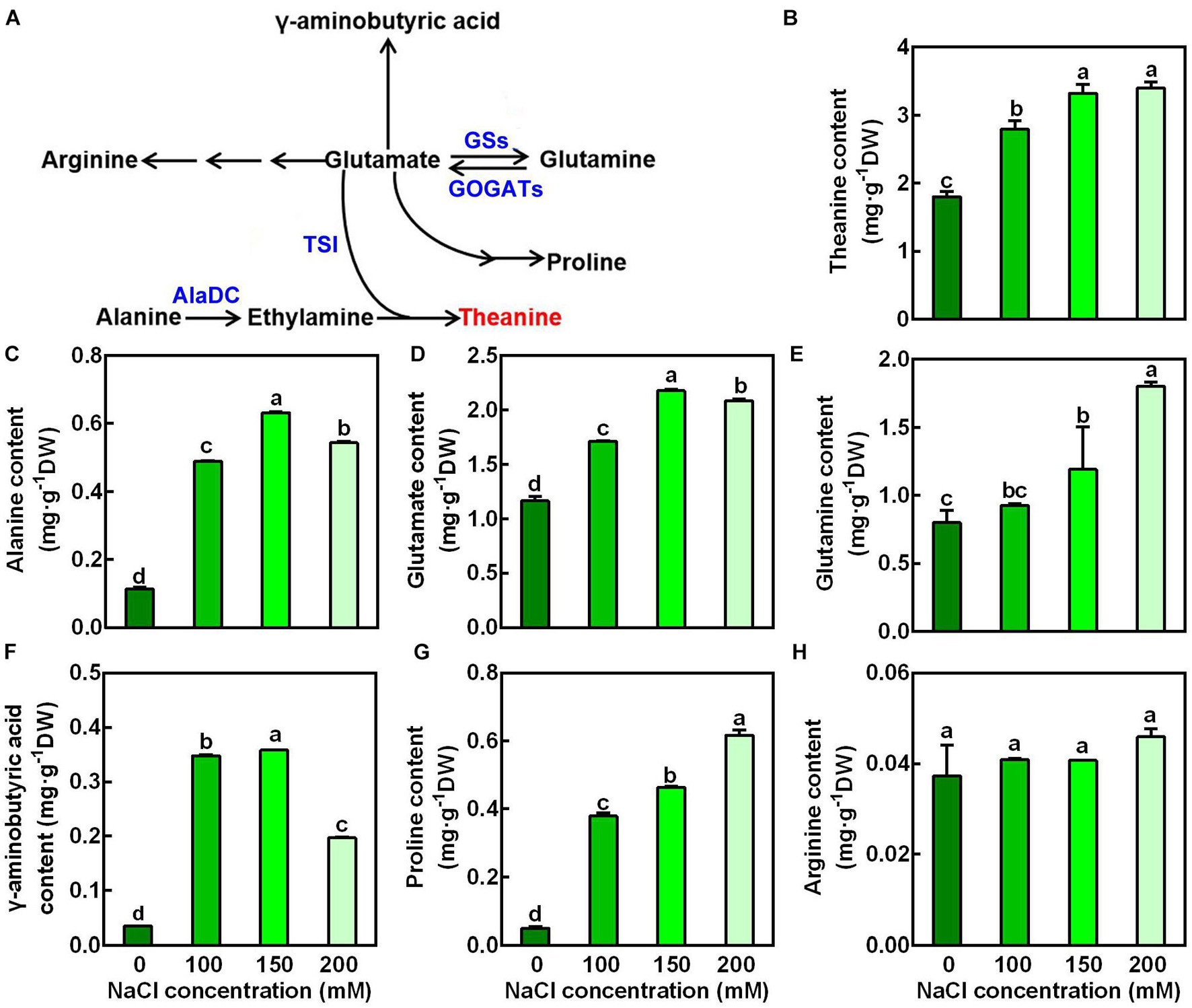
Figure 2. Effects of NaCl treatments on the accumulation of glutamate-pathway and theanine-related amino acids in the 2nd leaves of the new shoots. (A) Biosynthetic pathways of glutamate-pathway and theanine-related amino acids. GS, GOGAT, AlaDC, and TSI represent genes encoding glutamine synthetase, glutamate synthase, alanine decarboxylase, and theanine synthatase, respectively. (B–H) Amino acid contents in the 2nd leaves of new shoots. The new shoots were treated in nutrient solution containing 0, 100, 150, or 200 mM NaCl for 3 days before the leaves were collected for amino acid content measurement. Theanine (B), alanine (C), glutamate (D), glutamine (E), γ-aminobutyric acid (F), proline (G), arginine (H) were detected by the High-Speed Amino Acid Analyzer system. Data are means ± SE of three biological replicates. Bars with different letters are significantly different at P < 0.05 according to Duncan’s multiple range test.
To ascertain the effect of salt stress on the theanine biosynthesis, transcript levels of genes encoding theanine synthatase (TS), alanine decarboxylase (AlaDC), glutamine synthetase (GS), and glutamate synthase (GOGAT) (Figure 2A) were analyzed by real-time RT-PCR. Interestingly, compared to the control condition, the expression of theanine biosynthetic pathway genes, including CsAlaDC, CsGOGAT1, CsGOGAT2, and CsTSI was significantly induced by salt stress in a concentration-dependent manner (Figure 3). The expression of CsGSII-1.1 was also slightly induced; however, the expression of CsGSII-1.2, CsGSII-1.2, and CsGSII-2 was largely repressed by salt stress. These results implied salt stress increased theanine accumulation was probably through induced theanine biosynthesis in the new shoots of tea plants.
