- 1College of Horticulture and Landscape Architecture, Zhongkai University of Agriculture and Engineering, Guangzhou, China
- 2Institute of Oceanography, Minjiang University, Fuzhou, China
- 3He Xiangning College of Art and Design, Zhongkai University of Agriculture and Engineering, Guangzhou, China
- 4Department of Environmental Horticulture and Mid-Florida Research and Education Center, Institute of Food and Agricultural Sciences, University of Florida, Apopka, FL, United States
Cobalt is a transition metal located in the fourth row of the periodic table and is a neighbor of iron and nickel. It has been considered an essential element for prokaryotes, human beings, and other mammals, but its essentiality for plants remains obscure. In this article, we proposed that cobalt (Co) is a potentially essential micronutrient of plants. Co is essential for the growth of many lower plants, such as marine algal species including diatoms, chrysophytes, and dinoflagellates, as well as for higher plants in the family Fabaceae or Leguminosae. The essentiality to leguminous plants is attributed to its role in nitrogen (N) fixation by symbiotic microbes, primarily rhizobia. Co is an integral component of cobalamin or vitamin B12, which is required by several enzymes involved in N2 fixation. In addition to symbiosis, a group of N2 fixing bacteria known as diazotrophs is able to situate in plant tissue as endophytes or closely associated with roots of plants including economically important crops, such as barley, corn, rice, sugarcane, and wheat. Their action in N2 fixation provides crops with the macronutrient of N. Co is a component of several enzymes and proteins, participating in plant metabolism. Plants may exhibit Co deficiency if there is a severe limitation in Co supply. Conversely, Co is toxic to plants at higher concentrations. High levels of Co result in pale-colored leaves, discolored veins, and the loss of leaves and can also cause iron deficiency in plants. It is anticipated that with the advance of omics, Co as a constitute of enzymes and proteins and its specific role in plant metabolism will be exclusively revealed. The confirmation of Co as an essential micronutrient will enrich our understanding of plant mineral nutrition and improve our practice in crop production.
Introduction
Cobalt is an essential nutrient for prokaryotes, human beings, and other mammals but has not been considered an essential micronutrient for plants. Instead, this element, along with other elements, such as aluminum (Al), selenium (Se), silicon (Si), sodium (Na), and titanium (Ti), has been considered as a beneficial element for plant growth (Pilon-Smits et al., 2009; Lyu et al., 2017). An element that can improve plant health status at low concentrations but has toxic effects at high concentrations is known as a beneficial element (Pais, 1992). For an element to be considered essential, it must be required by plants to complete its life cycle, must not be replaceable by other elements, and must directly participate in plant metabolism (Arnon and Stout, 1939). It has been well-documented that there are 92 naturally occurring elements on the earth, wherein 82 of which have been found in plants (Reimann et al., 2001). Plants are able to absorb elements from soils either actively or passively due to their sessile nature. The occurrence of an element in plants, particularly in shoots, must have a purpose. Active transport of an element from roots to shoots may indicate a certain role it plays in plants. As stated in the study by Bertrand (1912), potentially, every element has a biological function that can be assessed properly against a background of a deficiency state, and every element is toxic when present at high enough concentrations, which is known as Bertrand's rule of metal necessity.
Significant progress has been made in plant mineral nutrition since the publication of Bertrand's rule (Bertrand, 1912) and the essentiality concept (Arnon and Stout, 1939). Among the beneficial elements, cobalt (Co) could potentially be an essential plant micronutrient. Co is a core element of cobalamin (vitamin B12 and its derivatives) and a cofactor of a wider range of enzymes and a component of different proteins in prokaryotes and animals (Maret and Vallee, 1993; Kobayashi and Shimizu, 1999; Harrop and Mascharak, 2013; Odaka and Kobayashi, 2013). Co-containing enzymes and proteins in plants require further investigation and clarification. Rhizobia and other nitrogen (N)-fixation bacteria require Co and cobalamin for fixing atmosphere dinitrogen (N2) into ammonia (NH3), providing plants with the essential macronutrient of N. Co plays a vital role in interaction with iron (Fe), nickel (Ni), and zinc (Zn) in maintaining cellular homeostasis. Similar to other essential micronutrients, plants respond to Co concentrations in soil: at low concentrations, it promotes plant growth but causes phytotoxicity at higher concentrations. However, it is different from other beneficial elements, as plants do exhibit Co deficiency when grown in soils with limited supply.
The objective of this article was to concisely review the importance of Co as a plant micronutrient including its role in N fixation, the occurrence of coenzyme or proteins, and its effects on plant growth as well as Co deficiency and toxicity. We intended that this review could raise an awareness that Co is a potentially essential micronutrient of plants, and further research is needed to confirm this proposition.
Cobalt and Nitrogen-Fixation in Plants
Cobalt was isolated by Brandt in 1735 and recognized as a new element by Bergman in 1780 (Lindsay and Kerr, 2011). The importance of Co to living things was realized in the 1930s during the investigation of ruminant livestock nutrition in Australia (Underwood and Filmer, 1935). Co was discovered to be essential for animals as it is a component of cobalamin. Five scientists were awarded Nobel Prizes for the investigation of cobalamin (Carpenter, 2004).
Cobalt Is a Core Element of Cobalamin
Cobalamin is a large molecule (C63H88O14N14PCo) comprised of a modified tetrapyrrole ring known as corrin with Co3+ in the center (Osman et al., 2021). Co is not inter-exchangeable with other metals in the cobalamin and cannot be released from the ring unless the ring is broken (Yamada, 2013), implying the significance of Co to cobalamin. There are two biologically active forms of cobalamin, namely, methylcobalamin and adenosylcobalamin in ruminants (Gonzalez-Montana et al., 2020). In human beings, Co is a cofactor of two enzymes, namely, ethylmalonyl-CoA mutase (MCM) and methionine synthase. MCM catalyzes the reversible isomerisation of l-methylmalonyl-CoA to succinyl-CoA. A deficiency of MCM causes an inherited metabolism disorder commonly known as methylmalonic aciduria. Methionine synthase utilizes cobalamin as a cofactor to produce methionine from homocysteine (Table 1). Reduced activity of this enzyme leads to megaloblastic anemia (Tjong et al., 2020). Ruminant animals produce vitamin B12 if there is an appropriate supply of Co in their diet. It was reported that 3 to 13% of the Co was incorporated into cobalamin by bacteria in the ruminant animals (Huwait et al., 2015).
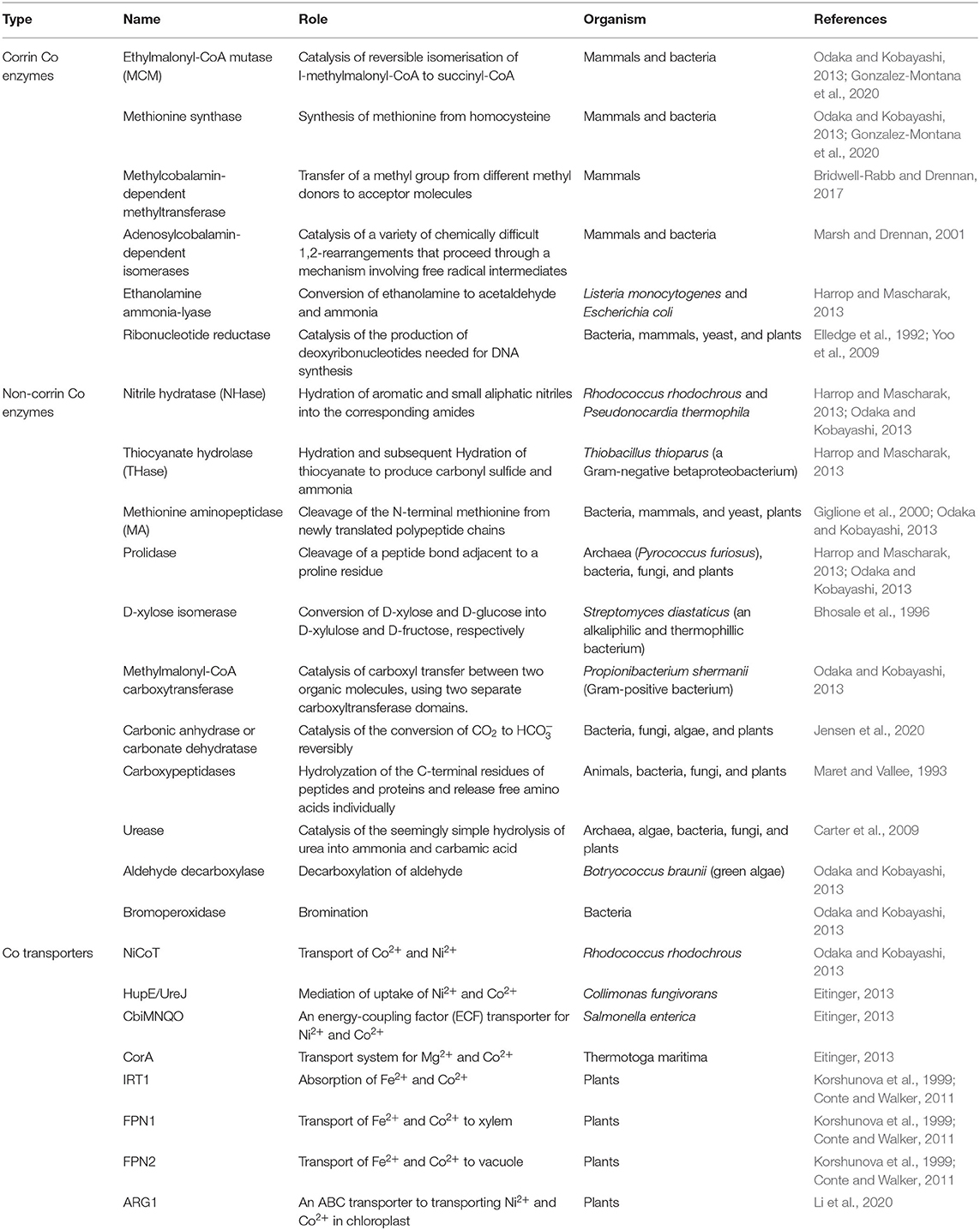
Table 1. Cobalt-containing enzymes, proteins, and transporter relevant or potentially relevant to plant metabolisms.
Cobalamin Biosynthesis in Bacteria and Archaea
The natural forms of vitamin B12 are 1,5-deoxyadenosylcobalamin, hydroxycobalamin, and methylcobalamin (Nohwar et al., 2020). They are synthesized by a selected subset of bacteria and archaea (Heal et al., 2017; Guo and Chen, 2018), which include Bacillus, Escherichia, Fervidobacterium, Kosmotoga, Lactobacillus, Mesotoga, Nitrosopumilus, Petrotoga, Propionibacterium, Proteobacteria, Pseudomonas, Rhodobacter, Rhizobium, Salmonella, Sinorhizobium, Thermosipho, and Thermotoga (Doxey et al., 2015; Fang et al., 2017). Cyanocobalamin is not a natural form but commercially synthesized B12. The production of vitamin B12 by these microbes involves about 30 enzymatic steps through either aerobic or anaerobic pathways. In addition to being essential for fat and carbohydrate metabolism and synthesis of DNA, vitamin B12 is a cofactor of many enzymes. There are more than 20 cobalamin-dependent enzymes in those prokaryotes including diol dehydratase, ethanolamine ammonia-lyase, glutamate, and methylmalonyl-CoA mutase, methionine synthase, and ribonucleotide reductase (Marsh, 1999) (Table 1). These enzymes catalyze a series of transmethylation and rearrangement reactions (Rodionov et al., 2003). Thus, Co is essential for those archaea and bacteria.
Cobalt Plays an Important Role in Biological Nitrogen Fixation
Biological N fixation is a process of converting N2 from the atmosphere into plant-usable form, primarily NH3. Biological N fixation (BNF) is carried out by a group of prokaryotes known as diazotrophs, which are listed in Table 2, including bacteria, mainly Rhizobium, Frankia, Azotobacter, Mycobaterium, Azospirillum, and Bacillus; Archaea, such as Methanococcales, Methanobacteriatles, and Methanomicrobiales, and cyanobacteria, like Anabaena, Nostoc, Toypothrix, and Anabaenopsis (Soumare et al., 2020). N2-fixing organisms are also classified into three categories: symbiotic, endophytic, and associated groups (Figure 1). Such classifications may not be accurate as some of them, such as those from Acetobacter and Azospirillum, could be associated, as well as endophytic bacteria.
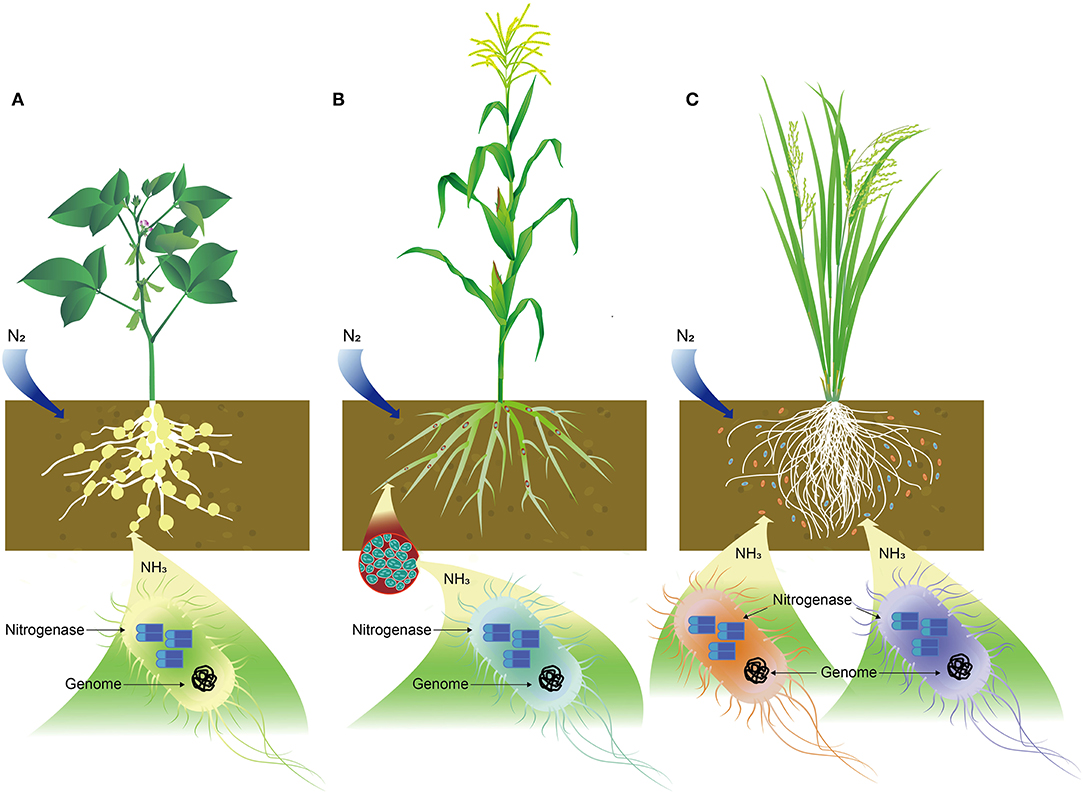
Figure 1. Schematic illustration of nitrogen (N) fixation in bacteria. (A) Symbiotic relationship of Rhizobium with soybean in N fixation, (B) endophytic bacteria, like Azospirillum lipoferum in corn plant root for N fixation, and (C) N-fixing bacteria, such as Azotobacter and Azospirillum associated with rice plant roots where cobalt or cobalamin plays important role in N fixation.
Cobalt Is Essential for Symbiotic Bacteria in N Fixation
There are two major symbioses between N2-fixing bacteria and higher plants, one is rhizobia with leguminous plants and the other is Frankia with actinorhizal plants (Wall, 2000). The former involves more than 1,700 plant species in the family Fabaceae, which includes some economically important crops, such as alfalfa, beans, peas, and soybeans. More than 220 species are actinorhizal plants, which are mainly trees and shrubs forming symbiotic relationships with Frankia.
