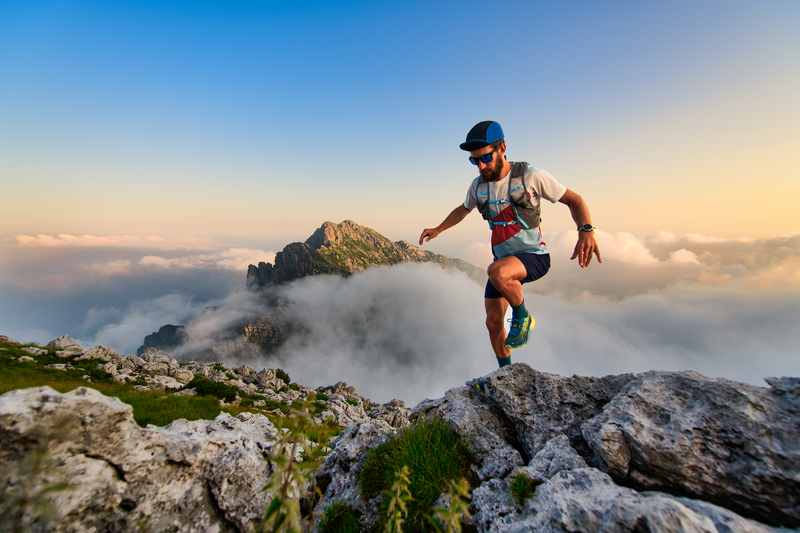
95% of researchers rate our articles as excellent or good
Learn more about the work of our research integrity team to safeguard the quality of each article we publish.
Find out more
REVIEW article
Front. Plant Sci. , 26 August 2021
Sec. Plant Metabolism and Chemodiversity
Volume 12 - 2021 | https://doi.org/10.3389/fpls.2021.733198
This article is part of the Research Topic Phenylpropanoid Systems Biology and Biotechnology View all 34 articles
Tricin (3',5'-dimethoxyflavone) is a specialized metabolite which not only confers stress tolerance and involves in defense responses in plants but also represents a promising nutraceutical. Tricin-type metabolites are widely present as soluble tricin O-glycosides and tricin-oligolignols in all grass species examined, but only show patchy occurrences in unrelated lineages in dicots. More strikingly, tricin is a lignin monomer in grasses and several other angiosperm species, representing one of the “non-monolignol” lignin monomers identified in nature. The unique biological functions of tricin especially as a lignin monomer have driven the identification and characterization of tricin biosynthetic enzymes in the past decade. This review summarizes the current understanding of tricin biosynthetic pathway in grasses and tricin-accumulating dicots. The characterized and potential enzymes involved in tricin biosynthesis are highlighted along with discussion on the debatable and uncharacterized steps. Finally, current developments of bioengineering on manipulating tricin biosynthesis toward the generation of functional food as well as modifications of lignin for improving biorefinery applications are summarized.
Flavonoids are a large group of plant-specialized metabolites that are ubiquitous in vascular plants and are also found in non-vascular plant lineages except hornworts (Yonekura-Sakakibara et al., 2019). Structurally, they are featured by a basic diphenylpropane (C6–C3–C6) backbone, which is usually made up of two benzene rings (A-ring and B-ring) and a middle pyrone ring (C-ring; Alseekh et al., 2020). Flavonoids are assigned to different classes according to the oxidation states in the C-rings (Schijlen et al., 2004). At least nine major classes, namely, flavanones, flavones, dihydroflavonols, flavonols, flavan-3-ols, leucoanthocyanidins, anthocyanidins, isoflavones, and aurones, have been described (Figure 1A; Yang et al., 2018; Nakayama et al., 2019).
Figure 1. Flavonoids in plants. (A) Structures of the major classes of flavonoids in plants. (B) Structures of tricin and flavone C-glycosides as the major flavone-derived metabolites in grasses. A-, B- and C-rings as well as the numbering system used for flavonoid molecules are indicated. R, R1, R2: H, OH, or OCH3.
In grasses, flavones are the predominant class of flavonoids accumulated in stems and leaves (Harborne and Hall, 1964; Tohge et al., 2017), whereas 3-hydroxylated flavonoids, such as flavonols and anthocyanidins, which are widely distributed in other plant lineages, are usually not accumulated due to the absence of flavanone 3-hydroxylase (F3H) expression (Deboo et al., 1995; Shih et al., 2008; Wang et al., 2020b). Grass flavones are present in the forms of flavone O-conjugates and flavone C-glycosides (Figure 1B; Harborne and Hall, 1964; Brazier-Hicks et al., 2009; Dong et al., 2014). Flavone O-conjugates harbor sugar or monolignol moieties linked to flavone aglycones through glycosidic or ether bonds (Li et al., 2016; Lan et al., 2019). 3',5'-Substituted flavone O-conjugates, in particular, tricin O-conjugates, are widely present (Dong et al., 2014; Li et al., 2016). On the other hand, flavone C-glycosides contain sugar moieties directly attached to C6 and/or C8 of the flavone backbones via C–C linkages (Besson et al., 1985; Cummins et al., 2006; Brazier-Hicks et al., 2009). Such flavone C-glycosides could be 3'-substituted but are rarely 3',5'-substituted (Dong et al., 2014). Unlike flavone O-conjugates, flavone C-glycosides are resistant to enzymatic or acid hydrolysis.
The flavone tricin has been drawing attention due to its widespread and abundant occurrence as soluble O-conjugates in grasses, and more remarkably, its unique incorporation in lignin polymers in cell walls of grasses and some other species. Soluble tricin was first isolated as an aglycone from leaves of a rust-resistant wheat cultivar (Triticum dicoccum; cv. Khapli; Anderson and Perkin, 1931). It was later found to be widely distributed in grasses and could also be detected in other monocots (e.g., Cyperaceae members), some dicots (e.g., Medicago species), and lycophytes (e.g., Lycopodium japonicum) [reviewed by Wollenweber and Dörr, (2008); Zhou and Ibrahim, (2010); Li et al. (2016)]. Soluble tricin-type metabolites usually exist as aglycone or tricin O-glycosides (predominately 5-O-, 7-O- and/or 4'-O-glucosides), tricin-oligolignols (predominately 4'-O-oligolignols and their derivatives), and their O-glycosides [reviewed by Zhou and Ibrahim, (2010); Li et al. (2016); Lan et al., (2019)]. Tricin C-glycosides (Theodor et al., 1981; Markham et al., 1987; Peterson and Rieseberg, 1987; Sun et al., 2013b), tricin sulfate, and tricin O-glycoside-O-sulfates (Harborne, 1975; Harborne and Williams, 1976; Barron et al., 1988; Galland et al., 2014) were also identified. In plants, soluble tricin-type metabolites were reported to function as defensive compounds against fungal pathogens (Kong et al., 2010), weeds (Kong et al., 2004), and insects (Adjei-Afriyie et al., 2000; Bing et al., 2007).
In the last decade, tricin was discovered to be incorporated into lignins (del Río et al., 2012), which are abundant structural polymers deposited together with cellulose and hemicelluloses in secondary cell walls of vascular plants. Tricin-integrated lignin (tricin-lignin; predominately 4'-O-conjugated to the β-position of the monolignol-derived phenylpropane units) is extensively distributed in grasses and is also detected in some non-grass monocot species [e.g., coconut (Cocos nucifera), curaua (Ananas erectifolius), and vanilla (Vanilla planifolia and V. phalaenopsis)] and the dicot alfalfa (Medicago sativa; Mao et al., 2013; You et al., 2013; Lan et al., 2016b). Tricin is the first lignin monomer known to be generated outside the monolignol biosynthetic pathways (del Río et al., 2012, 2020; Lan et al., 2015, 2016a, 2019). Currently, the physiological functions of tricin in cell wall lignins remain largely unknown.
To humans, tricin is considered promising nutraceutical due to its anticancer (Hudson et al., 2000; Yue et al., 2020), antioxidant (Ajitha et al., 2012), anti-inflammatory (Shalini et al., 2012, 2016), antiviral (Yazawa et al., 2010; Akuzawa et al., 2011), and antihistaminic activities [reviewed by Zhou and Ibrahim, (2010); Lan et al. (2016, 2019); Jiang et al. (2020)]. The potential use of tricin as a chemopreventive agent was notably well investigated (Hudson et al., 2000; Cai et al., 2004, 2005, 2009; Oyama et al., 2009; Chung et al., 2018; Tanaka et al., 2019; Wu and Tian, 2019; Yue et al., 2020). Tricin has been shown to be suitable for clinical development because of its excellent pharmacological efficacy (Cai et al., 2009) and low toxicity (Verschoyle et al., 2006), whereas its low bioavailability could be overcome by prodrug modifications (Ninomiya et al., 2011).
Elucidating the biosynthetic pathway for tricin is the pre-requisite for genetic manipulation of soluble and lignin-integrated tricin in different biotechnological applications. Here, we delineate the current understandings on tricin biosynthesis and discuss the present development and future prospects regarding the biotechnological aspects of engineering the biosynthetic pathway.
