- Departamento de Bioquímica y Biología Molecular, Facultad de Farmacia, Universidad de Sevilla, Seville, Spain
Ozone is a destructive pollutant, damaging crops, and decreasing crop yield. Therefore, there is great interest in finding strategies to alleviate ozone-induced crop losses. In plants, ozone enters leaves through the stomata and is immediately degraded into reactive oxygen species (ROS), producing ROS stress in plants. ROS stress can be controlled by ROS-scavenging systems that include enzymatic or non-enzymatic mechanisms. Our research group has developed a product from rice bran, a by-product of rice milling which has bioactive molecules that act as an antioxidant compound. This product is a water-soluble rice bran enzymatic extract (RBEE) which preserves all the properties and improves the solubility of proteins and the antioxidant components of rice bran. In previous works, the beneficial properties of RBEE have been demonstrated in animals. However, to date, RBEE has not been used as a protective agent against oxidative damage in agricultural fields. The main goal of this study was to investigate the ability of RBEE to be used as a biostimulant by preventing oxidative damage in plants, after ozone exposure. To perform this investigation, pepper plants (Capsicum annuum) exposed to ozone were treated with RBEE. RBEE protected the ozone-induced damage, as revealed by net photosynthetic rate and the content of photosynthetic pigments. RBEE also decreased the induction of antioxidant enzyme activities in leaves (catalase, superoxide dismutase, and ascorbate peroxidase) due to ozone exposure. ROS generation is a common consequence of diverse cellular traumas that also activate the mitogen-activated protein kinase (MAPK) cascade. Thus, it is known that the ozone damages are triggered by the MAPK cascade. To examine the involvement of the MAPK cascade in the ozone damage CaMPK6-1, CaMPK6-2, and CaMKK5 genes were analyzed by qRT-PCR. The results showed the involvement of the MAPK pathway in both, not only in ozone damage but especially in its protection by RBEE. Taken together, these results support that RBEE protects plants against ozone exposure and its use as a new biostimulant could be proposed.
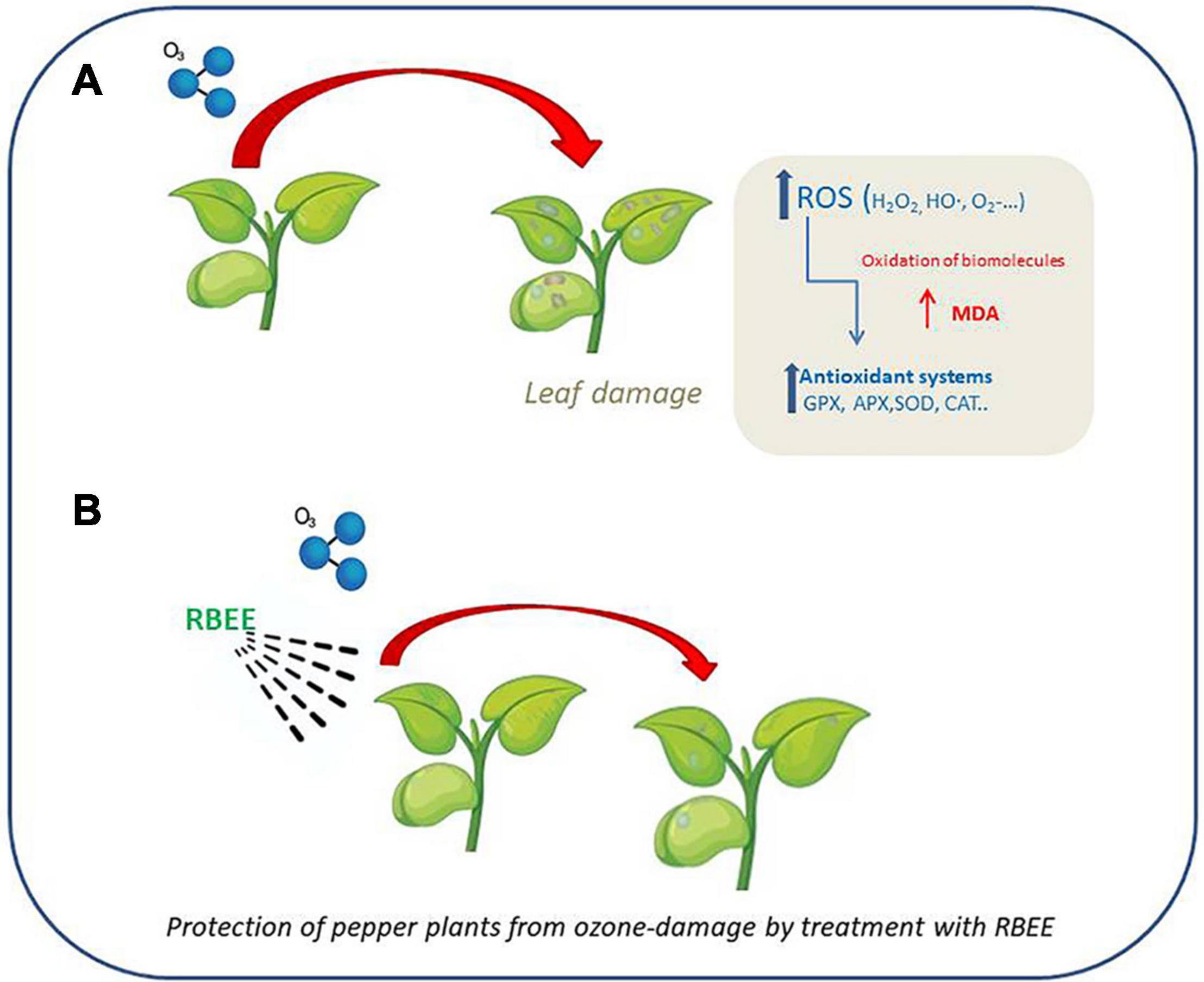
Graphical Abstract. Explanatory diagram of the protective action of RBEE against the oxidizing agent ozone.
Introduction
Ozone (O3) is a destructive pollutant with negative effects on human and ecosystem health that induces abiotic stress in plants – affecting photosynthetic carbon assimilation, stomatal conductance, and plant growth – that damages crops and decreases crop yield (Ainsworth et al., 2012).
In plants, O3 enters through the stomata and is degraded into secondary reactive oxygen species (ROS), including H2O2, O2⋅–, and HO⋅, in the apoplastic space (Vainonen and Kangasjärvi, 2015). High levels of ROS can lead to ROS stress that causes direct or indirect ROS-mediated damage on a variety of molecules including lipid peroxidation in cellular membranes, protein denaturation, carbohydrate oxidation, and pigment breakdown (Sharma et al., 2012). Even more, ROS stress can eventually lead to changes in gene expression and even cell death. However, ROS are generated in the metabolism of plant cells and therefore plants need systems that scavenge oxidizing species. Constitutive enzymatic and non-enzymatic systems in cells protect them from ROS-induced damage and are inducible under biotic and abiotic stress that triggers a rapid increase in ROS, including O3 exposure (Sachdev et al., 2021). Enzymatic ROS-scavenging mechanisms include antioxidant enzymes such as superoxide dismutase (SOD), catalase (CAT), ascorbate peroxidase (APX), glutathione peroxidase (GP), guaiacol peroxidase (GPX) and dehydroascorbate reductase (DHAR) (Gill and Tuteja, 2010).
Specific ROS also act as “second messengers” in signal transduction pathways that lie downstream initial event in cells. The mitogen-activated protein kinase (MAPK) cascade is one of the signaling pathways sensitive to the cell’s redox status. Therefore ROS generation is a common consequence of diverse cellular traumas that activate the MAPK cascade and studies in different plants have demonstrated that O3 exposure activates some components of the MAPK cascade (Marcus et al., 2000; Liu et al., 2015; Liu and He, 2017).
Studies on the effect of O3 on agricultural productivity showed an estimated 5–15% crop yield loss in the United States (Avnery et al., 2011) and a 5% in Europe (Mills et al., 2007). To alleviate the drop in crop productivity, the main defense strategy over the past four decades has been the application of chemicals, mainly synthetic antioxidants (fungicides, insecticides, herbicides, nematocides, growth regulators, antitranspirants, antioxidants from the rubber industry, etc.) (Didyk and Blum, 2010). These xenobiotic products have varied protection capacity with some producing ineffective or even detrimental side effects. The most effective product was found to be EDU (ethylene diurea– (N-[2-(2-oxo-1-imidazolidinyl) ethyl]-N0 phenylurea), developed by the duPont Chemical company (Archambault et al., 2000) whose main mechanism of action is not yet clear. Currently, more effective alternatives are being sought, such as extracts of plant origin, which do not generate environmental toxicity.
Rice Bran is a by-product of rice (Oryza sativa) milling that retains most of the bioactive compounds present in rice grain. These bioactives include naturally occurring antioxidants, mainly γ-oryzanol, tocopherols, tocotrienols, and polyphenols. Besides that, rice bran is also a good source of protein and fat (Goufo and Trindade, 2014). However, the low bioavailability of its biopolymers and bioactive compounds due to its high insolubility is the main drawback when considering rice bran as a biostimulant. Using enzymatic technology, our group has developed a process that enables obtaining a stable, water-soluble rice bran enzymatic extract (RBEE) from rice bran. RBEE has shown a high content in bioactive compounds, particularly phytosterols, γ–oryzanol, and tocols, and an increased content in peptides and free amino acids (Santa-María et al., 2010).
According to its content in bioactive compounds, RBEE has shown both in vivo and in vitro functional properties such as antioxidant capacity (Santa-María et al., 2010), hypocholesterolemic activity (Revilla et al., 2009), and antiproliferative and inmunoactivatory abilities (Revilla et al., 2013). Beneficial effects found in cells and animal models, especially those derived from its antioxidant capacity, lead us to consider the use of RBEE as a biostimulant in agriculture.
This work aims to evaluate the biostimulant capacity of RBEE. To this end, we propose studying the role of RBEE in plant protection against abiotic stress mediated by ROS, specifically in O3 exposure. We chose pepper plants (Capsicum annuum) since pepper is a vegetable crop of great agricultural and economic importance being the second most traded spice in the world. Heavy losses in pepper production are frequently caused by abiotic stresses including O3 exposition, in fact Capsicum pepper cultivation is almost entirely located in regions where O3 concentration is increasing to phytotoxic levels (Bortolin et al., 2016). For these reasons pepper plants are an interesting research target to evaluate the negative effects of O3.
