- 1Stress Physiology Phenomics Centre, Department of Botany, University of Karachi, Karachi, Pakistan
- 2Institute of Oceanography, Minjiang University, Fuzhou, China
- 3Muhammad Ajmal Khan Institute of Sustainable Halophyte Utilization, University of Karachi, Karachi, Pakistan
- 4Department of Horticultural Sciences, Faculty of Agriculture and Environment, The Islamia University of Bahawalpur, Bahawalpur, Pakistan
- 5Environmental Horticulture Department and Mid-Florida Research and Education Center, IFAS, University of Florida, Apopka, FL, United States
- 6School of Health and Biomedical Sciences, Discipline of Laboratory Medicine, RMIT University, Melbourne, VIC, Australia
The present study aimed to witness the plant–microbe interaction associated with salt tolerance in crops. We isolated the endophytic microbe from the root zone of halophytic grass. Later, the salt tolerance of the endophyte was tested in the saline medium and was identified using nucleotide sequencing (GenBank under the accession numbers: SUB9030920 AH1_AHK_ITS1 MW570850: SUB9030920 AH1_AHK_ITS4 MW570851). Rice and maize seeds were coated with identified endophyte Aspergillus terreus and were sown in separate plastic pots. Later 21-day-old seedlings were subjected to three NaCl concentrations, including 50, 100, and 150 mM salt stress. Under saline conditions, A. terreus showed a substantial increase in growth, biomass, relative water content, oxidative balance, and photochemical efficiency of rice and maize plants. The data reflected that the stimulation of gibberellic acid (GA) in treated leaves may be the main reason for the upregulation of photosynthesis and the antioxidant defense cascade. The data also depict the downregulation of oxidative damage markers malondialdehyde, hydrogen peroxide in rice and maize plants. Conclusively, salt-tolerant endophytic fungus A. terreus explicitly displayed the positive plant–microbe interaction by developing salt tolerance in rice and maize plants. Salt tolerance by endophytic fungus coincides with the enhanced GA concentration, which illustrated the stimulated physiological mechanism and gene in response to the extreme environmental crisis, resulting in improved crop productivity.
Introduction
Exposure to extreme climatic episodes is a serious problem for plant survival in arid or semi-arid regions worldwide. In the last several decades, these drastic changes in the environment and increases in the population have negatively impacted agricultural productivity. Pitman and Lauchli (2002) reported that crop loss due to salinity is challenging to measure, but it is anticipated to increase by 2050. Similarly, abiotic stress, such as salinity and drought, exacerbates these conditions and has been shown to substantially decline agricultural production (Tavakkoli et al., 2011; Abideen et al., 2020). Among the abiotic stress, salinity has affected nearly 1,128 million hectares of land (Wicke et al., 2011). It has previously been reported that crop plants at all stages of their life are sensitive to arid and saline areas, reducing growth (Nawaz et al., 2010). For example, monocot crops such as maize (Zea mays L.) and rice (Oryza sativa L.) can be considered as the most sensitive or moderately sensitive crops for suboptimal dry saline lands (Shafi et al., 2013). It is known that long-term saline irrigation causes adverse effects on plant physiology and the production of plant metabolites that ultimately affect the total biomass production (Hussain et al., 2019).
In the last few decades, scientists have been searching for sustainable solutions to mitigate salt-related consequences in crops to enhance yield. Several studies have recently suggested alternative and innovative ideas protect plants from the adverse effects of salinity stress (Yu et al., 2019). One strategy is to explore endophytic fungi, which establish a symbiotic relationship with the host plant by providing plant pathogen preventions and synthesis of nutrients that offer favorable conditions to resist sodium chloride (NaCl) toxicity in plants (Afzal et al., 2013; Yu et al., 2019). Physiologically, reactive oxygen species (ROS) increase under salt stress (Majeed et al., 2010; Umar and Siddiqui, 2018). Studies have shown that endophytic fungi can produce biologically active and structurally diverse natural-for-plant defense systems against environmental stresses (Schulz, 2002; Bilal et al., 2019; Yu et al., 2019).
Improving agricultural technology is the only solution to overcome the food demand in a saline environment. Sustainable management practices, genetic engineering, traditional breeding, biofertilizers, and micro-organisms are being used to reduce the saline impact in important crop plants (Tscharntke et al., 2012; Cho et al., 2014; Kamkar, 2016; Yasmeen and Siddiqui, 2018). Planting salt-tolerant crop cultivars had limited success despite significant efforts. Studies suggested that soil micro-organisms are better for increasing soil fertility and crop yield under a saline environment (Vejan et al., 2016; Yasmeen and Siddiqui, 2018). Endophytic fungi are the organisms inhabited in the rhizosphere plants and cultured from almost all crop and wild plants (Ananda and Sridhar, 2002; Wolf and Rashid, 2008). The roots of halophytes also make a symbiotic relationship with fungi. These fungi are considered to be salt tolerant as they are present in saline habitats. It has previously been reported that some fungi play a crucial role in enhancing abiotic stress tolerance in plants (Waqas et al., 2012; Bilal et al., 2019; Tisarum et al., 2020), but their ability to tolerate salt stress in maize and rice is limited. It was suggested that endophytic fungi of saline habitat could enhance salt tolerance, likely due to the symbiotic relationship with the host plant by optimizing water and improving physiological performance under stress conditions. However, little information exists regarding the fungal endophyte ecological, physiological behavior, and growth responses to alleviate salt stress in most essential food crops such as rice and maize (Tisarum et al., 2020).
Rice and maize are the most important cereal crops widely cultivated after wheat (Farooq et al., 2015). The world production of maize has reached 1.15 billion tons and 782 million tons for rice. Rice and maize are grown in a wide range of soil and extreme climatic conditions. Both are important crop plants in the Poaceae family which is moderately sensitive to salt stress (Chinnusamy et al., 2005). Furthermore, broad intraspecific genetic variation for salt resistance exists in rice and maize (Mansour et al., 2005).
A halophyte is a group of plants naturally living in a saline habitat that show diverse growth responses to increasing salinity, ranging from inhibition to dramatic stimulation. All halophytes display a range of salt tolerance with a common feature regulating cellular Na+, Cl–, and K+ concentrations to adjust external water potential. The genus Aspergillus is a filamentous endophytic fungus, which is common in soil and plants (Yoo et al., 2018). Due to its minimal nutritional requirements, this fungus can grow on organic and even inorganic materials contaminated by trace organic compounds. It was previously observed that biocontrol agents, i.e., A. terreus promoted plant growth (Waqas et al., 2015; Halo et al., 2018). Endophytes living in the plant’s rhizosphere living in a saline habitat could provide natural remedies and establish salt tolerance in crop plants. The modulation of physiological and biochemical cascade owing to the stress tolerance by the applied endophyte (A. terreus) was studied in the present research. The current study exploited the information obtained from the physiological and biochemical attributes that witness the microbes (endophyte)-associated metabolic modification, which spells out the novelty of the current approach. The study also enhanced the level of understanding regarding the photochemical attributes during the induction of salt tolerance by endophytes. Therefore, the aim of the present study not only was to isolate and identify the endophyte from the saline habitat but also to scrutinize the effect of salt-tolerant endophytes to mitigate salt stress in two different crop plants belonging to the same family. Furthermore, this study also provides comparative physiological and biochemical details in this regard. It was assumed that endophytes from the halophyte rhizosphere may be more salt resistant and it was checked by in vitro experiment.
Materials and Methods
Seed Selection
Rice (Oryza sativa L.) var. Kernel and maize (Zea mays L.) var. NT6621 was obtained in August 2017, from the Plant Protection Department, Karachi, Pakistan. Forty seeds of each rice and maize were surface sterilized in 100 ml of 10% concentrated sodium hypochlorite solution in a beaker for 3 min and washed meticulously by using autoclaved distilled water (100 ml) three times before the beginning of the experiment.
Culture Collection and Their Purification
The Aspergillus terreus was collected from the Cenchrus ciliaris L. root zone and was later purified in the Botany Department, University of Karachi (Figure 1). The soil samples were transferred to the laboratory in plastic bags, placed on moist Whatman’s filter paper (sterile) in Petri-plates, and then kept in a humid chamber at 28-30°C for 7 days. The soil dilution plate method was used for isolation and purification. In this regard, the soil was suspended in sterilized water, making a 10% suspension, maintained through agitation using a shaker for 5 min. Later, a series of dilutions (1/10, 1/100, 1/1,000….) was prepared using the suspension until the desired final dilution was achieved. Finally, a dilution factor of 104 to 106 was considered suitable for isolating fungi and bacteria. Aliquots of the final zoospore suspension were prepared by using the dilution method as previously described by Shelford et al. (2005, 2006) using the following equation.
Potato dextrose agar (PDA) was used to culture the fungus on petri-dishes at 28°C for 7 days and checked every 2 days for the emergence of endophytic fungi. The pH of the PDA was maintained to 5.6 before inoculation. The conidia were sub-cultured in a 500-ml flask containing 100 ml of the medium containing 30 g glucose, 3 g yeast extract, 0.39 g KH2PO4, 1.42 g Na2HPO4.12H2O, 0.060 g MgSO4.7H2O, 0.70 g NH4NO3, and 1.0 g KCl per liter (Fargues et al., 1992). A. terreus culture was prepared using PDA containing 100, 200, and 300 mM NaCl to examine the salt tolerance capacity (Figure 1). The isolate of A. terreus at 300 mM NaCl was incubated at 28-30°C for 24-98 h for further experiments. Subsequently, both rice and maize seeds were coated with A. terreus using 2% gum-Arabic adhesive. The colony-forming unit was maintained at 46.3 × 10–6 conidia/mL of A. terreus.
Isolation and DNA Extraction From Aspergillus terreus
To identify the A. terreus, total genomic DNA was extracted from the mycelium (Lee and Taylor, 1990). The internal transcribed spacer (ITS) regions were amplified using the primer pairs (White et al., 1990; Hong et al., 2005). The temperature profile for the ITS was an initial denaturation step at 95°C (10 min), 35 cycles of denaturation for 30 s at 95°C, annealing at 55°C (30 s) and extension at 72°C (90 s), and final extension step of 10 min at 72°C. The sequencing and purification of PCR products were carried out at Macrogen, Korea. Finally, sequences generated from the analysis were submitted in GenBank under the accession numbers: SUB9030920 AH1_AHK_ITS1 MW570850: SUB9030920 AH1_AHK_ITS4 MW570851.