Rhizobia are gram-negative bacteria encompassing Rhizobium, Azorhizobium, Sinorhizobium, Bradyrhizobium, and Mesorhizobium (Table 2, Figure 1A). Co was identified to be essential for Rhizobium in the 1950s and 1960s (Ahmed and Evans, 1960; Reisenauer, 1960). Rhizobium uses nitrogenase to catalyze the conversion of N2 to NH3, which can be readily absorbed and assimilated by plants. Three enzymes, namely, methionine synthase, methyl malonyl-CoA mutase, and ribonucleotide reductase in Rhizobium and Bradyrhizobium species, are known to be cobalamin-dependent and significantly affect nodulation and N fixation. Early studies showed that four soybean seedlings inoculated with rhizobia supplemented with 1 μg/L Co were healthy and produced 25.3 g of dry weight. On the contrary, four rhizobia-inoculated seedlings devoid of Co encountered N-deficiency symptoms and produced 16.6 g of dry weight, a 34.4% reduction in biomass due to the absence of Co (Ahmed and Evans, 1959). A close relationship was established amongst Co supply, cobalamin content in Rhizobium, leghemoglobin formation, N fixation, and plant growth (Kliewer and Evans, 1963a,b). The deficiency in Co significantly affects methionine synthase by reducing methionine synthesis, which subsequently decreases protein synthesis and produces smaller-sized bacteroids (bacteria in the nodules capable of N fixation) (Marschner, 2011). Methyl malonyl-CoA mutase catalyzes the production of leghemoglobin. If Co becomes limited, leghemoglobin synthesis is directly affected, resulting in reduced N fixation and ultimately a shortage of N supply. This is because leghemoglobin can protect nitrogenase from oxygen by limiting its supply (Hopkins, 1995). Ribonucleotide reductase is a cobalamin-dependent enzyme that catalyzes the reduction of ribonucleotides to deoxyribonucleotides, which is a rate-limiting step in DNA synthesis (Kolberg et al., 2004).
The genus Frankia is composed of gram-positive and gram-variable actinomycetes (Wall, 2000). It infects plants through root hairs and produces nodules in the pericycle. Frankia in nodules develops vesicles in which nitrogenase is suited (Huss-Danell, 1997). Co is needed for the synthesis of cobalamin which is in turn needed for N fixation. Actinomyceters are known as active producers of cobalamin (Hewitt and Bond, 1966). N fixation by actinorhizal plants appears to be comparable to the magnitude as that of the legumes (Wall, 2000).
Other symbioses occur in cyanobacteria with Gunnera and cycads. The genus Nostoc infected specialized gland organs located on the stems of Gunnera, such as G. chilensis and G. magellanica (Johansson and Bergman, 1994). Cyanobacteria also form symbiotic relationships with cycads in a special type of root system called coralloid roots (Chang et al., 2019). It has been well-documented that cyanobacteria require Co for the biosynthesis of cobalamin (Cavet et al., 2003).
Cobalt and Endophytic Bacteria in N Fixation
A group of N2-fixing bacteria can form an endophytic relationship with many crop plants (Table 2, Figure 1B). By definition, any bacterium could be considered to be an endophytic diazotroph if (1) it can be isolated from surface-disinfected plant tissue or extracted inside the plants, (2) it proves to be located inside the plant, either intra- or inter-cellularly by in-situ identification, and (3) it fixes N2, as demonstrated by acetylene reduction and/or 15N-enrichment (Hartmann et al., 2000; Gupta et al., 2012). Common N2-fixing endophytic bacteria include Azoarcus spp. BH72 and Pseudomonas stutzeri A1501 in rice (Wang et al., 2016; Pham et al., 2017), Achromobacter spp. EMC1936 in tomato (Abdel-Rahman et al., 2017), Azospirillum lipoferum 4B in maize (Garcia et al., 2017), Burkholderia phytofirmans PsJN in grape plants (Compant et al., 2008), Enterobacter cloacae ENHKU01 in pepper (Santoyo et al., 2016), Gluconoacetobacter diazotrophicus PaI5 in sugarcane (James et al., 2001). Other bacteria, such as Herbaspirillum, Klebsiella, and Serratia also are implicated in N2 fixation (Rothballer et al., 2008; Franche et al., 2009). These bacteria possess either iron or vanadium nitrogenase that fixes N2 into NH3.
The complete genome of Azoarcus sp. BH72 (Krause et al., 2006), G. diazotrophicus PAl 5 (Bertalan et al., 2009), Herbaspirillum seropedicae SmR1 (Pedrosa et al., 2011), and S. marcesens RSC-14 (Khan et al., 2017) were sequenced. Among them, genomic and proteomic profiles of Azoarcus sp., Gluconoacetobacter diazotrophicus, Herbaspirillum seropedicae, and Serratia marcesens have been studied (Krause et al., 2006; Gupta et al., 2012). These bacteria have co-transport systems for Co2+, Zn2+, and Cd2+ or Ca2+, Co2+, Zn2+, and Cd2+ as well as putative receptors for vitamin B12. Comparative genomic analyses of Ni, Co, and vitamin B12 utilization showed that both metals are widely used by the bacteria and archaea, with the most common prokaryotic transporter being Cbi/NikMNQO. Ni-Fe hydrogenase, Ni-dependent urease, B12-dependent ribonucleotide reductase, methionine synthase, and methymalonly-CoA mutase are the most widespread metalloproteins for Ni and Co (Zhang et al., 2009). Thus, Co is needed by these bacteria.
Cobalt and Plant Associated N2 Fixing Bacteria
Associated N2-fixing bacteria include Azotobacter, Azospirillum, Beijerinckia, Burkholderia, Clostridium, Herbaspirillum, Gluconacetobacter, Methanosarcina, and Paenibacillus (Table 2, Figure 1C). These bacteria are associated with the roots of a wide range of plants, including corn, rice, sugarcane, and wheat (Aasfar et al., 2021). Among them, the genus Azotobacter was first reported in 1901 and has been used as a biofertilizer thereafter (Gerlach and Vogel, 1902). Notable species found in soils are A. chroococcum, A. vinelandii, A. beigerinckii, A. armeniacus, A. nigricans, and A. paspali (Das, 2019). The genome of A. vinelandii DJ has been sequenced (Setubal et al., 2009). N fixation in these species is under aerobic conditions, and two-component proteins of Mo-dependent nitrogenase catalyze N2 into NH3. Co and vitamin B12 were found to be required by A. vinelandii OP. Additionally, 5,6-dimethylbenzimidazolylcobamide coenzyme was identified in this species, which might play an important role in N fixation (Nicholas et al., 1962). Furthermore, higher concentrations of Co were needed for A. vinelandii to fix N2 than was needed for the utilization of ammonium compounds (Evans and Kliewer, 1964). Co at a concentration of 0.1 mg/L was reported to increase N fixation in A. chroococcum in Jensen's medium (Iswaran and Rao Sundara, 1964). Culture of A. chroococcum in half-strength N-free Jensen's broth showed that N fixation was enhanced after supplemented with Co at 12.5 mg/L or 25 mg/L (Orji et al., 2018). Azotobacters were able to biosynthesize a series of vitamins, including B12 in chemically-defined media and dialyzed soil media (Gonzalez-Lopez et al., 1983; El-Essawy et al., 1984). In addition to A. vinelandii and A. chroococcum, Pseudomonas fluorescens, Bacillus megaterium, Bacillus firmus, and Sinorhizobium meliloti also produce cobalamin (Palacios et al., 2014), and the synthesized cobalamin may implicate the enhanced N fixation in these bacteria.
Azosprillum is another important genus of plant-associated N2-fixing bacteria. A. brasilense cultured on medium supplemented with 0.2 mM Co was able to accumulate Co up to 0.1 to 0.6 mg per gram of dry biomass (Kamnev et al., 2001). 57Co emission Mössbauer spectroscopy (EMS) studies of Co in Azospirillum brasilense Sp245 showed that Co activated glutamine synthetase to have two different Co forms at its active sites. In vitro, biochemical and spectroscopic analyses showed that Co2+ is among the divalent cations, along with Mg2+ and Mn2+, most effective in supporting the activity of glutamine synthetase at different adenylylation states, a key enzyme of N metabolism (Antonyuk et al., 2001).
Nitrogen Fixing Bacteria and Crop Productivity
Nitrogen is an essential macronutrient for plants. The application of synthetic N fertilizers has greatly enhanced crop production but also has caused serious environmental problems, such as groundwater contamination and surface water eutrophication (Hansen et al., 2017). As a result, exploring the potential of BNF becomes increasingly important. The symbiotic relationship between rhizobia and legume crops was considered the most important BNF system and estimated to contribute to 227 to 300 kg N/ha/year (Roughley et al., 1995; Herridge et al., 2008). N2 fixation by actinorhizal plants was estimated to be 240-350 kg N/ha/year (Wall, 2000).
Nitrogen fixation by plant-associated diazotrophs has been estimated to be 60 kg N/ha/year (Gupta et al., 2006; Reed et al., 2011). Moreover, the abundance of associated diazotrophs, such as Azotobacter species in the soil provides not only N (Din et al., 2019) but also phosphorus and plant growth regulators, which resulted in a yield increase of up to 40% in cereals and pulse crops (Yanni and El-Fattah, 1999; Choudhury and Kennedy, 2004; Kannan and Ponmurugan, 2010; Ritika and Dey, 2014; Wani et al., 2016; Velmourougane et al., 2019). Such beneficial effects have been harnessed ecologically in the engineering of Azotobacter species for fixing plant needed N, while reducing the reliance on synthetic N fertilizers for crop production in an environmentally friendly manner (Wani et al., 2016; Bageshwar et al., 2017; Ke et al., 2021).
Endophytic bacteria also contribute significantly to N input. Azoarcus is an endophytic N2-fixing diazotroph, and its action in roots of kallar grass increased hay yield up to 20–40 t/ha/year without N fertilizer application in saline-sodic, alkaline soils (Hurek and Reinhold-Hurek, 2003). Gluconoacetobacter diazotrophicus (Acetobacter diazotrophicus) is the main contributor in sugarcane and can fix up to 150 kg N/ha/year (Dobereiner et al., 1993; Muthukumarasamy et al., 2005). Many C-4 energy plants, such as Miscanthus sacchariflorus, Spartina pectinate, and Penisettum purpureum can harbor endophytic bacteria, which support the N requirement of these plants (Kirchhof et al., 1997). Gupta et al. (2012) reported that N derived from the air by endophytic bacteria for rice ranged from 9.2 to 47% depending on bacterial species. These results indicate that endophytic diazotrophs have a great potential to enhance the productivity of non-leguminous crops.
The aforementioned bacteria essentially act as the same as gut bacteria in mammals by living between plant cells as endophytes, close association with roots, or symbiotically and become indispensable for plant growth and development. Microorganisms are associated with all plant organs (Wei et al., 2017), but roots have the largest number and greatest range of microbes. Thus, a plant growing under field conditions is a community, not an individual. Such associations are collectively termed “phytomicrobiome.” The phytomicrobiome is integral for plant growth and function. Microbes play important roles in plant nutrient acquisition, biotic and abiotic stress management, physiology regulation through microbe-to-plant signals, and growth regulation via the production of phytohormones. The foregoing discussion documents the role of Co plays in N2 fixing rhizosphere bacteria. If we accept that coevolution exists between microbes and plants and the phytomicrobione in general, Co should be considered as an essential element to plants as it is required by symbiotic, endophytic, and associated bacteria.
Cobalt Coenzymes and Proteins
Cobalamin is a cofactor of adenosylcobalamin-dependent isomerases, ethanolamine ammonia-lyase, methylcobalamin-dependent methyltransferase, and ribonucleotide reductase in animals and bacteria (Table 1). Co is also a cofactor of non-corrin coenzymes or metalloproteins including aldehyde decarboxylase, bromoperoxidase-esterase, D-xylose isomerase, methionine aminopeptidase (MA), methylmalonyl-CoA carboxytransferase, nitrile hydratase (NHase), prolidase, and thiocyanate hydrolase (THase) in animals, bacteria, and yeasts. However, cobalamin-dependent enzymes or Co-proteins in plants remain obscure.
Cobalt Proteins in Plants
There are several lines of evidence suggesting that plants may have cobalamin-dependent enzymes and Co-containing proteins: (1) The ancestor of the chloroplast is cyanobacteria (Falcón et al., 2010), and Co is required by this group of bacteria. The speculation is that Co may be needed by plants. (2) Plants have been documented to utilize cobalamin produced by symbiotic, endophytic, and associated N2 fixing bacteria. Cobalamin concentrations of 37, 26, and 11 μg/100 g dry weight were detected in Hippophae rhammoides, Elymus, and Inula helenium, respectively (Nakos et al., 2017). There is a possibility that cobalamin-dependent enzymes may occur in plants. Poston (1977) reported the identification of leucine 2,3-aminomutase in extracts of bean seedlings. Its activity was stimulated by coenzyme B12 but inhibited by unknown factors. The inhibition was removed by the addition of B12, suggesting the presence of a cobalamin-dependent enzyme in higher plants. Subsequently, two coenzyme B12-dependent enzymes: leucine 2,3-aminomutase and methylmalonyl-CoA mutase were reported in potato tubers (Poston, 1978), but methylmalonyl-CoA mutase was found to be a phosphatase (Paizs et al., 2008). (3) Co is required by lower plants, which is to be discussed in the following section. (4) Plants can take up and transport cobalamin (Mozafar, 1994; Sato et al., 2004). A recent study using fluorescent analogs to follow the uptake and transport of cobalamin showed that Lepidium sativum can absorb cobalamin (Lawrence et al., 2018). Seed priming with cobalamin provided significant protection against the salt stress of common beans (Keshavarz and Moghadam, 2017). The incorporation of Co in plant tissue culture media significantly improves plantlet production (Bartolo and Macey, 1989). (5) Co as a metal cofactor of some additional enzymes and proteins are briefly discussed below (Table 1).
Carbonic anhydrase or carbonate dehydratase (CA, EC: 4.2.1.1) is a metalloenzyme catalyzing the conversion of CO2 to reversibly in many organisms including plants, particularly C4 and CAM plants. Eight different CA classes have been described as α-, β-, γ-, δ-, ζ-, η-, θ-, and a recently described ι-CA in microalgae. The metalloenzymes commonly use Zn2+ as a metal cofactor. However, Zn2+ in γ class can be replaced by Co2+ and Fe2+ in prokaryotes, fungi, algae, and plants, but in δ class is only can be replaced by Co2+ in marine phytoplankton (Jensen et al., 2020).
Carboxypeptidases (CPSs, EC: 3.4.16–3.4.18) are proteases hydrolyzing the C-terminal residues of peptides and proteins and release free amino acids individually. CPSs are divided into serine (EC: 3.4.16), metal (EC: 3.4.17), and cysteine (EC: 3.4.18) and occur in animals, bacteria, fungi, and plants. One Zn atom is essential to the catalytic activity of native carboxypeptidase A. Zn can be removed by dialysis at low pH or with chelating agents at neutral pH, which results in the inactivation of the enzyme. The re-addition of the metal restores the dual activities of carboxypeptidase toward peptides and esters. Co was found to be more active than Zn in the enzyme toward peptides and has nearly the same activity toward esters, indicating that Co in the active site is virtually identical to that of Zn in the native enzyme (Maret and Vallee, 1993).
Methionine aminopeptidase (MAP, EC 3.4.11.18) is widely documented in animals, bacteria, yeast, and plants. It is a Co-dependent enzyme responsible for the cleavage of the N-terminal methionine from newly translated polypeptide chains. Two classes of MAPs (MAP1 and MAP2) were reported in bacteria, and at least one MAP1 and one MAP2 occur in eukaryotes (Giglione and Meinnel, 2001). In Arabidopsis, there are four MAP1s (MAP1A, MAP1B, MAP1C, and MAP1D) and two MAP2s (MAP2A and MAP2B), along with two class 1 peptide deformylases (PDF1A and PDF1B). The plant MAP proteins show significant similarity to the eubacterial counterparts except for MAP1A and two MAP2s. It has been documented that the substrate specificity of PDFs and both organellar and cytosolic MAPs in plants are similar to that of their bacterial counterparts (Giglione et al., 2000). The MAP from Salmonella typhimurium is stimulated only by Co2+, not by Mg2+, Mn2+, or Zn2+ and is inhibited by metal ion chelator EDTA. E. coli MAP is a monomeric protein of 29 kDa consisting of 263 residues that possess two Co2+ ions in its active site (Permyakov, 2021).