Pepper plants exposed to O3 were treated with RBEE and antioxidant enzyme activities and specific functional parameters were analyzed. Treatment with RBEE improved physiological parameters, such as net photosynthetic rate and photosynthetic pigments content in plants exposed to with O3. RBEE treatment also decreased the induction of antioxidant enzyme activities in leaves (CAT, SOD, GPX, and APX) due to O3 exposure.
Additionally, to search whether MAPK cascade is involved in the protective role of RBEE against O3-induced damage we analyzed the transcriptional expression of CaMPK6-1, CaMPK6-2, and CaMKK5 genes. The high expression of genes studied strongly support that RBEE protective capacity involved the MAPK signaling cascade.
Materials and Methods
Rice Bran Enzymatic Extract Preparation
Rice bran (O. sativa var. indica) raw material was provided by Herba Ricemills, S.L.U (Sevilla, Spain). Rice bran is obtained during the polishing/milling of raw rice grains once their husks have been stripped. Rice bran was processed by enzymatic hydrolysis using the hydrolytic agent (Biocom, Spain) subtilisin (EC 3.4.21.62), a protease from Bacillus licheniformis as hydrolytic agent (Biocom, Spain) in a bioreactor with controlled temperature (60°C) and pH (pH 8), using the pH-stat method (Parrado et al., 2006). The processing of this product follows different steps, including solid separation and concentration. The final product RBEE is a brown syrup that is completely water-soluble.
Rice bran enzymatic extract bran macro and micronutrient composition was characterized as previously described (Parrado et al., 2006).
Plant Treatment
Capsicum annum L. var. grossum (pepper) plants were raised from seeds in plastic pots containing an organic commercial substrate (Gramoflor GmbH und Co., KG.) and Osmocote® (NPK 15-9-12), and grown inside the University of Seville Glasshouse General Services on a phytoclimatic chamber, with a controlled temperature of 18–22°C, 50% relative humidity, adequate irrigation with tap water and a photoperiod of 16 h light (1200 μmol.m–2. s–1)/8 h darkness. After 8 days of transplantation, 20 pepper plants were selected and divided in 4 treatments (5 plants per treatment): control plants (C), plants treated with RBEE, control plants under O3 exposition (C + O3), and plants treated with RBEE under O3 exposition (RBEE + O3).
To evaluate the protection capacity of the treatment with RBEE, RBEE and RBEE + O3 plants were foliar sprayed with an aqueous solution of RBEE at 0.1% a total of four times at 5-day intervals. At the same time, control plants (C and C + O3) were sprayed with distilled water the same times. After 5 days of the last spray treatment, C + O3 and RBEE + O3 plants were transferred to another phytoclimatic chamber with an O3 generator (ZONOSISTEM GM 5000 O3 Generator) attached and exposed to three consecutive fumigations with 100 ppB of O3 for 6 h (from 10:00 a.m. to 4:00 p.m.). After O3 fumigation, plants of all treatments were sprayed again with the corresponding solution (RBEE 0.1% or distilled water).
Finally, 24 h after the last exposure to O3, foliar samples were taken from each plant and the analyses described below were carried out.
Physiological Status in Plants
Determination of Net Photosynthetic Rate
Twenty-four hours after the las ozone treatment, the net photosynthetic rate (AN) was measured in plants using an IRGA (LI-6400XT, LI-COR Inc., Nev., EEUU) with a light chamber for the leaf (Li-6400-02B, Li-Cor Inc.). Measurements (n = 20) were performed between 10 a.m. and 2 p.m. hours under a photosynthetic photon flux density of 1500 μmol.m–2.s–1, a deficit of vapor pressure of 2–3 kPa, a temperature around 25°C, and a CO2 concentration environment of 400 μmol.mol–1 air. Each measurement was recorded after the stabilization of the exchange of gases was equilibrated (120 s).
Chlorophyll Content in Leaves
Chlorophyll content was extracted from random leaves from plants of each treatment. Fifty milligrams of leaves were homogenized in acetone 100% (v/v) and saline solution 0.9% (w/v) using a homogenizer (Hiscox and Israelstam, 1979). The total chlorophyll content was determined at 652 nm by using the absorbance coefficient of extinction 34.5 cm–1⋅ μg–1 (Arnon, 1949):
Delayed Fluorescence Measurements
Delayed fluorescence (DF) was detected using a plant imaging system (NightShade LB 985, Berthold Technologies, Germany) equipped with a deeply cooled CCD camera according to López-Jurado et al. (2020). From plants of each treatment, 2–3 intact leaves of approximately the same size were separated and placed in the plant imaging system. The leaves were illuminated for 20 s with light supplied from far red (730 nm), red (660 nm), green (565 nm), and blue (470 nm) LED panels at 2, 105, 40, and 110 μmol.m–2.s–1, respectively. Immediately after the LEDs were turned off, DF was measured, and the recorded intensities of light were converted to counts per second (cps). Data were then normalized to each leaf area to obtain comparable cps values across treatments.
Oxidative Stress Evaluation in Plants
Determination of Lipid Peroxidation
To quantify lipid peroxidation in leaf homogenates malondialdehyde (MDA) content was determined using the thiobarbituric acid reactive substances (TBARS) assay (Esterbauer and Cheeseman, 1990). Samples of 1 g of leaves were homogenized with 2 ml of 0.1% trichloroacetic acid (TCA). The homogenates were centrifuged at 8000 × g for 10 min at 4°C, and the supernatants were filtered through a 0.2 mm aseptic filter. Then, 0.3 ml of each sample was mixed with 0.9 ml of 20% TCA containing 0.5% TBA. The solutions were heated at 95°C for 1 h, immediately cooled, and centrifuged as above. Finally, the absorbance at 532 and 600 nm of the supernatants was recorded, and MDA concentration was calculated by subtracting the non-specific absorption at 600 nm from the absorption at 532 nm by using the absorbance coefficient of extinction 156 mM–1 cm–1. The results obtained were expressed in nmol.g–1 fresh weight (FW).
Antioxidant Enzymes Analyses
To observe the stress of plants, enzymatic activities of APX, SOD, guaiacol peroxidase (GPX), and CAT were measured as described by Duarte et al. (2015). Briefly, vegetal extract was extracted in extraction buffer (50 mM sodium phosphate buffer; pH 7.6) from 500 mg of leaves. Catalase activity was determined at 240 nm in a reaction solution containing the assay buffer (50 mM sodium phosphate buffer, pH 7.0) and 100 mM H2O2. APX activity was assayed in the assay buffer with 12 mM H2O2 and 0.25 mM L-ascorbate and measured at 290 nm. SOD activity was determined by monitorization of the pyrogallol oxidation at 325 nm by the addition of 3 mM pyrogallol. Guaiacol peroxidase activity was measured at 470 nm in a reaction mixture containing the assay buffer, 2 mM H2O2, and 20 mM guaiacol. To determine the auto-oxidation of the substrates, control assays were performed in absence of enzymatic extract samples (Duarte et al., 2015). Finally, the total protein content in the enzymatic extracts was measured according to Bradford (1976).
Oxidative Stress Index
Oxidative stress index (OSI) is a parameter that expresses the global oxidative stress in plants (Pérez-Palacios et al., 2017). It was calculated with the formula described in Paredes-Páliz et al. (2018). Values greater than 1 indicated that the leaves were stressed, whereas values less than 1 indicated that the leaves were without oxidative stress.
Plant RNA Extraction and qRT-PCR Assay
RNA was extracted from 100 mg of leaves using RNeasy® Plant Mini Kit (Qiagen, Germany) following the manufacturer’s instructions. Then, RNA samples were treated with DNA-freeTM kit (ThermoFisher, United States) to remove the residual DNA. Immediately, RNA samples were retrotranscripted to cDNA using QuantiTect® Reverse Transcription kit (Qiagen, Germany) according to the manufacturer’s instructions, and the expression of CaMPK6-1, CaMPK6-2 (Arabidopsis orthologs AtMPK6, Liu et al., 2015), and CaMKK5 (Arabidopsis orthologs AtMKK5, Liu et al., 2015) genes were analyzed by qPCR by triplicate. qPCR was performed using SensiFASTTM SYBR® No-ROX kit (Bioline, France) and primers described in Table 2 following the supplier’s instructions, in a LightCycler® 480 II thermo-cycler (Roche, Switzerland) under the next conditions: 95°C for 2 min and 50 cycles at 95°C for 5 s, followed by 60°C for 10 s, and finally 72°C for 15 s. The beta-tubulin housekeeping gene was used to normalize results from different samples. Primers used in these assays are described in Table 1. Expression signals were quantified and normalized using LightCycler® 480 Software version 1.5 (Roche). The expression fold was calculated according to Livak and Schmittgen (2001):
Statistical Analysis
Statistical analysis was performed using Statistica software version 6.0 (StatSoft Inc.). First, the normality was checked by the Kolmogorov–Smirnov test. The means of the different treatments were compared using two-way ANOVA and the statistic differences were carried out by Tukey test (F-test).
Results
Rice Bran Enzymatic Extract Chemical Characterization
The enzymatic process of obtaining the new water-soluble biostimulant (RBEE) from rice bran has been previously shown (Parrado et al., 2006). The biological tool involved in the enzymatic process for obtaining the RBEE is subtilisine (EC 3.4.21.62). This protease extracts, solubilizes, and hydrolyzes the initial insoluble proteins in brans, reducing the size of original rice bran proteins to soluble peptides. This process also led to the solubilization of hydrophobic compounds as lipids and bioactive metabolites in an emulsion (w/o). The chemical composition is shown in Table 2.
The two major components in RBEE are the proteins and lipids in equal amounts. Protein fraction is mainly comprised of peptides <5 kDa (see Supplementary Figure SM1). These peptides interact with the lipid fraction that allows fatty acids and hydrophobic bioactive compounds such as polyphenols, phytosterols, tocopherols, and tocotrienols (Revilla et al., 2009) to be soluble in water, leading to a greater bioavailability.
Rice bran is rich in prominent bioactives such as phytosterols. Interestingly, our RBEE retains major phytosterols described in rice bran (Santa María et al., 2016), the most abundant phytochemical found being γ–oryzanol (Table 2).