Same as other flavonoids, tricin is a downstream metabolite of the general phenylpropanoid pathway (Figure 2) in which ʟ-phenylalanine is first deaminated into cinnamate by phenylalanine ammonia-lyase (PAL; Camm and Towers, 1973; Elkind et al., 1990), followed by cinnamate 4-hydroxylase (C4H)-catalyzed para-hydroxylation of the aromatic ring to form p-coumarate (Russell and Conn, 1967; Russell, 1971; Schilmiller et al., 2009). Afterward, 4-coumarate:coenzymeA ligase (4CL) catalyzes the conversion of p-coumarate into p-coumaroyl-CoA, which serves as the precursor for the biosynthesis of different specialized metabolites, including flavonoids and lignin (Gui et al., 2011; Li et al., 2015). It is long believed that certain 4CL isoforms are specific for flavonoid biosynthesis (Hu et al., 1998; Ehlting et al., 1999; Sun et al., 2013a; Li et al., 2015).
Figure 2. General phenylpropanoid pathway and early flavonoid biosynthetic pathway. PAL, ʟ-phenylalanine ammonia-lyase; PTAL, ʟ-phenylalanine/ʟ-tyrosine ammonia-lyase; C4H, cinnamate 4-hydroxylase; 4CL, 4-hydroxycinnamate:CoA ligase; CHS, chalcone synthase; and CHI, chalcone isomerase. In green: general phenylpropanoid pathway. In purple: flavonoid biosynthetic pathway. In blue: monolignol biosynthetic pathway.
An alternative pathway using ʟ-tyrosine as a substrate to produce phenylpropanoids is also present in grasses (Figure 2; Barros et al., 2016). Bifunctional phenylalanine/tyrosine ammonia-lyases (PTAL) in maize and Brachypodium distachyon catalyze the deamination of ʟ-tyrosine to form p-coumarate, while at the same time, these enzymes also harbor PAL activities (Rosler et al., 1997; Barros et al., 2016). PALs and PTALs are highly conserved in grasses, suggesting the co-existence of two parallel pathways for phenylpropanoid production in Poaceae (Barros et al., 2016). In addition, results from feeding experiments using 13C-labelled ʟ-phenylalanine and ʟ-tyrosine in B. distachyon have suggested that PTAL is likely to be associated with the generation of grass-specific cell-wall-bound p-coumarate units (Barros et al., 2016). It is unknown whether tricin (soluble and lignin-bound) is derived from the PAL and/or PTAL pathway.
The initial biosynthetic steps and enzymes for flavonoid skeleton formation are highly conserved in the plant kingdom. Chalcone synthase (CHS), a prototype in the type III polyketide synthase superfamily, catalyzes sequential condensation of three malonyl-CoAs with p-coumaroyl-CoA to form naringenin chalcone (Figure 2). Chalcone isomerase (CHI)-catalyzed or occasionally spontaneous isomerization further converts naringenin chalcone into naringenin (a flavanone), which is the first flavonoid structure formed in the biosynthetic pathway. Naringenin is the precursor for all other flavonoids, including tricin. It was shown that deficiency of CHSs in maize and rice resulted in depletion in the accumulation of soluble and/or lignin-integrated tricin (Eloy et al., 2017; Wang et al., 2020a). Although it was not examined previously, CHIs are expected to be involved in tricin biosynthesis based on their conserved catalytic functions in the generation of all classes of flavonoids in plants.
Flavone O-conjugates and flavone C-glycosides are biosynthesized in separate pathways. Early radiotracer experiments on Lamnaceae plants revealed that 14C-labelled flavanone aglycones could be simultaneously converted into flavone O-glycosides and C-glycosides (Wallace and Grisebach, 1973), whereas 14C-labelled flavone aglycones could only be O-glycosylated but could not be C-glycosylated (Wallace et al., 1969). Accordingly, it was proposed that O-glycosylation occurs at the terminal step after the flavone aglycone is generated, whereas C-glycosylation takes place before flavone skeleton formation. Subsequently, crude enzyme extracts prepared from Fagopyrum esculentum cotyledons were shown to utilize 2-hydroxyflavanones, instead of flavanones or flavones, as substrates for C-glycosylation (Kerscher and Franz, 1987, 1988). These early speculations were substantiated by the characterization of flavone C-glycoside biosynthetic pathway in grasses a few decades later. To synthesize flavone C-glycosides, flavanones are first converted into 2-hydroxyflavanones by flavanone 2-hydroxylases (F2H; Figure 3), which are cytochrome P450 (CYP) monooxygenases belonging to the subfamily CYP93G (Du et al., 2010; Morohashi et al., 2012). Afterward, 2-hydroxyflavanones or their open ring isomers are C-glycosylated by C-glucosyltransferase, followed by dehydration to generate the flavone skeletons (Brazier-Hicks et al., 2009; Du et al., 2010; Ferreyra et al., 2013). Meanwhile, it was demonstrated that a rice mutant deficient in OsF2H was depleted in the accumulation of various flavone C-glycosides, but the production of tricin O-conjugates was not affected (Du et al., 2010). Evidently, flavone O-conjugates are synthesized in a separate pathway independent from flavone C-glycosides.
Figure 3. Flavone C-glycoside biosynthetic pathway. F2H, flavanone 2-hydroxylase; F3'H, flavonoid 3'-hydroxylase; FOMT, flavonoid O-methyltransferase; CGT, C-glycosyltransferase; DHT, dehydratase; and Glc, glucose. R: H, OH, or OCH3. In green: general phenylpropanoid pathway. In purple: flavonoid biosynthetic pathway.
Structural changes required for converting naringenin into tricin involve desaturation of the C2–C3 bond in the C-ring to generate the flavone nucleus, 3'- and 5'-hydroxylations in the B-ring, and subsequently 3'- and 5'-O-methylations. Two different types of enzymes, flavone synthase I (FNSI) and flavone synthase II (FNSII), were expected to convert flavanones into flavones by direct introduction of the C2–C3 double bond (Figure 4). FNSIs are Fe2+- and 2-oxoglutarate-requiring soluble enzymes, whereas FNSIIs are CYP enzymes bound to endoplasmic reticulum membranes (Martens and Mithöfer, 2005). Meanwhile, tricetin, a 3',5'-dihydroxylated flavone, was long proposed to be an intermediate along the tricin biosynthetic pathway (Cummins et al., 2006; Zhou and Ibrahim, 2010; Galland et al., 2014). Accordingly, sequential B-ring hydroxylations were expected to be catalyzed by flavonoid 3',5'-hydroxylases (F3'5'Hs). As all known F3'5'Hs accept different classes of flavonoids as substrates, 3',5'-hydroxylations might take place before and/or after flavone formation. Afterward, sequential 3',5'-O-methylations of tricetin presumably catalyzed by flavonoid O-methyltransferases would occur to produce tricin (Kim et al., 2006; Lin et al., 2006; Zhou et al., 2006, 2008, 2009). Collectively, the reaction steps for tricin biosynthesis were initially proposed to be: naringenin → apigenin → luteolin → tricetin → selgin → tricin (Galland et al., 2014) and/or naringenin → eriodictyol → dihydrotricetin → tricetin → selgin → tricin (Figure 4; Cummins et al., 2006; Zhou and Ibrahim, 2010).
Figure 4. Originally proposed tricin biosynthetic pathway. FNSI, flavone synthase I; FNSII, flavone synthase II; F3'H, flavonoid 3'-hydroxylase; F3'5'H, flavonoid 3',5'-hydroxylase; and FOMT, flavonoid O-methyltransferase. In green: general phenylpropanoid pathway. In purple: flavonoid biosynthetic pathway. Dotted arrows: originally proposed tricin biosynthetic pathway.
Using rice (Oryza sativa) as a model system, FNSII was identified to be the primary enzyme generating the flavone nucleus for tricin biosynthesis in grasses (Figure 5; Lam et al., 2014). Recombinant OsFNSII catalyzes direct conversions of flavanones, i.e., naringenin and eriodictyol, into apigenin and luteolin, respectively, in vitro (Brazier-Hicks and Edwards, 2013; Lam et al., 2014). In addition, over-expression of OsFNSII in Arabidopsis resulted in the accumulation of flavones (apigenin, luteolin, and chrysoeriol) O-glycosides which are normally not present in wild-type plants (Lam et al., 2014). Further analyses of the rice OsFNSII knockout mutant revealed substantial depletion of soluble tricin O-conjugates as well as tricin-lignin in cell walls, demonstrating the direct and predominant involvement of OsFNSII in the generation of both soluble and lignin-integrated tricin in rice (Lam et al., 2014, 2017). Moreover, the OsFNSII mutant accumulated soluble naringenin but not the other flavanones, e.g., eriodictyol (Lam et al., 2014), and generated altered lignins incorporated with naringenin (Lam et al., 2017), indicating that the in planta substrate of OsFNSII is primarily naringenin.
Figure 5. Current understanding on tricin biosynthetic pathway in grasses. FNSII, flavone synthase II; A3'H/C5'H, apigenin 3'-hydroxylase/chrysoeriol 5'-hydroxylase; F3'H, flavonoid 3'-hydroxylase; FOMT, flavonoid O-methyltransferase; COMT, caffeic acid O-methyltransferases; and CAldOMT, 5-hydroxyconiferaldehyde O-methyltransferase. In green: general phenylpropanoid pathway. In purple: flavonoid biosynthetic pathways. Dotted arrows: originally proposed tricin biosynthetic pathway.