Prolidase (PEPD, EC 3.4.13.9) hydrolyze peptide bonds of imidodipeptides with C-terminal proline or hydroxyproline, thus liberating proline. PEPD has been identified in fungi, plants (Kubota et al., 1977), archaea, and bacteria. The preferable substrate requires metal ions Mn2+, Zn2+, or Co2+.
Peroxidases are isoenzymes present in all organisms, which catalyze redox reactions that cleave peroxides; specifically, it breaks down hydrogen peroxide. The study of Han et al. (2008) found that Co2+ at a concentration below 0.1 mM increased horseradish peroxidase activity because Co2+ binds with some amino acids near or in the active site of the enzyme.
Urease is an enzyme occurring in selected archaea, algae, bacteria, fungi, and plants. It catalyzes the hydrolysis of urea into ammonia and carbamic acid. The active site of urease contains two Ni2+ atoms that are bridged by a carbamylated lysine residue and a water molecule (Carter et al., 2009). The study of Watanabe et al. (1994) reported that urease activity of cucumber leaves was markedly reduced when Ni concentration became <100 ng/L, but supplementing Co restored urase activity. Additionally, urease was also activated by both Co and manganese (Mn) through in vitro assay (Carter et al., 2009).
Cobalt transporters. Transporters specifically for Co have not been reported. The current understanding is that Co can be transported through Fe transporters (Figure 2). In Arabidopsis thaliana, Co is taken up from the soil into epidermal cells of roots by IRON-REGULATED TRANSPORTER 1 (IRT1), which is commonly known for absorption of Fe (Korshunova et al., 1999). Once Co is absorbed inside cells, Ferroportins, FPN1, and FPN2 are responsible for its further movement. IREG1/FPN1 is localized to the plasma membrane and expressed in the steel, indicating it is responsible for the loading of Fe to xylem, and FPN2 is situated the in vacuolar membrane and involved in buffering Fe concentration in the cytosol (Morrissey et al., 2009). Truncated FPN2 causes an elevated level of Co in shoots, while the loss of FPN1 abolishes Co accumulation in shoots. A double mutant of fpn1 fpn2 is unable to sequester Co in root vacuole and cannot transport Co to shoots. These results suggest that Co is likely absorbed and transported in the same way as Fe in plants (Figure 2). Additionally, an ATP-binding cassette (ABC) transporter from Arabidopsis has also been reported to transport Co, Ni, and Pb (Morel et al., 2009). Co movement in leaves is also associated with Ni, and Ni and Co movement in or out of chloroplasts are through an ABC transporter in the mediation of ionic homeostasis in the chloroplast of rice (Li et al., 2020).
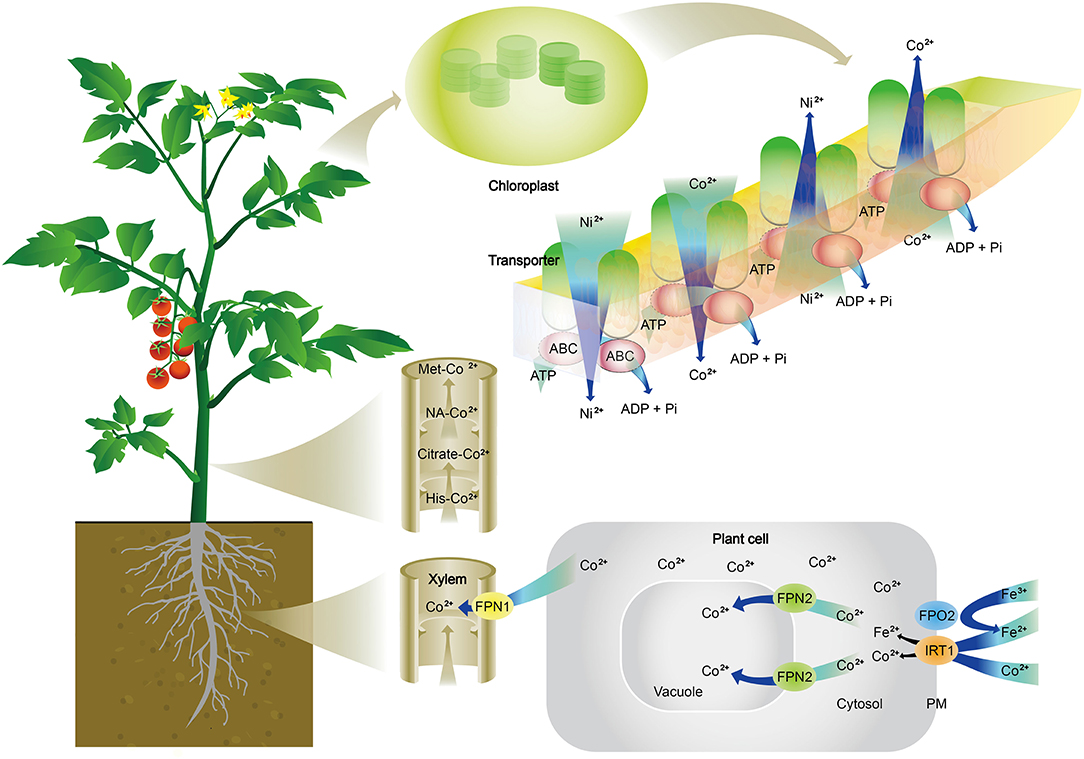
Figure 2. Schematic illustration of cobalt (Co2+) absorption, transport, and distribution in plants. Co2+ is absorbed from the soil into epidermal cells of roots by an iron transporter (IRT1). Once Co2+ is absorbed inside cells, Ferroportins (FPN1 and FPN2) are responsible for its further movement. FPN2 transports Co2+ into vacuoles, resulting in the sequestration of Co in root cells. FPN1 is to load Co2+ into the xylem. In the xylem, Co2+ is complexed with citrate, histidine (His), methionine (Met), or nicotianamine (NA) to be translocated to shoots. Co2+ is released in leaves and participate in metabolisms, which are often associated with nickel (Ni) and iron (Fe). It is shown here the Ni and Co movement in or out of chloroplasts through ATP-binding cassette (ABC) transporter in the mediation of ionic homeostasis in the chloroplast.
Cobalt Substitution of Other Metals
A characteristic of Co is its ability to substitute for other transition metals in a large number of enzymes. Maret and Vallee (1993) listed 37 Co-substituted metalloproteins, of which 24 are native to Zn, nine to copper (Cu), and four to Fe. These enzymes mainly occur in animals, bacteria, and yeast, while a few are in plants. Such a characteristic is closely related to the properties of Co with other metals. The ionic radius of Co2+ is 0.76 Å, which is similar to 0.74 Å of Zn2+, 0.69 Å for Cu2+, and 0.76 Å for Fe2+. Additionally, based on the available Protein Data Bank structures with Co2+, the study Khrustalev et al. (2019) found that Co2+ is commonly bound by cation traps. The traps are formed by relatively negatively charged regions of random coil between a β stand and α helix and between two β strands in which His, Asp, and Glu residues are situated. On the other hand, these sites are also occupied by other metals ions, such as Cu2+, Mg2+, Mn2+, and Zn2+, which play significant roles as catalysts. As a result, Co2+ could rather readily substitute for these ions in the active sites of enzymes. Additionally, based on the FIND-SITE-metal, a program for the prediction of the metal-binding site, the study of Brylinski and Skolnick (2011) found that Zn, due to a lower coordination number preference, is typically chelated with Cys and His, and His residues have a strong preference for Co, Cu, Fe, Ni, and Zn atoms. Thus, Co is able to replace Cu, Fe, Ni, and Zn in the active sites of enzymes. For example, Co addition alleviated Zn limitation in production of Thalassiosira weissflogii, which was due to Co substitution of Zn in the main isoform of carbonic anhydrase (Yee and Morel, 1996). Co substitution of Zn was also reported in two northeast Pacific isolates of diatoms Pseudo-nitzschia delicatissima UNC1205 and Thalassiosira spp. UNC1203 (Kellogg et al., 2020). Co2+ has been used as a spectroscopically active substitute for Zn2+ in enzymes (Bennett, 2010). Substitution of tetrahedral Zn2+ by higher-coordinate Co2+ often results in a catalytically active species, sometimes with catalytic properties perhaps unexpectedly similar to those of the native enzyme. In the vast majority of cases, no other transition ion than Co2+ provides a better substitute for Zn2+ (Maret and Vallee, 1993; Bennett, 2010). Due to these reasons, Co specific enzymes or proteins have not been conclusively identified. With the advance of omics, functions of a large number of gene sequences have not been assigned. Using the FIND-SITE-metal, a program developed for prediction of the metal-binding site, Brylinski and Skolnick (2011) predicted that about 10,953 putative metal-binding proteins in human proteome were bound with Ca, 10,534 bound with Mg, 8,681 with Zn, 1,863 with Fe, 1,246 with Mn, 652 with Co, 476 with Cu, and 403 with Ni. The predicted binding proteins with Co are greater than Cu and Ni in humans. Based on this assignment in the human proteome, it could be extremely difficult to believe that there are no Co-containing enzymes and proteins in plants.
Cobalt Is Essential for Lower Plants
Lower plants are commonly known as non-vascular plants because they do not have xylem and phloem vascular systems. Non-vascular plants are generally divided into bryophytes and algae.
Bryophytes
Bryophytes are seedless plants including Anthocerotophyta (hornworts), Bryophyta (mosses), and Marchantiophyta (liverworts) (Davies et al., 2020). This group of plants is able to absorb Co from air, soil, and water. In an early geochemical survey performed in Wisconsin and adjacent states and Missouri and Kentucky in the US, the study of Shacklette (1965) documented that the mean concentration of Co in 38 samples of liverworts and mosses was 32 mg/kg, and the concentration in the lower plants was closely related to the amount of the element in the soil, suggesting they act as a bioindicator of Co concentration in the environment (Baker, 1981). Mosses sampled from streams of the Idaho Cobalt Belt (U.S.) showed that Co concentrations in the plants almost perfectly correlated with those in the sediments, and the maximum content of Co (2,000 mg/kg) in moss ash corresponded to the maximum concentration of 320 mg/kg in the sediment (Erdman and Modreski, 1984). Mosses, such as Bryum argenteum and Hypnum cupressiforme were also considered to be bioindicators for monitoring heavy metal contamination in the air (Andić et al., 2015). Interestingly, the accumulation of Co did not cause any physiological damages to plants, but their growth was further enhanced.
The ability to take up Co could be related to the non-vascular nature and unidentified transporter. A radiolabel study showed that the total amount of 60Co accumulated in P. commune and D. scoparium under given conditions were 7.1 and 6.1 mg/kg, respectively. More than 95% of 60Co in D. scoparium was localized extracellular, while 70% of 60Co in P. commune was localized extracellular and about 20% localized intracellularly. These results showed that Co was largely adsorbed extracellularly, and there were unidentified transporters regulating the transport of Co into intracellular sites.
The enhanced growth could be in part attributed to the symbiotic relationship with cyanobacteria. Some bryophytes, primarily liverworts, and hornworts can form a symbiosis with cyanobacteria, such as Nostoc spp. After infection, Nostoc underwent some morphological and physiological changes by reducing growth rate and CO2 fixation but enhancing the fixation of N2 as well as releasing fixed N compounds to the plants. Cyanobacteria, like rhizobia, require cobalamin as a cofactor for nitrogenase complex to fix N2 (Böhme, 1998). Thus, cyanobacteria-bryophyte symbioses require Co.
Algae
Algae constitute a polyphyletic group ranging from unicellular microalgae, like chlorella and diatoms to multicellular forms, such as the giant kelp, seaweeds, and charophytes (Barsanti and Gualtieri, 2006). Co is essential to some marine algal species, including charophyte, diatoms, and dinoflagellates (Nagpal, 2004). Green alga Chlorella salina exhibited two phases of uptake of Co2+ (Garnham et al., 1992). The initial phase was rapid and independent of metabolism, and the second phase was slow and dependent on metabolism. Competition studies showed that the Co2+ uptake system was different from that for Mg2+, Mn2+, and Zn2+. The greatest amount of Co was associated with the cell wall. Co concentrations in the cytosol were 0.17 mM but 2.89 mM in the vacuole, suggesting that Co transport was well-controlled in C. salina. In the work of Czerpak et al. (1994), they studied the responses of a freshwater green alga Chlorella pyrenoidosa to different levels of Co and found that Co in a range from 5 to 50 mM significantly enhanced the growth of Chlorella pyrenoidosa, including 150–160 and 50–60% increase in fresh and dry weights, respectively. Such increase was related to the increase of chlorophylls a and b by 45–65%, water-soluble proteins by 19–20%, total carotenoids 55–65%, and monosaccharides content 55–60%, when compared with the culture devoid of Co. Although mechanisms behind the stimulating effects have not been elucidated, it is likely due to the biosynthesis of cobalamin that enhanced alga growth. Two cobalamin coenzyme 5′-deoxyadenosylcobalamin and methylcobalamin occurred in green alga C. vulgaris, and the addition of cobalamin significantly stimulated green alga growth (Watanabe et al., 1997). Moreover, C. vulgaris grown in Bold's basal medium supplemented with 2 and 2.5 μM CoCl2 produced 166.23 and 173.32 μg vitamin B12 per 100 g dry weight (Jalilian et al., 2019). Additionally, many algal species require different combinations of cobalamin, vitamin B1, and B7 (Croft et al., 2005) as they do not have pathways to synthesize cobalamin or may use alternative cobalamin-independent routes bypassing the need for the vitamin (Cruz-Lopez and Maske, 2016; Yao et al., 2018). As Co is a constituent of cobalamin, Co is required by those algae.
Some algal species, such as those in the genera Coccomyxa and Elliptochloris as well as diatoms form symbiotic relationships with cyanobacteria (Grube et al., 2017). Co is required for the growth of cyanobacteria, such as Anabaenza cylindrica Lemm (Holm-Hansen et al., 1954) and Prochlorococcus (Hawco et al., 2020) as they need it for N fixation in specialized cells called heterocysts. Thus, algal species symbiotic with cyanobacteria require Co for N-fixation.
Cobalt Improves the Growth of Higher Plants
Cobalt content in the crust of the earth ranges from 15 to 30 mg/kg (Roberts and Gunn, 2014). Co in soils is closely related to the weathering of parental minerals, such as cobaltite, smaltite, and erythrite (Bakkaus et al., 2005) as well as Co pollution (Mahey et al., 2020). Co in the surface soils of the world varies from 4.5 to 12 mg/kg with the highest level occurring in heavy loamy soils and the lowest in organic and light sandy soils (Kabata-Pendias and Mukherjee, 2007). However, Co in reference soil samples was found to differ from 5.5 to 29.9 mg/kg in the United States (U.S.) and 5.5 to 97 mg/kg in Chinese soils (Govindaraju, 1994). Pilon-Smits et al. (2009) suggested that soil Co concentrations generally range from 15 to 25 mg/kg.
Cobalt in Higher Plants
Plants absorb Co. Table 3 lists Co concentrations in over 140 non-hyperaccumulating species ranging from 0.04 to 274 mg/kg. Average concentrations of Co in grasses vary from 60 to 270 μg/kg and in clover differ from 100 to 570 μg/kg across Australia, Finland, Germany, Great Britain, Japan, New Zealand, Poland, Sweden, and the US (Kabata-Pendias and Mukherjee, 2007). Legumes absorb more Co than grasses. Plants that accumulate metals to a level 100-fold higher than those typically recorded in common plants are known as hyperaccumulators (Brooks, 1998).