Other bioactive molecules such as tocopherols and tocotrienols (vitamin E) are also present in rice bran. Seven homologs of tocols were identified in RBEE (α-, β-, γ-, and δ-tocopherols and α-, γ-, and δ-tocotrienols). Among these, γ-tocotrienols were the most abundant, followed by α-tocopherols and α-tocotrienols (Table 2).
Rice bran enzymatic extract components also include polyunsaturated fatty acids, so linoleic acid and linolenic acid constitute 35.76% of total fatty acid content (Table 2). Finally, RBEE also contains flavonoids (flavonols, 0.62 mg/g; flavanols, 2.90 mg/g) and phenolic acids (20.14 mg/g).
Accordingly, the enzymatic process increases the bioavailability of RB without losing bioactive components.
Physiological Status in Plants
After the experiments, the physiological status in pepper plants was determined by assaying diverse parameters, such as net photosynthetic rate, chlorophyll content, and DF.
The net photosynthetic rate was significantly affected by O3 exposure (Figure 1A). After the treatments with RBEE, plants showed similar values to the control plants indicating that the RBEE does not interfere with the photosynthesis. However, when the plants were exposed to O3 this rate significantly decreased. This decrease was recovered by 88% in the plants exposed to O3 and treated with RBEE.
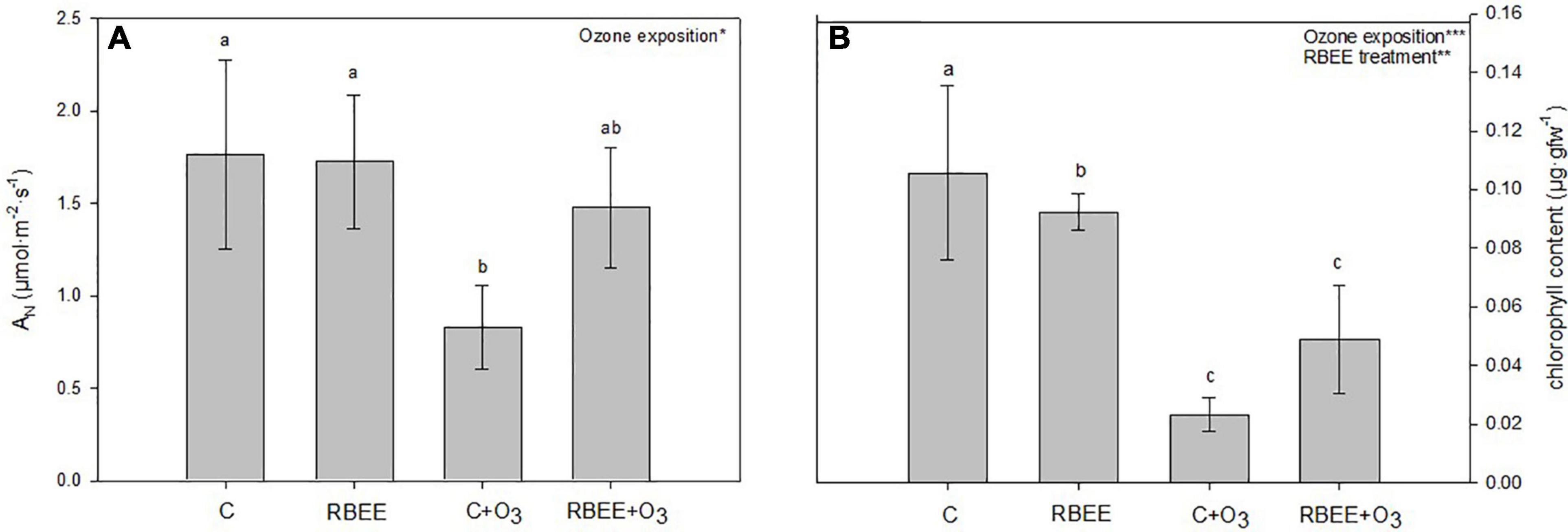
Figure 1. Physiological parameters. (A) Net photosynthetic rate (AN) and (B) total chlorophyll content in pepper plants in response to two conditions of ozone (O3) (0 and 100 ppm) under a treatment without and with rice bran enzymatic extract (RBEE). Values represent mean ± SD, n = 5. Different letters indicate means that are significantly different from each other (two-way ANOVA, O3 exposition × RBEE treatment; HSD test, P < 0.05). O3 exposition and RBEE treatment in the corner of the panel indicate main or interaction significant effects (*P < 0.05; **P < 0.01; ***P < 0.0001).
The total chlorophyll content in plants was significantly affected by both O3 exposure and RBEE treatment (Figure 1B). Leaves of control plants treated with ozone showed 4.6-folds less content of total chlorophyll than non O3-exposed control plants. However, despite RBEE treatment did not recover the content of total chlorophyll under O3 conditions, this treatment improved this content by 53%.
To monitor plant stress status, DF closely related to photosynthetic reactions and chlorophyll content was also measured (Zhang et al., 2019). Both RBEE treatment and O3 exposure produced a significant decrease in DF emissions. The lowest measurements were detected in untreated, O3-fumigated plants, and the RBEE and RBEE + O3 plants showed a less substantial decrease in DF emissions than the C + O3 plants (Figures 2A,B). Although O3 also produced a loss of DF signals in the RBEE-treated plants, this decrease was much less marked compared to C + O3 plants, highlighting a lower stress state of these plants.
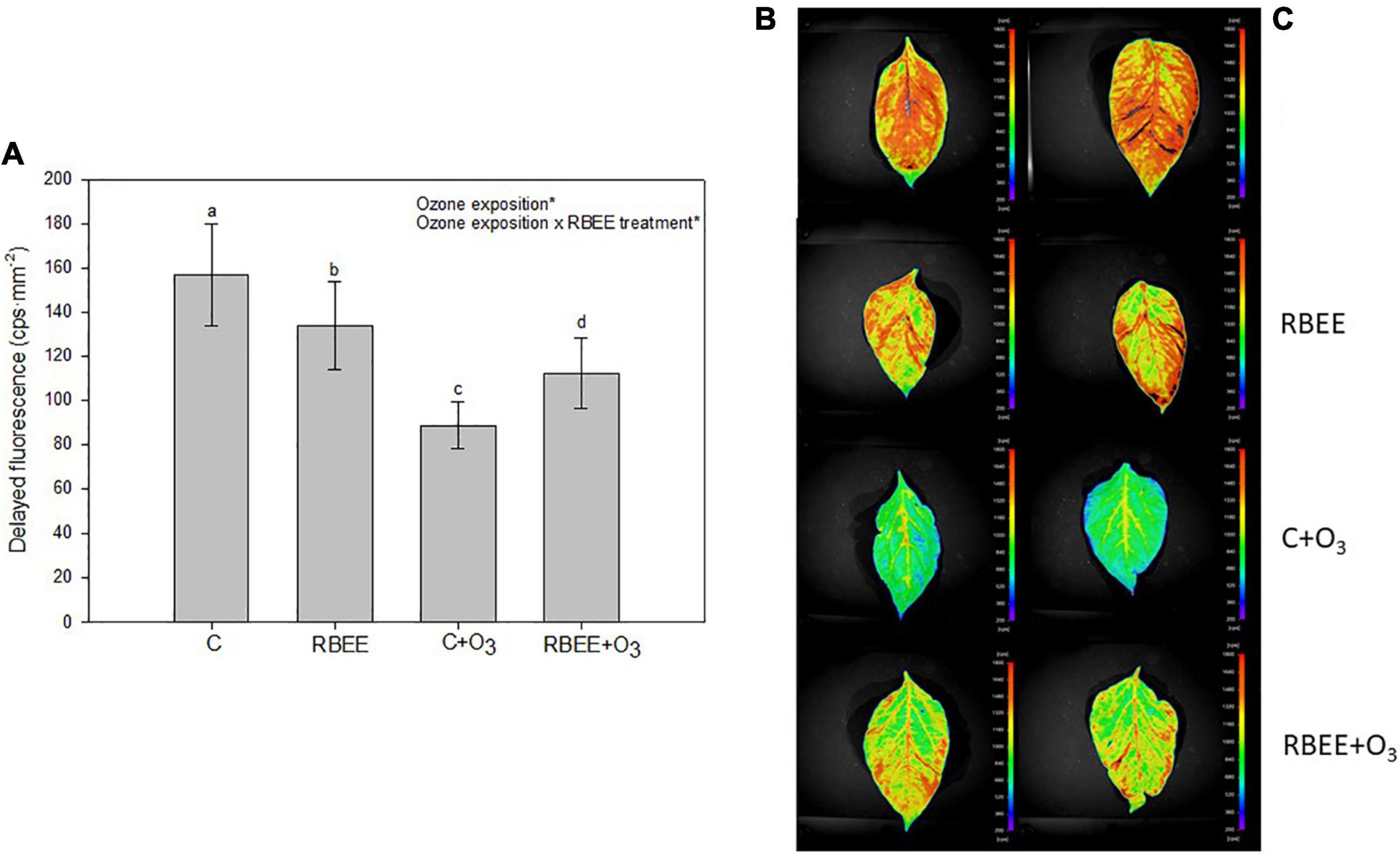
Figure 2. Delayed fluorescence in leaves of pepper plants in response to two conditions of ozone (O3) (0 and 100 ppm) under a treatment without and with rice bran enzymatic extract (RBEE). (A) Counts per second (cps) values for each treatment. Values represent mean ± SD, n = 5. Different letters indicate means that are significantly different from each other (two-way ANOVA, O3 exposition × RBEE treatment; HSD test, P < 0.05). O3 exposition and ozone exposition × RBEE treatment in the corner of the panel indicate main or interaction significant effects (*P < 0.0001); (B) photographs taken by the plant imaging system NightShade LB 985. The color scale mirrors the detected counts per second (cps) of delayed fluorescence emission in leaves.
These results suggest that RBEE protects plants against photosynthetic damages by O3 exposure and maintain the physiological status of plants under these conditions. With the naked eye, although with less significant differences, we could also observe the attenuation of the visible foliar symptoms caused by ozone in the plants treated with RBEE before ozonization. C + O3 plants showed a more widespread chlorosis, as well as the appearance of small brown spots that were not present on RBEE + O3 plants (Supplementary Figure SM2).
