- 1Laboratorio de Fisiología Vegetal, Departamento de Botánica, Facultad de Ciencias Naturales y Oceanográficas, Universidad de Concepción, Concepción, Chile
- 2Research Group on Plant Biology Under Mediterranean Conditions, Departament de Biologia, Institute of Agro-Environmental Research and Water Economy, Universitat de les Illes Balears, Illes Balears, Spain
- 3Departamento de Ingeniería Topográfica y Cartografía, Escuela Técnica Superior de Ingenieros en Topografía, Geodesia y Cartografía, Universidad Politécnica de Madrid, Madrid, Spain
- 4Department of Biology, Wilfrid Laurier University, Waterloo, ON, Canada
- 5Max-Planck-Institut für Molekulare Pflanzenphysiologie, Potsdam, Germany
- 6Centre for Research in Agricultural Genomics (CRAG), CSIC-IRTA-UAB-UB, Barcelona, Spain
- 7Institut de Recerca i Tecnología Agroalimentàries (IRTA), Edifici CRAG, Barcelona, Spain
The alternative oxidase pathway (AOP) is associated with excess energy dissipation in leaves of terrestrial plants. To address whether this association is less important in palustrine plants, we compared the role of AOP in balancing energy and carbon metabolism in palustrine and terrestrial environments by identifying metabolic relationships between primary carbon metabolites and AOP in each habitat. We measured oxygen isotope discrimination during respiration, gas exchange, and metabolite profiles in aerial leaves of ten fern and angiosperm species belonging to five families organized as pairs of palustrine and terrestrial species. We performed a partial least square model combined with variable importance for projection to reveal relationships between the electron partitioning to the AOP (τa) and metabolite levels. Terrestrial plants showed higher values of net photosynthesis (AN) and τa, together with stronger metabolic relationships between τa and sugars, important for water conservation. Palustrine plants showed relationships between τa and metabolites related to the shikimate pathway and the GABA shunt, to be important for heterophylly. Excess energy dissipation via AOX is less crucial in palustrine environments than on land. The basis of this difference resides in the contrasting photosynthetic performance observed in each environment, thus reinforcing the importance of AOP for photosynthesis.
Introduction
Current life on Earth would not be possible without the evolution of biochemical processes that maintained energy entry in plants during land colonization (Delwiche and Cooper, 2015; De Vries et al., 2016; De Vries and Archibald, 2018; Gago et al., 2019). The earliest terrestrial plant ancestor, a charophycean alga, emerged from water approximately 500 million years ago (Bhattacharya and Medlin, 1998; Yoon et al., 2004; Harholt et al., 2016; Morris et al., 2018; Reski, 2018), undergoing physiological, structural, and biochemical changes to cope with the transition from an aqueous to a gaseous medium (Kenrick and Crane, 1997; Pires and Dolan, 2012; Vermeij, 2016). Among physiological and structural modifications from the first colonizing vascular land plants, specialized sexual organs, different kinds of leaves and roots, stomata, vascular and structural tissues allowed increases in plant size and water use efficiency (Kenrick et al., 2012; Assouline and Or, 2013; Proctor, 2014; Arteaga-Vazquez, 2016; Brodribb et al., 2020). At the biochemical level, changes in metabolic pathways favored the synthesis of phenolic compounds, lignin, plant hormones, isoprenes, heat shock proteins or superoxide dismutase to favor photosynthetic performance and plant growth under a highly stressful terrestrial environment (Lowry et al., 1980; Kenrick and Crane, 1997; Waters, 2003; Weng and Chapple, 2010; Bowman et al., 2017). As plant gas exchange involves water loss, survival in the dry atmosphere required that plants overcame desiccation forcing the first colonizing terrestrial plants to be close to sources of water, until new adaptations allowed their spread into the dry atmosphere of terrestrial habitats (Brodribb et al., 2020). In the meantime, the antioxidant systems were enhanced in land plants allowing them to survive several deleterious types of environmental stresses worldwide that induce oxidative stress and damage to the photosynthetic apparatus (Asada, 2006; Thomas et al., 2008; Gill and Tuteja, 2010; Zandalinas et al., 2021).
Currently, several metabolic pathways are identified as major energy-dissipating systems conferring metabolic adaptation in response to a large entry of sunlight energy in leaves (Niyogi, 1999; Raghavendra and Padmasree, 2003; Scheibe, 2004; Noguchi and Yoshida, 2008). Among these pathways, mitochondrial metabolism stands out for its interaction with photosynthesis, photorespiration and nitrogen assimilation (Raghavendra and Padmasree, 2003; Florez-Sarasa et al., 2016; O’Leary et al., 2020). In the mitochondrial electron transport system, oxygen consumption takes place simultaneously through the activities of cytochrome oxidase (COX) and alternative oxidase (AOX). Several studies in genetically engineered AOX-modified terrestrial model plants have suggested a role of AOX activity in optimizing photosynthesis under stress (Dahal and Vanlerberghe, 2018; Del-Saz et al., 2018a) by favoring the dissipation of excess energy and thus balancing cellular redox metabolism (Raghavendra and Padmasree, 2003; Del-Saz et al., 2018a; Vanlerberghe et al., 2020). In fact, there is in vivo evidence of a fine tuning of respiratory metabolism via AOX activity in leaves of crops and model terrestrial plant species exposed to abiotic stress as a mechanism to dissipate excess energy (Florez-Sarasa et al., 2012, 2016; Del-Saz et al., 2018a, b). Indeed, across the divergence of the plant kingdom, AOX is widespread and conserved, and it is of vital importance for plants (McDonald and Vanlerberghe, 2006; Del-Saz et al., 2018a; Selinski et al., 2018). Notably, AOX is hypothesized to have originated among anaerobic bacteria in an anoxic atmosphere, being important for redox homeostasis during the transition to an oxygen-rich atmosphere 2.45 billion years ago during the Great Oxidation Event (Moore et al., 2002; Finnegan et al., 2003; Catling and Claire, 2005).
Several clades that appeared during the diversification of terrestrial plants, which include bryophytes, ferns and angiosperms, returned to aquatic environments, necessitating physiological, structural and biochemical modifications (Robe and Griffiths, 2000; Rascio, 2002; Maberly, 2014). This transition from terrestrial to aquatic habitats occurred gradually with dynamic environmental changes that provided habitats in the palustrine wetland system and emergent heterophyllous amphibious plants, which are characterized by submerged and aerial leaves, and are precursors of the fully submerged habit (Maberly and Spence, 1989; Maberly, 2014). The fully submerged habit led many aquatic leaves to display metabolic adaptations to enhance carbon gain (Bowes and Salvucci, 1989; Keeley and Santamaría, 1992; Maberly and Madsen, 2002; Huang et al., 2020) and the aeration status to allow oxidative phosphorylation (Gibbs and Greenway, 2003). It is unknown whether the transition from land to the amphibious condition involved respiratory and metabolic adjustments when oxygen was not a limiting factor. Such adjustments could have happened due to the contrasting redox conditions that characterize both environments. Terrestrial plants are less often shaded by canopy trees and more often exposed to drought events (Valladares and Niinemets, 2007; Schlesinger and Bernhardt, 2020), resulting in vegetation adapted to both different sunlight energy input and soil water conditions. Indeed, variation in vegetation type is more affected by climate in terrestrial habitats than in palustrine habitats (Schlesinger and Bernhardt, 2020), which may support our idea of higher potential risks for redox balance in terrestrial habitats. With this in mind, comparisons of respiratory metabolism in terrestrial vascular plants and their close amphibian relatives could provide clues to different metabolic routes important for the leaf biochemistry in each ecosystem under aerobic conditions. These comparisons could be performed in leaves of amphibious plants because part of their foliage photosynthesizes and respires in the same gaseous medium as leaves of terrestrial plants. In this sense, the combination of “omics” technologies together with measurements of photosynthesis and respiration is optimal for further understanding of the metabolic regulation of plant physiological processes under different environmental conditions (Florez-Sarasa et al., 2012, 2016, 2019; Del-Saz et al., 2016; Flexas and Gago, 2018; Clemente-Moreno et al., 2019).
No previous study has evaluated the in vivo respiratory activities in ferns and palustrine angiosperms. In the present study, we compared ten species of ferns and angiosperms organized as pairs of palustrine and terrestrial species (from the same family). The in vivo respiratory activities, photosynthesis, and metabolite profiling of aerial leaves were determined using the oxygen isotope discrimination technique, leaf gas exchange and gas chromatography coupled to mass spectrometry (GC-MS), respectively. Further, to outline the climatic space occupied by these species, we overlapped values of mean annual temperature (MAT) and annual precipitation with Whittaker’s biomes classification (Whittaker, 1970; Wright et al., 2004). The main objective was to assess respiratory differences between terrestrial and palustrine plant species. In addition, relationships between metabolic routes and the AOX pathway were identified given their importance for leaf biochemistry in terrestrial and palustrine environments. We hypothesize that in terrestrial plants, these relationships could be important for the regulation of water conservation and redox state; whilst in palustrine plants, these relationships could be important for non-stress roles related to the adaptation to intermediate habitats between land and water (e.g., heterophylly).
Materials and Methods
Plant Material and Experimental Design
We selected five families of vascular plants, which consisted of one terrestrial species and its palustrine counterpart: (1) Acanthus mollis L. and Hygrophila stricta (Vahl) L. in Acanthaceae (angiosperm); (2) Arum italicum Mill. and Anubias heterophylla Engl. in Araceae (angiosperm); (3) Trachelium caeruleum L. and Lobelia cardinalis L. in Campanulaceae (angiosperm); (4) Polypodium cambricum L. and Leptochilus pteropus (Blume) Fraser-Jenk, in Polypodiaceae (fern); and (5) Pteris vittata L. and Ceratopteris thalictroides L. (Brongn) in Pteridaceae (fern) (Table 1). In the middle of autumn, terrestrial plant species were collected in the field with their underlying substrate (soil) at various coordinates in Mallorca (Spain; Table 1), and placed in plastic bags to be immediately transported to the University of Balearic Islands (Mallorca) where they were transplanted into plastic pots, using a sterile soil–peat mixture (3: 1 v/v). Then, the pots were maintained in a growth chamber under controlled conditions of 25°C, moderate light intensity of 350 μmol m–2 s–1 of photosynthetic photon flux density (PPFD), relative humidity above 40%, 12 h photoperiod, and watered to full soil capacity every 3–4 days. At the same time, commercial amphibious plants were distributed inside the same growth chamber as the terrestrial plants in different 34 × 45 cm water-tanks containing 20 ± 5 cm water-level, rooted in gravel/substrate for aquarium plants, and maintained under a moderate irradiance of 100 μmol m–2 s–1, according to the low light demand required for growing aquarium species as described in previous studies (Mommer et al., 2005; Koga et al., 2020). Four to six plants per terrestrial and palustrine species were maintained under different availability of light energy and water in each habitat. By doing this, we generated contrasting redox environments according to their different predominance in biomes with contrasting canopy openness and water availability as outlined in next subsection. All plants developed aerial leaves under growth chamber conditions until the beginning of experiments in the middle of winter. The upper-most fully expanded aerial leaves of all species were used for gas exchange, in vivo respiration, and metabolic profiling analyses.
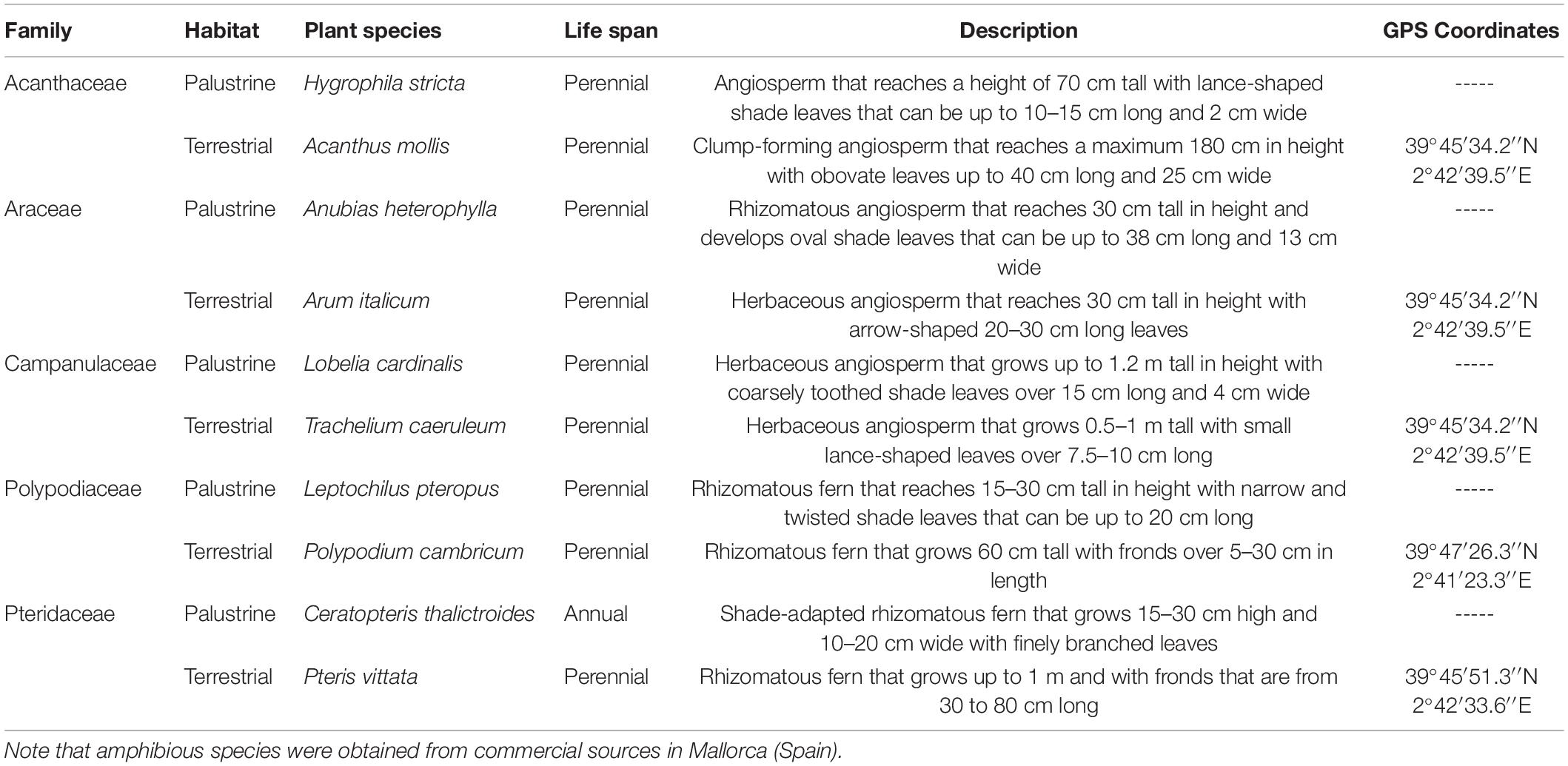
Table 1. Classification, collection, and life histories of the different plant species used in this study.