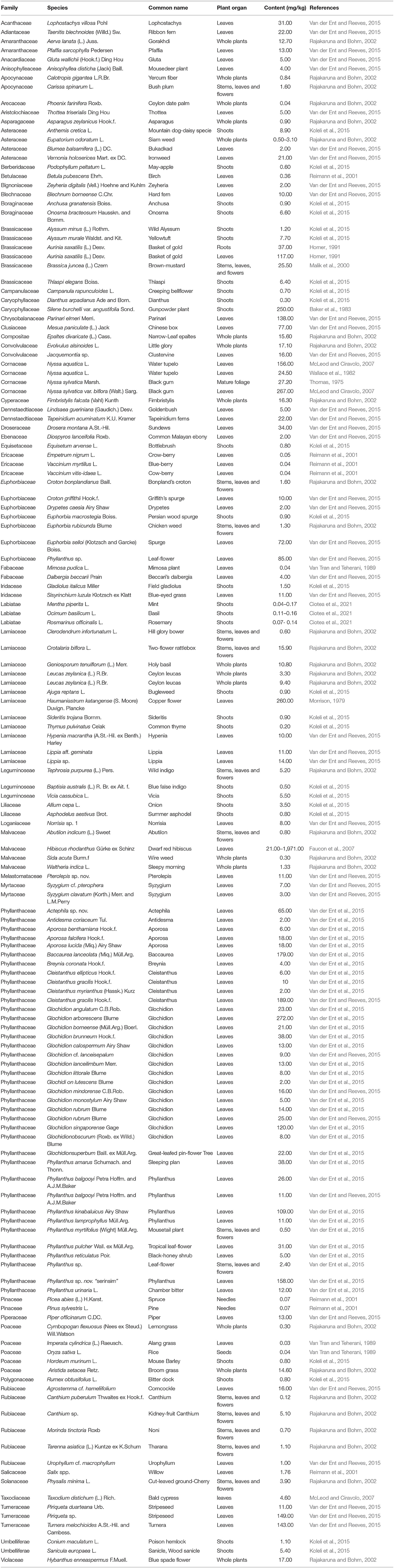
Table 3. The concentration of cobalt in higher plants with the exclusion of cobalt hyperaccumulators.
As discussed above, Co specific transporters have not been reported, and a schematic diagram for Co absorption and translocation is presented in Figure 2. After absorption by roots, Co is either sequestrated in the vacuole of root cells or transported to shoots. Co that is being transported to shoots is chelated with ligands. Co has little affinity with phytochelatins (Chen et al., 1997; Cheng et al., 2005), thus the ligands are not likely Co-S bonds. The study by Collins et al. (2010) reported that Co2+ was complexed with carboxylic acids, which were transported from roots to shoots in wheat or tomato plants. Other ligands are citrate or malate as well as non-proteinogenic amino acids, such as histidine and nicotianamine (Figure 2). Co has low mobility within the leaf tissue and is largely distributed in the vascular system of tomato and wheat leaves (Collins et al., 2010). Co transport from roots to shoots is well-controlled. Using radiolabeled 57Co, Page and Feller (2005) studied Co transport in wheat plants and found that 80% of 57Co remained in roots after 4 days of culture, and 50% was retained in the roots after 50 days; during which, some 57Co moved to the apical part of the main roots, suggesting that the loading of Co to the xylem is well-controlled, probably by FPN1 in wheat plants. In another study, Collins et al. (2010) reported that tomato and wheat plants grown in a nutrient solution containing 2.94 mg/L Co had 4,423 μg/kg and 9,319 μg/kg of Co in roots, respectively; but shoot concentrations of Co were 1,581 μg/kg and 395 μg/kg, respectively. This means that 35.7% of Co absorbed by tomato and 4.2% of Co absorbed wheat plants were transported from roots to shoots. Furthermore, for the 1,581 μg/kg Co in tomato shoots, 846 μg/kg was in the stem, 492 μg/kg in old leaves, only 243 μg/kg in young leaves, indicating that only 5.5% of absorbed Co is transported to actively growing shoots of tomato plants. These transport patterns are like those of titanium (Lyu et al., 2017) which are strictly controlled by plants. These findings imply that plants probably have unidentified transporters specifically for the transport of Co. Due to its toxicity at higher concentrations, the rigorous control of the transport and distribution would ensure that only an appropriate amount of Co could be transported to actively growing shoots. On the other hand, why was more Co transported to dicot tomato shoots than monocot wheat shoots? One explanation could be that different plants have different ligands for complexing Co, and Co complexed by ligands in tomato was more mobile than that in wheat. Another explanation could be that tomato plants need more Co to fulfill some unidentified roles in shoots. Further research is needed to verify these propositions.
To maintain ionic homeostasis in shoots, particularly in chloroplasts, plants develop mechanisms to mediate Co in chloroplasts. An ARG1 transporter, belonging to the ATP-binding cassette, was identified in rice (Li et al., 2020), which was able to modulate the levels of Co and Ni in chloroplasts to prevent excessive Co and Ni from competing with metal cofactors in chlorophyll and metal-binding proteins in photosynthesis (Figure 2).
Plant Growth Improvement
Cobalt at low concentrations can also promote the growth of non-leguminous crops (Table 4). Co applied to a sandy soil at 1 mg/kg enhanced shoot and root dry weights of wheat by 33.7 and 35.8%, respectively compared with the control (Aery and Jagetiy, 2000), and the same Co rate applied to a sandy loam soil increased shoot and root dry weights of wheat by 27.9 and 39.6%, respectively, compared with the control. The yield and essential oil contents of parsley (Petroselinum crispum) increased considerably after the application of Co at 25 mg/kg soil (Helmy and Gad, 2002). Plant height, branch numbers, and fruit numbers as well as anthocyanin and flavonoids contents of Hibiscus sabdariffa significantly increased after application of Co at 20 and 40 mg/kg (Aziz et al., 2007). Application of 50, 100, 150, 200, and 250 mg/kg Co to corn plants showed that the root length, shoot height, and the number of cobs and seeds per plant increased when plants were applied with 50 mg/kg Co, but these parameters decreased with 100 mg/kg Co and above (Jaleel et al., 2009). Co applied at 10 mg/kg significantly enhanced the growth of two onion cultivars, bulb yields, bulb length, and bulb quality, such as nutrient and essential oil contents. Bulb diameter and bulb weights were much higher than the control treatment (Attia et al., 2014), but Co concentrations higher than 10 mg/kg significantly reduced the promotive effects.
Explanations for the improved growth of non-leguminous plants vary but can be summarized as follows: (1) enhanced tolerance to abiotic stresses, (2) activation of antioxidative enzymes, (3) substitution of active metals, and (4) hormesis. Application of Co has been reported to alleviate drought, salt, heavy metal stresses, thus plant growth is not adversely affected. Co has been reported to suppress plant uptake of cadmium (Chmielowska-Bak et al., 2014). Co application increased free proline accumulation, which counteracted the salt stress. In general, abiotic stresses often cause plant imbalance between production and accumulation of reactive oxygen species (ROS), including superoxide anion (), hydroxyl radical (OH−), and hydrogen peroxide (H2O2) (Sachdev et al., 2021). ROS can activate the antioxidant system of the plant, thus minimizing the damages (Tewari et al., 2002; Choudhury et al., 2017). The antioxidant system includes enzymatic antioxidants: ascorbate peroxidase, catalase, dehydroascorbate reductase, general peroxidases, glutathione reductase, monodehydroascorbate reductase, and superoxide dismutase as well as non-enzymatic antioxidants, mainly ascorbic acid, α-tocopherol, carotenoids, reduced glutathione, plastoquinone/ubiquinone, and flavonoids (García-Caparrós et al., 2020). The action of the antioxidant system could be the first line of defense against the adverse effects. Therefore, it is not surprising to notice increased activities of ascorbate peroxidase, catalase, peroxidase, and superoxide dismutase (Hasanuzzaman et al., 2020). Co applied at appropriate concentrations can activate antioxidative enzymes, thus reducing ROS-caused damage. As discussed previously, Co may substitute other nutrient elements when such nutrients have limited availability. Baxter et al. (2008) showed that when Arabidopsis plants are grown under a low Fe concentration, the shoot concentration of Co increased, which was concomitant with the increased expression of Fe transporter IRT1. Additionally, Co contribution to hormesis has been proposed (Shahid et al., 2020). Due to the limited research on Co to date, these explanations may not be on target and incomplete. Our proposition is that the application of the appropriate amount of Co may stimulate rhizosphere bacteria (either symbiotic, endophytic, or associated ones) to fix N2, increase soil N, and enhance plant growth. Concomitantly, Co enzymes may be triggered to conduct proper biochemical and physiological activities, such as carbonate dehydratase may enhance photosynthesis and Co-peroxidase may activate the enzymatic antioxidant system. As a result, healthy growing plants would take up more nutrients from the soil and improve their growth and overall stress tolerance.
Other Performance Enhancement
Cobalt has been shown to have other beneficial effects on plants. Co as a component of preservative solutions can improve the postharvest quality of floriculture crops by prolonging the vase life of cut flowers. Cut fronds of Delta maidenhair fern (Adiantum raddianum) placed in deionized water became wilted in just 3 days because of the vascular blockage at the basal end of the petiole. The wilting, however, could be delayed for up to 8 days by adding 1 mM Co as Co(NO3)2 to the water (Fujino and Reid, 1983). The delay of senescence is attributed to the antibacterial activity of Co (Van Doorn et al., 1991). Co addition to preservative solutions increased leaf diffusive resistance, reduced xylem blockage, sustained water flow and uptake, and prolonged vase life of cut flowers of Rosa hybrida “Samantha”. Reddy (1988) suggested that partial closure of stomata by Co was responsible for reducing the water loss/water uptake ratio, and thereby maintaining a higher water potential in the cut roses. Co was also reported to slow the senescence process in harvested lettuce (Tosh et al., 1979). Co3+ has been reported to form Co-complexes, which have antiviral activities (Chang et al., 2010). In addition to antibacterial and antiviral activities, Co shows inhibitory activity to 1-aminocyclopropane-1-carboxylic acid (ACC) oxidase. Ethylene is synthesized from amino acid methionine by two key enzymes, ACC synthase, and ACC oxidase. Co can block the conversion of ACC to ethylene by inhibiting ACC oxidase activity in the ethylene biosynthesis pathway (Lau and Yang, 1976; Serek et al., 2006), thus increasing the vase life of cut flowers.
Cobalt Deficiency Occurs in Plants
Cobalt deficiency does occur in plants. Its deficiency symptoms include leaf chlorosis and necrosis, growth retardation, and reduced crop yield, resembling N-deficiency in plants (Liu, 1998). Co deficient legumes have reduced plant size, smaller and pale-yellow leaves, and smaller pods compared with non-deficiency plants. Root growth is also affected by exhibiting an overall reduction of root volume and root lengths. Nodule size and numbers are less abundant than the plants without Co deficiency. Co deficiency causes reduced synthesis of methionine, thus limiting protein synthesis and contributing to the smaller-sized bacteroids (Marschner, 2011). Sweet lupin is particularly sensitive to Co deficiency (Robson et al., 1979). In field-grown lupins, Co deficiency reduced bacteroid number per gram of nodule (Chatel et al., 1978) and affected nodule development and function at different levels (Dilworth et al., 1979). Co deficiency in legumes can be assessed by analysis of Co contents in shoots. In general, deficient symptom appears when shoot Co falls in a range from 0.04 (Ozanne et al., 1963) to 0.02 mg/kg based on dry weight (Robson et al., 1979). To correct Co deficiency in leguminous crops, application of Co in a range of 1.8 to 145.6 g per hectare was reported (Havlin et al., 2013).
Cobalt deficiency also occurs in non-leguminous plants. Co deficiency causes growth retardation in rubber trees and tomato plants (Wilson and Nicholas, 1967). Symptoms of Co deficiency in corn and wheat showed leaf chlorosis and reduced growth (Wilson and Nicholas, 1967). Low leaves may become necrotic, root systems are reduced with decreased number of N2 fixing bacteria. Grasses with low contents of Co can result in Co deficiency of sheep and cattle. For countries, like South Australia, Sierra Leone, Malta, New Zealand, and Finland, where soils have low Co contents (Sillanpaa and Jansson, 1992), application of Co could improve forage grass growth and enrich tissue Co content. Thus, the feeding of ruminants with healthy grass can reduce Co deficiency (Lee, 1951; Dewey et al., 1958). Due to low Co concentrations in plants, Co deficiency in grazing animals may occur, which can be corrected by mixing Co salts with fertilizers or sand carriers to broadcast it over grazed pastures.
Cobalt Toxicity in Plants
Cobalt at high concentrations causes cytotoxicity and phytotoxicity in plants, which is similar to Cu, Ni, and Zn. Cytotoxicity is the inhibition of mitosis and damage of chromosomes, and disruption of the endoplasmic reticulum of root tip cells (Rauser, 1981; Smith and Carson, 1981; Akeel and Jahan, 2020). Phytotoxicity varies depending on plant species and the concentration of Co in plant organs. Leguminous plants generally exhibit chlorosis or pale-white color on young leaves, and tomatoes show either interveinal chlorosis or diffused chlorosis on young leaves (Akeel and Jahan, 2020).
Cobalt toxicity to plants is uncommon in natural soils, but it happens when plants grow in Co contaminated soils. Soil contamination by Co is mainly from mining and smelting activities, disposal of sewage sludge, and the use of chemical fertilizers (Hamilton, 1994). As discussed above, plants can control Co absorption, transport, and distribution. However, when Co in contaminated soils becomes highly available, Co may gain a competitive advantage over Fe, resulting in more Co being absorbed than Fe through IRT1. With increasing concentrations of Co inside cells, FPN2 may not be able to effectively sequester Co into the vacuole, resulting in more Co to transport from roots to shoots. Li et al. (2020) showed that Co concentrations in shoots of barley, oilseed rape (Brassica napus), and tomato were linearly correlated with the soil solution Co. As a result, excessive Co in shoots may initially cause oxidative stress, resulting in increased anti-oxidative enzyme activities (Tewari et al., 2002). As the stress progresses, Co may compete with Fe or Mg in the chloroplast by decreasing chlorophyll content (Lwalaba et al., 2017), which causes Fe deficiency with newly growing leaves to be yellowish in color. As reported by Sree et al. (2015), Co is able to inhibit the activity of enzymes involved in the biosynthesis of chlorophyll intermediates, like 5-aminolevulinic acid and protoporphyrin, which will reduce net photosynthetic activities. Co also adversely affects the translocation of P, S, Cu, Mn, and Zn from roots to shoots (Chatterjee and Chatterjee, 2000). All these factors, acting together, can result in phytotoxicity and significantly reduce plant growth.
Different plants show different abilities to tolerate Co. Oat (Avena sativa) plants were adversely affected when grown in a soil solution containing 0.14 mg/L Co (Anderson et al., 1973). Rice (Oryza sativa) plants would develop toxic symptoms when grown in soils with Co ranging from 25 and 50 mg/kg (Kitagishi and Yamane, 1981). The contents of Co could be used for predicting the development of toxicity (Akeel and Jahan, 2020). Toxic symptoms occurred in bush beans when tissue Co contents ranged from 43 to 142 mg/kg (Wallace et al., 1977); similarly, 6 mg/kg in barley seedlings (Davis et al., 1978), and 19 to 32 mg/kg in Sudan grass (Gough et al., 1979). In general, tissue Co contents between 30 and 40 mg/kg are considered critical levels for the potential development of Co toxicity (Macnicol and Beckett, 1985). However, due to evolutionary adaptation, Co hyperaccumulators do not develop toxic symptoms at this concentration level. Co contents in leaves of Rinorea cf. bengalensis can be 1,200 mg/kg (Paul et al., 2020), and Glochidion cf. sericeum can accumulate 1,500 mg/kg Co (Van der Ent et al., 2018). Co hyperaccumulators are not the focus of this article. The reader is referred to publications by Brooks (1977), Brooks et al. (1977, 1980), Baker (1981, 1987), Lange et al. (2017), and Yamaguchi et al. (2019) for more information.
Conclusions and Future Perspectives
Cobalt in soils ranges from 15 to 25 mg/kg, wherein plant roots can absorb Co from soils and transport absorbed Co from roots to shoots in a controlled manner. Co concentrations in shoots vary with plant species but are comparable to those of essential elements of Cu, Ni, and Zn. Co was well-documented as a constituent of cobalamin, which is required by symbiotic, endophytic, and associated bacteria in the fixation of N2. Biological N fixation contributed significantly to the production of economically important crops, including beans, soybeans, rice, corn, barley, wheat, and sugarcane. The current view of plant-microbe association as a phytomicrobiome resulted from millions of years of co-evolution. The coevolution between plants and N2 fixing bacteria should remind us of the critical role Co plays and its potential essentiality to plant growth and development. Additionally, plants must have Co enzymes or proteins that are specifically responsible for Co metabolism. Due to its similar properties to other transition elements, its biological roles in plants have been largely ignored and simply attributed to its ability to substitute for those elements.
