- 1University of Angers, Institut Agro, INRAE, IRHS, SFR QUASAV, Angers, France
- 2Université Paris-Saclay, INRAE, AgroParisTech, Institut Jean-Pierre Bourgin (IJPB), Versailles, France
Shoot branching is highly dependent on environmental factors. While many species show some light dependence for branching, the rosebush shows a strict requirement for light to allow branching, making this species an excellent model to further understand how light impinges on branching. Here, in the first part, we provide a review of the current understanding of how light may modulate the complex regulatory network of endogenous factors like hormones (SL, IAA, CK, GA, and ABA), nutrients (sugar and nitrogen), and ROS to control branching. We review the regulatory contribution of microRNAs (miRNAs) to branching in different species, highlighting the action of such evolutionarily conserved factors. We underline some possible pathways by which light may modulate miRNA-dependent regulation of branching. In the second part, we exploit the strict light dependence of rosebush for branching to identify putative miRNAs that could contribute to the photocontrol of branching. For this, we first performed a profiling of the miRNAs expressed in early light-induced rosebush buds and next tested whether they were predicted to target recognized regulators of branching. Thus, we identified seven miRNAs (miR156, miR159, miR164, miR166, miR399, miR477, and miR8175) that could target nine genes (CKX1/6, EXPA3, MAX4, CYCD3;1, SUSY, 6PFK, APX1, and RBOHB1). Because these genes are affecting branching through different hormonal or metabolic pathways and because expression of some of these genes is photoregulated, our bioinformatic analysis suggests that miRNAs may trigger a rearrangement of the regulatory network to modulate branching in response to light environment.
Introduction
Important agronomic traits such as yield, visual and sanitary qualities, harvest index and even organoleptic quality are determined by plant architecture in general and shoot branching in particular (Boumaza et al., 2010; Garbez et al., 2015; Zhu and Wagner, 2020). A lot of research efforts are therefore produced to decipher mechanisms that control branching (Rameau et al., 2015; Roman et al., 2016; Le Moigne et al., 2018; Schneider et al., 2019; Wang M. et al., 2020; Barbier et al., 2021).
Branching relies on the ability of an axillary bud, a structure containing a miniature shoot comprising a meristem, short internodes and immature leaves, to break dormancy and to grow through cell proliferation and expansion into a new branch. Dormancy is complex and includes endo-, para-, and eco-dormancies that can in part overlap (Lang et al., 1987; Cline, 1997). While endodormancy is controlled by mechanisms endogenous to the bud, paradormancy is due to the control of other organs on a given bud, as it is the case in apical dominance. Apical dominance can be lifted by stem beheading (Dun et al., 2006) or by exogenous application of chemical products (Ophir et al., 2009; Suttle, 2009; Walton et al., 2009). Ecodormancy relies on environmental control over a bud. Bud ability to grow out is therefore controlled both by internal factors [i.e., genetic background, hormones, metabolites, reactive oxygen species (ROS)] (Girault et al., 2010; Mason et al., 2014; Barbier et al., 2015; Li-Marchetti et al., 2015; Porcher et al., 2021) and also external ones. Among them, nutrients and water availability (Demotes-Mainard et al., 2013), temperature (Djennane et al., 2014), and light (Djennane et al., 2014; Roman et al., 2016; Porcher et al., 2021) are major determinants. Responses to abiotic factors are indeed critical for the plant to adapt its own development to resource availability, seasons and environmental stresses and to compete with other plants.
Light signal is particularly informative for the plant since it varies in intensity, quality, direction, and duration (Leduc et al., 2014). The shade avoidance syndrome triggered by decreased photosynthetic radiations and R/FR (Red/Far Red) ratio leads for example to axillary bud outgrowth inhibition. Dependence on light for axillary bud growth is varying from one species to another. Some species are able to show axillary bud growth in darkness where in the rosebush, light is particularly essential for this. Indeed, in this species axillary bud outgrowth and organogenesis are totally inhibited and no axillary branches are produced in the absence of light (Girault et al., 2008). Beside rosebush’s primary importance among ornamentals, this response has also made it an excellent model for exploring light control of axillary bud outgrowth in plants (Demotes-Mainard et al., 2016; Huché-Thélier et al., 2016).
At the molecular level, most knowledge on axillary bud outgrowth and Its photocontrol has been gained at the transcriptional level in the rosebush (Girault et al., 2010; Djennane et al., 2014; Roman et al., 2016; Schneider et al., 2019; Porcher et al., 2020, 2021). Yet, as for other developmental processes, post-transcriptional regulations are likely important and need to be further explored. Some studies have revealed post-transcriptional regulation in axillary bud outgrowth control with post-transcriptional regulation of Rosa BRANCHED1 (RhBRC1) in interaction with sugars and regulation of its 3′UTR region with the potential role of PUMILIO RNA-BINDING PROTEIN FAMILY 4 (RhPUF4) (Wang et al., 2019b).
MicroRNA (miRNA) regulation is a major component of post-transcriptional regulation of all biological processes in eukaryotes. First discovered in Caenorhabditis elegans (Wightman et al., 1993), miRNAs are small (20–21 nucleotides) single stranded non-coding RNAs. In Arabidopsis thaliana, 728 mature miRNAs have been identified (Kozomara et al., 2019). MiRNAs modulate the expression of their target genes through binding to their mRNA, causing either transcript cleavage or translation inhibition (Voinnet, 2009). Some miRNAs and their targets show a high conservation within plants while non-conserved ones can be found only in a group of plants, a species or even be specific to a landrace (Rajagopalan et al., 2006; Fahlgren et al., 2007). Conserved miRNAs target preferentially genes coding for transcription factors that play important roles in developmental control, while non-conserved target genes code for much more diverse functions (Zhang and Zhang, 2012).
Some knowledge has been gained on the roles of miRNAs in meristem initiation (see reviews by Zhang et al., 2006; Wang et al., 2011; Wu, 2013; Li and Zhang, 2016; Liu et al., 2018) as well as on leaf organogenesis (Pulido and Laufs, 2010; Maugarny-Calès and Laufs, 2018; Yang et al., 2018), two processes that contribute to axillary bud initiation and development (Sussex and Kerk, 2001). However, little is known about the roles of miRNAs during axillary bud outgrowth per se. Here, we provide a survey of the current knowledge on this subject, first sketching how the main mechanisms regulating axillary bud outgrowth may be connected to light control. Next, we provide a comprehensive view of axillary bud outgrowth regulation by miRNAs and, exploring the wider literature, we provide hypotheses on how light controls miRNAs activity and may contribute to the photocontrol of axillary bud outgrowth. In a second part, based on our expertise in rosebush and using in silico analysis based on the miRNA profiling we performed, we further explored the rose genome to identify and discuss novel miRNAs-gene target couples that may play a potential role in the regulation of axillary bud outgrowth and in its photocontrol in rosebush. Thus, we provide novel hypotheses on the miRNA-mediated regulation of bud growth photocontrol on which future research may stand.
Axillary Bud Outgrowth and its Light Control: Current Knowledge on Main Actors
Several reviews have recently described in depth the current knowledge on the processes controlling axillary bud outgrowth in plants and their control by light (Leduc et al., 2014; Schneider et al., 2019; Wang M. et al., 2020; Kotov et al., 2021). As a pre-requisite to discuss their possible regulation by miRNAs, we provide here a brief overview of the main actors involved in the photocontrol of axillary bud outgrowth with a particular focus on rosebush. Main actors and their interactions are presented in Figure 1.
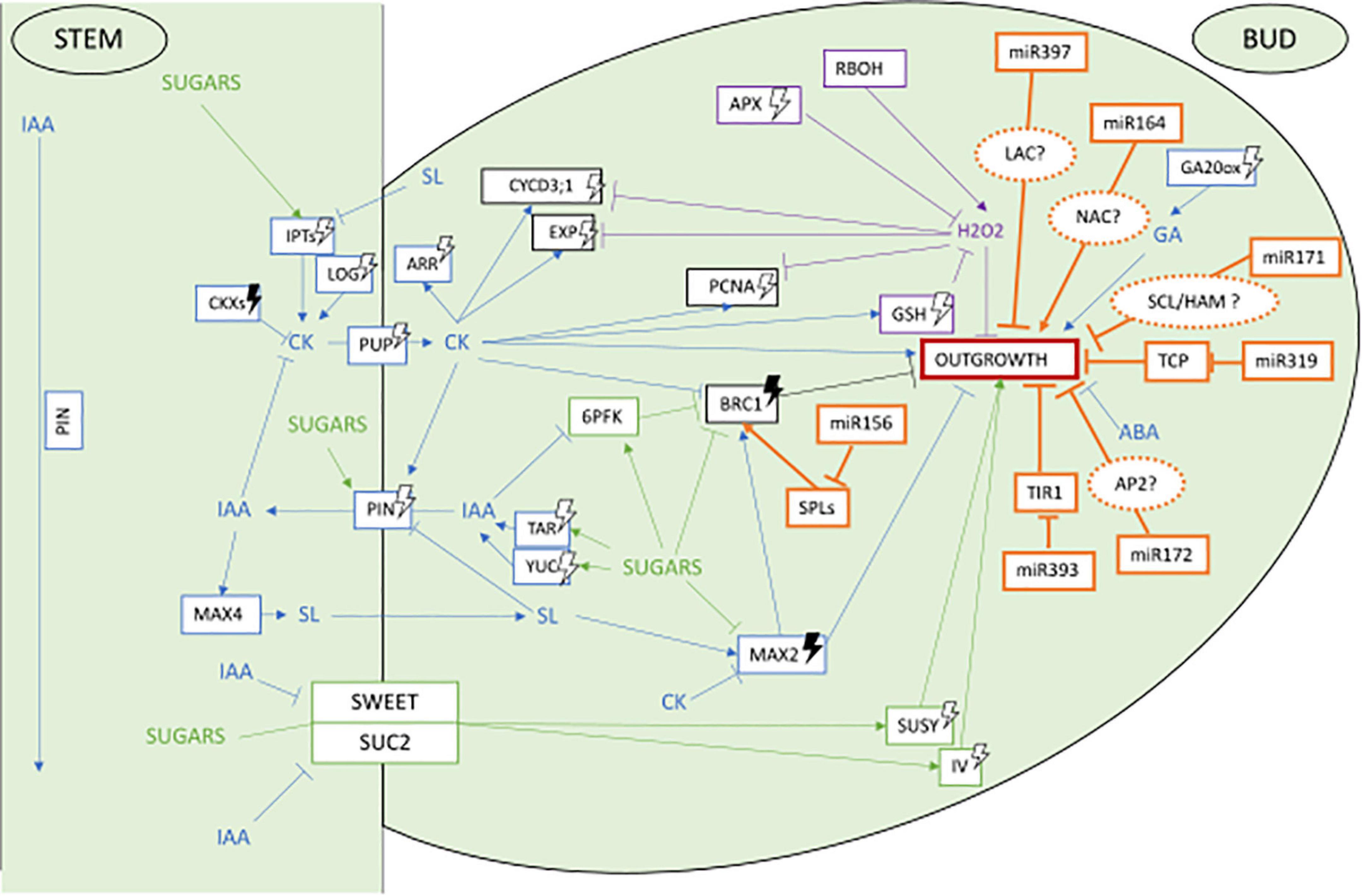
Figure 1. Network of main actors controlling axillary bud outgrowth in the rosebush Rosa ‘Radrazz’* and the potential targets of miRNAs based on literature in other plant species** Potential gene targets of these miRNAs in the control of bud outgrowth are represented in dotted orange circles. miRNAs involved in control of bud outgrowth according to the literature are represented in orange. Blue represents hormonal pathway, green sugars pathway, purple ROS pathway. Arrows head means induction, straight lines mean repression, white lightning bolt means induction by light, darked lightning bolts means induction by darkness. *Girault et al. (2008), Girault et al. (2010), Henry et al. (2011), Choubane et al. (2012), Rabot et al. (2012), Rabot et al. (2014), Djennane et al. (2014), Barbier et al. (2015), Roman et al. (2016), Roman et al. (2017), Porcher et al. (2020), Porcher et al. (2021), Wang M. et al. (2021). **Jiao et al. (2010), Miura et al. (2010), Wang et al. (2010), Wei et al. (2012), Xia et al. (2012), Curaba et al. (2013), Zhou et al. (2013), Zhang et al. (2013), Aung et al. (2015), Wang L. et al. (2015), Wang Y. et al. (2015), Huang et al. (2017), Kravchik et al. (2019), Sun et al. (2019), Cui et al. (2020), Lian et al. (2021), Wang R. et al. (2021), Zhan et al. (2021).
Main Actors and Their Roles in the Control of Axillary Bud Outgrowth
Axillary bud outgrowth is controlled by a complex interplay between several main actors such as hormones, nutrients in particular sugars and ROS (Figure 1).