Species Spatial Distribution
In order to assess the abundance of both terrestrial and palustrine plant species in locations and biomes with different environmental conditions, we studied the spatial distribution of these species considering data of MAT and mean annual precipitation (MAP) from the years 1980 to 2010. Different numbers of records among species were obtained from GBIF (Global Biodiversity Information Facility1): A. italicum (32875), P. cambricum (17980), L. cardinalis (5375), P. vittata (3906), A. mollis (2628), T. caeruleum (2559), C. thalictroides (2037), L. pteropus (196), A. heterophylla (55), and H. stricta (7). For greater accuracy, we increased the number of records of palustrine plants in Araceae and Acanthaceae, by substituting Higrophylla stricta (7) for Higrophylla ringens (1264) and Anubias heterophylla (55) for Anubias spp. Schott. (617) because of their similar distribution records (Supplementary Figure 1). Then, a random selection of records equalized the number of samples in each family and habitat; 2000 in Campanulaceae; 1500 in Pteridaceae; 1000 in Acanthaceae; 600 in Araceae, and 150 in Polypodiaceae. Finally, the spatial distribution of records randomly selected was studied with QGIS, a GIS software that combines species occurrences from GBIF with climate layers from WorldClim2. QGIS rasterized species occurrences and extracted MAT and MAP data across all grid cells of the species occurrence region, at a spatial resolution of 30 arc-seconds (∼1 km). Then, species classification into biomes was performed from a Whittaker diagram of MAT and MAP (Wright et al., 2004).
Leaf Gas Exchange Measurements
Leaf gas exchange with Chla fluorescence measurements were recorded every day from 10 am to 2 pm during the last 2 weeks of the experiment with an open infrared gas-exchange analyzer system (Li-6400; Li-Cor Inc., Lincoln, NE, United States) equipped with a leaf chamber fluorometer (Li-6400-40, Li-Cor Inc.) using aerial leaves of terrestrial and amphibious plants under light-saturating photosynthetic photon flux density (PPFD) of 1000 and 400 μmol m–2 s–1, respectively (to avoid photodamage as a consequence of a high PPFD), with 10% blue light, a vapor pressure deficit (VPD) of 1.35 ± 0.32 kPa, a CO2 concentration (Ca) of 400 μmol CO2 mol–1, and 25°C air temperature. Net photosynthesis (AN) and stomatal conductance (gs) were determined after a steady state was reached (after c. 20 min). Once the gas exchange stabilized, five readings were taken in four to six plants per species, and averaged to be considered as the mean of the measured plant. Intrinsic WUEi was calculated as the ratio between AN and gs. After a minimum 30 min under dark conditions, leaf dark respiration (Rdark) was measured in three to five plants per species with at least five readings per plant, and estimations of leaf carbon balance were obtained from the ratio of Rdark to AN.
The quantum efficiency of the photosystem II (PSII)-driven electron transport was determined using the equation ΦPSII = (Fm′ - Fs)/Fm′, where Fs is the steady-state fluorescence in the light (PPFD = 1000 and 400 μmol quanta m–2 s–1 for terrestrial and palustrine plants, respectively) and Fm′ is the maximum fluorescence obtained with a light-saturating pulse (8000 μmol quanta m–2 s–1). The electron transport rate (ETR) was calculated as ETR = ΦPSII × PPFD × αβ, where α is the leaf absorptance, assumed to be 0.84, and β is the distribution of absorbed energy between the two photosystems, assumed to be 0.5 (Gallé and Flexas, 2010). At least five readings in two to four plants per species were taken and averaged to be considered as ETR values of the measured plant. The average ETR value for each species was used for estimations of the ratio of ETR to AN.
Respiration and Oxygen-Isotope Fractionation Measurements
For respiratory measurements, the aerial leaves of terrestrial and palustrine plants were harvested and cut into pieces after 30 min in darkness to be placed in a 3 ml stainless-steel closed cuvette maintained at a constant temperature of 25°C. Air samples were sequentially removed from the cuvette and fed into the mass spectrometer (Delta XPlus; Thermo LCC, Bremen, Germany). Changes in the 18O/16O ratios and O2 concentration were obtained to calculate the oxygen-isotope fractionation and the electron partitioning to the AOP (τa), allowing calculations of the in vivo activities of AOP and cytochrome oxidase pathway (COP) as described in Del-Saz et al. (2017a). Both end point fractionation values of the AOP (Δa) and the capacity of the alternative pathway (Valt) were determined in leaves of terrestrial and palustrine plants treated with a solution of 10 mM potassium cyanide (KCN) for 30 min. For land plants, Δa values (n = 3) of 29.9 ± 0.2‰, 30.0 ± 0.2‰, 30.2 ± 0.5‰, 30.6 ± 0.2‰ and 30.3 ± 0.4‰ were obtained for P. cambricum, P. vittata, A. italicum, A. mollis, and T. caeruleum, respectively. For palustrine plants, Δa values of 32.5 ± 0.3‰, 30.8 ± 0.3‰, 31.2 ± 0.8‰, 31.4 ± 0.1‰, and 29.6 ± 0.2‰ were obtained for A. heterophylla, C. thalictroides, H. stricta, L. cardinalis, and L. pteropus, respectively. On the other hand, an assumed value of 20.0‰ for the end point fractionation values of the COP (Δc) was used for the electron partitioning calculations as this has been shown to be fairly constant in most of the leaves and species examined (Ribas-Carbó et al., 2005). Total mitochondrial ATP production (ATPtotal) together with ATP production via COP (ATPcop) and AOP (ATPaop) were modeled from the activities of the COP and AOP of each measurement, assuming that electron flow through the AOP drives the synthesis of 11 ATP for each 6 O2 consumed whilst 29 ATP are formed for each 6 O2 consumed via COP (Del-Saz et al., 2017b). Values presented are the mean of six to eight measurements performed in four to six plants per species that were performed from 9 am to 6 pm on the same days as gas exchange measurements were performed during the last 2 weeks of the experiment. In addition, the engagement of AOP (ρ) was calculated as a percentage of the ratio of the in vivo activity of AOP (valt) to Valt.
Metabolite Profiling
Terrestrial leaves of palustrine and terrestrial plants were simultaneously sampled after 30 min in darkness on the last day of the experimental period, immediately frozen in liquid nitrogen, and stored at –80°C until further analysis. Metabolite extractions, derivatization and gas chromatography time of flight-mass spectrometry (GC-TOF-MS) analyses were carried out as previously described (Lisec et al., 2006). The GC-TOF-MS system was composed of a CTC CombiPAL autosampler, an Agilent 6890N gas chromatograph, and a LECO Pegasus III time-of-flight mass spectrometer running in EI + mode. Metabolites were identified by comparison with database entries of standards (Kopka et al., 2005; Schauer et al., 2005). The data of each terrestrial species were normalized to the mean of its respective palustrine counterpart (i.e., the value of all metabolites for each palustrine species was set to 1). The data represent averages of three to six measurements corresponding to material harvested from three to six individual plants per species.
Statistical Analysis
Data of AN, WUEi, total respiration (Vt), in vivo activity of COP (vcyt), ATPcop, and ATPtotal, were log-transformed to meet homoscedasticity. A two-way analysis of variance (p < 0.05) was performed with habitat level (terrestrial, palustrine) and plant family (Acanthaceae, Araceae, Campanulaceae, Polypodiaceae, and Pteridaceae) as fixed factors (Table 2), and Tukey’s post hoc test (p < 0.05) was used to determine differences in each respiratory and photosynthetic parameter between species (Figures 2, 3, Tables 3, 4, and Supplementary Tables 2, 3). Student’s t-tests were used for statistical analyses in Table 5 in order to compare data from terrestrial species with data from the respective palustrine counterpart in each family. To generate individual fold change data from the physiological parameters, we normalized each measurement of the terrestrial counterpart to the mean of the respective palustrine species, as for the GC-MS metabolite analyses, and Pearson coefficients were obtained with JMP®, Version 12.1.0 (SAS Institute Inc., Cary, NC, United States, 1989–2007; Table 6). Associations between the respiratory parameters and the metabolite profile were explored by applying the Partial Least Square (PLS) sparse regression as defined previously (Saccenti et al., 2014). Missing data in the metabolome dataset were imputed by employing a random forest imputation method before PLS analysis (Gromski et al., 2014). The “pls” package in R software was used to develop the PLS regression analysis. Also, this package includes a function to implement the variable importance for the projection (VIP) for single-response orthogonal score plsr models (Wehrens and Mevik, 2007).
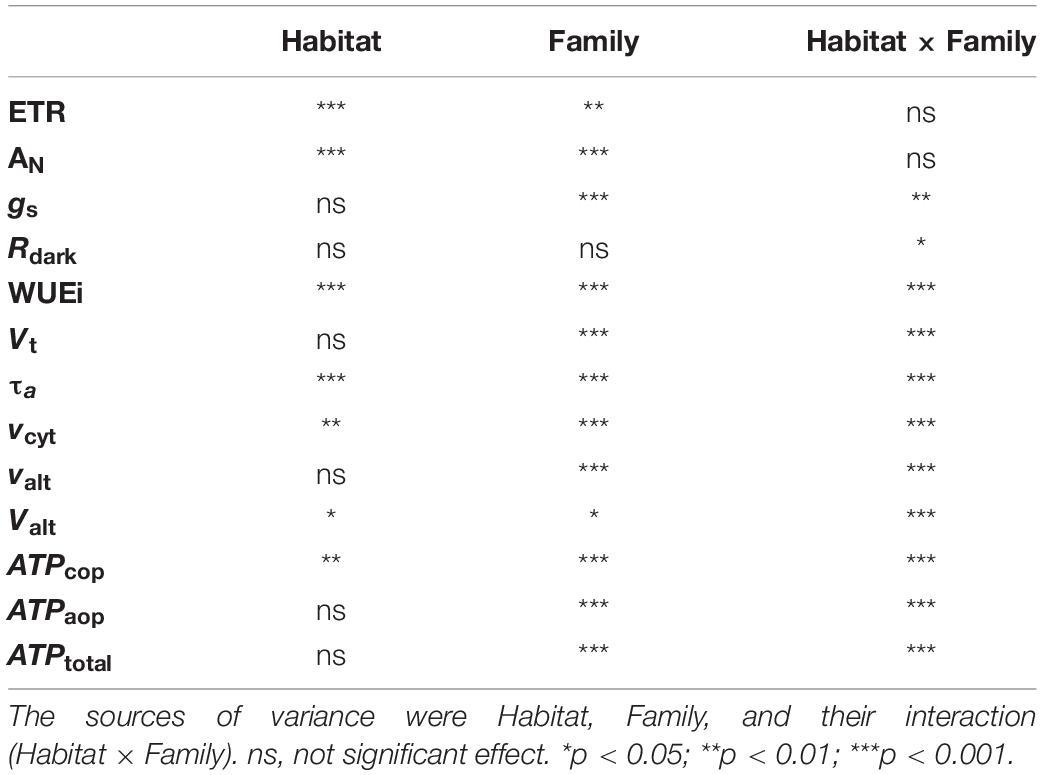
Table 2. Significance of sources of variation after two-way analysis of variance analyses for each parameter.
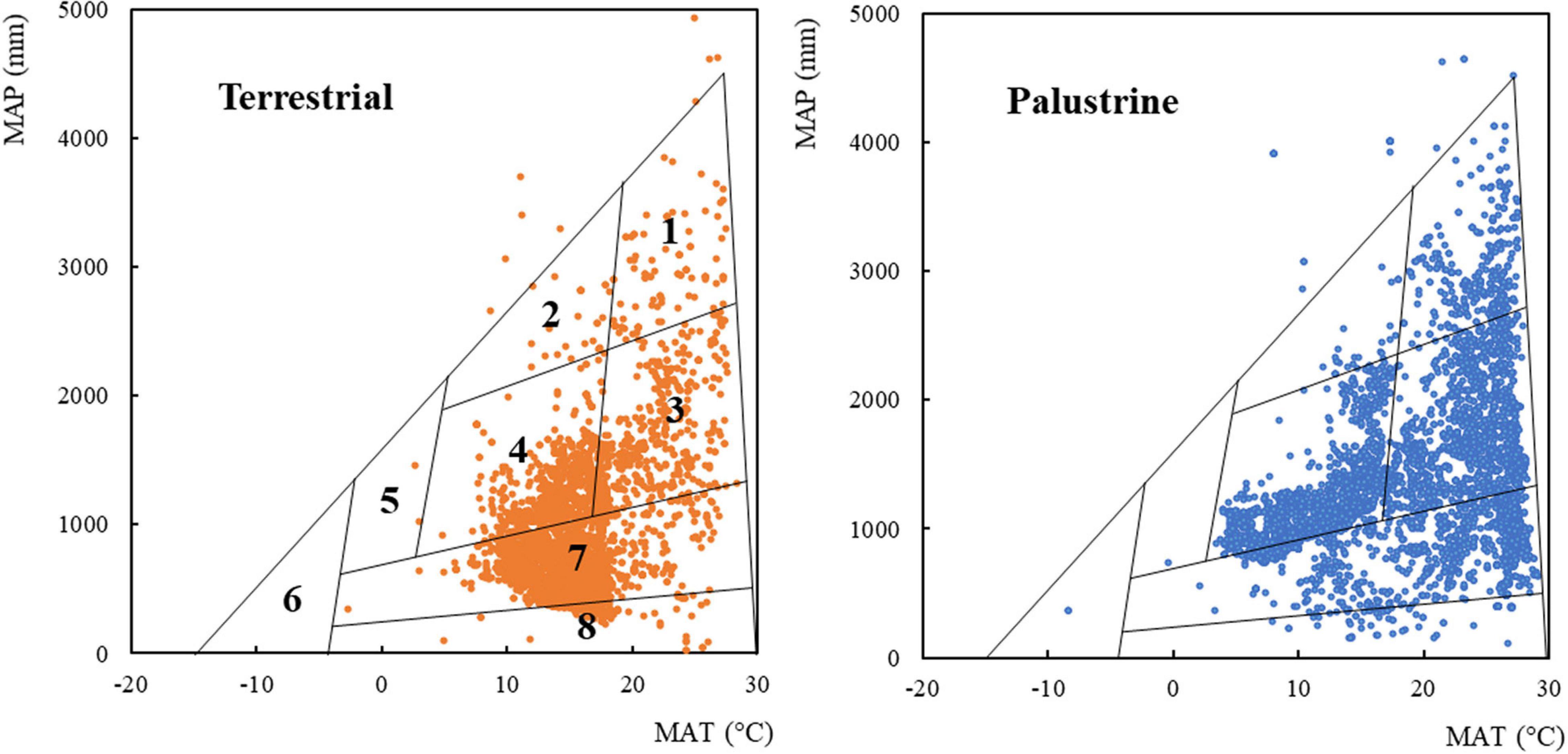
Figure 1. The boundaries of global biome type in relation to the climate factors mean annual temperature (MAT) and mean annual precipitation (MAP; Whittaker, 1970; Wright et al., 2004). For each habitat (terrestrial and palustrine), 5250 plant records (randomly selected and equalized, see section “Materials and Methods”) are overlaid on the climate envelopes of Whittaker’s biomes. Terrestrial and palustrine records are represented as brown and blue dots, respectively. (1) Tropical rainforest; (2) temperate rainforest; (3) tropical seasonal forest; (4) temperate forest; (5) boreal forest; (6) tundra; (7) woodland, shrubland, and grassland; (8) desert. Biome boundaries are only approximate. Specific abundances in each type of biome can be found in Supplementary Table 1.