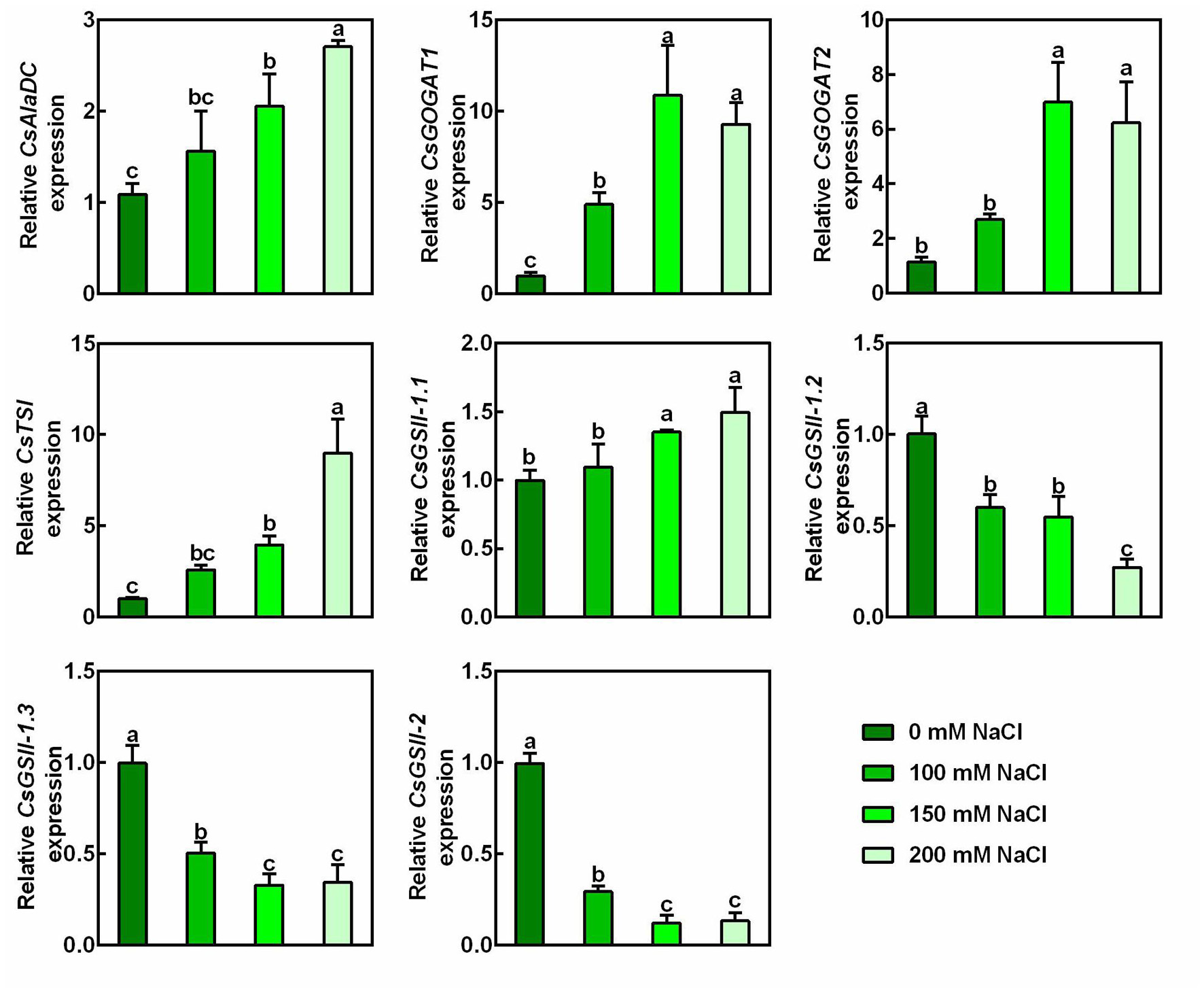
Figure 3. Effects of NaCl treatments on expression of theanine biosynthetic genes in the 2nd leaves of the new shoots. The new shoots of tea plants were treated in nutrient solution containing 0, 100, 150, or 200 mM NaCl. The expression of CsAlaDC (TEA005658), CsGOGAT1 (TEA003892), CsGOGAT2 (TEA026779), CsTSI (TEA015198), CsGSII-1.1 (TEA015580), CsGSII-1.2 (TEA032123), CsGSII-1.3 (TEA032217), and CsGSII-2 (TEA028194) in the 2nd leaves 2 days after treatment was analyzed by qRT-PCR. Expression levels were presented as values relative to control samples, after normalization to the internal control gene CsGAPDH (TEA025584). Data are means ± SE of three biological replicates. Bars with different letters are significantly different at P < 0.05 according to Duncan’s multiple range test.
Given that 150 mM NaCl significantly inhibited Fv/Fm and chlorophyll accumulation, and induced theanine biosynthesis, this concentration of NaCl was chosen for the further experiments.
Exogenous Theanine Application Alleviated Salt Stress Damage to the New Shoots of Tea Plants
Previously studies showed salt stress-induced proline and GABA improve tolerance to salt stress in plants (Moukhtari et al., 2020; Wu et al., 2020). To examine whether theanine plays a role in enhancing salt stress tolerance in the new shoots of tea plants, the effects of 10 mM exogenous theanine on the salt stress sensitivity, Fv/Fm, and chlorophyll level in the absence and presence of 150 mM NaCl treatment were investigated. Under salt stress treatment, exogenous theanine application significantly alleviated the damage of salt stress to the new shoots. This is evidenced by that exogenous theanine improved the performance, Fv/Fm and chlorophyll content of the new shoots in response to salt stress (Figures 4A–D). In the molecular level, the expression of salt stress-responsive genes, such as CsRD22, CsDREB2C, CsDREB1, and CsTSI, was reduced to the control level by theanine (Figures 4 E–H). In addition, application of 1 mM theanine to the model plant Arabidopsis also improved the salt stress tolerance of the seedlings (Supplementary Figure 1). It is noteworthy that exogenous theanine application under normal condition did not showed obvious effects on the new shoots of tea plants and Arabidopsis seedlings (Figures 4A–H and Supplementary Figure 1). These results indicated that theanine improved salt stress tolerance of the new shoots of tea plants.
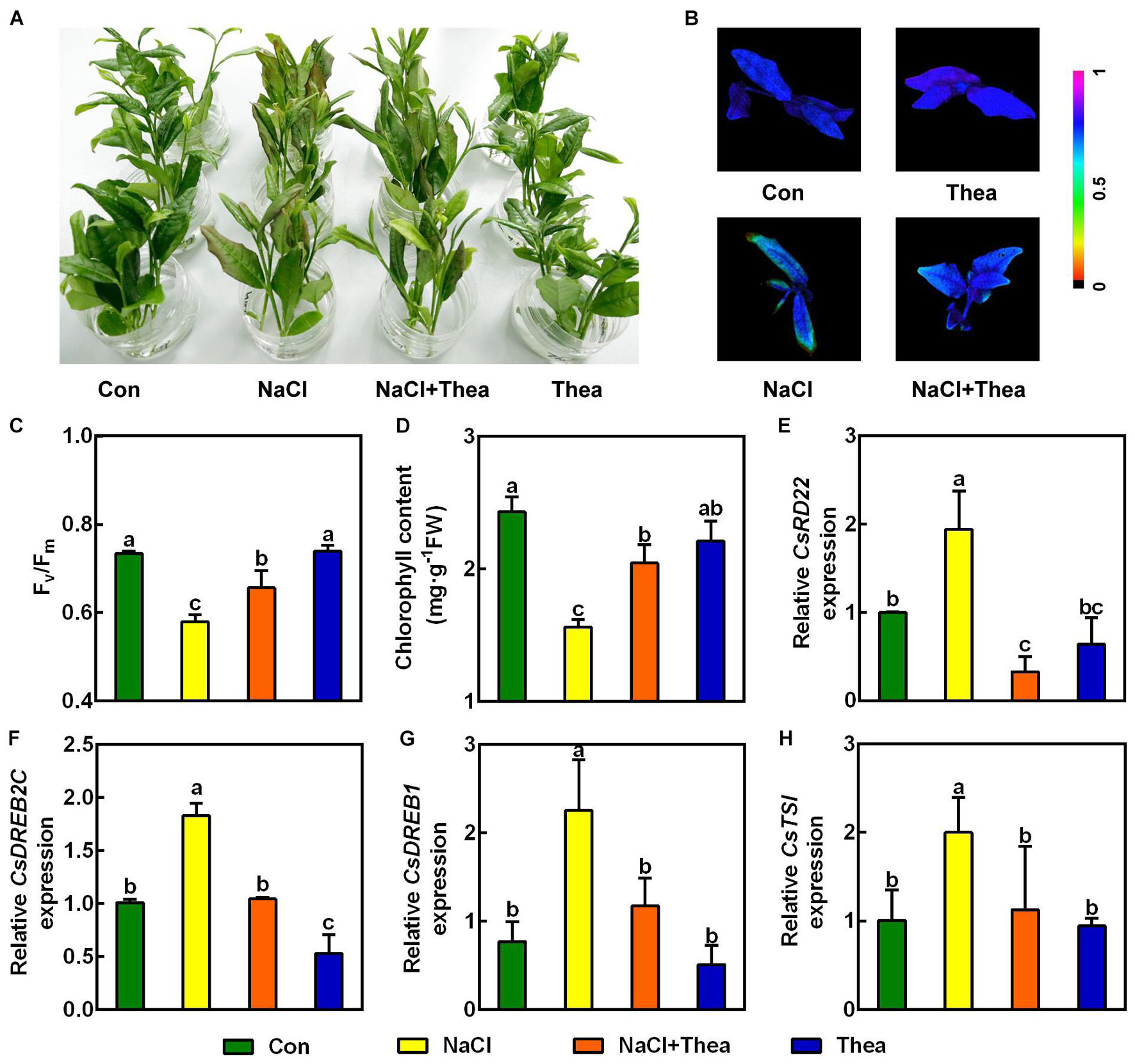
Figure 4. Exogenous theanine alleviated salt stress to the new shoots of tea plants. The new shoots of tea plants were treated in nutrient solution containing 150 mM NaCl (NaCl), 10 mM theanine (Thea), 150 mM NaCl and 10 mM Thea (NaCl + Thea), with normal nutrient solution as control (Con). (A) Phenotypes of the new shoots. Photographs were taken 3 days after treatment. (B,C) Pseudo-color image and statistical analysis of Fv/Fm of the 2nd leaves 2 days after treatment. The Pseudo-color gradient shows the degree of damage: 1, no damage; 0, strong damage. (D) Chlorophyll contents in the 2nd leaves. The contents were determined 3 days after treatment. (E–H) The expression of CsRD22 (TEA005584), CsDREB2C (TEA000861), CsDREB1 (TEA010806), and CsTSI (TEA015198) in 2nd leaves. The expression was analyzed 2 days after treatment by qRT-PCR. Expression levels were presented as values relative to Con samples, after normalization to the internal control gene CsGAPDH (TEA025584). Data are means ± SE of three biological replicates. Bars with different letters are significantly different at P < 0.05 according to Duncan’s multiple range test.