Concerning hormones, strong interactions between auxin (IAA), cytokinins (CK), and strigolactones (SL) form a core regulatory hormonal network instrumental for the release of apical dominance and induction of axillary bud outgrowth (Domagalska and Leyser, 2011; Barbier et al., 2019, Figure 1, blue lines). A first proposed mechanism suggests IAA inhibits axillary bud outgrowth indirectly through repression of CK synthesis and promotion of SL synthesis and signaling, both hormones being able to enter axillary buds and to act directly on axillary buds (Beveridge et al., 2000; Brewer et al., 2009; Ferguson and Beveridge, 2009). The balance of their opposing effects (CK promoting and SL repressing bud growth) would determine the fate (paradormancy vs. outgrowth) of the axillary bud. Through a second suggested mechanism, the basipetal flux of IAA produced by the apical meristem would also cause inhibition of axillary bud outgrowth by acting on the polarized distribution of the IAA efflux transporters PIN-FORMED (PIN) proteins in the axillary bud and by preventing it to export its own auxin (Bennett et al., 2006; Prusinkiewicz et al., 2009). In addition, a repressive role of ABA on axillary bud outgrowth was demonstrated in several species such as tomato, bean, arabidopsis, and rosebush (Tucker, 1976; Knox and Wareing, 1984; Gocal et al., 1991; Le Bris et al., 1999; Chatfield et al., 2000; Corot et al., 2017). In rosebush, a continued de novo synthesis of ABA contributes to maintaining bud endodormancy through control of cell cycle arrest in G2 phase (Le Bris et al., 1999). Application of ABA on the stem of rosebush and of other species also inhibits outgrowth of non-endodormant axillary buds nearby (Chatfield et al., 2000; Cline and Oh, 2006; Corot et al., 2017) while beheading of the main shoot causes a decrease in ABA contents in axillary buds along with their outgrowth (Knox and Wareing, 1984; Gocal et al., 1991). Last, gibberellins (GA) are hormones that control meristem cell differentiation in leaf primordia and internode elongation, both processes taking place during axillary bud outgrowth (Koornneef and van der Veen, 1980; Dodsworth, 2009). In rosebush, GA synthesis under the control of RoGA20-oxidase (RoGA20ox) and RoGA3ox and reduction of GA catabolism through down-regulation of RoGA2ox were shown to contribute to axillary bud outgrowth (Choubane et al., 2012).
As providers of energy, osmolytes and cell wall material, sugars are other key players in axillary bud outgrowth control (Bonicel et al., 1987; Marquat et al., 1999; Le Hir et al., 2006, Figure 1, green lines). In the rosebush, pea and sorgho, defoliation, decapitation or in vitro experiments showed axillary bud outgrowth is dependent on sucrose availability (Girault et al., 2010; Henry et al., 2011; Kebrom et al., 2012; Rabot et al., 2012; Mason et al., 2014). In rosebush Rosa ‘Radrazz’, SUCROSE TRANSPORTER 2 (RhSUC2) and RhSWEET10 control sucrose transport to the bud (Henry et al., 2011; Roman et al., 2016) where RhSUSY and VACUOLAR INVERTASE 1 (RhVI1) catabolize sucrose into fructose and glucose for growth (Girault et al., 2010; Rabot et al., 2014). Promoting effects of non-metabolizable sucrose analogs on axillary bud outgrowth in rosebush suggests that sucrose may also play a signaling role during axillary bud outgrowth (Rabot et al., 2012; Barbier et al., 2015). Sucrose interacts with the hormonal control of axillary bud outgrowth (Barbier et al., 2015). Using detached rosebush buds in vitro, Barbier et al. (2015) showed that sucrose upregulates auxin synthesis [TRYPTOPHAN AMINOTRANSFERASE RELATED 1 (RhTAR1), YUCCA 1 (RhYUC1)] and auxin efflux [PIN-FORMED 1 (RhPIN1), SERINE/THREONINE PROTEIN PHOSPHATASE 2A (RhPP2A), PINOID (RhPID)] genes in buds and represses strigolactones MORE AXILLARY BRANCHES 2 (RwMAX2) signaling gene. Promoting effect of sucrose onto axillary bud outgrowth also relies on repression of a main negative regulator of axillary bud outgrowth BRANCHED1 (BRC1) (Aguilar-Martínez et al., 2007; Finlayson et al., 2010; Dun et al., 2012; Brewer, 2015; Wang et al., 2019a) by sucrose (Barbier et al., 2015; Wang M. et al., 2021).
Evidence is also repetitively brought showing that the oxidative metabolism contributes to the control of axillary bud outgrowth (Figure 1, purple lines). In grape, for example, application of sodium azide or hydrogen cyanamide or heat shock on endodormant buds represses CATALASE (CAT) scavenging activity, causing accumulation of hydrogen peroxide (H2O2) that promotes bud outgrowth (Or et al., 2002; Ophir et al., 2009; Pérez et al., 2009; Vergara et al., 2012; Sudawan et al., 2016; Meitha et al., 2018). In non-endodormant buds, H2O2 appears to play an opposite role. Hence, in tomato, rboh mutants in which the capacity to produce apoplastic H2O2 is altered, a highly branched phenotype is observed suggesting that H2O2 contributes to axillary bud outgrowth inhibition (Sagi et al., 2004; Chen et al., 2016). In non-endodormant rosebush axillary buds subjected to apical dominance, a high content of H2O2 is present and contributes to bud arrest. Upon stem decapitation, activation of ROS scavenging activity is observed that causes a rapid decrease in H2O2 content along with axillary bud outgrowth (Porcher et al., 2020). In rosebush buds, scavenging activity is mainly due to the ascorbate–glutathione cycle (AsA–GSH). Transcription of glutathione biosynthesis genes RhGSH1 as RhGSH2 as well as of ascorbate peroxidase RhAPX1 and glutathione reductase RhGR1 are indeed actively transcribed in axillary buds after plant beheading and during axillary bud outgrowth while catalase RhCAT is not. These up-regulations are followed by increased corresponding enzymatic activities (Porcher et al., 2020).
Suppression of bud dormancies allows axillary bud outgrowth characterized by resumption of DNA replication and cell cycle (Shimizu and Mori, 1998; González-Grandío et al., 2013). Increased transcriptional activity of PROLIFERATING CELL NUCLEAR ANTIGEN (PCNA), CELL DIVISION CYCLE 2 (CDC2), CYCLIN B (CYCB, Devitt and Stafstrom, 1995), and CYCLIN D3 (CYCD3) takes for example rapidly place in pea (Shimizu and Mori, 1998), apple trees (Li et al., 2018) and in Rosa buds (Roman et al., 2016; Porcher et al., 2020) upon dormancy break. Cell division along with cell wall expansion contribute to growth of organ primordia and lead to protrusion of young leaves out of buds scales. In rosebush, up-regulation of EXPANSINS RhEXPA1, 2, and 3 take place in axillary buds within a few hours after stem decapitation and is promoted by CK (Roman et al., 2016, 2017).
Light Regulation of Main Actors of Axillary Bud Outgrowth
Spectral quality and light intensity both impact axillary bud outgrowth in a range of herbaceous and perennial species (Leduc et al., 2014; Demotes-Mainard et al., 2016; Huché-Thélier et al., 2016). Unlike other species such as Arabidopsis, tomato, and poplar (Girault et al., 2008), axillary buds of rosebush have an absolute need for light to grow out (Girault et al., 2008). Total inhibition of axillary bud outgrowth under darkness and promotion by light offers a great opportunity to examine the light control of bud outgrowth in this species and to deepen our knowledge of this ecodormancy in plants (Leduc et al., 2014; Demotes-Mainard et al., 2016; Huché-Thélier et al., 2016; Bertheloot et al., 2020).
In the rosebush, red (R) and blue (B) lights promote axillary bud outgrowth while far-red (FR) light is inhibitory (Girault et al., 2008). These responses are consistent with the shade avoidance syndrome of plants. In rosebush, monochromatic blue or red light alone is also able to trigger and sustain all processes involved in axillary bud outgrowth, suggesting cross links and redundancy in the transduction pathways of R and B lights (Abidi et al., 2013). The acrotonic outgrowth pattern observed in rosebush can also be deeply modified by localized dark treatment along the rosebush stem (Djennane et al., 2014) or temporary exposure to low light prior to full light exposure (Demotes-Mainard et al., 2013), indicating fine spatial regulation of axillary bud outgrowth patterns along the stem by light.
Transcriptional control of light over hormones, sugars, ROS, cell division and growth was demonstrated in the rosebush using contrasted lighting conditions (darkness vs. white light, monochromatic vs. white light) and exogenous hormonal and sugar treatments. From these studies, demonstration was made that CK are initial targets of the light control pathway (Roman et al., 2016). More precisely, bud exposure to light triggers a rapid and strong upregulation of genes involved in CK synthesis [ISOPENTENYLTRANSFERASE (RhIPT3, RhIPT5)], activation [LONELY GUY 8 (RhLOG8)], and transport [PURINE PERMEASE 5 (RhPUP5)] and to the repression of CK catabolism gene CYTOKININ OXIDASE/DEHYDROGENASE 1 (RhCKX1). This leads to CK accumulation in nodes and buds which then causes an up-regulation of IAA biosynthesis and transport (RhYUC1, RhPIN1), sugar (RhSUSY, RhVI, RhSUC2, and RhSWEET10) synthesis and transport genes, and repression of axillary bud outgrowth inhibitory genes (RhBRC1 and SL signaling RwMAX2). CK upregulation during light control of rosebush axillary bud outgrowth also acts through ROS scavenging (Porcher et al., 2021). In particular, the AsA-GSH pathway is activated by light and CK, causing a decrease in H2O2 content in bud (Porcher et al., 2021). These light regulations finally lead to axillary bud outgrowth through activation of cell cycle RhPCNA, and cell expansion RhEXPA genes (Roman et al., 2016, 2017). Additionally, in response to reduced light intensity, Corot et al. (2017) showed that inhibition of axillary bud outgrowth in the rosebush is associated with reduced levels of CK and increased levels of ABA. Exogenous delivery of CK and ABA to the stem confirmed their antagonistic action in the control of axillary bud outgrowth by light intensity (Corot et al., 2017). Light regulation of GA also contributes to the photocontrol of axillary bud outgrowth in the rosebush through upregulation of the expressions of biosynthesis genes GA20ox and GA3ox and repression of catabolic gene GA2ox (Choubane et al., 2012).
In dark, conversely, repression of axillary bud outgrowth results from up-regulation of repressor genes such as RhBRC1 and SL signaling RwMAX2 as well as repression of ROS scavenging AsA-GSH pathway (Djennane et al., 2014; Roman et al., 2016; Porcher et al., 2021). Repression of sucrose transport and catabolism under darkness leads to a switch toward sorbitol metabolism, seemingly as a survival mechanism. Strong upregulation of NAD-DEPENDANT SORBITOL DEHYDROGENASE (RhNAD-SDH) transcriptional activity is indeed observed upon exposure of buds to darkness (Girault et al., 2010).
Extending Our Knowledge of Conserved miRNAs and Their Regulation of Axillary Bud Outgrowth to Rosebush
Beside the regulatory pathways discussed above, the role of miRNAs in bud outgrowth control was more recently discovered. Numerous miRNAs showing high degree of similarity in their sequence were found in the genomes of many plant species and thus defined as conserved miRNAs (Weber, 2004; Zhang et al., 2006). Some of these were identified as key players in axillary bud outgrowth. Yet, the role of these miRNAs has been explored only in a few model species such as Arabidopsis, maize, and rice, the two latter concentrating on a grass specific branching process, called tillering. Here, we provide an up-to-date summary of roles of conserved miRNAs in branching and provide new data of the putative role of these conserved miRNAs in rosebush. Branching phenotypes of miRNAs and target gene-resistant mutants are summarized in Table 1.
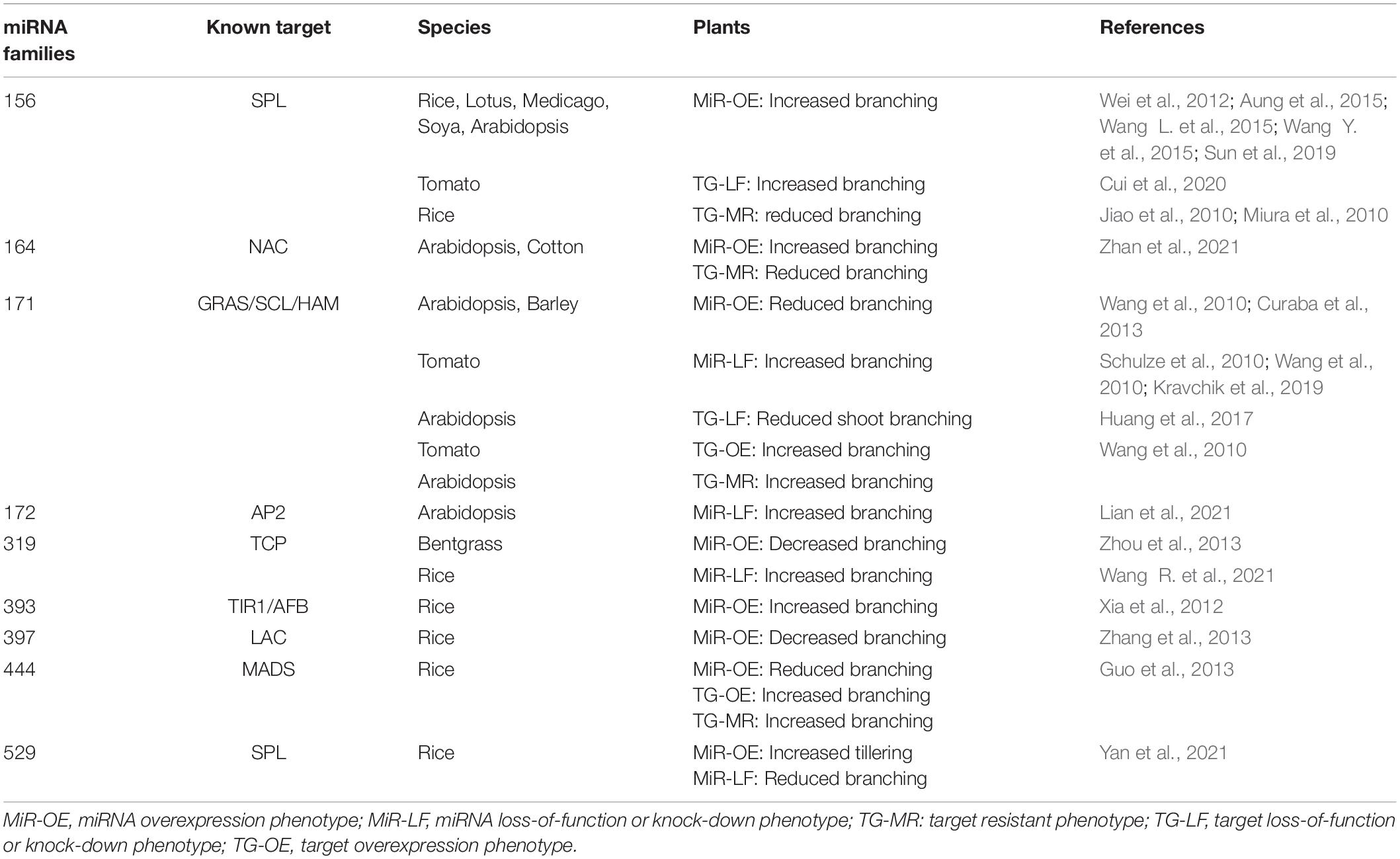
Table 1. Main conserved miRNA families and their associated targets causing shoot branching modulation.