OsFNSII, or CYP93G1, is a P450 enzyme belonging to the same CYP93G subfamily as OsF2H, or CYP93G2. Using naringenin as a common substrate, OsFNSII and OsF2H are the branch-point enzymes for the biosynthesis of tricin O-conjugates and flavone C-glycosides, respectively (Figures 3, 5). Phylogenetic analysis revealed that OsFNSII and OsF2H form two separate clades, each containing highly conserved sequences from the grass family (Figure 6A; Lam et al., 2017). Hence, sub-functionalization of CYP93G members probably preceded lineage divergence within Poaceae, resulting in the widespread distribution of the two classes of flavone-derived metabolites in grasses today. It is noteworthy that grass FNSIIs and F2Hs have a different phylogenetic origin from dicot FNSIIs and F2Hs, all of which exclusively belong to the CYP93B subfamily (Figure 6A; Kitada et al., 2001; Martens and Mithöfer, 2005; Zhang et al., 2007; Fliegmann et al., 2010; Wu et al., 2016; Zhao et al., 2016; Jiang et al., 2019). Noteworthily, grass species do not contain any CYP93B members and dicots do not have CYP93G members (Du et al., 2016).
Figure 6. Phylogeny of tricin biosynthetic enzymes. Phylogenetic trees of (A) FNSIIs and F2Hs; (B) A3'H/C5'Hs, F3'Hs, and F3'5'Hs; and (C) COMT/CAldOMTs in grasses and dicots, constructed based on previously published studies (Lam et al., 2017, 2019b; Lui et al., 2020). The unrooted phylogenetic trees were built by neighbor-joining method using MEGAX (Kumar et al., 2018). Bootstrapping with 1,000 replicates was performed. Scale bar denotes 0.1 substitutions per site.
Functionally, redundant enzymes other than FNSII are likely to be involved in tricin biosynthesis in grasses. For example, the rice OsFNSII mutant still accumulated soluble tricin and other flavones in anthers albeit at reduced levels compared with wild type (Wang et al., 2020a), while it shows substantial depletion of soluble tricin O-conjugates and tricin-lignin in vegetative tissues (Lam et al., 2014, 2017). In fact, two rice FNSIs were shown to catalyze the conversion of naringenin into apigenin in vitro (Kim et al., 2008; Lee et al., 2008b). In addition, maize possesses an FNSI (ZmFNSI-1) which shows in vitro FNS activities and results in the accumulation of flavones when over-expressed in Arabidopsis (Ferreyra et al., 2015; Righini et al., 2019).
In the plant kingdom, 3',5'-substituted flavonoids are patchily distributed, because F3'5'Hs, the enzymes responsible for catalyzing 5'-hydroxylation, are only present in isolated plant lineages (Tanaka and Brugliera, 2013). This is in contrast to the ubiquitous nature of flavonoid 3'-hydroxylases (F3'H; exclusively members of the CYP75B subfamily) that gives rise to the prevalence of 3'-substituted flavonoids (Tanaka and Brugliera, 2013). There have been strong interests for the investigation of F3'5'Hs as they are the key enzymes for the generation of delphinidin-derived anthocyanins, which confer blue or violet coloration in plant tissues, such as flowers and fruits (Tanaka and Brugliera, 2013). For ornamental purposes, transgenic expression of foreign F3'5'Hs has been employed to engineer novel blue or violet color in roses (Rosa hybrida), chrysanthemums (chrysanthemum morifolium), and carnations (Dianthus caryophyllus), all of which naturally lack delphinidin-derived anthocyanins (Katsumoto et al., 2007; Brugliera et al., 2013; Noda et al., 2013; Tanaka and Brugliera, 2013).
The canonical F3'5'Hs are CYP enzymes belonging to the CYP75A subfamily (Tanaka and Brugliera, 2013). Apparently, CYP75A-encoding genes have been lost repeatedly or became non-functional in many lineages during evolution (Tanaka and Brugliera, 2013). In rice, the only CYP75A member (CYP75A11) did not show any F3'5'H functions in in vitro enzyme assays or in CYP75A11 over-expressing transgenic Arabidopsis plants (Lam et al., 2015). On the other hand, a rice CYP75B member (CYP75B4) solely contributes to the 5'-hydroxylation activity during tricin biosynthesis, as evidenced by in planta metabolite analysis. For example, the CYP75B4 T-DNA knockout mutant is completely devoid of soluble selgin and tricin O-conjugates in vegetative tissues (Lam et al., 2015) and tricin-lignin in cell walls (Lam et al., 2019a). In addition, transgenic Arabidopsis co-expressing CYP75B4 and OsFNSII accumulates O-conjugates of selgin and tricin (Lam et al., 2015). Meanwhile, apigenin produced by OsFNSII using naringenin as a preferred in planta substrate was initially expected to undergo sequential B-ring hydroxylations to form tricetin as a tricin precursor. However, while CYP75B4 3'-hydroxylates apigenin to luteolin, it fails to 5'-hydroxylate luteolin to tricetin (Lam et al., 2015). Instead, CYP75B4 catalyzes 5'-hydroxylation of chrysoeriol to produce selgin (Lam et al., 2015). Chrysoeriol could be generated by 3'-O-methylation of luteolin, whereas selgin could undergo 5'-O-methylation to generate tricin. The flavonoid B-ring O-methylation reactions are known to be catalyzed by several O-methyltransferases in rice (see B-ring O-methylations below). Collectively, tricin biosynthetic pathway in rice has been re-established as: naringenin → apigenin → luteolin → chrysoeriol → selgin → tricin (Figure 5; Lam et al., 2015, 2019a). Meanwhile, chrysoeriol O-linked derivatives accumulates in rice vegetative tissues (Galland et al., 2014; Lam et al., 2015, 2019a; Eloy et al., 2017), whereas tricetin and its O-linked derivatives (e.g., O-conjugates) are rarely detected in grasses (Galland et al., 2014; Lam et al., 2015, 2019a; Eloy et al., 2017), supporting that chrysoeriol, instead of tricetin, is an intermediate along the tricin biosynthetic pathway.
CYP75B4 is a flavone-specific bifunctional B-ring hydroxylase in rice. It displays very weak 3'-hydroxylase activity toward naringenin while converting apigenin to luteolin readily (Lam et al., 2015; Park et al., 2016). In addition, its 5'-hydroxylation activity was restricted to chrysoeriol, but not any other 3'-methoxylated or 3'-hydroxylated flavonoids (Lam et al., 2015). Hence, the enzyme is now dedicated as apigenin 3'-hydroxylase/chrysoeriol 5'-hydroxylase (A3'H/C5'H). The dual catalytic activities have also been demonstrated in the highly conserved orthologs in sorghum (CYP75B97) and switchgrass (CYP75B11; Figure 6B), indicating that similar enzymology and intermediates were recruited for tricin biosynthesis in the grass family (Lam et al., 2019a). Further evidence indicated that the 3'-hydroxylation reaction (apigenin → luteolin) for tricin biosynthesis is also predominantly contributed by A3'H/C5'H. Thus, the rice CYP75B4 mutant accumulates elevated amounts of soluble apigenin metabolites along with the incorporation of apigenin into cell wall lignins (Lam et al., 2015, 2019a). On the other hand, CYP75B3, the only other CYP75B member in rice, is a canonical F3'H which catalyzes in vitro 3'-hydroxylation of a wide range of flavonoids including apigenin (Shih et al., 2008; Lam et al., 2015, 2019a; Park et al., 2016). However, CYP75B3 loss-of-function mutants are preferentially deficient in 3'-substituted flavone (luteolin and chrysoeriol) C-glycosides, while their production of soluble and lignin-integrated tricin remains unaffected (Lam et al., 2019a). Apparently, CYP75B3 primarily functions together with OsF2H along the separate biosynthetic pathway for flavone C-glycosides (Figure 3).
The highly conserved A3'H/C5'Hs in grasses are distinctive from other F3'5'Hs with regard to their phylogeny and catalytic properties. They are phylogenetically distant from CYP75A F3'5'Hs and were likely recruited through neofunctionalization of an ancestral CYP75B F3'H protein (Figure 6B). Similarly, several Asteraceae species had acquired CYP75B F3'5'Hs independently through convergent evolution, leading to delphinidin-derived anthocyanin pigmentation (Seitz et al., 2006; Seitz et al., 2015). In addition, the grass A3'H/C5'Hs are substrate specific for both 3'-hydroxylation (apigenin) and 5'-hydroxylation (chrysoeriol), while CYP75A and Asteraceae CYP75B F3'5'Hs could utilize a variety of non-substituted, 3'-hydroxylated and 3'-methoxylated flavonoids as substrates. Intriguingly, the unique catalytic properties of A3'H/C5'Hs are reminiscent of the bifunctional phenylpropanoid meta-hydroxylase (CYP788A1) required for syringyl (S)-lignin biosynthesis in the spikemoss Selaginella moellendorffii. CYP788A1 is involved in both 3- and 5-hydroxylations of phenylpropanoids, but it could only catalyze 5-hydroxylation after 3-O-methylation (Weng et al., 2008, 2010).
Several cation-independent OMTs in grasses were found to catalyze in vitro O-methylation of flavones in grasses (Kim et al., 2006; Lin et al., 2006; Zhou et al., 2006, 2008, 2009). Interestingly, these enzymes have been annotated as caffeic acid O-methyltransferases (COMT) or 5-hydroxyconiferaldehyde O-methyltransferases (CAldOMT) as they also show in vitro O-methylation activities toward 5-hydroxyconiferaldehyde, 5-hydroxyferulic acid, and caffeic acid, which are intermediates in the monolignol pathway; hence, they are also involved in S-lignin biosynthesis (Figure 7A; Collazo et al., 1992; Piquemal et al., 2002; Ma and Xu, 2008; Sattler et al., 2012; Koshiba et al., 2013).