Further research is warranted to (1) identify specific roles of Co plays in diazotrophs, with an emphasis on endophytic and associated bacteria, (2) ascertain Co-containing enzymes and proteins that are implicated in metabolisms of both lower and higher plants, (3) determine the interactions of Co with other transition metals in the regulation of enzymatic activities, (4) recognize Co as an essential micronutrient for plant growth, and (5) develop nutrient management programs by incorporating a group of particular N fixing bacteria with the appropriate amount of Co as plant-specific fertilizers for improving crop production. With the advance in omics, these tasks should be accomplished in the near future. The recognition of Co as an essential micronutrient would enrich our understanding of plant mineral nutrition and enhance crop productivity.
Author Contributions
XH, XW, and JC wrote the manuscript. JL prepared figures. All authors contributed to the acquisition and interpretation of available literature and the conception of the work, revised the manuscript, and approved this final version.
Funding
This study was supported in part by the Students Innovation and Entrepreneurship Training Program at Zhongkai University of Agriculture and Engineering with grant number 202111347007 and the General Project of the Natural Science Foundation of Fujian Province with grant number 2020J01867.
Conflict of Interest
The authors declare that the research was conducted in the absence of any commercial or financial relationships that could be construed as a potential conflict of interest.
Publisher's Note
All claims expressed in this article are solely those of the authors and do not necessarily represent those of their affiliated organizations, or those of the publisher, the editors and the reviewers. Any product that may be evaluated in this article, or claim that may be made by its manufacturer, is not guaranteed or endorsed by the publisher.
Acknowledgments
The authors would like to thank Ms. Terri A. Mellich for critical review of this manuscript.
References
Aasfar, A., Bargaz, A., Yaakoubi, K., Hilali, A., Bennis, I., Zeroual, Y., et al. (2021). Nitrogen fixing azotobacter species as potential soil biological enhancers for crop nutrition and yield Stability. Front. Microbiol. 12:628379. doi: 10.3389/fmicb.2021.628379
Abdel-Rahman, H., Salem, A., Moustafa, M. M., and El-Garhy, H. A. (2017). A novice Achromobacter sp. EMCC1936 strain acts as a plant-growth-promoting agent. Acta Physiol. Plant. 39:61. doi: 10.1007/s11738-017-2360-6
Adams, D. G., Bergman, B., Nierzwicki-Bauer, S. A., Duggan, P. S., Rai, A. N., and Schußler, A. (2013). “Cyanobacteria–bryophyte symbioses,” in The Prokaryotes, eds M. Dworkin, S. Falkow, E. Rosenberg, K. Schleifer, and E. Stackebrandt (Berlin; Heidelberg: Springer), 359–400.
Aery, N. C., and Jagetiy, B. L. (2000). Effect of cobalt treatments on dry matter production of wheat and DTPA extractable cobalt content in soils. Commun Soil Sci. Plant Anal. 31, 1275–1286. doi: 10.1080/00103620009370512
Ahmed, S., and Evans, H. J. (1959). Effect of Cobalt on growth of soybeans in the absence of supplied nitrogen. Biochem. Biophys. Res. Comm. 1, 271–275. doi: 10.1016/0006-291X(59)90036-1
Ahmed, S., and Evans, H. J. (1960). The plants grown under symbiotic conditions. Proc. Natl. Acad. Sci. U.S.A. 47, 24–36. doi: 10.1073/pnas.47.1.24
Akeel, A., and Jahan, A. (2020). “Role of cobalt in plants: its stress and alleviation,” in Contaminants in Agriculture, eds M. Naeem, A. Ansari, and S. Gill (Cham: Springer), 339–352.
Anderson, A., Meyer, D., and Mayer, F. (1973). Heavy metal toxicities: levels of nickel, cobalt and chromium in the soil and plants associated with visual symptoms and variation in growth of an oat crop. Aust. J. Agric. Res. 24, 557–571. doi: 10.1071/AR9730557
Andić, B., Dragićević, S., Stešević, D., Jančić, D., and Krivokapić, S. (2015). Comparative analysis of trace elements in the mosses – Bryum argenteum Hedw. and Hypnum cupressiforme Hedw. in Podgorica (Montenegro). J. Mater. Environ. Sci. 6, 333–342.
Andrews, M., and Andrews, M. E. (2017). Specificity in legume-rhizobia symbioses. Intl. J. Mol. Sci. 18:705. doi: 10.3390/ijms18040705
Antonyuk, L. P., Smirnova, V. E., Kamnev, A. A., Serebrennikova, O. B., Vanoni, M. A., Zanetti, G., et al. (2001). Influence of divalent cations on the catalytic properties and secondary structure of unadenylylated glutamine synthetase from Azospirillum brasilense. Biometals 14, 13–22. doi: 10.1023/A:1016640522299
Arnon, D. I., and Stout, P. R. (1939). The essentiality of certain elements in minute quantity for plants with special reference to copper. Plant Physiol. 14, 371–375. doi: 10.1104/pp.14.2.371
Aslmoshtaghi, E. (2014). Effects of daffodil flowers and cobalt chloride on vase life of cut rose. J. Chem. Health Risks 4, 1–6.
Atta-Aly, M. A. (1998). Soaking summer squash seeds in sow concentrations of cobalt solution before sowing increased plant growth, femaleness, and fruit yield via increasing plant ethylene Level. J. Plant Growth Regul. 17, 25–32 doi: 10.1007/PL00007008
Attia, S. A. A., Gad, N., and Abdel-Rahman, H.M. (2014). Effect of cobalt on growth, yield and production quality with mitotic and meiotic divisions in two onion cultivars. Curr. Sci. Int. 3, 122–131.
Aziz, E. E., Gad, N., and Nadia, M. B. (2007). Effect of cobalt and nickel on plant growth, yield and flavonoid content of Hibiscus sabdariffa L. Aust. J. Basic Appl. Sci. 1, 73–78.
Bageshwar, U. K., Srivastava, M., Pardha-Saradhi, P., Paul, S., Gothandapani, S., Jaat, R. S., et al. (2017). An environmentally friendly engineered Azotobacter strain that replaces a substantial amount of urea fertilizer while sustaining the same wheat yield. Appl. Environ. Microbiol. 83:e00590–17. doi: 10.1128/AEM.00590-17
Baker, A. (1981). Accumulators and excluders-strategies in the response of plants to heavy metals. J. Plant Nutr. 3, 643–654. doi: 10.1080/01904168109362867
Baker, A. J. M., Brooks, R. R., Pease, A. J., and Malaisse, F. (1983). Studies on Cu and cobalt tolerance in three closely related taxa within the genus Silene L. (Caryophyllaceae) from Zare. Plant Soil 73, 377–385. doi: 10.1007/BF02184314
Bakkaus, E., Gouget, B., Gallien, J. P., Khodja, H., Carrot, F., Morel, J. L., et al. (2005). Concentration and distribution of cobalt in higher plants: the use of micro-PIXE spectroscopy. Nucl. Instrum. Methods Phys. Res. Sect. B 231, 350–356. doi: 10.1016/j.nimb.2005.01.082
Barsanti, L., and Gualtieri, P. (2006). Algae: Anatomy, Biochemistry and Biotechnology. Boca Raton, FL: Taylor and Francis Group.
Bartolo, W., and Macey, M. (1989). Cobalt requirement in tissue culture of three species: Brassica oleracea L., Passifora mollissima Bailey, and Saintpaulia ioantha Wendl. J. Hort. Sci. 64, 643–647. doi: 10.1080/14620316.1989.11516003
Basu, M., and Bhadoria, P. B. S. (2008). Performance of groundnut under nitrogen fixing and phosphorous microbial inoculates with different levels of cobalt in alluvial soils of eastern India. Agron. Res. 6, 15–25.
Baxter, I. R., Vitek, O., Lahner, B., Muthukumar, B., Borghi, M., Morrissey, J., et al. (2008). The leaf ionome as a multivariable system to detect a plant's physiological status. Proc. Natl. Acad. Sci. U.S.A. 105, 12081–12086. doi: 10.1073/pnas.0804175105
Bennett, B. (2010). “EPR of cobalt-substituted zinc enzymes,” in Metals in Biology: Application of High Resolution EPR to Metalloenzymes, ed G. R. Hanson (New York, NY: Springer), 345–370. doi: 10.1007/978-1-4419-1139-1
Bertalan, M., Albano, R., de Pádua, V., Rouws, L., Rojas, C., Hemerly, A., et al. (2009). Complete genome sequence of the sugarcane nitrogen-fixing endophyte Gluconacetobacter diazotrophicus Pal5. BMC Genomics 10:450. doi: 10.1186/1471-2164-10-450
Bhosale, S., Rao, M., and Deshpande, V. (1996). Molecular and industrial aspects of glucose isomerase. Microbiol. Rev. 60, 280–300.
Boddey, R. M., and Dobereiner, J. (1988). Nitrogen fixation associated with grasses and cereals: recent results and perspectives for future research. Plant Soil 108, 53–65. doi: 10.1007/BF02370099
Böhme, H. (1998). Regulation of nitrogen fixation in heterocyst-forming cyanobacteria. Trends Plant Sci. 3. doi: 10.1016/S1360-1385(98)01290-4
Bolle-Jones, E., and Mallikarjuneswara, V. (1957). A beneficial effect of Co on the growth of the rubber plant. Nature 179, 738–739. doi: 10.1038/179738a0
Bridwell-Rabb, J., and Drennan, C. (2017). Vitamin B12 in the spotlight again. Curr. Opin. Chem. Biol. 37, 63–70. doi: 10.1016/j.cbpa.2017.01.013
Brooks, R. R. (1977). Copper and cobalt uptake by Haumaniastrum species. Plant Soil 48, 541–544. doi: 10.1007/BF02187261
Brooks, R. R. (1998). Plants that Hyperaccumulate Heavy, Metals: Their Role in Phytoremediation, Microbiology, Archaeology, Mineral Exploration and Phytomining. Oxon: CAB International.
Brooks, R. R., McCleave, J. A., and Schofield, E. K. (1977). Cobalt and nickel uptake by the Nyssaceae. Taxon 26, 197–201. doi: 10.2307/1220551
Brooks, R. R., Reeves, R. D., Morrison, R. S., and MalaJsse, F. (1980). Hyperaccumulation of copper and cobalt: a review. Bull. Soc. Roy. Bot. Belg. 113, 166–172.
Brylinski, M., and Skolnick, J. (2011). FINDSITE-metal: integrating evolutionary information and machine learning for structure-based metal binding site prediction at the proteome level. Proteins 79, 735–751. doi: 10.1002/prot.22913
Bulantseva, E. A., Glinka, E. M., Protsenko, M. A., and Sal'kova, E. G. (2001). A protein inhibitor of polygalacturonase in apple fruits treated with aminoethoxyvinylglycine and cobalt chloride. Prikl. Biokhim. Mikrobiol. 37, 100–104. doi: 10.1023/A:1002808912247
Carpenter, K. J. (2004). The Nobel Prize and the Discovery of Vitamins. NobelPrize.org. Nobel Prize Outreach AB2021. Stockholm. Available online at: https://www.nobelprize.org/prizes/themes/the-nobel-prize-and-the-discovery-of-vitamins (accessed August 28, 2021).
Carter, E. L., Flugga, N., Boer, J. L., Mulrooney, S. B., and Hausinger, R. P. (2009). Interplay of metal ions and urease. Metallomics 1, 207–221. doi: 10.1039/b903311d
Cavet, J. S., Borrelly, G. P., and Robinson, N. J. (2003). Zn, Cu and Co in cyanobacteria: selective control of metal availability. FEMS Microbiol. Rev. 27, 165–181. doi: 10.1016/S0168-6445(03)00050-0
Chang, A. C. G., Chen, T., Li, N., and Duan, J. (2019). Perspectives on endosymbiosis in coralloid roots: association of cycads and cyanobacteria. Front. Microbiol. 10:1888. doi: 10.3389/fmicb.2019.01888
Chang, E., Simmers, C., and Knight, D. (2010). Cobalt complexes as antiviral and antibacterial agents. Pharmaceuticals 3, 1711–1728. doi: 10.3390/ph3061711
Chatel, D. L., Robson, A. D., Gartrell, J. W., and Dilworth, M. J. (1978). The effect of inoculation on cobalt application on the growth of and nitrogen fixation of sweet lupins. Aust. J. Agric. Res. 29, 1191–1202. doi: 10.1071/AR9781191
Chatterjee, J., and Chatterjee, C. (2000). Phytotoxicity of cobalt, chromium and copper in caulifower. Environ. Pollut. 109, 69–74. doi: 10.1016/S0269-7491(99)00238-9
Chen, J., Zhou, J., and Goldsbrough, P. (1997). Characterization of phytochelatin synthase from tomato. Physiol. Plant 101, 165–192. doi: 10.1111/j.1399-3054.1997.tb01833.x
Cheng, Y. S., Yan, Y. B., and Liu, J. Y. (2005). Spectroscopic characterization of metal bound phytochelatin analogue (Glu-Cys)(4)-Gly. J. Inorg. Biochem. 99, 1952–1962. doi: 10.1016/j.jinorgbio.2005.06.016
Chmielowska-Bak, J., Lefèvre, I., Lutts, S., Kulik, A., and Deckert, J. (2014). Effect of cobalt chloride on soybean seedlings subjected to cadmium stress. Acta Soc. Bot. Pol. 83, 201–207. doi: 10.5586/asbp.2014.027
Choudhury, A., and Kennedy, I. (2004). Prospects and potentials for systems of biological nitrogen fixation in sustainable rice production. Biol. Fertil. Soils 39, 219–227. doi: 10.1007/s00374-003-0706-2
Choudhury, F. K., Rivero, R. M., Blumwald, E., and Mittler, R. (2017). Reactive oxygen species, abiotic stress and stress combination. Plant J. 90, 856–867. doi: 10.1111/tpj.13299
Ciotea, D., Ungureanu, E., Mustatea, G., and Popa, M. E. (2021). Incidence of lead, cadmium, chromium, nickel and cobalt in basil, rosemary and peppermint seasonings from Romanian market. Bull. Univ. Agric. Sci. Vet. Med. Cluj-Napoca. Food Sci. Technol. 78, 19–32. doi: 10.15835/buasvmcn-fst:2021.0002
Collins, R. N., Bakkaus, E., Carrière, M., Khodja, H., Proux, O., and Morel, J. L. (2010). Uptake, localization, and speciation of cobalt in Triticum aestivum L. (wheat) and Lycopersicon esculentum M. (tomato). Environ. Sci. Technol. 44, 2904–2910. doi: 10.1021/es903485h
Compant, S., Kaplan, H., Sessitsch, A., Nowak, J., Ait Barka, E., and Clément, C. (2008). Endophytic colonization of Vitis vinifera L. by Burkholderia phytofirmans strain PsJN: from the rhizosphere to inflorescence tissues. FEMS Microbiol. Ecol. 63, 84–93. doi: 10.1111/j.1574-6941.2007.00410.x
Conte, S. S., and Walker, E. L. (2011). Transporters contributing to iron trafficking in plants. Mol. Plant 4, 1–13. doi: 10.1093/mp/ssr015
Croft, M. T., Lawrence, A. D., Raux-Deery, E., Warren, M. J., and Smith, A. G. (2005). Algae acquire vitamin B12 through a symbiotic relationship with bacteria. Nature 438, 90–93. doi: 10.1038/nature04056
Cruz-Lopez, R., and Maske, H. (2016). The vitamin B1 and B12 required by the marine dinoflagellate lingulodinium polyedrum Can be provided by its associated bacterial community in culture. Front. Microbiol. 7:560. doi: 10.3389/fmicb.2016.00560
Czerpak, R., Bajguz, A., Chodkowski, K., and Popow, H. (1994). Influence of nickel and cobalt on the growth and biochemical changes of Chlorella pyrenoidosa (Chlorophyceae). Pol. Arch. Hydrobiol. 41, 161–169.