Pot Experiments
Rice and maize seeds were sown in separate plastic pots; each treatment had four pots or replicates (n = 4) filled with 500 g of sterile sandy loam soil (9% clay,17% silt, and 74% sand). The experiment was conducted at the Stress Physiology Phenomic Centre, Department of Botany, at the University of Karachi at ambient environmental conditions. The experiments outlined in this research were carried out in a randomized design to assess the impact of the endophytic fungus on host plants. Before the sowing of seeds, the soil was autoclaved to avoid any microbial contamination. Before the experiment, 4.10% soil organic carbon and 0.83% total nitrogen were recorded. For soil organic content measurement, 1 g of soil sample was transferred into a 250-ml conical flask having 10 ml of each 1 N potassium dichromate and concentrated H2SO4. After 30 min, 50 ml of deionized water, 3 ml of concentrated H3PO4, and 0.5 ml of 1% diphenylamine indicator were added. Then, titrated slowly with 1 N FeSO4 solution up to a green color was achieved. Total nitrite and nitrate-N were determined by acid digestion. For the estimation, the extracted supernatant is reacted with sulfanilic acid and methyl anthranilate as coupling agents to form an azo dye, which is measured at 493 nm using Shimadzu UV–Vis spectrophotometer (pH 7.6 and EC 1.7 dS/m) were recorded using a soil sensor (SDI-12 hydra probe II, Stevens Water, United States) before the beginning of the experiment. For salinity treatments, 150, 100, and 50 mM NaCl concentrations were applied in gradual increments, which are almost equal to 14.5, 9.7, and 4.4 dS/m. A NaCl solution was applied to 21-day-old seedlings using 25 mM NaCl each day, and soil moisture was maintained regularly. Three NaCl levels, including 50, 100, and 150 mM, were implemented by gradual increment, that is, 25 mM NaCl per day, until the desired concentration was achieved. After that, more than 70% soil moisture content was maintained using 60 ± 5 ml distilled water in respective pots on alternate days. Soil moisture contents and electrical conductivity were measured using the soil sensor SDI-12 hydra probe II. Plants without A. terreus treatments were rinsed with distilled water and served as control plants. Each experimental setup was exposed under an average day–night temperature (25 ± 4°C and 15 ± 3°C), respectively. Root, shoot length, biomass, physiological parameters, and antioxidant enzymes activities were determined.
Harvest and Growth Parameters
After 2 weeks of salt exposure, plants were harvested, and growth parameters, such as root, shoot length, and biomass, were determined. Plant material was dried in an oven at 80°C for 24 h. The fresh leaves of each plant were immediately frozen in liquid nitrogen and stored at -20°C for further biochemical analyses.
Extraction and Estimation of Total Pigments
Five hundred milligrams of fresh leaves were ground in 10 ml of 96% methanol (Batch # 322415 Sigma–Aldrich) and then centrifuged at 4,000 rpm for 10 min. Chlorophyll a (Ca), chlorophyll b (Cb), total chlorophyll [Chl(a+b)], and total carotenoids (Cx+c) were determined according to the method previously outlined in Lichtenthaler (1987). The supernatant (2 ml) was collected, and the absorbance was read at 666, 653, and 470 nm on the spectrophotometer, respectively (UV-1100, Roctec, China). The levels of these pigments were calculated according to the following formulas.
Where, Ca = Chlorophyll a, Cb = Chlorophyll b, Chl(a+b) = total chlorophyll, Cx+c = Total carotenoids.
Quantum Yield Photochemical Efficiency and Stomatal Conductance
The measurement of chlorophyll fluorescence emission from 20 randomly selected young leaves of each rice and maize were monitored using a chlorophyll fluorescence meter, model OS-30p+ (Opti-Science, United States), before harvesting. A leaf, adapted to dark conditions for 30 min using leaf clips, was initially exposed to the modulated measuring beam of far-red light (LED source with a typical peak at wavelength 735 nm). The original (Fo) and maximum (Fm) fluorescence yields were measured under a weak modulated red light (0.5 μmol/m2/s) with 1.6 s pulses of saturating light (6.8 μmol/m2/s PAR). The variable fluorescence yield (Fv) was calculated using the equation Fm–Fo. The ratio of the variable to maximum fluorescence (Fv/Fm) was calculated as the dark-adapted quantum yield of photochemical efficiency photochemistry, and performance index and non-photochemical quenching were calculated as previously described by Maxwell and Johnson (2000). Similarly, stomatal conductance of 20 randomly selected leaves of each treated and control plants of rice and maize were examined by taking the middle portion on the lower surface of the leaves using a leaf porometer (Model SC-1, Decagon).
Free Proline Determination
The proline content was assessed according to the methods previously described by Bates et al. (1973). Leaf samples (0.5 g) were homogenized in 5 ml sulfosalicylic acid (3% w/v) and the homogenate was then filtered using a Whatman #2 filter paper. Subsequently, 2 ml of extract, 2 ml of glacial acetic acid, and 2 ml of acid ninhydrin (1.25 g ninhydrin in 30 ml concentrated glacial acetic acid and 20 mL 6 M phosphoric acid) were added. They were heated in a boiling water bath at 100°C for 1 h. As soon as an observable brick red color developed, the reaction mixture was cooled. Four milliliters of toluene were mixed vigorously with a test tube stirrer for 15–20 s to extract the reaction mixture. The toluene with chromophores was subsequently separated, and absorbance was recorded at 520 nm against the toluene blank. The proline contents were calculated using the following formula:
Hydrogen Peroxide Content
Change in hydrogen peroxide content was measured according to the method previously described by Velikova et al. (2000). A 100 mg of freshly harvested leaf samples were homogenized in 3 ml of 0.1% (w/v) trichloroacetic acid in a chilled water bath and then centrifuged at 12,000 g for 15 min. Later, 0.5 ml of 10 mM phosphate buffer (pH 7.0) and 1 ml of 1 M potassium iodide (KI) were added to 0.5 ml of the supernatant. The change in the absorbance of the supernatant was read at 390 nm.
Leaf Relative Water Content
Randomly, four-leaf strips of 4 × 2 cm2 of rice and 1.2 cm2 of maize from the mid-veins and the edge were excised, and fresh weights (FW) were determined. For turgid weight (TW), leaves were left in an airtight 90-mm plastic Petri plate filled with distilled water for 12 h. Samples were then dried at 80°C for 48 h in the oven and determined dry weight (DW). The relative water content was determined using the method previously described by Barrs and Weatherley (1962) calculated using the following formula:
Malondialdehyde Content
Measurements of lipid peroxidation in the leaf tissue were determined as the amount of malondialdehyde (MDA) produced by the thiobarbituric acid (TBA) reaction (Dhindsa et al., 1981). Fresh leaves (0.25 g) were homogenized with 0.1% trichloroacetic acid (TCA) in a pestle and mortar and then centrifuged at 10,000 × g for 5 min. One milliliter supernatant was taken, and 4 ml of 20% TCA containing 0.5% TBA was subsequently added. This mixture was heated (95°C) for 30 min in a water bath and immediately cooled. The absorbance of the clear solution was read at 532 and 600 nm. The concentration of MDA was calculated using its extinction coefficient of 155/mM/cm.
Enzyme Assays
Five hundred milligrams of leaves were crushed and homogenized in prechilled pestle and mortar in 10 ml of protein extraction buffer containing Tris-HCl pH 6.8, 50 mg polyvinylpyrrolidone, and 0.05 mM ethylenediaminetetraacetic acid (EDTA). The homogenate was centrifuged at 12,000 rpm for 10 min using a Smart R-17, Hanil centrifuge machine. The supernatant (5 ml) was collected and used to determine the activities of catalase (CAT), ascorbate peroxidase (APX), and superoxide dismutase (SOD). The total protein was measured using the Bradford assay as previously described by Bradford (1976).
Assay of Catalase Activity
Catalase (EC 1.11.1.6) activity was estimated using the method previously described by Patterson et al. (1984). The decomposition of H2O2 was measured at 240 nm taking Δε as 43.6/mM/cm. The reaction mixture (3.0 ml) consisted of 10.5 mM H2O2 in 0.05 M potassium phosphate buffer (pH 7.0), which was initiated after adding 0.1 ml of the enzyme extract at 25°C. The decrease in absorbance at 240 nm was used to calculate the activity. One unit of CAT activity is defined as the amount of enzyme that catalyzes the conversion of 1 mM of H2O2/min at 25°C.
Assay of Ascorbate Peroxidase Activity
Ascorbate peroxidase (EC 1.11.1.11) activity was carried out using the method previously described by Nakano and Asada (1981). The reaction mixture (2.0 mL) contained 50 mM potassium phosphate buffer (pH 7.0), 0.2 mM EDTA, 0.5 mM ascorbic acid, and 0.25 mM H2O2. The reaction was initiated after the addition of 0.1 ml of the enzyme extract at 25°C. The decrease in absorbance at 290 nm for 1 min was recorded and the amount of ascorbate oxidized was calculated from the extinction coefficient 2.8 mM/cm. The unit of activity was expressed as a micromole of ascorbic acid oxidized per minute at 25°C.
Assay of Superoxide Dismutase Activity
Superoxide dismutase (EC 1.15.1.1) activity was carried out according to the method previously described by Beyer and Fridovich (1987). The reaction mixture consisted of 27 ml of 0.05 M potassium phosphate buffer (pH 7.8), 1.5 ml of L-methionine (300 mg per 2.7 ml), 1.0 ml of nitroblue tetrazolium salt (14.4 mg per 10 ml), and 0.75 ml of Triton X-100. Aliquots (1.0 ml) of this mixture were transferred into glass tubes, followed by adding 20 ml of the enzyme extract and 10 ml of riboflavin (4.4 mg per 100 ml). The reaction mixture was mixed and then illuminated for 15 min in an aluminum foil-lined box containing 25 W fluorescent tubes. The sample was substituted with 20 ml of buffer in the control tube, and the absorbance was measured at 560 nm. The reaction was quenched by switching off the light and placing the tubes in the dark. An increase in the absorbance due to the formation of formazan was measured at 560 nm. Under the described conditions, the increase in absorbance in the control was taken as 100% and the enzyme activity in the samples was calculated by determining the percentage inhibition per minute. One unit of SOD was the amount of enzyme that causes a 50% inhibition of the rate for reduction of the nitroblue tetrazolium salt under the conditions of the assay.
Assay of Guaiacol Peroxidase Activity
Guaiacol peroxidase (GPX; EC 1.11.17) activity was measured spectrophotometrically at 25°C using the method previously described by Tatiana et al. (1999). The reaction mixture (2.0 ml) consisted of 0.05 M potassium phosphate buffer (pH 7.0), 2 mM H2O2, and 2.7 mM guaiacol. The reaction was initiated by the addition of 0.1 ml of the enzyme extract. The initial rate of guaiacol oxidation was measured by the formation rate of tetra guaiacol and was measured at 470 nm (Δε = 26.6 mM/cm). One unit was defined as the amount of enzyme required to catalyze one micromole of hydrogen peroxide conversion, with guaiacol as hydrogen donor, per minute under specified conditions.
Extraction and Estimation of GA3
Leaves from each treatment and control were extracted using ethyl acetate three times (50 + 25 + 25) using Rachev et al. (1993). Subsequently, the extract was separated using anhydrous sodium sulfate (5 g) and evaporated under reduced pressure at 45°C at 15 rpm. The residual extract was re-dissolved in HPLC grade acetonitrile and kept for subsequent thin layer chromatography (TLC) analysis. TLC’s quantitative estimation of gibberellins was carried out using standard gibberellins (Cavell et al., 1967). Benzene: n-butanol:acetic acid (6:3:1) was used as the mobile phase and the developed spots were visualized under UV (254 nm) after spraying with a mixture of ethanol:concentrated sulfuric acid in a (95:5) ratio.