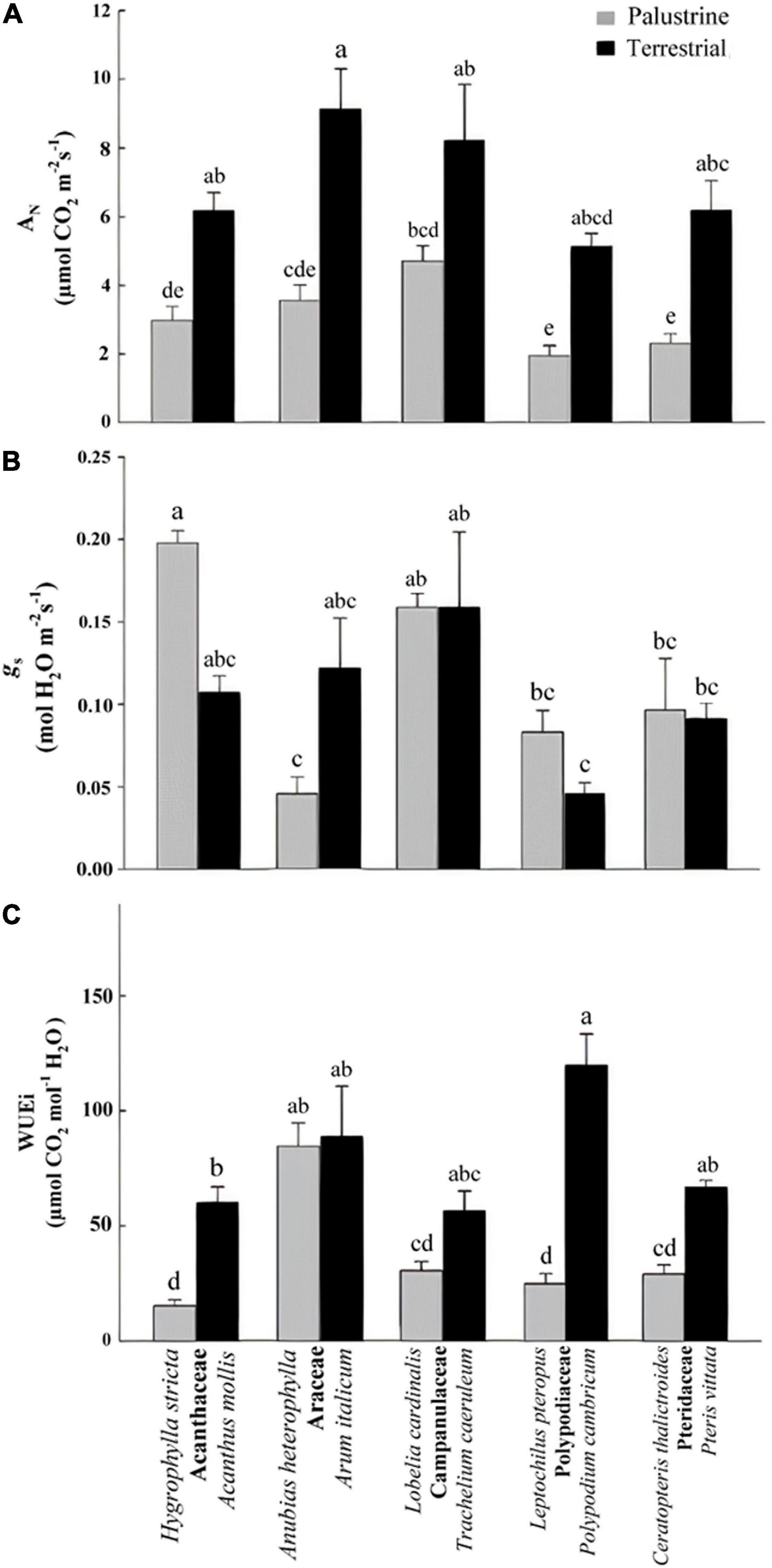
Figure 2. (A) Net photosynthesis (AN), (B) stomatal conductance (gs), and (C) intrinsic water-use efficiency (WUEi) in all palustrine and terrestrial species tested in this study. In (C), values were calculated from mean values of AN and gs. Four to six plants were used to characterize each species. Different letters indicate significant differences with a p-value < 0.05 determined by a post hoc Tukey–Kramer’s test.
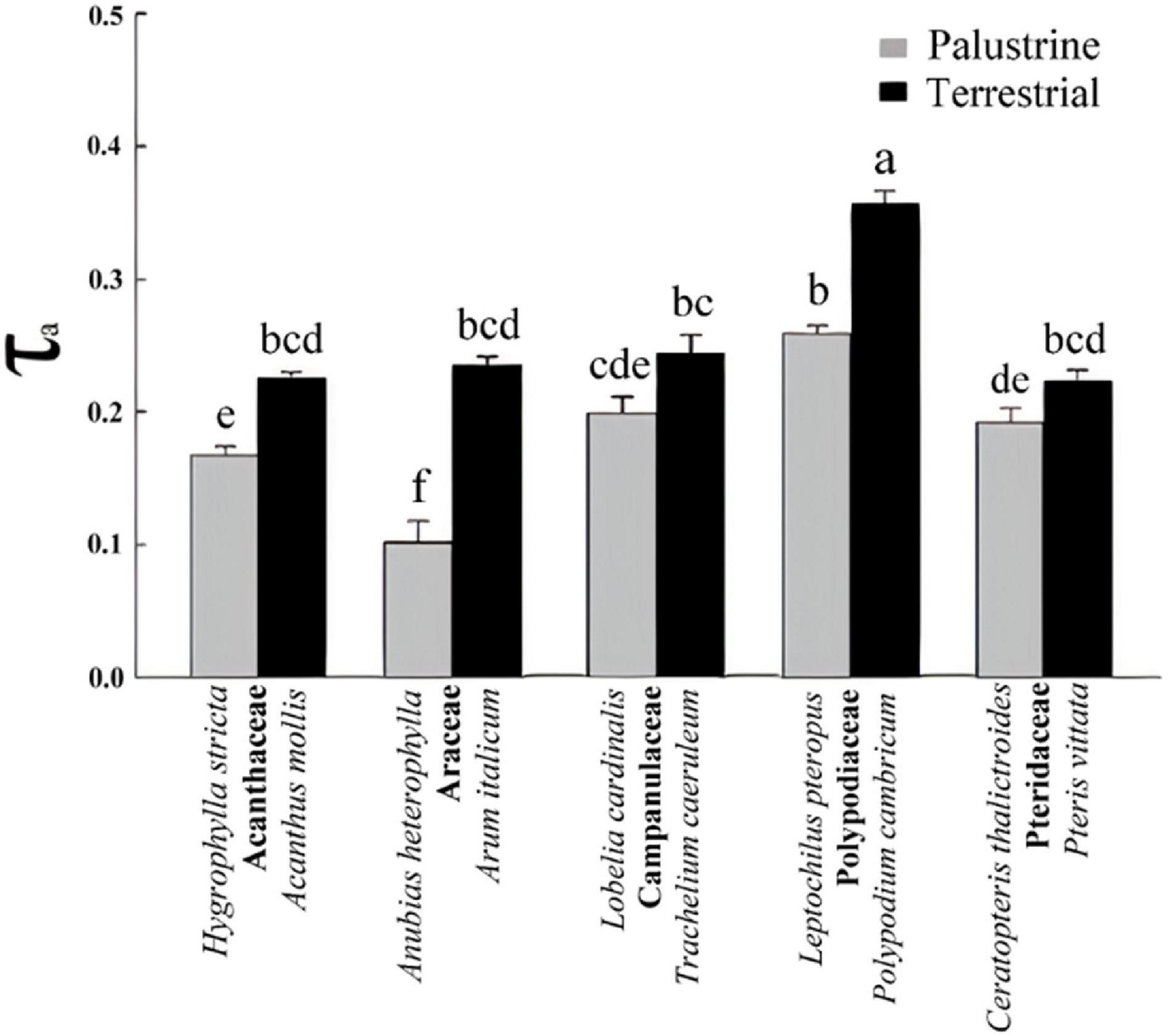
Figure 3. Electron partitioning to the alternative pathway (τa) in all palustrine and terrestrial species tested in this study. Values are the mean of six to eight measurements obtained from 4 to 6 plants per species. Different letters indicate significant differences with a p-value < 0.05 determined by a post hoc Tukey–Kramer’s test.
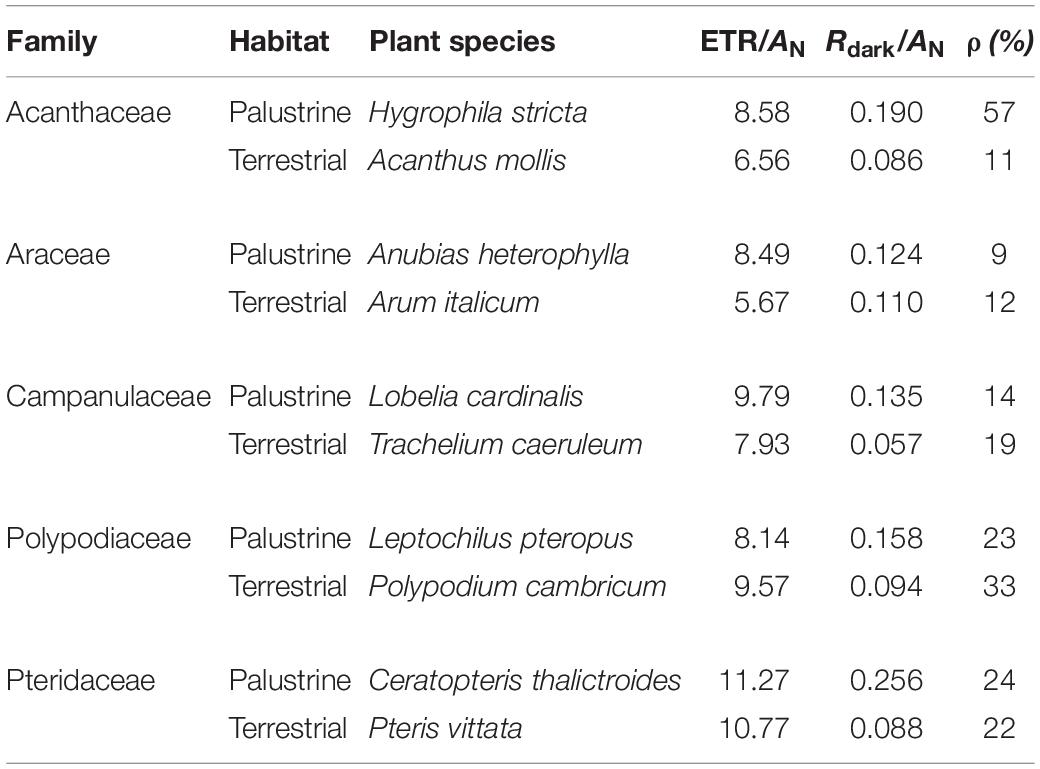
Table 3. General characteristics of the studied terrestrial and palustrine plant species: the ratio of electron transport rate (ETR) to net photosynthesis (AN), the ratio of dark respiration (Rdark) to AN, and the ratio of valt to Valt (ρ).
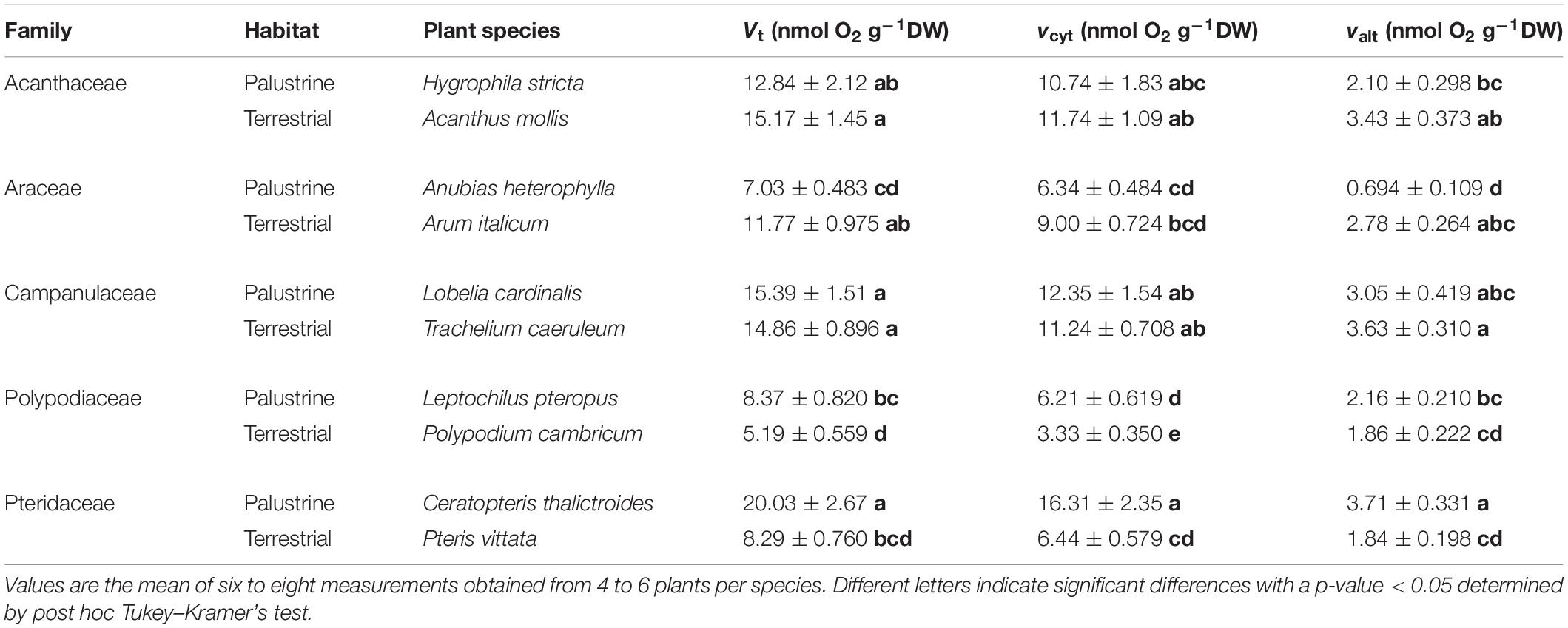
Table 4. Total respiration (Vt) and the in vivo activities of cytochrome oxidase (vcyt) and alternative oxidase (valt) in aerial leaves of ten different terrestrial and palustrine plant species (see section “Materials and Methods”).