The study of tillering in rice (Oryza sativa) has been instrumental in uncovering the role of miRNAs in bud outgrowth. To-date, miR156 is one of the best characterized miRNA families. MiR156 plays a major role in regulating shoot branching by targeting a subset of the SQUAMOSA PROTEIN-LIKE (SPLs) genes. SPL transcription factors are found in all green plants and are involved in many developmental regulatory processes such as phase transition and flowering, plastochron control, leaf development, fruit ripening and response to stresses (Wang and Wang, 2015). SPL genes are ordered into nine clades, of which six are regulated by miR156 (Preston and Hileman, 2013). In rice, increased expression level of OsSPL14 resulting from a mutation in its miR156 binding site leads to reduced branching from vegetative buds (Jiao et al., 2010; Miura et al., 2010). Interestingly, this mutation has opposite effects on branching during the reproductive phase, as it leads to increased inflorescence (panicle) branching and altogether forms the molecular basis of a quantitative locus known as WEALTHY FARMER’S PANICLE or IDEAL PLANT ARCHITECTURE 1 (IPA1) that improves rice productivity. On the contrary, high miR156 levels increase tiller formation in the dominant Corngrass1 maize mutant (Chuck et al., 2007). An increased branching resulting from miR156 overexpression was also reported in other species including Arabidopsis (Wei et al., 2012; Tian et al., 2014), alfalfa (Aung et al., 2015), lotus (Wang Y. et al., 2015), soya (Sun et al., 2019), and tomato. In this last species, the increased branching phenotype can be reverted by expression of a miR156-resistant SPL13 gene (Cui et al., 2020). Modulation of miR156/SPL interaction affects branching through both initiation and outgrowth of the axillary buds (Wang L. et al., 2015). At least three pathways have been associated with such increased branching. First, UNBRANCHED3, the maize ortholog of OsSPL14, directly targets and represses the expression of cytokinin biosynthesis and signaling genes while promoting expression of cytokinin degradation genes, thus resulting in lower cytokinin levels and signaling (Du et al., 2017). Second, OsSPL7, another target of miR156 in rice, represses the expression of OsGH3.8 which codes for an enzyme conjugating auxin to aspartate (Dai Z. et al., 2018). Hence, miR156 may affect auxin/cytokinin balance that is important for both formation and growth of the axillary meristems. Last, OsSPL14 directly promotes the expression of OsTB1, the rice homolog of bud outgrowth repressor AtBRC1 (Lu et al., 2013). In this last case, miR156 repression of OsSPL14 would reduce AtBRC1 inhibitory effect on bud outgrowth.
The study of tillering in rice has revealed additional miRNAs that contribute to the regulation of branching in this species (Yue et al., 2017). MiR529 that partially overlaps with miR156 also targets a subset of SPL genes. MiR529 is found in monocots but was specifically lost in some dicots (Morea et al., 2016). Recently, Yan et al. (2021) showed that modulation of miR529 activity increases rice tillering in a similar way as miR156.
Increased tillering was also observed in rice lines overexpressing miR393. This miRNA targets genes coding for auxin receptors [TRANSPORT INHIBITOR RESPONSE 1 (OsTIR1) and AUXIN SIGNALING F-BOX 2 (OsAFB2)]. Accordingly, rice lines overexpressing miR393 show a reduced response to auxin and lower expression of the OsTB1 gene, which may account for increased axillary bud growth (Li et al., 2016).
Modified auxin signaling is also observed in rice plants expressing a miR160-resistant version of the AUXIN RESPONSE FACTOR (OsARF18) that develop less tillers (Huang et al., 2016).
While overexpression of miR156, miR529 or miR393 increases rice tillering, overexpression of the monocot-specific miR444 has an opposite effect (Guo et al., 2013). miR444 targets OsMADS57 which is expressed in developing buds where OsMADS57 represses expression of D14 gene coding for the SL receptor. Hence, miR444 overexpression may lead to increased SL response and thus inhibition of bud outgrowth.
Finally, overexpression of miR397 that targets rice OsLAC, a laccase polymerizing monolignols into lignin (Berthet et al., 2011; Zhao et al., 2013) also leads to a slight reduction in rice tiller number (Zhang et al., 2013).
Study in Arabidopsis thaliana also revealed the potential role of three other miRNAs in the control of branching. For example, miR171 was shown to target genes coding for the GRAS transcription factors SCARECROW-LIKE SCL6-II, SCL6-III, and SCL6-IV [also named LOST MERISTEMS1-3 (LOM) or HAIRY MERISTEM (HAM)] (Schulze et al., 2010; Wang et al., 2010). These proteins are required for proper meristem function, including axillary meristem formation, and act by promoting the specification of the stem cells in the apical region of the meristem (Zhou et al., 2018; Han et al., 2020). Arabidopsis lines overexpressing miR171 show a massive reduction in the number of secondary branches, which is reverted by the expression of a miR171-resistant version of any of the 3 HAM genes (Wang et al., 2010). In tomato also, reduced miR171 activity or overexpression of the tomato HAM gene SlGRAS24 leads to increased branching suggesting that miR171 represses HAM gene expression to limit branching in these species (Huang et al., 2017; Kravchik et al., 2019). SlGRAS24 modulates auxin and gibberellin signaling, but whether it affects the formation of the axillary meristem, or its outgrowth is not known yet (Huang et al., 2017). The function of miR171 in shoot branching inhibition appears to be conserved in monocots, as overexpression of this miRNA reduces branching in barley (Curaba et al., 2013).
In Arabidopsis, miR164 targets a subset of the NAC genes, including CUP-SHAPED COTYLEDON (CUC) genes that are important for a wide range of meristem-related developmental processes. Recent studies performed in cotton and in the heterologous Arabidopsis system suggest that repression of CUC2 by miR164 is important for branch outgrowth (Zhan et al., 2021). Specifically, CUC2 interaction with BRC1 may modulate ABA levels to control bud growth (Zhan et al., 2021).
An antagonistic role of miR172 and of miR156 in the regulation of Arabidopsis branching was also demonstrated (Wang et al., 2009). The quintuple mutant of the five MIR172 genes indeed shows an increased branching as does a line overexpressing MIR156b (Wei et al., 2012; Lian et al., 2021). MiR172a and d are the MIR172 genes playing a major role in the repression of branching in Arabidopsis (Lian et al., 2021).
Research in other less studied plant species has also brought additional knowledge on the role of other miRNAs in branching. For instance, in bentgrass (Agrostis stolonifera), overexpression of miR319 leads to a slight decrease in tillering (Zhou et al., 2013). MiR319 targets a subset of the TEOSINTE BRANCHED1/CYCLOIDEA/PCF (TCP) transcription factor family which are involved among others in the transition from cell proliferation to differentiation during plant development (Palatnik et al., 2003). In line with these data, recent work in rice shows that inactivation of miR319 correlates with increased expression of OsTCP21 and increased number and length of tiller buds (Wang R. et al., 2021). In Camellia sinensis, the expression of MIR319c is significantly reduced during bud outgrowth while that of its target TCP2 is significantly upregulated (Liu et al., 2019).
Altogether, it appears that several conserved miRNAs contribute to branching control. While in some cases, their effect could be precisely attributed to meristem initiation or to their later outgrowth (Wang L. et al., 2015), this has not been systematically analyzed (Huang et al., 2017; Lian et al., 2021). In addition, as some of these miRNA/target couples are involved in many processes, the effect on branching could be sometimes indirect. This may be the case for example for miR156 that affects the duration of the vegetative phase and therefore the number of nodes bearing axillary meristems.
As described in part 1, a lot of information has been gained on axillary bud outgrowth regulatory pathways and its photocontrol through study of the rosebush Rosa ‘Radrazz’. However, the role of miRNAs in rosebush axillary bud outgrowth has not yet been explored. As a first step toward this, we analyzed whether the conserved miRNAs described in Table 1 were also expressed in rosebush axillary buds and if they could interact with the same known targets. To answer these questions and since no annotation of the mature miRNA sequences is available on the reference Rosa genome (Rosa chinensis, Hibrand Saint-Oyant et al., 2018; Raymond et al., 2018), we performed a high throughput profiling of the miRNA components of Rosa ‘Radrazz’ axillary buds. To induce axillary bud outgrowth, stems were beheaded to release apical dominance and were grown in light. We collected buds samples right after beheading and 6 h after beheading. This time point was chosen as it was late enough after beheading to allow key molecular mechanisms of the outgrowth process and of the light control to take place (Barbier et al., 2015; Roman et al., 2016; Porcher et al., 2020, 2021; Method detailed in Supplementary Method I) and early enough to minimize feed-back loop regulations of miRNAs that could be activated following resumption of axillary meristem outgrowth. From the sequenced small RNAs, prediction and annotation of the Rosa mature miRNAs sequences were achieved using Arabidopsis thaliana miRNAs database1 (Supplementary Data 1). Annotated Rosa miRNAs sequences were next aligned against orthologs of the known target genes described in literature (Table 1) and found in Rosa chinensis genome using target prediction web server’s psRNAtarget (Dai X. et al., 2018).2 Together this allowed us to take a snapshot of miRNAs present in buds upon suppression of apical dominance and during an early phase of bud growth and then predict their targets in Rosa (Table 2).
Our bioinformatic analysis shows that out of the 9 miRNAs families previously described as involved in the control of bud outgrowth (as mentioned above), 7 were found in Rosa (Table 2). The two missing families, miR444 and mi529, are also missing in other dicots. This result reinforces the hypothesis that miR529 was lost in eudicots during evolution and that miR444 is only present in monocots (Sunkar et al., 2005; Ortiz-Morea et al., 2013). Each Rosa miRNA family is represented by several members (i.e., 14 in miR156 family; 7 in miR164 family). Similar numbers of members were found in Arabidopsis except for miR171, 172, and 319 families in which a much lower number of members were identified in Arabidopsis (6, 9, and 3 members, respectively) in comparison to Rosa. This may suggest differences in the tuning of the regulations controlled by these miRNA families between Rosa and Arabidopsis.
Binding ability of Rosa miRNAs to known targets was predicted by searching sequences complementary to the miRNAs in the putative targets. Same target gene families were found in the Rosa genome as described for other species (Table 1). These findings suggest that regulations involving these miRNA families and their associated targets may also be conserved in Rosa axillary buds.
Bioinformatic analysis allowed identification of several members of each target gene family in Rosa (Table 2). Further experimental investigations are needed for confirmation of their interaction.
Results are summed up in orange in Figure 1 showing the network of main actors controlling axillary bud outgrowth in the rosebush Rosa ‘Radrazz’ and the potential roles of miRNAs based on literature in other plant species and the miRNA/target pairs we identified here.
