- 1Botany Area, Department of Molecular Biology and Biochemical Engineering, Universidad Pablo de Olavide, Seville, Spain
- 2Grainger Bioinformatics Center, Department of Science and Education, The Field Museum, Chicago, IL, United States
- 3Allan Herbarium, Manaaki-Whenua Landcare Research, Lincoln, New Zealand
- 4Department of Biology (Botany), Universidad Autónoma de Madrid, Madrid, Spain
- 5Centro de Investigación en Biodiversidad y Cambio Global (CIBC-UAM), Universidad Autónoma de Madrid, Madrid, Spain
- 6Instituto de Botánica Darwinion (ANCEFN-CONICET), San Isidro, Argentina
Carex subgenus Psyllophorae is an engaging study group due to its early diversification compared to most Carex lineages, and its remarkable disjunct distribution in four continents corresponding to three independent sections: sect. Psyllophorae in Western Palearctic, sect. Schoenoxiphium in Afrotropical region, and sect. Junciformes in South America (SA) and SW Pacific. The latter section is mainly distributed in Patagonia and the Andes, where it is one of the few Carex groups with a significant in situ diversification. We assess the role of historical geo-climatic events in the evolutionary history of the group, particularly intercontinental colonization events and diversification processes, with an emphasis on SA. We performed an integrative study using phylogenetic (four DNA regions), divergence times, diversification rates, biogeographic reconstruction, and bioclimatic niche evolution analyses. The crown age of subg. Psyllophorae (early Miocene) supports this lineage as one of the oldest within Carex. The diversification rate probably decreased over time in the whole subgenus. Geography seems to have played a primary role in the diversification of subg. Psyllophorae. Inferred divergence times imply a diversification scenario away from primary Gondwanan vicariance hypotheses and suggest long-distance dispersal-mediated allopatric diversification. Section Junciformes remained in Northern Patagonia since its divergence until Plio-Pleistocene glaciations. Andean orogeny appears to have acted as a northward corridor, which contrasts with the general pattern of North-to-South migration for temperate-adapted organisms. A striking niche conservatism characterizes the evolution of this section. Colonization of the SW Pacific took place on a single long-distance dispersal event from SA. The little ecological changes involved in the trans-Pacific disjunction imply the preadaptation of the group prior to the colonization of the SW Pacific. The high species number of the section results from simple accumulation of morphological changes (disparification), rather than shifts in ecological niche related to increased diversification rates (radiation).
Introduction
The Neotropic is one of the most biodiverse regions, containing seven of the 36 currently recognized biodiversity hotspots of Earth (Koenig, 2016), and is considered an evolutionary hub for research about the origin of biological diversity (Rull, 2008). Most available phylogeographical studies focusing on plants along South America (SA) have been primarily restricted to the Tropical Andes region (Muellner et al., 2005; Marcheli and Gallo, 2006; Acosta and Premoli, 2010; Table 1), while Patagonia remains among the least phylogeographically studied regions of SA despite having been affected more than other areas of the continent by historical environmental changes, both recently and over geological time scales (Rabassa, 2008; Sérsic et al., 2011). The interest in understanding the historical processes impacting Patagonian biota has been increasing in the last decades, with a special focus on animals (e.g., rodents, Kim et al., 1998; lizards, Morando et al., 2004, 2007; Breitman et al., 2012; fishes, Cussac et al., 2004; Ruzzante et al., 2008; birds, Calderón et al., 2014; Cadena et al., 2020). However, understanding of the evolutionary history and processes of plant diversification in this region has not bloomed until recently (Azpilicueta et al., 2009; Jakob et al., 2009; Tremetsberger et al., 2009; Cosacov et al., 2010; Sede et al., 2012; Nicola et al., 2014, 2019; Soliani et al., 2015; López and Bonasora, 2017; Table 1).
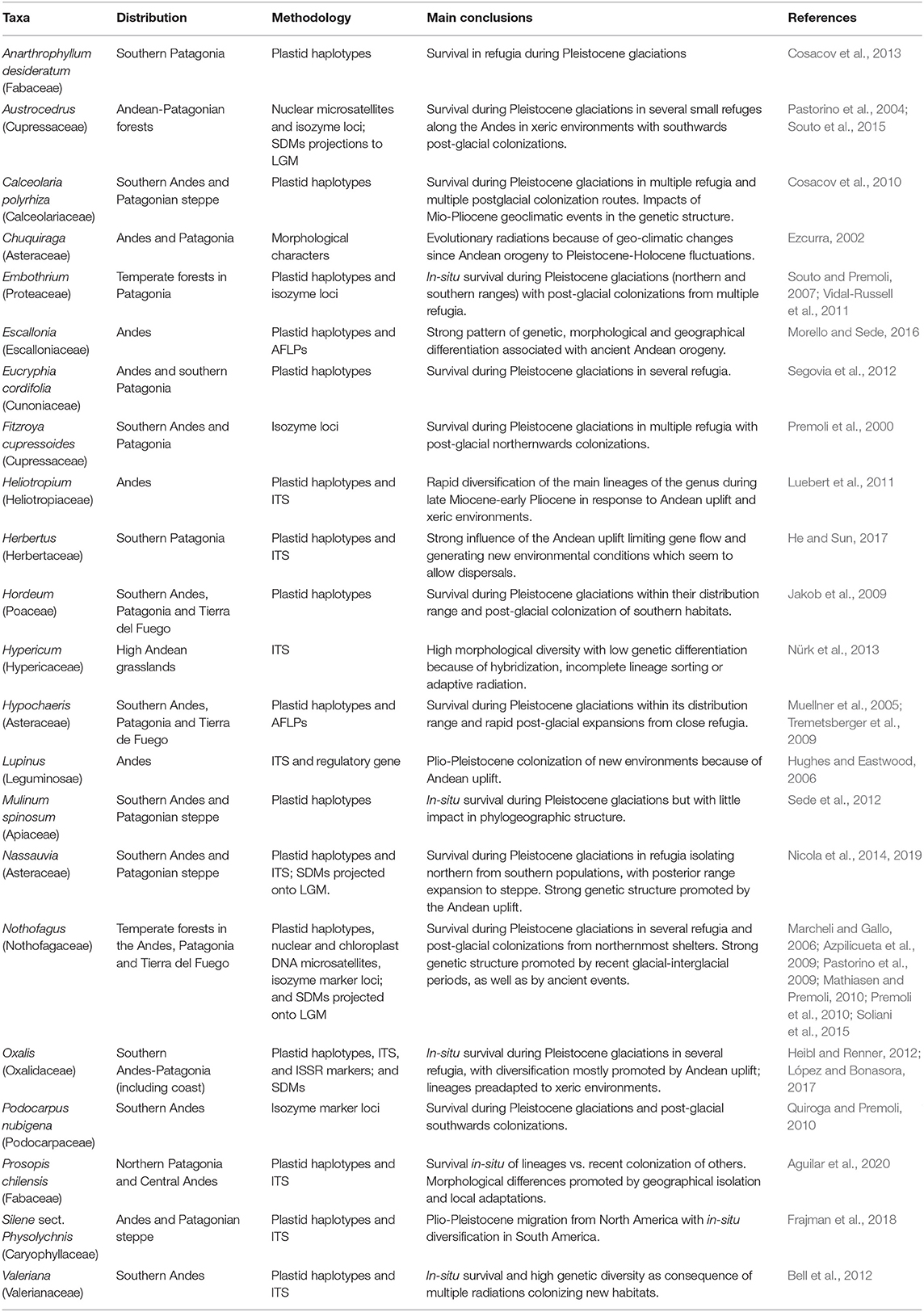
Table 1. Review of current South American phylogeographical knowledge of plants based on published articles with a focus on the Andes and Patagonia regions.
Different biogeographic affinities among SA and other landmasses (intercontinental disjunctions) have previously been described. One of the most intensely studied disjunctions has been the amphitropical pattern (including the bipolar disjunction). This involves taxa distributed at medium and high latitudes of both the hemispheres. It has mainly been explained through long-distance dispersal (LDD) likely by birds and dated back to cold periods of the Plio-Pleistocene (Simpson et al., 2005, 2017; Spalik et al., 2010; Villaverde et al., 2017a). Another less explored pattern is the trans-Caribbean disjunction. It is observed in organisms distributed in regions adjacent to the northern and southern shores of the Caribbean Sea. It has been associated with bird dispersal through the American-Atlantic flyway (Jiménez-Mejías et al., 2018, 2020). Another very striking disjunction pattern is that involving SA with New Zealand (NZ), the often so-called Gondwanan disjunction. In the 19th century, Hooker (1853) and Darwin (1859) discussed vicariance vs. dispersal-based explanations for organisms displaying disjunct distributions among SA, NZ, and also Africa (McLoughlin, 2001; Givnish et al., 2004; Specht, 2006). On the one hand, such distributions were often attributed to vicariance enabled by the tectonic dynamics of Gondwanan landmasses (Biffin et al., 2010; Noben et al., 2017). On the other hand, recent molecular dating studies have confirmed that LDD explains some of the Gondwanan distribution patterns better than vicariance-like scenarios, especially in plants (McGlone et al., 2001; Sanmartín and Ronquist, 2004). Accordingly, it has been shown that some species have reached both sides of the Southern Pacific (SA and NZ) by direct LDD in relatively recent times (Knapp et al., 2005; Otero et al., 2019). Alternatively, migration between these landmasses via stepping-stones across Antarctica has also been argued (de la de la Estrella et al., 2019). Just a few groups of ancient (Cretaceous) origin seem to have Gondwanan distributions explained by tectonic vicariance, such as Araucariaceae (Biffin et al., 2010; Kranitz et al., 2014).
Within SA, the Andean orogenesis had a great impact on species distribution (Antonelli et al., 2009). However, it was a slow uneven process, with the northern and southern parts of the range undergoing uplift at remarkably different times (middle Eocene-early Oligocene and Miocene-Pleistocene, respectively; Antonelli et al., 2009; Mora et al., 2020). Phylogeographic studies have shown that this mountain range acted on the one hand as a dispersal route in some groups of plants (Bell and Donoghue, 2005; Nürk et al., 2013), and on the other hand, as a barrier against vicariance triggering rapid diversification (Hughes and Eastwood, 2006). At the southernmost part of the continent, Patagonia was also dramatically affected by several geoclimatic events during the Cenozoic. The widening of the Drake Passage between the Southern Cone and Antarctica (49–17 million years ago, mya) caused changes in the ocean circulation intensifying the circum-Antarctic current, freezing Antarctica (ca. 30 mya; Cantrill and Poole, 2012), and subsequently cooling the surrounding landmasses. This may have facilitated the establishment and diversification of species with more cold-temperate climate preferences. From the late Oligocene to early Miocene, the shorelines of Patagonia underwent repeated shifting, continually creating new environmental opportunities, with the flatlands being repeatedly flooded by the sea in the form of marine transgressions and promoting the fragmentation of these areas (Encinas et al., 2018). At the end of the Cenozoic, the previously mentioned Andean orogeny in Patagonia introduced a cold and dry climate toward the east promoting the replacement of Miocene subtropical savannas by arid steppes, with taxa adapted to cooler xeric environments (tussock grasses and shrubs; Barreda and Palazzesi, 2007; Sede et al., 2012). This event also contributed to the retreat of ancient forests toward the more humid Andean slopes after the Patagonian desertification (Rabassa, 2008). In more recent times, different glaciations and associated climatic events have affected the distribution and divergence of species since the late Miocene (Ramos and Ghiglione, 2008; Martínez and Kutschker, 2011; Ponce et al., 2011; Breitman et al., 2012). The oldest known Patagonian glaciation took place around the Mio-Pliocene boundary (5–7 mya; Rabassa et al., 2005). This was subsequently followed by the large late Pliocene glaciations (ca. 3.5 mya; Rabassa et al., 2005). Later, the Quaternary glacial-interglacial cycles finished shaping present species distribution patterns (Markgraf et al., 1995; Hewitt, 2000; Rabassa, 2008). The most important was the Greatest Patagonian Glaciation (1–1.2 mya), exclusive to the Southern Cone, involving a large expansion of ice sheets in the Patagonian steppe. The Last Glacial Maximum (LGM, c. 18-21 ka) also influenced Patagonia and took place there and in the Northern Hemisphere, simultaneously (Rabassa et al., 2011). The absence of a continuous ice sheet during the LGM in southern SA and the occurrence of interglacial periods are associated with the movement of species following a more stable climate and the presence of refugia mostly north and east (Markgraf, 1983; Markgraf et al., 1995; Holderegger and Thiel-Egenter, 2009; Ponce et al., 2011). However, other studies point to several species presenting a reversed pattern, with refugia in higher (southern) latitudes with postglacial recolonizations to the north (Jakob et al., 2009; Tremetsberger et al., 2009; Cosacov et al., 2010; Sérsic et al., 2011; Breitman et al., 2012; Sede et al., 2012; Vera-Escalona et al., 2012; Frajman et al., 2018), which contrast with what is documented for the Northern Hemisphere (Hewitt, 2004). Even species endemic to Southern Patagonia (S Patagonia; incl. Tierra del Fuego) seem to have had glacial refugia exclusively in that same area during the Pleistocene (Muellner et al., 2005).
To illustrate the biogeographic history of this region and the implied speciation patterns, it is important to understand how geo-climatic events have interacted with the ecological requirements of plant species and how this has affected the evolution of lineages through time. Some species unable to adapt to new environmental conditions are forced to move in search of an environment with similar conditions to their original requirements (niche conservatism; Wiens and Graham, 2005; Kozak and Wiens, 2006; Losos, 2008; Wiens et al., 2010), while others are able to adapt to new environmental conditions, persisting in their original niche as the conditions change, or colonizing newly available habitats (niche shifts; Pearman et al., 2008; Spalink et al., 2016). Despite the undoubted role of geography and climate shaping distribution patterns in SA, the change in environmental niche seems to have also played a role in the diversification of species at more local and regional scales (Aguilar et al., 2020; Luebert et al., 2020). That is to say, geographical isolation could have triggered divergence of disjunct populations and eventual speciation via local adaptation (Frajman et al., 2018; Aguilar et al., 2020).