Oxidative Stress Level in Plants
To observe the oxidative stress after O3 exposure and RBEE treatment, antioxidant enzyme activities were measured. Both variables had significant effects on these activities (Figure 3). As expected, O3-exposed plants showed a significant increase in their enzymatic activities in comparison with the control to alleviate the oxidative stress caused by ozone, underline that SOD and APX showed more activity than the other enzymes. Nevertheless, the activity of enzymes decreased both in an O3 environment and when plants were treated with RBEE but no exposed to O3, with the insignificant exception of the APX activity without ozone exposure (Figure 3B). In absence of O3, the activities decrease 16, 26, and 17%, in CAT, GPX, and SOD, respectively. In O3-exposed plants, the decreases were 41, 49, 10, and 34% in CAT, APX, GPX, and SOD, respectively, being the APX activity the most affected.
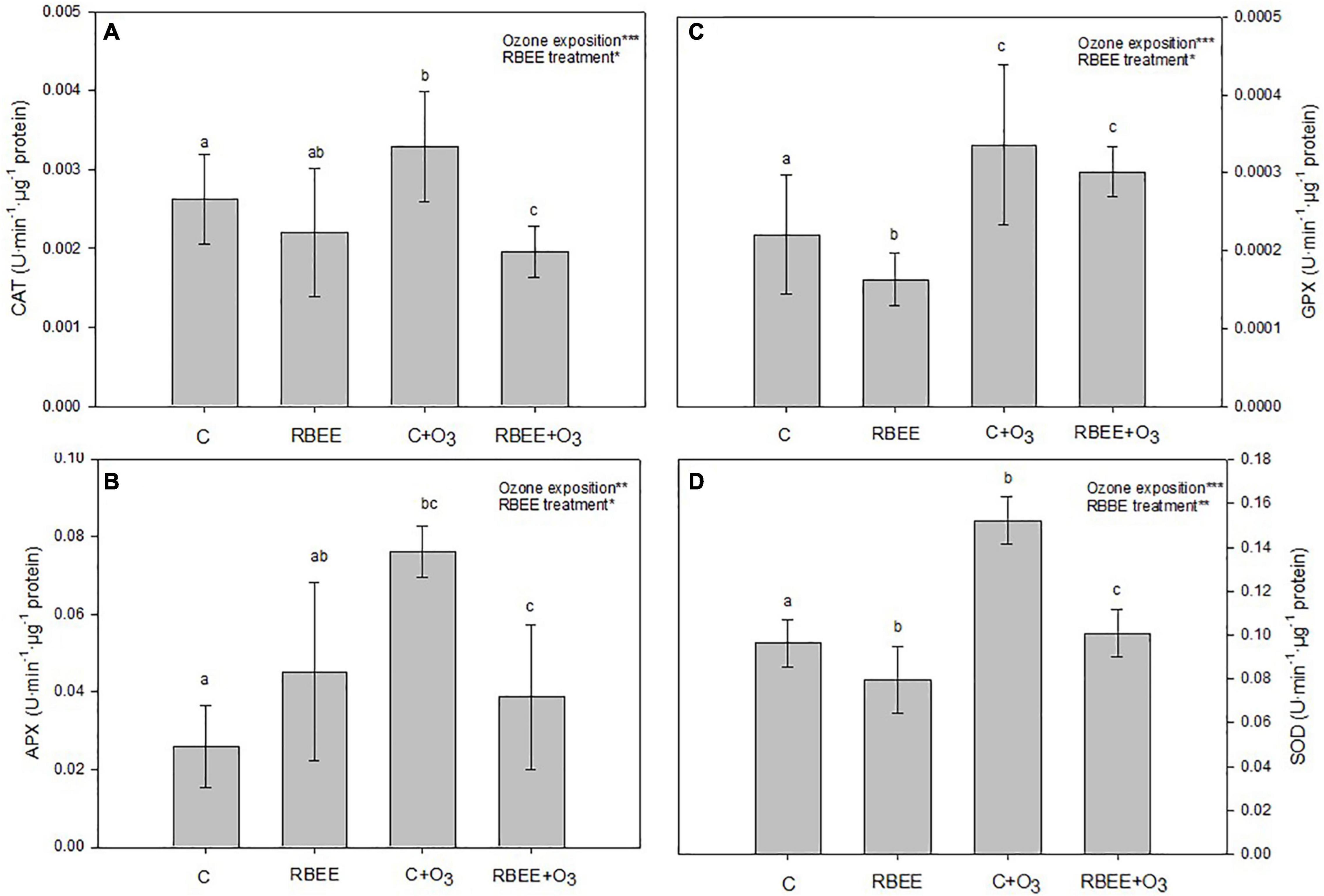
Figure 3. Antioxidant enzyme activities. (A) Catalase (CAT), (B) ascorbate peroxidase (APX), (C) guaiacol peroxidase (GPX), and (D) superoxide dismutase (SOD) activities from leaves of pepper plants in response to two conditions of ozone (O3) (0 and 100 ppm) under a treatment without and with rice bran enzymatic extract (RBEE). Values represent mean ± SD, n = 5. Different letters indicate means that are significantly different from each other (two-way ANOVA, O3 exposition × RBEE treatment; HSD test, P < 0.05). O3 exposition and RBEE treatment in the corner of the panels indicate main or interaction significant effects (*P < 0.01; **P < 0.001; ***P > 0.0001).
To check the lipid peroxidation of plants, the content of MDA, commonly used as a marker of oxidative stress (Oszlányi et al., 2020), was measured in our leaf samples. As shown in Figure 4A, higher concentrations of MDA were recorded in the C + O3 plants, which presented a significant increase in MDA content compared to all other experimental plants. On the other hand, the plants sprayed with RBEE showed similar levels of lipid peroxidation to those of the control plants, showing the protective effect of RBEE treatment against oxidative stress caused by O3 exposure.
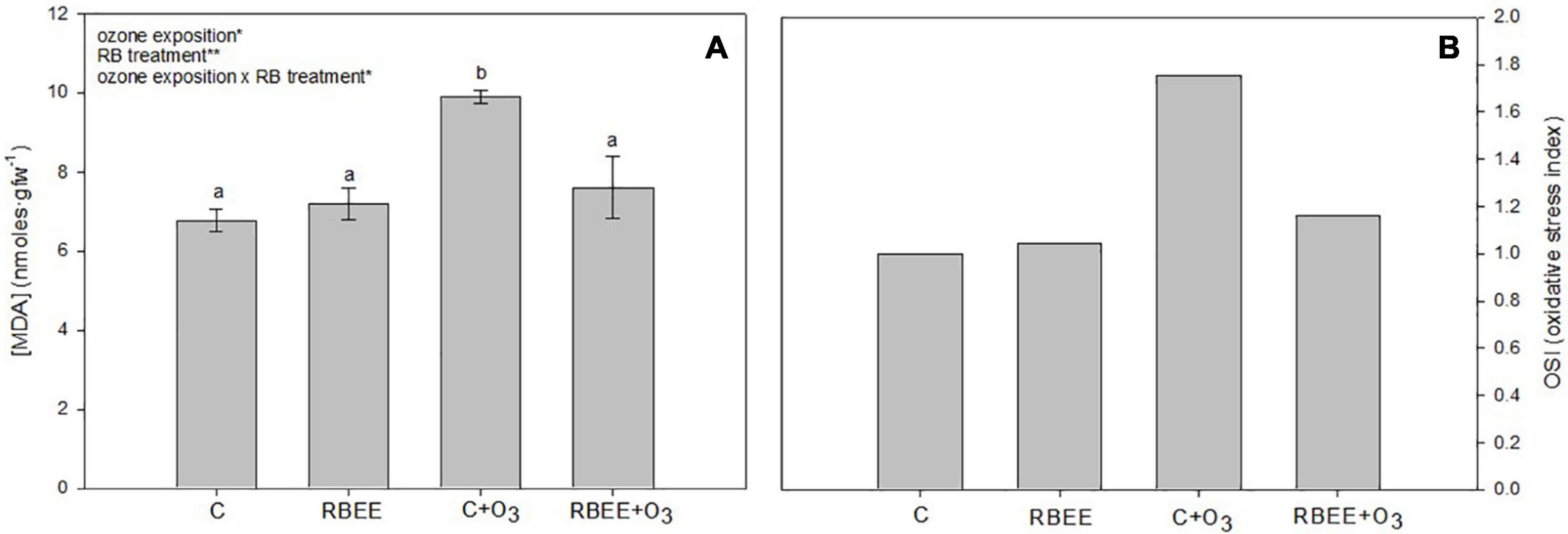
Figure 4. Malondialdehyde concentration (MDA) (A) and oxidative stress index (OSI) (B) in leaves of pepper plants in response to two conditions of ozone (O3) (0 and 100 ppm) under a treatment without and with rice bran enzymatic extract (RBEE). Values represent mean ± SD, n = 5. Different letters indicate means that are significantly different from each other (two-way ANOVA, O3 exposition × RBEE treatment; HSD test, P < 0.05). O3 exposition, RBEE treatment, and O3 exposition × RBEE treatment in the corner of the panels indicate main or interaction significant effects (*P < 0.01; **P < 0.001).
All these positive effects of RBEE in plants in an O3 environment were also reflected in the OSI (Figure 4B). The OSI shows that O3-exposed plants treated with RBEE contain more similar stress levels to that of the control plants. These results support the protective effects of RBEE against O3-induced oxidative damage. Furthermore, RBEE application in leaves did not produce any oxidative stress in pepper plants.
Mitogen-Activated Protein Kinase Genes Expression
This study also checked the expression of some MAPK genes involved in oxidative stress in plants (Liu and He, 2017). As suspected, expression of CaMPK6-1, CaMPK6-2, and CaMKK5 genes increased in leaves of O3-exposed plants (Figure 5). RBEE did not give rise to the expression of these genes in plants in the absence of an O3 environment. Surprisingly, however, after O3 exposure, RBEE treated leaves showed a huge overexpression of these MAPK genes, these being expressions of 4.89, 55.31, and 6.39-folds higher than in the O3-exposed control plant for CaMPK6-1, CaMPK6-2, and CaMKK5 genes, respectively (Figure 5). This result could indicate that the O3-oxidated RBEE produces the induction of gene expression by acting as a transcription inductor. However, this fact should be studied to understand the mechanism involved in this process.