Figure 7. Current understanding on lignin biosynthesis in grasses. (A) Monolignol biosynthetic pathways. Major activity of COMT/CAldOMT in monolignol biosynthetic pathways is indicated. (B) Radical coupling of tricin and monolignols to produce tricin-lignin polymers. 4CL, 4-hydroxycinnamate:CoA ligase; C3H, p-coumarate 3-hydroxylase; HCT, p-hydroxycinnamoyl-CoA:quinate/shikimate esterase; APX, ascorbate peroxidase; C3'H, p-coumaroyl ester 3-hydroxylase; CSE, caffeoyl shikimate esterase; CCoAOMT, caffeoyl-CoA O-methyltransferase; CAld5H, coniferaldehyde 5-hydroxylase; COMT, caffeic acid O-methyltransferases; CAldOMT, 5-hydroxyconiferaldehyde O-methyltransferase; CCR, cinnamoyl-CoA reductase; CAD, cinnamyl alcohol dehydrogenase; PMT, p-coumaroyl-CoA:monolignol transferase; LAC, laccase; and PRX, peroxidase. In green: general phenylpropanoid pathway. In purple: flavonoid biosynthetic pathways. In blue: monolignol biosynthetic pathways. T, tricin; M, monolignols and their derivatives; pCA, p-coumarate; and Ac, acetate.
Recently, knockout and knockdown mutant analyses have demonstrated that grass COMT/CAldOMTs are actually bifunctional enzymes required for both tricin and S-lignin biosynthesis (Fornalé et al., 2016; Eudes et al., 2017; Daly et al., 2019; Lam et al., 2019b). Rice and sorghum deficient in COMT/CAldOMT accumulated reduced levels of soluble tricin but increased levels of selgin (mono-methoxylated) and luteolin (non-methoxylated) when compared with wild-type controls (Lam et al., 2015; Eudes et al., 2017). In addition, maize, rice, and sorghum plants deficient in COMT/CAldOMT were depleted in both tricin-lignin and S-lignin (Fornalé et al., 2016; Eudes et al., 2017; Lam et al., 2019b). Apparently, the highly conserved grass COMT/CAldOMT orthologs (Figure 6C) have likely evolved dual catalytic functions for the two parallel biosynthetic pathways of flavonoids and monolignols, contributing to the widespread occurrence of soluble and lignin-integrated tricin metabolites in the grass family nowadays.
Based on the revised tricin biosynthetic pathways and the new findings in the COMT/CAldOMT-deficient grass plants, the catalytic activities of COMT/CAldOMTs were re-examined. Recombinant COMT/CAldOMTs in rice and sorghum were found to catalyze 3'-O-methylation of luteolin and 5'-O-methylation of selgin (Kim et al., 2006; Lin et al., 2006; Zhou et al., 2006; Eudes et al., 2017; Lam et al., 2019b), which are the substrates of COMT/CAldOMTs in the tricin biosynthetic pathway (Figure 5). Meanwhile, rice OsCAldOMT1 shows comparable catalytic efficiencies toward selgin and 5-hydroxyconiferaldehyde, which are the substrates of COMT/CAldOMTs in tricin and monolignol biosynthetic pathway, respectively (Figures 5, 7A), further suggesting the bifunctional roles of COMT/CAldOMTs in tricin and monolignol biosynthesis in grasses (Lam et al., 2019b).
Functionally redundant OMTs other than COMT/CAldOMTs appear to be present for the biosynthesis of tricin in grasses as tricin-derived metabolites, including tricin-lignin, are not completed depleted in the COMT/CAldOMT loss-of-function mutants in maize, sorghum, and rice (Lam et al., 2015, 2019b; Fornalé et al., 2016; Eudes et al., 2017). In fact, several cation-dependent caffeoyl-CoA O-methyltransferase (CCoAOMT)-related enzymes could catalyze 3',5'-O-methylation using various flavone substrates (Lee et al., 2008a), but their involvement in tricin biosynthesis in planta requires further investigations.
Based on the types of soluble tricin metabolites detected in grasses, O-glycosylations and O-conjugations with monolignols and their acylated derivatives represent the predominant structural modifications of tricin (Dong et al., 2014; Lan et al., 2016a; Eloy et al., 2017; Peng et al., 2017). These modifications occur after the formation of tricin aglycone (Hong et al., 2007; Jiang et al., 2016; Lan et al., 2016a).
O-Glycosylations of flavonoids are usually catalyzed by uridine diphosphate (UDP)-dependent glycosyltransferases (UGT; family 1 glycosyltransferases 1; GT1; Ko et al., 2006; Yonekura-Sakakibara and Hanada, 2011; Kim et al., 2015), which utilize UDP sugars as sugar donors (Yang et al., 2018). A number of UGTs from rice (Ko et al., 2006, 2008; Hong et al., 2007; Luang et al., 2013; Chen et al., 2014; Peng et al., 2017) and wheat (Shi et al., 2020) are capable of catalyzing the conjugation of sugars, usually glucose, to one or multiple hydroxyl groups of tricin in vitro and/or when over-expressed in transgenic plants. Single-nucleotide polymorphisms (SNPs) in several putative UGTs were also found to be directly associated with the variations of flavone O-glycoside accumulation in different natural cultivars and/or recombinant inbred lines of rice (Chen et al., 2014; Dong et al., 2014; Peng et al., 2017; Li et al., 2019) and wheat (Shi et al., 2020). The different O-glycosylations could enhance solubility and stability, and might be involved in regulating storage, transport, and detoxification of tricin (Yonekura-Sakakibara and Hanada, 2011).
In addition to sugars, tricin conjugates with monolignols and their derivatives, leading to the formation of soluble tricin-oligolignols along with insoluble tricin-lignin in the cell walls. The soluble tricin-oligolignols in grasses have been found to be either optically active (Wenzig et al., 2005; Xiong et al., 2011) or inactive (racemic; Lan et al., 2016a). The optically active tricin-oligolignols, which have been often referred to as “flavonolignans” (Begum et al., 2010; Chambers et al., 2015; Csupor et al., 2016), may be formed by oxidative radical coupling of tricin with monolignols or their derivatives with the assistance of dirigent proteins, similar to the biosynthesis of lignans (Davin and Lewis, 2003; Umezawa, 2003; Paniagua et al., 2017), in which dirigent proteins serve as auxiliary proteins for guiding the regioselective and stereoselective coupling of phenoxy radicals from monolignols and their analogs. For example, the absolute configuration of a diastereomeric pair of β–O–4 neolignan-type flavonolignans, threo-(−)-guaiacylglycerol-β-tricin ether [(−)-salcolin A], and erythro-(−)-guaiacylglycerol-β-tricin ether [(−)-salcolin B] isolated from Sinocalamus affinis (Poaceae) were determined as 7''S,8''S and 7''R,8''S, respectively (Xiong et al., 2011). This strongly suggests that the coupling between tricin and coniferyl alcohol radicals to form 4'–O–8'' bond proceeds enantioselectively, probably mediated by a dirigent protein, giving rise to the optically active quinonemetide, which are then attacked by water non-stereoselectively, giving rise to both (−)-(7''S,8''S)-salcolin A and (−)-(7''R,8''S)-salcolin B (Figure 8). This is in line with the recent findings that a dirigent protein, AtDIR12/AtDP1, was involved in the formation of arylglycerol-β-aryl ether (β–O–4) type neolignans in Arabidopsis (Yonekura-Sakakibara et al., 2021). However, from Avena sativa, (−)-salcolin A and (+)-salcolin B were isolated (Wenzig et al., 2005). In this case, the diastereomers should have opposite absolute configuration at 8'' position, forming (−)-(7''S,8''S)-salcolin A and (+)-(7''S,8''R)-salcolin B (Figure 8). During their formation, the radical coupling should afford racemic quinonemethide in terms of 8'' position, and the following water addition at 7'' position should be diastereoselective to give rise to the optically active diastereomers (Wenzig et al., 2005). On the other hand, optically inactive tricin-oligolignols are generated solely by radical coupling (Figure 7B) and are considered to exist at least partially as the precursors for the generation of tricin-lignin polymers (see Tricin-lignin formation below; Lan et al., 2016a).
Figure 8. A possible mechanism for the formation of optically active salcolin A and salcolin B through radical coupling of tricin and coniferyl alcohol. Different possible stereoisomers of salcolin A and salcolin B are shown.