Carex L. (Cyperaceae) is one of the most diverse and widely distributed plant groups on Earth (ca. 2000 spp; Egorova, 1999; Ball and Reznicek, 2002), with a cosmopolitan distribution and only absent from Antarctica and a few tropical areas (Roalson et al., 2021). It has its highest species richness in cold-temperate regions of the Northern Hemisphere and inhabits a great diversity of habitats (Martín-Bravo et al., 2019). Subgenus Psyllophorae (Degl.) Peterm. is one of the main lineages of the genus Carex (Villaverde et al., 2020), but with a relatively small number of species (56 species according to Roalson et al., 2021) compared to other subgenera [Carex, Vignea (P. Beauv. ex T. Lestib.) Peterm.; >500 species, Roalson et al., 2021]. It displays a striking disjunct distribution (Figure 1) as is present in the Western Palearctic (sect. Psyllophorae Degl.), Afrotropical region (sect. Schoenoxiphium Nees), as well as SA and SW Pacific [sect. Junciformes (Boeckeler) Kük]. A fourth group (Curvula-clade) has been included in this subgenus but its placement still needs further study because of the extremely short branch supporting the group (Roalson et al., 2021). Subgenus Psyllophorae has been dated back to the late Oligocene with relatively deep nodes (Martín-Bravo et al., 2019). The group as a whole seems to match two remarkable distribution patterns. On the one hand, a Rand-Flora pattern (Mairal et al., 2017) is drawn between the Western Palearctic (sect. Psyllophora) and Afrotropical (sect. Schoenoxiphium) distribution. On the other hand, a reminiscent pattern of Gondwanan distribution (Sanmartín and Ronquist, 2004) is suggested for the circum-Antarctic disjunction between SA and SW Pacific (sect. Junciformes), and Tropical Africa (sect. Schoenoxiphium).
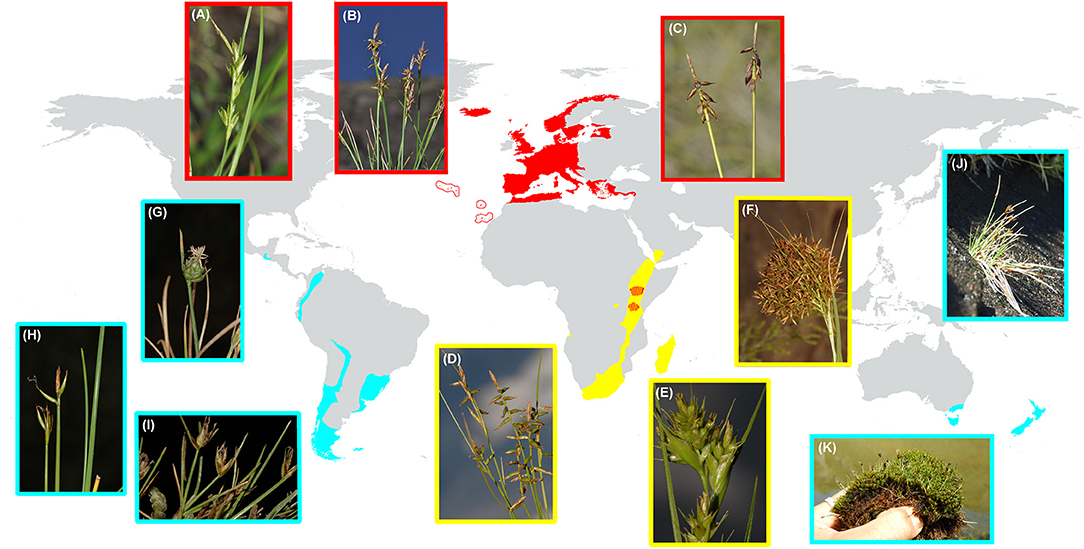
Figure 1. Distribution map of Carex subg. Psyllophorae including its three sections: (1) Psyllophorae in Western Palearctic; (2) Schoenoxiphium in the Afrotropical region; and (3) Junciformes in SA and SW Pacific. The colors of each section are according to Figure 2A (AAR analysis). Photographs display the morphological variation in each section: (A–C sect. Psyllophorae) (A) C. distachya Desf., Spain, Ávila, Arenas de S. Pedro, (B) C. macrostyla Lapeyr., Spain, Lerida, Aran Valley, (C) C. pulicaris L., W Iceland; (D–F sect. Schoenoxiphium), (D) C. killickii Nelmes, Lesotho, Roma-Semonkong, (E) C. dregeana Kunth, South Africa, Drakensberg, Cathedral Peak, (F) C. multispiculata Luceño and Martín-Bravo, South Africa, Kwazulu-Natal, Cathedral Peak; (G–K sect. Junciformes) (G) C. andina Phil, Chile, Santiago, Nevado Valley, (H) C. camptoglochin V. I. Krecz., Chile, Punta Arenas, (I) C. vallis-pulchrae var. barrosiana G. A. Wheeler, Argentina, Tierra del Fuego, Ushuaia, (J) C. acicularis Boott, NZ, Eyre mountains, (K) C. enysii Petrie, NZ, Harris mountains. AAR, ancestral area reconstruction, NZ, New Zealand.
Section Junciformes include 28 species (Roalson et al., 2021). It is mostly endemic to SA, with its center of diversity in Patagonia (Barros, 1935, 1969; Moore, 1968, 1983), few taxa reaching tropical latitudes through the Andes (Wheeler and Guaglianone, 2003; Jiménez-Mejías and Roalson, 2016) and C. phalaroides Kunth entering marginally in Central America (Jiménez-Mejías et al., 2018). In addition, this section is also distributed in SW Pacific (SE Australia, Tasmania, and NZ). It was previously treated as two old different sections mixed in distribution (sects. Aciculares G. A. Wheeler and Junciformes s.s.) due to apparent morphological differences (Wheeler, 1989). In addition, two species of uncertain phylogenetic position have also been recently ascribed to the current sect. Junciformes (C. phalaroides and C. camptoglochin V. I. Krecz; Jiménez-Mejías et al., 2016a; Roalson et al., 2021). This group is especially interesting from an evolutionary and biogeographical point of view taking into account its disjunction distribution in the Southern Hemisphere, and the fact that it is the second-largest Carex section in SA [after sect. Uncinia (Pers.) Baill.; Jiménez-Mejías et al., 2018]. Both groups, together with sect. Fecundae Kük., constitute the only examples of significantly speciose Carex groups (more than 15 species) in SA. The origin of sect. Junciformes has been dated back to the Early Miocene (c. 17 mya; Martín-Bravo et al., 2019), making it also one of the oldest sections of the genus. Species of this group mostly inhabit dry and cold habitats, such as steppe grasslands, fellfields, and rocky outcrops, with fewer species inhabiting mesic and humid environments like bogs, swamps, or forest understories (Barros, 1969; Moore and Edgar, 1970; Wheeler, 1989; Curtis and Morris, 1994).
Carex subg. Psyllophorae represents an ideal group to study the biogeographic history and evolution of the genus in the Southern Hemisphere, in contrast to its predominant diversification in the Northern Hemisphere. The relatively old origin of the subgenus added to its remarkable distribution pattern makes it an interesting case study to evaluate the role of the different geo-climatic events shaping the diversity of the group, paying special attention to the colonization processes resulting in its currently Gondwanan/Rand-Flora reminiscent disjunct distribution pattern as well as diversification of Carex in SA. In particular, we will assess whether the relatively large number of species of sect. Junciformes in SA matches any of the types of evolutionary radiation (Simões et al., 2016) and, if so, what triggers could be related to such radiation. Finally, we will try to elucidate the process originating the remarkable disjunction between SA and SW Pacific. To this end, we will perform phylogenetic, biogeographic, and bioclimatic niche evolution analyses.
Materials and Methods
Sampling, DNA Amplification, and Alignment
We mainly relied on herbarium materials from 15 herbaria (A, BR, CHR, CONC, GOET, E, M, MA, MO, MSB, NY, SI, UPOS, and WS; codes according to Thiers, 2020) to study 45 species (49 taxa) belonging to subg. Psyllophorae (Supplementary Table 1): 22 species plus four varieties from sect. Junciformes (all except C. archeri Boott, C. boelckeiana Barros, and C. moorei G. A. Wheeler); all 7 species of sect. Psyllophorae; and 17 included in sect. Schoenoxiphium (all except C. acocksii C. Archer, C. chermezonii Luceño and Martín-Bravo, C. gordon-grayae Luceño, Márq.-Corro and Sánchez-Villegas, and C. sciocapensis Luceño, Márq.-Corro and Sánchez-Villegas; see Luceño et al., 2021 for more information). Our dataset comprises 87% of all the extant diversity known for subg. Psyllophorae (following Roalson et al., 2021 with some modifications; 53 species) including all main lineages and representing the geographical and morphological variability of the subgenus. Carex phalaroides s.l. constitutes a complex species much in need of detailed study; so we opted for a synthetic view and considered it as a single species (herein C. gibertii G. A. Wheeler, C. moesta Kunth, and C. hypsipedos C. B. Clarke are treated as C. phalaroides). We used as outgroup one or two representatives of each of the other five Carex subgenera (Villaverde et al., 2020): C. hypolytroides Ridl. and C. siderosticta Hance (subg. Siderostictae Waterway), C. canescens L. and C. gibba Wahlenb. (subg. Vignea), C. flava L. and C. dissitiflora Franch. (subg. Carex), C. arctogena Harry Sm. (subg. Euthyceras Peterm.), and C. meridensis (Steyerm.) J. R. Starr (subg. Uncinia Pers.).
We used four DNA regions, the nrDNA ITS and ETS, and the ptDNA matK and rps16. These markers were selected because they have been used successfully in previous studies in Carex in general and in subg. Psyllophorae in particular (Gehrke et al., 2010; Jiménez-Mejías et al., 2016a; Villaverde et al., 2017b; Márquez-Corro et al., 2020). DNA extraction and sequence amplification followed Jiménez-Mejías et al. (2016a), except for rps16 amplification that was performed as indicated in Shaw et al. (2005). All PCR products were sequenced by Macrogen (Madrid, Spain). Sequence chromatograms were manually edited using Geneious v. 11.0.2 (Biomatters Ltd., Auckland, New Zealand).
Four independent matrices were compiled, each one containing sequences for one of the DNA regions (ITS, ETS, matK, or rps16). The sequences were automatically aligned with Muscle v.3.8.425 (Edgar, 2004) and alignments were manually corrected in the flanking regions of indels. Informative indels were coded as a binary character using SeqState v.1.4.1. according to Simmons and Ochoterena (2000) simple coding method. A complete multiaccession matrix (105 accessions, 23.26% missing data) was built concatenating all markers.
Phylogenetic Dating and Diversification Rate Analyses
We performed maximum likelihood (ML) and Bayesian Inference (BI) phylogenetic analyses using RAxML v.8.2 (Stamatakis, 2014) and MrBayes v.3.2 (Ronquist et al., 2012), as implemented in CIPRES Science Gateway (Miller et al., 2010), with parameters detailed in Supplementary Data 1.
We inferred divergence times in subg. Psyllophorae with a Bayesian analysis implemented in BEAST v.1.10 (Suchard et al., 2018) establishing three primary calibration points based on reliable Carex fossils (Jiménez-Mejías et al., 2016b): C. colwellensis (Eocene: 38.0-33.9 mya) for the crown node of the genus, C. marchica (Early Miocene: 23.0-16.0 mya) and C. hartauensis (late Oligocene: 28.1-23 mya) for the stem node of subg. Vignea and Carex, respectively (Table 2). We also used a secondary calibration point for the crown node of subg. Psyllophora (24.41 mya; Martín-Bravo et al., 2019). Prior node age distributions and analyses settings are detailed in Supplementary Data 1.
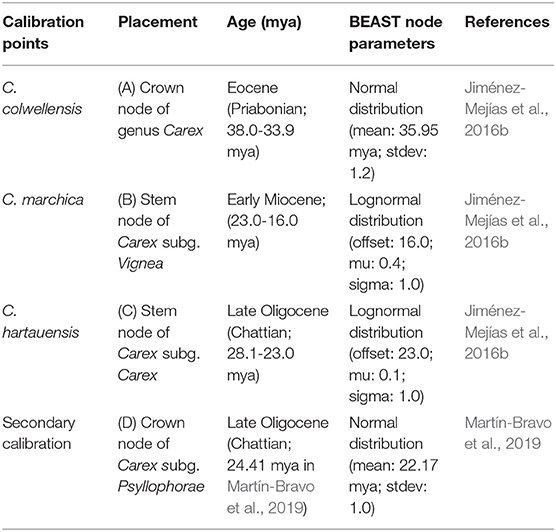
Table 2. Three calibration points based on fossils and a secondary one were used to date the phylogenetic tree of Carex subg. Psyllophorae using BEAST.
Diversification rates for subg. Psyllophorae were estimated on the dated singleton tree using the speciation-extinction model implemented in BAMM (BAMMtools package; Rabosky et al., 2014) using R v.3.6 (R Development Core Team, 2019). This analysis was performed in order to estimate the diversification rates by the effective sample size of the log-likelihood and number of shift events along branches using reversible jump Markov chain Monte Carlo (rjMCMC) to infer sample mixtures of distinct evolutionary rate dynamics across the branches. Four chains of 1.000.000 generations, saving trees each 1,000 (see Supplementary Data 1 for more information) were performed.
Bioclimatic Niche Analyses
Occurrences belonging to sect. Junciformes (Supplementary Table 2) were downloaded from GBIF (https://www.gbif.org/), iDiGBio (https://www.idigbio.org), and the ALA database (https://www.ala.org.au/). In addition, we manually georeferenced 154 vouchers from 14 herbaria (A, CONC, E, LIL, M, MICH, MO, MSB, NY, SI, TRIER, UPOS, US, and WS), as well as 115 records obtained from literature sources (Barros, 1948, 1950, 1957; Wheeler, 1988, 1989, 1998; Wheeler and Muñoz-Schick, 1990; Wheeler and Guaglianone, 2003; Wheeler and Beck, 2011; Jiménez-Mejías et al., 2020). We cleaned the resulting database by removing duplicates and unreliable records after visual inspection, and finally, 616 occurrences were recovered (Supplementary Table 2).