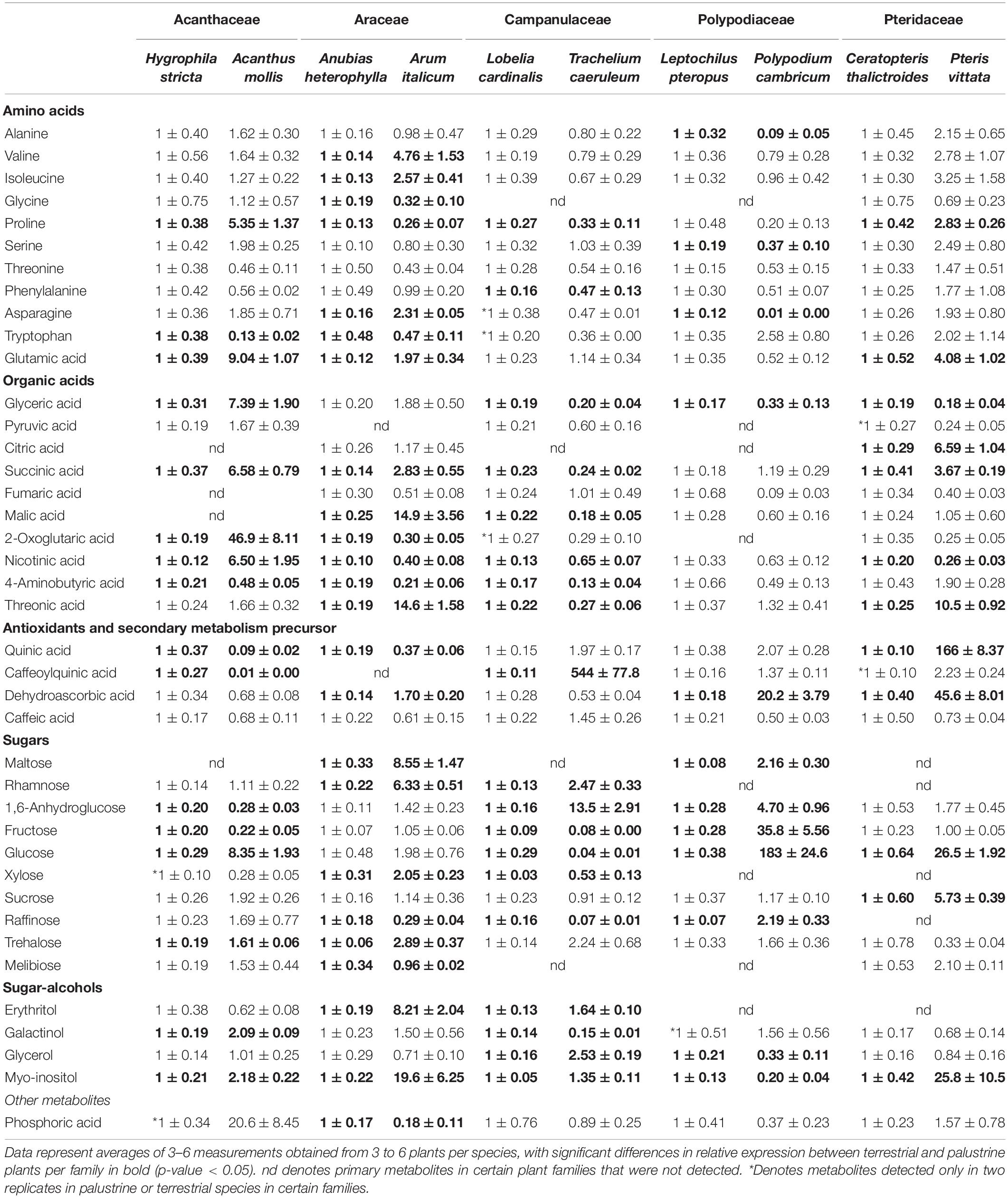
Table 5. Relative metabolite levels in leaves of 10 terrestrial and palustrine plant species belonging to five families of ferns and angiosperms as measured by GC-MS (see section “Materials and Methods”).
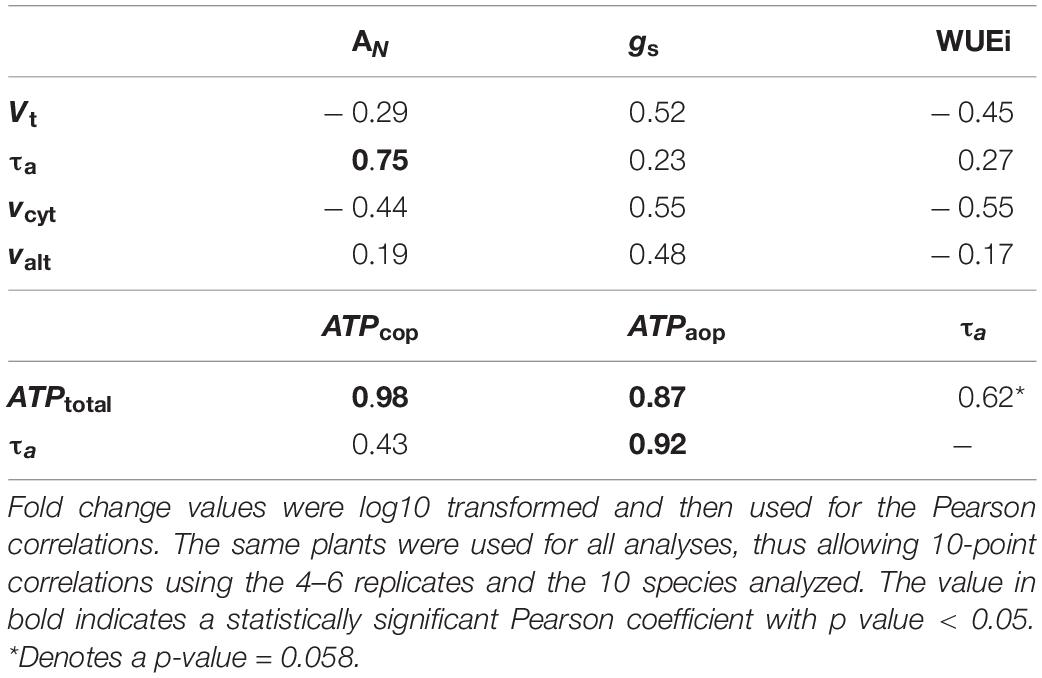
Table 6. Pearson correlation coefficients between fold changes in photosynthetic parameters levels (AN, gs, WUEi) and in vivo respiratory parameters levels (Vt, vcyt, τa, valt), and between fold changes in respiratory parameters (τa and ATPtotal) and ATP synthesis through each pathway (ATPcop and ATPaop), in leaves of ten species of palustrine and terrestrial vascular plants (Table 1).
Results
Spatial Patterns
A species classification into biomes was obtained from a Whittaker diagram of MAT and MAP (Figure 1 and Supplementary Table 1; Wright et al., 2004). We observed species records in all biomes, especially in shrubland, temperate forest, tropical seasonal forest, woodland, and desert (25.6, 24.0, 22.2, 12.6, and 9.89% total records). A low register was found in tropical rainforest, grassland, temperate rainforest, boreal forest, and tundra (4.06, 1.11, 0.48, 0.03, and 0.02% total records). In general, palustrine species were more abundant than terrestrial species in biomes with values of MAP ≥ 1000 mm, such as temperate forest (33.0% palustrine vs. 15.1% terrestrial), tropical seasonal forest (32.3% palustrine vs. 12.2% terrestrial), and tropical rainforest (6.40% palustrine vs. 1.72% terrestrial). In biomes with values of MAP ≤ 1000 mm, palustrine species were more abundant only in woodland (19.3% palustrine vs. 5.96% terrestrial), whilst terrestrial species were more abundant than palustrine species in arid biomes such as shrubland (46.4% terrestrial vs. 4.72% palustrine) and desert (17.6% terrestrial vs. 2.16% palustrine). Specific abundances in each type of biome can be found in Supplementary Table 1.
Leaf Gas Exchange
Regarding net photosynthesis (AN), comparisons between groups showed no differences between angiosperms (Acanthaceae, Araceae, Campanulaceae) and ferns (Polypodiaceae, Pteridaceae) in terrestrial habitats; however among palustrine species, AN was significantly lower in the two ferns species compared to the angiosperm L. cardinalis (Campanulaceae; Figure 2A). When comparing between counterparts in each family, AN was significantly higher (by 2.5-fold) in terrestrial species of Acanthaceae, Araceae, Polypodiaceae, and Pteridaceae. Regarding gs among terrestrial species, this parameter was significantly lower in the fern P. cambricum (Polypodiaceae) only when compared with the angiosperm T. caeruleum (Campanulaceae). Contrary to what was observed for AN, no differences were found in gs when comparing between counterparts in each family (Figure 2B).
With regard to WUEi, no major differences were observed between ferns and angiosperms in terrestrial habitats; whilst among palustrine species, the two ferns species showed a significantly lower WUEi when compared to the angiosperm A. heterophylla (Araceae; Figure 2C). Very similar to the trends observed for AN, WUEi was significantly higher (by 3.7-fold) in terrestrial counterparts of Acanthaceae, Polypodiaceae, and Pteridaceae, with the terrestrial fern P. cambricum (Polypodiaceae) showing the highest values of WUEi, and both the palustrine angiosperm H. stricta (Acanthaceae) and fern L. pteropus (Polypodiaceae) displaying the lowest values of WUEi (Figure 2C). On the other hand, palustrine plants showed higher averaged values of ETR/AN (9.25) and Rdark/AN (0.173) than terrestrial plants (ETR/AN = 8.10, Rdark/AN = 0.087) mainly because their small AN, and secondary, because the lack of major variations in Rdark and ETR (Tables 2, 3 and Supplementary Table 2).
Respiration and Electron Partitioning to the Alternative Oxidase Pathway
A high heterogeneity was found in Vt, vcyt, and valt among all species. Considering that most of Vt takes place via COX activity, a similar heterogeneity was found in vcyt and Vt, with both varying significantly by 3.3 and 2.7-fold, across species in the terrestrial and palustrine environments, respectively. Both valt and τa showed less variability than vcyt and Vt across terrestrial species (2.0 and 1.6-fold, respectively). In palustrine environments, higher variability was found in valt, differing significantly 5.4-fold across species, whilst τa showed similar variability to vcyt and Vt (2.6-fold). When comparing between counterparts in each family, Vt was significantly higher in terrestrial counterparts of Araceae (by 1.7-fold), and in palustrine counterparts from both fern families, Polypodiaceae and Pteridaceae (by 1.6-fold and 2.4-fold respectively; Table 2), differing slightly from vcyt, which was no different in terrestrial counterparts of Araceae (Table 4). A different pattern was observed for valt, which was significantly higher in the terrestrial counterpart of Araceae (4.0-fold) and in the palustrine counterpart of Pteridaceae (2.0-fold). A similar behavior was observed for ATP production modeled from vcyt and valt (Supplementary Table 3). Regarding τa, the terrestrial counterparts of Acanthaceae, Araceae, and Polypodiaceae showed significantly higher values than their palustrine counterparts, 1.4, 2.3, and 1.4-fold, respectively. It is worth mentioning that in Polypodiaceae, the two ferns showed the highest values of τa in each habitat (Figure 3). On the other hand, leaves of H. stricta showed the highest engagement of AOP (ρ) (57%) mainly because the low Valt, followed by leaves of plants in Polypodiaceae and Pteridaceae (25.5%) that showed variability in Valt and valt, and by leaves of plants in Campanulaceae and of terrestrial plants in Araceae and Acanthaceae (14%) that displayed large Valt. The palustrine A. heterophylla showed the lowest ρ (9%) because the low valt (Tables 3, 4 and Supplementary Table 2).
In order to better understand the changes in photosynthetic parameters driving the species-specific response of the respiratory parameters, fold changes of AN, gs and WUEi values were correlated with fold changes of Vt, τa, vcyt, and valt as described in the statistical analyses section. The only significant correlation (r = 0.75) can be found between AN and τa. Similarly, to study whether AOP contributes significantly to ATP synthesis, fold changes of τa and ATPtotal values were correlated with fold changes of τa, ATPcop and ATPaop. Significant correlations can be found between ATPtotal and energy synthesis by each pathway (ATPcop and ATPaop; r = 0.98 and 0.87), and between τa and ATPaop (r = 0.98; Table 6).
Relative Metabolite Levels
By using GC-MS-based metabolite profiling from the aerial leaves of palustrine and terrestrial plants, we annotated 40 metabolites (Supplementary Table 5), including sugars, amino acids, organic acids, antioxidants and secondary metabolite precursors, as well as sugar-alcohols (Table 5). Although the identification of 17 metabolites (glycine, asparagine, tryptophan, phosphoric acid, pyruvic acid, citric acid, malic acid, fumaric acid, 2-oxoglutaric acid, quinic acid caffeoyl, maltose, rhamnose, xylose, raffinose, melibiose, erythritol, and galactinol) were only partly detected (n = 2) or not detected at all (nd) in certain species, they were considered for a general interpretation of the results. Significant changes (Student’s t test, p < 0.05) in metabolite levels were observed for each metabolite, in the comparison between terrestrial and palustrine counterparts in each family, with the exception of threonine, pyruvic acid, fumaric acid, and caffeic acid.
Focusing on photosynthetic routes, we observed that Campanulaceae, the only family which showed no significant differences in AN between palustrine and terrestrial counterparts, showed the largest number of metabolites (19), mainly sugars and organic acids, with reduced levels in the terrestrial species when compared to the palustrine counterpart (Table 5). In contrast, terrestrial species of Acanthaceae, Araceae, Polypodiaceae, and Pteridaceae, with higher values of AN than their palustrine counterparts, showed higher levels of sugars such as sucrose, fructose or glucose (Table 5), suggesting a higher energy status. We also observed that Araceae, with significantly higher gs in the terrestrial counterpart, was the only family also showing higher levels of metabolites such as malate and maltose, which are considered of interest due to their roles in determining stomatal movement (Fernie and Martinoia, 2009; Araújo et al., 2011; Gago et al., 2016).