Tricin is incorporated into lignin polymers in grass cell walls by radical coupling (Lan et al., 2015), essentially the same way lignification takes place solely with monolignols (coniferyl alcohol, sinapyl alcohol, and p-coumaryl alcohol) in typical non-grass vascular plants (i.e., gymnosperms, dicots, and non-grass monocots). The compatibility of tricin with radical coupling was demonstrated by biomimetic oxidations of tricin with monolignols using peroxidase/hydrogen peroxide and silver (I) oxide as oxidants (Lan et al., 2015). Tricin was found to cross-couple to monolignols exclusively via the 4'–O–β-coupling mode (Figure 7B; del Río et al., 2012; Lan et al., 2015), probably because the radical from the 4'-hydroxyl group of tricin is more stabilized than the other possible radicals as supported by a density functional theory study (Elder et al., 2020). Thus, in plant cell walls, it is expected that tricin is first oxidized by phenol oxidases, presumably laccases (LAC) and/or peroxidases (PRX; Figure 7A; Tobimatsu and Schuetz, 2019), and then coupled with monolignol radicals or acylated monolignol radicals to form tricin-(4'–O–β)-linked phenylpropane units in the lignin polymers (Figure 7B). As tricin is unable to undergo dehydrodimerization, and it does not cross-couple directly with growing lignin polymers, tricin predominantly incorporates into the starting ends of the final lignin polymer chains (Lan et al., 2015). Thus, tricin is expected to serve as a nucleation site for lignification (Lan et al., 2015; Berstis et al., 2021).
Lignin-integrated tricin content in grasses was estimated to be around 0.5–7mg/g whole cell wall or 2–33mg/g lignin by thioacidolysis (Lan et al., 2016b). These contents are several folds higher than extractable tricin content (Lan et al., 2016b), suggesting that the majority of tricin synthesized in grasses is incorporated into lignin polymers in cell walls.
In contrast to their prevalence in grasses and other monocot lineages, tricin-derived metabolites are only sporadically distributed in dicots. Metabolomics studies have reported their occurrences in several dicot lineages, spanning from basal dicots, like individual Ranunculus spp. (Li et al., 2005; Aslam et al., 2012), to two core dicot lineages: rosids [e.g., Agelaea pentagyna (family: Connaraceae), Medicago legumes and Trigonella foenum-graecum (family: Fabaceae); Kuwabara et al., 2003], and asterids [e.g., Artemisia vulgaris (family: Asteraceae), Leucas cephalotes (family: Lamiaceae), and Lonicera japonica (family: Caprifoliaceae)] (Miyaichi et al., 2006). Meanwhile, tricin-lignin is only detected in leaves of alfalfa, albeit at much lower quantity than those in grasses (Lan et al., 2016b). Intriguingly, although tricin is restricted to certain dicot lineages, its flavone precursors, including apigenin, luteolin, and/or chrysoeriol, are widely distributed in non-tricin-accumulating dicots (Harborne, 1974). Hence, the occurrences of tricin derivatives are probably resulting from independent and convergent recruitment of novel enzyme activities in those isolated tricin-accumulating dicot lineages.
Three possible types of dicot enzymes, FNSIs, FNSIIs, and F2Hs, have been described for flavone nucleus formation (Martens et al., 2001; Martens and Mithöfer, 2005; Zhang et al., 2007; Ferreyra et al., 2015; Li et al., 2020a), but their contribution to tricin biosynthesis remains elusive in tricin-producing dicots. Both FNSIs (Britsch, 1990; Martens et al., 2001; Miyahisa et al., 2006; Yun et al., 2008) and FNSIIs (Kitada et al., 2001; Fliegmann et al., 2010; Wu et al., 2016; Zhao et al., 2016; Jiang et al., 2019) catalyze direct desaturation of flavanones into flavones, whereas F2Hs converts flavanones to 2-hydroxyflavanones which were proposed to be intermediates for generating the flavone skeleton (Akashi et al., 1998; Zhang et al., 2007).
Initially identified in parsley (Petroselinum crispum), FNSIs were long presumed to be confined to Apiaceae (Britsch, 1990; Martens et al., 2001; Yun et al., 2008). However, they were subsequently isolated from other dicots, including Arabidopsis (Ferreyra et al., 2015) and Morus notabilis (Li et al., 2020). Interestingly, angiosperm FNSIs outside Apiaceae are apparently phylogenetically unrelated to FNSIs in Apiaceae and non-vascular plants; thus, FNSIs were probably evolved convergently in distant plant lineages (Li et al., 2020). Meanwhile, all the known dicot FNSIIs and F2Hs are CYP enzymes belonging to the CYP93B subfamily (Kitada et al., 2001; Martens and Mithöfer, 2005; Zhang et al., 2007; Fliegmann et al., 2010; Wu et al., 2016; Zhao et al., 2016; Jiang et al., 2019). FNSIIs are present in most flavone-accumulating dicots, such as Gerbera hybrids (Martens and Forkmann, 1999), Lonicera japonica, L. macranthoides (Wu et al., 2016), Glycine max (Fliegmann et al., 2010; Jiang et al., 2010), Glycyrrhiza echinate (Akashi et al., 1999), Salvia miltiorrhiza (Deng et al., 2018), and Scutellaria baicalensis (Zhao et al., 2016). On the other hand, F2Hs were only reported in a few dicot species, including G. echinata (Akashi et al., 1998), Chrysanthemum indicum (Jiang et al., 2019), and M. truncatula (Zhang et al., 2007). It remains to be investigated whether FNSI, FNSII, and/or F2H are required for tricin biosynthesis which is restricted to isolated dicot lineages, such as the Medicago legumes.
Considerable knowledge about the 3'- and 5'-hydroxylation reactions required for tricin biosynthesis in Medicago legumes has come to light recently (Lui et al., 2020). Canonical CYP75A F3'5'Hs are not involved in the B-ring modifications, but instead, a group of Medicago-unique CYP75B proteins, including M. truncatula MtFBH-4 as well as alfalfa (M. sativa) MsFBH-4 and MsFBH-10, are utilized. In in vitro enzyme assays, these CYP proteins catalyze 3'-hydroxylation of different flavonoid classes (flavanone, flavone, and flavonol) and 5'-hydroxylation of their 3'-methoxylated derivatives which include chrysoeriol. Furthermore, apigenin is converted to 3'- and 5'-substituted flavones (i.e., luteolin, chrysoeriol, selgin, and tricin) when these CYP75B proteins are transiently expressed in Nicotiana benthamiana leaves. Consistent with these findings, M. truncatula MtFBH-4 knockout mutants are completely depleted in tricin O-glycosides, hence establishing an indispensable role of MtFBH-4 in tricin biosynthesis. Basically, the same reaction steps that occur in grasses (Figure 5) have been acquired independently by the Medicago legumes to produce tricin.
The Medicago-unique CYP75B enzymes required for tricin biosynthesis are distinct from the grass A3'H/C5'Hs with regard to their catalytic properties and phylogenetic origins (Lam et al., 2015; Lui et al., 2020). For example, the 5'-hydroxylase activity is restricted to chrysoeriol for the grass enzymes but is extended to other 3'-methoxylated flavonoids for the Medicago enzymes. Interestingly, the Thr-to-Gly substitution in the substrate recognition site 6 domain is critical for these Medicago enzymes to catalyze the 5'-hydroxylation reactions (Lui et al., 2020). On the other hand, the equivalent position is replaced by a Leu residue in the grass A3'H/C5'Hs (Lui et al., 2020), but it is unknown whether this could account for their more specific substrate preference for 5'-hydroxylation. Meanwhile, the Medicago-unique CYP enzymes have likely acquired the novel 5'-hydroxylase activities through neofunctionalization of redundant CYP75B F3'Hs following the divergence of the Medicago genus from other lineages in the legume family (Lui et al., 2020). Convergent evolution of CYP75B F3'5'H had also occurred independently in several Asteraceae lineages for the generation of delphinidin-derived blue/violet pigments (Seitz et al., 2006, 2015). By sharp contrast, A3'H/C5'Hs are highly conserved amongst grasses, consistent with prevalence of tricin in the grass family (Lam et al., 2019a). It would be intriguing to decipher the enzymology and evolution of B-ring hydroxylations for tricin biosynthesis in other isolated dicot lineages.
The enzymes responsible for the 3'- and 5'-O-methylation reactions remain elusive for tricin biosynthesis in dicots. It is possible that they are also COMT/CAldOMT enzymes, as in the case for the grass bifunctional OMTs. In fact, Arabidopsis knockout mutant analyses demonstrated the dual roles of COMT/CAldOMT in the production of monolignols and flavonoids (Do et al., 2007; Tohge et al., 2007; Nakatsubo et al., 2008). However, there is no tricin accumulation in Arabidopsis, presumably due to the absence of F3'5'H enzymes. Meanwhile, the expression of an endogenous COMT gene is upregulated in transgenic alfalfa over-expressing the gene encoding N-acetylserotonin O-methyltransferase (MsASMT1), which catalyzes the final step in melatonin biosynthesis (Cen et al., 2020). In addition to increased melatonin formation, the transgenic alfalfa plants produced elevated amounts of various soluble chrysoeriol- and tricin-derived metabolites (Cen et al., 2020), which might be resulting from increased COMT activities. However, FgCOMT1 isolated from the tricin-accumulating legume fenugreek (Trigonella foenum-graecum; Kuwabara et al., 2003) could O-methylate 5-hydroxyferulic acid but not quercetin (a 3'-hydroxylated flavonol) or tricetin in vitro (Qin et al., 2012). Over-expression of FgCOMT1 in Arabidopsis atomt1 knockout mutant only partially restored the accumulation of sinapoyl aldehyde and sinapic acid (intermediates of the monolignol biosynthetic pathway) but not isorhamnetin (a 3'-methoxylated flavonol; Qin et al., 2012).