We retrieved 37 bioclimatic and ecophysiological variables from WorldClim2 (Fick and Hijmans, 2017) and Envirem (Title and Bemmels, 2018) to characterize the bioclimatic niche of our study group. Our variable selection procedure (Supplementary Data 1) retained five bioclimatic variables that contributed more than the rest to the variability of our data, and have easy biological interpretation for our species: Annual mean temperature (AMT), temperature annual range (TAR), mean temperature of the driest quarter (MTDQ), annual precipitation (AP), and terrain roughness index (TRI). These variables illustrate species-climatic limits regarding water availability (AP), temperature optimum (AMT), and limits (TAR, MTDQ), as well as microclimatic determinants driven by topography (TRI). We conducted a principal component analysis (PCA) of the retained variables using the package ggplot2 (Wickham, 2006) and the function prcomp in R for visualizing the environmental space of sect. Junciformes.
We performed ancestral state reconstruction of ecological preferences for sect. Junciformes using the dated pruned singleton tree and the mean value for each retained variable in each species as implemented in the package phytools in R (Revell, 2012; Figure 2A). We previously assessed two models of continuous trait evolution [Brownian motion (BM) and Ornstein-Uhlenbeck (OU)] and selected the best using corrected Akaike Information Criterion (AICc) (fitContinuous function in geiger package; Harmon et al., 2008).
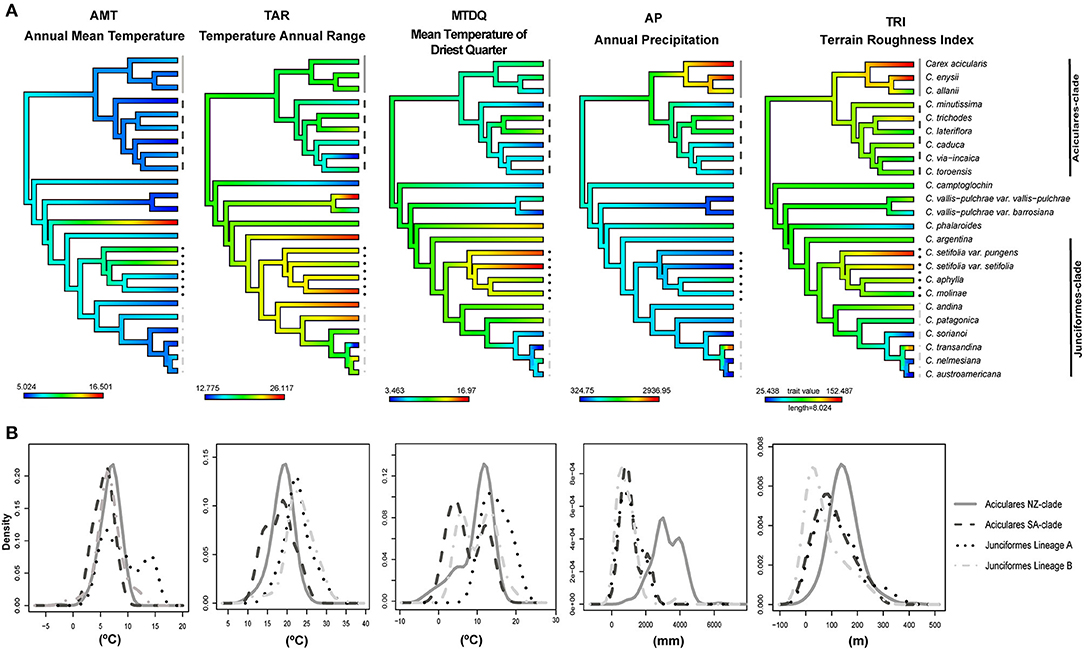
Figure 2. (A) Bioclimatic niche reconstruction at ancestral nodes for five continuous environmental variables of the calibrated tree for the Carex sect. Junciformes. The ML reconstruction is represented as gradational colors along the branches. Higher values are displayed in red, intermediate in green, and low in blue. (B) Comparison of density plots expressing the frequency of distribution for the same uncorrelated variables in the four clades belonging to Carex sect. Junciformes. These clades are also represented in Figure 3C to the right of the phylogeny. ML, maximum likelihood.
In order to study fine-level ecological differences within sect. Junciformes, we obtained and plotted response curves for each retained variable for the main sister lineages (Figure 2B) and single species (Supplementary Figures 1, 2). To characterize the environmental space for a whole lineage, we merged all occurrences of the corresponding species. To further evaluate the Phylogenetic Niche Conservatism hypothesis between sister lineages from SA and NZ within the Aciculares-clade (see Results), we also performed tests of similarity and equivalence (Warren et al., 2008; Broennimann et al., 2012) implemented in the package ecospat (Di Cola et al., 2017). We also measured the niche overlap between these sister clades with Schoener's D index (Schoener, 1968).
Biogeographic Analyses
Ancestral area reconstruction (AAR) was performed using the package BioGeoBEARS (Matzke, 2014a) in R. We performed two different biogeographic reconstructions focusing on two different evolutionary-geographic scales. First, we performed a large-scale analysis based on our complete sampling for the whole subg. Psyllophorae, coding species distribution according to six biogeographical regions (Figure 3A): North America, SA, Eastern Palearctic, Western Palearctic, Afrotropical region, and Australasia (including NZ). For species in subg. Psyllophorae, we obtained distributions from the World Checklist of Selected Plant Families (WCSP) (Govaerts et al., 2020), while for each outgroup tip, the coded distribution was obtained from the ancestral area of the correspondent subgenus as inferred by Martín-Bravo et al. (2019). Second, we focused only on sect. Junciformes to explore in detail its biogeography, delimiting subregions within the Neotropics and NZ, based on their current (Supplementary Figure 3) and inferred present potential distribution (Supplementary Figure 4; see Supplementary Data 1 for methodological details). As a result, we considered seven areas (Figure 3C): Northern Andes, Central Andes, Northern Patagonia (N Patagonia), S Patagonia, Atlantic South America, Falklands, and NZ. As input data, we used the pruned calibrated tree and a file recording the presence/absence of species in each area.
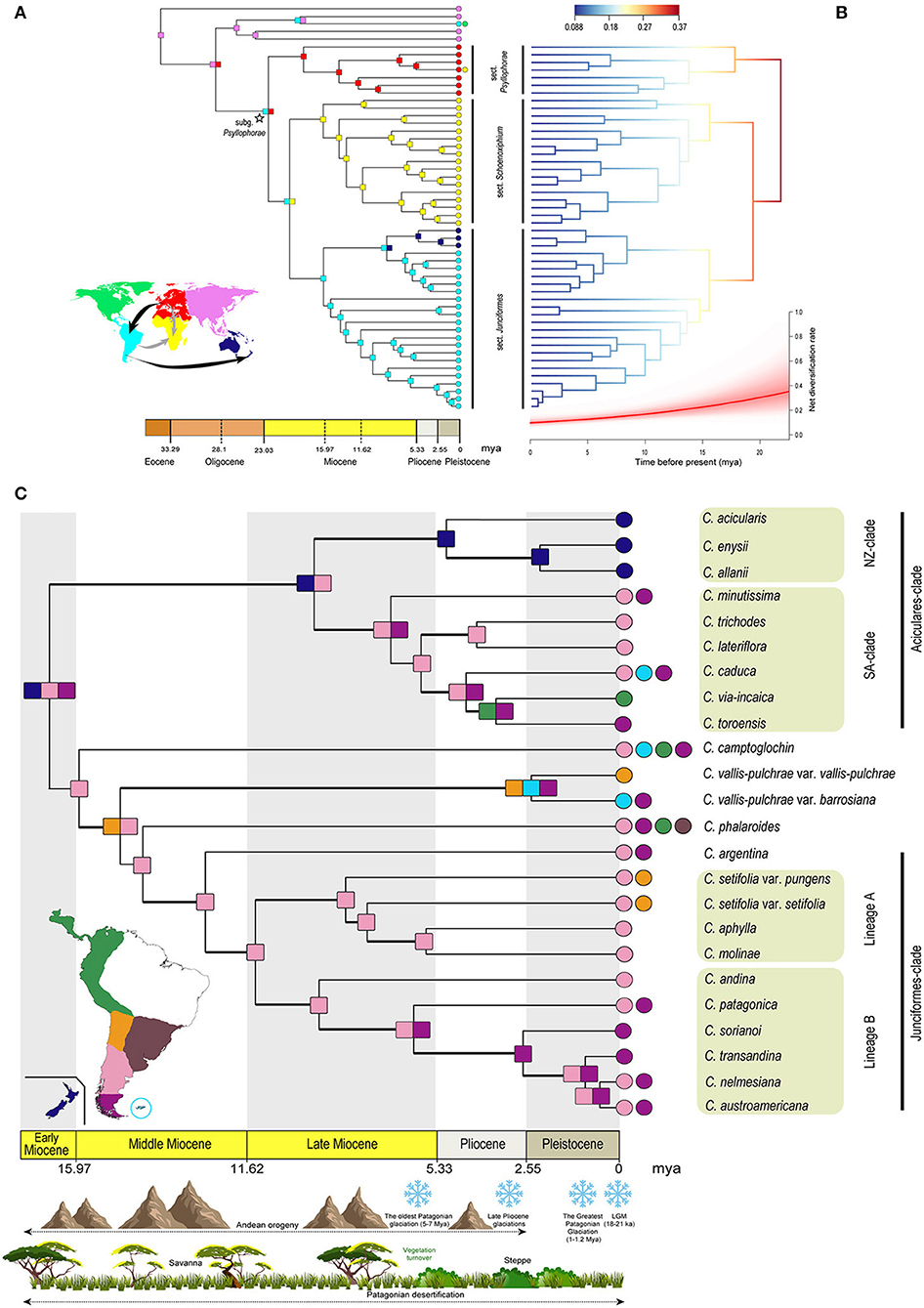
Figure 3. (A) Chronogram based on the dated phylogeny of Carex subg. Psyllophorae. Square nodes and circle terminals with different colors represent the most probable area or combination of areas inferred by the DIVA-like model in the AAR using BioGeoBEARS. Colors are according to the world map representing the regions coded for the biogeographic analysis. Arrows in the map show the direction of migration among the different regions inferred by the AAR. Arrows in black color display a clear colonization route inferred by the AAR, while ones in gray color display uncertainty. Two crossed lines on the gray arrow from Western Palearctic to the Afrotropical region display an uncertain dispersal or vicariance pattern between both the continents. (B) Phylorate plot from the analysis of diversification rate using BAMM, based on the dated phylogeny of Carex subg. Psyllophorae (excluding outgroup). Tree branch color indicates the model-averaged net diversification rates along the branches. Below is represented the net diversification rate through time with a red line displaying the mean and the shaded range of its rjMCMC confidence interval. (C) Chronogram based on the dated phylogeny including only Carex sect. Junciformes. Square nodes and circle terminals with different colors represent the most probable area or combination of areas inferred by the DEC model in AAR specifically performed for SA and NZ. Colors are according to the map representing the South American and NZ regions coded for the biogeographic analysis. The main historical geo-climatic events since the origin of this group are shown with representative drawings in the timeline. Bold branches represent well-supported nodes (PP > 0.9). DIVA, dispersal-vicariance analysis; AAR, ancestral area reconstruction; BAMM, Bayesian Analysis of Macroevolutionary Mixtures; rjMCMC, reversible jump Markov chain Monte Carlo; DEC, dispersal-extinction cladogenesis; SA, South America; NZ, New Zealand.
Both datasets were analyzed under three different models: Dispersal-Extinction-Cladogenesis (DEC; Ree and Smith, 2008), a likelihood version of the Dispersal-Vicariance analysis (DIVA-like; Ronquist, 1997), and a likelihood interpretation of BayArea model (Landis et al., 2013) implemented in BioGeoBEARS (BAYAREA-like; Matzke, 2014a), and each of these in combination with the founder-event speciation (+J model; Matzke, 2014b). The fit of the different models was tested using AICc (Burnham and Anderson, 2002).
Results
Phylogenetic, Divergence Time and Diversification Analyses
Subgenus Psyllophorae was recovered in a strongly supported monophyletic group (Supplementary Figure 5) including sects. Psyllophorae, Schoenoxiphium, and Junciformes, which also formed well-supported clades. Section Psyllophorae (Clade A) was sister to Clade B which in turn contained sects. Junciformes and Schoenoxiphium as sister groups (see Supplementary Data 2 for a detailed description of phylogenetic results).
Divergence times obtained with BEAST are shown in Supplementary Figure 6. The crown age of subg. Psyllophorae coincided with the early Miocene (mean = 22.55 mya, 95% highest probability density (HPD) = 20.76–24.44 mya). The most recent common ancestors (MRCA) of sect. Psyllophorae (Clade A; mean = 18.40 mya, 95% HPD = 15.01-21.56 mya) and sects. Schoenoxiphium and Junciformes (crown node of Clade B; mean = 20.04 mya, 95% HPD = 17.25-22.84 mya) were inferred to have diversified just after the subgenus as a whole, still in the early Miocene. Interestingly, both sections from the Southern Hemisphere were originated almost at the same time in the early Miocene (Clade B1: sect. Schoenoxiphium, mean = 16.10 mya, 95% HPD = 12.5-19.47, and Clade B2: sect. Junciformes, mean = 16.05 mya, 95% HPD = 12.86-19.17 mya). Within sect. Junciformes, we found two main clades diversified in late Miocene (Aciculares-clade, mean = 8.60 mya, 95% HPD = 5.6–11.89 mya; core Junciformes, mean = 10.25 mya, 95% HPD = 7.89–12.82 mya). On the one hand, Junciformes-clade (including the monospecific lineage of C. argentina) seems to have diversified during the middle Miocene (mean = 11.66 mya, 95% HPD = 9.04–14.38 mya). The MRCA of core Junciformes was dated to the late Miocene (mean = 10.25 mya, 95% HPD = 7.89–12.82 mya), and in turn, the Lineages A and B derived from this ancestor were also inferred to have differentiated in the late Miocene (Lineage A: mean = 7.71 mya, 95% HPD = 5.6-10 mya; Lineage B: mean = 8.46 mya, 95% HPD = 6.14-10.9 mya). On the other hand, within Aciculares-clade, the diversification of SA-clade was during the late Miocene (mean = 6.44 mya, 95% HPD = 4.06–8.95 mya), whereas NZ-clade probably occurred a bit later during early Pliocene (mean = 4.87 mya, 95% HPD = 2.79–7.28 mya).