Determination of Na+, K+, and Cl– Ions
Sodium, potassium, and chloride ions were determined in leaf tissues (Chaudhary et al., 1996). Five hundred milligrams of randomly collected dry leaves were homogenized in 10 ml of double-distilled water at 25°C for 10 min. The mixture was centrifuged for 15 min at 3,000 × g and the supernatant filtered through Whatman no.1 filter paper. Subsequently, the filtrate was used to estimate Cl– by titration with the AgNO3 solution using 5% K2CrO4 as an indicator, whereas Na+ and K+ by flame photometer (Jenway, PFP-7).
Statistical Analyses
The data generated from the treated and control groups (n = 4) were subjected to statistical analyses using the software SPSS Version 20 (IBM, United States). A two-way ANOVA was used to determine significant differences among means within and among each treatment. The significance of the applied Bonferroni test has been represented on bar graphs (Figures 2–6). Same letters denoted on bar graphs show non-significant differences (p < 0.05) within a treatment (Capital letters for A. terreus, small letters without A. terreus, only salinity), (*) denotes significant differences, and (ns) denotes non-significant among the treatments (with and without P).
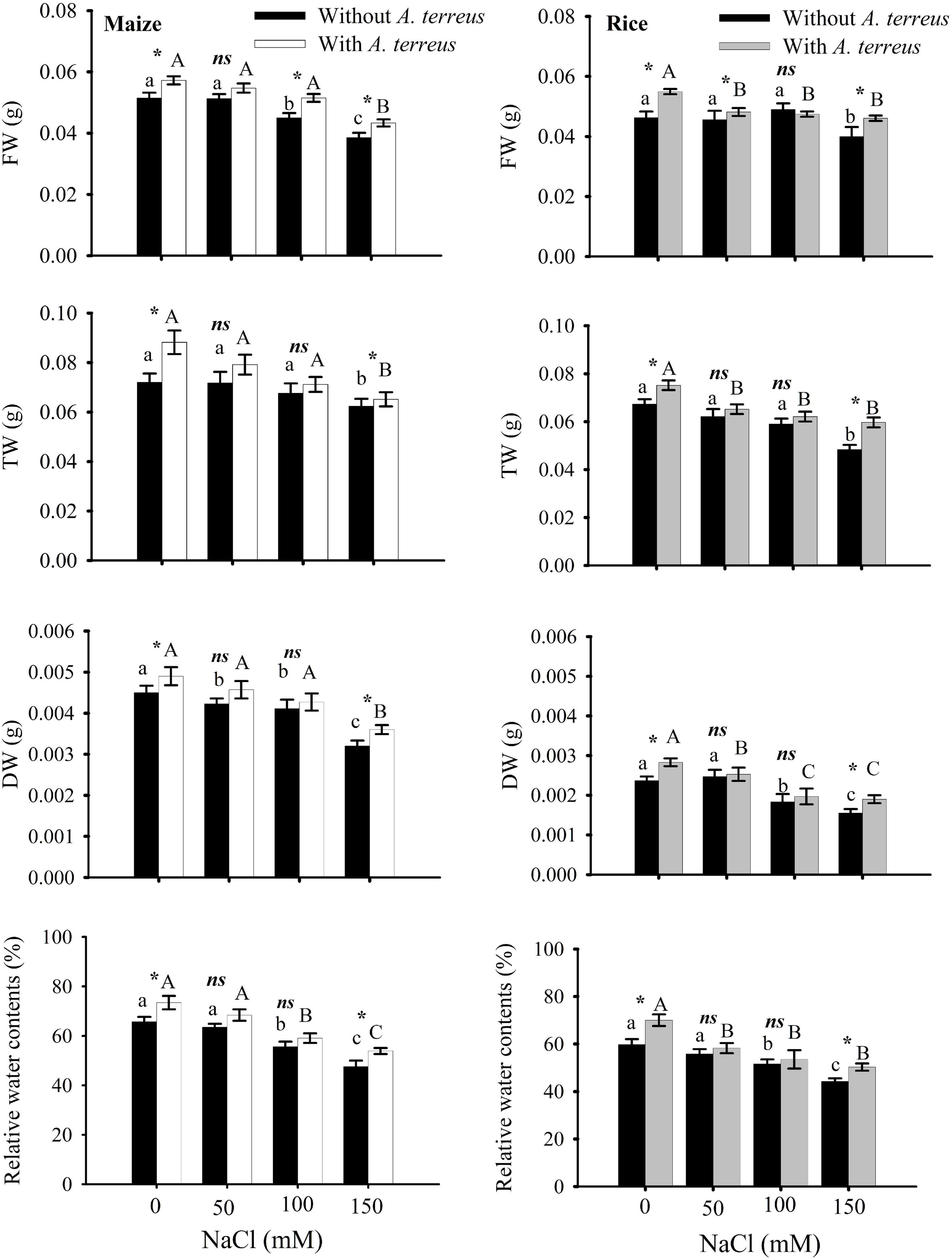
Figure 2. Effect of Aspergillus terreus seed treatments on relative water content and biomass accumulation of maize (Zea mays L.) var. NT6621 and rice (Oryza sativa L.) var. The kernel is in a saline environment. Vertical lines on bar graphs denote mean standard error (±). Same letters on bar graphs denote non-significant differences within a treatment (capital letters for A. terreus, small letters without A. terreus only salinity), (*) denotes significant differences, and (ns) stands for non-significant among the treatments (with and without A. terreus).
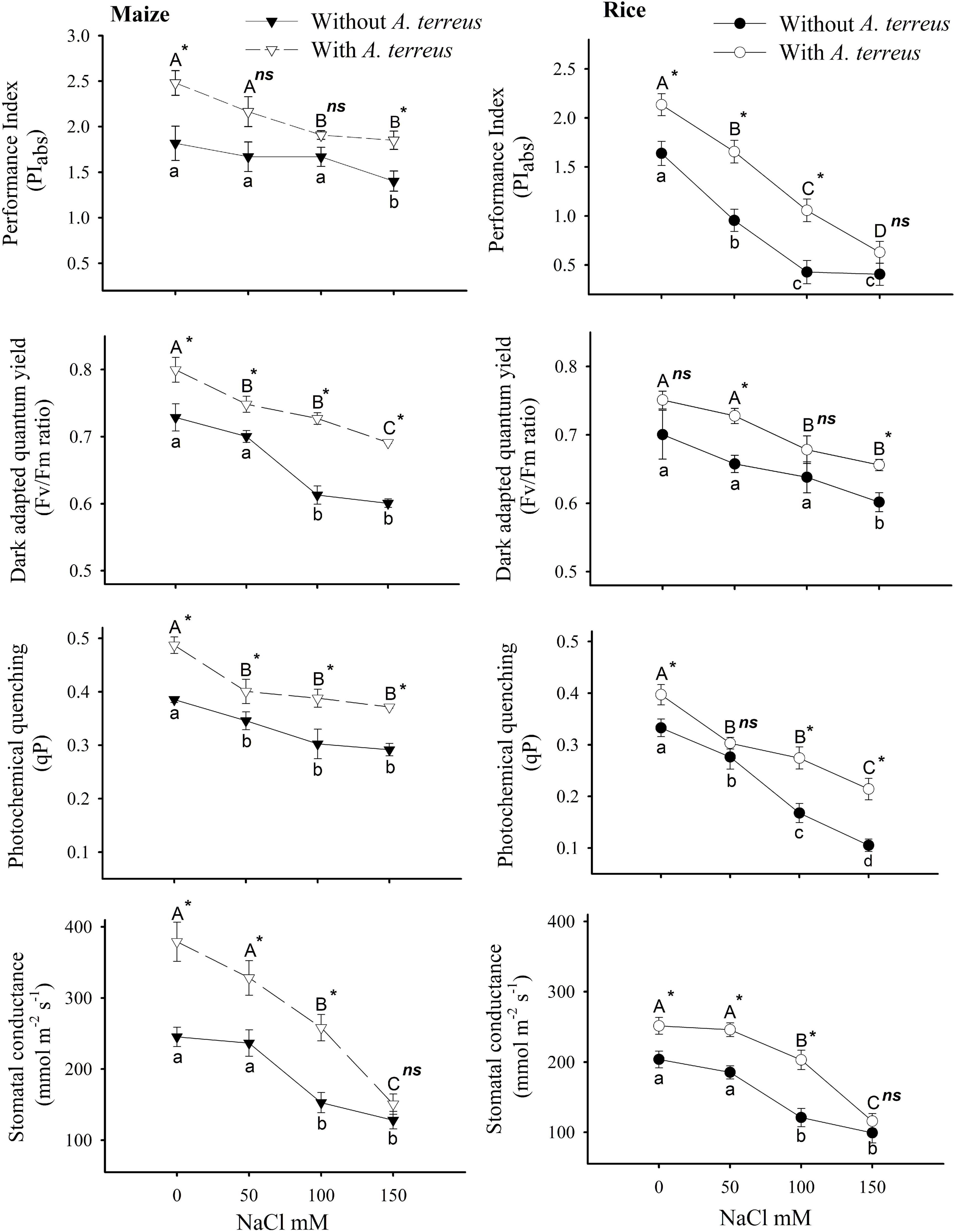
Figure 3. The effect of Aspergillus terreus seed treatments on the photosynthetic attributes of maize (Zea mays L.) var. NT6621 and rice (Oryza sativa L.) var. Kernel under saline conditions. Vertical lines on line graphs denote mean (±) standard error. Same letters on the bar graphs denote non-significant differences within a treatment (capital letters for with A. terreus, small letters without A. terreus only salinity), (*) denotes significant differences, and (ns) denotes non-significant among treatments (with and without A. terreus).