Crosstalk Between Light Signaling Pathway and miRNAs Encoding Gene Expression
Light is a major environmental cue that controls bud outgrowth in many plant species and light regulation of axillary bud outgrowth at transcriptional level has been well documented in rosebush (Girault et al., 2008; Rabot et al., 2014; Roman et al., 2016; Porcher et al., 2020). Yet, little is known to-date on the involvement of miRNAs in light-mediated axillary bud outgrowth mechanisms. Therefore, we summarize here the present knowledge on light effects on the miRNA pathway in general, affecting for instance the stability or functionality of the core proteins involved in the maturation of miRNAs (Sánchez-Retuerta et al., 2018; Park et al., 2021). In addition to such a general effect on the whole miRNA pathway, light also affects the expression of some MiRNA genes specifically. Red (R) and Blue (B) light signal transduction engages photoreceptors, including phytochromes (PHY) and cryptochromes (CRY) and their downstream effectors as ELONGATED HYPOCOTYL 5 (HY5) and PHYTOCHROME INTERACTING FACTORS (PIF4). PHYB plays a main role in the photocontrol of bud outgrowth (Kebrom and Mullet, 2016). Recently, Holalu et al. (2020) reported that PIF4 and PIF5 contribute to the suppression of branching resulting from phyB loss-of-function and a low R/FR ratio. This phenotype is correlated to BRC1 expression induction by PIF4/PIF5 and to abscisic acid (ABA) accumulation in axillary buds. Interestingly, several reports indicated that modulation of miRNA expression may contribute to the PHY-mediated light response, raising the hypothesis that this may contribute to branching modulation. For instance, analysis of miRNAs and their PHYB-mediated targets in rice leaves identified a total of 135 miRNAs differentially expressed between the WT and phyB mutant (Sun et al., 2015). This finding suggests that these miRNAs are directly or indirectly controlled by PHYB and participate in PHYB-mediated light signaling. In the same line, HY5 and PIFs transcription factors were reported to directly control the expression of several miRNA genes (Zhang et al., 2011; Xie et al., 2017). More recently, in Arabidopsis, PIF4 was reported to promote expression of genes encoding miR156/157, miR160, miR165/166, miR167, miR170/171, and miR394 and to reduce the expression of the genes encoding miR172 and miR319 by binding to the promoters of these miRNA genes (Sun et al., 2018). Compared to wild type, corresponding miRNA mutants had altered hypocotyl phenotypes, supporting a role for specific miRNAs in photomorphogenesis (Sun et al., 2018). In the extremophile plant Eutrema salsugineum under long-term action of R, Pashkovskiy et al. (2021) reported an increase in the expression of PHYA, PHYB, and PHYC as well as of PIF4 and PIF5 together with that of miR395, miR408 and miR165. In addition, they also observed a decrease in HY5, miR171, miR157, and miR827 expressions. These data suggest that the quantity of these miRNAs in E. salsugineum is light-regulated in a PHY and PIF-mediated manner. Also, in Solanum tuberosum leaves, R light can induce miR398, 399, 408, 482, 8036, and 8049 expressions (Qiao et al., 2017). Among miRNAs whose expression is regulated by light, some (miRNA156, 171, 172, and 319) are involved in branching control (Table 1). These observations thus indicate that light via a PHY-dependent pathway may control bud outgrowth through modifying the expression of several miRNAs.
A key event in bud outgrowth is the initiation and expansion of leaves. While no experimental data report direct interaction between light signaling and miRNAs biogenesis in the control of leaf organogenesis during bud outgrowth, some works demonstrate that light regulates leaf expansion (Romanowski et al., 2021), and this partly occurs through miRNAs regulation (Pashkovskiy et al., 2016). Hence, Pashkovskiy et al. (2016) reported that blue light causes a significant increase in miR167 expression which decreases the level of auxin-dependent ARF6 transcripts in Arabidopsis leaves, thereby allowing leaf expansion. ARF transcription factors play an important role in the auxin-mediated gene transcription pathway by interacting with Aux/IAAs proteins, a key pathway involved in bud outgrowth (Vanneste and Friml, 2009; Dierck et al., 2016). In the same line, blue light (B) specifically down-regulates miR156 and miR157 and upregulates their target genes SPL9 and SPL15 during Brassica rapa subsp. Rapa seedling development (Zhou et al., 2016). These results, combined to increase shoot branching phenotype caused by miR156 overexpression in Brassica napus (Wei et al., 2010), support the hypothesis of a crosstalk between light signaling and miRNAs regulation and suggest miR156/SPL couple acts as regulatory hub of bud outgrowth in response to light. More recently, Dong et al. (2020), found 20 miRNAs differentially expressed in tomato leaves after blue light treatment. Among them, sly-miR9472-3p expression is up-regulated by blue light treatment and is negatively correlated to the expression of its target gene IPT5, a cytokinin biosynthesis gene which plays a crucial role in promoting axillary bud outgrowth (Barbier et al., 2015; Roman et al., 2016). All these data suggest that miRNAs may well be involved in the light control of leaf expansion during bud outgrowth.
Bioinformatic Analysis of Putative New miRNAs in the Control and Photocontrol of Bud Outgrowth in Rosa
As described above, rosebush is a pertinent model to understand how light controls axillary bud outgrowth. Since little has been described on the role of miRNAs in the photocontrol of axillary bud outgrowth, we took advantage of the rose model to further examine whether components of the axillary bud outgrowth regulatory network (Figure 1) may be targets of post-transcriptional regulation via miRNAs.
To address this question, full length sequences of genes from this network were identified on Rosa chinensis genomes (Hibrand Saint-Oyant et al., 2018; Raymond et al., 2018) and confronted against the mature miRNAs sequences we identified in Rosa axillary bud from small RNA-seq using target prediction web server’s psRNAtarget (Dai X. et al., 2018; see text footnote 2) (Supplementary Table 1).
Our bioinformatic analysis reveals that 9 out of the sixty-five genes related to axillary bud outgrowth and its photocontrol in Rosa are predicted to be targeted by 7 miRNA families. These families are conserved in plants. Out of these 7 miRNAs, only miR156 and miR164 have already been described as involved in control of bud outgrowth (Table 1). On the contrary, five miRNAs (miR159, miR166, miR399, miR477, and miR8175) were not yet identified for a possible role in the control of bud outgrowth but were characterized for other functions. This could be either because their contribution to axillary bud outgrowth could be masked by the action of other more prominent miRNAs or, alternatively, because the strict light dependence of rosebush axillary bud outgrowth involves novel regulatory circuits that are less conserved in other species, and thus less characterized. MiR159 has been reported in growth transition, programmed cell death, and seed germination (Reyes and Chua, 2007; Alonso-Peral et al., 2010; Guo et al., 2017). MiR166 is implicated in different developmental processes such as regulation of shoot meristem development (Zhang and Zhang, 2012), root growth and development (Boualem et al., 2008; Singh et al., 2014) and seed maturation (Tang et al., 2012). MiR399 has been studied for its role in phosphate homeostasis and starvation (Baek et al., 2013; Tian et al., 2018). For the two last, miR477 and miR8175, to date little data is available concerning their biological function. MiR477 is implicated in plant immunity in Camellia sinensis and in Gossypium hirsutum (Hu et al., 2020; Wang S. et al., 2020), while miR8175 has been studied in Arabidopsis thaliana roots, and found to be upregulated by high-light intensity (Anna et al., 2019).
Among the nine genes identified as predicted targets, three genes participate in hormone signaling including two genes involved in the cytokinin pathway (RhCKX1, RhCKX6) both targeted by miR159a_1 and one in the strigolactones pathway (RwMAX4) targeted by six miR166 members. These genes are involved in the repression of bud outgrowth in Rosa. Interestingly, none of the genes involved in auxin and gibberellin pathways are found as potential targets of post-transcriptional regulation via miRNAs. Concerning sugar control, two genes (RhSUSY1, Rh6PFK) are targeted by two different miRNAs: miR8175 and miR166e-5p, respectively. Besides hormonal and sugar control, two genes involved in ROS metabolism are potential targets of miRNAs. The first one, RhAPX1, encoding antioxidant enzyme is targeted by three miR164 isoforms (miR164a_4, 164b, and 164e-5p), the other one (RhRBOHB1) encoding a NADPH oxidase is targeted by miR399e_5. Finally, two genes involved in the cell division (RhCYCD3;2) and expansion (RhEXPA3) are identified as potential targets of miR477e and miR156j_1, respectively. The validation of the interaction between these 7 miRNAs and the predicted rosebush targets awaits experimental confirmation. It is however noteworthy that, in agreement with our findings, miR159 was previously shown as targeting CKX with negative correlation of their expression in response to pathogens in poplar (Zhao et al., 2012). Furthermore, suppressing miR159 leads to a decreased expression of the CK synthesis gene, OsIPT, of CK signaling genes OsRR and of the CK degradation genes OsCKX in rice (Zhao et al., 2017). Taken together, these data suggest that miR159 may play a role in cytokinin metabolism and signaling and support our prediction of miR159 targeting two CKX in rosebush during bud outgrowth.
Our study reveals that even though few genes of the regulatory network of axillary bud outgrowth appear under miRNA regulation, most of the pathways identified in Rosa are potential targets of miRNA regulation (Figure 2). This suggests that miRNAs act on multiple pathways of the complex network regulating bud outgrowth in rosebush. Interestingly, six of the nine identified genes targeted by miRNAs have a light regulated expression (Supplementary Table 1). This brings up the hypothesis that their respective associated miRNAs might potentially act in the light transduction pathway (Figure 2). Further studies addressing the light regulation of these miRNAs in rosebush will be required to progress in the understanding of their role during axillary bud outgrowth.
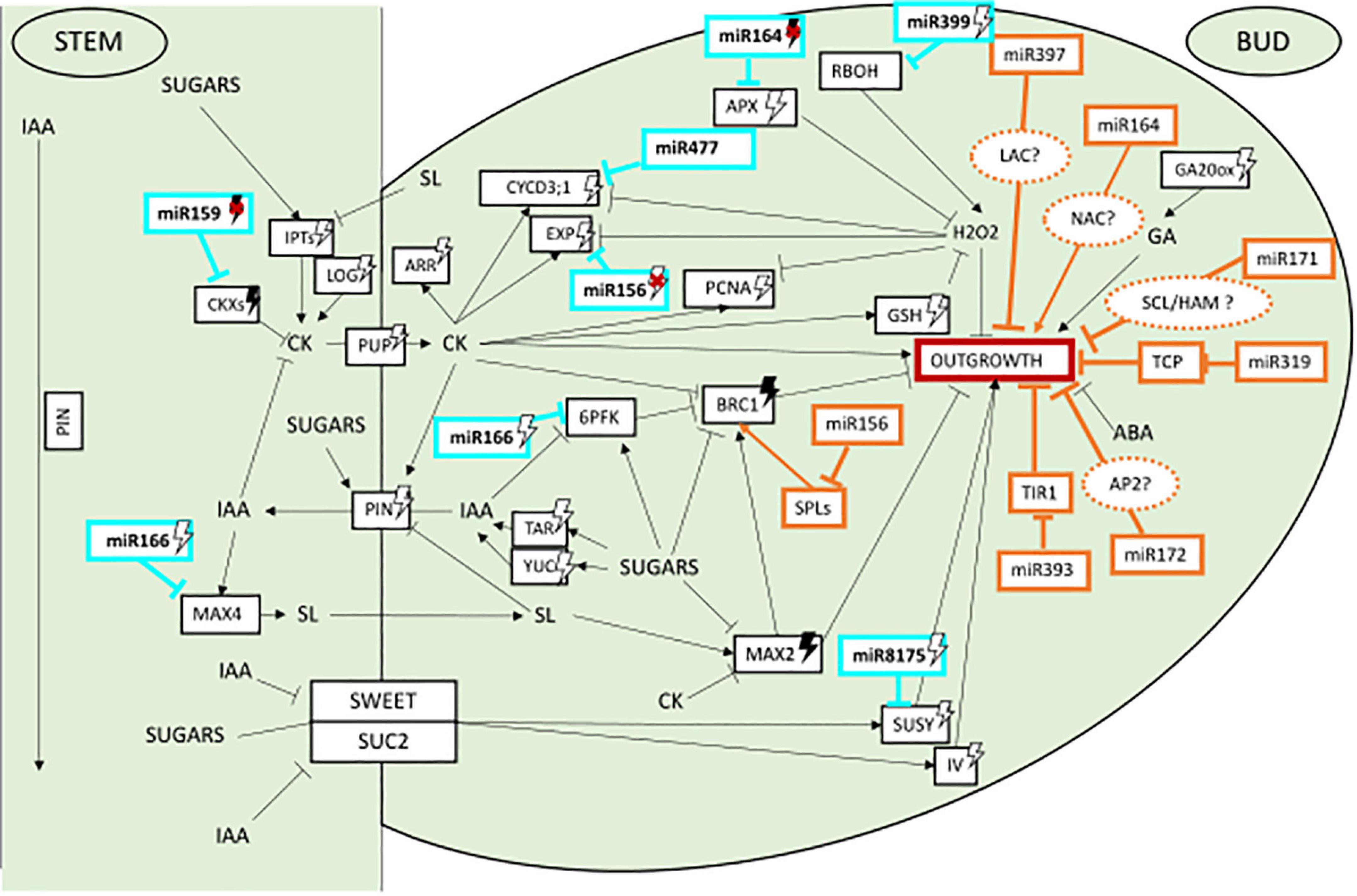
Figure 2. Potential role of miRNAs in the control of axillary bud outgrowth and their light regulation in plants. In bright blue miRNAs identified in the present study as putative novel regulators in control of bud outgrowth. Black arrows heads mean induction, straight lines mean repression, white lightning bolt means induction by light, darked lightning bolt means induction by darkness, red cross means repression of the miRNA.
The other two miRNAs identified here, miR156 and miR164 were already described to play a role in regulating shoot branching (as mentioned above). While miR156j_1 Rosa isoform and the 3 miR164 Rosa isoforms are predicted to bind to their conserved targets SPL and NAC (Laufs et al., 2004; Wang and Wang, 2015; Blein and Laufs, 2016; Xu et al., 2016), new targets were also identified by our analysis: EXPA3 and APX1, respectively, both genes being light-regulated during bud outgrowth in Rosa (Supplementary Table 1). The Arabidopsis orthologs of these Rosa targets (AtEXP15 and AtAPX1) lack a binding site for the miRNA (data not shown). Therefore, it is attractive to speculate that the addition of these new targets may have appeared by evolution of their sequence to create a novel miRNA binding site. The resulting bifurcated pathway downstream the miRNA, with one conserved branch (SPL or NAC) and one branch more species-specific (EXPA3 or APX1) could provide robustness to the effect of the miRNA on bud outgrowth.