Regarding respiratory routes, in Araceae, the only family showing higher Vt in the terrestrial counterpart, the lack of change and decrease in citrate and 2-oxoglutarate levels, respectively, together with increases in downstream intermediates (succinate and malate) suggests a high TCA cycle activity (Table 5). This pattern was significantly different (increased citrate levels with no changes in 2-oxoglutarate and malate) in the two terrestrial fern species that displayed lower Vt and vcyt, when compared to their palustrine counterparts, presumably due to lower TCA cycle decarboxylation activity. In this comparison, pronounced differences in γ-aminobutyric acid (GABA) levels – which are intimately connected to TCA cycle activity – between ferns and angiosperms suggest a different role for the GABA-shunt. In addition, the large accumulation of sugars such as sucrose, glucose, and fructose in ferns (Table 4) coincided with an accumulation of antioxidant and secondary metabolism precursors such as quinic acid and dehydroascorbic acid, likely indicative of a reduction in sugar oxidation by glycolysis and the TCA cycle while also promoting the accumulation of antioxidant and secondary metabolism precursors (Table 5). Notably, in Araceae, the only family showing higher values of valt in the terrestrial counterpart, we observed higher levels of metabolites such as valine, isoleucine, and malate, which are considered of interest due to their positive correlation with valt in previous studies (Florez-Sarasa et al., 2012; Del-Saz et al., 2016).
Given the observed general tendency of several physiological parameters to correlate with several metabolites (Figures 2A, 3 and Table 5), we further investigated the observed respiratory patterns for each habitat group employing PLS statistical modeling combined with variable importance for projection (VIP) as a criterion to elucidate metabolite relevance from the generated models (Gago et al., 2016). This modeling helps to highlight putative metabolic networks that differentially drive the respiratory processes in the terrestrial as compared to the palustrine species studied. We used Vt, vcyt, valt, and τa as response variables and, after cross-validation (CV) of the generated models by the PLS, only models for τa can be considered robust due to the display of a R2 higher than 0.6, for both terrestrial (R2 = 0.62) and palustrine (R2 = 0.7) habitats. For palustrine species, significant associations with phosphoric acid, proline, glucose, malic acid, glyceric acid, quinic acid, quinic acid caffeoyl, fructose, GABA, and threonine were observed (Figure 4 and Supplementary Table 4). For terrestrial species, associations with τa were observed for trehalose, sucrose, glucose, threonic acid and glycerol (Supplementary Table 4). Interestingly, sugar metabolism was importantly related to τa for both lifestyle strategies, glucose being the only metabolite significantly associated in both; despite sugar metabolism in each family differing in the other metabolite associations. Terrestrial species associated mostly with levels of trehalose and sucrose, while palustrine species were mainly associated with phosphoric acid and proline.
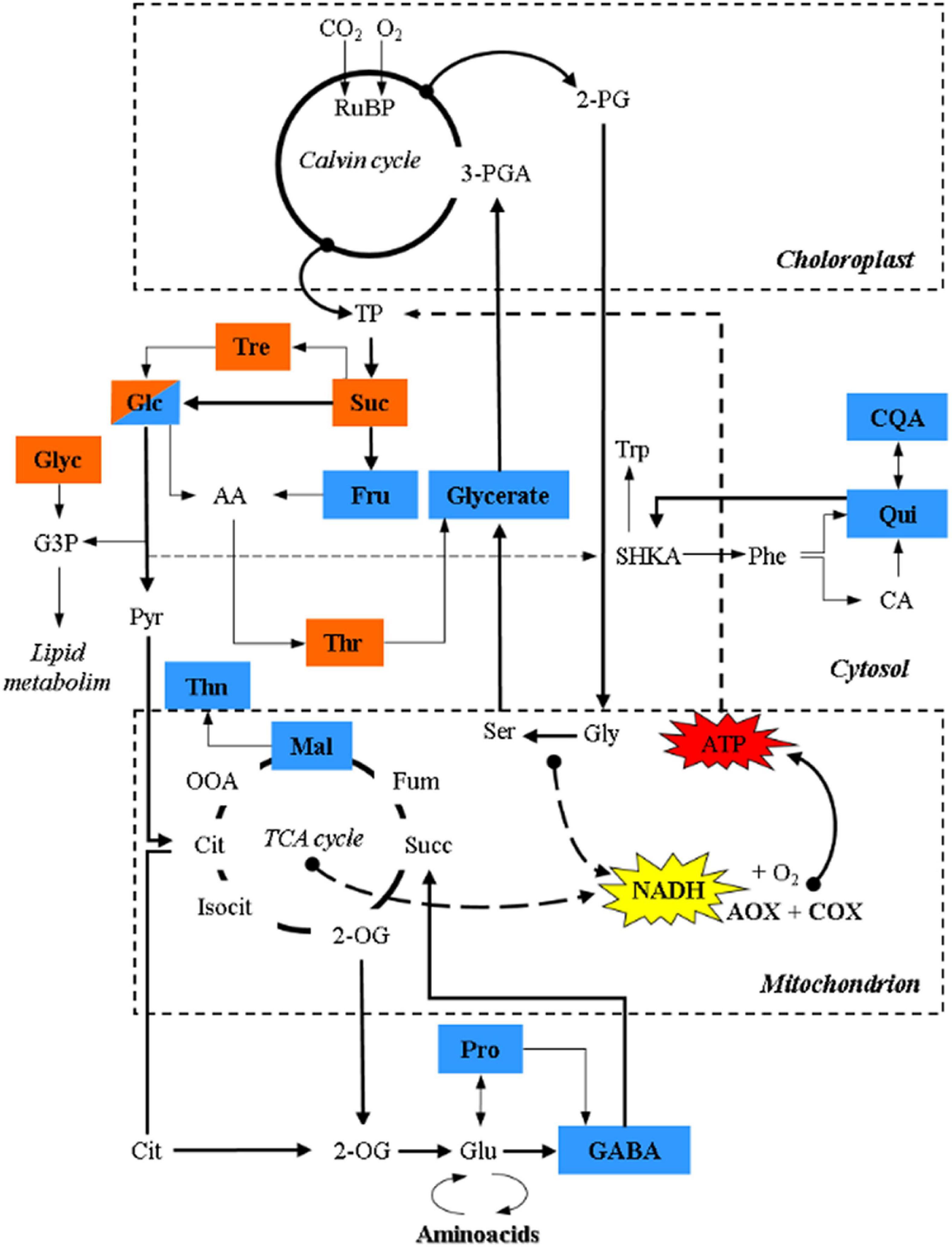
Figure 4. Schematic representation of the TCA cycle and its connection with metabolites, related to sugar metabolism, photorespiration and secondary metabolism, showing significant relationships with τa identified with a PLS approach through multivariate regression modeling. Brown and blue colors denote significant relationships with τa in terrestrial and palustrine environments, respectively. 2-PG, 2-Phosphoglycolate; 3-PGA, 3-Phosphoglyceric acid; RuBP, RuBisCO; TP, triose phosphate; Suc, sucrose; Tre, Trehalose; Glc, Glucose; AA, Ascorbic acid; Fru, fructose; Thr, threonic acid; Gly, glycine; Glyc, glycerol; G3P, glycerol 3-phosphate; SHKA, shikimate; Trp, tryptophan; Phe, phenylalanine; CA, caffeic acid; Qui, quinic acid; CQA, caffeoylquinic acid; Pyr, pyruvate; Cit, citrate; OOA, oxaloacetate; Mal, malate; Thn, threonine; Fum, fumarate; Suc, succinate; 2-OG, 2-oxoglutarate; Pro, proline; GABA, γ-aminobutyric acid; Glu, glutamate.
Discussion
Habitats Are Associated With Different AN, Water Use Efficiency and Electron Partitioning to Alternative Oxidase Pathway
In order to characterize terrestrial and palustrine species under the contrasting redox conditions that broadly differentiate both habitats, we decided to maintain plants under different light intensities to fall close to an optimum for each lifestyle. This is because palustrine plants are more often covered by dense canopy trees in humid forests than terrestrial plants in semi-arid Mediterranean forests, according to spatial distribution of plant records and sample collection coordinates of terrestrial plants (Figure 1 and Table 1). Besides, in humid forest, ground layer plant species may display shade adaptations like low light saturation and light compensation points (Chazdon and Pearcy, 1991; Meng et al., 2014), which led us to photosynthetically characterize these species at different PPFD. We did not expose plants to changing light intensities because it is well known that changes in growth light intensity does not affect oxygen isotope discrimination or τa as observed in leaves of Arabidopsis thaliana (Florez-Sarasa et al., 2011) and of sun and shade species (Noguchi et al., 2001). However, we ensured that experimental conditions were non-stressful, and enough to allow ETR/AN values typical of irrigated plants, positive leaf carbon balance and low AOP engagement (and enough overcapacity) in all species (Table 3).
As leaves of terrestrial plants have large energy input because in air the light level is high, the terrestrial species A. mollis, A. italicum, P. cambricum, and P. vittata showed higher AN than their palustrine counterparts H. stricta, A. heterophylla, L. pteropus, and C. thalictroides in Acanthaceae, Araceae, Polypodiaceae, and Pteridaceae, respectively (Figure 2A). This coincided with higher levels of sugars (e.g., sucrose, fructose, and glucose; Table 5), which were considered as markers of high photosynthetic activity (Gago et al., 2016). In contrast, no differences in AN were found between T. caeruleum and L. cardinalis in Campanulaceae, which coincides with important reductions in sugars and organic acids in T. caeruleum with respect to L. cardinalis (Table 5). Because the higher AN, WUEi, the ratio between AN and gs, was found to be larger in Acanthaceae, Polypodiaceae, and Pteridaceae (Figure 2C), which could be in line with previous studies describing a differential regulation of ecosystem (WUE) among biomes. In arid ecosystems, WUE is primarily controlled by evaporation; whilst in sub-humid regions, WUE is mostly regulated by assimilation (Yang R. et al., 2016), which could be partly due to a different predominance of palustrine and terrestrial records displaying contrasting values of WUEi (Figure 1 and Figure 2C) agreeing with the idea of water losses acting as a driving force for the evolution in land plants of gas exchange regulation system (Raven, 2002; Berry et al., 2010; Assouline and Or, 2013).
Contrary to AN, total respiration (Vt) was not higher in the terrestrial species of Acanthaceae, Araceae, Polypodiaceae, and Pteridaceae than in their palustrine counterparts. Differences in Vt were found among families in each habitat and between ferns and angiosperms (Table 4), similar to previous studies (Choy-Sin and Suan, 1974; Boyce and Mohamed, 1987; Davey et al., 2004; Hilman and Angert, 2016; Zhu et al., 2021). Variability was also found regarding valt and vcyt (Table 2). Respiration in leaves is highly variable among species as it depends on leaf characteristics such as leaf lifespan, nitrogen content, growth forms, and differential nutritional requirements, regardless of lifestyle or biome (Grime and Hunt, 1975; Reich et al., 1998; Lusk and Reich, 2000; Millenaar et al., 2001; Wright et al., 2004; Atkin et al., 2015). Moreover, the carbon cost for leaf growth and maintenance may differ among species (Lambers et al., 2008). This is why τa, which represents the contribution of AOX to Vt, represents a better proxy to evaluate the importance of AOX activity for plant respiration when comparing among different plant species. In vivo AOX activity accounted for 10-36% of Vt in both palustrine and terrestrial species considered here, which is within the range of values observed under both stressful and non-stressful conditions in terrestrial species (10–50%; Del-Saz et al., 2018a), and here, it was strongly influenced by habitat (Table 2). The contribution of AOX to Vt was significantly higher in terrestrial species from Acanthaceae, Araceae, and Polypodiaceae (Figure 3). In model terrestrial plants, previous studies reported τa increases under abiotic stressors mainly due to reductions in vcyt because the COX pathway is more sensitive to stressors than the AOX pathway (Del-Saz et al., 2018a), which helps to explain the different effect of habitat on both vcyt and valt (Table 2). Considering the highest values of AN and τa observed among terrestrial species (Figures 2A, 3) and the significant Pearson coefficient between these parameters (Table 6), the AOP is likely more important for the dissipation of excess energy in terrestrial plants than in palustrine plants, which is in line with previous studies describing higher oxygen isotope discrimination in sun leaves than in shade leaves (Noguchi et al., 2001). Moreover, this coincided with metabolic increases in the levels of several sugars and AN (Figure 2A and Table 5). Interestingly, τa was variable among terrestrial and palustrine species (Figure 3), suggesting that valt is coupled to fundamental metabolic processes under non-stress conditions that may differ among species (Florez-Sarasa et al., 2016). Regarding the differences observed between groups, previous studies suggested that the post-translational regulation of AOXs in ferns may differ from those of angiosperms because of the presence of a SerI residue instead of a CysI residue in the majority of the AOX protein sequences analyzed, which could presumably affect valt (Neimanis et al., 2013).
The Electron Partitioning to the Alternative Oxidase Pathway Is Linked to Habitat-Specific Metabolic Routes
A PLS approach through multivariate regression modeling identified significant relationships only between τa and several metabolites in each habitat (Figure 4 and Supplementary Table 4). In terrestrial plants, significant relationships were identified only between τa and metabolites related to sugar metabolism (sucrose, glucose, and trehalose). All of these carbohydrates are closely linked to glycolytic activity or sucrose synthesis that are highly dependent on leaf ATP synthesis or requirements (Lunn et al., 2006; Dimroth and von Ballmoos, 2008; Lim et al., 2020). In addition, the accumulation of these sugars likely confers osmotolerance and redox homeostasis in both ecosystems (Robe and Griffiths, 2000). Sucrose is a metabolic precursor of trehalose, via trehalose-6-phosphate, which acts as a signal for high carbon availability in the form of sucrose (Schluepmann et al., 2004; Lunn et al., 2006; Paul et al., 2010; Fichtner and Lunn, 2021), which is in line with the high rates of AN observed in terrestrial plants (Figure 2A). Trehalose is hydrolyzed by trehalase into glucose, and together with fructose (a product of the reactions catalyzed by both invertase and sucrose synthase) are metabolic precursors of ascorbic acid (AA), one of the most abundant antioxidants in plants (Smirnoff and Wheeler, 2000; Hossain et al., 2017). AA can be metabolized to compounds like threonate (Hancock and Viola, 2005; DeBolt et al., 2006; Smirnoff, 2018) which showed a significant relationship with τa in terrestrial plants. Notably, previous studies under salinity conditions highlighted a relationship between the AOP and erythronic acid (Del-Saz et al., 2016), a degradation product of AA (Green and Fry, 2005), reinforcing the role of the AOP in mitochondrial AA synthesis (Millar et al., 2003; Bartoli et al., 2006; Del-Saz et al., 2016). In addition, threonate is also a precursor of osmoprotectants (Guerrier et al., 2000; Jouve et al., 2004; Muscolo et al., 2015). On the other hand, τa in terrestrial plants also showed a significant relationship with glycerol, which is a lipid precursor, that similar to trehalose, is thought to be produced as a consequence of an enhanced CO2 assimilation in the Calvin-Benson cycle and/or from starch degradation (Liska et al., 2004), which corresponds to the highest values of photosynthesis, foliar carbon balance and oxygen isotope discrimination observed in terrestrial plants (Figures 1A, 3 and Table 3).