Bayesian Analysis of Macroevolutionary Mixtures (BAMM) analyses estimated a similar and constant diversification pattern along the three sections (Psyllophorae, Schoenoxiphium, and Junciformes; Figure 3B) of subg. Psyllophorae. In addition, it did not detect significant shifts in diversification rate.
Bioclimatic Niche Analyses
The PCA (Supplementary Figure 7) of the variables using mean values for all occurrences per species of sect. Junciformes displayed a clear trend toward variables related to temperature along the first principal component, which explained 42.1% of the total variance. Precipitation (AP) and terrain roughness (TRI) were negatively correlated with this axis and displayed lower values than temperature ones, while they contributed positively to the second principal component that showed 32.4% of the total variance explained. Therefore, the cumulative proportion of the variance explained by both principal components was 74.5%.
The best evolutionary model for bioclimatic reconstruction over sect. Junciformes phylogenetic tree according to the corrected AICc value was the Ornstein–Uhlenbeck model (BM = 112.10, log-likelihood = −53.76; OU = −34.99, log-likelihood = 20.76). The bioclimatic niche evolution of the five selected variables on the obtained phylogeny is shown in Figure 2A, as well as density functions exhibiting the frequency of the distribution of the bioclimatic variables in Figure 2B. In general, similar values across internal nodes of the phylogeny were retrieved for each independent variable (Figure 2A). This is also depicted by the mostly overlapping and relatively narrow peaks for the different sister clades (Aciculares-SA vs. NZ; Junciformes lineages A vs. B; see also Supplementary Figures 1, 2) in the response curves (Figure 2B). The deep nodes along the phylogeny displayed medium values on their bioclimatic preferences, while tips revealed the existence of more extreme values (Figure 2A).
Despite the general scenario of relatively uniform bioclimatic values across sect. Junciformes, some degree of heterogeneity was observed for certain variables in particular lineages. Thus, TAR was the variable that showed the highest environmental heterogeneity along the phylogeny, with contrasting bioclimatic preferences for Junciformes-clade (C. andina Phil., C. molinae Phil., C. patagonica Speg., and C. setifolia Kunze). The monospecific lineage of C. phalaroides showed high values for AMT in comparison to low-medium values in the rest of the species of sect. Junciformes. In addition, closely related species of Aciculares NZ-clade presented contrasting bioclimatic preferences across different variables, such as AP and TRI, as well as TAR and TRI for some species belonging to Junciformes-clade (C. setifolia and C. transandina). Finally, the high values displayed in the irregularity of terrain (TRI) were associated with species that inhabit a wider altitude range across NZ and SA. In addition, C. setifolia revealed higher values of MTDQ in comparison to medium-lower ones for the other tips. Otherwise, C. transandina G. A. Wheeler displayed contrasting values for AP and TRI, more similar to species from Aciculares NZ-clade than Junciformes Lineage B to which it is phylogenetically more related (Figure 2A and Supplementary Figure 2).
The PCA comparing the e-space for NZ and SA clades (Aciculares-clade) revealed a large overlap between both groups within both principal components (67% explained of the total variance; Supplementary Figure 8). This niche overlapping was also revealed by Schoener's D index (D = 0.523). In addition, pairwise statistical comparison for similarity and equivalence tests suggested that these clades were also significantly more similar and equivalent than expected by chance (p < 0.01).
Biogeographic Analyses
Dispersal-vicariance analysis (DIVA-like model) yielded lower corrected AICc values than DEC and BAYAREA-like model (76.84, 81.15, and 111.8, respectively), with ΔAICc > 2 (Burnham and Anderson, 2002). DIVA-like and DEC models resulted in biologically congruent reconstructions. However, the BAYAREA-like model displayed scarce uncertainty for node inferences although this model is more limited in the number of evolutionary scenarios considered (i.e., vicariance is not considered). In order to simplify the results, we mainly focused on the DIVA-like model (Figure 3A). Results from DEC and BAYAREA-like models are presented in Supplementary Figures 9, 10, respectively. The stem node of subg. Psyllophorae (Oligocene) was restricted to the Palearctic, from where it dispersed to SA (crown node widespread in the Western Palearctic and SA, early Miocene). The differentiation of sect. Psyllophorae took place in the Western Palearctic from the early Miocene. For the clade embracing sects. Schoenoxiphium and Junciformes, the ancestor was recovered as widespread in the Afrotropical region and SA during the early Miocene. The differentiation of each of these two sections implied the constriction of their crown node to one of the two landmasses. While the arrival to SA necessarily implied a LDD event from the Old World, the early Miocene colonization of the Afrotropical region remains obscure, since we cannot rule out the dispersal from SA or dispersal/vicariance from the Western Palearctic. Finally, a LDD event to SW Pacific from SA across the Mio-Pliocene boundary led to the more recent diversification of the NZ-clade (Figure 3A). Within-area lineage diversification from the middle Miocene onwards was inferred for the remaining subclades within the three sections commented above (Figure 3A and Supplementary Figures 9, 10).
Regarding the biogeographic reconstruction for sect. Junciformes, the DEC model yielded lower AICc values than the DIVA-like and BAYAREA-like models (141.2, 142.4, and 143.3, respectively), although with ΔAICc <2 between DEC and DIVA-like models. Thus, we decided to focus on the DEC model (Figure 3C). Results for DIVA-like and BAYAREA-like models are presented in Supplementary Figures 11, 12, respectively. A widespread ancestral area including Patagonia as a whole and NZ was inferred for the crown node of sect. Junciformes at the late early Miocene, although uncertainty was present under the three biogeographic models tested with ranges probabilities below 0.10 (Figure 3C and Supplementary Figures 11, 12). DIVA-like model inferred N Patagonia, while BAYAREA-like model inferred both N-S Patagonia and Central Andes.
In the Aciculares-clade and the DEC model, a widespread area in N Patagonia and NZ was inferred for the MRCA (late Miocene). On the one hand, a LDD event from N Patagonia gave rise to the NZ-clade at the Mio-Pliocene boundary. On the other hand, from the late Miocene onwards, the diversification of SA-clade took place within N-S Patagonia, with at least two later Plio-Pleistocene independent range expansions (to the Falklands -C. caduca Boott- and Northern Andes -C. via-incaica Jim. Mejías and Roalson-). Remarkably, a similar scenario of Patagonian diversification was inferred for the Junciformes-clade, although starting earlier (from the Middle Miocene) than in the Aciculares-clade, and restricted to the N Patagonia. Subsequent expansions took place southwards to the S Patagonia (Pleistocene; Lineage B), and northwards to the Central Andes (from the Late Miocene; C. setifolia). The isolated lineages of C. camptoglochin, C. vallis-pulchrae, and C. phalaroides underwent unprecedented range expansions within the section, including multiple extra-Patagonian regions.
We preferred models not accounting for the J parameter for the AAR analyses as these results should be treated with caution (Ree and Sanmartín, 2018).
Discussion
Systematic Implications for Subg. Psyllophorae
Our sanger-based phylogenetic findings suggest that subg. Psyllophorae, as delimited by Roalson et al. (2021), is a well-supported monophyletic group (Supplementary Figure 5), but not including C. baldensis L. and C. curvula All. The placement of these two species in this subgenus needs further study and it was considered provisional by Roalson et al. (2021) as they were placed on a very short branch in the phylogenomic reconstructions (Villaverde et al., 2020). Three well-supported major clades are identified within subg. Psyllophorae, each one representing a section (Psyllophorae, Schoenoxiphium, and Junciformes), unlike previous phylogenies where lower taxonomic and molecular sampling yielded less robust reconstructions (Jiménez-Mejías et al., 2016a; Martín-Bravo et al., 2019). The prolonged isolated evolution of some species (Supplementary Figure 6) has yielded a remarkable morphological differentiation within sections (e.g., C. distachya, C. camptoglochin, and C. phalaroides), which has traditionally caused taxonomic problems in their sectional delimitation (Wheeler and Guaglianone, 2003; Luceño, 2008; Silveira and Longui-Wagner, 2012). The monophyly of sect. Schoenoxiphium is well-supported (Clade B1; Supplementary Figure 5), which agrees with previous studies focusing on that group (Luceño et al., 2021, and references therein) despite resolution problems reported for its inner phylogenetic structure. Meanwhile, C. camptoglochin and C. phalaroides are nested within sect. Junciformes clade, supporting their treatment within that section (Roalson et al., 2021). Section Aciculares as conceived by Wheeler (1989) is not monophyletic due to the position of C. transandina and C. vallis-pulchrae with respect to the members of sect. Aciculares (Supplementary Figure 5). As currently circumscribed, sect. Junciformes is primarily characterized by the presence of inflorescences formed by a single androgynous spike, usually dense, and with a staminate tip concealed by the female part. The only exception is C. phalaroides, with multispicate inflorescences, which seems a reversion to the ancestral state of the genus (Roalson et al., 2021). In addition, C. camptoglochin, which displays reflexed utricles with a protruding rachilla that may play an epizoochorous role (Villaverde et al., 2017a), has been traditionally included in sect. Leucoglochin Dumort. primarily because of this distinctive morphological character (Wheeler and Guaglianone, 2003).
Large-Scale Biogeography in Subg. Psyllophorae: Rand-Flora vs. Gondwanan Patterns
Subgenus Psyllophorae is inferred as one of the oldest lineages of Carex, dated to the early Miocene (22.55 mya), with previous studies retrieving even slightly older ages around the Mio-Oligocene boundary (24.4 mya; Martín-Bravo et al., 2019). In congruence with the origin and early diversification of Carex in the Eastern Palearctic (Martín-Bravo et al., 2019), our results point to an ancient migration from the Northern to the Southern Hemisphere, specifically from the Palearctic to SA (Figure 3A). Thus, although the biogeographic reconstruction inferred the Western Palearctic and SA continent as the ambiguous ancestral area for the crown node of subg. Psyllophorae, the immediate parent node was inferred to be exclusively distributed in the Northern Hemisphere (Eastern Palearctic or Western Palearctic). In a previous reconstruction using a representative sampling for the whole genus, the Western Palearctic was revealed as the origin of subg. Psyllophorae (Martín-Bravo et al., 2019).
Allopatric differentiation is suggested for the three main sectional lineages of subg. Psyllophorae originating in different regions (sect. Psyllophorae in the Western Palearctic, sect. Schoenoxiphium in the Afrotropical region, and sect. Junciformes in SA) and diversifying almost exclusively within each one (except sect. Junciformes, which also colonizes the SW Pacific for more than 15 million years (Figure 3A). A remarkably synchronous timing of the origin was inferred for these three main lineages (16–18 mya).
As expected, a primary (tectonic) Gondwanan disjunction has to be ruled out for the Southern Hemisphere lineages (sects. Schoenoxiphium and Junciformes) because their ages long postdate the split of the southern supercontinent (c. 16 mya vs. 135–105 mya; McLoughlin, 2001), unlike what is found in other, much older groups of plants (Araucariaceae, Biffin et al., 2010; Dicksonia, Noben et al., 2017). It is unclear whether the Afrotropical region was colonized from SA by LDD or from the Western Palearctic (Figure 3A). In the latter case, since this colonization occurred in the early Miocene (c. 20 mya) the disjunction is probably too old to fit a climate-driven Rand-Flora vicariance (Mairal et al., 2017), while LDD may not be ruled out (Míguez et al., 2017). Moreover, the origin of sect. Schoenoxiphium seems to be in central Southern Africa, which also dismisses the Rand-Flora pattern (Márquez-Corro et al., 2020). An additional, more recent (probably Pleistocene) colonization of the Afrotropical region from the Western Palearctic by LDD can be identified within sect. Psyllophora involving C. peregrina Link (Figure 3A). Remarkably, this constitutes the only recent out-of-continent dispersal within the subgenus.
Phylogeography of Sect. Junciformes: Diversification in South America and Colonization of New Zealand
The diversification of sect. Junciformes within SA started close to their origin in the middle Miocene and was characterized by relatively constant cladogenesis (especially in the Junciformes-clade), while diversification rate decreased over time (Figures 3B,C). The group remained confined and diversified in N Patagonia for several million years (Figure 3C). This long-permanence and within-area diversification is known in other groups at comparative evolutionary levels (Mathiasen and Premoli, 2010; Otero et al., 2019; Benítez-Benítez et al., 2021). The persistence of the group in N Patagonia and lack of evidence of early presence at lower latitudes suggest that sect. Junciformes colonized the Southern Cone from the Northern Hemisphere by direct LDD from the Western Palearctic (Figures 3A,C). Contrastingly, most cold-adapted Neotropical plant groups used the American cordillera as a corridor into SA (Zemlak et al., 2008; Xu et al., 2009; He and Sun, 2017). It would not be until the latest stages of the orogeny that the Andes served as a natural passageway for multiple colonizations in sect. Junciformes, including northward migrations from Patagonia (C. camptoglochin, C. setifolia, C. vallis-pulchrae, and C. via-incaica). This could have been favored by the concurrent, Pliocene cooling of the low-latitude Andes (Roberts et al., 2017). Both, the early permanence of sect. Junciformes in N Patagonia and the posterior colonizations within SA entailed no strong ecological innovations but a relative niche conservatism (Figures 2, 3C). Our bioclimatic reconstructions suggest that such ecological conservation is favored by selection (Figure 2A, OU model; Blomberg et al., 2020). Accordingly, the changing environment in SA (Rabassa, 2008) instead of inducing niche shifts in sect. Junciformes entailed the range expansion as similar suitable niches became available. By contrast the entrance of C. phalaroides in multiple areas out of Patagonia implied striking changes in bioclimatic preferences, especially regarding AMT (Figure 2A). This is expectable since this species inhabits radically different, much more temperate habitats (Atlantic Forest, Pampas, montane tropical forest) in comparison to the other species belonging to sect. Junciformes.