Exogenous Theanine Reduced Reactive Oxygen Species Accumulation in the Salt Stressed Leaves
Reactive oxygen species are induced by salt stress, which leads to growth stunt and even cell death (Considine and Foyer, 2021). To explore the mechanism underlying the improved salt stress tolerance by theanine, we examined the effects of theanine on ROS levels. By histochemical staining with diaminobenzidine (DAB) and nitroblue tetrazolium (NBT) staining, the accumulation of H2O2 and in the 2nd leaves were, respectively, analyzed. As expected, compared to the shoots under control condition, NaCl-treated leaves accumulated more H2O2 and , while NaCl and theanine co-treatment greatly reduced H2O2 and accumulation (Figures 5A,B). Consistently, theaine also reduced thiobarbituric acid-reactive substances (TBARS; an indicator of lipid peroxidation) accumulation in the salt stressed leaves (Figure 5C). These results suggested that theanine alleviated salt stress-induced oxidative damage to new shoots of tea plants.
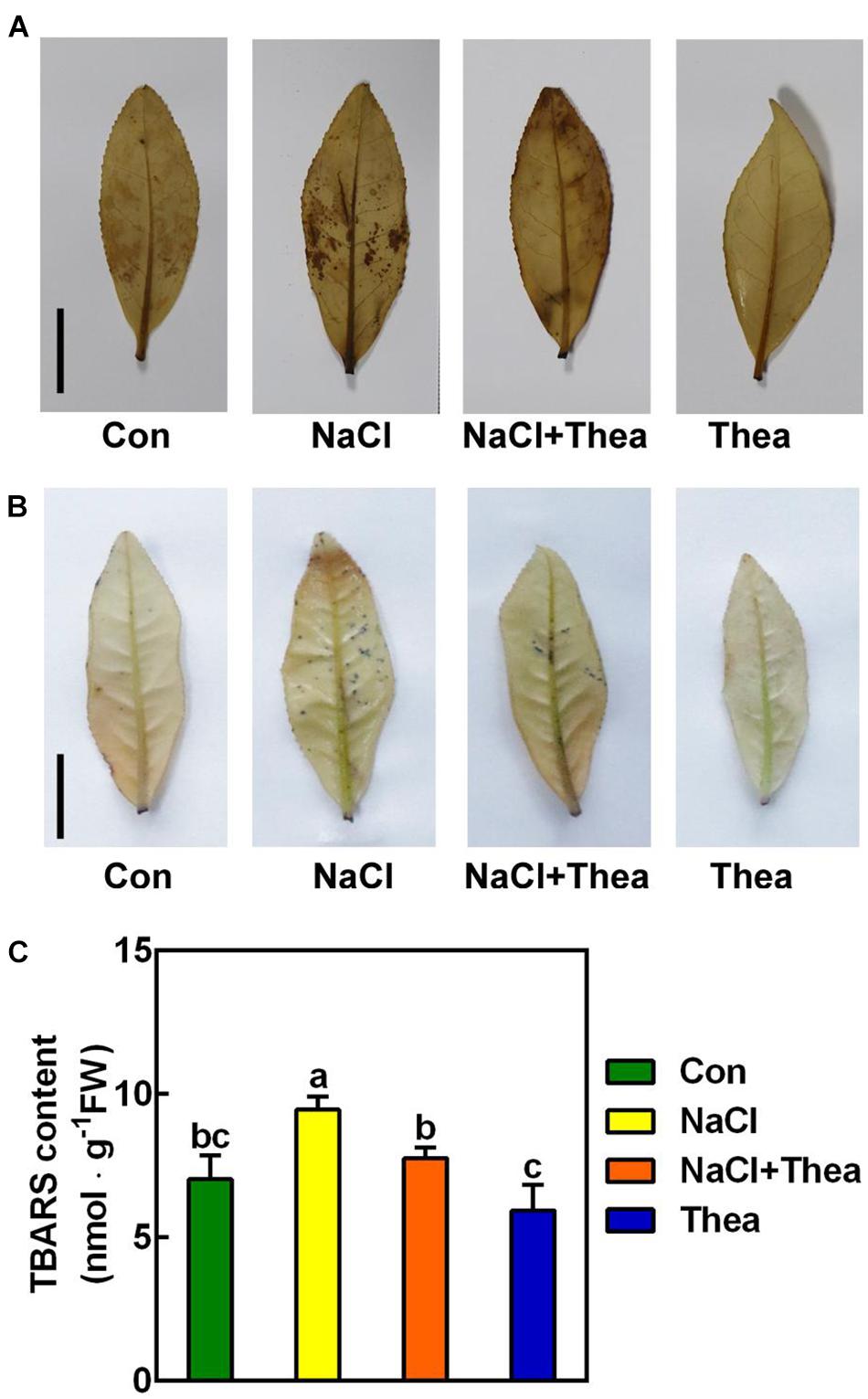
Figure 5. The effects of exogenous theanine on reactive oxygen species (ROS) accumulation in the leaves of new shoot exposed to salt stress. The new shoots of tea plants were treated in nutrient solution containing 150 mM NaCl (NaCl), 10 mM theanine (Thea), 150 mM NaCl, and 10 mM Thea (NaCl + Thea), with normal nutrient solution as control (Con). (A,B) H2O2 (A) and (B) accumulation in the 2nd leaves of new shoots exposed to Con, NaCl, NaCl + Thea, and Thea. Three days after treatment, the leaves were stained with DAB and NBT, respectively, and were immediately photographed. Scale bar = 2 cm. (C) Thiobarbituric acid-reactive substances (TBARS; an indicator of lipid peroxidation) contents in the 2nd leaves. The contents were measured 3 days after treatment. At least three individual samples were analyzed. Data are means ± SE of at least three bilogical replicates. Bars with different letters are significantly different at P < 0.05 according to Duncan’s multiple range test.
To explore how exogenous theanine reduced ROS accumulation, we examined the activities of ROS scavenging enzymes, including SOD, CAT, and APX. The results showed that salt stress increased SOD, CAT, and APX activities, and exogenous theanine further improved the activities of these enzymes (Figures 6A–C). At the level of gene expression, salt stress also significantly induced the expression of CsCAT and CsAPX, and exogenous theanine further significantly induced the expression of CsSOD, CsCAT, and CsAPX (Figures 6D–F). Therefore, exogenous theanine induced the expression of genes encoding ROS scavenging enzymes and increased the activities of these enzymes, under salt stress condition. These results supported the notion that salt stress-induced theanine contributes to salt stress tolerance via (at least partially) ROS scavenging pathway.