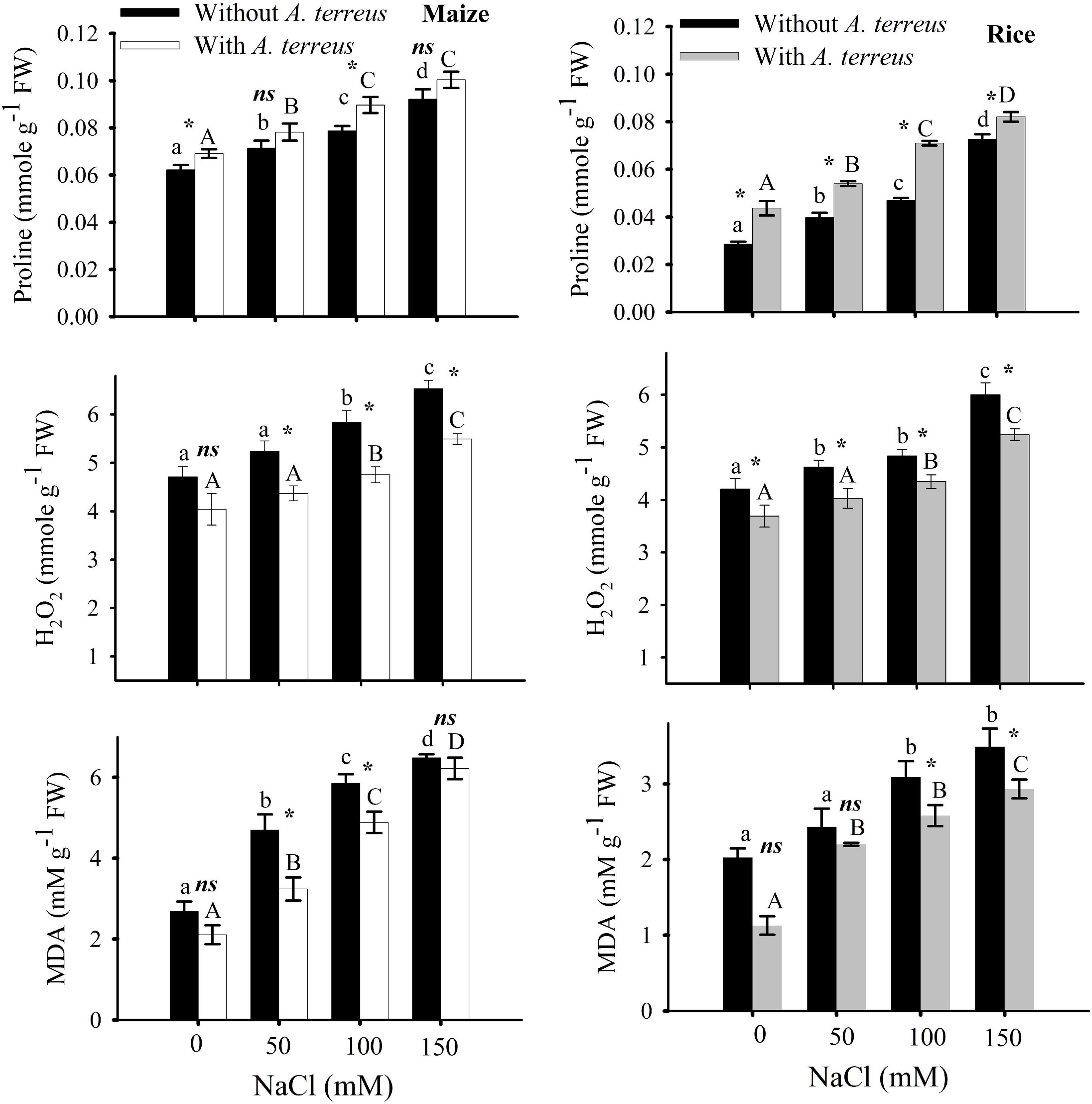
Figure 4. Effect of Aspergillus terreus seed treatments on the proline, H2O2, and MDA contents of maize (Zea mays L.) var. NT6621 and rice (Oryza sativa L.) var. Kernel under saline conditions. Vertical lines on bargraphs denote mean (±) standard error. The same letters on the bar graph denote non-significant differences within a treatment (capital letters for A. terreus, small letters for without A. terreus only salinity), (*) denotes significant differences, and (ns) denotes non-significant among treatments (with and without A. terreus).
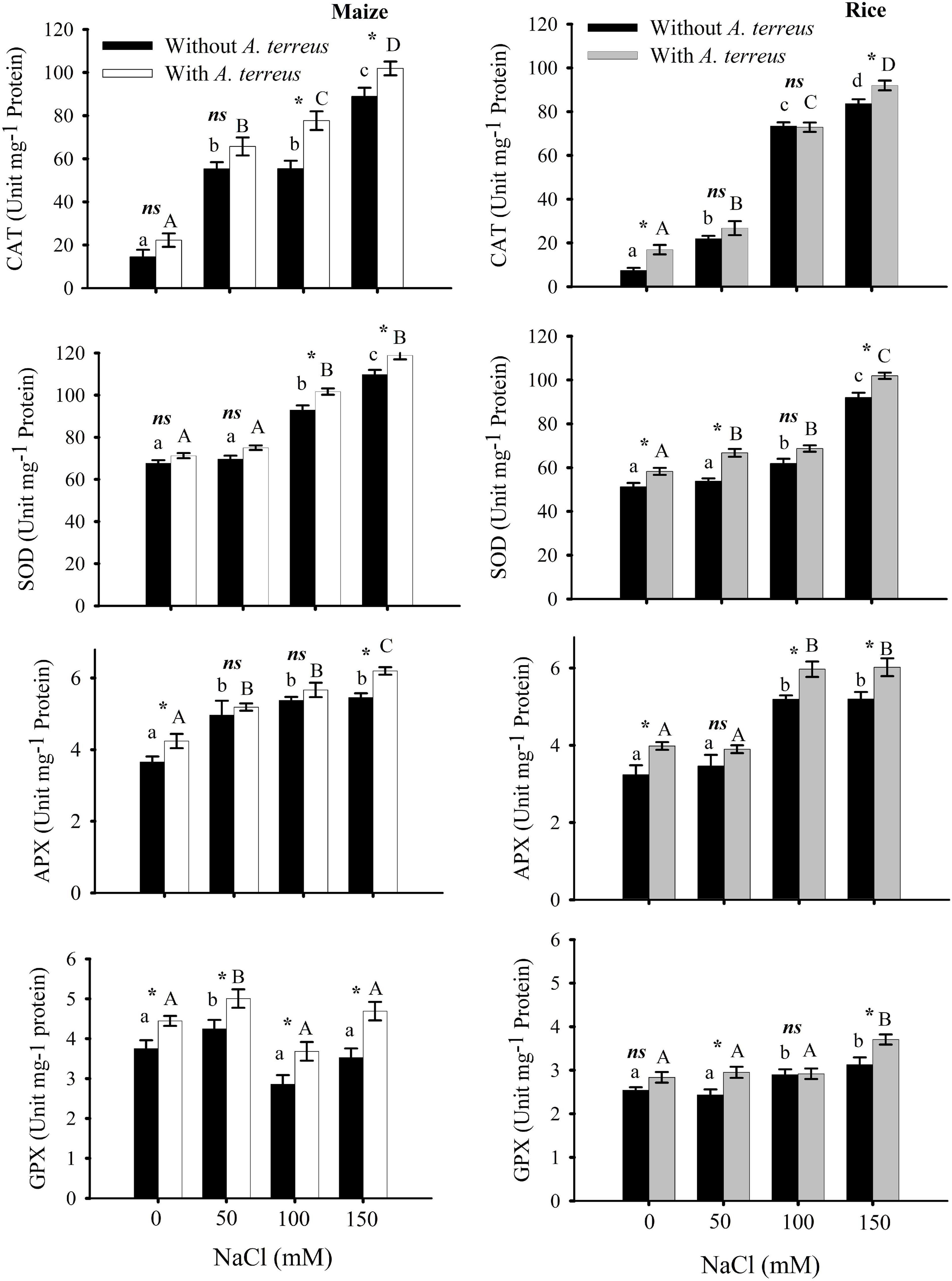
Figure 5. The effect of Aspergillus terreus seed treatments on antioxidants enzymes of maize (Zea mays L.) var. NT6621 and rice (Oryza sativa L.) var. Kernel under saline conditions. Vertical lines on bar graphs denote mean (±) standard error. Same letters on the bar graph denote non-significant differences within a treatment (capital letters for A. terreus, small letters without A. terreus only salinity), (*) denotes significant differences, and (ns) denotes non-significant among treatments (with and without A. terreus).
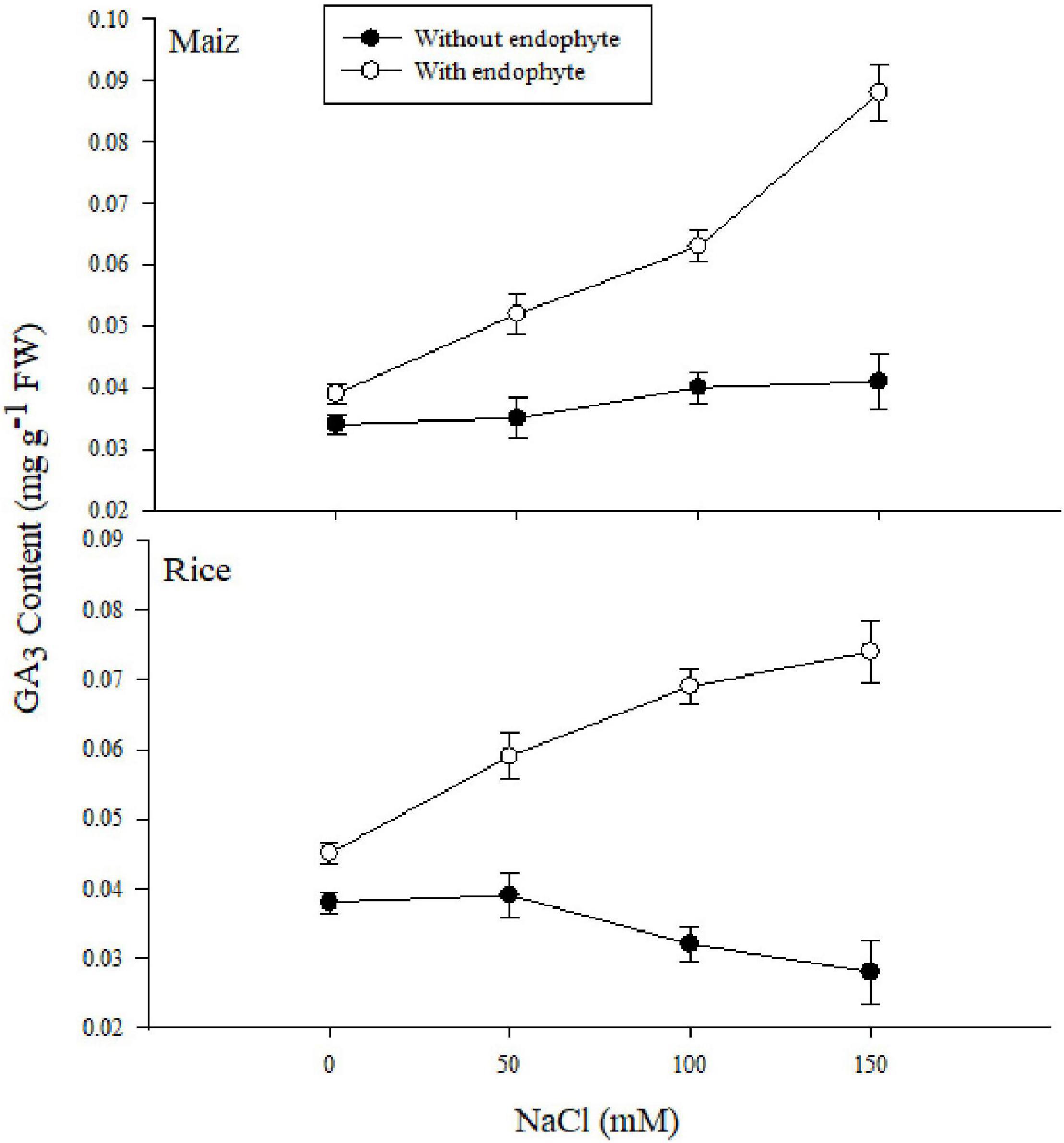
Figure 6. The effect of Aspergillus terreus seed treatments on total gibberellic acid of maize (Zea mays L.) var. NT6621 and rice (Oryza sativa L.) var. Kernel under saline conditions. Vertical line online graph represents mean SE (±).