Palustrine plants displayed a higher energy efficiency of respiration bearing in mind their lower τa, the significant Pearson coefficient between ATPaop and ATPtotal (Table 6), and the highest VIP value obtained from the relationship between τa and phosphate (Supplementary Table 4), perhaps indicative of a tendency to save phosphorus during oxidative phosphorylation for the benefit of ATP synthesis via COX. Besides, we identified relationships between τa and primary metabolites related to sugar metabolism, photorespiration, secondary metabolism, the TCA cycle and ammonium assimilation. Precisely, we found a significant relationship between τa and glycerate, corresponding to the described role of AOP in dissipating reducing equivalents from photorespiration (Watanabe et al., 2016; Timm and Hagemann, 2020), and suggesting a role of photorespiration in palustrine plants as previously described (Maberly and Spence, 1989). The relationships between τa and acyl-quinic acids (Qui, CQA; Figure 4) in palustrine plants suggest a participation of the AOP in modulating carbon supply for these chlorogenic acids, whose accumulation is associated with enhanced tolerance to oxidative stress (Tamagnone et al., 1998; Niggeweg et al., 2004), and competes with the accumulation of shikimate and derived metabolites (Marsh et al., 2009), such as phenylalanine and tryptophan. The reversible esterification of caffeoyl-CoA (whose metabolic precursor is CA) with Qui produces CQA. By the conversion of Qui to shikimate (Clifford et al., 2017), the shikimate pathway provides precursors for the synthesis of tryptophan that in turn is a metabolic precursor for the biosynthesis of auxins. In heterophyllous amphibious plants, auxin synthesis may be enhanced due to alterations in the perception of blue light in submerged leaves. This is part of a mechanism to coordinate, together with other plant hormones, phenotypic plasticity in leaf form or heterophylly (Nakayama et al., 2012, 2014, 2017; Li et al., 2019, 2021). On the other hand, the significant relationships between τa and malate, GABA, and proline suggest that the AOP could also be related to the carbon supply for both the TCA cycle and ammonium assimilation. Through the mitochondrial 2-OG/malate transporter, malate can facilitate GABA transport (Ramesh et al., 2018; Bown and Shelp, 2020), whose synthesis mainly occurs from glutamate by the cytosolic glutamate decarboxylase, alternatively through polyamine degradation (Yang Y. et al., 2016), or by the oxidation of proline to glutamate in mitochondria (Fait et al., 2008; Shelp et al., 2012). Moreover, both GABA and proline may act as osmoprotectants and their catabolism in mitochondria can provide reducing equivalents as substrates for the AOP (Studart-Guimarães et al., 2007; Michaeli et al., 2011; Florez-Sarasa et al., 2021), which is in agreement with the relationships identified between τa and these metabolites in palustrine plants (Figure 4 and Supplementary Table 4). On top of this, GABA can act as a transducer of environmental stress signals leading to the activation of genes for ethylene and abscisic acid biosynthesis (Kinnersley and Turano, 2000; Forde and Lea, 2007). Overall, the relationships between τa and metabolites related to hormone biosynthesis and signaling in palustrine environments could be especially relevant for heterophyllous amphibious plants. All these signaling metabolites, together with gibberellins, mediate perception and responses to fluctuations of water levels, and control the synthesis of new developing aerial leaves in the transition from a submerged to an aerial habit (Cox et al., 2004; Jackson, 2008; Chater et al., 2014; Kim et al., 2018). Whilst some evidence has suggested that plant hormones such as abscisic acid, ethylene, gibberellins, and auxins are part of signaling networks controlling AOX expression (Ivanova et al., 2014; Berkowitz et al., 2016), their control of in vivo AOX activity remains, even in model terrestrial plants, to be tested.
Conclusion
Here we performed a comparative study of photosynthesis, WUEi, and respiration in palustrine and terrestrial species of angiosperms and ferns widely distributed across biomes, and maintained at different availability of energy and water in their habitats. Our experimental design does not allow the identification of the most important primary force (light or water) driving associations between the respiratory parameters and the metabolites. However, under different redox conditions that broadly characterize their habitats in nature, we found evidence of a large entry of energy into leaves of terrestrial plants considering their higher values of AN, WUEi, and τa, as well as their significant relationships between τa and metabolites related to both sugar metabolism and osmotolerance. In palustrine plants, changes in τa could modulate the supply of carbon skeletons from sugars to metabolic routes involved in the production of hormones and signaling molecules important for heterophylly (e.g., the shikimate pathway and GABA shunt). Further experiments are needed in amphibious plants in order to study the precise regulation of the AOX pathway during the development of new aerial leaves during their emergence from water. In addition, the low τa observed together with the identification of τa relationships with phosphoric acid and other respiratory parameters suggests that mitochondrial electron partitioning contributes to maximizing the ATP yield of respiration in palustrine plants.
Dedication
We would like to honor this manuscript to Prof. James N. Siedow. Jim taught me how to take science so seriously that only Duke basketball was at the same level. Jim could simultaneously smash you with the toughest question of the world, or plant biochemistry, and ensure that you could find the answer by yourself. The velocity of his brain was so high that by the time anyone could catch up with him, he was already smashing with the next joke. His jokes were always sharp, incisive, and funny. And, “so, What’s your point?” – MR-C.
Data Availability Statement
The raw data supporting the conclusions of this article will be made available by the authors, without undue reservation.
Author Contributions
JF, JG, MR-C, and ND-S conceived and designed the idea of this experiment. MC identified and recollected all plant species. CD carried out the gas-exchange measurements. ND-S carried out the measurements of respiration. IF-S carried out the metabolic analysis. JG carried out the PLS approach. AR-M carried out the spatial distribution analysis. ND-S, JO, and CS wrote the first draft of the manuscript with subsequent inputs from all co-authors. All authors have read and agreed to the published version of the manuscript.
Funding
This work was mainly supported by Ministerio de Economía y Competitividad (MINECO, Spain) (project PGC2018-093824-B-C41) and the ERDF (FEDER). Others funding were provided by FONDECYT No. 1191118 from National Agency for Research and Development (ANID) and the Chilean Scholarship Program/Becas de doctorado nacional/2017–21180329, the European Union’s Horizon 2020 Research and Innovation Program under the Marie Skłodowska-Curie grant agreement no. 753301, the ‘Ramon y Cajal’ contract RYC2019-027244-I/AEI/10.13039/501100011033 and the European Social Fund.
Conflict of Interest
The authors declare that the research was conducted in the absence of any commercial or financial relationships that could be construed as a potential conflict of interest.
Publisher’s Note
All claims expressed in this article are solely those of the authors and do not necessarily represent those of their affiliated organizations, or those of the publisher, the editors and the reviewers. Any product that may be evaluated in this article, or claim that may be made by its manufacturer, is not guaranteed or endorsed by the publisher.
Acknowledgments
We are very grateful to Dr. Biel Martorell at the Serveis Cientifico-Tecnics of the Universitat de les Illes Balears for his help while running IRMS experiments, and to Chris Baldwin for his help with English corrections. Thanks to Bruce Osborne for fruitful discussions during the International Plant Ecophysiology Colloquium held at Katalapi’s Park.
Supplementary Material
The Supplementary Material for this article can be found online at: https://www.frontiersin.org/articles/10.3389/fpls.2021.752795/full#supplementary-material
Footnotes
References
Araújo, W. L., Tohge, T., Ishizaki, K., Leaver, C. J., and Fernie, A. R. (2011). Protein degradation–an alternative respiratory substrate for stressed plants. Trends Plant Sci. 16, 489–498. doi: 10.1016/j.tplants.2011.05.008
Arteaga-Vazquez, M. A. (2016). Land plant evolution: listen to your elders. Curr. Biol. 26, 26–29. doi: 10.1016/j.cub.2015.12.001
Asada, K. (2006). Production and scavenging of reactive oxygen species in chloroplasts and their functions. Plant Physiol. 141, 391–396. doi: 10.1104/pp.106.082040
Assouline, S., and Or, D. (2013). Plant water use efficiency over geological time-evolution of leaf stomata configurations affecting plant gas exchange. PLoS One 8:e67757. doi: 10.1371/journal.pone.0067757
Atkin, O. K., Bloomfield, K. J., Reich, P. B., Tjoelker, M. G., Asner, G. P., Bonal, D., et al. (2015). Global variability in leaf respiration in relation to climate, plant functional types and leaf traits. New Phytol. 206, 614–636. doi: 10.1111/nph.13253
Bartoli, C. G., Yu, J., Gomez, F., Fernández, L., McIntosh, L., and Foyer, C. H. (2006). Inter-relationships between light and respiration in the control of ascorbic acid synthesis and accumulation in Arabidopsis thaliana leaves. J. Exp. Bot. 57, 1621–1631. doi: 10.1093/jxb/erl005
Berkowitz, O., De Clercq, I., Van Breusegem, F., and Whelan, J. (2016). Interaction between hormonal and mitochondrial signaling during growth, development and in plant defense responses. Plan Cell Environ. 39, 127–1139. doi: 10.1111/pce.12712
Berry, J. A., Beerling, D. J., and Franks, P. J. (2010). Stomata: key players in the earth system, past and present Curr. Opin. Plant Biol. 13, 232–239. doi: 10.1016/j.pbi.2010.04.013
Bhattacharya, D., and Medlin, L. (1998). Algal phylogeny and the origin of land plants. Plant Physiol. 116, 9–15. doi: 10.1104/pp.116.1.9
Bowes, G., and Salvucci, M. E. (1989). Plasticity in the photosynthetic carbon metabolism of submersed aquatic macrophytes. Aquat. Bot. 34, 233–266. doi: 10.1016/0304-3770(89)90058-2
Bowman, J. L., Kohchi, T., Yamato, K. T., Jenkins, J., Shu, S., Ishizaki, K., et al. (2017). Insights into land plant evolution garnered from the Marchantia polymorpha genome. Cell. 171, 287–304. doi: 10.1016/j.cell.2017.09.030
Bown, A. W., and Shelp, B. J. (2020). Does the GABA Shunt Regulate Cytosolic GABA? Trends Plant Sci. 25, 422–424. doi: 10.1016/j.tplants.2020.03.001
Boyce, A., and Mohamed, M. H. (1987). Photosynthetic and respiratory characteristics of Malayan sun and shade ferns. New Phytol. 105, 81–88. doi: 10.1111/j.1469-8137.1987.tb00112.x
Brodribb, T. J., Carriquí, M., Delzon, S., McAdam, S. A. M., and Holbrook, N. M. (2020). Advanced vascular function discovered in a widespread moss. Nat. Plants. 6, 273–279. doi: 10.1038/s41477-020-0602-x
Catling, D. C., and Claire, M. W. (2005). How Earth’s atmosphere evolved to anoxic state: a status report. Earth Planet Sci. Lett. 237, 1–20. doi: 10.1016/j.epsl.2005.06.013
Chater, C. C., Oliver, J., Casson, S., and Gray, J. E. (2014). Putting the brakes on: abscisic acid as a central environmental regulator of stomatal development. New Phytol. 202, 376–391. doi: 10.1111/nph.12713
Chazdon, R. L., and Pearcy, R. W. (1991). The Importance of Sunflecks for Forest Understory Plants. BioScience 41, 760–766. doi: 10.2307/1311725
Choy-Sin, H., and Suan, W. Y. (1974). Photosynthesis and respiration of ferns in relation to their habitat. Amen. Fern J. 64, 40–48. doi: 10.2307/1546761
Clemente-Moreno, M. J., Omranian, N., Sáez, P., Figueroa, C. M., Del-Saz, N., Elso, M., et al. (2019). Cytochrome respiration pathway and sulphur metabolism sustain stress tolerance to low temperature in the Antarctic species Colobanthus quitensis. New Phytol. 225, 754–768. doi: 10.1111/nph.16167
Clifford, M. N., Jaganath, I. B., Ludwig, I. A., and Crozier, A. (2017). Chlorogenic acids and the acyl-quinic acids: discovery, biosynthesis, bioavailability and bioactivity. Nat. Prod. Rep. 34, 1391–1421. doi: 10.1039/C7NP00030H
Cox, M. C., Benschop, J. J., Vreeburg, R. A., Wagemaker, C. A., Moritz, T., Peeters, A. J., et al. (2004). The roles of ethylene, auxin, abscisic acid, and gibberellin in the hyponastic growth of submerged Rumex palustris petioles. Plant Physiol. 136, 2948–2960. doi: 10.1104/pp.104.049197