In the late Pliocene and during the Pleistocene, several groups (Junciformes-clade Lineage B and Aciculares-clade SA-clade) underwent in situ diversification either in N or S Patagonia. In addition, these clades present changes in bioclimatic preferences at very shallow levels, especially in the Junciformes-clade Lineage B (Figure 2). These could be interpreted as a result of local adaptation in glacial refugia, as reported in other plant genera in different regions around the world (Tremetsberger et al., 2009; Premoli et al., 2010; Kremer, 2016), as well as in other Carex groups (Benítez-Benítez et al., 2018). Many plant groups were able to survive Patagonian glaciations in high latitude refugia (see references in Table 1) since ice sheets were generally not extensive after the Greatest Patagonian Glaciation (1–1.2 mya; Rabassa, 2008). During the Pleistocene, major Patagonian rivers have been invoked as barriers for allopatric speciation in plants (Jakob et al., 2009; Sede et al., 2012; Cosacov et al., 2013), but these do not seem to have acted as barriers in Carex given its striking capacity for LDD (Villaverde et al., 2017a). In other plant groups, glacial survival in Patagonia seems to be conditioned by the aridification and establishment of the steppe (Jakob et al., 2009; Cosacov et al., 2010; Sede et al., 2012). In sect. Junciformes, the steppe may have played a certain role as a barrier since these species hardly penetrate this biome (Supplementary Figure 3).
Section Junciformes colonized SW Pacific (NZ and probably also SE Australia and Tasmania) from SA (Aciculares-NZ clade; Figure 3A), a biogeographic pattern also reported in other families of plants (Von Hagen and Kadereit, 2001; Meudt and Simpson, 2006; Otero et al., 2019). Whether the colonization took place by direct LDD or stepping stone is unclear, despite the absence of sect. Junciformes in circum-Antarctic archipelagos seem to point to a direct LDD as the most plausible explanation. In any case, inferred divergence ages (late Miocene-Pliocene; Supplementary Figure 6) also rule out the role of Antarctica as the last tundra remnants of this region are reported to disappear around the Middle Miocene (Lewis et al., 2008; but see Barrett, 2013). New Zealand species inhabit alpine habitats which became available with the orogeny of the Southern Alps starting in the Pliocene (Heenan and McGlone, 2013), which mostly matches the crown node age inferred for this group (Figure 3C and Supplementary Figure 6). Interestingly, the colonization of NZ entailed a remarkable long-term niche conservatism within Aciculares-clade for different variables (AMT, TAR, MTDQ; Figure 2), which indicates that the lineage was somehow preadapted to the newly colonized habitats. Nonetheless, a certain degree of local adaptation is revealed by the somewhat different values for AP and TRI (Figure 2).
Diversification Patterns in Subg. Psyllophorae and Their Relation to Niche Evolution
The phylogenetic reconstruction of subg. Psyllophorae shows relatively synchronous cladogenesis through time and an unbalanced topology, with a relatively poor lineage (sect. Psyllophorae) sister to a much more diversified group (sects. Schoenoxiphium-Junciformes; Figure 3B). This has sometimes resulted in a ladderized rather than bifurcating topology (Crisp and Cook, 2005; Vargas and Zardoya, 2014), with frequently long branches and deep nodes originating old monotypic and/or species-poor lineages. Their stem nodes date as old as the early-middle Miocene. Interestingly, this pattern has been found in the three sections: Psyllophorae (C. distachya Desf., c. 18 mya; C. illegitima Ces., 12 mya); Schoenoxiphium (C. multispiculata Luceño and Martín-Bravo - C. lancea (Thunb.) Baill., c. 11 mya), and Junciformes (C. camptoglochin, c. 15 mya; C. vallis-pulchrae, c. 14 mya; C. phalaroides, c. 14 mya).
Diversification rates seem to progressively decrease in subg. Psyllophorae (Figure 3B), which agrees with the previous findings in Martín-Bravo et al. (2019) using a larger sampling of Carex species but a smaller set of sect. Junciformes taxa. As stated before, sect. Junciformes is one of the only three Neotropical Carex groups with more than 15 species (see Introduction). This lack of significant increase in diversification rates points to a process of disparification rather than true evolutionary radiation (Simões et al., 2016). Thus, its current relatively high diversity would be simply the result of the progressive accumulation of changes during its long evolutionary history.
The allopatric distribution of the three sections in different landmasses (Figure 1) could have imposed differential intrinsic/extrinsic factors influencing their diversification. The limited ecological diversification revealed by our bioclimatic niche analysis in sect. Junciformes (Figure 2B) provides insights into the intrinsic conditionants of its evolution. A relatively conserved niche in this lineage, perhaps constrained by its own ecological limits, may have prevented a significant increase in diversification rates. It means that the ecologically suitable space could have been early filled. In particular, the case of the NZ-clade is remarkable, since the group hardly diversified after colonizing NZ, also involving limited ecological (Figure 2) and morphological change (Hamlin, 1962; Edgar, 1970), which strongly contrasts with other spectacular cases of morphological/ecological adaptive radiations within NZ (Wagstaff et al., 2002; Glenny, 2004; Meudt and Simpson, 2006). Furthermore, other Carex groups (sects. Spirostachyae and Uncinia; Roalson et al., 2021) underwent remarkable radiations in NZ, but they could have arrived earlier to the archipelago than NZ-clade of sect. Junciformes (Martín-Bravo et al., 2019), perhaps preventing the establishment of its species through high density blocking (Slingsby and Verboom, 2006; Pender et al., 2021).
Further studies are needed to characterize in detail the niche of the other sections of subg. Psyllophorae. On the one hand, sect. Schoenoxiphium was inferred to have originated in the Drakensberg range in South Africa, where its current center of diversity is still located (Márquez-Corro et al., 2020). In this area, species distributions are often overlapping, but with frequent turnover along various ecological gradients (elevation, wetness, forest to grassland; Luceño et al., 2021). Thus, speciation in this lineage appears to have been sympatric and driven by ecological factors. On the other hand, sect. Psyllophorae combines widely distributed species (C. distachya and C. pulicaris) which are partly sympatric with several allopatric narrowly distributed species (Jiménez-Mejías and Luceño, 2011). Interestingly, while it is the poorest diversified section within subg. Psyllophorae, its species display remarkably different ecological preferences, ranging from Mediterranean shrublands to mountain bogs (Borges et al., 2008; Luceño, 2008; Silva et al., 2010; Verdcourt, 2010; Gehrke, 2011).
Conclusion
The present study provides new insights into the biogeographic and diversification patterns of the Southern Hemisphere and in particular Patagonia, one of the least studied areas of SA. It also sheds light on the phylogenetic structure of one of the oldest lineages of Carex (subg. Psyllophorae, dated to the early Miocene), which early diversified allopatrically in three different continents: sect. Psyllophorae in Western Palearctic, sect. Schoenoxiphium in Afrotropical region, and sect. Junciformes in SA. In particular, the early diversification of the latter section involved the differentiation of a group of species mostly restricted to a single landmass (SA), with its diversity centre in N Patagonia and a single direct LDD to SW Pacific. This trans-Pacific disjunction entails a striking niche conservatism, which implies that these species seem to have been preadapted to ecological requirements made available around the time of arrival, but probably limited subsequent in situ diversification. Later diversification in Patagonia pointed to an important role of Plio-Pleistocene glaciations and the triggering of multiple colonizations toward other SA regions. Furthermore, the Andes acted as a corridor toward the north, an inverse pattern to that reported for the colonization of most Northern Hemisphere cold-adapted plants into SA. The section as a whole seems to have developed geographic speciation with slight ecological differentiation as bioclimatic variables show relatively homogeneous values among species of the same lineage/clade. However, we cannot rule out certain local adaptations for the group at the microevolutionary level.
Data Availability Statement
The original contributions presented in the study are included in the article/Supplementary Materials, further inquiries can be directed to the corresponding author/s.
Author Contributions
CB-B carried out the laboratory work, performed the analyses, and drafted the manuscript. PJ-M and SM-B conceived the idea, collected plant material, and drafted the manuscript. AO carried out biogeographic analyses. PG-M collected occurrences data for bioclimatic niche evolution analyses. ML, KF, and SD collected plant material. All authors contributed to the writing of the final version.
Funding
This support was carried out with financial support by the Spanish Ministry of Science and Innovation (project PID2020-113897GB-I00) and the Regional Government of Madrid, Spain (Macondo SI1/PIJ/2019-00333). CB-B was supported by a Predoctoral Fellowship Program grant (FPU16/01257) from the Spanish Ministry of Universities, and SM-B by a José Castillejo grant (CAS19/00253), from the Spanish Ministry of Science and Innovation.
Conflict of Interest
The authors declare that the research was conducted in the absence of any commercial or financial relationships that could be construed as a potential conflict of interest.
Publisher's Note
All claims expressed in this article are solely those of the authors and do not necessarily represent those of their affiliated organizations, or those of the publisher, the editors and the reviewers. Any product that may be evaluated in this article, or claim that may be made by its manufacturer, is not guaranteed or endorsed by the publisher.
Acknowledgments
The authors thank all the staff from herbaria A, BR, CHR, CONC, GOET, E, M, MA, MO, MSB, NY, SI, UPOS, and WS for granting them access to their collections and for providing them plant material. They also thank D. Penneckamp for the Carex trichodes sample. The authors thank the staff of UPOS and the botanical laboratory for technical support, especially M. Míguez, C. Barciela, E. Ritoré, as well as the preliminary work performed by C. De La Cuadra.
Supplementary Material
The Supplementary Material for this article can be found online at: https://www.frontiersin.org/articles/10.3389/fpls.2021.735302/full#supplementary-material
References
Acosta, M. C., and Premoli, A. C. (2010). Evidence of chloroplast capture in South American Nothofagus (subgenus Nothofagus, Nothofagaceae). Mol. Phylogenet. Evol. 54, 235–242. doi: 10.1016/j.ympev.2009.08.008
Aguilar, D. L., Acosta, M. C., Baranzelli, M. C., Sérsic, A. N., Delatorre-Herrera, J., Verga, A., et al. (2020). Ecophylogeography of the disjunct South American xerophytic tree species Prosopis chilensis (Fabaceae). Biol. J. Linnean Soc. 129, 793–809. doi: 10.1093/biolinnean/blaa006
Antonelli, A., Nylander, J. A. A., Persson, C., and Sanmartin, I. (2009). Tracing the impact of the Andean uplift on Neotropical plant evolution. Proc. Nat. Acad. Sci. U.S.A. 106, 9749–9754. doi: 10.1073/pnas.0811421106
Azpilicueta, M. M., Marchelli, P., and Gallo, L. A. (2009). The effects of Quaternary glaciations in Patagonia as evidenced by chloroplast DNA phylogeography of Southern beech Nothofagus obliqua. Tree Genet. Genomes 5, 561–571. doi: 10.1007/s11295-009-0209-x
Ball, P. W., and Reznicek, A. A. (2002). “Carex” in Flora of North America 23. eds. Editorial Committee. New York, NY: Oxford University Press.
Barreda, V., and Palazzesi, L. (2007). Patagonian vegetation turnovers during the paleogene-early neogene: origin of arid-adapted floras. Botanical Rev. 73, 31–50. doi: 10.1663/0006-8101(2007)73<31:PVTDTP>2.0.CO;2
Barrett, P. J. (2013). Resolving views on Antarctic Neogene glacial history - the Sirius debate. Earth Environ. Sci. Trans. R. Soc. Edinb. 104, 31–53. doi: 10.1017/S175569101300008X
Barros, M. (1935). “Ciperáceas argentinas II, géneros Kyllingia, Scirpus, Carex” Anales del Museo Argentino de Ciencias Naturales. Buenos Aires.
Barros, M. (1969). “Cyperaceae,” in Flora Patagónica, Parte II, Typhaceae a Orchidaceae (exceptio Gramineae), ed M. N. Correa (Buenos Aires: Colección Científica del Instituto Nacional de Tecnología Agropecuaria).
Bell, C., and Donoghue, M. J. (2005). Phylogeny and biogeography of Valerianaceae (Dipsacales) with special reference to the South American valerians. Organ. Diversity Evolut. 5, 147–159. doi: 10.1016/j.ode.2004.10.014
Bell, C. D., Kutschker, A., and Arroyo, M. T. K. (2012). Phylogeny and diversification of Valerianaceae (Dipsacales) in the southern Andes. Mol. Phylogenet. Evol. 63, 724–737. doi: 10.1016/j.ympev.2012.02.015
Benítez-Benítez, C., Escudero, M., Rodríguez-Sánchez, F., Martín-Bravo, S., and Jiménez-Mejías, P. (2018). Pliocene-Pleistocene ecological niche evolution shapes the phylogeography of a Mediterranean plant group. Mol. Ecol. 27, 1696–1713. doi: 10.1111/mec.14567
Benítez-Benítez, C., Martín-Bravo, S., Bjorå, C. S., Gebauer, S., Hipp, A. L., Hoffmann, M. H., et al. (2021). Geographical vs. ecological diversification in Carex section Phacocystis (Cyperaceae): patterns hidden behind a twisted taxonomy. J. Syste. Evolut. 59, 642–667. doi: 10.1111/jse.12731
Biffin, E., Hill, R. S., and Lowe, A. J. (2010). Did Kauri (Agathis: Araucariaceae) Really survive the Oligocene drowning of New Zealand? Syst. Biol. 59, 594–602. doi: 10.1093/sysbio/syq030
Blomberg, S. P., Rathnayake, S. I., and Moreau, C. M. (2020). Beyond Brownian Motion and the Ornstein-Uhlenbeck process: Stochastic diffusion models for the evolution of quantitative characters. Am. Nat. 195, 145–165. doi: 10.1086/706339
Borges, P. A. V., Abreu, C., Aguiar, A. M. F., Carvalho, P., Jardim, R., Melo, I., et al. (2008). A list of the terrestrial Fungi, Flora and Fauna of Madeira and selvagens archipelagos. Portugal: Direccão Regional do Ambiente da Madeira and Universidade dos Açores.