Results
The effects of A. terreus with and without salt stress on maize and rice plants were examined in this experiment. Growth in root and shoot length declined significantly in salt stress (NaCl) treatments as compared to positive control. However, seeds treated with A. terreus showed a significant increase in shoot lengths of maize up to 30% and rice up to 21% under salt stress as compared to untreated plants (Supplementary Table 1). Similarly, the application of A. terreus improved the root lengths in both plants (maize 26%, rice 29%) against salt stress. At 150 mM NaCl, a plant treated with A. terreus showed up to a 13% increase in rice and a 10% increase in maize fresh weight (FW) as compared to untreated plants (Figure 2). Dry weight (DW) also increased in rice (18%) and maize (10%) at 150 mM when plants were treated with A. terreus. Leaf relative water content (RWC) also increased in maize and rice plants under salt stresses when plants were exposed to endophyte A. terreus.
It was evident from the data that A. terreus mitigates the deleterious effect of NaCl stress in both maize and rice plants improving chlorophyll content under salt stress conditions (Supplementary Table 2). The A. terreus in maize plants increased the total chlorophyll up to 24% under salt stress environment. The use of A. terreus enhanced chlorophyll ‘a’ and ‘b’ displaying a substantial increase in maize (36%) and rice (38%) at 150 mM NaCl as compared to untreated plants.
However, the application of endophyte substantially improved the performance index (PIabs) under salt stress compared to endophyte untreated plants. In both plants, the variable fluorescence (Fv/Fm) ratio was minimally affected it was in the range of 0.6–0.7 under salt stress. Photochemical quenching (qP) exhibited more significant variations under salt stress, especially in rice plants. A sharp decline (almost two folds) in qP was observed in rice as the salt concentration increased. Application of endophyte in maize plants expressed better qP values around 0.3 under salt stresses than rice plants, where qP values decreased up to 0.1. These results suggested that maize plants had better photosynthetic performance than rice plants under a salt stress environment. However, the use of A. terreus in both plants improved the photosynthetic performance under salt stress conditions (Figure 3).
In stress without A. terreus-free proline content was increased in both maize and rice and it was in the range of 0.02–0.0727 (Figure 4). However, the use of endophyte (A. terreus) in rice and maize alleviated the adverse effects of salinity and showed substantial increases in proline; almost 9-12% in maize and 11-34% in rice under salt stress conditions. It was noticed from the data that the amount of hydrogen peroxide (H2O2) was greatly decreased in maize (19%) in the presence of A. terreus under saline conditions compared to rice plants. Seeds treated with A. terreus showed a minimum H2O2 (almost 19% in maize and 14% in rice) at all NaCl treatments as compared to A. terreus untreated plants. MDA content of A. terreus-treated plants was decreased in both maize and rice as compared to untreated plants (Figure 4). It appears that the A. terreus treatment mitigated the salinity effects and reduced MDA up to 44% in maize and 79% in rice under salt stress environment (Figure 4).
Antioxidant enzymes including CAT, APX, SOD, and guaiacol peroxidase (GPX) activities were measured at different NaCl concentrations with and without A. terreus (Figure 5). Observations revealed that both maize and rice plants CAT, APX, and SOD activities substantially increased with an increasing NaCl concentration in the presence of endophyte A. terreus. Maize plants expressed 12–28% CAT, 7–9% SOD; 4–12% APX; 15–25% GPX activities. At 50 mM NaCl, the CAT activities of maize plants were two-fold higher than rice plants. In the same way, GPX activities of maize were also greater than rice plants under salt stress. These results showed that maize plants had greater antioxidant activity than rice (Figure 6).
The gibberellic acid (GA) content increased in both maize and rice under salt stress due to the application of A. terreus (Figure 6). However, a gradual increase was observed in both rice and maize. Results suggested that maize plants had higher GA under 150 mM NaCl treatments with and without A. terreus compared to rice in each treatment.
Salt stress affects photosynthesis and plants’ growth due to ion toxicity and osmotic drought. Under saline conditions, Na+, K+, and Cl– concentrations were examined in both rice and maize plants (Table 1). Results showed that maize and rice had accumulated substantial amounts of Na + and Cl- ions under NaCl stresses. However, after the use of endophyte A. terreus, the Na+ accumulation was reduced up to 103% in maize and 73% in rice plants under salt stress. A. terreus showed a more significant reduction in Na+ and Cl– ions in maize than in rice plants. Plants exposed to salt stress without A. terreus showed the highest amounts of Na+ and Cl– as compared to plants inoculated with A. terreus. Results expressed that rice and maize had lower Na+ concentrations as compared to Cl– under salt stress. Similarly in salt stress, a minimal decline in K+ ion was also observed in both plants without A. terreus (Table 1). The A. terreus-inoculated maize plants expressed a lesser decline in K+ ion accumulation as compared to rice under salt stress. These outcomes showed that the K+ concentration was higher in maize compared to rice under salt stress. The higher concentration of K+ in maize than rice might be due to the inoculation of A. terreus, which maintains the membrane integrity and averts the leakage of K+ under salt stress conditions. The Na+/K+ ratio help in salt tolerance in plants. The Na+/K+ ratio was increased under salt stress as compared to control plants. The maize plants have a lower Na+/K+ ratio as compared to rice plants.
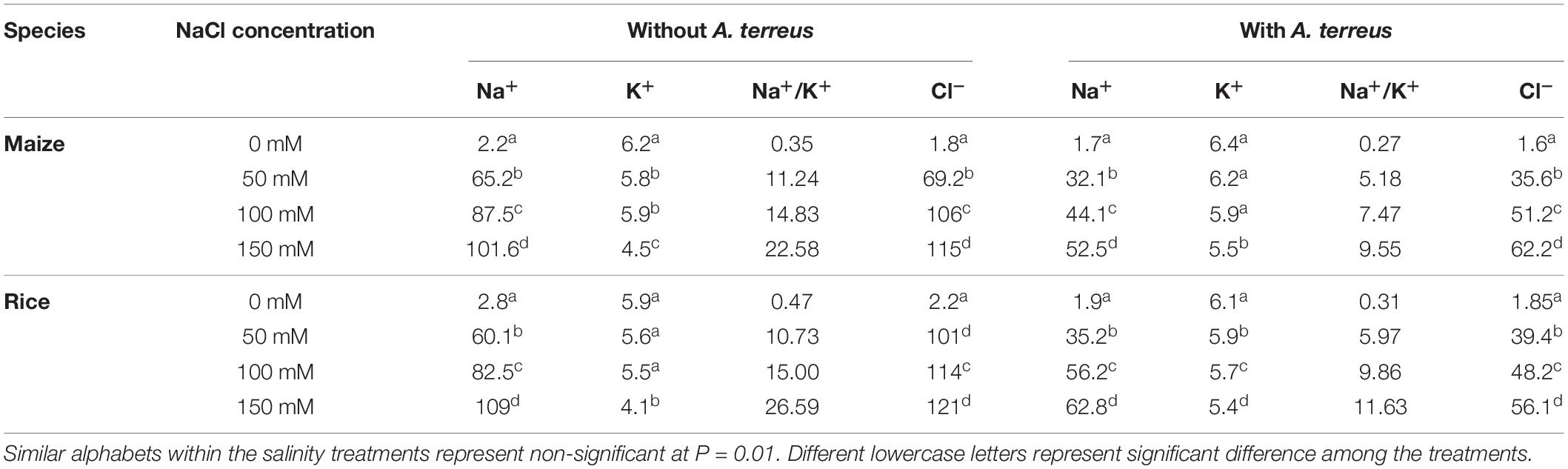
Table 1. Changes in Na+, K+, and Cl– concentration (mM) of rice and maize due to Aspergillus terreus seed treatments under saline conditions.
Discussion
Salt-tolerant endophytic fungus A. terreus was applied in two different crops, i.e., maize and rice belonging to the same family. The plants’ growth and physiological responses under saline stress conditions were determined. It was observed that salinity caused a substantial reduction in the growth and biomass of those plants without A. terreus treatment. The application of A. terreus mitigates salt-related consequences in plants, which results in considerable increases in growth, biomass production (up to 30% in maize; 25% in rice), and RWC (11% maize; 14% rice) under saline conditions (Supplementary Table 1).
Endophytic fungus negates the salt stress in plants by activating the antioxidant system, increasing the level of osmoprotectants, modulation of phytohormone profile, and reducing the salt-induced root respiration (Jogawat et al., 2013; Rewald et al., 2015; Ghaffari et al., 2016; Zhang et al., 2019). These cascades of effects are in feedback to enhance the plant growth and resistance against salt stress. The present study observed the increased biomass content in maize and rice plants treated with endophytic fungus. This explicates the modification in the root architecture system, by the endophytic fungus to improve the plant’s nutrient uptake which results in increased accumulation of biomass content (Gupta et al., 2020). Improved root architecture system by endophytic fungus with different compositions of rhizo-deposits (exudates) also enables the rice and maize plants to utilize water resources of the salt-exploited areas until it cannot be avoided (Jogawat et al., 2013). This imparted the effect on the plant water status for regulation of salt acquisitions and translocation with the increased RWC of the plants treated with endophytes (Figure 2). The current study also witnessed the increased root length in rice and maize plants colonized with endophytes which negated the inhibition of cell division and elongation caused by salt acquisitions in the soil (Rahnama et al., 2011). The increased shoot length of the plants colonized with endophytes was due to the phytohormone modulations. Inoculation of endophytes strategizes the interplay of phytohormone to regulate the physiological and metabolic process against salt stress in plants. From Figure 6, it is evident that the increased bioactive gas was responsible for the greater shoot length in maize and rice plants (Waqas et al., 2012). The greater increment in maize as compared to rice plants might be linked with the better symbiotic association of an endophytic fungus with the maize than the rice (Rho et al., 2018).
Higher chlorophyll content (a and b) as well as carotenoids in rice and maize plans colonized with A. terreus spell out the induction of salt tolerance in the chloroplast by endophyte inoculation (Supplementary Table 2). Excessive Na + ion accumulation in chloroplast deters the photosynthetic activity, which was evident from the low chlorophyll and carotenoid content of plants without endophyte inoculation (Ali et al., 2012). The current findings suggest that the biosynthetic enzymes of the chlorophyll pathway could be more active and functional in rice and maize plants, which were inoculated with A. terreus against salt stress (Chaves et al., 2009). The enhanced level of gs (Figure 3) with the A. terreus inoculation depicted the tolerance of osmotic shock and increased leaf area caused by the salt stress (Chaves et al., 2009). Higher gs favor the enhancement of photosynthetic attributes by providing more CO2 assimilation and rubisco activity (Seemann and Critchley, 1985). The increased photosynthetic attributes in plants inoculated with endophyte displayed the reduced risk of photosystem II damage, which is often caused by salt stress. The higher performance index (PIabs), dark-adapted quantum yield (Fv/Fm), and photochemical quenching (qP) of photosynthetic apparatus were due to less accumulation of electrons in the thylakoid membrane and reduced disposal of energy dissipation among the plants colonized with endophytes (Habib et al., 2017). These photochemical attributes displayed the protection of D1 and D2 proteins of photosystem II by A. terreus against salt stress.