Cereals contribute to more than half of the world population’s daily caloric intake, but the commonly consumed polished grains, which are mainly consisting of endosperms, are poor in phytochemicals and minerals (Awika, 2011). Their consumption as staple food in developing countries is associated with micronutrient malnutrition due to the lack of dietary diversity (Bhullar and Gruissem, 2013). To overcome this problem, biofortification through metabolic engineering has been pursued to introduce different phytochemicals and minerals in endosperms of cereal grains (Bhullar and Gruissem, 2013; Saltzman et al., 2013). As a prime example, golden rice engineered with the β-carotene biosynthetic pathway in endosperm was developed to combat vitamin A deficiency (Ye et al., 2000; Paine et al., 2005; Owens, 2018). Following the success of golden rice, cereal crops that accumulate high contents of iron, zinc, and various carotenoids in the edible endosperm have been developed using genetic engineering (Wirth et al., 2009; Johnson et al., 2011; Saltzman et al., 2013; Blancquaert et al., 2015; Singh et al., 2017; Zhu et al., 2018). Recently, transgenic rice with endosperms fortified with flavonoids, anthocyanins, or stilbenoids was also successfully engineered (Baek et al., 2013; Ogo et al., 2013; Zhu et al., 2017), representing potential functional staple food containing different health-beneficial phenolics.
Although tricin and its derivatives have been characterized with many different health-promoting properties (Cai et al., 2004; Duarte-Almeida et al., 2007; Yazawa et al., 2011; Murayama et al., 2012; Jung et al., 2014, 2015; Lee et al., 2015; Shalini et al., 2016), they are rarely present in human diets. Tricin is abundant in vegetative tissues of grasses but is not present in cereal endosperm due to the absence of expression of genes required for tricin biosynthesis (Ogo et al., 2013). Primary dietary sources of tricin include whole cereal grains such as rice, wheat, oat, and barley, in which small amounts of tricin are preserved in the bran (pericarp, testa, aleurone, and embryo; Poulev et al., 2018, 2019), as well as some grass-derived food products, such as sugarcane juice (Duarte-Almeida et al., 2007) and barley leaf powders (Zeng et al., 2018).
Functional food crops that are fortified with tricin could be generated by engineering the entire biosynthetic pathway in edible tissues. Previously, transgenic rice seeds that accumulate tricin were generated by expression of genes from multiple species encoding rice PAL, rice CHS, parsley FNSI, soybean FNSII, blue viola F3'5'H, and rice COMT/CAldOMT (Ogo et al., 2013). Recent establishment of the endogenous biosynthetic pathways in grasses (Lam et al., 2014, 2015, 2019a) and Medicago legumes (Lui et al., 2020) as well as further elucidation of the regulatory mechanism should facilitate more effective metabolic engineering in plants or edible tissues that do not naturally produce tricin-type metabolites.
Grasses show great potential as a source of lignocellulosic biomass. A large amount of lignocellulose is produced annually as agricultural residues from worldwide cultivation of grass grain crops, including maize, wheat, rice, barley, and sorghum, as well as grass sugar crops, such as sugarcane and sweet sorghum. In addition, grass energy crops, such as Miscanthus, Erianthus, switchgrass, and bamboo, which show notably high biomass productivity, are attractive lignocellulose feedstocks for various biorefinery applications (Tye et al., 2016; Bhatia et al., 2017; Umezawa, 2018; Umezawa et al., 2020). Because of the prominent impacts of lignin on the usability of lignocellulose in both polysaccharide- and lignin-oriented biorefinery applications, bioengineering approaches to control lignin content and structure in grass cell walls have been actively investigated (Umezawa, 2018, 2020; Halpin, 2019; Coomey et al., 2020). However, due to our limited knowledge regarding the biological functions and physicochemical properties of tricin-lignin, it is still uncertain how tricin-lignin influences the usability of grass biomass. Thus far, not much has been examined on the effects of manipulating tricin biosynthesis on the utilization properties of grass biomass for different biorefinery applications.
As tricin could serve as a nucleation site for lignification, reducing the content of tricin used for lignification may result in reduction of lignin content and biomass recalcitrance, which may in turn improve the production of fermentable sugars from biomass in the polysaccharide-oriented biorefinery processes (Halpin, 2019). Indeed, tricin-depleted rice mutants deficient in FNSII (Lam et al., 2017) or A3'H/C5'H (Lam et al., 2017, 2019a) displayed reduced lignin content and improved cell wall digestibility. In contrast, however, tricin-depleted maize mutant deficient in CHS showed increased lignin level and reduced cell wall digestibility in leaves albeit no alteration in either lignin content or cell wall digestibility in stems (Eloy et al., 2017). The altered lignin content in the CHS-deficient maize leaf cell walls was attributed at least partially to the consequence of the increased carbon flux toward the branching monolignol biosynthesis pathway upon the blockage of the entry of the flavonoid pathway where CHS plays the major role (Eloy et al., 2017). These studies on tricin-depleted grass mutants implicated that disrupting tricin biosynthetic genes not only impedes the formation of tricin-lignin but also affects the formation of the core lignin polymer units derived from monolignols, although the mechanisms underlying this phenomenon remain unclear. Further manipulations of different tricin biosynthetic genes in different grass species are imperative to determine the precise relationships between tricin, lignin content and composition, and cell wall digestibility in tricin-depleted grasses.
On the other hand, increasing the levels of tricin serving as initiation sites for lignin polymerization would theoretically reduce the molecular weight of the lignin polymers, which may potentially improve the efficiency of lignin deconstruction in the polysaccharide-oriented biorefinery processes (Berstis et al., 2021). A recent computational study determined that the bond strengths of the 4'–O–β linkages between the tricin- and monolignol-derived lignin polymer units are comparable to the major β–O–4 linkages connecting the internal monolignol-derived lignin polymer units, suggesting that introduction of more tricin units in lignin polymers is unlikely to increase the energy for lignin depolymerization (Berstis et al., 2021). Nonetheless, whether such tricin bioengineering strategy to attenuate lignin molecular weight and depolymerization efficiency requires further exploration.
Meanwhile, grass crops bioengineered toward high tricin-lignin content could bring benefits in the lignin-oriented biorefinery approaches by amplifying the supply of tricin or tricin-derived aromatic chemicals. It has been estimated that large quantity of tricin could be released from grass lignins (Ralph, 2020; del Río et al., 2020). However, challenges ahead include developing technologies for efficient extraction and isolation of tricin from grass lignins to meet the stringent purity specifications as well as industrializing the production with maximized cost effectiveness and minimized environmental impacts. As the most abundant aromatic polymers on Earth, lignin has a great potential to serve as starting materials for sustainable production of bulk or functionalized aromatic chemicals (Ragauskas et al., 2014; Rinaldi et al., 2016; Umezawa et al., 2020). Accordingly, chemical and biochemical approaches to depolymerize lignin into useful low molecular weight aromatic compounds have been extensively pursued (Schutyser et al., 2018; Sun et al., 2018; Renders et al., 2019; Abu-Omar et al., 2021). As these studies have mostly focused on the conversions of the major monolignol-derived phenylpropane units in lignin, the consequences of lignin-integrated tricin units in various catalytic and bio-catalytic lignin depolymerization strategies remain an intriguing subject for further investigations.
PL, YT, and CL wrote the manuscript with help from all the other authors. All authors contributed to the article and approved the submitted version.
This work was supported in part by the Research Grants Council of Hong Kong, China (grant nos. GRF17113217, GRF17126918, and GRF17104720), HKU seed fund (grant no. 201910159284), the Japan Society for the Promotion of Science (grant nos. #JP16H06198 and #JP20H03044), and Research Institute for Sustainable Humanosphere, Kyoto University (the Research Grant for Mission Research on Sustainable Humanosphere).
The authors declare that the research was conducted in the absence of any commercial or financial relationships that could be construed as a potential conflict of interest.
All claims expressed in this article are solely those of the authors and do not necessarily represent those of their affiliated organizations, or those of the publisher, the editors and the reviewers. Any product that may be evaluated in this article, or claim that may be made by its manufacturer, is not guaranteed or endorsed by the publisher.