Breitman, M. F., Avila, L. J., Sites, J. W., and Morando, M. (2012). How lizards survived blizzards: Phylogeography of the Liolaemus lineomaculatus group (Liolaemidae) reveals multiple breaks and refugia in southern Patagonia and their concordance with other codistributed taxa. Mol. Ecol. 21, 6068–6085. doi: 10.1111/mec.12075
Broennimann, O., Fitzpatrick, M. C., Pearman, P. B., Petitpierre, B., Pellissier, L., Yoccoz, N. G., et al. (2012). Measuring ecological niche overlap from occurrence and spatial environmental data: Measuring niche overlap. Global Ecol. Biogeograp. 21, 481–497. doi: 10.1111/j.1466-8238.2011.00698.x
Burnham, K. P., and Anderson, D. R. (2002). Model Selection and Multimodel Inference: a Practical Information-Theoretical Approach. New York, NY: Springer.
Cadena, C. D., Cuervo, A. M., Céspedes, L. N., Bravo, G. A., Krabbe, N., Schulenberg, T. S., et al. (2020). Systematics, biogeography, and diversification of Scytalopus tapaculos (Rhinocryptidae), an enigmatic radiation of Neotropical montane birds. The Auk. 137:ukz077. doi: 10.1093/auk/ukz077
Calderón, L., Quintana, F., Cabanne, G. S., Lougheed, S. C., and Tubaro, P. L. (2014). Phylogeography and genetic structure of two Patagonian shag species (Aves: Phalacrocoracidae). Mol. Phylogenet. Evol. 72, 42–53. doi: 10.1016/j.ympev.2013.12.011
Cantrill, D. J., and Poole, I. (2012). The Vegetation of antarctica Through Geological Time. Cambridge: Cambridge University Press.
Cosacov, A., Johnson, L. A., Paiaro, V., Cocucci, A. A., Córdoba, F. E., and Sérsic, A. N. (2013). Precipitation rather than temperature influenced the phylogeography of the endemic shrub Anarthrophyllum desideratum in the Patagonian steppe. J. Biogeogr. 40, 168–182. doi: 10.1111/j.1365-2699.2012.02776.x
Cosacov, A., Sérsic, A. N., Sosa, V., Johnson, L. A., and Cocucci, A. A. (2010). Multiple periglacial refugia in the Patagonian steppe and post-glacial colonization of the Andes: The phylogeography of Calceolaria polyrhiza: Phylogeography of Calceolaria polyrhiza. J. Biogeogr. 37, 1463–1477. doi: 10.1111/j.1365-2699.2010.02307.x
Crisp, M. D., and Cook, L. G. (2005). Do early branching lineages signify ancestral traits? Trends Ecol. Evol. 20, 122–128. doi: 10.1016/j.tree.2004.11.010
Curtis, W. M., and Morris, D. I. (1994). The Student's Flora of Tasmania, Part 4B. Hobart: Government Printer.
Cussac, V., Ortubay, S., Iglesias, G., Milano, D., Lattuca, M. E., Barriga, J. P., et al. (2004). The distribution of South American galaxiid fishes: The role of biological traits and post-glacial history: The distribution of South American galaxiid fishes. J. Biogeogr. 31, 103–121. doi: 10.1046/j.0305-0270.2003.01000.x
de la Estrella, M., Buerki, S., Vasconcelos, T., Lucas, E. J., and Forest, F. (2019). The role of antarctica in biogeographical reconstruction: a point of view. Int. J. Plant Sci. 180, 63–71. doi: 10.1086/700581
Di Cola, V., Broennimann, O., Petitpierre, B., Breiner, F. T., D'Amen, M., Randin, C., et al. (2017). Ecospat: An R package to support spatial analyses and modeling of species niches and distributions. Ecography 40, 774–787. doi: 10.1111/ecog.02671
Edgar, E. (1970). “Cyperaceae,” in Flora of New Zealand. Vol. II. Indigenous Tracheophyta: Monocotyledones except Gramineae, eds L. B. Moore and E. Edgar (Wellington: Botany Division DSIR).
Edgar, R. C. (2004). MUSCLE: Multiple sequence alignment with high accuracy and high throughput. Nucleic Acids Res. 32, 1792–1797. doi: 10.1093/nar/gkh340
Egorova, T. V. (1999). The sedges (Carex L.) of Russia and adjacent states (within the limits of the former USSR). St. Louis: St. Petersburg State Chemical-Pharmaceutical Academy and Missouri Botanical Garden.
Encinas, A., Folguera, A., Bechis, F., Finger, K. L., Zambrano, P., Pérez, F., et al. (2018). “The Late Oligocene-Early Miocene marine transgression of Patagonia,” in The Evolution of the Chilean-Argentinean Andes, ed. A. Folguera, E. Contreras Reyes, N. Heredia, A. Encinas, S. Iannelli, V. Oliveros et al. (Cham: Springer).
Ezcurra, C. (2002). Phylogeny, morphology, and biogeography of chuquiraga, an andean-patagonian genus of asteraceae-barnadesioideae. Botanical Rev. 68, 153–170. doi: 10.1663/0006-8101(2002)068<0153:PMABOC>2.0.CO;2
Fick, S. E., and Hijmans, R. J. (2017). WorldClim 2: New 1-km spatial resolution climate surfaces for global land areas. Int. J. Climatol. 37, 4302–4315. doi: 10.1002/joc.5086
Frajman, B., Schönswetter, P., Weiss-Schneeweiss, H., and Oxelman, B. (2018). Origin and Diversification of South American Polyploid Silene Sect. Physolychnis (Caryophyllaceae) in the Andes and Patagonia. Front. Genet. 9:639. doi: 10.3389/fgene.2018.00639
Gehrke, B. (2011). Synopsis of Carex (Cyperaceae) from sub-Saharan Africa and Madagascar. Botan. J. Linnean Soc. 166, 51–99. doi: 10.1111/j.1095-8339.2011.01116.x
Gehrke, B., Martín-Bravo, S., Muasya, M., and Luceño, M. (2010). Monophyly, phylogenetic position and the role of hybridization in Schoenoxiphium Nees (Cariceae, Cyperaceae). Mol. Phylogenet. Evol. 56, 380–392. doi: 10.1016/j.ympev.2010.03.036
Givnish, T. J., Millam, K. C., Evans, T. M., Hall, J. C., Chris Pires, J., Berry, P. E., et al. (2004). Ancient vicariance or Recent Long-Distance Dispersal? Inferences about phylogeny and South American–African disjunctions in Rapateaceae and Bromeliaceae based on ndh F sequence data. Int. J. Plant Sci. 165, S35–S54. doi: 10.1086/421067
Glenny, D. (2004). A revision of the genus Gentianella in New Zealand. NZ. J. Bot. 42, 361–530. doi: 10.1080/0028825X.2004.9512910
Govaerts, R., Jiménez-Mejías, P., Koopman, J., Simpson, D., Goetghebeur, P., Wilson, K., et al. (2020). World Checklist of Cyperaceae. Available online at: http://wcsp.science.kew.org/ (accessed July 16, 2020).
Hamlin, B. G. (1962). Studies in New Zealand Carices - VI. Subgenus Primocarex Kükenthal. Transact. R. Soc. New Zeal. Botany 1, 269–277.
Harmon, L. J., Weir, J. T., Brock, C. D., Glor, R. E., and Challenger, W. (2008). GEIGER: Investigating evolutionary radiations. Bioinformatics 24, 129–131. doi: 10.1093/bioinformatics/btm538
He, X., and Sun, Y. (2017). Contrasting patterns of postglacial range shifts between the northern and southern hemisphere in Herbertus (Herbertaceae, Marchantiophyta). System. Biodivers. 15, 541–551. doi: 10.1080/14772000.2017.1291542
Heenan, P. B., and McGlone, M. S. (2013). Evolution of New Zealand alpine and open-habitat plant species during the late Cenozoic. New Zeal. J. Ecol. 37, 105–113. Available online at: https://newzealandecology.org/nzje/
Heibl, C., and Renner, S. S. (2012). Distribution models and a dated phylogeny for Chilean Oxalis species reveal occupation of new habitats by different lineages, not rapid adaptive radiation. Syst. Biol. 61, 823–834. doi: 10.1093/sysbio/sys034
Hewitt, G. M. (2000). The genetic legacy of the Quaternary ice ages. Nature 405, 907–913. doi: 10.1038/35016000
Hewitt, G. M. (2004). Genetic consequences of climatic oscillations in the Quaternary. Philosoph. Transact. R. Soc. Lond. Series B Biol. Sci. 359, 183–195. doi: 10.1098/rstb.2003.1388
Holderegger, R., and Thiel-Egenter, C. (2009). A discussion of different types of glacial refugia used in mountain biogeography and phylogeography. J. Biogeogr. 36, 476–480. doi: 10.1111/j.1365-2699.2008.02027.x
Hughes, C., and Eastwood, R. (2006). Island radiation on a continental scale: Exceptional rates of plant diversification after uplift of the Andes. Proc. Nat. Acad. Sci. U.S.A. 103, 10334–10339. doi: 10.1073/pnas.0601928103
Jakob, S. S., Martinez-Meyer, E., and Blattner, F. R. (2009). Phylogeographic analyses and paleodistribution modeling indicate Pleistocene in situ survival of Hordeum species (Poaceae) in southern Patagonia without genetic or spatial restriction. Mol. Biol. Evol. 26, 907–923. doi: 10.1093/molbev/msp012
Jiménez-Mejías, P., Alegría-Olivera, J. J., Beltrán, H. W., Cano, A., Granda-Paucar, A., Maldonado Fonkén, M. S., et al. (2020). Chorological and nomenclatural notes on Peruvian Carex (Cyperaceae). Caldasia 42, 63–69. doi: 10.15446/caldasia.v42n1.76771
Jiménez-Mejías, P., Benítez-Benítez, C., Beltrán, H., Cano Echevarría, A., Donadío, S., Escudero, M., et al. (2018). Carex (Cyperaceae) in South America: Diversity, Phylogenetics and Biogeography of a Boreotemperate Element in the Neotropics. [Poster presented at the 6th International Conference on Comparative Biology of Monocotyledons]. [Natal, Rio Grande do Norte, Brazil].
Jiménez-Mejías, P., Hahn, M., Lueders, K., Starr, J. R., Brown, B. H., Chouinard, B. N., et al. (2016a). Megaphylogenetic specimen-level approaches to the Carex (Cyperaceae) phylogeny using ITS, ETS, and matK: Implications for classification. Syst. Bot. 41, 500–518. doi: 10.1600/036364416X692497
Jiménez-Mejías, P., and Luceño, M. (2011). “Cyperaceae,” in Euro+Med Plantbase - The Information Resource for Euro-Mediterranean Plant Diversity. Available online at: http://ww2.bgbm.org/EuroPlusMed/query.asp
Jiménez-Mejías, P., Martinetto, E., Momohara, A., Popova, S., Smith, S. Y., and Roalson, E. H. (2016b). A commented synopsis of the Pre-Pleistocene fossil record of Carex (Cyperaceae). Botanical Rev. 82, 258–345. doi: 10.1007/s12229-016-9169-7
Jiménez-Mejías, P., and Roalson, E. H. (2016). Two new species of Carex (Cyperaceae) from northern South America. Phytotaxa 266:21. doi: 10.11646/phytotaxa.266.1.3
Kim, I., Phillips, C. J., Monjeau, J. A., Birney, E. C., Noack, K., Pumo, D. E., et al. (1998). Habitat islands, genetic diversity, and gene flow in a Patagonian rodent. Mol. Ecol. 7, 667–678. doi: 10.1046/j.1365-294x.1998.00369.x
Knapp, M., Stöckler, K., Havell, D., Delsuc, F., Sebastiani, F., and Lockhart, P. J. (2005). Relaxed molecular clock provides evidence for long-distance dispersal of nothofagus (Southern Beech). PLoS Biol. 3:e14. doi: 10.1371/journal.pbio.0030014
Kozak, K. H., and Wiens, J. J. (2006). Does niche conservatism promote speciation? A case study in North American salamanders. Evolution 60, 2604–2621. doi: 10.1111/j.0014-3820.2006.tb01893.x
Kranitz, M. L., Biffin, E., Clark, A., Hollingsworth, M. L., Ruhsam, M., Gardner, M. F., et al. (2014). Evolutionary diversification of new caledonian araucaria. PLoS ONE 9:e110308. doi: 10.1371/journal.pone.0110308
Kremer, A. (2016). Microevolution of European temperate oaks in response to environmental changes. C. R. Biol. 339, 263–267. doi: 10.1016/j.crvi.2016.04.014
Landis, M. J., Matzke, N. J., Moore, B. R., and Huelsenbeck, J. P. (2013). Bayesian analysis of biogeography when the number of areas is large. Syst. Biol. 62, 789–804. doi: 10.1093/sysbio/syt040
Lewis, A. R., Marchant, D. R., Ashworth, A. C., Hedenas, L., Hemming, S. R., Johnson, J. V., et al. (2008). Mid-Miocene cooling and the extinction of tundra in continental Antarctica. Proc. Nat. Acad. Sci. U.S.A. 105, 10676–10680. doi: 10.1073/pnas.0802501105
López, A., and Bonasora, M. G. (2017). Phylogeography, genetic diversity and population structure in a Patagonian endemic plant. AoB PLANTS 9:plx017. doi: 10.1093/aobpla/plx017
Losos, J. B. (2008). Phylogenetic niche conservatism, phylogenetic signal and the relationship between phylogenetic relatedness and ecological similarity among species. Ecol. Lett. 11, 995–1003. doi: 10.1111/j.1461-0248.2008.01229.x
Luceño, M. (2008). “Carex,” in Flora Iberica, eds S. Castroviejo, M. Luceño, A. Galán de Mera, P. Jiménez-Mejías, F. J. Cabezas, and L. Medina (Madrid: Real Jardín Botánico).