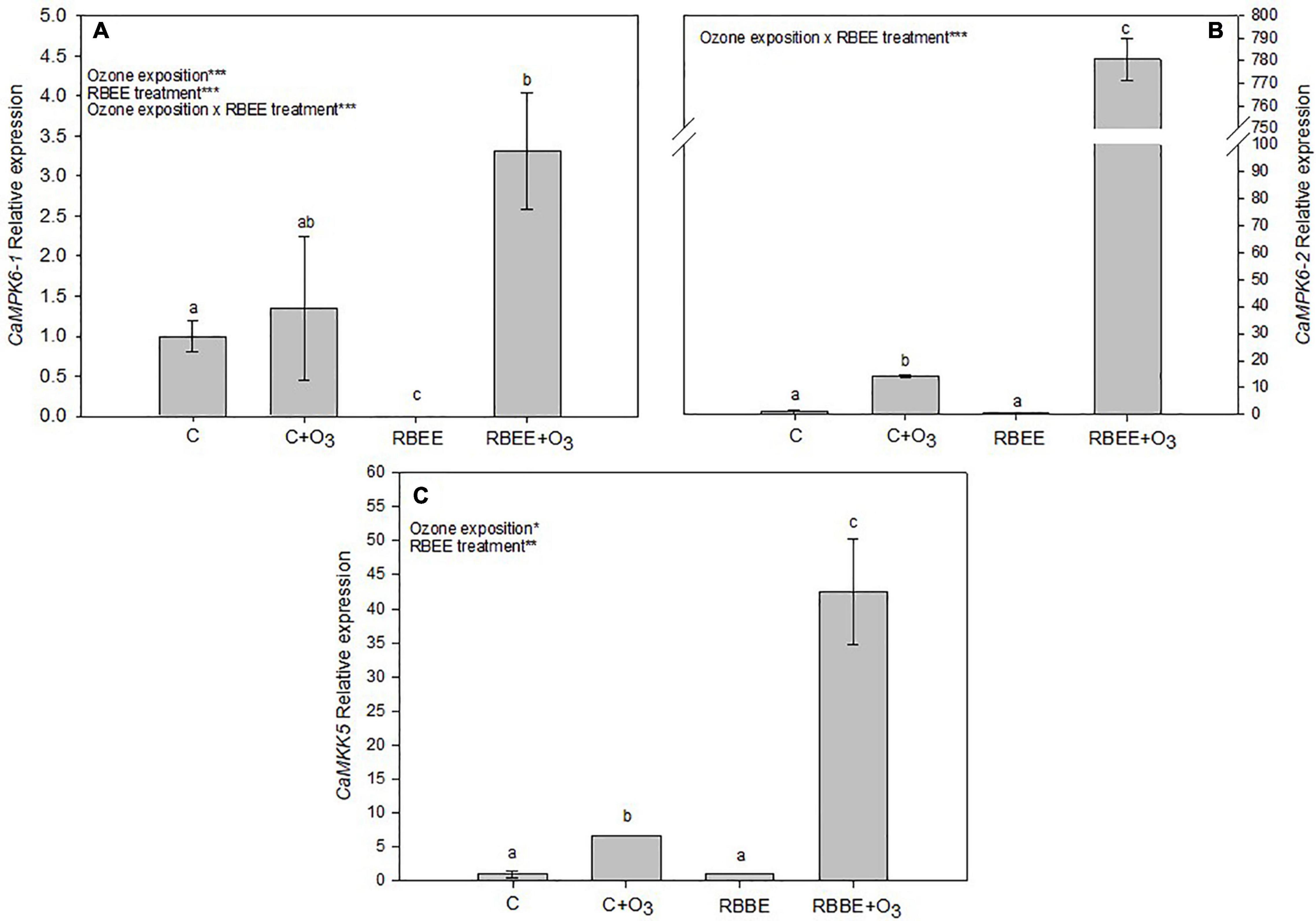
Figure 5. Relative expession of (A) CaMPK6-1, (B) CaMPK6-2, and (C) CaMKK5 in leaves of pepper plants in response to two conditions of ozone (O3) (0 and 100 ppm) under a treatment without and with rice bran enzymatic extract (RBEE). For each gene, the expression level is relative to that of control plants (without RBEE treatment and without O3 exposition) considered as 1. Values represent relative expression ± SD, n = 3. Different letters indicate means that are significantly different from each other (two-way ANOVA, O3 exposition × RBEE treatment; HSD test, P < 0.05). O3 exposition, RBEE treatment, and O3 exposition × RBEE treatment in the corner of the panels indicate main or interaction significant effects (*P < 0.05; **P < 0.001; ***P < 0.0001).
Discussion
Biostimulants have been defined as “a formulated product of biological origin that improves plant productivity as a consequence of the novel, or emergent properties of the complex of constituents, and not as a sole consequence of the presence of known essential plant nutrients, plant growth regulators, or plant protective compounds” (Yakhin et al., 2017). Present results show a new product, an enzymatic extract from rice bran that protects against O3-induced damage. O3 treatment induces a decrease in net photosynthetic rate, chlorophyll content, and DF in pepper leaves which was partially reversed after foliar treatment with RBEE (Figures 1A,B, 2A, respectively). Thus, we can propose that RBEE may exert a biostimulant effect on pepper plants mainly based on its bioactive compounds.
Rice bran enzymatic extract, the enzymatically obtained extract from rice bran, shows a notable total antioxidant capacity (Revilla et al., 2009) and has been evaluated as a protector against lipid and protein oxidation in rat brain homogenate (Parrado et al., 2003). Additionally, we tested the antioxidant capacity of RBEE in two cell models: keratinocyte monolayers and reconstructed human epidermis both irradiated with UVB, and found that RBEE decreased lipid peroxidation in both systems (Santa-María et al., 2010).
Rice bran enzymatic extract is rich in prominent bioactive molecules. Many components of the extract may contribute to its antioxidant capacity. The most abundant phytochemical is γ-oryzanol, which is a natural antioxidant composed of ferulic acid esters of sterols and triterpene alcohols with high scavenging activity of free radicals, mainly mediated by ferulic acid moiety (Lemus et al., 2014; Minatel et al., 2016; Massarolo et al., 2017; Zduńska et al., 2018). RBEE also contains tocols (both tocopherols and tocotrienols) that act as antioxidants due to their ability to donate phenolic hydrogens (electrons) to lipid radicals (Xu et al., 2001). Polyunsaturated fatty acids which act as radical scavenging agents are also present in RBEE, contributing to the antioxidant activity. So, other natural substances such as Rosa rubiginosa oil (Franco et al., 2007), grape seed oil (Bail et al., 2008), and soybean-germ oil (Chen et al., 2019) are used for cosmetic purposes due to their polyunsaturated fatty acid content, which acts as a radical scavenging agent.
Rice bran enzymatic extract is also rich in PHs. PHs exhibit different antioxidant and free radicals scavenging activities (Li et al., 2008), mainly conferred by some nitrogenous compounds contained therein such as glycine, betaine, and proline (du Jardin, 2015). Moreover, PHs have shown an ability to enhance antioxidant mechanisms in plants (Gurav and Jadhav, 2013). It is worth noting that PHs, mainly those resulting from the enzymatic hydrolysis of protein substrates into low molecular weight peptides and free amino acids, have shown multiple biostimulant capabilities. Protein hydrolysates are included within the biostimulant classification (du Jardin, 2015). The direct effects in plants include modulating N uptake and assimilation, acting on signaling pathways in the root, regulating enzymes involved in this process (du Jardin, 2015), possessing hormonal activity similar to auxin and gibberellin (Colla et al., 2014), and producing antioxidant activity (Li et al., 2008; Gurav and Jadhav, 2013). In addition, when applied to soils, PHs have shown indirect effects on plant growth and nutrition by increasing the availability of nutrients and their acquisition by the roots – enhancing the microbial activity and biomass of the soil, soil respiration, and their fertility in general (du Jardin, 2015; Rodríguez-Morgado et al., 2015).
It is worthing to note that mixture of these bioactive molecules, as is the case of RBEE, has been reported to greatly enhance the antioxidant activity of rice bran oil (Perez-Ternero et al., 2017).
According to the RBEE antioxidant capacity, we have selected an acute O3 treatment as an abiotic stressor. O3 is degraded in the apoplast into secondary ROS (Vainonen and Kangasjärvi, 2015) and high levels of ROS can lead to ROS stress that induces the enzymatic and non-enzymatic-systems that protect cells from ROS (Sachdev et al., 2021). Accordingly all the enzymatic activities assayed – CAT, GPX, APX, and SOD – were induced after O3 treatment. Induction was significantly reversed by the foliar treatment with RBEE (Figure 3). The effect on enzymatic activities could be correlated with the antioxidant effect of RBEE, thus the lipid peroxidation induced by O3 treatment was significantly alleviated by RBEE (Figure 4A). Consequently, the antioxidant effect is reflected in the OSI values (Figure 4B).
Unlike other enzymes assayed, a surprising result was the induction of APX after the application of RBEE in plants not treated with O3 (Figure 3B). We can speculate that this APX induction may be due to a hormetic effect induced by RBEE, stimulating a cellular system that could be essential for plant defense under stress. At the physiological level, hormesis is an adaptive response of an organism to a low-level stress factor accompanied by over-compensation when homeostasis is interrupted (Mattson, 2008; Calabrese, 2009; Wiegant et al., 2012). Hormesis is the cellular response in plants that occurs after an initial exposure to low levels of biotic or abiotic stressors – such as herbicides, temperature, chemicals, and radiation – which predisposes them to stimulate cellular defense mechanisms at subsequent sources of stress (Agathokleous and Calabrese, 2020; Berry and López-Martínez, 2020). For example, low doses of some herbicides such as 2,4-dichlorophenoxyacetic acid, glyphosate, and paraquat have been shown to trigger auxin production and antioxidant defense. The biostimulant-induced hormesis response of beneficial organisms allows plants to tolerate stress (Ahkami et al., 2017) through activation of secondary metabolisms and gene expression to recover homeostasis (Vargas-Hernandez et al., 2017). Application of biostimulants at right time can therefore facilitate increased plant growth while combined application of multiple biostimulants can be effective in reducing environmental drastic impacts (Dong et al., 2020).