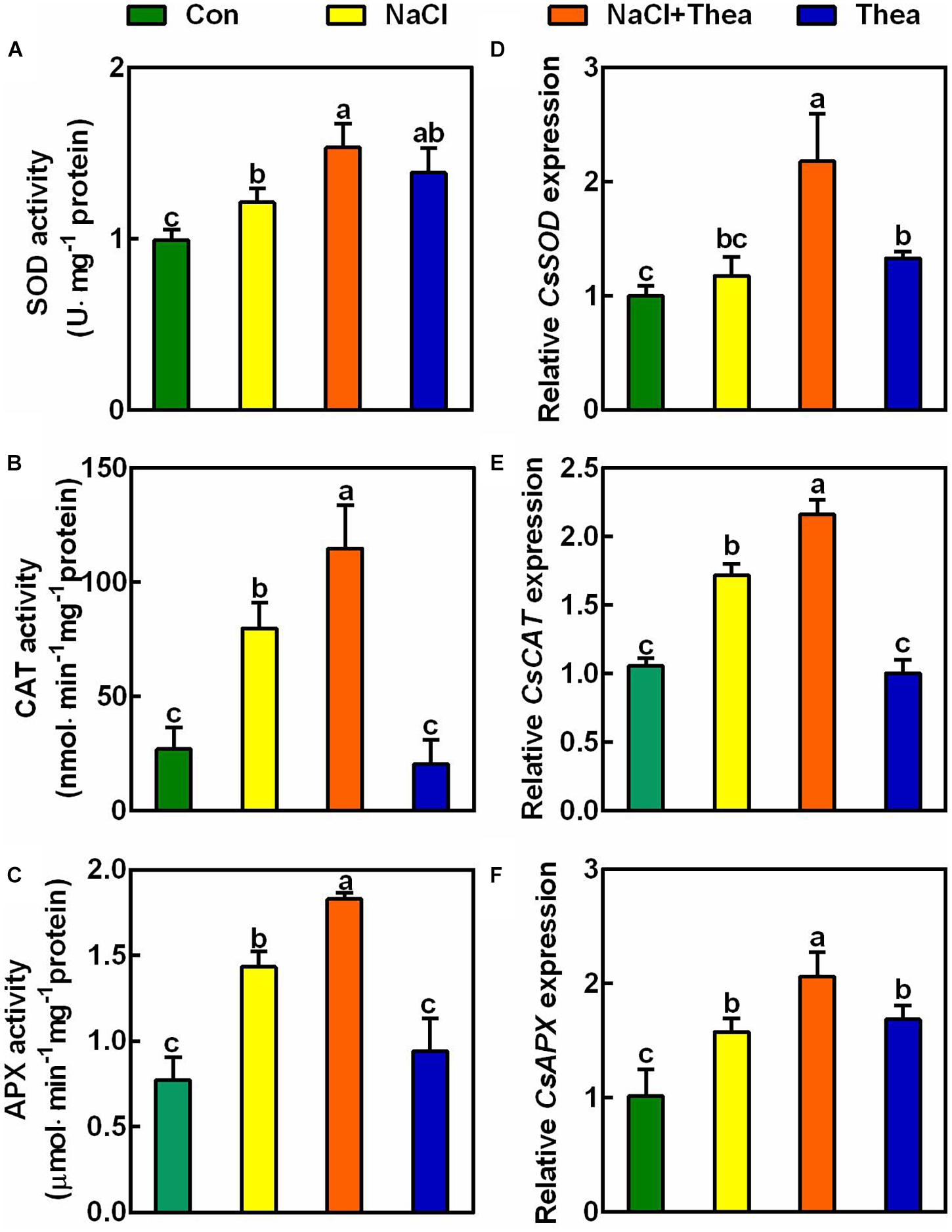
Figure 6. The effects of exogenous theanine on the activities of ROS scavenging enzymes and the expression of genes encoding the enzymes. The new shoots of tea plants were treated for 3 days in nutrient solution containing 150 mM NaCl (NaCl), 10 mM theanine (Thea), 150 mM NaCl and 10 mM Thea (NaCl + Thea), with normal nutrient solution as control (Con). (A–C) The activities of SOD, CAT, and APX in the 2nd leaves of the new shoots. (D–F) The expression of CsSOD (TEA00629), CsCAT (TEA002986), and CsAPX (TEA000543) in the 2nd leaves. The expression was analyzed 2 days after treatment by qRT-PCR. Expression levels were presented as values relative to Con samples, after normalization to the internal control gene CsGAPDH (TEA025584). Data are means ± SE of three biological replicates. Bars with different letters are significantly different at P < 0.05 according to Duncan’s multiple range test.
Genetic Evidence Revealed That Catalase Is Required for Theanine-Induced Salinity Tolerance
Catalases are highly responsive to stresses and are critical for scavenging H2O2 in plants (Mhamdi et al., 2012; Su et al., 2018). There are three genes CAT1, CAT2, and CAT3 encoding catalases in Arabidopsis. cat2cat3 double mutant and cat1cat2cat3 triple mutant were obtained in Arabidopsis (Su et al., 2018). To investigate the role of ROS scavenging pathway in theanine-induced salt stress tolerance, we used these double and triple mutants for further study, given that we cannot knock down or overexpress the CsCATs in tea plants. Similar to the wild-type (WT), cat2cat3 and cat1cat2cat3 mutants also exhibited hypersensitivity to NaCl treatment (Figure 7). However, unlike WT, theanine did not improve salt stress tolerance of cat2cat3 and cat1cat2cat3 mutants, in terms of primary root growth and chlorophyll content (Figures 7A–C). These results indicated that catalase-mediated H2O2 scavenging pathway is required for theanine-induced salt stress tolerance in Arabidopsis, and implied that salt stress-induced theanine improves salt stress tolerance by modulating H2O2 scavenging in tea plants.