Das, H. K. (2019). Azotobacters as biofertilizer. Adv. Appl. Microbiol. 108, 1–43. doi: 10.1016/bs.aambs.2019.07.001
Davies, K. M., Jibran, R., Zhou, Y., Albert, N. W., Brummell, D. A., Jordan, B. R., et al. (2020). The evolution of flavonoid biosynthesis: a bryophyte perspective. Front. Plant Sci. 11:7. doi: 10.3389/fpls.2020.00007
Davis, R., Beckett, P., and Wollan, E. (1978). Critical levels of twenty potentially toxic elements in young spring barley. Plant Soil 49, 395–408. doi: 10.1007/BF02149747
Dewey, D. W., Lee, H. J., and Marston, H. R. (1958). Provision of cobalt to ruminants by means of heavy pellets. Nature 181, 1367–1371. doi: 10.1038/1811367a0
Dilworth, M. J., Robson, A. D., and Chatel, D. L. (1979). Cobalt and nitrogen fixation in Lupinus angustifolius L. II. Nodule formation and function. New Phytol. 83, 63–79. doi: 10.1111/j.1469-8137.1979.tb00727.x
Din, M., Nelofer, R., Salman, M., Abdullah, K. F. H, and Khan, A. (2019). Production of nitrogen fixing Azotobacter (SR-4) and phosphorus solubilizing Aspergillus niger and their evaluation on Lagenaria siceraria and Abelmoschus esculentus. Biotechnol. Rep. 22:e00323. doi: 10.1016/j.btre.2019.e00323
Dobereiner, J., Reis, V., Paula, M., and Olivares, F. (1993). “Endophytic diazotrophs in sugarcane cereals and tuber crops,” in New Horizons in Nitrogen Fixation, eds R. Palacios, J. Moor, and W. Newton (Dordrecht: Kluwer), 671–674.
Doxey, A. C., Kurtz, D. A., Lynch, M. D., Sauder, L. A., and Neufeld, J. D. (2015). Aquatic metagenomes implicate Thaumarchaeota in global cobalamin production. ISME J. 9, 461–471. doi: 10.1038/ismej.2014.142
Eitinger, T. (2013). “Cobalt transporters,” in Encyclopedia of Metalloproteins, eds R. H. Kretsinger, V. N. Uversky, and E. A. Permyakov (New York, NY: Springer), 678–682.
El-Essawy, A., El-Sayed, M., and Mohamed, Y. (1984). Production of cyanocobalamine by Azotobacter chroococcum. Zentralblatt für Mikrobiol. 139, 335–342. doi: 10.1016/S0232-4393(84)80011-6
Elledge, S., Zhou, Z., and Allen, J. (1992). Ribonucleotide reductase: regulation, regulation, regulation. Trends. Biochem. Sci 17, 119–123. doi: 10.1016/0968-0004(92)90249-9
Erdman, J. A., and Modreski, P. J. (1984). Copper and cobalt in aquatic mosses and stream sediments from the Idaho Cobalt Belt. J. Geochem. Explor. 20, 75–84. doi: 10.1016/0375-6742(84)90091-8
Evans, H. J., and Kliewer, M. (1964). Vitamin B12 compounds in relation to the requirements of cobalt for higher plants and nitrogen-fixing organisms. Annals N. Y. Acad. Sci. 112, 735–755. doi: 10.1111/j.1749-6632.1964.tb45052.x
Falcón, L., Magallón, S., and Castillo, A. (2010). Dating the cyanobacterial ancestor of the chloroplast. ISME J. 4,777–783. doi: 10.1038/ismej.2010.2
Fang, H., Kang, J., and Zhang, D. (2017). Microbial production of vitamin B12: a review and future perspectives. Microb. Cell. Fact. 16:15. doi: 10.1186/s12934-017-0631-y
Faucon, M. P., Shutcha, M. N., and Meerts, P. (2007). Revisiting copper and cobalt concentrations in supposed hyperaccumulators from SC Africa: influence of washing and metal concentrations in soil. Plant Soil 301, 29–36. doi: 10.1007/s11104-007-9405-3
Franche, C., Lindstrom, K., and Elmerich, C. (2009). Nitrogen- fixing bacteria associated with leguminous and non-leguminous plants. Plant Soil 321, 35–59. doi: 10.1007/s11104-008-9833-8
Fujino, D. W., and Reid, M.S. (1983). Factors affecting the vase life of fronds of maidenhair fern. Sci. Hort. 21, 181–188. doi: 10.1016/0304-4238(83)90164-4
Gad, N. (2006). Increasing the efficiency of nitrogen fertilization through cobalt application to pea plant. Res. J. Agri. Biol. Sci. 2, 433–442.
Gad, N. (2012a). Physiological and chemical response of groundnut (Arachis hypogaea) to Cobalt Nutrition. World Appl. Sci. J. 20, 327–335.
Gad, N. (2012b). Role and importance of cobalt nutrition on groundnut (Arachis hypogaea) production. World Appl. Sci. J. 20, 359–367. doi: 10.5829/idosi.wasj.2012.20.03.2819
Gad, N., and Hassan, N. M. K. (2013). Role of cobalt and organic fertilizers amendments on tomato production in the newly reclaimed soil. World Appl. Sci. J. 22, 1527–1533.
Gad, N., and Kandil, H. (2008). Response of sweet potato (Ipomoea batatas L.) plants to different levels of cobalt. Aust. J. Basic Appl. Sci. 2, 949–955.
Gad, N., and Kandil, H. (2009). The influence of cobalt on sugar beet (Beta vulgaris L.) production. Intl. J. Acad. Res. 1, 52–58.
Garcia, M. M., Pereira, L. C., Braccini, A. L., Angelotti, P., Suzukawa, A. K., Marteli, D. C., et al. (2017). Effects of Azospirillum brasilense on growth and yield compounds of maize grown at nitrogen limiting conditions. Revista de Ciências Agrárias 40, 353–362. doi: 10.19084/RCA16101
García-Caparrós, P., De Filippis, L., Gul, A., Hasanuzzaman, M., Ozturk, M., Altay, V., et al. (2020). Oxidative stress and antioxidant metabolism under adverse environmental conditions: a review. Bot. Rev. 40, 353–362. doi: 10.1007/s12229-020-09231-1
Garnham, G. W., Codd, G. A., and Gadd, G. M. (1992). Kinetics of uptake and intracellular location of cobalt, manganese and zinc in the estuarine green alga Chlorella salina. Appl. Microbiol. Biotechnol. 37, 270–276. doi: 10.1007/BF00178183
Giglione, C., and Meinnel, T. (2001). Organellar peptide deformylases: universality of the N-terminal methionine cleavage mechanism. Trends Plant Sci. 6, 566–572. doi: 10.1016/S1360-1385(01)02151-3
Giglione, C., Serero, A., Pierre, M., Boisson, B., and Meinnel, T. (2000). Identification of eukaryotic peptide deformylases reveals universality of N-terminal protein processing mechanisms. EMBO J. 19, 5916–5929. doi: 10.1093/emboj/19.21.5916
Gonzalez-Lopez, J., Salmeron, V., Moreno, J., and Ramos-Cormenzana, A. (1983). Amino acids and vitamins produced by Azotobacter vinelandii ATCC 12837 in chemically-defined media and dialysed soil media. Soil Biol. Biochem. 15, 711–713. doi: 10.1016/0038-0717(83)90037-8
Gonzalez-Montana, J. R., Escalera-Valente, F., Alonso, A. J., Lomillos, J. M., Robles, R., and Alonso, M. E. (2020). Relationship between vitamin B12 and cobalt metabolism in domestic ruminant: an update. Animals 10:1855. doi: 10.3390/ani10101855
Gough, L., Shacklette, H., and Case, A. (1979). Element concentrations toxic to plants, animals and man. LiSGS Bull 1466, 80–84.
Govindaraju, K. (1994). Compilation of working values and sample description for 383 geostandards. Geostand Newslett. 18, 1–158. doi: 10.1111/j.1751-908X.1994.tb00502.x
Grover, S., and Purves, W. K. (1976). Cobalt and plant development: interactions with ethylene in hypocotyl growth. Plant Physiol. 57, 886–889. doi: 10.1104/pp.57.6.886
Grube, M., Seckbach, J., and Muggia, L. (2017). Algal and Cyanobacteria Symbioses. Singapore: World Scientific.
Guo, M., and Chen, Y. (2018). Coenzyme cobalamin: biosynthesis, overproduction and its application in dehalogenation—a review. Rev. Environ. Sci. Biotechnol. 17, 259–284. doi: 10.1007/s11157-018-9461-6
Gupta, G., Panwar, J., Akhtar, M. S., and Jha, P. N. (2012). “Endophytic nitrogen-fixing bacteria as biofertilizer,” in Sustainable Agriculture Reviews, ed E. Lichtfouse (Dordrecht: Springer), 183–221.
Gupta, V., Roper, M., and Roget, D. (2006). Potential for non-symbiotic N2-fixation in different agroecological zones of southern Australia. Aust. J. Soil Res. 44, 343–354. doi: 10.1071/SR05122
Hamilton, E. I. (1994). The geobiochemistry of cobalt. Sci. Total Environ. 150, 7–39. doi: 10.1016/0048-9697(94)90126-0
Han, H. Y., Xu, W. A., Lu, Z. R., Zou, F., and Li, S. (2008). Activation and inactivation of horseradish peroxidase by cobalt ions. J. Biomol. Struct. Dyn. 26, 83–92. doi: 10.1080/07391102.2008.10507226
Hansen, B., Thorling, L., Schullehner, J., Termansen, M., and Dalgaard, T. (2017). Groundwater nitrate response to sustainable nitrogen management. Sci. Rep. 7:8566. doi: 10.1038/s41598-017-07147-2
Harrop, T. C., and Mascharak, P. K. (2013). “Cobalt-containing enzymes,” in Encyclopedia of Metalloproteins, eds R. H. Kretsinger, V. N. Uversky, and E. A. Permyakov (New York, NY: Springer), 684–690.
Hartmann, A., Stoffels, M., Eckert, B., Kirchhof, G., and Schloter, M. (2000). “Analysis of the presence and diversity of diazotrophic endophytes,” in Prokaryotic Nitrogen Fixation: A Model System for the Analysis of a Biological Process, ed E. W. Triplett (Wymondham: Horizon Scientific Press), 727–736.
Hasanuzzaman, M., Bhuyan, M. H. M. B., Zulfiqar, F., Raza, A., Mohsin, S. M., Mahmud, J. A., et al. (2020). Reactive oxygen species and antioxidant defense in plants under abiotic stress: revisiting the crucial role of a universal defense regulator. Antioxidants 9:681. doi: 10.3390/antiox9080681
Havlin, J. L., Tisdale, S. L., Nelson, W. L., and Beaton, J. D. (2013). Soil Fertility and Fertilizers. Upper Saddle River, NJ: Prentice Hall, Inc.
Hawco, N. J., McIlvin, M. M., Bundy, R. M., Tagliabue, A., Goepfert, T. J., Moran, D. M., et al. (2020). Minimal cobalt metabolism in the marine cyanobacterium Prochlorococcus. Proc. Natl. Acad. Sci. 117, 15740–15747. doi: 10.1073/pnas.2001393117
Heal, K. R., Wei, Q., Ribalet, F., Bertagnolli, A. D., Coyote-Maestas, W., Hmelo, L. R., et al. (2017). Two distinct pools of B12 analogs reveal community interdependencies in the ocean. Proc. Natl. Acad. Sci. U.S.A. 114, 364–369. doi: 10.1073/pnas.1608462114
Helmy, L. M., and Gad, N. (2002). Effect of cobalt fertilization on the yield, quality the essential oil composition of parsley leaves. Arab. Univ. J. Agric. Sci. Ain. Shams Univ Cairo 10, 779–802.
Herridge, D. F., Peoples, M. B., and Boddey, R. M. (2008). Global inputs of biological nitrogen fixation in agricultural systems. Plant Soil 311, 1–18. doi: 10.1007/s11104-008-9668-3
Hewitt, E. J., and Bond, G. (1966). The cobalt requirement of non-legume root nodule plants. J. Exp. Bot. 17, 480–491. doi: 10.1093/jxb/17.3.480
Holm-Hansen, O., Gerloff, G. C., and Skoog, F. (1954). Cobalt as an essential element for blue-green algae. Physiol. Plant 7, 665–675. doi: 10.1111/j.1399-3054.1954.tb07727.x
Homer, F. A. (1991). Comparative studies of nickel, cobalt, and copper uptake by some nickel hyperaccumulators of the genus Alyssum. Plant Soil 138, 195–205. doi: 10.1007/BF00012246
Hurek, T., and Reinhold-Hurek, B. (2003). Azoarcus sp. strain BH72 as a model for nitrogen-fixing grass endophytes. J. Biotechnol. 106, 169–178. doi: 10.1016/j.jbiotec.2003.07.010
Huss-Danell, K. (1997). Tansley Review No. 93. Actinorhizal symbioses and their N2 fixation. New Phytol. 136, 375–405. doi: 10.1046/j.1469-8137.1997.00755.x
Huwait, A. E., Kumosani, A. T., Moselhy, S. S., Mosaoa, M. R., and Yaghmoor, S. S. (2015). Relationship between soil cobalt and vitamin B12 levels in the liver of livestock in Saudi Arabia: role of competing elements in soils. Afr. Health Sci. 15, 993–998. doi: 10.4314/ahs.v15i3.38
Hyodo, H., and Fukasawa, R. (1985). Ethylene production in kiwi fruit (Actinidia chinensis cultivar. Hayward). J. Jap. Soc. Hortic. Sci. 54, 209–215. doi: 10.2503/jjshs.54.209
Iswaran, V., and Rao Sundara, W. V. B. (1964). role of cobalt in Nitrogen fixation by Azotobacter chroococcum. Nature 203:549. doi: 10.1038/203549a0
Jaleel, C. A., Jayakumar, K., Zhao, C.-X., and Iqbal, M. (2009). Low concentration of cobalt increases growth, biochemical constituents, mineral status and yield in Zea mays. J. Sci. Res. 1, 128–137. doi: 10.3329/jsr.v1i1.1226
Jalilian, N., Najafpour, G., and Khajouei, M. (2019). Enhanced vitamin B12 production using Chlorella vulgaris. IJE Transac. Basics 32, 1–9. doi: 10.5829/ije.2019.32.01a.01
Jamali, B., and Rahemi, M. (2011). Carnation flowers senescence as influenced by nickel, cobalt and silicon. J. Biol. Environ. Sci. 5, 147–152.
James, E. K., Olivares, F. L., de Oliveira, A. L., dos Reis, F. B. Jr, da Silva, L. G., and Reis, V. M. (2001). Further observations on the interaction between sugar cane and Gluconacetobacter diazotrophicus under laboratory and greenhouse conditions. J. Exp. Bot. 52, 747–760. doi: 10.1093/jexbot/52.357.747
Jayakumar, K., Abdul Jaleel, C., Azooz, M. M., Vijayarengan, P., Gomathinayagam, M., and Panneerselvam, R. (2009). Effect of different concentrations of cobalt on morphological parameters and yield components of soybean. Glob. J. Mol. Sci. 4, 10–14.
Jayakumar, K., Rajesh, M., Baskaran, L., and Vijayarengan, P. (2013). Changes in nutritional metabolism of tomato plants exposed to increasing concentration of cobalt chloride. Acta Physiol. Plant. 4, 62–69.
Jensen, E. L., Maberly, S. C., and Gontero, B. (2020). Insights on the functions and ecophysiological relevance of the diverse carbonic anhydrases in microalgae. Int. J. Mol. Sci. 21:2922. doi: 10.3390/ijms21082922
Johansson, C., and Bergman, B. (1994). Reconstitution of the symbiosis of Gunnera manicata Linden: cyanobacterial specificity. New Phytol. 126, 643–652. doi: 10.1111/j.1469-8137.1994.tb02960.x
Kabata-Pendias, A., and Mukherjee, A. B. (2007). Trace Elements From Soils to Human. Berlin; Heidelberg: Springer-Verlag.
Kamnev, A., Tarantilis, P., Antonyuk, L., Bespalova, L., Polissiou, M., Colina, M., et al. (2001). Fourier transform Raman spectroscopic characterisation of cells of the plant-associated soil bacterium Azospirillum brasilense Sp7. J. Mol. Struct. 563, 199–207. doi: 10.1016/S0022-2860(00)00877-2
Kannan, T., and Ponmurugan, P. (2010). Response of paddy (Oryza sativa L.) varieties to Azospirillum brasilense inoculation. J. Phytol. 2, 8–13.