A decrease in MDA (44% maize; 19% rice) and H2O2 (22% maize; 14% rice) in A. terreus-treated plants under a saline environment was observed. In the present study, plants treated with A. terreus had lower H2O2 contents than A. terreus-untreated plants under salinity stress (Figure 3). It was suggested that several plants develop several physiological and biochemical tactics to deal with stress in a stressful environment. This osmotic adjustment substance that exists in plant cells is more important as it is available in the free state and possesses high water solubility, low molecular weight, and helps in maintaining physiological pH. It was observed that plant cells tend to accumulate soluble osmotic substances such as proline, sugars, and sugar-containing alcohol, to alleviate osmotic stress caused by salt stress (Silveira et al., 2003). It was evident from the present study that the application of endophytes lowered the H2O2 contents indicating the relief of stress-related consequences (Ahmad et al., 2015; Tahjib-Ul-Arif et al., 2018). It is presumed that the application of endophytes minimizes the breakdown of aldehydic lipid products, causing a reduction of MDA and H2O2 values under saline conditions and mitigating salt stress. In this investigation, A. terreus-treated plants expressed lower MDA contents under saline environments. Our findings agreed with a study previously reported by Khan et al. (2012) that showed more than 40% decline in MDA production under saline conditions, which suggested lower cellular membrane damage in plants.
In abiotic stress such as salinity, elevated H2O2 production damages the protein and lipid molecules (Umar and Siddiqui, 2018). It is presumed that the application of A. terreus lowered the H2O2 production in salt stress due to more antioxidant enzyme activity. Moreover, it was reported that an endophyte such as Trichoderma enhances antioxidant enzymes such as glutathione S-transferases (GSTs) and peroxidase (POD) activities and lowers reactive oxygen species (ROS) production (Hajiboland et al., 2010; Wu and Sardo, 2010; Alqarawi et al., 2014). It was reported that maximum quantum yield and photosynthetic performance control the production of ROS (Kalaji et al., 2018). Photosynthetic performance and quantum yield are inter-related and are often decreased in abiotic stress and under elevated ROS levels. Fluctuating responses with respect to quantum yield and performance index in a stress environment are diversified and specific for some plant species (Behera et al., 2002). Substantial photosynthetic performance index and quantum yield in maize treated with A. terreus indicate that maize could develop a better symbiotic relationship with A. terreus in a saline environment compared to rice. In our study, it was detected that the presence of A. terreus in a saline environment increased the activity of antioxidant enzymes as compared to those saline media that did not have A. terreus. Earlier, it was reported that the activities of antioxidant enzymes such as catalase (CAT), superoxide dismutase (SOD), peroxidase (POD), and ascorbate peroxidase (APX) were increased in a saline environment (Umar and Siddiqui, 2018). It is presumed that the presence of A. terreus in a saline environment diminished H2O2 production due to elevated antioxidant enzyme activities as well as proline production. It was reported that antioxidant enzymes and the production of osmolytes and polyols, such as proline and sorbitol, are important physiological strategies for coping with the consequences of abiotic stress and maintaining ion homeostasis (Sairam et al., 2002; Rasool et al., 2013). Antioxidant activities are important physiological aspects playing a key role in coping with salt stress (Guo et al., 2018). An increase in antioxidant activities protects the cell against environmental stresses like drought and salt stress. A. terreus-treated plants showed significant increases ranging from 5 to 28% in antioxidant enzymes activities, including CAT, APX, SOD, and GPX, compared to non-inoculated plants under salt stresses (Figure 6). It was observed that the endophytic fungi increased antioxidant enzyme activities in plants under salt stress conditions (Rasool et al., 2013; Hashem et al., 2014). Physiologically, abiotic stresses cause oxidative damage to plants by producing ROS in plant cells, which are compensated by provoking an antioxidant system (Ahmad et al., 2015). The production of ROS was elevated in stress conditions causing denaturation of biomolecules (Becana et al., 2000; Majeed et al., 2010). In this regard, antioxidant enzymes such as SOD play a critical role in plants and functionally convert the superoxide radicals into water and oxygen (O2). Elevated SOD activity against salinity in the present study seems to be used in the de novo synthesis of antioxidant enzymatic proteins such as APX, CAT, and GPX (Verma and Dubey, 2003; Malecka et al., 2007; Majeed et al., 2010). Therefore, it may also be suggested that a combined effect of two or more antioxidant enzymes could provide complete detoxification of ROS to plants under salt stress conditions, as observed in rice and maize.
Plants treated with A. terreus had lower concentrations of Na+ and Cl– under salt stress than control plants favoring a better plant growth and photosynthetic performance. Results suggested that A. terreus improved the efficiency of plants to eliminate the Na+ and Cl– under salt stress. Both plants treated with A. terreus showed a greater concentration of K+ (3–24% increment), whereas lower Na+ concentrations (46–98% decline) compared to plants without A. terreus under salt stress. The increment in K+ suggests that A. terreus had greater potential to accumulate K+ ions under NaCl playing an important role in maintaining physiological processes under salt stress (Colpan et al., 2013). Furthermore, K+ ion minimizes oxidative stress and improves the root and shoot length (Jan et al., 2017). The essential criteria for salt tolerance in plants are higher amounts of K+ and Na+ selectivity by roots and distribution of ions in plant tissues under salt stress (Gu et al., 2016). The higher the accumulation of Na+ and Cl– ions are frequently assumed to be primarily responsible for reducing photosynthesis and growth of plants (Tsai et al., 2004; Hong et al., 2009).
Application of endophyte showed a substantial decrease in MDA. The higher K+ contents in the endophyte-treated plant are linked with reduced MDA contents in monocot plants (Jan et al., 2017). It is suggested that the A. terreus-treated plant showed higher K + contents that ultimately prevent membrane degradation in maize and rice plants under salt stress. Na+/K+ ratio under salt stress is an important trait to screen the genotypes. It was interesting that maize and rice plants inoculated with A. terreus showed a lower Na+/K+ ratio than uninoculated plants under salt stress. This indicated that the Na+ exclusion mechanism might be more active in A. terreus-inoculated plants. The salt tolerance in A. terreus-inoculated plants might be due to the lower rate of Na+ loading and better capacity to sequester as it enters the leaf (Alias et al., 2020). Fungus-treated plants, lower Na+/K+ ratio in maize plants under salt stress may have helped in better tolerance than rice plants.
Some of the vital stress parameters were selected and compared using Pearson’s correlations. Supplementary Table 3 explains the correlation analysis with and without A. terreus among the desired parameters. Proline, CAT, APX, and GPX were positively correlated with other RWC, Chl, PIabs, Fv/Fm, and stomatal conductance (gs), and RWC showed the highest negative correlation (–0.990** and –0.950**) with H2O2 and MDA under salt stress treatment. It was evident from the data that proline accumulation could be the main reason for higher RWC in plants treated with endophyte under saline habitat. Similarly, higher antioxidant activities also provide further evidence in this regard. It was suggested that an increased MDA depended on lower RWC under stress conditions. Furthermore, RWC positively correlated with Chl, PIabs, Fv/Fm, and gs (almost 0.95**), which expressed the importance of RWC in photosynthesis (Umar and Siddiqui, 2018). Higher RWC improves the photosynthetic efficiency by increasing chlorophyll contents and photochemical performance (Hajlaoui et al., 2009; Umar and Siddiqui, 2018). The correlation analysis showed that GA3 was positively correlated with CAT and SOD. The photosynthetic attributes were also significantly correlated with GA3 concentration. This correlation suggested that GA3 upregulated the antioxidant enzyme activities linked with better photosynthetic performance in maize and rice plants against A. terreus treatment.
The role of GA producing endophytes in crop plants such as maize and rice regarding upregulating physiological traits was not previously highlighted. In the present study, it was evident from the data that A. terreus improved substantial plant growth, photosynthetic performance index, and antioxidant system are linked with excessive production of GA in both the treated plants. Furthermore, it was suggested that A. terreus improved growth and provided physiological tolerance in crop plants due to more phytohormones (GA3) production under saline conditions. However, the concentration of GA production in both plants varies, affecting the physiological and growth response differently under the abiotic stress environment. Our current findings indicated that A. terreus established a more significant symbiotic relationship with maize than rice under saline conditions producing phytohormones, showing the possibility of using endophytic microbe in salt-affected soil. This profound eco-physiological benefit suggests that A. terreus could be considered a better plant biostimulant for improving maize seedling in saline soil than rice. Our findings also indicated that rice and maize could produce biomass for economic sustainability by using brackish water irrigation on saline soils avoiding competition with conventional agriculture.
Data Availability Statement
The datasets presented in this study can be found in online repositories. The names of the repository/repositories and accession number(s) can be found below: https://www.ncbi.nlm.nih.gov/genbank/, SUB9030920 AH1_AHK_ITS1 MW570850: SUB9030920 AH1_AHK_ITS4 MW570851.
Author Contributions
ZS, RY, and MU did physiological and photochemistry experiments. DD did proofreading and editing. AH and SD were involved in providing fungus and its molecular identification. All authors equally participated in the manuscript.
Conflict of Interest
The authors declare that the research was conducted in the absence of any commercial or financial relationships that could be construed as a potential conflict of interest.
The handling editor declared a past co-authorship with one of the authors FZ.
Publisher’s Note
All claims expressed in this article are solely those of the authors and do not necessarily represent those of their affiliated organizations, or those of the publisher, the editors and the reviewers. Any product that may be evaluated in this article, or claim that may be made by its manufacturer, is not guaranteed or endorsed by the publisher.
Acknowledgments
The authors would gratefully acknowledge the Plant Pathology Laboratory, Department of Botany, and the University of Karachi, Pakistan, for providing the Aspergillus terreus isolate. The authors wish to thank Higher Education Commission (HEC) sponsored project # 6586 for financial support to carry out this research.
Supplementary Material
The Supplementary Material for this article can be found online at: https://www.frontiersin.org/articles/10.3389/fpls.2021.770084/full#supplementary-material
References
Abideen, Z., Koyro, H. W., Huchzermeyer, B., Ahmed, M. Z., Zulfiqar, F., Egan, T., et al. (2020). Phragmites karka plants adopt different strategies to regulate photosynthesis and ion flux in saline and water deficit conditions. Plant Biosys. 155, 524–534. doi: 10.1080/11263504.2020.1762783
Afzal, S., Samrah, T., Viqar, S., Jehan, A., and Syed, E. H. (2013). Managing the root diseases of okra with endo-root plant growth promoting Pseudomonas and Trichoderma viride associated with healthy okra roots. Pak. J. Bot. 45, 1455–1460.
Ahmad, P., Abeer, H., Elsayed, F. A. A., Alqarawi, A. A., Riffat, J., Dilfuza, E., et al. (2015). Role of Trichoderma harzianum in mitigating NaCl stress in Indian mustard (Brassica juncea L.) through antioxidative defense system. Front. Plant. Sci. 6:868. doi: 10.3389/fpls.2015.00868
Ali, S., Charles, T. C., and Glick, B. R. (2012). Delay of flower senescence by bacterial endophytes expressing 1-aminocyclopropane-1-carboxylate deaminase. J. App. Microbiol. 113, 1139–1144. doi: 10.1111/j.1365-2672.2012.05409.x
Alias, E., Thomas, D., Menon, M. V., Prameela, P., and Sreelatha, A. K. (2020). Regulation of plant Na/K ratio for productivity enhancement in Pokkali rice. J. Trop. Agric. 58, 78–82.