Conclusion and Future Perspectives
Multiple regulators have been described as acting in concert to fine tune, in an intricate network, axillary bud outgrowth as well as its photoregulation. While this network is mostly built on integrating knowledge gained at the transcriptional level, little is known to date about how post-transcriptional regulation fits into this network. Here, we provide new information by integrating post-transcriptional regulation of the photocontrol bud growth into this network. Based on the literature published mainly on Arabidopsis, rice and maize combined with our bioinformatic analysis on Rosa we explored specifically the potential role of miRNAs in the control of bud growth and how they may manage to interact with this network. Thus, we identified through bioinformatic analysis some conserved miRNAs families and their associated target genes as good candidates to play a potential role in the control of axillary bud outgrowth in the rosebush. Interestingly, these miRNAs have the ability to target key molecular players in all major pathways regulating bud outgrowth (including hormones, sugars, and ROS) with a far broader spectrum compared to previously reported studies. Additionally, according to literature, several of those such as miR156, miR164, miR166, and miR8175 are under light regulation which leads us to suggest that they may participate in the light-mediated mechanisms of axillary bud outgrowth. Whether or not these novel miRNA-gene target couples physically interact and demonstrate a biological relevance in the regulation of bud outgrowth and its photocontrol is still to be addressed. To this end, we need to move toward functional analysis and use of mutants.
Involvement of post-transcriptional factors in bud outgrowth control is not limited to miRNAs. Indeed, some studies have brought to light the possible role of small interfering RNAs (siRNAs) in this process (Ortiz-Morea et al., 2013; Kamthan et al., 2015). In this purpose, future research should be conducted to identify precisely which small RNAs are involved using deep sequencing and understand how they interact with miRNAs in the control of axillary bud outgrowth.
The understanding of the mechanisms behind miRNA control of bud outgrowth could be a great opportunity for improving agriculturally important traits such as plant architecture (Boumaza et al., 2010; Leduc et al., 2014; Huché-Thélier et al., 2016). Notably, it was shown recently that plant 5′ of primary miRNA contains a short open reading-frame (ORF) that encodes a peptide called micro-peptide (miPEPs). These miPEPs can raise the mature miRNA level by enhancing the transcription of their associated primary miRNA in a specific manner (Lauressergues et al., 2015). For that miPEPs could be used as powerful tools to lead to agronomic traits improvement under miRNA control (Couzigou et al., 2015).
Data Availability Statement
The original contributions presented in the study are included in the article/Supplementary Material, further inquiries can be directed to the corresponding author/s.
Author Contributions
JM, PL, NL, and JLG designed the experiments and wrote the manuscript. JM performed the experiments and bio informatic analysis. PL, NL, and JLG supervised the work. All authors read and approved the final manuscript.
Funding
This work was supported by University of Angers and Pays de la Loire Region, project ROSAPEPS funded by RFI-Objectif Vegetal. The IJPB benefits from the support of Saclay Plant Sciences-SPS (ANR-17-EUR-0007). JM was the recipient of a Ph.D. scholarship from the Ministére de l‘Enseignement Supérieur et de la Recherche.
Conflict of Interest
The authors declare that the research was conducted in the absence of any commercial or financial relationships that could be construed as a potential conflict of interest.
Publisher’s Note
All claims expressed in this article are solely those of the authors and do not necessarily represent those of their affiliated organizations, or those of the publisher, the editors and the reviewers. Any product that may be evaluated in this article, or claim that may be made by its manufacturer, is not guaranteed or endorsed by the publisher.
Acknowledgments
We are grateful to Nathalie Brouard and Anita Lebrec for their help in bud sampling. We are most grateful to the ImorPhen core facility (Angers, France) for its technical support.
Supplementary Material
The Supplementary Material for this article can be found online at: https://www.frontiersin.org/articles/10.3389/fpls.2021.770363/full#supplementary-material
Footnotes
References
Abidi, F., Girault, T., Douillet, O., Guillemain, G., Sintes, G., Laffaire, M., et al. (2013). Blue light effects on rose photosynthesis and photomorphogenesis: blue light and rose development. Plant Biol. 15, 67–74. doi: 10.1111/j.1438-8677.2012.00603.x
Aguilar-Martínez, J. A., Poza-Carrión, C., and Cubas, P. (2007). Arabidopsis BRANCHED1 Acts as an Integrator of Branching Signals within Axillary Buds. Plant Cell 19, 458–472. doi: 10.1105/tpc.106.048934
Alonso-Peral, M. M., Li, J., Li, Y., Allen, R. S., Schnippenkoetter, W., Ohms, S., et al. (2010). The MicroRNA159-Regulated GAMYB-like Genes Inhibit Growth and Promote Programmed Cell Death in Arabidopsis. Plant Physiol. 154, 757–771. doi: 10.1104/pp.110.160630
Anna, B.-B., Grzegorz, B., Marek, K., Piotr, G., and Marcin, F. (2019). Exposure to High-Intensity Light Systemically Induces Micro-Transcriptomic Changes in Arabidopsis thaliana Roots. Int. J. Mol. Sci. 20:5131. doi: 10.3390/ijms20205131
Aung, B., Gruber, M. Y., Amyot, L., Omari, K., Bertrand, A., and Hannoufa, A. (2015). MicroRNA156 as a promising tool for alfalfa improvement. Plant Biotechnol. J. 13, 779–790. doi: 10.1111/pbi.12308
Baek, D., Kim, M. C., Chun, H. J., Kang, S., Park, H. C., Shin, G., et al. (2013). Regulation of miR399f Transcription by AtMYB2 Affects Phosphate Starvation Responses in Arabidopsis. Plant Physiol. 161, 362–373. doi: 10.1104/pp.112.205922
Barbier, F. F., Cao, D., Fichtner, F., Weiste, C., Perez-Garcia, M., Caradeuc, M., et al. (2021). HEXOKINASE1 signalling promotes shoot branching and interacts with cytokinin and strigolactone pathways. New Phytol. 231, 1088–1104. doi: 10.1111/nph.17427
Barbier, F. F., Dun, E. A., Kerr, S. C., Chabikwa, T. G., and Beveridge, C. A. (2019). An Update on the Signals Controlling Shoot Branching. Trends Plant Sci. 24, 220–236. doi: 10.1016/j.tplants.2018.12.001
Barbier, F., Péron, T., Lecerf, M., Perez-Garcia, M.-D., Barrière, Q., Rolčík, J., et al. (2015). Sucrose is an early modulator of the key hormonal mechanisms controlling bud outgrowth in Rosa hybrida. J. Exp. Bot. 66, 2569–2582. doi: 10.1093/jxb/erv047
Bennett, T., Sieberer, T., Willett, B., Booker, J., Luschnig, C., and Leyser, O. (2006). The Arabidopsis MAX Pathway Controls Shoot Branching by Regulating Auxin Transport. Curr. Biol. 16, 553–563. doi: 10.1016/j.cub.2006.01.058
Bertheloot, J., Barbier, F., Boudon, F., Perez-Garcia, M. D., Péron, T., Citerne, S., et al. (2020). Sugar availability suppresses the auxin-induced strigolactone pathway to promote bud outgrowth. New Phytol. 225, 866–879. doi: 10.1111/nph.16201
Berthet, S., Demont-Caulet, N., Pollet, B., Bidzinski, P., Cézard, L., Le Bris, P., et al. (2011). Disruption of LACCASE4 and 17 Results in Tissue-Specific Alterations to Lignification of Arabidopsis thaliana Stems. Plant Cell 23, 1124–1137. doi: 10.1105/tpc.110.082792
Beveridge, C. A., Symons, G. M., and Turnbull, C. G. N. (2000). Auxin Inhibition of Decapitation-Induced Branching Is Dependent on Graft-Transmissible Signals Regulated by Genes Rms1 and Rms2. Plant Physiol. 123, 689–698. doi: 10.1104/pp.123.2.689
Blein, T., and Laufs, P. (2016). “MicroRNAs (miRNAs) and Plant Development,” in eLS, (Chichester, UK: John Wiley & Sons, Ltd), eds John Wiley & Sons, Ltd. 1–11. doi: 10.1002/9780470015902.a0020106.pub2
Bonicel, A., Haddad, G., and Gagnaire, J. (1987). Seasonal variations of starch and major soluble sugars in the different organs of young poplars. Plant Physiol. Biochem. 25, 451–459.
Boualem, A., Laporte, P., Jovanovic, M., Laffont, C., Plet, J., Combier, J.-P., et al. (2008). MicroRNA166 controls root and nodule development in Medicago truncatula. Plant J. 54, 876–887. doi: 10.1111/j.1365-313X.2008.03448.x
Boumaza, R., Huché-Thélier, L., Demotes-Mainard, S., Coz, E. L., Leduc, N., Pelleschi-Travier, S., et al. (2010). Sensory profiles and preference analysis in ornamental horticulture: the case of the rosebush. Food Qual. Prefer. 21, 987–997. doi: 10.1016/j.foodqual.2010.05.003
Brewer, P. B. (2015). - Plant Architecture The Long and the Short of Branching in Potato. Curr. Biol. 25, R724–R725.
Brewer, P. B., Dun, E. A., Ferguson, B. J., Rameau, C., and Beveridge, C. A. (2009). Strigolactone Acts Downstream of Auxin to Regulate. bud outgrowth in pea and Arabidopsis. Plant Physiol. 150, 482–493.
Chatfield, S. P., Stirnberg, P., Forde, B. G., and Leyser, O. (2000). The hormonal regulation of axillary bud growth in Arabidopsis. Plant J. 24, 159–169. doi: 10.1046/j.1365-313x.2000.00862.x
Chen, X., Xia, X., Guo, X., Zhou, Y., Shi, K., Zhou, J., et al. (2016). Apoplastic H 2 O 2 plays a critical role in axillary bud outgrowth by altering auxin and cytokinin homeostasis in tomato plants. New Phytol. 211, 1266–1278. doi: 10.1111/nph.14015
Choubane, D., Rabot, A., Mortreau, E., Legourrierec, J., Péron, T., Foucher, F., et al. (2012). Photocontrol of bud burst involves gibberellin biosynthesis in Rosa sp. J. Plant Physiol. 169, 1271–1280. doi: 10.1016/j.jplph.2012.04.014
Chuck, G., Cigan, A. M., Saeteurn, K., and Hake, S. (2007). The heterochronic maize mutant Corngrass1 results from overexpression of a tandem microRNA. Nat. Genet. 39, 544–549. doi: 10.1038/ng2001
Cline, M. G. (1997). Concepts and terminology of apical dominance. Am. J. Bot. 84, 1064–1069. doi: 10.2307/2446149
Cline, M. G., and Oh, C. (2006). A Reappraisal of the Role of Abscisic Acid and its Interaction with Auxin in Apical Dominance. Ann. Bot. 98, 891–897. doi: 10.1093/aob/mcl173