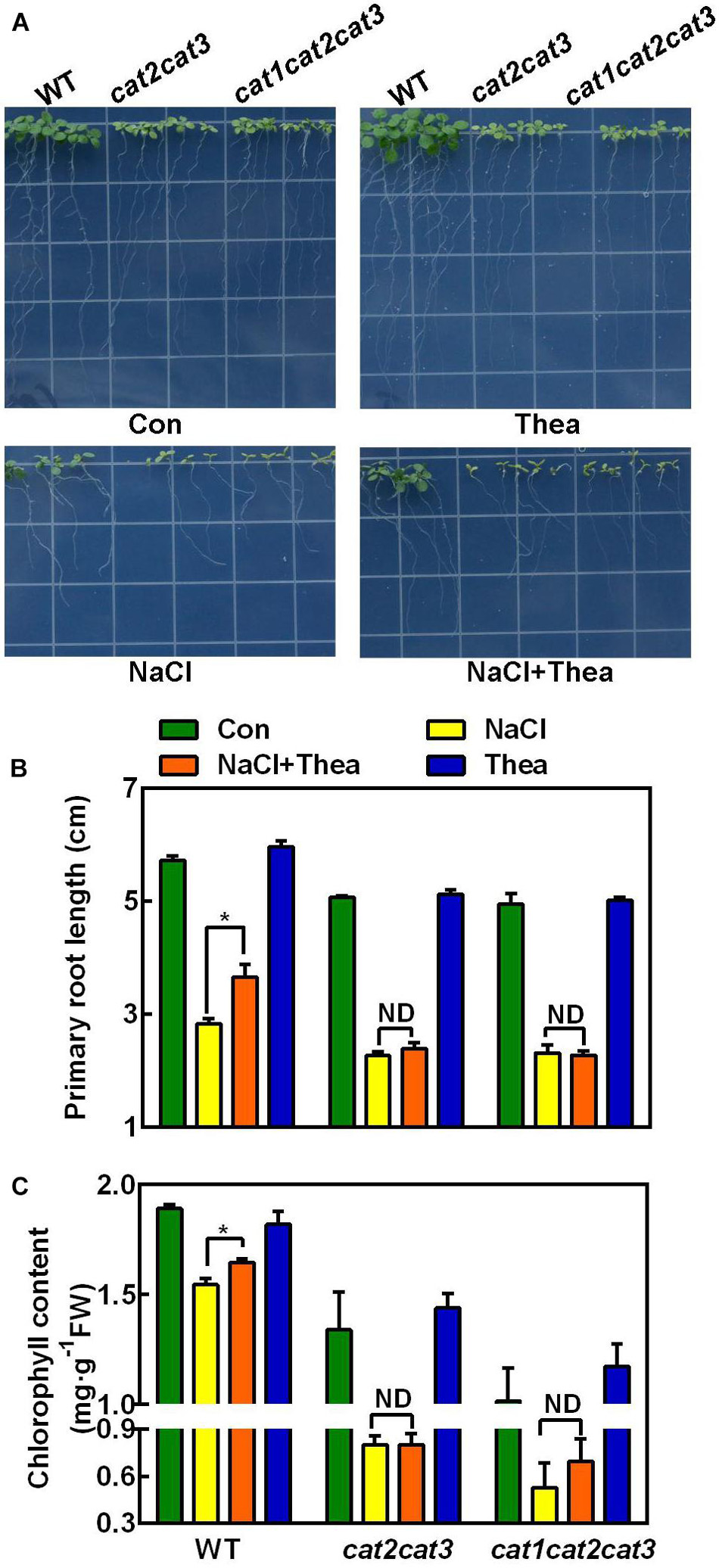
Figure 7. The effects of exogenous theanine on the salt stress tolerance of Arabidopsis wild-type (WT), cat2cat3 and cat1cat2cat3 mutants. (A) Phenotypes of WT Arabidopsis and cat2cat3 and cat1cat2cat3 mutants grown on MS medium containing 100 mM NaCl (NaCl), 100 mM NaCl and 1 mM theanine (NaCl + Thea), 1 mM theanine (Thea) for 10 days. The medium without NaCl and theanine was the control (Con). (B,C) Primary root length and total chlorophyll content in leaves of WT, cat2cat3 and cat1cat2cat3 mutants grown under the indicated conditions. Data are means ± SE of three biological replicates. Asterisks indicate significant differences from WT under NaCl treatment based on Student’s t-test (*P < 0.05). ND, no significant difference.
Discussion
Theanine has favorable physiological effects on human health, including antioxidant and immune-regulation properties (Deng et al., 2016; Gong et al., 2019). A positive correlation exists between theanine and antioxidative activity, suggesting that theanine plays a role in modulating redox homeostasis in animals (Li et al., 2012; Deng et al., 2016; Zeng et al., 2020). Previous results showed that theanine contents in tea plants were influenced by abiotic stress, such as salinity, drought, and heat stress (Deng et al., 2012; Wang et al., 2016; Li et al., 2018a). It is known that these abiotic stresses, especially salt stress, elicits the accumulation of ROS and results in redox imbalance (Bose et al., 2013; Considine and Foyer, 2021).
Under our experimental conditions, theanine accumulation was obviously induced by salt stress in the new shoots of tea plants (Figure 2B). Besides, salt stress improved the glutamate accumulation, a direct substrate for theanine biosynthesis, as well as the alanine, a precursor of ethylamine in tea plants (Figures 2C,D; Sasaoka and Kito, 1964; Takeo, 1974). These findings are consistent with the former result (Deng et al., 2012). We further showed that the induced expression of theanine biosynthetic genes CsAlaDC, CsGOGAT1, CsGOGAT2, and CsTSI could be responsible for the increased theanine biosynthesis and accumulation.
Salt stress significantly inhibits electron transport at the oxidizing side of photosystem II (PSII) and decreases the PSII activity, which leads to reduced photosynthesis (Xia et al., 2004). A decline in chlorophyll content can also decrease the photosynthesis in plant during salinity stress (Heuer and Plaut, 1989). Thereby, the growth and productivity of plants were severely affected by salt stress (Jamil et al., 2014). Interestingly, in this study, we found the application of theanine was able to relieve high salinity-induced damage to the new shoots, the reduction of maximum quantum efficiency of photosystem II (Fv/Fm) and chlorophyll content (Figures 4A–D). Additionally, the induction of salt stress-responsive genes including CsRD22, CsDREB2C, CsDREB1, and CsTSI was also significantly recovered by theanine (Figures 4E–H). Combined with the protective effect of theanine in model plant Arabidopsis wild-type seedlings against salt stress (Supplementary Figure 1), and the high abundance of theanine in tea plants, we speculate that theanine is a key regulator of salt stress resistance in tea plants.
Redox imbalance, such as the overproduction of ROS and lipid peroxidation, was induced by salt stress (Miller et al., 2010; Considine and Foyer, 2021). ROS, in turn, inhibit protein synthesis and damage the protein subunits and pigments of PSII (Murata et al., 2007). Previous studies strongly revealed that an efficient antioxidant system contributes to salt stress tolerance in plants (Miller et al., 2010). The health benefits of theanine in animals are associated with its antioxidant actions (Williams et al., 2019). The present study demonstrated that theanine counteracted NaCl-induced H2O2, and TBARS accumulation in the leaves of new shoots (Figure 5), and also increased the activities of SOD, CAT, and APX and the expression of the corresponding genes (Figures 6A–F). These results were consistent with the observations in animals. For example, theanine prevented ethanol-triggered ROS and MDA generation via restoring the antioxidant capability of hepatocytes (Li et al., 2012). The anti-inflammatory and antioxidative actions triggered by theanine were further confirmed in Escherichia coli-infected and D-galactose-induced liver dysfunction (Deng et al., 2016; Zeng et al., 2020). Together, our results suggested that theanine modulates oxidative damage in tea plants under salt stress condition.
Among the antioxidant enzymes, catalase is an important respiratory enzyme in plants, and its activity is closely related to plant resistance (Mhamdi et al., 2012). Chinese cabbage plants overexpressing maize catalase (ZmCAT) showed enhanced tolerance to high salinity (Tseng et al., 2007). Silencing GhWRKY46 in cotton by virus-induced gene silencing reduced the catalase activity, and resulted in hypersensitivity to salt stress (Li et al., 2021). It was also found that Arabidopsis cat2-1 mutant exhibited increased sensitivity to salt stress (Bueso et al., 2007). In the present study, we also found Arabidopsis cat2cat3 double mutant and cat1cat2cat3 triple mutant were hypersensitive to salt stress, and theanine could not enhance the salt stress tolerance of these mutants (Figure 7). This result provided genetic evidence that CAT-mediated redox homeostasis is required for theanine-induced salt stress tolerance.
Production of ROS was accelerated by over-reduced ferredoxin during photosynthetic electron transfer in the chloroplasts, impaired electron transport processes in the mitochondria and photorespiration in peroxisomes in plants (Ahmed et al., 2009). The nucleus-encoding ROS scavenging enzymes are responsible for maintaining the redox homeostasis in these organelles. In the present study, we showed that theanine application induced the expression of genes encoding SOD, APX, and CAT (Figures 6E–F). However, it is unclear how theanine induced the expression of these genes. Recently, the retrograde signaling from chloroplast to nucleus was reported to be critical for salt stress tolerance in plants (Park and Seo, 2021; Zhuang et al., 2021). This retrograde signaling is required for the induction of ROS scavenging pathway genes such as CSD2 (Zhuang et al., 2021). Interestingly, theanine is mainly distributed in cytosol and chloroplast in the new shoots of tea plants (Fu et al., 2021). It will be interesting to investigate whether theanine regulates the expression of SOD, APX, and CAT via chloroplast to nucleus retrograde signaling pathway.