Abu-Omar, M. M., Barta, K., Beckham, G. T., Luterbacher, J. S., Ralph, J., Rinaldi, R., et al. (2021). Guidelines for performing lignin-first biorefining. Energy Environ. Sci. 14, 262–292. doi: 10.1039/D0EE02870C
Adjei-Afriyie, F., Kim, C.-S., Takemura, M., Ishikawa, M., Tebayashi, S.-I., and Horiike, M. (2000). Probing stimulants from the rice plant towards the smaller brown planthopper, Laodelphax striatellus (fallen)(Homoptera: Delphacidae). Z. Naturforsch. C. J. Biosci. 55, 1038–1044. doi: 10.1515/znc-2000-11-1232
Ajitha, M. J., Mohanlal, S., Suresh, C. H., and Jayalekshmy, A. (2012). DPPH radical scavenging activity of tricin and its conjugates isolated from “Njavara” rice bran: A density functional theory study. J. Agric. Food Chem. 60, 3693–3699. doi: 10.1021/jf204826e
Akashi, T., Aoki, T., and Ayabe, S.-i. (1998). Identification of a cytochrome P450 cDNA encoding (2S)-flavanone 2-hydroxylase of licorice (Glycyrrhiza echinata L.; Fabaceae) which represents licodione synthase and flavone synthase II. FEBS Lett. 431, 287–290. doi: 10.1016/S0014-5793(98)00781-9
Akashi, T., Fukuchi-Mizutani, M., Aoki, T., Ueyama, Y., Yonekura-Sakakibara, K., Tanaka, Y., et al. (1999). Molecular cloning and biochemical characterization of a novel cytochrome P450, flavone synthase II, that catalyzes direct conversion of flavanones to flavones. Plant Cell Physiol. 40, 1182–1186. doi: 10.1093/oxfordjournals.pcp.a029505
Akuzawa, K., Yamada, R., Li, Z., Li, Y., Sadanari, H., Matsubara, K., et al. (2011). Inhibitory effects of tricin derivative from Sasa albo-marginata on replication of human cytomegalovirus. Antivir. Res. 91, 296–303. doi: 10.1016/j.antiviral.2011.06.014
Alseekh, S., de Souza, L. P., Benina, M., and Fernie, A. R. (2020). The style and substance of plant flavonoid decoration; towards defining both structure and function. Phytochemistry 174:112347. doi: 10.1016/j.phytochem.2020.112347
Anderson, J. A., and Perkin, A. G. (1931). CCCLXV.—The yellow colouring matter of khapli wheat, Triticum dicoccum. J. Chem. Soc. 2624–2625. doi: 10.1039/JR9310002624
Aslam, M. S., Choudhary, B. A., Uzair, M., and Ijaz, A. S. (2012). The genus ranunculus: a phytochemical and ethnopharmacological review. Int. J. Pharm. Pharm. Sci 4, 15–22.
Awika, J. M. (2011). “Major cereal grains production and use around the world,” in Advances in Cereal Science: Implications to Food Processing and Health Promotion. eds. J. M. Awika, V. Piironen, and S. Bean (Washington: ACS Publications), 1–13.
Baek, S.-H., Shin, W.-C., Ryu, H.-S., Lee, D.-W., Moon, E., Seo, C.-S., et al. (2013). Creation of resveratrol-enriched rice for the treatment of metabolic syndrome and related diseases. PLoS One 8:e57930. doi: 10.1371/journal.pone.0057930
Barron, D., Varin, L., Ibrahim, R. K., Harborne, J. B., and Williams, C. A. (1988). Sulphated flavonoids—an update. Phytochemistry 27, 2375–2395. doi: 10.1016/0031-9422(88)87003-1
Barros, J., Serrani-Yarce, J. C., Chen, F., Baxter, D., Venables, B. J., and Dixon, R. A. (2016). Role of bifunctional ammonia-lyase in grass cell wall biosynthesis. Nat. Plants 2:16050. doi: 10.1038/nplants.2016.50
Begum, S. A., Sahai, M., and Ray, A. B. (2010). “Non-conventional lignans: coumarinolignans, flavonolignans, and stilbenolignans,” in Fortschritte Der Chemie Organischer Naturstoffe/Progress in the Chemistry of Organic Natural Products. eds. A. Kinghorn, H. Falk, and J. Kobayashi (Vienna: Springer), 1–70.
Berstis, L., Elder, T., Dixon, R., Crowley, M., and Beckham, G. T. (2021). Coupling of flavonoid initiation sites with monolignols studied by density functional theory. ACS Sustain. Chem. Eng. 9, 1518–1528. doi: 10.1021/acssuschemeng.0c04240
Besson, E., Dellamonica, G., Chopin, J., Markham, K. R., Kim, M., Koh, H.-S., et al. (1985). C-glycosylflavones from Oryza sativa. Phytochemistry 24, 1061–1064. doi: 10.1016/S0031-9422(00)83183-0
Bhatia, R., Gallagher, J. A., Gomez, L. D., and Bosch, M. (2017). Genetic engineering of grass cell wall polysaccharides for biorefining. Plant Biotechnol. J. 15, 1071–1092. doi: 10.1111/pbi.12764
Bhullar, N. K., and Gruissem, W. (2013). Nutritional enhancement of rice for human health: the contribution of biotechnology. Biotechnol. Adv. 31, 50–57. doi: 10.1016/j.biotechadv.2012.02.001
Bing, L., Hongxia, D., Maoxin, Z., Di, X., and Jingshu, W. (2007). Potential resistance of tricin in rice against brown planthopper Nilaparvata lugens (Stål). Acta Ecol. Sin. 27, 1300–1306. doi: 10.1016/S1872-2032(07)60031-6
Blancquaert, D., Van Daele, J., Strobbe, S., Kiekens, F., Storozhenko, S., De Steur, H., et al. (2015). Improving folate (vitamin B9) stability in biofortified rice through metabolic engineering. Nat. Biotechnol. 33, 1076–1078. doi: 10.1038/nbt.3358
Brazier-Hicks, M., and Edwards, R. (2013). Metabolic engineering of the flavone-C-glycoside pathway using polyprotein technology. Metab. Eng. 16, 11–20. doi: 10.1016/j.ymben.2012.11.004
Brazier-Hicks, M., Evans, K. M., Gershater, M. C., Puschmann, H., Steel, P. G., and Edwards, R. (2009). The C-glycosylation of flavonoids in cereals. J. Biol. Chem. 284, 17926–17934. doi: 10.1074/jbc.M109.009258
Britsch, L. (1990). Purification and characterization of flavone synthase I, a 2-oxoglutarate-dependent desaturase. Arch. Biochem. Biophys. 282, 152–160. doi: 10.1016/0003-9861(90)90099-K
Brugliera, F., Tao, G.-Q., Tems, U., Kalc, G., Mouradova, E., Price, K., et al. (2013). Violet/blue chrysanthemums—metabolic engineering of the anthocyanin biosynthetic pathway results in novel petal colors. Plant Cell Physiol. 54, 1696–1710. doi: 10.1093/pcp/pct110
Cai, H., Al-Fayez, M., Tunstall, R. G., Platton, S., Greaves, P., Steward, W. P., et al. (2005). The rice bran constituent tricin potently inhibits cyclooxygenase enzymes and interferes with intestinal carcinogenesis in ApcMin mice. Mol. Cancer Ther. 4, 1287–1292. doi: 10.1158/1535-7163.MCT-05-0165
Cai, H., Hudson, E. A., Mann, P., Verschoyle, R. D., Greaves, P., Manson, M. M., et al. (2004). Growth-inhibitory and cell cycle-arresting properties of the rice bran constituent tricin in human-derived breast cancer cells in vitro and in nude mice in vivo. Br. J. Cancer 91, 1364–1371. doi: 10.1038/sj.bjc.6602124
Cai, H., Sale, S., Schmid, R., Britton, R. G., Brown, K., Steward, W. P., et al. (2009). Flavones as colorectal cancer chemopreventive agents—phenol-O-methylation enhances efficacy. Cancer Prev. Res. 2, 743–750. doi: 10.1158/1940-6207.CAPR-09-0081
Camm, E. L., and Towers, G. H. N. (1973). Phenylalanine ammonia lyase. Phytochemistry 12, 961–973. doi: 10.1016/0031-9422(73)85001-0
Cen, H., Wang, T., Liu, H., Wang, H., Tian, D., Li, X., et al. (2020). Overexpression of MsASMT1 promotes plant growth and decreases flavonoids biosynthesis in transgenic alfalfa (Medicago sativa L.). Front. Plant Sci. 11:489. doi: 10.3389/fpls.2020.00489
Chambers, C. S., Valentova, K., and Kren, V. (2015). “Non-taxifolin” derived flavonolignans: phytochemistry and biology. Curr. Pharm. Des. 21, 5489–5500. doi: 10.2174/1381612821666151002112720
Chen, W., Gao, Y., Xie, W., Gong, L., Lu, K., Wang, W., et al. (2014). Genome-wide association analyses provide genetic and biochemical insights into natural variation in rice metabolism. Nat. Genet. 46, 714–721. doi: 10.1038/ng.3007
Chung, D.-J., Wang, C.-J., Yeh, C.-W., and Tseng, T.-H. (2018). Inhibition of the proliferation and invasion of C6 glioma cells by tricin via the upregulation of focal-adhesion-kinase-targeting micro RNA-7. J. Agric. Food Chem. 66, 6708–6716. doi: 10.1021/acs.jafc.8b00604
Collazo, P., Montoliu, L., Puigdomènech, P., and Rigau, J. (1992). Structure and expression of the lignin O-methyltransferase gene from Zea mays L. Plant Mol. Biol. 20, 857–867. doi: 10.1007/BF00027157
Coomey, J. H., Sibout, R., and Hazen, S. P.(2020). Grass secondary cell walls, Brachypodium distachyon as a model for discovery. New Phytol. 227, 1649–1667. doi: 10.1111/nph.16603