Corot, A., Roman, H., Douillet, O., Autret, H., Perez-Garcia, M.-D., Citerne, S., et al. (2017). Cytokinins and Abscisic Acid Act Antagonistically in the Regulation of the Bud Outgrowth Pattern by Light Intensity. Front. Plant Sci. 8:1724. doi: 10.3389/fpls.2017.01724
Couzigou, J.-M., Lauressergues, D., Bécard, G., and Combier, J.-P. (2015). miRNA-encoded peptides (miPEPs): a new tool to analyze the roles of miRNAs in plant biology. RNA Biol. 12, 1178–1180. doi: 10.1080/15476286.2015.1094601
Cui, L., Zheng, F., Wang, J., Zhang, C., Xiao, F., Ye, J., et al. (2020). miR156a-targeted SBP-Box transcription factor SlSPL13 regulates inflorescence morphogenesis by directly activating SFT in tomato. Plant Biotechnol. J. 18, 1670–1682. doi: 10.1111/pbi.13331
Curaba, J., Talbot, M., Li, Z., and Helliwell, C. (2013). Over-expression of microRNA171 affects phase transitions and floral meristem determinancy in barley. BMC Plant Biol. 13:6. doi: 10.1186/1471-2229-13-6
Dai, X., Zhuang, Z., and Zhao, P. X. (2018). psRNATarget: a plant small RNA target analysis server (2017 release). Nucleic Acids Res. 46, W49–W54. doi: 10.1093/nar/gky316
Dai, Z., Wang, J., Yang, X., Lu, H., Miao, X., and Shi, Z. (2018). Modulation of plant architecture by the miR156f–OsSPL7–OsGH3.8 pathway in rice. J. Exp. Bot. 69, 5117–5130. doi: 10.1093/jxb/ery273
Demotes-Mainard, S., Huché-Thélier, L., Morel, P., Boumaza, R., Guérin, V., and Sakr, S. (2013). Temporary water restriction or light intensity limitation promotes branching in rose bush. Sci. Hortic. 150, 432–440. doi: 10.1016/j.scienta.2012.12.005
Demotes-Mainard, S., Péron, T., Corot, A., Bertheloot, J., Le Gourrierec, J., Pelleschi-Travier, S., et al. (2016). Plant responses to red and far-red lights, applications in horticulture. Environ. Exp. Bot. 121, 4–21. doi: 10.1016/j.envexpbot.2015.05.010
Devitt, M. L., and Stafstrom, J. P. (1995). Cell cycle regulation during growth-dormancy cycles in pea axillary buds. Plant Mol. Biol. 29, 255–265. doi: 10.1007/BF00043650
Dierck, R., De Keyser, E., De Riek, J., Dhooghe, E., Van Huylenbroeck, J., Prinsen, E., et al. (2016). Change in Auxin and Cytokinin Levels Coincides with Altered Expression of Branching Genes during Axillary Bud Outgrowth in Chrysanthemum. PLoS One 11:e0161732. doi: 10.1371/journal.pone.0161732
Djennane, S., Hibrand-Saint Oyant, L., Kawamura, K., Lalanne, D., Laffaire, M., Thouroude, T., et al. (2014). Impacts of light and temperature on shoot branching gradient and expression of strigolactone synthesis and signalling genes in rose: light and temperature effects on rose branching. Plant Cell Environ. 37, 742–757. doi: 10.1111/pce.12191
Dodsworth, S. (2009). A diverse and intricate signalling network regulates stem cell fate in the shoot apical meristem. Dev. Biol. 336, 1–9. doi: 10.1016/j.ydbio.2009.09.031
Domagalska, M. A., and Leyser, O. (2011). Signal integration in the control of shoot branching. Nat. Rev. Mol. Cell Biol. 12, 211–221. doi: 10.1038/nrm3088
Dong, F., Wang, C., Dong, Y., Hao, S., Wang, L., Sun, X., et al. (2020). Differential expression of microRNAs in tomato leaves treated with different light qualities. BMC Genomics 21:37. doi: 10.1186/s12864-019-6440-4
Du, Y., Liu, L., Li, M., Fang, S., Shen, X., Chu, J., et al. (2017). UNBRANCHED3 regulates branching by modulating cytokinin biosynthesis and signaling in maize and rice. New Phytol. 214, 721–733. doi: 10.1111/nph.14391
Dun, E. A., de Saint Germain, A., Rameau, C., and Beveridge, C. A. (2012). Antagonistic Action of Strigolactone and Cytokinin in Bud Outgrowth Control. Plant Physiol. 158, 487–498. doi: 10.1104/pp.111.186783
Dun, E. A., Ferguson, B. J., and Beveridge, C. A. (2006). Apical Dominance and Shoot Branching. Divergent Opinions or Divergent Mechanisms? Plant Physiol. 142, 812–819. doi: 10.1104/pp.106.086868
Fahlgren, N., Howell, M. D., Kasschau, K. D., Chapman, E. J., Sullivan, C. M., Cumbie, J. S., et al. (2007). High-Throughput Sequencing of Arabidopsis microRNAs: evidence for Frequent Birth and Death of MIRNA Genes. PLoS One 2:e219. doi: 10.1371/journal.pone.0000219
Ferguson, B. J., and Beveridge, C. A. (2009). Roles for Auxin, Cytokinin, and Strigolactone in Regulating Shoot Branching. Plant Physiol. 149, 1929–1944. doi: 10.1104/pp.109.135475
Finlayson, S. A., Krishnareddy, S. R., Kebrom, T. H., and Casal, J. J. (2010). Phytochrome Regulation of Branching in Arabidopsis. Plant Physiol. 152, 1914–1927. doi: 10.1104/pp.109.148833
Garbez, M., Galopin, G., Sigogne, M., Favre, P., Demotes-Mainard, S., and Symoneaux, R. (2015). Assessing the visual aspect of rotating virtual rose bushes by a labeled sorting task. Food Qual. Prefer. 40, 287–295. doi: 10.1016/j.foodqual.2014.06.008
Girault, T., Abidi, F., Sigogne, M., Pelleschi-Travier, S., Boumaza, R., Sakr, S., et al. (2010). Sugars are under light control during bud burst in Rosa sp.: photocontrol of sugars during bud burst. Plant Cell Environ. 33, 1339–1350. doi: 10.1111/j.1365-3040.2010.02152.x
Girault, T., Bergougnoux, V., Combes, D., Viemont, J.-D., and Leduc, N. (2008). Light controls shoot meristem organogenic activity and leaf primordia growth during bud burst in Rosa sp. Plant Cell Environ. 31, 1534–1544. doi: 10.1111/j.1365-3040.2008.01856.x
Gocal, G. F. W., Pharis, R. P., Yeung, E. C., and Pearce, D. (1991). Changes after Decapitation in Concentrations of Indole-3-Acetic Acid and Abscisic Acid in the Larger Axillary Bud of Phaseolus vulgaris L. cv Tender Green. Plant Physiol. 95, 344–350. doi: 10.1104/pp.95.2.344
González-Grandío, E., Poza-Carrión, C., Sorzano, C. O. S., and Cubas, P. (2013). BRANCHED1 Promotes Axillary Bud Dormancy in Response to Shade in Arabidopsis. Plant Cell 25, 834–850. doi: 10.1105/tpc.112.108480
Guo, C., Xu, Y., Shi, M., Lai, Y., Wu, X., Wang, H., et al. (2017). Repression of miR156 by miR159 Regulates the Timing of the Juvenile-to-Adult Transition in Arabidopsis. Plant Cell 29, 1293–1304. doi: 10.1105/tpc.16.00975
Guo, S., Xu, Y., Liu, H., Mao, Z., Zhang, C., Ma, Y., et al. (2013). The interaction between OsMADS57 and OsTB1 modulates rice tillering via DWARF14. Nat. Commun. 4:1566. doi: 10.1038/ncomms2542
Han, H., Yan, A., Li, L., Zhu, Y., Feng, B., Liu, X., et al. (2020). A signal cascade originated from epidermis defines apical-basal patterning of Arabidopsis shoot apical meristems. Nat. Commun. 11:1214. doi: 10.1038/s41467-020-14989-4
Henry, C., Rabot, A., Laloi, M., Mortreau, E., Sigogne, M., Leduc, N., et al. (2011). Regulation of RhSUC2, a sucrose transporter, is correlated with the light control of bud burst in Rosa sp.: sucrose transporter role in bud burst. Plant Cell Environ. 34, 1776–1789. doi: 10.1111/j.1365-3040.2011.02374.x
Hibrand Saint-Oyant, L., Ruttink, T., Hamama, L., Kirov, I., Lakhwani, D., Zhou, N. N., et al. (2018). A high-quality genome sequence of Rosa chinensis to elucidate ornamental traits. Nat. Plants 4, 473–484. doi: 10.1038/s41477-018-0166-1
Holalu, S. V., Reddy, S. K., Blackman, B. K., and Finlayson, S. A. (2020). Phytochrome interacting factors 4 and 5 regulate axillary branching via bud abscisic acid and stem auxin signalling. Plant Cell Environ. 43, 2224–2238. doi: 10.1111/pce.13824
Hu, G., Hao, M., Wang, L., Liu, J., Zhang, Z., Tang, Y., et al. (2020). The Cotton miR477- CBP60A Module Participates in Plant Defense Against Verticillium dahlia. Mol. Plant Microbe Interact. 33, 624–636. doi: 10.1094/MPMI-10-19-0302-R
Huang, J., Li, Z., and Zhao, D. (2016). Deregulation of the OsmiR160 Target Gene OsARF18 Causes Growth and Developmental Defects with an Alteration of Auxin Signaling in Rice. Sci. Rep. 6:29938. doi: 10.1038/srep29938
Huang, W., Peng, S., Xian, Z., Lin, D., Hu, G., Yang, L., et al. (2017). Overexpression of a tomato miR171 target gene SlGRAS24 impacts multiple agronomical traits via regulating gibberellin and auxin homeostasis. Plant Biotechnol. J. 15, 472–488. doi: 10.1111/pbi.12646
Huché-Thélier, L., Crespel, L., Gourrierec, J. L., Morel, P., Sakr, S., and Leduc, N. (2016). Light signaling and plant responses to blue and UV radiations—Perspectives for applications in horticulture. Environ. Exp. Bot. 121, 22–38. doi: 10.1016/j.envexpbot.2015.06.009
Jiao, Y., Wang, Y., Xue, D., Wang, J., Yan, M., Liu, G., et al. (2010). Regulation of OsSPL14 by OsmiR156 defines ideal plant architecture in rice. Nat. Genet. 42, 541–544. doi: 10.1038/ng.591
Kamthan, A., Chaudhuri, A., Kamthan, M., and Datta, A. (2015). Small RNAs in plants: recent development and application for crop improvement. Front. Plant Sci. 06:208. doi: 10.3389/fpls.2015.00208
Kebrom, T. H., and Mullet, J. E. (2016). Transcriptome Profiling of Tiller Buds Provides New Insights into PhyB Regulation of Tillering and Indeterminate Growth in Sorghum. Plant Physiol. 170, 2232–2250. doi: 10.1104/pp.16.00014
Kebrom, T. H., Chandler, P. M., Swain, S. M., King, R. W., Richards, R. A., and Spielmeyer, W. (2012). Inhibition of Tiller Bud Outgrowth in the tin Mutant of Wheat Is Associated with Precocious Internode Development. Plant Physiol. 160, 308–318. doi: 10.1104/pp.112.197954
Knox, J. P., and Wareing, P. F. (1984). Apical Dominance in Phaseolus vulgaris L.: the Possible Roles of Abscisic and Indole-3-Acetic Acid. J. Exp. Bot. 35, 239–244.
Koornneef, M., and van der Veen, J. H. (1980). Induction and Analysis of gibberellin Sensitive Mutants in Arabidopsis thaliana (L.) Heynh. Theor. Appl. Genet. 58, 257–263.
Kotov, A. A., Kotova, L. M., and Romanov, G. A. (2021). Signaling network regulating plant branching: recent advances and new challenges. Plant Sci. 307:110880. doi: 10.1016/j.plantsci.2021.110880
Kozomara, A., Birgaoanu, M., and Griffiths-Jones, S. (2019). miRBase: from microRNA sequences to function. Nucleic Acids Res. 47, D155–D162. doi: 10.1093/nar/gky1141
Kravchik, M., Stav, R., Belausov, E., and Arazi, T. (2019). Functional Characterization of microRNA171 Family in Tomato. Plants 8:10. doi: 10.3390/plants8010010
Lang, G., Early, J., Martin, G., and Darnell, R. (1987). Endo-, para-, and ecodormancy: physiological terminology and classification for dormancy research. HortScience 22, 371–377.