Many studies have reported that proline or GABA improve salt stress tolerance in plants (Moukhtari et al., 2020; Wu et al., 2020). In this study, we showed that salt stress induces theanine biosynthesis, and the induced theanine is associated with enhanced salt stress tolerance in the new shoots of tea plants. Theanine-induced salt stress tolerance is probably via modulating ROS homeostasis in a CAT-dependent ROS scavenging pathway (Figure 8). To our knowledge, this is the first report to link theanine with salt stress tolerance and ROS homeostasis in tea plants. This study provided new insights into the physiological role of theanine in tea plants.
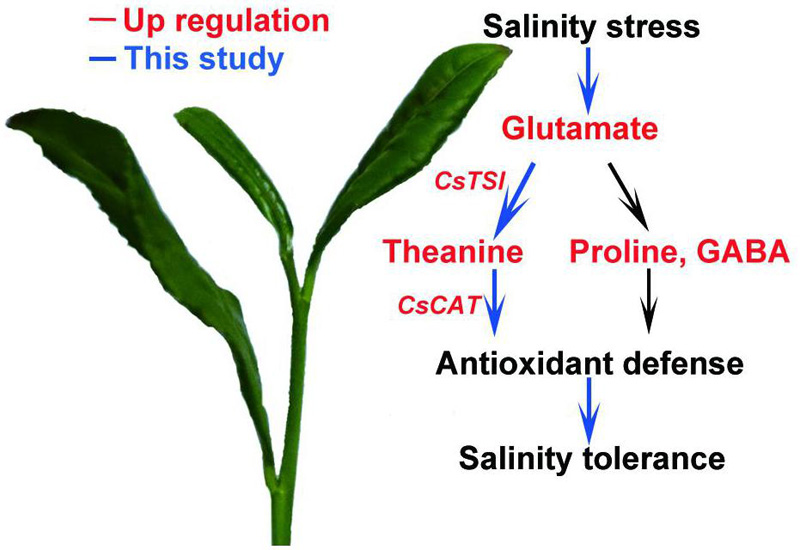
Figure 8. A proposed model of theanine-mediated tolerance to salt stress in tea plants. Salt stress increases glutamate accumulation and the expression of theanine biosynthetic genes to promote theanine biosynthesis. Theanine enhances plant high-salinity tolerance through a CAT-dependent ROS scavenging pathway.
Data Availability Statement
The original contributions presented in the study are included in the article/Supplementary Material, further inquiries can be directed to the corresponding author/s.
Author Contributions
ZZ and ZC conceived the study and designed the experiments. ZC, SL, JL, and TC performed the experiments. QG and TY participated in the preparation of plant materials. ZC wrote the manuscript. ZZ revised and finalized the manuscript. All authors read and approved the final version of the manuscript.
Funding
This work was supported by grants from the National Natural Science Foundation of China (3207624 and 3177073), Collaborative Innovation Project of Universities in Anhui Province (GXXT-2020-080), the Base of Introducing Talents for Tea Plant Biology and Quality Chemistry (D20026), and the Talent Research Fund Project of Hefei University (No. 18-19RC13).
Conflict of Interest
The authors declare that the research was conducted in the absence of any commercial or financial relationships that could be construed as a potential conflict of interest.
Publisher’s Note
All claims expressed in this article are solely those of the authors and do not necessarily represent those of their affiliated organizations, or those of the publisher, the editors and the reviewers. Any product that may be evaluated in this article, or claim that may be made by its manufacturer, is not guaranteed or endorsed by the publisher.
Acknowledgments
We thank Changle Ma in College of Life Sciences, Shandong Normal University, China for kindly providing the cat2cat3 and cat1cat2cat3 mutants.
Supplementary Material
The Supplementary Material for this article can be found online at: https://www.frontiersin.org/articles/10.3389/fpls.2021.770398/full#supplementary-material
References
Ahmed, P., Jaleel, C. A., Azooz, M. M., and Nabi, G. (2009). Generation of ROS and non-enzymatic antioxidants during abiotic stress in plants. Bot. Res. Intl. 2, 11–20.
Ashihara, H. (2015). Occurrence, biosynthesis and metabolism of theanine (γ-Glutamyl-L-ethylamide) in plants: a comprehensive review. Nat. Prod. Commun. 10, 803–810. doi: 10.1177/1934578X1501000525
Bai, P., Wei, K., Wang, L., Zhang, F., Ruan, L., Li, H., et al. (2019). Identification of a novel gene encoding the specialized alanine decarboxylase in tea (Camellia sinensis) plants. Molecules 24:540. doi: 10.3390/molecules24030540
Bao, H., Chen, X., Lv, S., Jiang, P., Feng, J., Fan, P., et al. (2015). Virus-induced gene silencing reveals control of reactive oxygen species accumulation and salt tolerance in tomato by γ-aminobutyric acid metabolic pathway. Plant Cell Environ. 38, 600–613. doi: 10.1111/pce.12419
Beauchamp, C., and Fridovich, I. (1971). Superoxide dismutase: improved assays and an assay applicable to acrylamide gels. Anal. Biochem. 44, 276–287. doi: 10.1016/0003-2697(71)90370-8
Ben, P., Zhang, Z., Zhu, Y., Xiong, A., Gao, Y., Mu, J., et al. (2016). L-Theanine attenuates cadmium-induced neurotoxicity through the inhibition of oxidative damage and tau hyperphosphorylation. Neurotoxicology 57, 95–103. doi: 10.1016/j.neuro.2016.09.010
Bose, J., Rodrigo-Moreno, A., and Shabala, S. (2013). ROS homeostasis in halophytes in the context of salinity stress tolerance. J. Exp. Bot. 65, 1241–1257. doi: 10.1093/jxb/ert430
Bradford, M. M. (1976). A rapid and sensitive method for the quantitation of microgram quantities of protein utilizing the principle of protein-dye binding. Anal. Biochem. 72, 248–254. doi: 10.1016/0003-2697(76)90527-3
Buege, J. A., and Aust, S. D. (1978). Microsomal lipid peroxidation. Methods Enzymol. 52, 302–310. doi: 10.1016/S0076-6879(78)52032-6
Bueso, E., Alejandro, S., Carbonell, P., Perez-Amador, M. A., and Serrano, R. (2007). The lithium tolerance of the Arabidopsis cat2 mutant reveals a cross-talk between oxidative stress and ethylene. Plant J. 52, 1052–1065. doi: 10.1111/j.1365-313X.2007.03305.x
Causapé, J., Quílez, D., and Aragüés, R. (2004). Assessment of irrigation and environmental quality at the hydrological basin level: ii. Salt and nitrate loads in irrigation return flows. Agr. Water Manage. 70, 211–228. doi: 10.1016/j.agwat.2004.06.006
Chang, C., Wang, B., Shi, L., Li, Y., Duo, L., and Zhang, W. (2010). Alleviation of salt stress-induced inhibition of seed germination in cucumber (Cucumis sativus L.) by ethylene and glutamate. J. Plant Physiol. 167, 1152–1156. doi: 10.1016/j.jplph.2010.03.018
Chen, L., Zhou, Z. X., and Yang, Y. J. (2007). Genetic improvement and breeding of tea plant (Camellia sinensis) in China: from individual selection to hybridization and molecular breeding. Euphytica 154, 239–248. doi: 10.1007/s10681-006-9292-3
Cheng, S., Fu, X., Wang, X., Liao, Y., Zeng, L., Dong, F., et al. (2017). Studies on biochemical formation pathway of the amino acid L-theanine in tea (Camellia sinensis) and other plants. J. Agric. Food Chem. 65, 7210–7216. doi: 10.1021/acs.jafc.7b02437
Considine, M. J., and Foyer, C. H. (2021). Stress effects on the reactive oxygen species (ROS)-dependent regulation of plant growth and development. J. Exp. Bot. 72, 5795–5806. doi: 10.1093/jxb/erab265
Cui, W., Zhang, J., Xuan, W., and Xie, Y. (2013). Up-regulation of heme oxygenase-1 contributes to the amelioration of aluminum-induced oxidative stress in Medicago sativa. J. Plant Physiol. 170, 1328–1336. doi: 10.1016/j.jplph.2013.05.014
Deb, S., Dutta, A., Phukan, B. C., Manivasagam, T., Justin Thenmozhi, A., Bhattacharya, P., et al. (2019). Neuroprotective attributes of L-theanine, a bioactive amino acid of tea, and its potential role in Parkinson’s disease therapeutics. Neurochem. Int. 129:104478. doi: 10.1016/j.neuint.2019.104478