Luceño, M., Villaverde, T., Márquez-Corro, J. I., Sánchez-Villegas, R., Maguilla, E., Escudero, M., et al. (2021). An integrative monograph of Carex section Schoenoxiphium (Cyperaceae). PeerJ 9:e11336. doi: 10.7717/peerj.11336
Luebert, F., Hilger, H. H., and Weigend, M. (2011). Diversification in the andes: age and origins of South American heliotropium lineages (Heliotropiaceae, Boraginales). Mol. Phylogenet. Evol. 61, 90–102. doi: 10.1016/j.ympev.2011.06.001
Luebert, F., Lörch, M., Acuña, R., Mello-Silva, R., Weigend, M., and Mutke, J. (2020). “Clade-specific biogeographic history and climatic niche shifts of the southern Andean-southern Brazilian disjunction in plants,” in Neotropical Diversification: Patterns and Processes, eds V. Rull and A. C. Carnaval (Cham: Springer International Publishing). doi: 10.1007/978-3-030-31167-4_24
Mairal, M., Sanmartín, I., and Pellissier, L. (2017). Lineage-specific climatic niche drives the tempo of vicariance in the Rand Flora. J. Biogeogr. 44, 911–923. doi: 10.1111/jbi.12930
Marcheli, P., and Gallo, L. (2006). Multiple ice-age refugia in a southern beech of South America as evidenced by chloroplast DNA markers. Conserv. Genet. 7, 591–603. doi: 10.1007/s10592-005-9069-6
Markgraf, V. (1983). Late and postglacial vegetational and paleoclimatic changes in subantarctic, temperate, and arid environments in Argentina. Palynology 7, 43–70. doi: 10.1080/01916122.1983.9989252
Markgraf, V., McGlone, M., and Hope, G. (1995). Neogene paleoenvironmental and paleoclimatic change in southern temperate ecosystems—A southern perspective. Trends Ecol. Evol. 10, 143–147. doi: 10.1016/S0169-5347(00)89023-0
Márquez-Corro, J. I., Jiménez-Mejías, P., Helme, N. A., Luceño, M., and Martín-Bravo, S. (2020). The systematic position of the enigmatic rare South African endemic Carex acocksii: Its relevance on the biogeography and evolution of Carex sect. Schoenoxiphium (Cyperaceae). South Afr. J. Botany 131, 475–483. doi: 10.1016/j.sajb.2020.03.027
Martín-Bravo, S., Jiménez-Mejías, P., Villaverde, T., Escudero, M., Hahn, M., Spalink, D., et al. (2019). A tale of worldwide success: Behind the scenes of Carex (Cyperaceae) biogeography and diversification. J. Syst. Evol. 57, 695–718. doi: 10.1111/jse.12549
Martínez, O. A., and Kutschker, A. (2011). The ‘Rodados Patagónicos’ (Patagonian shingle formation) of eastern Patagonia: Environmental conditions of gravel sedimentation: Patagonian gravels in eastern Patagonia. Biol. J. Linnean Soc. 103, 336–345. doi: 10.1111/j.1095-8312.2011.01651.x
Mathiasen, P., and Premoli, A. C. (2010). Out in the cold: Genetic variation of Nothofagus pumilio (Nothofagaceae) provides evidence for latitudinally distinct evolutionary histories in austral South America: Genetic structure of N. pumilio. Mol. Ecol. 19, 371–385. doi: 10.1111/j.1365-294X.2009.04456.x
Matzke, N. J. (2014a). BioGeoBEARS: BioGeography With Bayesian (and Likelihood) Evolutionary Analysis with R Scripts, v. 1.1.1. Available online at: https://rdrr.io/cran/BioGeoBEARS (accessed November 15, 2020).
Matzke, N. J. (2014b). Model selection in historical biogeography reveals that founder-event speciation is a crucial process in Island clades. Syst. Biol. 63, 951–970. doi: 10.1093/sysbio/syu056
McGlone, M. S., Duncan, R. P., and Heenan, P. B. (2001). Endemism, species selection and the origin and distribution of the vascular plant flora of New Zealand: Origin and distribution of the New Zealand flora. J. Biogeograp. 28, 199–216. doi: 10.1046/j.1365-2699.2001.00525.x
McLoughlin, S. (2001). The breakup history of Gondwana and its impact on pre-Cenozoic floristic provincialism. Aust. J. Bot. 49:271. doi: 10.1071/BT00023
Meudt, H. M., and Simpson, B. B. (2006). The biogeography of the austral, subalpine genus Ourisia (Plantaginaceae) based on molecular phylogenetic evidence: South American origin and dispersal to New Zealand and Tasmania. Biol. J. Linnean Soc. 87, 479–513. doi: 10.1111/j.1095-8312.2006.00584.x
Míguez, M., Gehrke, B., Maguilla, E., Jiménez-Mejías, P., and Martín-Bravo, S. (2017). Carex sect. Rhynchocystis (Cyperaceae): A miocene subtropical relict in the Western Palaearctic showing a dispersal-derived Rand Flora pattern. J. Biogeogr. 44, 2211–2224. doi: 10.1111/jbi.13027
Miller, M. A., Pfeiffer, W., and Schwartz, T. (2010). “Creating the CIPRES science gateway for inference of large phylogenetic trees,” in Proceedings of the Gateway Computing Environments Workshop, 14, 1–8. doi: 10.1109/GCE.2010.5676129
Moore, D. M. (1968). The vascular flora of the Falkland Islands. Br. Antarct. Sur. Sci. Rep. 60, 1–202.
Moore, D. M. (1983). Flora of Tierra del Fuego. Oswestry; St. Louis, MO: Anthony Nelson Ltd. and Missouri Botanical Garden.
Moore, L. B., and Edgar, E. (1970). Flora of New Zealand Volume II. Wellington: Indigenous Tracheophyta, Monocotyledons except Gramineae. Government Printer.
Mora, A., Villagómez, D., Parra, M., Caballero, V. M., Spikings, R., Horton, B. K., et al. (2020). “Late Cretaceous to Cenozoic uplifts of the Northern Andes: Paleogeographic implications,” in The Geology of Colombia, eds J. Gómez and D. Mateus-Zabala (Bogotá: Servicio Geológico Colombiano, Publicaciones Geológicas Especiales). doi: 10.32685/pub.esp.37.2019.04
Morando, M., Avila, L. J., Baker, J., and Sites, J. W. (2004). Phylogeny and phylogeography of the Liolaemus darwinii complex (Squamata: Liolaemidae): evidence for introgression and incomplete lineage sorting. Evolution 58, 842–859. doi: 10.1111/j.0014-3820.2004.tb00416.x
Morando, M., Avila, L. J., Turner, C. R., and Sites, J. W. (2007). Molecular evidence for a species complex in the patagonian lizard Liolaemus bibronii and phylogeography of the closely related Liolaemus gracilis (Squamata: Liolaemini). Mol. Phylogenet. Evol. 43, 952–973. doi: 10.1016/j.ympev.2006.09.012
Morello, S., and Sede, S. M. (2016). Genetic admixture and lineage separation in a southern Andean plant. AoB Plants 8:plw034. doi: 10.1093/aobpla/plw034
Muellner, A. N., Tremetsberger, K., Stuessy, T., and Baeza, C. M. (2005). Pleistocene refugia and recolonization routes in the southern Andes: Insights from Hypochaeris palustris (Asteraceae, Lactuceae): Hypochaeris palustris in the Southern Andes. Mol. Ecol. 14, 203–212. doi: 10.1111/j.1365-294X.2004.02386.x
Nicola, M. V., Johnson, L. A., and Pozner, R. (2019). Unraveling patterns and processes of diversification in the South Andean-Patagonian Nassauvia subgenus Strongyloma (Asteraceae, Nassauvieae). Mol. Phylogenet. Evol. 136, 164–182. doi: 10.1016/j.ympev.2019.03.004
Nicola, M. V., Sede, S. M., Pozner, R., and Johnson, L. A. (2014). Phylogeography and palaeodistribution modelling of Nassauvia subgenus Strongyloma (Asteraceae): Exploring phylogeographical scenarios in the Patagonian steppe. Ecol. Evol. 4, 4270–4286. doi: 10.1002/ece3.1268
Noben, S., Kessler, M., Quandt, D., Weigand, A., Wicke, S., Krug, M., et al. (2017). Biogeography of the Gondwanan tree fern family Dicksoniaceae-A tale of vicariance, dispersal and extinction. J. Biogeogr. 44, 2648–2659. doi: 10.1111/jbi.13056
Nürk, N. M., Scheriau, C., and Madriñán, S. (2013). Explosive radiation in high Andean Hypericum-rates of diversification among New World lineages. Front. Genet. 4:175. doi: 10.3389/fgene.2013.00175
Otero, A., Jiménez-Mejías, P., Valcárcel, V., and Vargas, P. (2019). Worldwide long-distance dispersal favored by epizoochorous traits in the biogeographic history of Omphalodeae (Boraginaceae). J. Syst. Evol. 57, 579–593. doi: 10.1111/jse.12504
Pastorino, M. J., Gallo, L. A., and Hattemer, H. H. (2004). Genetic variation in natural populations of Austrocedrus chilensis, a cypress of the Andean-Patagonian Forest. Biochem. Syst. Ecol. 32, 993–1008. doi: 10.1016/j.bse.2004.03.002
Pastorino, M. J., Marchelli, P., Milleron, M., Soliani, C., and Gallo, L. A. (2009). The effect of different glaciation patterns over the current genetic structure of the southern beech Nothofagus antarctica. Genetica 136, 79–88. doi: 10.1007/s10709-008-9314-2
Pearman, P. B., Guisan, A., Broennimann, O., and Randin, C. F. (2008). Niche dynamics in space and time. Trends Ecol. Evol. 23, 149–158. doi: 10.1016/j.tree.2007.11.005
Pender, J. E., Hipp, A. L., Hahn, M., and Starr, J. R. (2021). Trait evolution rates shape continental patterns of species richness in North America's most diverse angiosperm genus (Carex, Cyperaceae). J. Syst. Evol. 59, 763–775. doi: 10.1111/jse.12739
Ponce, J. F., Rabassa, J., Coronato, A., and Borromei, A. M. (2011). Palaeogeographical evolution of the Atlantic coast of Pampa and Patagonia from the last glacial maximum to the Middle Holocene: Palaeogeography of Patagonia since LGM. Biol. J. Linnean Soc. 103, 363–379. doi: 10.1111/j.1095-8312.2011.01653.x
Premoli, A. C., Kitzberger, T., and Veblen, T. T. (2000). Isozyme variation and recent biogeographical history of the long-lived conifer Fitzroya cupressoides. J. Biogeogr. 27, 251–260. doi: 10.1046/j.1365-2699.2000.00402.x
Premoli, A. C., Mathiasen, P., and Kitzberger, T. (2010). Southernmost Nothofagus trees enduring ice ages: Genetic evidence and ecological niche retrodiction reveal high latitude (54°S) glacial refugia. Palaeogeogr. Palaeoclimatol. Palaeoecol. 298, 247–256. doi: 10.1016/j.palaeo.2010.09.030
Quiroga, M. P., and Premoli, A. C. (2010). Genetic structure of Podocarpus nubigena (Podocarpaceae) provides evidence of Quaternary and ancient historical events. Palaeogeogr. Palaeoclimatol. Palaeoecol. 285, 186–193. doi: 10.1016/j.palaeo.2009.11.010
R Development Core Team (2019). R: A LANGUAGE and Environment for Statistical Computing. Austria: R Foundation for Statistical Computing. Available online at: https://www.r-project.org/ (accessed November 20, 2020).
Rabassa, J. (2008). “Late cenozoic glaciations in Patagonia and Tierra del Fuego,” in Developments in Quaternary Sciences, Vol. 11 (Amsterdam: Elsevier). doi: 10.1016/S1571-0866(07)10008-7
Rabassa, J., Coronato, A., and Martínez, O. (2011). Late Cenozoic glaciations in Patagonia and Tierra del Fuego: An updated review: Late Cenozoic Patagonian glaciations. Biol. J. Linnean Soc. 103, 316–335. doi: 10.1111/j.1095-8312.2011.01681.x
Rabassa, J., Coronato, A. M., and Salemme, M. (2005). Chronology of the Late Cenozoic Patagonian glaciations and their correlation with biostratigraphic units of the Pampean region (Argentina). J. South Am. Earth Sci. 20, 81–103. doi: 10.1016/j.jsames.2005.07.004
Rabosky, D. L., Grundler, M., Anderson, C., Title, P., Shi, J. J., Brown, J. W., et al. (2014). BAMMtools: An R package for the analysis of evolutionary dynamics on phylogenetic trees. Methods Ecol. Evolution 5, 701–707. doi: 10.1111/2041-210X.12199
Ramos, V. A., and Ghiglione, M. C. (2008). “Tectonic evolution of the patagonian Andes,” in Developments in Quaternary Sciences, Vol. 11 (Amsterdam: Elsevier). doi: 10.1016/S1571-0866(07)10004-X
Ree, R. H., and Sanmartín, I. (2018). Conceptual and statistical problems with the DEC+J model of founder-event speciation and its comparison with DEC via model selection. J. Biogeograp. 45, 741–749. doi: 10.1111/jbi.13173
Ree, R. H., and Smith, S. A. (2008). Maximum likelihood inference of geographic range evolution by dispersal, local extinction, and cladogenesis. Syst. Biol. 57, 4–14. doi: 10.1080/10635150701883881
Revell, L. J. (2012). Phytools: An R package for phylogenetic comparative biology (and other things): phytools: R package. Methods Ecol. Evolut. 3, 217–223. doi: 10.1111/j.2041-210X.2011.00169.x
Roalson, E. H., Jiménez-Mejías, P., Hipp, A. L., Benítez-Benítez, C., Bruederle, L. P., Chung, K.-S., et al. (2021). A framework infrageneric classification of Carex (Cyperaceae) and its organizing principles. J. Syst. Evol. 59, 726–762. doi: 10.1111/jse.12722
Roberts, N. J., Barendregt, R. W., and Clague, J. J. (2017). Multiple tropical Andean glaciations during a period of late Pliocene warmth. Sci. Rep. 7:41878. doi: 10.1038/srep41878
Ronquist, F. (1997). Dispersal-Vicariance analysis: a new approach to the quantification of historical biogeography. Syst. Biol. 46:9.