Kazemi, M. (2012). Effect of cobalt, silicon, acetylsalicylic acid and sucrose as novel agents to improve vase-life of Argyranthemum flowers. Trends Appl. Sci. Res. 7, 579–583. doi: 10.3923/tasr.2012.579.583
Kazemi, M., and Ameri, A. (2012). Effect of Ni, CO, SA and sucrose on extending the vase-life of lily cut flower. Iran. J. Energy Environ. 3, 162–166. doi: 10.5829/idosi.ijee.2012.03.02.0258
Ke, J., Wang, B., and Yoshikuni, Y. (2021). Microbiome engineering: synthetic biology of plant-associated microbiomes in sustainable agriculture. Trends Biotechnol. 39, 244–261. doi: 10.1016/j.tibtech.2020.07.008
Kellogg, M. M., Mcllvin, M. R., Vedamati, J., Twining, B. S., Moffett, J. W., Marchetti, A., et al. (2020). Efficient zinc/cobalt inter-replacement in northeast Pacific diatoms and relationships to high surface dissolved Co: Zn ratio. Limnol. Oceanogr. 65, 2557–2582. doi: 10.1002/lno.11471
Keshavarz, H., and Moghadam, R. S. G. (2017). Seed priming with cobalamin (vitamin B12) provides significant protection against salinity stress in the common bean. Rhizosphere 3, 143–149. doi: 10.1016/j.rhisph.2017.04.010
Khan, A. R., Park, G. S., Asaf, S., Hong, S. J., Jung, B. K., and Shin, J. H. (2017). Complete genome analysis of Serratia marcescens RSC-14: a plant growth-promoting bacterium that alleviates cadmium stress in host plants. PloS ONE 12:e0171534. doi: 10.1371/journal.pone.0171534
Khrustalev, V., Khrustaleva, T., Poboinev, V., Karchevskaya, C., Shablovskaya, E., and Terechova, T. (2019). Cobalt(ii) cation binding by proteins. Metallomics 11, 1743–1752. doi: 10.1039/C9MT00205G
Kirchhof, G., Reis, V., Baldani, J., Eckert, B., Döbereiner, J., and Hartmann, A. (1997). Occurrence, physiological and molecular analysis of endophytic diazotrophic bacteria in gramineous energy plants. Plant Soil 194, 45–55. doi: 10.1023/A:1004217904546
Kitagishi, K., and Yamane, I. (1981). Heavy Metal Pollution in Soils of Japan. Tokyo: Japan Science Society Press.
Kliewer, M., and Evans, H. (1963a). Cobamide coenzyme contents of soybean nodules and nitrogen fixing bacteria in relation to physiological conditions. Plant Physiol. 38, 99–104. doi: 10.1104/pp.38.1.99
Kliewer, M., and Evans, H. J. (1963b). Identification of cobamide coenzyme in nodules of symbionts and isolation of the B12 coenzyme from Rhizobium meliloti. Plant Physiol. 38, 55–59. doi: 10.1104/pp.38.1.55
Kobayashi, M., and Shimizu, S. (1999). Cobalt proteins. Eur. J. Biochem. 261, 1–9. doi: 10.1046/j.1432-1327.1999.00186.x
Kolberg, M., Strand, K. R., Graff, P., and Andersson, K. K. (2004). Structure, function, and mechanism of ribonucleotide reductases. Biochim. Biophys. Acta 1699, 1–34. doi: 10.1016/S1570-9639(04)00054-8
Koleli, N., Demir, A., Kantar, C., Atag, G. A., Kusvuran, K., and Binzet, R. (2015). “Heavy metal accumulation in serpentine flora of Mersin-Findikpinari (Turkey) – Role of ethylenediamine tetraacetic Acid in facilitating extraction of nickel,” in Soil Remediation and Plants (New York, NY, USA, Academic Press). doi: 10.1016/b978-0-12-799937-1.00022-x
Korshunova, Y. O., Eide, D., Clark, W. G., Guerinot, M. L., and Pakrasi, H. B. (1999). The IRT1 protein from Arabidopsis thaliana is a metal transporter with a broad substrate range. Plant Mol. Biol. 40, 37–44. doi: 10.1023/A:1026438615520
Krause, A., Ramakumar, A., Bartels, D., Battistoni, F., Bekel, T., Boch, J., et al. (2006). Complete genome of the mutualistic, N 2-fixing grass endophyte Azoarcus sp. strain BH72. Nat. Biotechnol. 24, 1384–1390. doi: 10.1038/nbt1243
Kubota, Y., Shoji, S., and Motohara, K. (1977). Purification and properties of prolidase for germinating soybeans. Yakugaku Zasshi 97, 111–115. doi: 10.1248/yakushi1947.97.1_111
Lange, B., van der Ent, A., Baker, A. J., Echevarria, G., Mahy, G., Malaisse, F., et al. (2017). Copper and cobalt accumulation in plants: a critical assessment of the current state of knowledge. New Phytol. 213, 537–551. doi: 10.1111/nph.14175
Lau, O. L., and Yang, S. F. (1976). Inhibition of ethylene production by cobaltous ion. Plant Physiol. 58, 114–117. doi: 10.1104/pp.58.1.114
Lawrence, A. D., Nemoto-Smith, E., Deery, E., Baker, J. A., Schroeder, S., Brown, D. G., et al. (2018). Construction of fluorescent analogs to follow the uptake and distribution of cobalamin (vitamin B12) in bacteria, worms, and plants. Cell Chem. Biol. 25, 941–951. e946. doi: 10.1016/j.chembiol.2018.04.012
Lee, H. J. (1951). Cobalt and copper deficiencies affecting sheep in South Australia. J. Agri. Sci. Austr. 54, 475–532.
Li, H., Liu, Y., Qin, H., Lin, X., Tang, D., Wu, Z., et al. (2020). A rice chloroplast-localized ABC transporter ARG1 modulates cobalt and nickel homeostasis and contributes to photosynthetic capacity. New Phytol. 228, 163–178. doi: 10.1111/nph.16708
Liu, J. (1998). Cobalt: physiological effects and uptake mechanisms in plants [Ph.D. thesis]. The University of Adelaide, Adelaide, SA, Australia.
Lwalaba, J. L. W., Zvogbo, G., Mulembo, M., Mundende, M., and Zhang, G. (2017). The effect of cobalt stress on growth and physiological traits and its association with cobalt accumulation in barley genotypes differing in cobalt tolerance. J. Plant. Nutr. 40, 2192–2199. doi: 10.1080/01904167.2017.1346676
Lyu, S., Wei, X., Chen, J., Wang, C., Wang, X., and Pan, D. (2017). Titanium as a beneficial element for crop production. Front. Plant. Sci. 8:597. doi: 10.3389/fpls.2017.00597
Macnicol, R., and Beckett, P. (1985). Critical tissue concentrations of potentially toxic elements. Plant Soil 85, 107–129. doi: 10.1007/BF02197805
Mahey, S., Kumar, R., Sharma, M., Kumar, V., and Bhardwaj, R. (2020). A critical review on toxicity of cobalt and its bioremediation strategies. SN Appl. Sci. 2:1279. doi: 10.1007/s42452-020-3020-9
Malik, M., Chaney, R. L., Brewer, E. P., Li, Y. M., and Angle, J. S. (2000). Phytoextraction of Soil Cobalt Using Hyperaccumulator Plants. Int. J. Phytorem. 2, 319–329. doi: 10.1080/15226510008500041
Mandujano-Piña, M., Colinas-León, M. T., Castillo-González, A. M., Alía-Tejacal, I., and Valdéz-Aguilar, L. A. (2012). Cobalt as senescence retardant in postharvest of oriental hybrid Lilium. Revista Chapingo. Serie Hort. 18, 239–252. doi: 10.5154/r.rchsh.2010.09.034
Maret, M., and Vallee, B. (1993). Cobalt as probe and label of proteins. Methods Enzymol. 226, 52–71. doi: 10.1016/0076-6879(93)26005-T
Marschner, P. (2011). Marschner's Mineral Nutrition of Higher Plants. Amsterdam: Elsevier/Academic Press.
Marsh, E. N. (1999). Coenzyme B12 (cobalamin)-dependent enzymes. Essays Biochem. 34, 139–154. doi: 10.1042/bse0340139
Marsh, E. N. G., and Drennan, C. L. (2001). Adenosyl cobalamin dependent isomerases: new insights into structure and mechanism. Curr. Opin. Chem. Biol. 5, 499–505. doi: 10.1016/S1367-5931(00)00238-6
McLeod, K. W., and Ciravolo, T. G. (2007). Cobalt uptake by Nyssa aquatica, N. sylvatica var. biflora, and Taxodium distichum seedlings. Wetlands 27, 40–43. doi: 10.1672/0277-5212(2007)27[40:CUBNAN]2.0.CO;2
Mehrafarin, A., Rezazadeh, S., Naghdi Badi, H., Gh, N., Z, E., and Qaderi, A. (2021). A review on biology, cultivation and biotechnology of fenugreek (Trigonella foenum-graecum L.) as a valuable medicinal plant and multipurpose. J. Med. Pl. 10, 6–24.
Mohandas, S. (1985). Effect of presowing seed treatment with molybdenum and cobalt on growth, nitrogen and yield in bean (Phaseolus vulgaris L.). Plant Soil 86, 283–285. doi: 10.1007/BF02182905
Morel, M., Crouzet, J., Gravot, A., Auroy, P., Leonhardt, N., Vavasseur, A., et al. (2009). AtHMA3, a P1B-ATPase allowing Cd/Zn/Co/Pb vacuolar storage in Arabidopsis. Plant Physiol. 149, 894–904. doi: 10.1104/pp.108.130294
Morrison, R. S. (1979). Copper and cobalt uptake by metallophytes from Zaïre. Plant Soil 53, 535–539. doi: 10.1007/BF02140724
Morrissey, J., Baxter, I., Lee, J., Li, L., Lahner, B., Grotz, N., et al. (2009). The ferroportin metal efflux proteins function in iron and cobalt homeostasis in Arabidopsis. Plant Cell 21, 3326–3338. doi: 10.1105/tpc.109.069401
Mozafar, A. (1994). Enrichment of some B-vitamins in plants with application of organic fertilizers. Plant Soil 167, 305–331. doi: 10.1007/BF00007957
Murr, D. P., Venkatarayappa, T., and Tsujita, M. J. (1979). Counteraction of bent-neck of cut roses with cobalt nitrate.” Can. J. Plant Sci. 59, 1169–1171. doi: 10.4141/cjps79-184
Muthukumarasamy, R., Cleenwerck, I., Revathi, G., Vadivelu, M., Janssens, D., Hoste, B., et al. (2005). Natural association of Gluconacetobacter diazotrophicus and diazotrophic Acetobacter peroxydans with wetland rice. Syst. Appl. Microbiol. 28, 277–286. doi: 10.1016/j.syapm.2005.01.006
Nagpal, N. (2004). Water Quality Guidelines for Cobalt. Victoria, BC: Ministry of Water, Land and Air Protection, Water Protection Section, Water, Air and Climate Change Branch.
Nakos, M., Pepelanova, I., Beutel, S., Krings, U., Berger, R. G., and Scheper, T. (2017). Isolation and analysis of vitamin B12 from plant samples. Food Chem. 216, 301–308. doi: 10.1016/j.foodchem.2016.08.037
Nicholas, D., Kobayashi, M., and Wilson, P. (1962). Cobalt requirement for inorganic nitrogen metabolism in microorganisms. Proc. Nat. Acad. Sci. 48, 1537–1542. doi: 10.1073/pnas.48.9.1537
Nohwar, N., Khandare, R. V., and Desai, N. S. (2020). Media optimization studies and production of adenosylcobalamin (Vitamin B12) by environment friendly organism Rhizobium spp. J. Appl. Biol. Biotech. 8, 38–47.
Odaka, M., and Kobayashi, M. (2013). “Cobalt proteins, overview,” in Encyclopedia of Metalloproteins, eds R. H. Kretsinger, V. N. Uversky, and E. A. Permyakov (New York, NY: Springer), 670–678.
Orji, J., Ngumah, C., Asor, H., and Anuonyemere, A. (2018). Effects of cobalt and manganese on biomass and nitrogen fixation yields of a free-living nitrogen fixer-Azotobacter chroococcum. Eur. J. Biol. Res. 8, 7–13.
Osman, D., Cooke, A., Young, T., Deery, E., Robinson, N., and Warren, M. (2021). The requirement for cobalt in vitamin B12: a paradigm for protein metalation. BBA-Mol. Cell. Res. 1868:118896. doi: 10.1016/j.bbamcr.2020.118896
Ozanne, P. G., Greenwood, E. A. N., and Shaw, T. C. (1963). The cobalt requirement of Subterranean clover in the field. Aust. J. Agric. Res. 14, 39–50. doi: 10.1071/AR9630039
Page, V., and Feller, U. (2005). Selective transport of zinc, manganese, nickel, cobalt and cadmium in the root system and transfer to the leaves in young wheat plants. Ann. bot. 96, 425–434. doi: 10.1093/aob/mci189
Pais, I. (1992). Criteria of essentiality, beneficiality and toxicity of chemical elements. Acta Aliment. 21, 145–152.
Paizs, C., Diemer, T., and Rétey, J. (2008). The putative coenzyme B12- dependent methylmalonyl-CoA mutase from potatoes is a phosphatase. Bioorg. Chem. 36, 261–264. doi: 10.1016/j.bioorg.2008.06.002
Palacios, O. A., Bashan, Y., and de-Bashan, L. E. (2014). Proven and potential involvement of vitamins in interactions of plants with plant growth-promoting bacteria—an overview. Biol. Fertil. Soils. 50, 415–432. doi: 10.1007/s00374-013-0894-3
Paul, A. L. D., Nkrumah, P. N., Echevarria, G., Erskine, P. D., Chaney, R. L., Spiers, K. M., et al. (2020). Cobalt hyperaccumulation in Rinorea cf. Bengalensis (Violaceae) from sabah: Accumulation potential and tissue and cellular-level distribution of cobalt. Plant Soil 455, 289–303. doi: 10.1007/s11104-020-04629-7
Pedrosa, F. O., Monteiro, R. A., Wassem, R., Cruz, L. M., Ayub, R. A., Colauto, N. B., et al. (2011). Genome of Herbaspirillum seropedicae strain SmR1, a specialized diazotrophic endophyte of tropical grasses. PLoS Genet. 7:e1002064. doi: 10.1371/journal.pgen.1002064
Permyakov, E. A. (2021). Metal binding proteins. Encyclopedia 1, 261–292. doi: 10.3390/encyclopedia1010024
Pham, V. T. K., Rediers, H., Ghequire, M. G. K., Nguyen, H. H., De Mot, R., Vanderleyden, J., et al. (2017). The plant growth-promoting effect of the nitrogen-fixing endophyte Pseudomonas stutzeri A15. Arch. Microbiol. 199, 513–517. doi: 10.1007/s00203-016-1332-3
Pilon-Smits, E. A., Quinn, C. F., Tapken, W., Malagoli, M., and Schiavon, M. (2009). Physiological functions of beneficial elements. Curr. Opin. Plant. Biol. 12, 267–274. doi: 10.1016/j.pbi.2009.04.009
Poston, J. M. (1977). Leucine 2, 3-aminomutase: a cobalamin-dependent enzyme present in bean seedlings. Science 195, 301–302. doi: 10.1126/science.195.4275.301
Poston, J. M. (1978). Coenzyme B12-dependent enzymes in potatoes: leucine 2, 3-aminomutase and methylmalonyl-coa mutase. Phytochemistry 17, 401–402. doi: 10.1016/S0031-9422(00)89324-3
Puri, A., Padda, K. P., and Chanway, C. P. (2018). “Nitrogen-fixation by endophytic bacteria in agricultural crops: recent advances,” in Nitrogen in Agriculture-Updates, eds A. Khan, S. Fahad (Rijeka: In Tech Publisher), 73–94. doi: 10.5772/intechopen.71988
Raj, A. S. (1987). Cobalt nutrition of pigeonpea and peanut in relation to growth and yield. J. Plant Nutr. 10, 2137–2145. doi: 10.1080/01904168709363764
Rajakaruna, N., and Bohm, B. A. (2002). Serpentine and its vegetation: a preliminary study from Sri Lanka. J. Appl. Bot. Angewand. Bot. 76, 20–28.