Dahal, K., and Vanlerberghe, G. C. (2018). Improved chloroplast energy balance during water deficit enhances plant growth: more crop per drop. J. Exp. Bot. 69, 1183–1197. doi: 10.1093/jxb/erx474
Davey, P. A., Hunt, S., Hymus, G. J., DeLucia, E. H., Drake, B. G., Karnosky, D. F., et al. (2004). Respiratory oxygen uptake is not decreased by an instantaneous elevation of [CO2], but is increased with long-term growth in the field at elevated [CO2]. Plant Physiol. 134, 520–527. doi: 10.1104/pp.103.030569
De Vries, J., and Archibald, J. M. (2018). Plant evolution: landmarks on the path to terrestrial life. New Phytol. 217, 1428–1434. doi: 10.1111/nph.14975
De Vries, J., Stanton, A., Archibald, J. M., and Gould, S. B. (2016). Streptophyte terrestrialization in light of plastid evolution. Trends Plant Sci. 21, 467–476. doi: 10.1016/j.tplants.2016.01.021
DeBolt, S., Cook, D. R., and Ford, C. M. (2006). L-Tartaric acid synthesis from vitamin C in higher plants. PNAS. 103, 5608–5613. doi: 10.1073/pnas.0510864103
Del-Saz, N. F., Florez-Sarasa, I., Clemente-Moreno, M. J., Mahdhbi, H., Flexas, J., Fernie, A. R., et al. (2016). Salinity tolerance is related to cyanide-resistant alternative respiration in Medicago truncatula under sudden severe stress. Plant Cell Environ. 39, 2361–2369. doi: 10.1111/pce.12776
Del-Saz, N. F., Ribas-Carbó, M., Martorell, G., Fernie, A. R., and Florez-Sarasa, I. (2017a). Measurements of electron partitioning between cytochrome and alternative oxidase pathways in plant tissues. Plant Respiration and Internal Oxygen 2017, 203–217. doi: 10.1007/978-1-4939-7292-0_17
Del-Saz, N. F., Romero-Munar, A., Alonso, D., Aroca, R., Baraza, E., Flexas, J., et al. (2017b). Respiratory ATP cost and benefit of arbuscular mycorrhizal symbiosis with Nicotiana tabacum at different growth stages and under salinity. J. Plant Physiol. 218, 243–248. doi: 10.1016/j.jplph.2017.08.012
Del-Saz, N. F., Ribas-Carbó, M., McDonald, A. E., Lambers, H., Fernie, A. R., and Florez-Sarasa, I. (2018a). An in vivo perspective of the role(s) of the alternative oxidase pathway. Trends Plant Sci. 23, 206–219. doi: 10.1016/j.tplants.2017.11.006
Del-Saz, N. F., Romero-Munar, A., Cawthray, G. R., Palma, F., Aroca, R., Baraza, E., et al. (2018b). Phosphorus concentration coordinates a respiratory bypass, synthesis and exudation of citrate, and the expression of high-affinity phosphorus transporters in Solanum lycopersicum. Plant Cell Environ. 41, 865–875. doi: 10.1111/pce.13155
Delwiche, C. F., and Cooper, E. D. (2015). The evolutionary origin of a terrestrial flora. Curr. Biol. 25, 899–910. doi: 10.1016/j.cub.2015.08.029
Dimroth, P., and von Ballmoos, C. (2008). ATP synthesis by decarboxylation phosphorylation. Results Probl. Cell Differ. 45, 153–184. doi: 10.1007/400_2007_045
Fait, A., Fromm, H., Walter, D., Galili, G., and Fernie, A. R. (2008). Highway or byway: the metabolic role of the GABA shunt in plants. Trends Plant Sci. 13, 14–19. doi: 10.1016/j.tplants.2007.10.005
Fernie, A. R., and Martinoia, E. (2009). Malate: jack of all trades or master of a few? Phytochemistry. 70, 828–832. doi: 10.1016/j.phytochem.2009.04.023
Fichtner, F., and Lunn, J. E. (2021). The role of trehalose 6-phosphate (Tre6P) in plant metabolism and development. Annu. Rev. Plant Biol. 72, 1–24. doi: 10.1146/annurev-arplant-050718-095929
Finnegan, P. M., Umbach, A. L., and Wilce, J. A. (2003). Prokaryotic origins for the mitochondrial alternative oxidase and plastid terminal oxidase nuclear genes. FEBS Letters. 555, 425–430. doi: 10.1016/S0014-5793(03)01309-7
Flexas, J., and Gago, J. (2018). A role for ecophysiology in the ‘omics’ era. Plant J. 96, 251–259. doi: 10.1111/tpj.14059
Florez-Sarasa, I., Flexas, J., Rasmusson, A. G., Umbach, A. L., Siedow, J. N., and Ribas-Carbó, M. (2011). In vivo cytochrome and alternative pathway respiration in leaves of Arabidopsis thaliana plants with altered alternative oxidase under different light conditions. Plant Cell Environ. 34, 1373–1383. doi: 10.1111/j.1365-3040.2011.02337.x
Florez-Sarasa, I., Obata, T., Del-Saz, N. F., Reichheld, J. P., Meyer, E. H., Rodriguez-Concepcion, M., et al. (2019). The Lack of Mitochondrial Thioredoxin TRXo1 Affects In Vivo Alternative Oxidase Activity and Carbon Metabolism under Different Light Conditions. Plant Cell Physiol. 60, 2369–2381. doi: 10.1093/pcp/pcz123
Florez-Sarasa, I., Welchen, E., Racca, S., Gonzalez, D. H., Vallarino, J. G., Fernie, A. R., et al. (2021). Cytochrome c Deficiency Differentially Affects the In Vivo Mitochondrial Electron Partitioning and Primary Metabolism Depending on the Photoperiod. Plants. 10, 444. doi: 10.3390/plants10030444
Florez-Sarasa, I., Araujo, W. L., Wallstrom, S. V., Rasmusson, A. G., Fernie, A. R., and Ribas-Carbo, M. (2012). Light-responsive metabolite and transcript levels are maintained following a dark-adaptation period in leaves of Arabidopsis thaliana. New Phytol. 195, 136–148. doi: 10.1111/j.1469-8137.2012.04153.x
Florez-Sarasa, I., Ribas-Carbo, M., Del-Saz, N., Schwahn, K., Nikoloski, Z., Fernie, A. R., et al. (2016). Unravelling the in vivo regulation and metabolic role of the alternative oxidase pathway in C3 species under photoinhibitory conditions. New Phytol. 212, 66–79. doi: 10.1111/nph.14030
Forde, B. G., and Lea, P. J. (2007). Glutamate in plants: metabolism, regulation, and signalling. J. Exp. Bot. 58, 2339–2358. doi: 10.1093/jxb/erm121
Gago, J., Carriquí, M., Nadal, M., Clemente-Moreno, M. J., Coopman, R. E., Fernie, A. R., et al. (2019). Photosynthesis optimized across land plant phylogeny. Trends Plant Sci. 24, 948–957. doi: 10.1016/j.tplants.2019.07.002
Gago, J., Daloso, D. M., Figueroa, C. M., Flexas, J., Fernie, A. R., and Nikoloski, Z. (2016). Relationships of leaf net photosynthesis, stomatal conductance, and mesophyll conductance to primary metabolism: a multispecies meta-analysis approach. Plant Physiol. 171, 265–279. doi: 10.1104/pp.15.01660
Gallé, A., and Flexas, J. (2010). “Gas-Exchange and Chlorophyll Fluorescence Measurements in Grapevine Leaves in the Field,” in Methodologies and Results in Grapevine Research, eds S. Delrot, H. Medrano, E. Or, L. Bavaresco, and S. Grando (Dordrecht: Springer), doi: 10.1007/978-90-481-9283-0_8
Gibbs, J., and Greenway, H. (2003). Mechanisms of anoxia tolerance in plants. I. Growth, survival and anaerobic catabolism. Funct. Plant Biol. 30, 1–47. doi: 10.1071/PP98095
Gill, S. S., and Tuteja, N. (2010). Reactive oxygen species and antioxidant machinery in abiotic stress tolerance in crop plants. Plant Physiol. Biochem. 48, 909–930. doi: 10.1016/j.plaphy.2010.08.016
Green, M. A., and Fry, S. C. (2005). Vitamin C degradation in plant cells via enzymatic hydrolysis of 4-O-oxalyl-L-threonate. Nature 433, 83–87. doi: 10.1038/nature03172
Grime, J. P., and Hunt, R. (1975). Relative growth-rate: its range and adaptive significance in a local flora. J. Ecol. 63, 393–422. doi: 10.2307/2258728
Gromski, P. S., Xu, Y., Kotze, H. L., Correa, E., Ellis, D. I., Armitage, E. G., et al. (2014). Influence of missing values substitutes on multivariate analysis of metabolomics data. Metabolites 4, 433–452. doi: 10.3390/metabo4020433
Guerrier, G., Brignolas, F., Thierry, C., Courtois, M., and Kahlem, G. (2000). Organic solutes protect drought-tolerant Populus× euramericana against reactive oxygen species. J. Plant Physiol. 156, 93–99. doi: 10.1016/S0176-1617(00)80277-1
Hancock, R. D., and Viola, R. (2005). Biosynthesis and catabolism of L-ascorbic acid in plants. Crit. Rev. Plant Sci. 24, 167–188. doi: 10.1080/07352680591002165
Harholt, J., Moestrup, O., and Ulvskov, P. (2016). Why Plants Were Terrestrial from the Beginning. Trends Plant Sci. 21, 96–101. doi: 10.1016/j.tplants.2015.11.010
Hilman, B., and Angert, A. (2016). Measuring the ratio of CO2 efflux to O2 influx in tree stem respiration. Tree Physiol. 36, 1422–1431. doi: 10.1093/treephys/tpw057
Hossain, M. A., Munné-Bosch, S., Burritt, D. J., Diaz-Vivancos, P., Fujita, M., and Lorence, A. (2017). Ascorbic acid in plant growth, development and stress tolerance. Berlin: Springer, doi: 10.1007/978-3-319-74057-7
Huang, W., Han, S., Xing, Z., and Li, W. (2020). Responses of leaf anatomy and CO2 concentrating mechanisms of the aquatic plant Ottelia cordata to variable CO2. Front. Plant Sci. 11:1261. doi: 10.3389/fpls.2020.01261
Ivanova, A., Law, S. R., Narsai, R., Duncan, O., Lee, J. H., Zhang, B., et al. (2014). A functional antagonistic relationship between auxin and mitochondrial retrograde signaling regulates alternative oxidase1a expression in Arabidopsis. Plant Physiol. 165, 1233–1254. doi: 10.1104/pp.114.237495
Jackson, M. B. (2008). Ethylene-promoted elongation: an adaptation to submergence stress. Ann. Bot. 101, 229–248. doi: 10.1093/aob/mcm237
Jouve, L., Hoffmann, L., and Hausman, J. F. (2004). Polyamine, carbohydrate, and proline content changes during salt stress exposure of aspen (Populus tremula L.): involvement of oxidation and osmoregulation metabolism. Plant Biol. 7, 74–80. doi: 10.1055/s-2003-44687
Keeley, J. E., and Santamaría, L. (1992). Carbon: freshwater plants. Plant Cell Environ. 15, 1021–1035. doi: 10.1111/j.1365-3040.1992.tb01653.x
Kenrick, P., and Crane, P. R. (1997). The origin and early evolution of plants on land. Nature 389, 33. doi: 10.1038/37918
Kenrick, P., Wellman, C. H., Schneider, H., and Edgecombe, G. D. (2012). A timeline for terrestrialization: consequences for the carbon cycle in the Palaeozoic. Philos. Trans. R. Soc. B: Biol. Scie. 367, 519–536. doi: 10.1098/rstb.2011.0271
Kim, J., Joo, Y., Kyung, J., Jeon, M., Park, J. Y., Lee, H. G., et al. (2018). A molecular basis behind heterophylly in an amphibious plant, Ranunculus trichophyllus. PLoS genetics. 14:e1007208. doi: 10.1371/journal.pgen.1007208
Kinnersley, A. M., and Turano, F. J. (2000). Gamma aminobutyric acid (GABA) and plant responses to stress. Crit. Rev. Plant Sci. 19, 479–509. doi: 10.1080/07352680091139277
Koga, H., Doll, Y., Hashimoto, K., Toyooka, K., and Tsukaya, H. (2020). Dimorphic Leaf Development of the Aquatic Plant Callitriche palustris L. Through Differential Cell Division and Expansion. Front. Plant Sci. 11:269. doi: 10.3389/fpls.2020.00269
Kopka, J., Schauer, N., Krueger, S., Birkemeyer, C., Usadel, B., Bergmüller, E., et al. (2005). GMD@ CSB. DB: the Golm metabolome database. Bioinformatics. 21, 1635–1638. doi: 10.1093/bioinformatics/bti236
Lambers, H., Chapin, F. S., and Pons, T. L. (2008). Plant Physiological Ecology. New York, NY: Springer-Verlag, 10–95.
Li, G., Hu, S., Hou, H., and Kimura, S. (2019). Heterophylly: phenotypic plasticity of leaf shape in aquatic and amphibious plants. Plants. 8, 420. doi: 10.3390/plants8100420
Li, G., Hu, S., Zhao, X., Kumar, S., Li, Y., Yang, J., et al. (2021). Mechanisms of the Morphological Plasticity Induced by Phytohormones and the Environment in Plants. Int. J. Mol. Sci. 22, 765. doi: 10.3390/ijms22020765
Lim, S. L., Voon, C. P., Guan, X., Yang, Y., Gardeström, P., and Lim, B. L. (2020). In planta study of photosynthesis and photorespiration using NADPH and NADH/NAD+ fluorescent protein sensors. Nature communications. 11, 1–12. doi: 10.1038/s41467-020-17056-0
Lisec, J., Schauer, N., Kopka, J., Willmitzer, L., and Fernie, A. R. (2006). Gas chromatography mass spectrometry-based metabolite profiling in plants. Nature Protoc. 1, 387–396. doi: 10.1038/nprot.2006.59
Liska, A. J., Shevchenko, A., Pick, U., and Katz, A. (2004). Enhanced photosynthesis and redox energy production contribute to salinity tolerance in Dunaliella as revealed by homology-based proteomics. Plant Physiol. 136, 2806–2817. doi: 10.1104/pp.104.039438
Lowry, B., Hebant, C., and Lee, D. (1980). The origin of land plants -a new look at an old problem. Taxon 29, 183–197. doi: 10.2307/1220280
Lunn, J. E., Feil, R., Hendriks, J. H. M., Gibon, Y., Morcuende, R., Osuna, D., et al. (2006). Sugar-induced increases in trehalose 6-phosphate are correlated with redox activation of ADP glucose pyrophosphorylase and higher rates of starch synthesis in Arabidopsis thaliana. Biochem. J. 397, 139–148. doi: 10.1042/BJ20060083
Lusk, C. H., and Reich, P. B. (2000). Relationships of leaf dark respiration with light environment and tissue nitrogen content in juveniles of 11 cold-temperate tree species. Oecologia 123, 318–329.
Maberly, S. C. (2014). The fitness of the environments of air and water for photosynthesis, growth, reproduction and dispersal of photoautotrophs: an evolutionary and biogeochemical perspective. Aquat. Bot. 118, 4–13. doi: 10.1016/j.aquabot.2014.06.014
Maberly, S. C., and Madsen, T. V. (2002). Aquatic freshwater angiosperm carbon concentrating mechanisms: processes and patterns. Funct. Plant Biol. 29, 393–405. doi: 10.1071/PP01187
Maberly, S. C., and Spence, D. H. N. (1989). Photosynthesis and photorespiration in freshwater organisms: amphibious plants. Aquat. Bot. 34, 267–286.