Ronquist, F., Teslenko, M., van der Mark, P., Ayres, D. L., Darling, A., Höhna, S., et al. (2012). MrBayes 3.2: efficient bayesian phylogenetic inference and model choice across a large model space. Syst. Biol. 61, 539–542. doi: 10.1093/sysbio/sys029
Rull, V. (2008). Speciation timing and neotropical biodiversity: The Tertiary-Quaternary debate in the light of molecular phylogenetic evidence: Speciation timing and neotropical biodiversity. Mol. Ecol. 17, 2722–2729. doi: 10.1111/j.1365-294X.2008.03789.x
Ruzzante, D. E., Walde, S. J., Gosse, J. C., Cussac, V. E., Habit, E., Zemlak, T. S., et al. (2008). Climate control on ancestral population dynamics: Insight from Patagonian fish phylogeography: Historical population dynamics of Patagonian fish. Mol. Ecol. 17, 2234–2244. doi: 10.1111/j.1365-294X.2008.03738.x
Sanmartín, I., and Ronquist, F. (2004). Southern hemisphere biogeography inferred by event-based models: plant versus animal patterns. Syst. Biol. 53, 216–243. doi: 10.1080/10635150490423430
Schoener, T. W. (1968). The anolis lizards of bimini: resource partitioning in a complex fauna. Ecology 49, 704–726. doi: 10.2307/1935534
Sede, S. M., Nicola, M. V., Pozner, R., and Johnson, L. A. (2012). Phylogeography and palaeodistribution modelling in the Patagonian steppe: The case of Mulinum spinosum (Apiaceae): Phylogeography and palaeodistribution modelling of Mulinum spinosum. J. Biogeogr. 39, 1041–1057. doi: 10.1111/j.1365-2699.2011.02662.x
Segovia, R. A., Pérez, M. F., and Hinojosa, L. F. (2012). Genetic evidence for glacial refugia of the temperate tree Eucryphia cordifolia (Cunoniaceae) in southern South America. Am. J. Bot. 99, 121–129. doi: 10.3732/ajb.1100013
Sérsic, A. N., Cosacov, A., Cocucci, A. A., Johnson, L. A., Pozner, R., Avila, L. J., et al. (2011). Emerging phylogeographical patterns of plants and terrestrial vertebrates from Patagonia: Phylogeographical patterns from Patagonia. Biol. J. Linnean Soc. 103, 475–494. doi: 10.1111/j.1095-8312.2011.01656.x
Shaw, J., Lickey, E. B., Beck, J. T., Farmer, S. B., Liu, W., Miller, J., et al. (2005). The tortoise and the hare II: Relative utility of 21 noncoding chloroplast DNA sequences for phylogenetic analysis. Am. J. Bot. 92, 142–166. doi: 10.3732/ajb.92.1.142
Silva, L., Moura, M., Schaefer, H., Rumsey, F., and Dias, E. F. (2010). “List of vascular plants (Tracheobionta),” in A List of the Terrestrial and Marine Biota From the Azores, eds P. A. V. Borges, A. Costa, R. Cunha, R. Gabriel, V. Gonçalves, A. F. Martins et al. (Cascáis: Principia) 117–146.
Silveira, G. H., and Longui-Wagner, H. M. (2012). O gênero Carex L. (Cyperaceae) no Rio Grande do Sul, Brasil. Brazil. J. Biosci. 10, 373–417.
Simmons, M. P., and Ochoterena, H. (2000). Gaps as characters in sequence-based phylogenetic analyses. Syst. Biol. 49, 369–381. doi: 10.1093/sysbio/49.2.369
Simões, M., Breitkreuz, L., Alvarado, M., Baca, S., Cooper, J. C., Heins, L., et al. (2016). The evolving theory of evolutionary radiations. Trends Ecol. Evol. 31, 27–34. doi: 10.1016/j.tree.2015.10.007
Simpson, B. B., Tate, J. A., and Weeks, A. (2005). The biogeography of Hoffmannseggia (Leguminosae, Caesalpinioideae, Caesalpinieae): A tale of many travels: The biogeography of Hoffmannseggia, Leguminosae. J. Biogeogr. 32, 15–27. doi: 10.1111/j.1365-2699.2004.01161.x
Simpson, M. G., Johnson, L. A., Villaverde, T., and Guilliams, C. M. (2017). American amphitropical disjuncts: Perspectives from vascular plant analyses and prospects for future research. Am. J. Bot. 104, 1600–1650. doi: 10.3732/ajb.1700308
Slingsby, J. A., and Verboom, G. A. (2006). Phylogenetic relatedness limits co-occurrence at fine spatial scales: evidence from the Schoenoid sedges (Cyperaceae: Schoeneae) of the Cape Floristic Region, South Africa. Am. Nat. 168, 14–27. doi: 10.1086/505158
Soliani, C., Tsuda, Y., Bagnoli, F., Gallo, L. A., Vendramin, G. G., and Marcella, P. (2015). Halfway encounters: Meeting points of colonization routes among the southern beeches Nothofagus pumilio and N. antarctica. Mol. Phylogenet. Evolut. 85, 197–207. doi: 10.1016/j.ympev.2015.01.006
Souto, C. P., Kitzberger, T., Arbetman, M. P., and Premoli, A. C. (2015). How do cold-sensitive species endure ice ages? Phylogeographic and paleodistribution models of postglacial range expansion of the mesothermic drought-tolerant conifer Austrocedrus chilensis. New Phytol. 208, 960–972. doi: 10.1111/nph.13508
Souto, C. P., and Premoli, A. C. (2007). Genetic variation in the widespread Embothrium coccineum (Proteaceae) endemic to Patagonia: Effects of phylogeny and historical events. Aust. J. Bot. 55, 809–817. doi: 10.1071/BT06183
Spalik, K., Piwczyński, M., Danderson, C. A., Kurzyna-Młynik, R., Bone, T. S., and Downie, S. R. (2010). Amphitropic amphiantarctic disjunctions in Apiaceae subfamily Apioideae. J. Biogeogr. 37, 1977–1994. doi: 10.1111/j.1365-2699.2010.02334.x
Spalink, D., Drew, B. T., Pace, M. C., Zaborsky, J. G., Starr, J. R., Cameron, K. M., et al. (2016). Biogeography of the cosmopolitan sedges (Cyperaceae) and the area-richness correlation in plants. J. Biogeogr. 43, 1893–1904. doi: 10.1111/jbi.12802
Specht, C. (2006). Gondwanan Vicariance or Dispersal in the Tropics? The biogeographic history of the tropical monocot family costaceae (Zingiberales). Aliso 22, 633–644. doi: 10.5642/aliso.20062201.50
Stamatakis, A. (2014). RAxML v. 8: A tool for phylogenetic analysis and post-analysis of large phylogenies. Bioinformatics 30, 1312–1313. doi: 10.1093/bioinformatics/btu033
Suchard, M. A., Lemey, P., Baele, G., Ayres, D. L., Drummond, A. J., and Rambaut, A. (2018). Bayesian phylogenetic and phylodynamic data integration using BEAST 1.10. Virus Evolut. 4:16. doi: 10.1093/ve/vey016
Title, P. O., and Bemmels, J. B. (2018). ENVIREM: An expanded set of bioclimatic and topographic variables increases flexibility and improves performance of ecological niche modeling. Ecography 41, 291–307. doi: 10.1111/ecog.02880
Tremetsberger, K., Urtubey, E., Terrab, A., Baeza, C. M., Ortiz, M. Á., Talavera, M., et al. (2009). Pleistocene refugia and polytopic replacement of diploids by tetraploids in the Patagonian and Subantarctic plant Hypochaeris incana (Asteraceae, Cichorieae). Mol. Ecol. 18, 3668–3682. doi: 10.1111/j.1365-294X.2009.04298.x
Vargas, P., and Zardoya, R. (2014). The Tree of Life: Evolution and Classification of Living Organisms. Massachussets: Sinauer.
Vera-Escalona, I., D'Elía, G., Gouin, N., Fontanella, F. M., Muñoz-Mendoza, C., Sites, J. W., et al. (2012). Lizards on ice: evidence for multiple refugia in Liolaemus pictus (Liolaemidae) during the last glacial maximum in the southern andean beech forests. PLoS ONE 7:e48358. doi: 10.1371/journal.pone.0048358
Verdcourt, B. (2010). “Carex,” in Flora of Tropical East Africa, eds H. J. Beentje and S. A. Ghazanfar (London: Richmond Kew Publishing).
Vidal-Russell, R., Souto, C. P., and Premoli, A. C. (2011). Multiple Pleistocene refugia in the widespread Patagonian tree Embothrium coccineum (Proteaceae). Aust. J. Bot. 59, 299–314. doi: 10.1071/BT10303
Villaverde, T., Escudero, M., Martín-Bravo, S., Jiménez-Mejías, P., Sanmartín, I., Vargas, P., et al. (2017a). Bipolar distributions in vascular plants: A review. Am. J. Bot. 104, 1680–1694. doi: 10.3732/ajb.1700159
Villaverde, T., Jiménez-Mejías, P., Luceño, M., Waterway, M. J., Kim, S., Lee, B., et al. (2020). A new classification of Carex (Cyperaceae) subgenera supported by a HybSeq backbone phylogenetic tree. Botan. J. Linnean Soc. 194, 141–163. doi: 10.1093/botlinnean/boaa042
Villaverde, T., Maguilla, E., Escudero, M., Márquez-Corro, J. I., Jiménez-Mejías, P., Gehrke, B., et al. (2017b). New insights into the systematics of the Schoenoxiphium Clade (Carex, Cyperaceae). Int. J. Plant Sci. 178, 320–329. doi: 10.1086/691144
Von Hagen, K. B., and Kadereit, J. W. (2001). The phylogeny of Gentianella (Gentianaceae) and its colonization of the southern hemisphere as revealed by nuclear and chloroplast DNA sequence variation. Organ. Diversity Evolut. 1, 61–79. doi: 10.1078/1439-6092-00005
Wagstaff, S. J., Bayly, M., Garnock-Jones, P. J., and Albach, D. (2002). Classification, origin, and diversification of the New Zealand Hebes (Scrophulariaceae). Ann. Missouri Botan. Garden 89, 38–63. doi: 10.2307/3298656
Warren, D. L., Glor, R. E., and Turelli, M. (2008). Environmental niche equivalency versus conservatism: quantitative approaches to niche evolution. Evolution 62, 2868–2883. doi: 10.1111/j.1558-5646.2008.00482.x
Wheeler, G. A. (1988). The distribution of Carex acaulis Urv., C. barrosii Nelmes, and C. macrosolen Steudel (Cyperaceae) in Austral South America. Taxon 37, 127–131. doi: 10.2307/1220939
Wheeler, G. A. (1989). The taxonomy of Carex sect. Aciculares (Cyperaceae) in South America. System. Botany 14, 168–188. doi: 10.2307/2418904
Wheeler, G. A. (1998). Notes on Carex azuayae and C. enneastachya (Cyperaceae) from South America. Rhodora 100, 293–297.
Wheeler, G. A., and Beck, S. G. (2011). A new combination in Carex (Cyperaceae) and the first report of five other Cariceae from Bolivia. Rev. Soc. Bolivi. Botán. 5, 47–52.
Wheeler, G. A., and Guaglianone, E. R. (2003). Notes of South American Carex (Cyperaceae): C. camptoglochin and C. microglochin. Darwiniana 41, 193–206.
Wheeler, G. A., and Muñoz-Schick, M. (1990). Carex andina Philippi (Cyperaceae): its taxonomy, distribution and lectotypification. Gayana Botan. 47, 71–76.
Wickham, H. (2006). ggplot: An Implementation of the Grammar of Graphics in R Package version 2.0.0.
Wiens, J. J., Ackerly, D. D., Allen, A. P., Anacker, B. L., Buckley, L. B., Cornell, H. V., et al. (2010). Niche conservatism as an emerging principle in ecology and conservation biology. Ecol. Lett. 13, 1310–1324. doi: 10.1111/j.1461-0248.2010.01515.x
Wiens, J. J., and Graham, C. H. (2005). Niche conservatism: integrating evolution, ecology, and conservation biology. Annu. Rev. Ecol. Evol. Syst. 36, 519–539. doi: 10.1146/annurev.ecolsys.36.102803.095431
Xu, J., Pérez-Losada, M., Jara, C. G., and Crandall, K. A. (2009). Pleistocene glaciation leaves deep signature on the freshwater crab Aegla alacalufi in Chilean Patagonia. Mol. Ecol. 18, 904–918. doi: 10.1111/j.1365-294X.2008.04070.x
Zemlak, T. S., Habit, E. M., Walde, S. J., Battini, M. A., Adams, E. D. M., and Ruzzante, D. E. (2008). Across the southern Andes on fin: Glacial refugia, drainage reversals and a secondary contact zone revealed by the phylogeographical signal of Galaxias platei in Patagonia. Mol. Ecol. 17, 5049–5061. doi: 10.1111/j.1365-294X.2008.03987.x
Keywords: Andes, biogeography, disjunction, Gondwana, long-distance dispersal, niche conservatism
Citation: Benítez-Benítez C, Otero A, Ford KA, García-Moro P, Donadío S, Luceño M, Martín-Bravo S and Jiménez-Mejías P (2021) An Evolutionary Study of Carex Subg. Psyllophorae (Cyperaceae) Sheds Light on a Strikingly Disjunct Distribution in the Southern Hemisphere, With Emphasis on Its Patagonian Diversification. Front. Plant Sci. 12:735302. doi: 10.3389/fpls.2021.735302
Received: 02 July 2021; Accepted: 28 September 2021;
Published: 08 November 2021.
Edited by:
Lisa Pokorny, National Institute of Agricultural and Food Research and Technology, SpainReviewed by:
Isabel Larridon, Royal Botanic Gardens, Kew, United KingdomDaniel Spalink, Texas A&M University, United States
Copyright © 2021 Benítez-Benítez, Otero, Ford, García-Moro, Donadío, Luceño, Martín-Bravo and Jiménez-Mejías. This is an open-access article distributed under the terms of the Creative Commons Attribution License (CC BY). The use, distribution or reproduction in other forums is permitted, provided the original author(s) and the copyright owner(s) are credited and that the original publication in this journal is cited, in accordance with accepted academic practice. No use, distribution or reproduction is permitted which does not comply with these terms.
*Correspondence: Carmen Benítez-Benítez, Y2JlbmJlbjEmI3gwMDA0MDt1cG8uZXM=
†These authors have contributed equally to this work and share senior authorship