Laufs, P., Peaucelle, A., Morin, H., and Traas, J. (2004). MicroRNA regulation of the CUC genes is required for boundary size control in Arabidopsis meristems. Development 131, 4311–4322. doi: 10.1242/dev.01320
Lauressergues, D., Couzigou, J.-M., Clemente, H. S., Martinez, Y., Dunand, C., Bécard, G., et al. (2015). Primary transcripts of microRNAs encode regulatory peptides. Nature 520, 90–93. doi: 10.1038/nature14346
Le Bris, M., Michaux-Ferrière, N., Jacob, Y., Poupet, A., Barthe, P., Guigonis, J.-M., et al. (1999). Regulation of bud dormancy by manipulation of ABA in isolated buds of Rosa hybrida cultured in vitro. Funct. Plant Biol. 26, 273–281. doi: 10.1071/PP98133
Le Hir, R., Leduc, N., Jeannette, E., Viemont, J.-D., and Pelleschi-Travier, S. (2006). Variations in sucrose and ABA concentrations are concomitant with heteroblastic leaf shape changes in a rhythmically growing species (Quercus robur). Tree Physiol. 26, 229–238. doi: 10.1093/treephys/26.2.229
Le Moigne, M.-A., Guérin, V., Furet, P.-M., Billard, V., Lebrec, A., Spíchal, L., et al. (2018). Asparagine and sugars are both required to sustain secondary axis elongation after bud outgrowth in Rosa hybrida. J. Plant Physiol. 222, 17–27. doi: 10.1016/j.jplph.2017.12.013
Leduc, N., Roman, H., Barbier, F., Péron, T., Huché-Thélier, L., Lothier, J., et al. (2014). Light Signaling in Bud Outgrowth and Branching in Plants. Plants 3, 223–250. doi: 10.3390/plants3020223
Li, C., and Zhang, B. (2016). MicroRNAs in Control of Plant Development. J. Cell. Physiol. 231, 303–313. doi: 10.1002/jcp.25125
Li, G., Tan, M., Cheng, F., Liu, X., Qi, S., Chen, H., et al. (2018). Molecular role of cytokinin in bud activation and outgrowth in apple branching based on transcriptomic analysis. Plant Mol. Biol. 98, 261–274. doi: 10.1007/s11103-018-0781-2
Li, X., Xia, K., Liang, Z., Chen, K., Gao, C., and Zhang, M. (2016). MicroRNA393 is involved in nitrogen-promoted rice tillering through regulation of auxin signal transduction in axillary buds. Sci. Rep. 6:32158. doi: 10.1038/srep32158
Lian, H., Wang, L., Ma, N., Zhou, C.-M., Han, L., Zhang, T.-Q., et al. (2021). Redundant and specific roles of individual MIR172 genes in plant development. PLoS Biol. 19:e3001044. doi: 10.1371/journal.pbio.3001044
Li-Marchetti, C., Le Bras, C., Relion, D., Citerne, S., Huché-Thélier, L., Sakr, S., et al. (2015). Genotypic differences in architectural and physiological responses to water restriction in rose bush. Front. Plant Sci. 06:355. doi: 10.3389/fpls.2015.00355
Liu, H., Yu, H., Tang, G., and Huang, T. (2018). Small but powerful: function of microRNAs in plant development. Plant Cell Rep. 37, 515–528. doi: 10.1007/s00299-017-2246-5
Liu, S., Mi, X., Zhang, R., An, Y., Zhou, Q., Yang, T., et al. (2019). Integrated analysis of miRNAs and their targets reveals that miR319c/TCP2 regulates apical bud burst in tea plant (Camellia sinensis). Planta 250, 1111–1129. doi: 10.1007/s00425-019-03207-1
Lu, Z., Yu, H., Xiong, G., Wang, J., Jiao, Y., Liu, G., et al. (2013). Genome-Wide Binding Analysis of the Transcription Activator IDEAL PLANT ARCHITECTURE1 Reveals a Complex Network Regulating Rice Plant Architecture. Plant Cell 25, 3743–3759. doi: 10.1105/tpc.113.113639
Marquat, C., Vandamme, M., Gendraud, M., and Pétel, G. (1999). Dormancy in vegetative buds of peach: relation between carbohydrate absorption potentials and carbohydrate concentration in the bud during dormancy and its release. Sci. Hortic. 79, 151–162. doi: 10.1016/S0304-4238(98)00203-9
Mason, M. G., Ross, J. J., Babst, B. A., Wienclaw, B. N., and Beveridge, C. A. (2014). Sugar demand, not auxin, is the initial regulator of apical dominance. Proc. Natl. Acad. Sci. U. S. A. 111, 6092–6097. doi: 10.1073/pnas.1322045111
Maugarny-Calès, A., and Laufs, P. (2018). Getting leaves into shape: a molecular, cellular, environmental and evolutionary view. Development 145:dev161646. doi: 10.1242/dev.161646
Meitha, K., Agudelo-Romero, P., Signorelli, S., Gibbs, D. J., Considine, J. A., Foyer, C. H., et al. (2018). Developmental control of hypoxia during bud burst in grapevine: developmental hypoxia in grapevine buds. Plant Cell Environ. 41, 1154–1170. doi: 10.1111/pce.13141
Miura, K., Ikeda, M., Matsubara, A., Song, X.-J., Ito, M., Asano, K., et al. (2010). OsSPL14 promotes panicle branching and higher grain productivity in rice. Nat. Genet. 42, 545–549. doi: 10.1038/ng.592
Morea, E. G. O., da Silva, E. M., e Silva, G. F. F., Valente, G. T., Barrera Rojas, C. H., Vincentz, M., et al. (2016). Functional and evolutionary analyses of the miR156 and miR529 families in land plants. BMC Plant Biol. 16:40. doi: 10.1186/s12870-016-0716-5
Ophir, R., Pang, X., Halaly, T., Venkateswari, J., Lavee, S., Galbraith, D., et al. (2009). Gene-expression profiling of grape bud response to two alternative dormancy-release stimuli expose possible links between impaired mitochondrial activity, hypoxia, ethylene-ABA interplay and cell enlargement. Plant Mol. Biol. 71, 403–423. doi: 10.1007/s11103-009-9531-9
Or, E., Vilozny, I., Fennell, A., Eyal, Y., and Ogrodovitch, A. (2002). Dormancy in grape buds: isolation and characterization of catalase cDNA and analysis of its expression following chemical induction of bud dormancy release. Plant Sci. 162, 121–130. doi: 10.1016/S0168-9452(01)00542-8
Ortiz-Morea, F. A., Vicentini, R., Silva, G. F. F., Silva, E. M., Carrer, H., Rodrigues, A. P., et al. (2013). Global analysis of the sugarcane microtranscriptome reveals a unique composition of small RNAs associated with axillary bud outgrowth. J. Exp. Bot. 64, 2307–2320. doi: 10.1093/jxb/ert089
Palatnik, J. F., Allen, E., Wu, X., Schommer, C., Schwab, R., Carrington, J. C., et al. (2003). Control of leaf morphogenesis by microRNAs. Nature 425, 257–263. doi: 10.1038/nature01958
Park, S. J., Choi, S. W., Kim, G. M., Møller, C., Pai, H.-S., and Yang, S. W. (2021). Light-stabilized FHA2 suppresses miRNA biogenesis through interactions with DCL1 and HYL1. Mol. Plant 14, 647–663. doi: 10.1016/j.molp.2021.01.020
Pashkovskiy, P. P., Kartashov, A. V., Zlobin, I. E., Pogosyan, S. I., and Kuznetsov, V. V. (2016). Blue light alters miR167 expression and microRNA-targeted auxin response factor genes in Arabidopsis thaliana plants. Plant Physiol. Biochem. 104, 146–154. doi: 10.1016/j.plaphy.2016.03.018
Pashkovskiy, P., Ryazansky, S., Kartashov, A., Voloshin, R., Khudyakova, A., Kosobryukhov, A. A., et al. (2021). Effect of red light on photosynthetic acclimation and the gene expression of certain light signalling components involved in the microRNA biogenesis in the extremophile Eutrema salsugineum. J. Biotechnol. 325, 35–42. doi: 10.1016/j.jbiotec.2020.11.018
Pérez, V. I., Van Remmen, H., Bokov, A., Epstein, C. J., Vijg, J., and Richardson, A. (2009). The overexpression of major antioxidant enzymes does not extend the lifespan of mice. Aging Cell 8, 73–75. doi: 10.1111/j.1474-9726.2008.00449.x
Porcher, A., Guérin, V., Leduc, N., Lebrec, A., Lothier, J., and Vian, A. (2021). Ascorbate–glutathione pathways mediated by cytokinin regulate H2O2 levels in light-controlled rose bud burst. Plant Physiol. 186, 910–928. doi: 10.1093/plphys/kiab123
Porcher, A., Guérin, V., Montrichard, F., Lebrec, A., Lothier, J., and Vian, A. (2020). Ascorbate glutathione-dependent H2O2 scavenging is an important process in axillary bud outgrowth in rosebush. Ann. Bot. 126, 1049–1062. doi: 10.1093/aob/mcaa130
Preston, J. C., and Hileman, L. C. (2013). Functional Evolution in the Plant SQUAMOSA-PROMOTER BINDING PROTEIN-LIKE (SPL) Gene Family. Front. Plant Sci. 4:80. doi: 10.3389/fpls.2013.00080
Prusinkiewicz, P., Crawford, S., Smith, R. S., Ljung, K., Bennett, T., Ongaro, V., et al. (2009). Control of bud activation by an auxin transport switch. Proc. Natl. Acad. Sci. U. S. A. 106, 17431–17436. doi: 10.1073/pnas.0906696106
Pulido, A., and Laufs, P. (2010). Co-ordination of developmental processes by small RNAs during leaf development. J. Exp. Bot. 61, 1277–1291. doi: 10.1093/jxb/erp397
Qiao, Y., Zhang, J., Zhang, J., Wang, Z., Ran, A., Guo, H., et al. (2017). Integrated RNA-seq and sRNA-seq analysis reveals miRNA effects on secondary metabolism in Solanum tuberosum L. Mol. Genet. Genomics 292, 37–52. doi: 10.1007/s00438-016-1253-5
Rabot, A., Henry, C., Ben Baaziz, K., Mortreau, E., Azri, W., Lothier, J., et al. (2012). Insight into the Role of Sugars in Bud Burst Under Light in the Rose. Plant Cell Physiol. 53, 1068–1082. doi: 10.1093/pcp/pcs051
Rabot, A., Portemer, V., Péron, T., Mortreau, E., Leduc, N., Hamama, L., et al. (2014). Interplay of Sugar, Light and Gibberellins in Expression of Rosa hybrida Vacuolar Invertase 1 Regulation. Plant Cell Physiol. 55, 1734–1748. doi: 10.1093/pcp/pcu106
Rajagopalan, R., Vaucheret, H., Trejo, J., and Bartel, D. P. (2006). A diverse and evolutionarily fluid set of microRNAs in Arabidopsis thaliana. Genes Dev. 20, 3407–3425. doi: 10.1101/gad.1476406
Rameau, C., Bertheloot, J., Leduc, N., Andrieu, B., Foucher, F., and Sakr, S. (2015). Multiple pathways regulate shoot branching. Front. Plant Sci. 5:741. doi: 10.3389/fpls.2014.00741
Raymond, O., Gouzy, J., Just, J., Badouin, H., Verdenaud, M., Lemainque, A., et al. (2018). The Rosa genome provides new insights into the domestication of modern roses. Nat. Genet. 50, 772–777. doi: 10.1038/s41588-018-0110-3
Reyes, J. L., and Chua, N.-H. (2007). ABA induction of miR159 controls transcript levels of two MYB factors during Arabidopsis seed germination: miR159 regulation of ABA responses during germination. Plant J. 49, 592–606. doi: 10.1111/j.1365-313X.2006.02980.x
Roman, H., Girault, T., Barbier, F., Péron, T., Brouard, N., Pìnčík, A., et al. (2016). Cytokinins Are Initial Targets of Light in the Control of Bud Outgrowth. Plant Physiol. 172, 489–509. doi: 10.1104/pp.16.00530
Roman, H., Girault, T., Le Gourrierec, J., and Leduc, N. (2017). In silico analysis of 3 expansin gene promoters reveals 2 hubs controlling light and cytokinins response during bud outgrowth. Plant Signal. Behav. 12:e1284725. doi: 10.1080/15592324.2017.1284725
Romanowski, A., Furniss, J. J., Hussain, E., and Halliday, K. J. (2021). Phytochrome regulates cellular response plasticity and the basic molecular machinery of leaf development. Plant Physiol. 186, 1220–1239. doi: 10.1093/plphys/kiab112
Sagi, M., Davydov, O., Orazova, S., Yesbergenova, Z., Ophir, R., Stratmann, J. W., et al. (2004). Plant Respiratory Burst Oxidase Homologs Impinge on Wound Responsiveness and Development in Lycopersicon esculentum [W]. Plant Cell 16, 616–628. doi: 10.1105/tpc.019398
Sánchez-Retuerta, C., Suaréz-López, P., and Henriques, R. (2018). Under a New Light: regulation of Light-Dependent Pathways by Non-coding RNAs. Front. Plant Sci. 9:962. doi: 10.3389/fpls.2018.00962
Schneider, A., Godin, C., Boudon, F., Demotes-Mainard, S., Sakr, S., and Bertheloot, J. (2019). Light Regulation of Axillary Bud Outgrowth Along Plant Axes: an Overview of the Roles of Sugars and Hormones. Front. Plant Sci. 10:1296. doi: 10.3389/fpls.2019.01296
Schulze, S., Schäfer, B. N., Parizotto, E. A., Voinnet, O., and Theres, K. (2010). LOST MERISTEMS genes regulate cell differentiation of central zone descendants in Arabidopsis shoot meristems: LOM genes control meristem maintenance. Plant J. 64, 668–678. doi: 10.1111/j.1365-313X.2010.04359.x
Shimizu, S., and Mori, H. (1998). Analysis of Cycles of Dormancy and Growth in Pea Axillary Buds Based on mRNA Accumulation Patterns of Cell Cycle-Related Genes. Plant Cell Physiol. 39, 255–262. doi: 10.1093/oxfordjournals.pcp.a029365
Singh, A., Singh, S., Panigrahi, K. C. S., Reski, R., and Sarkar, A. K. (2014). Balanced activity of microRNA166/165 and its target transcripts from the class III homeodomain-leucine zipper family regulates root growth in Arabidopsis thaliana. Plant Cell Rep. 33, 945–953. doi: 10.1007/s00299-014-1573-z
Sudawan, B., Chang, C.-S., Chao, H., Ku, M. S. B., and Yen, Y. (2016). Hydrogen cyanamide breaks grapevine bud dormancy in the summer through transient activation of gene expression and accumulation of reactive oxygen and nitrogen species. BMC Plant Biol. 16:202. doi: 10.1186/s12870-016-0889-y
Sun, W., Xu, X. H., Wu, X., Wang, Y., Lu, X., Sun, H., et al. (2015). Genome-wide identification of microRNAs and their targets in wild type and phyB mutant provides a key link between microRNAs and the phyB-mediated light signaling pathway in rice. Front. Plant Sci. 6:372. doi: 10.3389/fpls.2015.00372
Sun, Z., Li, M., Zhou, Y., Guo, T., Liu, Y., Zhang, H., et al. (2018). Coordinated regulation of Arabidopsis microRNA biogenesis and red light signaling through Dicer-like 1 and phytochrome-interacting factor 4. PLoS Genet. 14:e1007247. doi: 10.1371/journal.pgen.1007247
Sun, Z., Su, C., Yun, J., Jiang, Q., Wang, L., Wang, Y., et al. (2019). Genetic improvement of the shoot architecture and yield in soya bean plants via the manipulation of GmmiR156b. Plant Biotechnol. J. 17, 50–62. doi: 10.1111/pbi.12946
Sunkar, R., Girke, T., Jain, P. K., and Zhu, J.-K. (2005). Cloning and Characterization of MicroRNAs from Rice. Plant Cell 17, 1397–1411. doi: 10.1105/tpc.105.031682
Sussex, I. M., and Kerk, N. M. (2001). The evolution of plant architecture. Curr. Opin. Plant Biol. 4, 33–37. doi: 10.1016/S1369-5266(00)00132-1
Suttle, J. C. (2009). Ethylene Is Not Involved in Hormone- and Bromoethane-Induced Dormancy Break in Russet Burbank Minitubers. Am. J. Potato Res. 86, 278–285. doi: 10.1007/s12230-009-9081-3
Tang, X., Bian, S., Tang, M., Lu, Q., Li, S., Liu, X., et al. (2012). MicroRNA–Mediated Repression of the Seed Maturation Program during Vegetative Development in Arabidopsis. PLoS Genet. 8:e1003091. doi: 10.1371/journal.pgen.1003091
Tian, C., Zhang, X., He, J., Yu, H., Wang, Y., Shi, B., et al. (2014). An organ boundary-enriched gene regulatory network uncovers regulatory hierarchies underlying axillary meristem initiation. Mol. Syst. Biol. 10:755. doi: 10.15252/msb.20145470
Tian, L., Liu, H., Ren, L., Ku, L., Wu, L., Li, M., et al. (2018). MicroRNA 399 as a potential integrator of photo-response, phosphate homeostasis, and sucrose signaling under long day condition. BMC Plant Biol 18:290. doi: 10.1186/s12870-018-1460-9
Tucker, D. J. (1976). ENDOGENOUS GROWTH REGULATORS IN RELATION TO SIDE SHOOT DEVELOPMENT IN THE TOMATO. New Phytol. 77, 561–568. doi: 10.1111/j.1469-8137.1976.tb04647.x
Vanneste, S., and Friml, J. (2009). Auxin: a Trigger for Change in Plant Development. Cell 136, 1005–1016. doi: 10.1016/j.cell.2009.03.001
Vergara, R., Parada, F., Rubio, S., and Pérez, F. J. (2012). Hypoxia induces H2O2 production and activates antioxidant defence system in grapevine buds through mediation of H2O2 and ethylene. J. Exp. Bot. 63, 4123–4131.