Deng, W. W., Wang, S., Chen, Q., Zhang, Z. Z., and Hu, X. Y. (2012). Effect of salt treatment on theanine biosynthesis in Camellia sinensis seedlings. Plant Physiol. Biochem. 56, 35–40. doi: 10.1016/j.plaphy.2012.04.003
Deng, Y., Xiao, W., Chen, L., Liu, Q., Liu, Z., and Gong, Z. (2016). In vivo antioxidative effects of L-theanine in the presence or absence of Escherichia coli-induced oxidative stress. J. Funct. Foods 24, 527–536. doi: 10.1016/j.jff.2016.04.029
Dluzniewska, P., Gessler, A., Dietrich, H., Schnitzler, J. P., Teuber, M., and Rennenberg, H. (2007). Nitrogen uptake and metabolism in Populus×canescens as affected by salinity. New Phytol. 173, 279–293. doi: 10.1111/j.1469-8137.2006.01908.x
Dong, C., Li, F., Yang, T., Feng, L., Zhang, S., Li, F., et al. (2020). Theanine transporters identified in tea plants (Camellia sinensis L.). Plant J. 101, 57–70. doi: 10.1111/tpj.14517
Fu, X., Liao, Y., Cheng, S., Xu, X., Grierson, D., and Yang, Z. (2021). Nonaqueous fractionation and overexpression of fluorescent-tagged enzymes reveals the subcellular sites of L-theanine biosynthesis in tea. Plant Biotechnol. J. 19, 98–108. doi: 10.1111/pbi.13445
Gong, Z., Lin, L., Liu, Z., Zhang, S., Liu, A., Chen, L., et al. (2019). Immune-modulatory effects and mechanism of action of L-theanine on ETEC-induced immune-stressed mice via nucleotide-binding oligomerization domain-like receptor signaling pathway. J. Funct. Foods 54, 32–40. doi: 10.1016/j.jff.2019.01.011
Groat, R. G., and Vance, C. P. (1981). Root nodule enzymes of ammonia assimilation in Alfalfa (Medicago sativa L.): development patterns and response to applied nitrogen. Plant Physiol. 67, 1198–1203. doi: 10.1104/pp.67.6.1198
Hayat, S., Hayat, Q., Alyemeni, M. N., Wani, A. S., Pichtel, J., and Ahmad, A. (2012). Role of proline under changing environments: a review. Plant Signal. Behav. 7, 1456–1466. doi: 10.4161/psb.21949
Heuer, B., and Plaut, Z. (1989). Photosynthesis and osmotic adjustment of two sugarbeet cultivars grown under saline conditions. J. Exp. Bot. 4, 437–440. doi: 10.1093/jxb/40.4.437
Jamil, M., Rehman, S. U., and Rha, E. S. (2014). Response of growth, PSII photochemistry and chlorophyll content to salt stress in four Brassica species. Life Sci. J. 11, 139–145.
Konishi, S., Miyamoto, S., and Taki, T. (1985). Stimulatory effects of aluminum on tea plants growth under low and high phosphorus supply. Soil Sci. Plant Nutr. 31, 361–368. doi: 10.1080/00380768.1985.10557443
Li, G., Ye, Y., Kang, J., Yao, X., Zhang, Y., Jiang, W., et al. (2012). L-Theanine prevents alcoholic liver injury through enhancing the antioxidant capability of hepatocytes. Food Chem. Toxicol. 50, 363–372. doi: 10.1016/j.fct.2011.10.036
Li, X., Wei, J. P., Ahammed, G. J., Zhang, L., Li, Y., Yan, P., et al. (2018a). Brassinosteroids attenuate moderate high temperature-caused decline in tea quality by enhancing theanine biosynthesis in Camellia sinensis L. Front. Plant Sci. 9:1016. doi: 10.3389/fpls.2018.01016
Li, X., Wei, J. P., Scott, E. R., Liu, J. W., Shuai, G., Li, Y., et al. (2018b). Exogenous melatonin alleviates cold stress by promoting antioxidant defense and redox homeostasis in Camellia sinensis L. Molecules 23:65. doi: 10.3390/molecules23010165
Li, Y., Chen, H., Li, S., Yang, C., and Wang, D. J. (2021). GhWRKY46 from upland cotton positively regulates the drought and salt stress responses in plant. Environ. Exp. Bot. 186:104438. doi: 10.1016/j.envexpbot.2021.104438
Lichtenthaler, H. K. (1987). Chlorophylls and carotenoids: pigments of photosynthetic biomembranes. Methods Enzymol. 148, 350–382. doi: 10.1016/0076-6879(87)48036-1
Lu, M., Han, J., Zhu, B., Jia, H., Yang, T., Wang, R., et al. (2019). Significantly increased amino acid accumulation in a novel albino branch of the tea plant (Camellia sinensis). Planta 249, 363–376. doi: 10.1007/s00425-018-3007-6
Mhamdi, A., Noctor, G., and Baker, A. (2012). Plant catalases: peroxisomal redox guardians. Arch. Biochem. Biophys. 525, 181–194. doi: 10.1016/j.abb.2012.04.015
Miflin, B. J., and Habash, D. Z. (2002). The role of glutamine synthetase and glutamate dehydrogenase in nitrogen assimilation and possibilities for improvement in the nitrogen utilization of crops. J. Exp. Bot. 53, 979–987. doi: 10.1093/jexbot/53.370.979
Miller, G., Suzuki, N., Ciftci-Yilmaz, S., and Mittler, R. J. P. (2010). Reactive oxygen species homeostasis and signalling during drought and salinity stresses. Plant Cell Environ. 33, 453–467. doi: 10.1111/j.1365-3040.2009.02041.x
Moukhtari, A. E., Cabassa-Hourton, C., Farissi, M., and Savouré, A. (2020). How does proline treatment promote salt stress tolerance during crop plant development. Front. Plant Sci. 11:1127. doi: 10.3389/fpls.2020.01127
Murata, N., Takahashi, S., Nishiyama, Y., and Allakhverdiev, S. I. (2007). Photoinhibition of photosystem II under environmental stress. Biochim. Biophys. Acta 1767, 414–421. doi: 10.1016/j.bbabio.2006.11.019
Narukawa, M., Morita, K., and Hayashi, Y. (2008). L-theanine elicits an umami taste with inosine 5′-monophosphate. Biosci. Biotechnol. Biochem. 72, 3015–3017. doi: 10.1271/bbb.80328
Park, K. Y., and Seo, S. Y. (2021). Translocation of chloroplast NPR1 to the nucleus in retrograde signaling for adaptive response to salt stress in tobacco. bioRxiv [Preprint] doi: 10.1101/2021.03.24.436779
Sasaoka, K., and Kito, M. (1964). Synthesis of theanine by tea seedling homogenate. Agric Biol Chem. 28, 313–317. doi: 10.1080/00021369.1964.10858238
Sharma, E., Joshi, R., and Gulati, A. (2018). L-Theanine: an astounding sui generis integrant in tea. Food Chem. 242, 601–610. doi: 10.1016/j.foodchem.2017.09.046
Silveira, J. A. G., de Almeida Viégas, R., da Rocha, I. M. A., de Oliveira Monteiro-Moreira, A. C., de Azevedo Moreira, R., and Oliveira, J. T. A. (2003). Proline accumulation and glutamine synthetase activity are increased by salt-induced proteolysis in cashew leaves. J. Plant Physiol. 160, 115–123. doi: 10.1078/0176-1617-00890
Su, T., Wang, P., Li, H., Zhao, Y., Lu, Y., Dai, P., et al. (2018). The Arabidopsis catalase triple mutant reveals important roles of catalases and peroxisome-derived signaling in plant development. J. Integr. Plant Biol. 60, 65–81. doi: 10.1111/jipb.12649
Szabados, L., and Savouré, A. (2010). Proline: a multifunctional amino acid. Trends Plant Sci. 15, 89–97. doi: 10.1016/j.tplants.2009.11.009
Takeo, T. (1974). L-alanine as a precursor of ethylamine in Camellia sinensis. Phytochemistry 13, 1401–1406. doi: 10.1016/0031-9422(74)80299-2
Takeo, T. (1979). Formation of amino acids induced by ammonia application and seasonal level fluctuation of amino acids contents in tea plant. Chagyo Kenkyu 56:70.