In plants, the mechanism of hormesis is still unknown. The most probable pathways for hormetic responses are the induction of ROS by mild stress which leads to the activation of antioxidant defenses, stress-signaling hormones, or adaptive growth responses (Poschenrieder et al., 2013). Thus, induction of low and sub-toxic concentrations of ROS by mild stressors, such as which occurs as a result of foliar application of RBEE, has shown the ability to develop a hormetic effect, activating antioxidative defense and adaptive responses. RBEE is rich in 18C-unsaturated fatty acids (UFAs): oleic (18:1), linoleic (18:2), and α-linolenic (18:3) acids (Table 2). Besides their roles as ingredients and modulators of cellular membranes, reserves of carbon and energy, stocks of extracellular barrier constituents (e.g., cutin and suberin), or precursors of various bioactive molecules (e.g., jasmonates and nitroalkenes), recent works have pointed the role of 18C-UFAs as regulators of stress signaling (He and Ding, 2020). Oleic acid has been implicated in plant immunity against pathogens (Mandal et al., 2012). Even more, linoleic acid may regulate plant defense through ROS production (Yaeno et al., 2004). In this context, we can assume that RBEE application may induce a mild increase in ROS due to UFAs content that underly the induction of APX activity.
Ascorbate peroxidase is an H2O2-scavenging enzyme and is indispensable for the protection of chloroplasts and other cell constituents from damage by H2O2 and hydroxyl radicals (OH.). APX has been identified in most higher plants and comprises a family of isoenzymes present in different plant cell compartments, including apoplast (Sofo et al., 2015). As compared to catalase, it is more vital in stress as it has a high affinity for hydrogen peroxide (Afzal et al., 2014). Accordingly, we have found that APX activity exceeds catalase activity 10 times (Figures 3A,B). After O3 enters the leaves through the stomata, it rises to the apoplast where it is immediately degraded into secondary ROS. Detoxification of ROS in the apoplast can therefore be considered as an early line of defense against O3 (Kangasjärvi et al., 2005; Castagna and Ranieri, 2009). APX uses ascorbate as its specific electron donor to reduce H2O2 to water. Ascorbate is believed to be the major redox buffer and ROS scavenger in the apoplast (Foyer and Noctor, 2009). Thus, we can speculate that pretreatment with RBEE induced ROS generation that slightly stimulates APX activity, probably at the apoplast, the gate of entrance, that facilitates posterior induction and consequently protection against O3 exposure.
The APX result could be working in tandem with the decrease in chlorophyll content and DF found in the same group of plants (Figures 1B, 2A). This effect could be due to linoleic acid present in RBEE as it has been shown that linoleic acid decreased the chlorophyll concentration and photosynthetic efficiency in Cylindrospermopsis raciborskii (Xu et al., 2016).
Protein phosphorylation mediated by protein kinase cascades is one of the most important post-translational modifications that coordinate response in cells. One relevant protein-kinase based amplification cascade is the MAPK cascade. The MAPK cascade is a complex signaling pathway hierarchically organized at least three sequentially acting serine/threonine kinases – a MAP kinase kinase kinase (MAPKKK), a MAP kinase kinase (MAPKK), and finally, the MAPK itself – with each phosphorylating, and hence activating, the next kinase. In this cascade, MAPKs phosphorylate specific substrate proteins, such as transcription factors and enzymes, and subsequently trigger cellular responses and rapidly transform upstream signals into appropriate intracellular responses (Liu et al., 2015). MAPK cascade is involved in many aspects of plant physiology, including cell division (Komis et al., 2011), plant growth and development (Xu and Zhang, 2015), plant resistance to pathogens (Bigeard et al., 2015) and insect herbivores (Hettenhausen et al., 2015), and plant response to abiotic stresses (Liu, 2012; Smékalová et al., 2014). It has been described as an interplay between MAPK cascade and ROS; so exogenous application of H2O2 or O3 activates components of MAPK cascades. On the other hand, manipulating MAPK cascades results in initiation of ROS responses (Šamajová et al., 2013). In this context, the present work analyses the role of the MAPK cascade in the protective effect of RBEE against O3 damage.
The majority of MAPK and MAPKK members are constitutively expressed in pepper plants. In leaves, the transcript level of CaMPK1 is the highest, followed by CaMPK6-2, and CaMPK19-2. Among MAPKK genes, CaMKK5 exhibits the highest transcript levels. Under the challenges of heat shock, salt stress, or pathogen inoculation, most of the MAPKs and MAPKKs in the pepper genome were significantly transcriptionally modified (Liu et al., 2015). Accordingly, after O3 exposure, we found induction of the studied MAPKs. It is worth noting the relevant role that MPK3/MPK6 play in ROS response and O3 sensitivity. AtMPK3/AtMPK6 in A. thaliana (Ahlfors et al., 2004) and the orthologs SIPK/WIPK in tobacco (Marcus et al., 2000), have been found to regulate for O3 sensitivity; in fact RNAi-mediated silencing of MPK6 renders the plant more sensitive to O3 (Miles et al., 2005). In these lines we found increased CaMAPK6-1 and CaMAPK6-2 after pepper plants exposition to O3. Moreover, induction of CaMKK5 may be related to CaMAPK6-1 and CaMAPK6-2. It has been described in Arabidopsis that MKK5 was involved in MPK3/MPK6 activation in response to O3 exposure (Vainonen and Kangasjärvi, 2015) and interestingly in pepper CaMPKK5 interacts with CaMPK3, CaMPK6-1, and CaMPK6-2 (Liu et al., 2015).
But surprisingly, after O3 exposure, RBEE treated leaves showed a huge expression of these MAPK genes, being higher than in the control plants under O3 in CaMPK6-1, CaMPK6-2, and CaMKK5 genes (Figure 5). As previously indicated, the MAPK cascade is involved in many aspects of plant physiology including the defense response against stress. The presence of multiple genes’ family members in genomes of different plant species encoding for MAPKKKs, MAPKKs, and MAPKs, and the fact that one MAPK cascade may be associated with more than one upstream or downstream partner (Liu et al., 2015) made it difficult to elucidate specific mechanism mediated by MAPK modules. How ROS activates MAPK cascades still remains unclear. It is possible that plants not only use MAPK cascades to transduce ROS signaling to gene expression and sometimes cell death, but also initiate the negative feedback regulation by MAPK cascades to maintain ROS homeostasis. The different combinations of the three tiers of kinases, distribution, time point-dependent activation, strength, duration, and availability of substrates of MAPK cascades may determine the feed-forward or feed-back outcomes (Liu and He, 2017). However, our results unequivocally indicate that both the damage induced by O3 and the activation of the protection systems against it induced by RBEE take place through the transcriptional induction of the MAPK cascade.
Conclusion
Rice bran enzymatic extract, an enzymatic extract of plant origin, reversed the O3-induced decrease in physiological parameters as net photosynthetic rate, chlorophyll content, and DF. Thus, the results of our study highlight the potential use of RBEE as an effective biostimulant plant protector against oxidative stress caused by O3. Present results also point out that MAPK cascade is involved in both, O3-induced damage and RBEE protection. However, more studies are needed to clarify how this kinase pathway is involved.
Thus, we contribute to the general efforts done in the last decade searching new non-chemical alternative products to protect crops against damages caused by environmental oxidative stress.
Data Availability Statement
The original contributions presented in the study are included in the article/Supplementary Material, further inquiries can be directed to the corresponding author.
Author Contributions
SM-B, SN-T, ER, AC, and JP designed the study. SM-B, SN-T, and LM performed the research. SM-B, SN-T, PC, ER, AC, and JP analyzed the data and wrote the manuscript. All authors contributed to the article and approved the submitted version.
Conflict of Interest
The authors declare that the research was conducted in the absence of any commercial or financial relationships that could be construed as a potential conflict of interest.
Publisher’s Note
All claims expressed in this article are solely those of the authors and do not necessarily represent those of their affiliated organizations, or those of the publisher, the editors and the reviewers. Any product that may be evaluated in this article, or claim that may be made by its manufacturer, is not guaranteed or endorsed by the publisher.
Funding
This study was supported by the Ministerio de Ciencia e Innovación (Spain), Proyectos I + D + i <<Retos Investigación>> Convocatoria 2018 RTI2018-097425-B100.
Acknowledgments
We are particularly grateful with Emilia Sanz-Rios for help with language corrections. We thank the University of Seville Greenhouse General Service (CITIUS) for their collaboration and providing the facilities.