Voinnet, O. (2009). Origin, Biogenesis, and Activity of Plant MicroRNAs. Cell 136, 669–687. doi: 10.1016/j.cell.2009.01.046
Walton, E. F., Wu, R.-M., Richardson, A. C., Davy, M., Hellens, R. P., Thodey, K., et al. (2009). A rapid transcriptional activation is induced by the dormancy-breaking chemical hydrogen cyanamide in kiwifruit (Actinidia deliciosa) buds. J. Exp. Bot. 60, 3835–3848. doi: 10.1093/jxb/erp231
Wang, C.-Y., Chen, Y.-Q., and Liu, Q. (2011). Sculpting the meristem: the roles of miRNAs in plant stem cells. Biochem. Biophys. Res. Commun. 409, 363–366. doi: 10.1016/j.bbrc.2011.04.123
Wang, H., and Wang, H. (2015). The miR156/SPL Module, a Regulatory Hub and Versatile Toolbox, Gears up Crops for Enhanced Agronomic Traits. Mol. Plant 8, 677–688. doi: 10.1016/j.molp.2015.01.008
Wang, J.-W., Czech, B., and Weigel, D. (2009). miR156-Regulated SPL Transcription Factors Define an Endogenous Flowering Pathway in Arabidopsis thaliana. Cell 138, 738–749. doi: 10.1016/j.cell.2009.06.014
Wang, L., Mai, Y.-X., Zhang, Y.-C., Luo, Q., and Yang, H.-Q. (2010). MicroRNA171c-Targeted SCL6-II, SCL6-III, and SCL6-IV Genes Regulate Shoot Branching in Arabidopsis. Mol. Plant 3, 794–806. doi: 10.1093/mp/ssq042
Wang, L., Sun, S., Jin, J., Fu, D., Yang, X., Weng, X., et al. (2015). Coordinated regulation of vegetative and reproductive branching in rice. Proc. Natl. Acad. Sci. U. S. A. 112, 15504–15509. doi: 10.1073/pnas.1521949112
Wang, M., Le Moigne, M.-A., Bertheloot, J., Crespel, L., Perez-Garcia, M.-D., Ogé, L., et al. (2019a). BRANCHED1: a Key Hub of Shoot Branching. Front. Plant Sci. 10:76. doi: 10.3389/fpls.2019.00076
Wang, M., Ogé, L., Voisine, L., Perez-Garcia, M.-D., Jeauffre, J., Hibrand Saint-Oyant, L., et al. (2019b). Posttranscriptional Regulation of RhBRC1 (Rosa hybrida BRANCHED1) in Response to Sugars is Mediated via its Own 3′ Untranslated Region, with a Potential Role of RhPUF4 (Pumilio RNA-Binding Protein Family). Int. J. Mol. Sci. 20:3808. doi: 10.3390/ijms20153808
Wang, M., Pérez-Garcia, M.-D., Davière, J.-M., Barbier, F., Ogé, L., Gentilhomme, J., et al. (2021). Outgrowth of the axillary bud in rose is controlled by sugar metabolism and signalling. J. Exp. Bot. 72, 3044–3060. doi: 10.1093/jxb/erab046
Wang, M., Zang, L., Jiao, F., Perez-Garcia, M.-D., Ogé, L., Hamama, L., et al. (2020). Sugar Signaling and Post-transcriptional Regulation in Plants: an Overlooked or an Emerging Topic? Front. Plant Sci. 11:578096. doi: 10.3389/fpls.2020.578096
Wang, R., Yang, X., Guo, S., Wang, Z., Zhang, Z., and Fang, Z. (2021). MiR319-targeted OsTCP21 and OsGAmyb regulate tillering and grain yield in rice. J. Integr. Plant Biol. 63, 1260–1272. doi: 10.1111/jipb.13097
Wang, S., Liu, S., Liu, L., Li, R., Guo, R., Xia, X., et al. (2020). miR477 targets the phenylalanine ammonia-lyase gene and enhances the susceptibility of the tea plant (Camellia sinensis) to disease during Pseudopestalotiopsis species infection. Planta 251:59. doi: 10.1007/s00425-020-03353-x
Wang, Y., Wang, Z., Amyot, L., Tian, L., Xu, Z., Gruber, M. Y., et al. (2015). Ectopic expression of miR156 represses nodulation and causes morphological and developmental changes in Lotus japonicus. Mol. Genet. Genomics 290, 471–484. doi: 10.1007/s00438-014-0931-4
Weber, M. J. (2004). New human and mouse microRNA genes found by homology search: new human and mouse microRNA gene. FEBS J. 272, 59–73. doi: 10.1111/j.1432-1033.2004.04389.x
Wei, S., Gruber, M. Y., Yu, B., Gao, M.-J., Khachatourians, G. G., Hegedus, D. D., et al. (2012). Arabidopsis mutant sk156 reveals complex regulation of SPL15 in a miR156-controlled gene network. BMC Plant Biol. 12:169. doi: 10.1186/1471-2229-12-169
Wei, S., Yu, B., Gruber, M. Y., Khachatourians, G. G., Hegedus, D. D., and Hannoufa, A. (2010). Enhanced Seed Carotenoid Levels and Branching in Transgenic Brassica napus Expressing the Arabidopsis miR156b Gene. J. Agric. Food Chem. 58, 9572–9578. doi: 10.1021/jf102635f
Wightman, B., Ha, I., and Ruvkun, G. (1993). Posttranscriptional regulation of the heterochronic gene lin-14 by lin-4 mediates temporal pattern formation in C. elegans. Cell 75, 855–862. doi: 10.1016/0092-8674(93)90530-4
Wu, G. (2013). Plant MicroRNAs and Development. J. Genet. Genomics 40, 217–230. doi: 10.1016/j.jgg.2013.04.002
Xia, K., Wang, R., Ou, X., Fang, Z., Tian, C., Duan, J., et al. (2012). OsTIR1 and OsAFB2 Downregulation via OsmiR393 Overexpression Leads to More Tillers, Early Flowering and Less Tolerance to Salt and Drought in Rice. PLoS One 7:e30039. doi: 10.1371/journal.pone.0030039
Xie, Y., Liu, Y., Wang, H., Ma, X., Wang, B., Wu, G., et al. (2017). Phytochrome-interacting factors directly suppress MIR156 expression to enhance shade-avoidance syndrome in Arabidopsis. Nat. Commun. 8:348. doi: 10.1038/s41467-017-00404-y
Xu, M., Hu, T., Zhao, J., Park, M.-Y., Earley, K. W., Wu, G., et al. (2016). Developmental Functions of miR156-Regulated SQUAMOSA PROMOTER BINDING PROTEIN-LIKE (SPL) Genes in Arabidopsis thaliana. PLoS Genet. 12:e1006263. doi: 10.1371/journal.pgen.1006263
Yan, Y., Wei, M., Li, Y., Tao, H., Wu, H., Chen, Z., et al. (2021). MiR529a controls plant height, tiller number, panicle architecture and grain size by regulating SPL target genes in rice (Oryza sativa L.). Plant Sci. 302:110728. doi: 10.1016/j.plantsci.2020.110728
Yang, T., Wang, Y., Teotia, S., Zhang, Z., and Tang, G. (2018). The Making of Leaves: how Small RNA Networks Modulate Leaf Development. Front. Plant Sci. 9:824. doi: 10.3389/fpls.2018.00824
Yue, E., Li, C., Li, Y., Liu, Z., and Xu, J.-H. (2017). MiR529a modulates panicle architecture through regulating SQUAMOSA PROMOTER BINDING-LIKE genes in rice (Oryza sativa). Plant Mol. Biol. 94, 469–480. doi: 10.1007/s11103-017-0618-4
Zhan, J., Chu, Y., Wang, Y., Diao, Y., Zhao, Y., Liu, L., et al. (2021). The miR164-GhCUC2-GhBRC1 module regulates plant architecture through abscisic acid in cotton. Plant Biotechnol. J. 19, 1839–1851. doi: 10.1111/pbi.13599
Zhang, B., Pan, X., Cobb, G. P., and Anderson, T. A. (2006). Plant microRNA: a small regulatory molecule with big impact. Dev. Biol. 289, 3–16. doi: 10.1016/j.ydbio.2005.10.036
Zhang, H., He, H., Wang, X., Wang, X., Yang, X., Li, L., et al. (2011). Genome-wide mapping of the HY5-mediated genenetworks in Arabidopsis that involve both transcriptional and post-transcriptional regulation: regulation of HY5-mediated gene networks. Plant J. 65, 346–358. doi: 10.1111/j.1365-313X.2010.04426.x
Zhang, Y.-C., Yu, Y., Wang, C.-Y., Li, Z.-Y., Liu, Q., Xu, J., et al. (2013). Overexpression of microRNA OsmiR397 improves rice yield by increasing grain size and promoting panicle branching. Nat. Biotechnol. 31, 848–852. doi: 10.1038/nbt.2646
Zhang, Z., and Zhang, X. (2012). Argonautes compete for miR165/166 to regulate shoot apical meristem development. Curr. Opin. Plant Biol. 15, 652–658. doi: 10.1016/j.pbi.2012.05.007
Zhao, J. P., Jiang, X. L., Zhang, B. Y., and Su, X. H. (2012). Involvement of microRNA-mediated gene expression regulation in the pathological development of stem canker disease in Populus trichocarpa. PLoS One 7:e44968. doi: 10.1371/journal.pone.0044968
Zhao, Q., Nakashima, J., Chen, F., Yin, Y., Fu, C., Yun, J., et al. (2013). LACCASE Is Necessary and Nonredundant with Peroxidase for Lignin Polymerization during Vascular Development in Arabidopsis. Plant Cell 25, 3976–3987. doi: 10.1105/tpc.113.117770
Zhao, Y., Wen, H., Teotia, S., Du, Y., Zhang, J., Li, J., et al. (2017). Suppression of microRNA159 impacts multiple agronomic traits in rice (Oryza sativa L.). BMC Plant Biol. 17:215. doi: 10.1186/s12870-017-1171-7
Zhou, B., Fan, P., Li, Y., Yan, H., and Xu, Q. (2016). Exploring miRNAs involved in blue/UV-A light response in Brassica rapa reveals special regulatory mode during seedling development. BMC Plant Biol. 16:111. doi: 10.1186/s12870-016-0799-z
Zhou, M., Li, D., Li, Z., Hu, Q., Yang, C., Zhu, L., et al. (2013). Constitutive Expression of a miR319 Gene Alters Plant Development and Enhances Salt and Drought Tolerance in Transgenic Creeping Bentgrass. Plant Physiol. 161, 1375–1391. doi: 10.1104/pp.112.208702
Zhou, Y., Yan, A., Han, H., Li, T., Geng, Y., Liu, X., et al. (2018). HAIRY MERISTEM with WUSCHEL confines CLAVATA3 expression to the outer apical meristem layers. Science 361, 502–506. doi: 10.1126/science.aar8638
Keywords: branching, light control, lateral meristem, post-transcriptional regulation, small RNAs, rose
Citation: Mallet J, Laufs P, Leduc N and Le Gourrierec J (2022) Photocontrol of Axillary Bud Outgrowth by MicroRNAs: Current State-of-the-Art and Novel Perspectives Gained From the Rosebush Model. Front. Plant Sci. 12:770363. doi: 10.3389/fpls.2021.770363
Received: 03 September 2021; Accepted: 13 December 2021;
Published: 31 January 2022.
Edited by:
Turgay Unver, FicusBio, TurkeyReviewed by:
Marcelo Carnier Dornelas, State University of Campinas, BrazilLili Zhuang, Nanjing Agricultural University, China
Munevver Dogramaci, Edward T. Schafer Agricultural Research Center, United States Department of Agriculture, Agricultural Research Service (USDA-ARS), United States
Copyright © 2022 Mallet, Laufs, Leduc and Le Gourrierec. This is an open-access article distributed under the terms of the Creative Commons Attribution License (CC BY). The use, distribution or reproduction in other forums is permitted, provided the original author(s) and the copyright owner(s) are credited and that the original publication in this journal is cited, in accordance with accepted academic practice. No use, distribution or reproduction is permitted which does not comply with these terms.
*Correspondence: José Le Gourrierec, am9zZS5nZW50aWxob21tZUB1bml2LWFuZ2Vycy5mcg==