Marsh, K. B., Boldingh, H. L., Shilton, R. S., and Laing, W. A. (2009). Changes in quinic acid metabolism during fruit development in three kiwifruit species. Funct. Plant Biol. 36, 463–470. doi: 10.1071/FP08240
McDonald, A. E., and Vanlerberghe, G. C. (2006). Origins, evolutionary history, and taxonomic distribution of alternative oxidase and plastoquinol terminal oxidase. Comp. Biochem. Physiol. Part D: Genom. Proteom. 1, 357–364. doi: 10.1016/j.cbd.2006.08.001
Meng, F., Cao, R., Yang, D., Niklas, K. J., and Sun, S. (2014). Trade-offs between light interception and leaf water shedding: a comparison of shade- and sun-adapted species in a subtropical rainforest. Oecologia. 174, 13–22. doi: 10.1007/s00442-013-2746-0
Michaeli, S., Fait, A., Lagor, K., Nunes−Nesi, A., Grillich, N., Yellin, A., et al. (2011). A mitochondrial GABA permease connects the GABA shunt and the TCA cycle, and is essential for normal carbon metabolism. Plant J. 67, 485–498. doi: 10.1111/j.1365-313X.2011.04612.x
Millar, A. H., Mittova, V., Kiddle, G., Heazlewood, J. L., Bartoli, C. G., Theodoulou, F. L., et al. (2003). Control of ascorbate synthesis by respiration and its implications for stress responses. Plant Physiol. 133, 443–447. doi: 10.1104/pp.103.028399
Millenaar, F. F., Gonzalez-Meler, M. A., Fiorani, F., Welschen, R., Ribas-Carbó, M., Siedow, J. N., et al. (2001). Regulation of alternative oxidase activity in six wild monocotyledonous species. An in vivo study at the whole root level. Plant Physiol. 126, 376–387. doi: 10.1104/pp.126.1.376
Mommer, L., de Kroon, H., Pierik, R., Bgöemann, G. M., and Visser, E. J. W. (2005). A functional comparison of acclimation to shade and submergence in two terrestrial plant species. New Phytol. 167, 197–206. doi: 10.1111/j.1469-8137.2005.01404.x
Moore, A. L., Albury, M. S., Crichton, P. G., and Affourtit, C. (2002). Function of the alternative oxidase: is it still a scavenger? Trends Plant Sci. 7, 478–481. doi: 10.1016/S1360-1385(02)02366-X
Morris, J. L., Puttick, M. N., Clark, J. W., Edwards, D., Kenrick, P., Pressel, S., et al. (2018). The timescale of early land plant evolution. PNAS. 115, 2274–2283. doi: 10.1073/pnas.1719588115
Muscolo, A., Junker, A., Klukas, C., Weigelt-Fischer, K., Riewe, D., and Altmann, T. (2015). Phenotypic and metabolic responses to drought and salinity of four contrasting lentil accessions. J. Exp. Bot. 66, 5467–5480. doi: 10.1093/jxb/erv208
Nakayama, H., Nakayama, N., Nakamasu, A., Sinha, N., and Kimura, S. (2012). Toward elucidating the mechanisms that regulate heterophylly. Plant Morphology. 24, 57–63. doi: 10.5685/plmorphol.24.57
Nakayama, H., Nakayama, N., Seiki, S., Kojima, M., Sakakibara, H., Sinha, N., et al. (2014). Regulation of the KNOX-GA gene module induces heterophyllic alteration in North American lake cress. The Plant Cell. 26, 4733–4748. doi: 10.1105/tpc.114.130229
Nakayama, H., Sinha, N. R., and Kimura, S. (2017). How do plants and phytohormones accomplish heterophylly, leaf phenotypic plasticity, in response to environmental cues. Front. Plant. Sci. 8:1717. doi: 10.3389/fpls.2017.01717Nasrulhaq
Neimanis, K., Staples, J. F., Hüner, N. P., and McDonald, A. E. (2013). Identification, expression, and taxonomic distribution of alternative oxidases in non-angiosperm plants. Gene. 526, 275–286. doi: 10.1016/j.gene.2013.04.072
Niggeweg, R., Michael, A. J., and Martin, C. (2004). Engineering plants with increased levels of the antioxidant chlorogenic acid. Nature Biotechnol. 22, 746–754. doi: 10.1038/nbt966
Niyogi, K. K. (1999). Photoprotection revisited: genetic and molecular approaches. Annu. Rev. Plant Physiol. Plant Mol. Biol. 50, 333–359. doi: 10.1146/annurev.arplant.50.1.333
Noguchi, K., Go, C. S., Terashima, I., Ueda, S., and Yoshinari, T. (2001). Activities of the cyanide-resistant respiratory pathway in leaves of sun and shade species. Aust. J. Plant Physiol. 28, 27–35. doi: 10.1071/PP00056
Noguchi, K., and Yoshida, K. (2008). Interaction between photosynthesis and respiration in illuminated leaves. Mitochondrion. 8, 87–99. doi: 10.1016/j.mito.2007.09.003
O’Leary, B. M., Asao, S., Millar, A. H., and Atkin, O. (2020). Core principles which explain variation in respiration across biological scales. New Phytol. 222, 670–686. doi: 10.1111/nph.15576
Paul, M. J., Jhurreea, D., Zhang, Y., Primavesi, L. F., Delatte, T., Schluepmann, H., et al. (2010). Up-regulation of biosynthetic processes associated with growth by trehalose 6-phosphate. Plant Signal. Behav. 5, 1–7. doi: 10.4161/psb.5.4.10792
Pires, N. D., and Dolan, L. (2012). Morphological evolution in land plants: new designs with old genes. Philosophical Transactions of the Royal Society B: Biological Sciences. 367, 508–518. doi: 10.1098/rstb.2011.0252
Proctor, M. C. F. (2014). “The Diversification of Bryophytes and Vascular Plants in Evolving Terrestrial Environments,” in Photosynthesis in Bryophytes and Early Land Plants. Advances in Photosynthesis and Respiration (Including Bioenergy and Related Processes), Vol. 37, eds D. Hanson and S. Rice (Dordrecht: Springer), doi: 10.1007/978-94-007-6988-5_4
Raghavendra, A. S., and Padmasree, K. (2003). Beneficial interactions of mitochondrial metabolism with photosynthetic carbon assimilation. Trends Plant Sci. 8, 546–553. doi: 10.1016/j.tplants.2003.09.015
Ramesh, S. A., Kamran, M., Sullivan, W., Chirkova, L., Okamoto, M., Degryse, F., et al. (2018). Aluminum-activated malate transporters can facilitate GABA transport. Plant Cell. 30, 1147–1164. doi: 10.1105/tpc.17.00864
Rascio, N. (2002). The underwater life of secondarily aquatic plants: some problems and solutions. Crit. Rev. Plant Sci. 21, 401–427. doi: 10.1080/0735-260291044296
Raven, J. A. (2002). Selection pressures on stomatal evolution. New Phytol. 153, 371–386. doi: 10.1046/j.0028-646X.2001.00334.x
Reich, P. B., Walters, M. B., Tjoelker, M. G., Vanderklein, D., and Buschena, C. (1998). Photosynthesis and respiration rates depend on leaf and root morphology and nitrogen concentration in nine boreal tree species differing in relative growth rate. Funct. Ecol. 12, 395–405. doi: 10.1046/j.1365-2435.1998.00209.x
Reski, R. (2018). Enabling the water-to-land transition. Nat. Plant. 4, 67–68. doi: 10.1038/s41477-018-0101-5
Ribas-Carbó, M., Robinson, S. A., and Giles, L. (2005). “The application of the oxygen isotope technique to assess respiratory pathway partitioning,” in Plant Respiration: From Cell to Ecosystem, vol 18. Advances in Photosynthesis and Respiration Series, Chap. 3, eds H. Lambers Ribas-Carb (Dordrecht: Springer), 31–42. doi: 10.1007/1-4020-3589-6_3
Robe, W. E., and Griffiths, H. (2000). Physiological and photosynthetic plasticity in the amphibious, freshwater plant, Littorella uniflora, during the transition from aquatic to dry terrestrial environments. Plant Cell Environ. 23, 1041–1054. doi: 10.1046/j.1365-3040.2000.00615.x
Saccenti, E., Hoefsloot, H. C., Smilde, A. K., Westerhuis, J. A., and Hendriks, M. M. (2014). Reflections on univariate and multivariate analysis of metabolomics data. Metabolomics. 10, 361–374. doi: 10.1007/s11306-013-0598-6
Schauer, N., Steinhauser, D., Strelkov, S., Schomburg, D., Allison, G., Moritz, T., et al. (2005). GC-MS libraries for the rapid identification of metabolites in complex biological samples. FEBS letters. 579, 1332–1337. doi: 10.1016/j.febslet.2005.01.029
Scheibe, R. (2004). Malate valves to balance cellular energy supply. Physiol. Plant. 120, 21–26. doi: 10.1111/j.0031-9317.2004.0222.x
Schlesinger, W. H., and Bernhardt, S. B. (2020). “Wetland Ecosystems,” in Biogeochemistry, Fourth Edition, Chap. 7, eds W. H. Schlesinger and S. B. Bernhardt (Cambridge, Ma: Academic Press), 249–291. doi: 10.1016/C2017-0-00311-7
Schluepmann, H., van Dijken, A., Aghdasi, M., Wobbes, B., Paul, M., and Smeekens, S. (2004). Trehalose mediated growth inhibition of Arabidopsis seedlings is due to trehalose-6-phosphate accumulation. Plant Physiol. 135, 879–890. doi: 10.1104/pp.104.039503
Selinski, J., Scheibe, R., Day, D. A., and Whelan, J. (2018). Alternative oxidase is positive for plant performance. Trends Plant Sci. 23, 588–597. doi: 10.1016/j.tplants.2018.03.012
Shelp, B. J., Mullen, R. T., and Waller, J. C. (2012). Compartmentation of GABA metabolism raises intriguing questions. Trends Plant Sci. 17, 57–59. doi: 10.1016/j.tplants.2011.12.006
Smirnoff, N. (2018). Ascorbic acid metabolism and functions: A comparison of plants and mammals. Free Rad. Biol. Med. 122, 116–129. doi: 10.1016/j.freeradbiomed.2018.03.033
Smirnoff, N., and Wheeler, G. L. (2000). Ascorbic acid in plants: biosynthesis and function. Crit. Rev. Plant Sci. 19, 267–290. doi: 10.1080/07352680091139231
Studart-Guimarães, C., Fait, A., Nunes-Nesi, A., Carrari, F., Usadel, B., and Fernie, A. R. (2007). Reduced expression of succinyl-coenzyme A ligase can be compensated for by up-regulation of the γ-aminobutyrate shunt in illuminated tomato leaves. Plant Physiol. 145, 626–639. doi: 10.1104/pp.107.103101
Tamagnone, L., Merida, A., Stacey, N., Plaskitt, K., Parr, A., Chang, C. F., et al. (1998). Inhibition of phenolic acid metabolism results in precocious cell death and altered cell morphology in leaves of transgenic tobacco plants. Plant Cell. 10, 1801–1816. doi: 10.1105/tpc.10.11.1801
Thomas, D. J., Boling, J., Crowell, C. M., Eubanks, L. M., McCarthy, N., McSpadden, T., et al. (2008). A test of the oxygen paradox using antioxidant-deficient cyanobacteria. Gravitation Space Biol. 21, 27–28.
Timm, S., and Hagemann, M. (2020). Photorespiration-how is it regulated and how does it regulate overall plant metabolism? J. Exp. Bot. 71, 3955–3965. doi: 10.1093/jxb/eraa183
Valladares, F., and Niinemets, U. (2007). “The architecture of plant crowns: from design rules to light capture and performance,” in Functional plant ecology, eds F. I. Pugnaire and F. Valladares (New York: Taylor and Francis), 101–150. doi: 10.1201/9781420007626.ch4
Vanlerberghe, G. C., Dahal, K., Alber, N. A., and Chadee, A. (2020). Photosynthesis, respiration and growth: A carbon and energy balancing act for alternative oxidase. Mitochondrion 52, 197–211. doi: 10.1016/j.mito.2020.04.001
Vermeij, G. J. (2016). Plant defenses on land and in water: why are they so different? Ann Bot. 117, 1099–1109. doi: 10.1093/aob/mcw061
Watanabe, C. K., Yamori, W., Takahashi, S., Terashima, I., and Noguchi, K. (2016). Mitochondrial Alternative Pathway-Associated Photoprotection of Photosystem II is Related to the Photorespiratory Pathway. Plant Cell Physiol. 57, 1426–1431. doi: 10.1093/pcp/pcw036
Waters, E. R. (2003). Molecular adaptation and the origin of land plants. Mol. Phylogenet. Evol. 29, 456–463. doi: 10.1016/j.ympev.2003.07.018
Wehrens, R., and Mevik, B. H. (2007). The pls package: principal component and partial least squares regression in R. J. Statist Softw. 18, 1–23.
Weng, J. K., and Chapple, C. (2010). The origin and evolution of lignin biosynthesis. New Phytol. 187, 273–285. doi: 10.1111/j.1469-8137.2010.03327.x
Whittaker, R. H. (1970). Communities and Ecosystems. 2nd Revised Edition. New York: Macmillan Publishing Co.
Wright, I. J., Reich, P. B., Westoby, M., Ackerly, D. D., Baruch, Z., Bongers, F., et al. (2004). The Worldwide Leaf Economics Spectrum. Nature. 428, 821–827. doi: 10.1038/nature02403
Yang, R., Hui, Q., and Gu, Z. (2016). Effects of ABA and CaCl2 on GABA accumulation in fava bean germinating under hypoxia-NaCl stress. Biosc. Biotechnol. Biochem. 80, 540–546. doi: 10.1080/09168451.2015.1116923
Yang, Y., Guan, H., Batelaan, O., McVicar, T. R., Long, D., Piao, S., et al. (2016). Contrasting responses of water use efficiency to drought across global terrestrial ecosystems. Sci. Rep. 6, 23284. doi: 10.1038/srep23284
Yoon, H. S., Hackett, J. D., Ciniglia, C., Pinto, G., and Bhattacharya, D. (2004). A molecular timeline for the origin of photosynthetic eukaryotes. Mol. Biol. Evol. 21, 809–818. doi: 10.1093/molbev/msh075
Zandalinas, I., Fritschi, B., and Mittler, R. (2021). Global Warming, Climate Change, and Environmental Pollution: Recipe for a Multifactorial Stress Combination Disaster. Trends Plant Sci. 2021, 11. doi: 10.1016/j.tplants.2021.02.011
Keywords: alternative oxidase pathway (AOP), cytochrome oxidase pathway (COP), electron partitioning to the AOP (τa), primary metabolism, terrestrial species, palustrine species, heterophylly
Citation: Del-Saz NF, Douthe C, Carriquí M, Ortíz J, Sanhueza C, Rivas-Medina A, McDonald A, Fernie AR, Ribas-Carbo M, Gago J, Florez-Sarasa I and Flexas J (2021) Different Metabolic Roles for Alternative Oxidase in Leaves of Palustrine and Terrestrial Species. Front. Plant Sci. 12:752795. doi: 10.3389/fpls.2021.752795
Received: 03 August 2021; Accepted: 15 October 2021;
Published: 04 November 2021.
Edited by:
Donald R. Ort, University of Illinois at Urbana-Champaign, United StatesReviewed by:
Xinguang Zhu, University of Chinese Academy of Sciences (UCAS), ChinaMarco Zancani, University of Udine, Italy
Copyright © 2021 Del-Saz, Douthe, Carriquí, Ortíz, Sanhueza, Rivas-Medina, McDonald, Fernie, Ribas-Carbo, Gago, Florez-Sarasa and Flexas. This is an open-access article distributed under the terms of the Creative Commons Attribution License (CC BY). The use, distribution or reproduction in other forums is permitted, provided the original author(s) and the copyright owner(s) are credited and that the original publication in this journal is cited, in accordance with accepted academic practice. No use, distribution or reproduction is permitted which does not comply with these terms.
*Correspondence: Nestor Fernandez Del-Saz, bmVzZmVybmFuZGV6QHVkZWMuY2w=; Jaume Flexas, amF1bWUuZmxleGFzQHVpYi5lcw==