Alqarawi, A. A., Abd Allah, E. F., and Hashem, A. (2014). Alleviation of salt-induced adverse impact via mycorrhizal fungi in ephedra aphylla forssk. J. Plant Interact. 9, 802–810. doi: 10.1080/17429145.2014.949886
Ananda, K., and Sridhar, K. R. (2002). Diversity of endophytic fungi in the roots of mangrove species on the west coast of India. Can. J. Microbiol. 48, 871–878. doi: 10.1139/w02-080
Barrs, H. D., and Weatherley, P. E. (1962). A re-examination of the relative turgidity technique for estimating water deficits in leaves. Austr. J. Biol. Sci. 15, 413–428. doi: 10.1071/bi9620413
Bates, L., Waldren, P. P., and Teare, J. D. (1973). Rapid determination of free proline of water stress studies. Plant Soil 39, 205–207. doi: 10.1007/bf00018060
Becana, M., Dalton, D. A., Moran, J. F., Iturbe-Ormaetxe, I., Matamoros, M. A., and Rubio, M. C. (2000). Reactive oxygen species and antioxidants in legume nodules. Physiol. Plant 109, 372–381. doi: 10.1034/j.1399-3054.2000.100402.x
Behera, R. K., Mishra, P. C., and Choudhury, N. K. (2002). High irradiance and water stress induce alterations in pigment composition and chloroplast activities of primary wheat leaves. J. Plant Physiol. 159, 967–973. doi: 10.1078/0176-1617-00823
Beyer, W. F., and Fridovich, I. (1987). Assaying for superoxide dismutase activity. some large consequences of minor changes in condition. Anal. Biochem. 61, 559–566. doi: 10.1016/0003-2697(87)90489-1
Bilal, S., Shahzad, R., Khan, A. L., Al-Harrasi, A., Kim, C. K., and Lee, I. J. (2019). Phytohormones enabled endophytic Penicillium funiculosum LHL06 protects Glycine max L. from synergistic toxicity of heavy metals by hormonal and stress-responsive proteins modulation. J. Hazard. Mater. 379:120824. doi: 10.1016/j.jhazmat.2019.120824
Bradford, M. M. (1976). A rapid and sensitive method for the quantitation of microgram quantities of protein utilizing the principle of protein-dye binding. Anal. Biochem. 72, 248–254. doi: 10.1006/abio.1976.9999
Cavell, B. D., MacMillan, J., Pryce, R. J., and Sheppard, A. C. (1967). Thin layer chromatography of gibberellic acids. Phytochemistry 6, 867–874.
Chaudhary, M. T., Wainwright, S. J., and Merrett, M. J. (1996). Comparative NaCl tolerance of Lucerne plants regenerated from salt-selected suspension cultures. Plant Sci. 114, 221–232. doi: 10.1016/0168-9452(96)04326-9
Chaves, M. M., Flexas, J., and Pinheiro, C. (2009). Photosynthesis under drought and salt stress: regulation mechanisms from whole plant to cell. Ann. Bot. 103, 551–560. doi: 10.1093/aob/mcn125
Chinnusamy, V., Jagendorf, A., and Zhu, J. K. (2005). Understanding and improving salt tolerance in plants. Crop Sci. 45, 437–448. doi: 10.2135/cropsci2005.0437
Cho, B. R., Lee, P., and Hahn, J. S. (2014). CK2-dependent inhibitory phosphorylation is relieved by Ppt1 phosphatase for the ethanol stress-specific activation of Hsf1 in Saccharomyces cerevisiae. Mol. Microbiol. 93, 306–316. doi: 10.1111/mmi.12660
Colpan, E., Zengin, M., and Ozbahce, A. (2013). The effects of potassium on the yield and fruit quality components of stick tomato. Hortic. Environ. Biotechnol. 54, 20–28. doi: 10.1007/s13580-013-0080-4
Dhindsa, R. H., Plumb-Dhindsa, R., and Thorpe, T. A. (1981). Leaf senescence correlated with increased level of membrane permeability, lipid peroxidation and decreased level of SOD and CAT. J. Exp. Bot. 32, 93–101. doi: 10.1093/jxb/32.1.93
Fargues, J., Maniania, N. K., Delmas, J. C., and Smits, N. (1992). Influence de la temperature sur la croissance in vitro d‘hyphomycetes entomopathogens. Agronomie 12, 557–564. doi: 10.1051/agro:19920708
Farooq, M., Hussain, M., Wakeel, A., and Siddiqui, K. H. M. (2015). Salt stress in maize: effects, resistance mechanisms, and management. A review. Agron. Sustain. Dev. 35, 461–481. doi: 10.1007/s13593-015-0287-0
Ghaffari, M. R., Ghabooli, M., Khatabi, B., Hajirezaei, M. R., Schweizer, P., and Salekdeh, G. H. (2016). Metabolic and transcriptional response of central metabolism affected by root endophytic fungus Piriformospora indica under salinity in barley. Plant Mol. Biol. 90, 699–717. doi: 10.1007/s11103-016-0461-z
Gu, M. F., Li, N., Shao, T. Y., Long, X. H., Brestic, M., Shao, H. B., et al. (2016). Accumulation capacity of ions in cabbage (Brassica oleracea L). supplied with sea water. Plant Soil Environ. 62, 314–320. doi: 10.17221/771/2015-pse
Guo, R., Wang, Z., Huang, Y., Fan, H., and Liu, Z. (2018). Biocontrol potential of saline-or alkaline-tolerant Trichoderma asperellum mutants against three pathogenic fungi under saline or alkaline stress conditions. Braz. J. Microbiol. 49, 236–245.
Gupta, S., Chaturvedi, P., Kulkarni, M. G., and Van Staden, J. (2020). A critical review on exploiting the pharmaceutical potential of plant endophytic fungi. Biotechnol. Adv. 39:107462. doi: 10.1016/j.biotechadv.2019.107462
Habib, N., Avraham-Davidi, I., Basu, A., Burks, T., Shekhar, K., Hofree, M., et al. (2017). Massively parallel single-nucleus RNA-seq with DroNc-seq. Nat. Methods 14, 955–958. doi: 10.1038/nmeth.4407
Hajiboland, R., Aliasgharzadeh, N., Laiegh, S. F., and Poschenrieder, C. (2010). Colonization with arbuscular mycorrhizal fungi improves salinity tolerance of tomato (Solanum Lycopersicum L.) plants. Plant Soil 331, 313–327. doi: 10.1007/s11104-009-0255-z
Hajlaoui, H., Denden, M., and El-Ayeb, N. (2009). Changes in fatty acids composition, hydrogen peroxide generation and lipid peroxidation of salt-stressed corn (Zea mays L). roots. Acta Physiol. Plant 31, 787–796. doi: 10.1007/s11738-009-0293-4
Halo, B. A., Al-Yahyai, R. A., and Al-Sadi, A. M. (2018). Aspergillus terreus inhibits growth and induces morphological abnormalities in Pythium aphanidermatum and suppresses pythium-induced damping-off of cucumber. Front. Microbiol. 9:95. doi: 10.3389/fmicb.2018.00095
Hashem, A., Abd-allah, E. F., Alqarawi, A. A., Al-Huqail, A. A., and Egamberdieva, D. (2014). Alleviation of abiotic salt stress in Ochradenus baccatus (Del.). by Trichoderma hamatum (Bonord.). Bainier. J. Plant Interact. 9, 857–868. doi: 10.1080/17429145.2014.983568
Hong, C. Y., Chao, Y. Y., Yang, M. Y., Cho, S. C., and Huei-Kao, C. (2009). Na+ but not Cl– or osmotic stress is involved in NaCl-induced expression of glutathione reductase in roots of rice seedlings. J. Plant Physiol. 166, 1598–1606. doi: 10.1016/j.jplph.2009.04.001
Hong, S. B., Go, S. J., Shin, H. D., Frisvad, J. C., and Samson, R. A. (2005). Polyphasic taxonomy of Aspergillus fumigatus and related species. Mycologia 97, 1316–1329. doi: 10.1080/15572536.2006.11832738
Hussain, S., Shaukat, M., Ashraf, M., Zhu, C., Jin, Q., and Zhang, J. (2019). “Salinity stress in arid and semi-arid climates, effects and management in field crops,” in Climate Change and Agriculture, eds S. Hussain, M. Shaukat, M. Ashraf, C. Zhu, Q. Jin, and J. Zhang (London: Intech Open).
Jan, A. U., Hadi, F., Nawaz, M. A., and Rahman, K. (2017). Potassium and zinc increase tolerance to salt stress in wheat (Triticum aestivum L.). Plant Physiol. Biochem. 116, 139–149. doi: 10.1016/j.plaphy.2017.05.008
Jogawat, A., Saha, S., Bakshi, M., Dayaman, V., Kumar, M., Dua, M., et al. (2013). Piriformospora indica rescues growth diminution of rice seedlings during high salt stress. Plant Signal. Behav. 8:e26891. doi: 10.4161/psb.26891
Kalaji, H. M., Rastogi, A., Živčák, M., Brestic, M., Daszkowska-Golec, A., Sitko, K., et al. (2018). Prompt chlorophyll fluorescence as a tool for crop phenotyping: an example of barley landraces exposed to various abiotic stress factors. Photosynthetica 56, 953–961. doi: 10.1007/s11099-018-0766-z
Kamkar, B. (2016). Sustainable development principles for agricultural activities. Adv. Plant Agric. Res. 3, 163–164.
Khan, A. L., Hamayun, M., Kang, S. M., Kim, Y. H., Jung, H. Y., Lee, J. H., et al. (2012). Endophytic fungal association via gibberellins and indole acetic acid can improve plant growth under abiotic stress: an example of Paecilomyces formosus LHL10. BMC Microbiol. 12:3. doi: 10.1186/1471-2180-12-3
Lee, S. B., and Taylor, J. W. (1990). “Isolation of DNA from fungal mycelia and single spores,” in PCR Protocols: A Guide to Methods and Applications, eds M. A. Innis, D. H. Gelfand, J. J. Sninsky, and T. J. White (New York, NY: Academic Press), 282–287. doi: 10.1016/b978-0-12-372180-8.50038-x
Lichtenthaler, H. K. (1987). Chlorophylls and carotenoids pigments of photosynthetic biomembranes. Method Enzymol. 148, 350–382. doi: 10.1515/znc-2001-11-1225
Majeed, A., Nisar, M. F., and Hussain, K. (2010). Effect of saline culture on the concentration of Na+, K+ and Cl- in Agrostis tolonifera. Curr. Res. J. Biol. Sci. 2, 76–82.
Malecka, A., Jarmuszkiewicz, W., and Tomaszewska, B. (2007). Antioxidative defense to lead stress in subcellular compartments of pea root cells. Acta Biochem. Pollut. 48, 687–698.
Mansour, M. M. F., Salama, K. H. A., Ali, F. Z. M., and Abou-Hadid, A. F. (2005). Cell and plant responses to NaCl in Zea mays (L). Cultivars differing in salt tolerance. Gen. Appl. Plant Physiol. 31, 29–41. doi: 10.1016/j.plaphy.2010.09.011
Maxwell, K., and Johnson, G. N. (2000). Chlorophyll fluorescence – a practical guide. J. Exp. Bot. 51, 659–668. doi: 10.1093/jxb/51.345.659
Nakano, Y., and Asada, K. (1981). Hydrogen peroxide is scavenged by ascorbate specific peroxidase in spinach chloroplasts. Plant Cell Physiol. 22, 867–880.