Rana, K. L., Divjot, K., Tanvir, K., Rubee, D., Nath, Y. A., Neelam, Y., et al. (2020). Endophytic microbes: biodiversity, plant growth-promoting mechanisms and potential applications for agricultural sustainability. Antonie van Leeuwenhoek 113, 1075–1107. doi: 10.1007/s10482-020-01429-y
Rauser, W. (1981). Entry of sucrose into minor veins of bean seedlings exposed to phytotoxic burdens of Co, Ni or Zn. J. Plant. Nutr. 3, 319–328. doi: 10.1080/01904168109362840
Reddy, T. V. (1988). Mode of action of cobalt extending the vase life of cut roses. Sci. Hort. 36, 303–313. doi: 10.1016/0304-4238(88)90065-9
Reed, S. C., Cleveland, C. C., and Townsend, A. R. (2011). Functional ecology of free-living nitrogen fixation: a contemporary perspective. Annu. Rev. Ecol. Evol. Syst. 42, 489–512. doi: 10.1146/annurev-ecolsys-102710-145034
Reimann, C., Koller, F., Frengstad, B., Kashulina, G., Niskavaara, H., and Englmaier, P. (2001). Comparison of the element compositin in several plant species and their substrate from a 1555000 km2 area in northern Europe. Sci. Total. Environ. 278, 87–112. doi: 10.1016/S0048-9697(00)00890-1
Reisenauer, H. M. (1960). Cobalt in nitrogen fixation by a legume. Nature 186, 375–376. doi: 10.1038/186375a0
Ritika, B., and Dey, U. (2014). Biofertilizer, a way towards organic agriculture: a review. Afr. J. Microbiol. Res. 8, 2332–2343. doi: 10.5897/AJMR2013.6374
Roberts, S., and Gunn, G. (2014). “Cobalt,” in Critical Metals Handbook, 1st Edn, ed G. Gunn (Nottingham: John Wiley and Sons), 122–147.
Robson, A. D., Dilworth, M. J., and Chatel, D. L. (1979). Cobalt and nitrogen fixation on Lupinus angusfifohus L. I. Growth nitrogen concentrations and cobalt distribution. New Phytol. 83, 53–62. doi: 10.1111/j.1469-8137.1979.tb00726.x
Rod, N. K., Gudadhe, N. N., Karmakar, N., Mehta, P. V., and Narwade, A. V. (2019). Cobalt chloride enhances crop duration, increases production, and productivity of chickpea. J. Plant. Nutr. 42, 40–57. doi: 10.1080/01904167.2018.1544258
Rodionov, D. A., Vitreschak, A. G., Mironov, A. A., and Gelfand, M. S. (2003). Comparative genomics of the vitamin B12 metabolism and regulation in prokaryotes. J. Bio. Chem. 278, 41148–41159. doi: 10.1074/jbc.M305837200
Rosenblueth, M., Ormeno-Orrillo, E., Lopez-Lopez, A., Rogel, M. A., Reyes-Hernandez, B. J., Martinez-Romero, J. C., et al. (2018). Nitrogen fixation in cereals. Front. Microbiol. 9:1794. doi: 10.3389/fmicb.2018.01794
Rothballer, M., Eckert, B., Schmid, M., Fekete, A., Schloter, M., Lehner, A., et al. (2008). Endophytic root colonization of gramineous plants by Herbaspirillum frisingense. FEMS Microbiol. Ecol. 66, 85–95. doi: 10.1111/j.1574-6941.2008.00582.x
Roughley, R. J., Gault, R. R., Gemell, L. G., Andrews, J. A., Brockwell, J., Dunn, B. W., et al. (1995). Autecology of Bradyrhizobium japonicum in soybean-rice rotations. Plant Soil 176, 7–14. doi: 10.1007/BF00017670
Sachdev, S., Ansari, S. A., Ansari, M. I., Fujita, M., and Hasanuzzaman, M. (2021). Abiotic stress and reactive oxygen species: generation, signaling and defense mechanisms. Antioxidants 10:277. doi: 10.3390/antiox10020277
Santoyo, G., Moreno-Hagelsieb, G., del Carmen Orozco-Mosqueda, M., and Glick, B. R. (2016). Plant growth-promoting bacterial endophytes. Microbiol. Res. 183, 92–99. doi: 10.1016/j.micres.2015.11.008
Saric, T., and Saciragic, B. (1969). Effect of oat seed treatment with microelements. Plant Soil 31, 185–187. doi: 10.1007/BF01373038
Sato, K., Kudo, K., and Muramatsu, K. (2004). Incorporation of a high level of vitamin B12 into a vegetable, kaiware daikon (Japanese radish sprout), by the absorption from its seeds. Biochim. Biophys. Acta. 1672, 135–137. doi: 10.1016/j.bbagen.2004.03.011
Serek, M., Woltering, E. J. E. C, Sisler, S. F., and Sriskandarajah, S. (2006). Controlling ethylene responses in flowers at the receptor level. Biotechnol. Adv. 24, 368–381. doi: 10.1016/j.biotechadv.2006.01.007
Setubal, J. C., Dos Santos, P., Goldman, B. S., Ertesvåg, H., Espin, G., Rubio, L. M., et al. (2009). Genome sequence of Azotobacter vinelandii, an obligate aerobe specialized to support diverse anaerobic metabolic processes. J. Bacteriol. 191, 4534–4545. doi: 10.1128/JB.00504-09
Shahid, M., Niazi, N. K., Rinklebe, J., Bundschuh, J., Dumat, C., and Pinelli, E. (2020). Trace elements-induced phytohormesis: a critical review and mechanistic interpretation. Crit. Rev. Environ. Sci. Technol. 50, 1984–1932. doi: 10.1080/10643389.2019.1689061
Sillanpaa, M., and Jansson, H. (1992). Status of Cadmium, Lead, Cobalt and Selenium in Soils and Plants of Thirty Countries. Rome: FAO
Singh, D. K., Singh, A. K., Singh, M., Bordoloi, L. J., and Srivastava, O. P. (2012). Production potential and nutrient uptake efficiency of Pea (Pisum sativum L) as influenced by different fertility levels and micronutrients. J. Indian Soc. Soil Sci. 60, 1–6.
Singh, Z., Lakhvir, S., Arora, C. L., Dhillon, B. S., Singh, Z., and Singh, L. (1994). Effect of cobalt, cadmium, and nickel as inhibitors of ethylene biosynthesis on floral malformation, yield and fruit quality of mango. J. Plant. Nutr. 17, 1659–1670. doi: 10.1080/01904169409364838
Smith, I. C., and Carson, B. L. (1981). Trace Metals in the Environment. Ann Arbor, MI: Ann Arbor Science Publishers.
Soumare, A., Diedhiou, A. G., Thuita, M., Hafidi, M., Ouhdouch, Y., Gopalakrishnan, S., et al. (2020). Exploiting biological nitrogen fixation: a route towards a sustainable agriculture. Plants 9:1011. doi: 10.3390/plants9081011
Sree, K. S., Keresztes, A., Mueller-Roeber, B., Brandt, R., Eberius, M., Fischer, W., et al. (2015). Phytotoxicity of cobalt ions on the duckweed Lemna minor- morphology, ion uptake, and starch accumulation. Chemosphere 131, 149–156. doi: 10.1016/j.chemosphere.2015.03.008
Steenhoudt, O., and Vanderleyden, J. (2000). Azospirillum, a free-living nitrogen-fixing bacterium closely associated with grasses: genetic, biochemical and ecological aspects. FEMS Microbiol. Rev. 24, 487–506. doi: 10.1111/j.1574-6976.2000.tb00552.x
Tewari, R., Kumar, P., Sharma, P., and Bisht, S. (2002). Modulation of oxidative stress responsive enzymes by excess. Plant Sci. 162, 381–388. doi: 10.1016/S0168-9452(01)00578-7
Tittle, F. L. (1987). Auxin-stimulated ethylene production in fern gametophytes and sporophytes. Physiol. Plant. 70, 499–502. doi: 10.1111/j.1399-3054.1987.tb02849.x
Tjong, E., Dimri, M., and Mohiuddin, S. S. (2020). Biochemistry, Tetrahydrofolate. Treasure Island, FL: StartPearls.
Tosh, S., Choudhuri, M. A., and Chatterjee, S. K. (1979). Retardation of lettuce (Lactuca sativa L.) leaf senescence by cobalt ions. Indian. J. Exp. Bio. 17, 1134–1136.
Trejo-Téllez, L. I., Gómez-Merino, F. C., Gómez-Pérez, V., and Castro-García, F. D. A. (2014). Cobalt in postharvest of gladiolus (Gladiolus grandiflorus Hort.). Rev. Mexicana Ciencias Agrícolas 28, 1575–1587.
Underwood, E. J., and Filmer, J. F. (1935). The determination of the biological potent element (cobalt) in limonite. Aust. Vet. J. 11, 84–92.
Van der Ent, A., Erskine, P., and Sumail, S. (2015). Ecology of nickel hyperaccumulator plants from ultramafic soils in Sabah (Malaysia). Chemoecology 25, 243–259. doi: 10.1007/s00049-015-0192-7
Van der Ent, A., Mak, R., de Jonge, M. D., and Harris, H. H. (2018). Simultaneous hyperaccumulation of nickel and cobalt in the tree Glochidion cf. sericeum (Phyllanthaceae): elemental distribution and chemical speciation. Sci. Rep. 8:9683. doi: 10.1038/s41598-018-26891-7
Van der Ent, A., and Reeves, R. D. (2015). Foliar metal accumulation in plants from copper-rich ultramafic outcrops: case studies from Malaysia and Brazil. Plant Soil 389, 401–418. doi: 10.1007/s11104-015-2385-9
Van Doorn, W. G., Zagory, D., and Reid, M. S. (1991). Role of ethylene and bacteria in vascular blockage of cut fronds from the fern Adiantum raddianum. Sci. Hort. 46, 161–169. doi: 10.1016/0304-4238(91)90102-5
Van Tran, L., and Teherani, D. K. (1989). Determination of trace elements in biological material by neutron activation analysis. J. Radio. Nucl. Chem. Let. 135, 443–448. doi: 10.1007/BF02164772
Velmourougane, K., Prasanna, R., Chawla, G., Nain, L., Kumar, A., and Saxena, A. K. (2019). Trichoderma–Azotobacter biofilm inoculation improves soil nutrient availability and plant growth in wheat and cotton. J. Basic Microbiol. 59, 632–644. doi: 10.1002/jobm.201900009
Vijayarengan, P., Jaleel, C. A., Zhao, C. X., Jayakumar, K., and Azooz, M. M. (2009). Biochemical variation in ground nut under Co application. Applications. Glob. J. Mol. Sci. 4, 19–22.
Wall, L. G. (2000). The actinorhizal symbiosis. J. Plant Growth Regul. 19, 167–182. doi: 10.1007/s003440000027
Wallace, A., Alexander, G. V., and Chaudhry, F. M. (1977). Phytotoxicity of cobalt, vanadium, silver and chromium. Commun. Soil Sci. Plant Anal. 8, 751–756. doi: 10.1080/00103627709366769
Wallace, A. E. M, Romney, D. C., Adriano, J. K. G., and Alexander, V. (1982). Sources of variation in mineral composition of selected plants inhabiting a floodplain at the Savannah River Site. Soil Sci. 134, 36–39. doi: 10.1097/00010694-198207000-00006
Wang, J. B., Tang, Y. L., Xu, J., Xu, G. C., Zou, Y., and Chen, H. (2015). Accumulation and distribution of cobalt in broad bean and its effects on the photosynthesis and antioxidant enzyme activities. Acta Bot. Boreali-Occidentalia Sinica 35, 963–970.
Wang, W., Zhai, Y., Cao, L., Tan, H., and Zhang, R. (2016). Endophytic bacterial and fungal microbiota in sprouts, roots and stems of rice (Oryza sativa L.). Microbiol. Res. 188, 1–8. doi: 10.1016/j.micres.2016.04.009
Wani, S. A., Chand, S., Wani, M. A., Ramzan, M., and Hakeem, K. R. (2016). “Azotobacter chroococcum–a potential biofertilizer in agriculture: an overview,” in Soil Science: Agricultural and Environmental Prospective, eds K. Hakeem, J. Akhtar, and M. Sabir (Cham: Springer), 333–348.
Watanabe, F., Abe, K., Takenaka, S., Tamura, Y., Maruyama, I., and Nakano, Y. (1997). Occurrence of cobalamin coenzymes in the photosynthetic green alga, Chlorella vulgaris. Biosci. Biotechnol. Biochem. 61, 896–897. doi: 10.1271/bbb.61.896
Watanabe, Y., Iizuka, T., and Shimada, N. (1994). Induction of cucumber leaf urease by cobalt. Soil Sci. Plant Nutr. 40, 545–548. doi: 10.1080/00380768.1994.10413333
Wei, X., Lyu, S., Yu, Y., Wang, Z., Liu, H., Pan, D., et al. (2017). Phylloremediation of air pollutants: exploiting the potential of plant leaves and leaf-associated microbes. Front. Plant Sci. 8:1318. doi: 10.3389/fpls.2017.01318
Wheeler, R. M., and Salisbury, F. B. (1981). Gravitropism in higher plant shoots: I. A Role for ethylene. Plant Physiol. 67, 686–690. doi: 10.1104/pp.67.4.686
Wilson, S. B., and Nicholas, D. J. D. (1967). A Cobalt reguirement for non-nodulated legumes and for wheat. Phytochemistry 6, 1057–1066. doi: 10.1016/S0031-9422(00)86062-8
Yamada, K. (2013). Cobalt: its role in health and disease. Met Ions Life Sci.13, 295–320. doi: 10.1007/978-94-007-7500-8_9
Yamaguchi, T., Tsukada, C., Takahama, K., Hirotomo, T., Tomioka, R., and Takenaka, C. (2019). Localization and speciation of cobalt and nickel in the leaves of the cobalt-hyperaccumulating tree Clethra barbinervis. Trees 33, 521–532. doi: 10.1007/s00468-018-1797-6
Yanni, Y., and El-Fattah, F. K. (1999). Towards integrated biofertilization management with free living and associative dinitrogen fixers for enhancing rice performance in the Nile delta. Symbiosis 27, 319–331.
Yao, S., Lyu, S., An, Y., Lu, J., Gjermansen, C., and Schramm, A. (2018). Microalgae-bacteria symbiosis in microalgal growth and biofuel production: a review. J. Appl. Microbiol. 126, 359–368. doi: 10.1111/jam.14095
Yee, D., and Morel, F. M. (1996). In vivo substitution of zinc by cobalt in carbonic anhydrase of a marine diatom. Limnol. Oceanogr. 41, 573–577. doi: 10.4319/lo.1996.41.3.0573
Yoo, S., Cho, S., Sugimoto, H., Li, J., Kusumi, K., Koh, H., et al. (2009). Rice virescent3 and stripe1 encoding the large and small subunits of ribonucleotide reductase are required for chloroplast biogenesis during early leaf development. Plant Physiol. 150, 388–401. doi: 10.1104/pp.109.136648
Keywords: cobalamin, cobalt, endophytes, essential nutrients, micronutrients, symbiosis, vitamin B12, transporter
Citation: Hu X, Wei X, Ling J and Chen J (2021) Cobalt: An Essential Micronutrient for Plant Growth? Front. Plant Sci. 12:768523. doi: 10.3389/fpls.2021.768523
Received: 31 August 2021; Accepted: 29 September 2021;
Published: 16 November 2021.
Edited by:
Antonios Chrysargyris, Cyprus University of Technology, CyprusReviewed by:
Timothy O. Jobe, University of Cologne, GermanyGuilan Duan, Research Center for Eco-Environmental Sciences (CAS), China
Copyright © 2021 Hu, Wei, Ling and Chen. This is an open-access article distributed under the terms of the Creative Commons Attribution License (CC BY). The use, distribution or reproduction in other forums is permitted, provided the original author(s) and the copyright owner(s) are credited and that the original publication in this journal is cited, in accordance with accepted academic practice. No use, distribution or reproduction is permitted which does not comply with these terms.
*Correspondence: Jianjun Chen, ampjaGVuJiN4MDAwNDA7dWZsLmVkdQ==; Xiangying Wei, eGlhbmd5aW5nd2VpJiN4MDAwNDA7bWp1LmVkdS5jbg==