Supplementary Material
The Supplementary Material for this article can be found online at: https://www.frontiersin.org/articles/10.3389/fpls.2021.749422/full#supplementary-material
References
Afzal, F., Khurshid, R., Ashraf, M., and Gul Kazi, A. (2014). “Reactive Oxygen Species and Antioxidants in Response to Pathogens and Wounding,” in Oxidative Damage to Plants Antioxid, ed. P. Ahamad (Amsterdam: Elsevier), 397–424. doi: 10.1016/B978-0-12-799963-0.00013-7
Agathokleous, E., and Calabrese, E. J. (2020). A global environmental health perspective and optimisation of stress. Sci. Total Environ. 704:135263. doi: 10.1016/j.scitotenv.2019.135263
Ahkami, A. H., Allen White, R., Handakumbura, P. P., and Jansson, C. (2017). Rhizosphere engineering: enhancing sustainable plant ecosystem productivity. Rhizosphere 3, 233–243. doi: 10.1016/J.RHISPH.2017.04.012
Ahlfors, R., Macioszek, V., XRudd, J., Brosché, M., Schlichting, R., Scheel, D., et al. (2004). Stress hormone-independent activation and nuclear translocation of mitogenactivated protein kinases in Arabidopsis thaliana during ozone exposure. Plant J. 40, 512–522. doi: 10.1111/J.1365-313X.2004.02229.X
Ainsworth, E. A., Yendrek, C. R., Sitch, S. S., Collins, W. J. C., and Emberson, L. D. (2012). The effects of tropospheric ozone on net primary productivity and implications for climate change. Annu. Rev. Plant Biol. 63, 637–661. doi: 10.1146/ANNUREV-ARPLANT-042110-103829
Archambault, D. J., Archambault, D. J., Slaski, J. J., and Li, X. (2000). Ozone protection in plants: the potential use of chemical protectants to measure atmospheric oxidant damage in Alberta crops. Edmonton: Alberta Environment. doi: 10.5962/bhl.title.115607
Arnon, D. I. (1949). Copper enzymes in isolated chloroplasts. Polyphenoloxidase in Beta vulgaris. Plant Physiol. 24, 1–15. doi: 10.1104/PP.24.1.1
Avnery, S., Mauzerall, D. L., Liu, J., and Horowitz, L. W. (2011). Global crop yield reductions due to surface ozone exposure: 2. Year 2030 potential crop production losses and economic damage under two scenarios of O3 pollution. Atmos. Environ. 45, 2297–2309. doi: 10.1016/J.ATMOSENV.2011.01.002
Bail, S., Stuebiger, G., Krist, S., Unterweger, H., and Buchbauer, G. (2008). Characterisation of various grape seed oils by volatile compounds, triacylglycerol composition, total phenols and antioxidant capacity. Food Chem. 108, 1122–1132. doi: 10.1016/J.FOODCHEM.2007.11.063
Berry, R., and López-Martínez, G. (2020). A dose of experimental hormesis: when mild stress protects and improves animal performance. Comp. Biochem. Physiol. A Mol. Integr. Physiol. 242:110658. doi: 10.1016/j.cbpa.2020.110658
Bigeard, J., Colcombet, J., and Hirt, H. (2015). Signaling mechanisms in pattern-triggered immunity (PTI). Mol. Plant 8, 521–539. doi: 10.1016/J.MOLP.2014.12.022
Bortolin, R. C., Caregnato, F. F., Divan Junior, A. M., Zanotto-Filho, A., Moresco, K. S., de Oliveira Rios, A., et al. (2016). Chronic ozone exposure alters the secondary metabolite profile, antioxidant potential, anti-inflammatory property, and quality of red pepper fruit from Capsicum baccatum. Ecotoxicol. Environ. Saf. 129, 16–24. doi: 10.1016/J.ECOENV.2016.03.004
Bradford, M. M. (1976). A rapid and sensitive method for the quantitation of microgram quantities of protein utilizing the principle of protein-dye binding. Anal. Biochem. 72, 248–254. doi: 10.1016/0003-2697(76)90527-3
Calabrese, E. J. (2009). Hormesis: a Conversation with a Critic. Environ. Health Perspect. 117:1339. doi: 10.1289/EHP.0901002
Castagna, A., and Ranieri, A. (2009). Detoxification and repair process of ozone injury: from O3 uptake to gene expression adjustment. Environ. Pollut. 157, 1461–1469. doi: 10.1016/J.ENVPOL.2008.09.029
Chen, J., Tang, G., Zhou, J., Liu, W., and Bi, Y. (2019). The characterization of soybean germ oil and the antioxidative activity of its phytosterols. RSC Adv. 9, 40109–40117. doi: 10.1039/C9RA08771K
Colla, G., Rouphael, Y., Canaguier, R., Svecova, E., and Cardarelli, M. (2014). Biostimulant action of a plant-derived protein hydrolysate produced through enzymatic hydrolysis. Front. Plant Sci. 5:448. doi: 10.3389/fpls.2014.00448
Didyk, N. P., and Blum, O. B. (2010). Natural antioxidants of plant origin against ozone damage of sensitive crops. Acta Physiol. Plant 33, 25–34. doi: 10.1007/S11738-010-0527-5
Dong, C., Wang, G., Du, M., Niu, C., Zhang, P., Zhang, X., et al. (2020). Biostimulants promote plant vigor of tomato and strawberry after transplanting. Sci. Hortic. 267:109355. doi: 10.1016/J.SCIENTA.2020.109355
du Jardin, P. (2015). Plant biostimulants: definition, concept, main categories and regulation. Sci. Hortic. 196, 3–14. doi: 10.1016/J.SCIENTA.2015.09.021
Duarte, B., Goessling, J., Marques, J., and Caçador, I. (2015). Ecophysiological constraints of Aster tripolium under extreme thermal events impacts: merging biophysical, biochemical and genetic insights. Plant Physiol. Biochem. 97, 217–228. doi: 10.1016/J.PLAPHY.2015.10.015
Esterbauer, H., and Cheeseman, K. (1990). Determination of aldehydic lipid peroxidation products: malonaldehyde and 4-hydroxynonenal. Methods Enzymol. 186, 407–421. doi: 10.1016/0076-6879(90)86134-H
Foyer, C., and Noctor, G. (2009). Redox regulation in photosynthetic organisms: signaling, acclimation, and practical implications. Antioxid. Redox Signal. 11, 861–905. doi: 10.1089/ARS.2008.2177
Franco, D., Pinelo, M., Sineiro, J., and Núñez, M. J. (2007). Processing of Rosa rubiginosa: extraction of oil and antioxidant substances. Bioresour. Technol. 98, 3506–3512. doi: 10.1016/J.BIORTECH.2006.11.012
Gill, S. S., and Tuteja, N. (2010). Reactive oxygen species and antioxidant machinery in abiotic stress tolerance in crop plants. Plant Physiol. Biochem. 48, 909–930. doi: 10.1016/J.PLAPHY.2010.08.016
Goufo, P., and Trindade, H. (2014). Rice antioxidants: phenolic acids, flavonoids, anthocyanins, proanthocyanidins, tocopherols, tocotrienols, γ-oryzanol, and phytic acid. Food Sci. Nutr. 2, 75–104. doi: 10.1002/FSN3.86
Guo, W., Chen, R., Gong, Z., Yin, Y., Ahmed, S., and He, Y. (2012). Exogenous abscisic acid increases antioxidant enzymes and related gene expression in pepper (Capsicum annuum) leaves subjected to chilling stress. Genet. Mol. Res. 11, 4063–4080. doi: 10.4238/2012.SEPTEMBER.10.5
Gurav, R. G., and Jadhav, J. P. (2013). A novel source of biofertilizer from feather biomass for banana cultivation. Environ. Sci. Pollut. Res. 20, 4532–4539. doi: 10.1007/s11356-012-1405-z
He, M., and Ding, N.-Z. (2020). Plant Unsaturated Fatty Acids: multiple Roles in Stress Response. Front. Plant Sci. 11:562785. doi: 10.3389/FPLS.2020.562785
Hettenhausen, C., Schuman, M., and Wu, J. (2015). MAPK signaling: a key element in plant defense response to insects. Insect Sci. 22, 157–164. doi: 10.1111/1744-7917.12128
Hiscox, J. D., and Israelstam, G. F. (1979). A method for the extraction of chlorophyll from leaf tissue without maceration. Can. J. Bot. 57, 1332–1334. doi: 10.1139/b79-163
Kangasjärvi, J., Jaspers, P., and Kollist, H. (2005). Signalling and cell death in ozone-exposed plants. Plant Cell Environ. 28, 1021–1036. doi: 10.1111/J.1365-3040.2005.01325.X
Komis, G., Illés, P., Beck, P., and Šamaj, J. (2011). Microtubules and mitogen-activated protein kinase signalling. Curr. Opin. Plant Biol. 14, 650–657. doi: 10.1016/J.PBI.2011.07.008
Lemus, C., Angelis, A., Halabalaki, M., and Skaltsounis, A. L. (2014). ‘γ-Oryzanol. An Attractive Bioactive Component from Rice Bran,’ in Wheat and Rice in Disease Prevention and Health, eds R. R. Watson, V. R. Preedy, and S. Zibadi (London: Elsevier Inc.), 409–430. doi: 10.1016/B978-0-12-401716-0.00032-5
Li, Y., Jiang, B., Zhang, T., Mu, W., and Liu, J. (2008). Antioxidant and free radical-scavenging activities of chickpea protein hydrolysate (CPH). Food Chem. 106, 444–450. doi: 10.1016/j.foodchem.2007.04.067
Liu, Y. (2012). Roles of mitogen-activated protein kinase cascades in ABA signaling. Plant Cell Rep. 31, 1–12. doi: 10.1007/S00299-011-1130-Y
Liu, Y., and He, C. (2017). A review of redox signaling and the control of MAP kinase pathway in plants. Redox Biol. 11:192. doi: 10.1016/J.REDOX.2016.12.009
Liu, Z., Shi, L., Liu, Y., Tang, Q., Shen, L., Yang, S., et al. (2015). Genome-wide identification and transcriptional expression analysis of mitogen-activated protein kinase and mitogen-activated protein kinase kinase genes in Capsicum annuum. Front. Plant Sci. 6:780. doi: 10.3389/FPLS.2015.00780
Livak, K., and Schmittgen, T. (2001). Analysis of relative gene expression data using real-time quantitative PCR and the 2(-Delta Delta C(T)) Method. Methods 25, 402–408. doi: 10.1006/METH.2001.1262
López-Jurado, J., Balao, F., and Mateos-Naranjo, E. (2020). Polyploidy-mediated divergent light-harvesting and photoprotection strategies under temperature stress in a Mediterranean carnation complex. Environ. Exp. Bot. 171:103956. doi: 10.1016/J.ENVEXPBOT.2019.103956
Mandal, M., Chandra-Shekara, A., Jeong, R., Yu, K., Zhu, S., Chanda, B., et al. (2012). Oleic acid-dependent modulation of NITRIC OXIDE ASSOCIATED1 protein levels regulates nitric oxide-mediated defense signaling in Arabidopsis. Plant Cell 24, 1654–1674. doi: 10.1105/TPC.112.096768