Nawaz, K., Hussain, K., Majeed, A., Khan, F., Afghan, S., and Kazim, A. (2010). Fatality of salt stress to plants, morphological, physiological and biochemical aspects. Afr. J. Biotechnol. 9, 5475–5480.
Patterson, B. D., Macrae, E. A., and Ferguson, I. B. (1984). Estimation of hydrogen peroxide in plant extracts using titanium (IV). Anal. Biochem. 139, 487–492. doi: 10.1016/0003-2697(84)90039-3
Pitman, M. G., and Lauchli, A. (2002). “Global impact of salinity and agricultural ecosystems,” in Salinity: Environment - Plants–Molecules, eds A. Lauchli and U. Luttge (Dordrecht: Springer).
Rachev, R. C. H., Rousava, R. P., Bojkova, S. V., and Gancheva, V. K. (1993). Isolation of gibberellic acid produced by Fusarium moniliforme. J. Nat. Prod. 56, 1168–1170. doi: 10.1021/np50097a023
Rahnama, A., Munns, R., Poustini, K., and Watt, M. (2011). A screening method to identify genetic variation in root growth response to a salinity gradient. J. Exp. Bot. 62, 69–77. doi: 10.1093/jxb/erq359
Rasool, S., Ahmad, A., Siddiqui, T. O., and Ahmad, P. (2013). Changes in growth, lipid peroxidation and some key antioxidant enzymes in chickpea genotypes under salt stress. Acta Physiol. Plant 35, 1039–1050. doi: 10.1007/s11738-012-1142-4
Rewald, B., Holzer, L., and Göransson, H. (2015). Arbuscular mycorrhiza inoculum reduces root respiration and improves biomass accumulation of salt-stressed Ulmus glabra seedlings. Urb. For. Urban Green. 14, 432–437. doi: 10.1016/j.ufug.2015.04.011
Rho, H., Hsieh, M., Kandel, S. L., Cantillo, J., Doty, S. L., and Kim, S.-H. (2018). Do endophytes promote growth of host plants under stress? A meta-analysis on plant stress mitigation by endophytes. Microbiol. Ecol. 75, 407–418. doi: 10.1007/s00248-017-1054-3
Sairam, R., Rao, K. V., and Srivastava, G. C. (2002). Differential response of wheat genotypes to long term salinity stress in relation to oxidative stress, antioxidant activity and osmolyte concentration. Plant Sci. 163, 1037–1046. doi: 10.1016/s0168-9452(02)00278-9
Schulz, B. (2002). Endophytic fungi, a source of novel biologically active secondary metabolites. Mycol. Res. 106, 996–1004. doi: 10.1017/s0953756202006342
Seemann, J. R., and Critchley, C. (1985). Effects of salt stress on the growth, ion content, stomatal behaviour and photosynthetic capacity of a salt-sensitive species, Phaseolus vulgaris L. Planta 164, 151–162. doi: 10.1007/BF00396077
Shafi, M., Khan, M. J., Bakht, J., and Khan, M. A. (2013). Response of wheat genotypes to salinity under field environment. Pak. J. Bot. 45, 787–794.
Shelford, T. J., de-Villiers, D. S., Langhans, R. W., and Albright, L. D. (2005). An Evaluation of Four Treatment Methods to Eliminate Pythium Aphanidermatum in Hydroponic Spinach using a Spinach Seedling Bioassay. ASABE Paper No. 054055. St. Joseph, MI: ASAE.
Shelford, T. J., de-Villiers, D. S., Langhans, R. W., and Albright, L. D. (2006). A Comparison of Three Treatment Systems for the Suppression of Pythium Aphanidermatum in Continuous Production of Hydroponic Babyleaf Spinach. ASABE Paper No. 064020. St. Joseph, MI: ASAE.
Silveira, J. A. G., de Almeida Viégas, R., da Rocha, I. M. A., Moreira, A. C. D. O. M., de Azevedo Moreira, R., and Oliveira, J. T. A. (2003). Proline accumulation and glutamine synthetase activity are increased by salt-induced proteolysis in cashew leaves. J. Plant Physiol. 160, 115–123. doi: 10.1078/0176-1617-00890
Tahjib-Ul-Arif, M., Siddiqui, M. N., Sohag, A. A. M., Arif Sakil, M., Mezanur Rahman, M., Arif Sadik Polash, M., et al. (2018). Salicylic acid-mediated enhancement of photosynthesis attributes and antioxidant capacity contributes to yield improvement of maize plants under salt stress. J Plant Growth Regul. 37, 1318–1330. doi: 10.1007/s00344-018-9867-y
Tatiana, Z., Yamashita, K., and Matsumoto, H. (1999). Iron deficiency Induced changes in ascorbate content and enzyme activities related to ascorbate metabolism in cucumber root. Plant Cell Physiol. 40, 273–280. doi: 10.1093/oxfordjournals.pcp.a029538
Tavakkoli, E., Fatehi, F., Coventry, S., Rengasamy, P., and McDonald, G. K. (2011). Additive effects of Na+ and Cl– ions on barley growth under salinity stress. J. Exp. Bot. 62, 2189–2203. doi: 10.1093/jxb/erq422
Tisarum, R., Theerawitaya, C., Samphumphuang, T., Polispitak, K., Thongpoem, P., Singh, H. P., et al. (2020). Alleviation of salt stress in upland rice (Oryza sativa L sp. indica cv. Leum Pua). using arbuscular mycorrhizal fungi inoculation. Front. Plant Sci. 11:348. doi: 10.3389/fpls.2020.00348
Tsai, Y. C., Hong, C. Y., Liu, L. F., and Kao, H. (2004). Relative importance of Na+ and Cl– in NaCl-induced antioxidant systems in roots of rice seedlings. Physiol. Plant 122, 86–94. doi: 10.1111/j.1399-3054.2004.00387.x
Tscharntke, T., Clough, Y., Wanger, T. C., Jackson, L., Motzke, I., Perfecto, I., et al. (2012). Global food security, biodiversity conservation and the future of agricultural intensification. Biol. Conserv. 151, 53–59. doi: 10.1016/j.biocon.2012.01.068
Umar, M., and Siddiqui, Z. S. (2018). Physiological performance of sunflower genotypes under combined salt and drought stress environment. Acta Bot. Croat. 77, 36–44. doi: 10.2478/botcro-2018-0002
Vejan, P., Abdullah, R., Khadiran, T., Ismail, S., and Boyce, A. N. (2016). Role of plant growth promoting rhizobacteria in agricultural sustainability—a review. Molecules 21:573. doi: 10.3390/molecules21050573
Velikova, V., Yordanov, I., and Edreva, A. (2000). Oxidative stress and some antioxidant systems in acid rain treated bean plants, protective role of exogenous polyamines. Plant Sci. 151, 59–66. doi: 10.1016/s0168-9452(99)00197-1
Verma, S., and Dubey, R. S. (2003). Lead toxicity induces lipid peroxidation and alters the activities of antioxidant enzymes in growing rice plants. Plant Sci. 164, 645–655.
Waqas, M., Khan, A. L., Kamran, M., Hamayun, M., Kang, S. M., Kim, Y. H., et al. (2012). Endophytic fungi produce gibberellins and indoleacetic acid and promotes host-plant growth during stress. Molecules 17, 10754–10773. doi: 10.3390/molecules170910754
Waqas, M., Khan, A. L., Shahzad, R., Ihsan Ullah Khan, A. R., and Lee, I. (2015). Endophytic fungi promote plant growth and mitigate the adverse effects of stem rot, an example of Penicillium citrnum and Aspergillus terreus. J. Plant Interact. 10, 280–287. doi: 10.1080/17429145.2015.1079743
White, T. J., Bruns, T., Lee, S., and Taylor, J. (1990). “Amplification and direct sequencing of fungal ribosomal RNA genes for phylogenetics,” in PCR Protocols, A Guide to Methods and Applications, eds M. A. Innis, D. H. Gelfand, J. J. Sninsky, and T. J. White (New York, NY: Academic Press), 315–322.
Wicke, B., Smeets, E., Dornburg, V., Vashev, B., Gaiser, T., Turkenburg, W., et al. (2011). The global technical and economic potential of bioenergy from salt-affected soils. Ene. Environ. Sci. 4, 2669–2681. doi: 10.1039/c1ee01029h
Wolf, H. D., and Rashid, R. (2008). Heavy metal accumulation in Littoraria scabra along polluted and pristine mangrove areas of Tanzania. Environ. Pollut. 152, 636–643. doi: 10.1016/j.envpol.2007.06.064
Wu, J., and Sardo, V. (2010). “Sustainable versus organic agriculture,” in Sociology, Organic Farming, Climate Change and Soil Science, ed. E. Lichtfouse (Dordrecht: Springer), 41–76. doi: 10.1007/978-90-481-3333-8_3
Yasmeen, R., and Siddiqui, Z. S. (2018). Ameliorative effects of Trichoderma harzianum on monocot crops under hydroponic saline environment. Acta Physiol. Plant 40:4. doi: 10.1007/s11738-017-2579-2
Yoo, S. J., Shin, D. J., Won, H. Y., Song, J., and Sang, M. K. (2018). Aspergillus terreus JF27 promotes the growth of tomato plants and induces resistance against Pseudomonas syringae pv. Tomato. Mycobiol. 46, 147–153. doi: 10.1080/12298093.2018.1475370
Yu, X., Zhang, W., Lang, D., Zhang, X., Cui, G., and Zhang, X. (2019). Interactions between endophytes and plants: beneficial effect of endophytes to ameliorate biotic and abiotic stresses in plants. J. Plant Biol. 62:2732.
Keywords: endophytes, Aspergillus terreus, growth regulator, antioxidant enzyme activity, physiological performance, salinity, crop salt resistance
Citation: Siddiqui ZS, Wei X, Umar M, Abideen Z, Zulfiqar F, Chen J, Hanif A, Dawar S, Dias DA and Yasmeen R (2022) Scrutinizing the Application of Saline Endophyte to Enhance Salt Tolerance in Rice and Maize Plants. Front. Plant Sci. 12:770084. doi: 10.3389/fpls.2021.770084
Received: 03 September 2021; Accepted: 21 December 2021;
Published: 17 February 2022.
Edited by:
Mirza Hasanuzzaman, Sher-e-Bangla Agricultural University, BangladeshReviewed by:
Bassam Taha Al-IESSA, Qatar University, QatarSneha Vinay Kumar Gupta, The University of Melbourne, Australia
Copyright © 2022 Siddiqui, Wei, Umar, Abideen, Zulfiqar, Chen, Hanif, Dawar, Dias and Yasmeen. This is an open-access article distributed under the terms of the Creative Commons Attribution License (CC BY). The use, distribution or reproduction in other forums is permitted, provided the original author(s) and the copyright owner(s) are credited and that the original publication in this journal is cited, in accordance with accepted academic practice. No use, distribution or reproduction is permitted which does not comply with these terms.
*Correspondence: Zamin Shaheed Siddiqui, emFtaW5zc0B1b2suZWR1LnBr; Xiangying Wei, eGlhbmd5aW5nd2VpQG1qdS5lZHUuY24=