Tseng, M. J., Liu, C. W., and Yiu, J. C. (2007). Enhanced tolerance to sulfur dioxide and salt stress of transgenic Chinese cabbage plants expressing both superoxide dismutase and catalase in chloroplasts. Plant Physiol. Biochem. 45, 822–833. doi: 10.1016/j.plaphy.2007.07.011
Tsushida, T., and Takeo, T. (1984). Ethylamine content of fresh tea shoots and made tea determined by high performance liquid chromatography. J. Agric. Food Chem. 35, 77–83. doi: 10.1002/jsfa.2740350113
Upadhyaya, H., and Panda, S. K. (2013). Abiotic stress response in tea [Camellia sinensis L (O) Kuntze]: an overview. Rev. Agr. Sci. 1, 1–10. doi: 10.7831/ras.1.1
Wang, R., Chen, S., Deng, L., Fritz, E., Hüttermann, A., and Polle, A. (2007). Leaf photosynthesis, fluorescence response to salinity and the relevance to chloroplast salt compartmentation and anti-oxidative stress in two poplars. Trees 21, 581–591. doi: 10.1007/s00468-007-0154-y
Wang, W., Xin, H., Wang, M., Ma, Q., Wang, L., Kaleri, N. A., et al. (2016). Transcriptomic analysis reveals the molecular mechanisms of drought-stress-induced decreases in Camellia sinensis leaf quality. Front. Plant Sci. 7:385. doi: 10.3389/fpls.2016.00385
Wei, C., Yang, H., Wang, S., Zhao, J., Liu, C., Gao, L., et al. (2018). Draft genome sequence of Camellia sinensis var. sinensis provides insights into the evolution of the tea genome and tea quality. Proc. Natl. Acad. Sci. U. S. A. 115, E4151–E4158. doi: 10.1073/pnas.1719622115
Williams, J., Sergi, D., McKune, A. J., Georgousopoulou, E. N., Mellor, D. D., and Naumovski, N. (2019). The beneficial health effects of green tea amino acid L-theanine in animal models: promises and prospects for human trials. Phytother. Res. 33, 571–583. doi: 10.1002/ptr.6277
Wu, X., Jia, Q., Ji, S., Gong, B., Li, J., Lv, G., et al. (2020). Gamma-aminobutyric acid (GABA) alleviates salt damage in tomato by modulating Na+ uptake, the GAD gene, amino acid synthesis and reactive oxygen species metabolism. BMC Plant Biol. 20:465. doi: 10.1186/s12870-020-02669-w
Xia, E. H., Li, F. D., Tong, W., Li, P. H., Wu, Q., Zhao, H. J., et al. (2019). Tea plant information archive: a comprehensive genomics and bioinformatics platform for tea plant. Plant Biotechnol. J. 17, 1938–1953. doi: 10.1111/pbi.13111
Xia, J., Li, Y., and Zou, D. (2004). Effects of salinity stress on PSII in Ulva lactuca as probed by chlorophyll fluorescence measurements. Aquat. Bot. 80, 129–137. doi: 10.1016/j.aquabot.2004.07.006
Xie, Y., Ling, T., Han, Y., Liu, K., Zheng, Q., Huang, L., et al. (2008). Carbon monoxide enhances salt tolerance by nitric oxide-mediated maintenance of ion homeostasis and up-regulation of antioxidant defence in wheat seedling roots. Plant Cell Environ. 31, 1864–1881. doi: 10.1111/j.1365-3040.2008.01888.x
Xie, Y., Mao, Y., Lai, D., Wei, Z., and Shen, W. (2012). H2 enhances Arabidopsis salt tolerance by manipulating ZAT10/12-mediated antioxidant defence and controlling sodium exclusion. PLoS One 7:e49800. doi: 10.1371/journal.pone.0049800
Yang, H. (2014). Effects of amino acid on seed germination and seedling growth of buckwheat under salt stress. Guizhou Agr. Sci. 42, 30–33.
Yang, T., Li, H., Tai, Y., Dong, C., Cheng, X., Xia, E., et al. (2020). Transcriptional regulation of amino acid metabolism in response to nitrogen deficiency and nitrogen forms in tea plant root (Camellia sinensis L.). Sci. Rep. 10:6868. doi: 10.1038/s41598-020-63835-6
Yang, T., Xie, Y., Lu, X., Yan, X., Wang, Y., Ma, J., et al. (2021). Shading promoted theanine biosynthesis in the roots and allocation in the shoots of the tea plant (Camellia sinensis L.) cultivar Shuchazao. J. Agric. Food Chem. 69, 4795–4803. doi: 10.1021/acs.jafc.1c00641
Zeng, L., Lin, L., Peng, Y., Yuan, D., Zhang, S., Gong, Z., et al. (2020). L-theanine attenuates liver aging by inhibiting advanced glycation end products in D-galactose-induced rats and reversing an imbalance of oxidative stress and inflammation. Exp. Gerontol. 131:110823. doi: 10.1016/j.exger.2019.110823
Keywords: Camellia sinensis L., salt stress, theanine biosynthesis, ROS scavenging enzymes, catalases
Citation: Chen Z, Lin S, Li J, Chen T, Gu Q, Yang T and Zhang Z (2021) Theanine Improves Salt Stress Tolerance via Modulating Redox Homeostasis in Tea Plants (Camellia sinensis L.). Front. Plant Sci. 12:770398. doi: 10.3389/fpls.2021.770398
Received: 03 September 2021; Accepted: 27 September 2021;
Published: 15 October 2021.
Edited by:
Deyu Xie, North Carolina State University, United StatesReviewed by:
Jay Prakash Awasthi, Assam University, IndiaMingjie Chen, Xinyang Normal University, China
Copyright © 2021 Chen, Lin, Li, Chen, Gu, Yang and Zhang. This is an open-access article distributed under the terms of the Creative Commons Attribution License (CC BY). The use, distribution or reproduction in other forums is permitted, provided the original author(s) and the copyright owner(s) are credited and that the original publication in this journal is cited, in accordance with accepted academic practice. No use, distribution or reproduction is permitted which does not comply with these terms.
*Correspondence: Zhaoliang Zhang, emhsemhhbmdAYWhhdS5lZHUuY24=
†These authors have contributed equally to this work