Marcus, A. S., Godfrey, P. M., and Brian, E. E. (2000). Ozone treatment rapidly activates MAP kinase signalling in plants. Plant J. 22, 367–376. doi: 10.1046/J.1365-313X.2000.00741.X
Massarolo, K. C., Denardi de Souza, T., Collazzo, C. C., Badiale Furlong, E., and de Souza Soares, L. A. (2017). The impact of Rhizopus oryzae cultivation on rice bran: gamma-oryzanol recovery and its antioxidant properties. Food Chem. 228, 43–49. doi: 10.1016/J.FOODCHEM.2017.01.127
Miles, G. P., Samuel, M. A., Zhang, Y., and Ellis, B. E. (2005). RNA interference-based (RNAi) suppression of AtMPK6, an Arabidopsis mitogen-activated protein kinase, results in hypersensitivity to ozone and misregulation of AtMPK3. Environ. Pollut. 138, 230–237. doi: 10.1016/J.ENVPOL.2005.04.017
Mills, G., Buse, A., Gimeno, B., Bermejo, V., Holland, M., Emberson, L., et al. (2007). A synthesis of AOT40-based response functions and critical levels of ozone for agricultural and horticultural crops. Atmos. Environ. 41, 2630–2643. doi: 10.1016/J.ATMOSENV.2006.11.016
Minatel, I. O., Francisqueti, F. V., Corrêa, C. R., and Lima, G. P. P. (2016). Antioxidant Activity of γ-Oryzanol: a Complex Network of Interactions. Int. J. Mol. Sci. 17:1107. doi: 10.3390/IJMS17081107
Oszlányi, R., Mirmazloum, I., Pónya, Z., Szegő, A., Jamal, S., Bat-Erdene, O., et al. (2020). Oxidative stress level and dehydrin gene expression pattern differentiate two contrasting cucumber F1 hybrids under high fertigation treatment. Int. J. Biol. Macromol. 161, 864–874. doi: 10.1016/J.IJBIOMAC.2020.06.050
Paredes-Páliz, K., Rodríguez-Vázquez, R., Duarte, B., Caviedes, M. A., Mateos-Naranjo, E., Redondo-Gómez, S., et al. (2018). Investigating the mechanisms underlying phytoprotection by plant growth-promoting rhizobacteria in Spartina densiflora under metal stress. Plant Biol. 20, 497–506. doi: 10.1111/plb.12693
Parrado, J., Miramontes, E., Jover, M., Gutierrez, J. F., Collantes de Terán, L., and Bautista, J. (2006). Preparation of a rice bran enzymatic extract with potential use as functional food. Food Chem. 98, 742–748. doi: 10.1016/J.FOODCHEM.2005.07.016
Parrado, J., Miramontes, E., Jover, M., Márquez, J. C., Mejias, M. A., De Teran, L. C., et al. (2003). Prevention of brain protein and lipid oxidation elicited by a water-soluble oryzanol enzymatic extract derived from rice bran. Eur. J. Nutr. 42, 307–314. doi: 10.1007/S00394-003-0424-4
Pérez-Palacios, P., Agostini, E., Ibáñez, S. G., Talano, M. A., Rodríguez-Llorente, I. D., Caviedes, M. A., et al. (2017). Removal of copper from aqueous solutions by rhizofiltration using genetically modified hairy roots expressing a bacterial Cu-binding protein. Environ. Technol. 38, 2877–2888. doi: 10.1080/09593330.2017.1281350
Perez-Ternero, C., Alvarez de Sotomayor, M., and Herrera, M. D. (2017). Contribution of ferulic acid, γ-oryzanol and tocotrienols to the cardiometabolic protective effects of rice bran. J. Funct. Foods 32, 58–71. doi: 10.1016/j.jff.2017.02.014
Poschenrieder, C., Cabot, C., Martos, S., Gallego, B., and Barceló, J. (2013). Do toxic ions induce hormesis in plants?. Plant Sci. 212, 15–25. doi: 10.1016/j.plantsci.2013.07.012
Revilla, E., Maria, C. S., Miramontes, E., Bautista, J., García-Martínez, A., Cremades, O., et al. (2009). Nutraceutical composition, antioxidant activity and hypocholesterolemic effect of a water-soluble enzymatic extract from rice bran. Food Res. Int. 42, 387–393. doi: 10.1016/J.FOODRES.2009.01.010
Revilla, E., Santa-María, C., Miramontes, E., Candiracci, M., Rodríguez-Morgado, B., Carballo, M., et al. (2013). Antiproliferative and immunoactivatory ability of an enzymatic extract from rice bran. Food Chem. 136, 526–531. doi: 10.1016/J.FOODCHEM.2012.08.044
Rodríguez-Morgado, B., Gómez, I., Parrado, J., García-Martínez, A. M., Aragón, C., and Tejada, M. (2015). Obtaining edaphic biostimulants/biofertilizers from different sewage sludges. Effects on soil biological properties. Environ. Technol. 36, 2217–2226. doi: 10.1080/09593330.2015.1024760
Sachdev, S., Ansari, S. A., Ansari, M. I., Fujita, M., and Hasanuzzaman, M. (2021). Abiotic Stress and Reactive Oxygen Species: generation, Signaling, and Defense Mechanisms. Antioxidants 10:277. doi: 10.3390/ANTIOX10020277
Šamajová, O., Plíhal, O., Al-Yousif, M., Hirt, H., and Šamaj, J. (2013). Improvement of stress tolerance in plants by genetic manipulation of mitogen-activated protein kinases. Biotechnol. Adv. 31, 118–128. doi: 10.1016/J.BIOTECHADV.2011.12.002
Santa María, C., Revilla, E., Rodríguez-Morgado, B., Castaño, A., Carbonero, P., Gordillo, B., et al. (2016). Effect of rice parboiling on the functional properties of an enzymatic extract from rice bran. J. Cereal Sci. 72, 54–59. doi: 10.1016/J.JCS.2016.09.010
Santa-María, C., Revilla, E., Miramontes, E., Bautista, J., García-Martínez, A., Romero, E., et al. (2010). Protection against free radicals (UVB irradiation) of a water-soluble enzymatic extract from rice bran. Study using human keratinocyte monolayer and reconstructed human epidermis. Food Chem. Toxicol. 48, 83–88. doi: 10.1016/J.FCT.2009.09.019
Sharma, P., Jha, A. B., Dubey, R. S., and Pessarakli, M. (2012). Reactive Oxygen Species, Oxidative Damage, and Antioxidative Defense Mechanism in Plants under Stressful Conditions. J. Bot. 2012:217037. doi: 10.1155/2012/217037
Smékalová, V., Doskočilová, A., Komis, G., and Šamaj, J. (2014). Crosstalk between secondary messengers, hormones and MAPK modules during abiotic stress signalling in plants. Biotechnol. Adv. 32, 2–11. doi: 10.1016/J.BIOTECHADV.2013.07.009
Sofo, A., Scopa, A., Nuzzaci, M., and Vitti, A. (2015). Ascorbate peroxidase and catalase activities and their genetic regulation in plants subjected to drought and salinity stresses. Int. J. Mol. Sci. 16, 13561–13578. doi: 10.3390/IJMS160613561
Vainonen, J. P., and Kangasjärvi, J. (2015). Plant signalling in acute ozone exposure. Plant Cell Environ. 38, 240–252. doi: 10.1111/PCE.12273
Vargas-Hernandez, M., Macias-Bobadilla, I., Guevara-Gonzalez, R. G., Romero-Gomez, S., de, J., Rico-Garcia, E., et al. (2017). Plant hormesis management with biostimulants of biotic origin in agriculture. Front. Plant Sci. 8:1762. doi: 10.3389/FPLS.2017.01762
Wiegant, F. A. C., de Poot, S. A. H., Boers-Trilles, V. E., and Schreij, A. M. (2012). Hormesis and Cellular Quality Control: a Possible Explanation for the Molecular Mechanisms that Underlie the Benefits of Mild Stress. Dose Response 11, 413–430. doi: 10.2203/DOSE-RESPONSE.12-030.WIEGANT
Xu, J., and Zhang, S. (2015). Mitogen-activated protein kinase cascades in signaling plant growth and development. Trends Plant Sci. 20, 56–64. doi: 10.1016/J.TPLANTS.2014.10.001
Xu, S., Yang, S.-Q., Yang, Y.-J., Xu, J.-Z., Shi, J.-Q., and Wu, Z.-X. (2016). Influence of linoleic acid on growth, oxidative stress and photosynthesis of the cyanobacterium Cylindrospermopsis raciborskii. N. Z. J. Mar. Freshw. Res. 51, 223–236. doi: 10.1080/00288330.2016.1197286
Xu, Z., Hua, N., and Godber, J. (2001). Antioxidant activity of tocopherols, tocotrienols, and gamma-oryzanol components from rice bran against cholesterol oxidation accelerated by 2,2′-azobis(2-methylpropionamidine) dihydrochloride. J. Agric. Food Chem. 49, 2077–2081. doi: 10.1021/JF0012852
Yaeno, T., Matsuda, O., and Iba, K. (2004). Role of chloroplast trienoic fatty acids in plant disease defense responses. Plant J. 40, 931–941. doi: 10.1111/J.1365-313X.2004.02260.X
Yakhin, O. I., Lubyanov, A. A., Yakhin, I. A., and Brown, P. H. (2017). Biostimulants in plant science: a global perspective. Front. Plant Sci. 7:2049. doi: 10.3389/fpls.2016.02049
Zduńska, K., Dana, A., Kolodziejczak, A., and Rotsztejn, H. (2018). Antioxidant Properties of Ferulic Acid and Its Possible Application. Skin Pharmacol. Physiol. 31, 332–336. doi: 10.1159/000491755
Keywords: ozone, ROS, rice bran, enzymatic extract, MAPK
Citation: Macias-Benitez S, Navarro-Torre S, CaballeroP, Martín L, Revilla E, Castaño A and Parrado J (2021) Biostimulant Capacity of an Enzymatic Extract From Rice Bran Against Ozone-Induced Damage in Capsicum annum. Front. Plant Sci. 12:749422. doi: 10.3389/fpls.2021.749422
Received: 29 July 2021; Accepted: 19 October 2021;
Published: 19 November 2021.
Edited by:
Maurizio Ruzzi, University of Tuscia, ItalyReviewed by:
George Komis, Palacký University Olomouc, CzechiaKamrun Nahar, Sher-e-Bangla Agricultural University, Bangladesh
Kundan Kumar, Birla Institute of Technology and Science, India
Copyright © 2021 Macias-Benitez, Navarro-Torre, Caballero, Martín, Revilla, Castaño and Parrado. This is an open-access article distributed under the terms of the Creative Commons Attribution License (CC BY). The use, distribution or reproduction in other forums is permitted, provided the original author(s) and the copyright owner(s) are credited and that the original publication in this journal is cited, in accordance with accepted academic practice. No use, distribution or reproduction is permitted which does not comply with these terms.
*Correspondence: Juan Parrado, cGFycmFkb0B1cy5lcw==
†These authors have contributed equally to this work and share first authorship