Csupor, D., Csorba, A., and Hohmann, J. (2016). Recent advances in the analysis of flavonolignans of Silybum marianum. J. Pharm. Biomed. Anal. 130, 301–317. doi: 10.1016/j.jpba.2016.05.034
Cummins, I., Brazier-Hicks, M., Stobiecki, M., Fraǹski, R., and Edwards, R. (2006). Selective disruption of wheat secondary metabolism by herbicide safeners. Phytochemistry 67, 1722–1730. doi: 10.1016/j.phytochem.2006.01.012
Daly, P., McClellan, C., Maluk, M., Oakey, H., Lapierre, C., Waugh, R., et al. (2019). RNAi-suppression of barley caffeic acid O-methyltransferase modifies lignin despite redundancy in the gene family. Plant Biotechnol. J. 17, 594–607. doi: 10.1111/pbi.13001
Davin, L. B., and Lewis, N. G. (2003). An historical perspective on lignan biosynthesis: monolignol, allylphenol and hydroxycinnamic acid coupling and downstream metabolism. Phytochem. Rev. 2, 257–288. doi: 10.1023/B:PHYT.0000046175.83729.b5
Deboo, G. B., Albertsen, M. C., and Taylor, L. P. (1995). Flavanone 3-hydroxylase transcripts and flavonol accumulation are temporally coordinate in maize anthers. Plant J. 7, 703–713. doi: 10.1046/j.1365-313X.1995.07050703.x
del Río, J. C., Rencoret, J., Gutiérrez, A., Elder, T., Kim, H., and Ralph, J. (2020). Lignin monomers from beyond the canonical monolignol biosynthetic pathway: another brick in the wall. ACS Sustain. Chem. Eng. 8, 4997–5012. doi: 10.1021/acssuschemeng.0c01109
del Río, J. C., Rencoret, J., Prinsen, P., Martínez, Á. T., Ralph, J., and Gutiérrez, A. (2012). Structural characterization of wheat straw lignin as revealed by analytical pyrolysis, 2D-NMR, and reductive cleavage methods. J. Agric. Food Chem. 60, 5922–5935. doi: 10.1021/jf301002n
Deng, Y., Li, C., Li, H., and Lu, S. (2018). Identification and characterization of flavonoid biosynthetic enzyme genes in salvia miltiorrhiza (Lamiaceae). Molecules 23:1467. doi: 10.3390/molecules23061467
Do, C.-T., Pollet, B., Thévenin, J., Sibout, R., Denoue, D., Barrière, Y., et al. (2007). Both caffeoyl coenzyme A 3-O-methyltransferase 1 and caffeic acid O-methyltransferase 1 are involved in redundant functions for lignin, flavonoids and sinapoyl malate biosynthesis in Arabidopsis. Planta 226, 1117–1129. doi: 10.1007/s00425-007-0558-3
Dong, X., Chen, W., Wang, W., Zhang, H., Liu, X., and Luo, J. (2014). Comprehensive profiling and natural variation of flavonoids in rice. J. Integr. Plant Biol. 56, 876–886. doi: 10.1111/jipb.12204
Du, Y., Chu, H., Chu, I. K., and Lo, C. (2010). CYP93G2 is a flavanone 2-hydroxylase required for C-glycosyl-flavone biosynthesis in rice. Plant Physiol. 154, 324–333. doi: 10.1104/pp.110.161042
Du, H., Ran, F., Dong, H.-L., Wen, J., Li, J.-N., and Liang, Z. (2016). Genome-wide analysis, classification, evolution, and expression analysis of the cytochrome P450 93 family in land plants. PLoS One 11:e0165020. doi: 10.1371/journal.pone.0165020
Duarte-Almeida, J. M., Negri, G., Salatino, A., de Carvalho, J. E., and Lajolo, F. M. (2007). Antiproliferative and antioxidant activities of a tricin acylated glycoside from sugarcane (Saccharum officinarum) juice. Phytochemistry 68, 1165–1171. doi: 10.1016/j.phytochem.2007.01.015
Ehlting, J., Büttner, D., Wang, Q., Douglas, C. J., Somssich, I. E., and Kombrink, E. (1999). Three 4-coumarate: coenzyme A ligases in Arabidopsis thaliana represent two evolutionarily divergent classes in angiosperms. Plant J. 19, 9–20. doi: 10.1046/j.1365-313X.1999.00491.x
Elder, T., del Rio, J. C., Ralph, J., Rencoret, J., Kim, H., Beckham, G. T., et al. (2020). Coupling and reactions of lignols and new lignin monomers: a density functional theory study. ACS Sustain. Chem. Eng. 8, 11033–11045. doi: 10.1021/acssuschemeng.0c02880
Elkind, Y., Edwards, R., Mavandad, M., Hedrick, S. A., Ribak, O., Dixon, R. A., et al. (1990). Abnormal plant development and down-regulation of phenylpropanoid biosynthesis in transgenic tobacco containing a heterologous phenylalanine ammonia-lyase gene. Proc. Natl. Acad. Sci. U. S. A. 87, 9057–9061. doi: 10.1073/pnas.87.22.9057
Eloy, N. B., Voorend, W., Lan, W., Saleme, M. D. L. S., Cesarino, I., Vanholme, R., et al. (2017). Silencing CHALCONE SYNTHASE impedes the incorporation of tricin into lignin and increases lignin content. Plant Physiol. 173, 998–1016. doi: 10.1104/pp.16.01108
Eudes, A., Dutta, T., Deng, K., Jacquet, N., Sinha, A., Benites, V. T., et al. (2017). SbCOMT (Bmr 12) is involved in the biosynthesis of tricin-lignin in sorghum. PLoS One 12:e0178160. doi: 10.1371/journal.pone.0178160
Ferreyra, M. L. F., Emiliani, J., Rodriguez, E. J., Campos-Bermudez, V. A., Grotewold, E., and Casati, P. (2015). The identification of maize and Arabidopsis type I flavone synthases links flavones with hormones and biotic interactions. Plant Physiol. 169, 1090–1107. doi: 10.1104/pp.15.00515
Ferreyra, M. L. F., Rodriguez, E., Casas, M. I., Labadie, G., Grotewold, E., and Casati, P. (2013). Identification of a bifunctional maize C-and O-glucosyltransferase. J. Biol. Chem. 288, 31678–31688. doi: 10.1074/jbc.M113.510040
Fliegmann, J., Furtwängler, K., Malterer, G., Cantarello, C., Schüler, G., Ebel, J., et al. (2010). Flavone synthase II (CYP93B16) from soybean (Glycine max L.). Phytochemistry 71, 508–514. doi: 10.1016/j.phytochem.2010.01.007
Fornalé, S., Rencoret, J., García-Calvo, L., Encina, A., Rigau, J., Gutiérrez, A., et al. (2016). Changes in cell wall polymers and degradability in maize mutants lacking 3'- and 5'-O-methyltransferases involved in lignin biosynthesis. Plant Cell Physiol. 58, 240–255. doi: 10.1093/pcp/pcw198
Galland, M., Boutet-Mercey, S., Lounifi, I., Godin, B., Balzergue, S., Grandjean, O., et al. (2014). Compartmentation and dynamics of flavone metabolism in dry and germinated rice seeds. Plant Cell Physiol. 55, 1646–1659. doi: 10.1093/pcp/pcu095
Gui, J., Shen, J., and Li, L. (2011). Functional characterization of evolutionarily divergent 4-coumarate: coenzyme A ligases in rice. Plant Physiol. 157, 574–586. doi: 10.1104/pp.111.178301
Halpin, C. (2019). Lignin engineering to improve saccharification and digestibility in grasses. Curr. Opin. Biotechnol. 56, 223–229. doi: 10.1016/j.copbio.2019.02.013
Harborne, J. B. (1975). Flavonoid sulphates: a new class of Sulphur compounds in higher plants. Phytochemistry 14, 1147–1155. doi: 10.1016/S0031-9422(00)98585-6
Harborne, J. B., and Hall, E. (1964). Plant polyphenols—XII.: The occurrence of tricin and of glycoflavones in grasses. Phytochemistry 3, 421–428. doi: 10.1016/S0031-9422(00)83627-4
Harborne, J. B., and Williams, C. A. (1976). Flavonoid patterns in leaves of the Gramineae. Biochem. Syst. Ecol. 4, 267–280. doi: 10.1016/0305-1978(76)90051-X
Hong, B.-S., Kim, J.-H., Kim, N.-Y., Kim, B.-G., Chong, Y.-H., and Ahn, J.-H. (2007). Characterization of uridine-diphosphate dependent flavonoid glucosyltransferase from Oryza sativa. BMB Rep. 40, 870–874. doi: 10.5483/BMBRep.2007.40.6.870
Hu, W.-J., Kawaoka, A., Tsai, C.-J., Lung, J., Osakabe, K., Ebinuma, H., et al. (1998). Compartmentalized expression of two structurally and functionally distinct 4-coumarate: CoA ligase genes in aspen (Populus tremuloides). Proc. Natl. Acad. Sci. U. S. A. 95, 5407–5412. doi: 10.1073/pnas.95.9.5407
Hudson, E. A., Dinh, P. A., Kokubun, T., Simmonds, M. S. J., and Gescher, A. (2000). Characterization of potentially chemopreventive phenols in extracts of brown rice that inhibit the growth of human breast and colon cancer cells. Cancer Epidemiol. Biomark. Prev. 9, 1163–1170.
Jiang, N., Doseff, A. I., and Grotewold, E. (2016). Flavones: from biosynthesis to health benefits. Plan. Theory 5:27. doi: 10.3390/plants5020027
Jiang, Y., Ji, X., Duan, L., Ye, P., Yang, J., Zhan, R., et al. (2019). Gene mining and identification of a flavone synthase II involved in flavones biosynthesis by transcriptomic analysis and targeted flavonoid profiling in Chrysanthemum indicum L. Ind. Crop. Prod. 134, 244–256. doi: 10.1016/j.indcrop.2019.04.009
Jiang, B., Song, J., and Jin, Y. (2020). A flavonoid monomer tricin in Gramineous plants: metabolism, bio/chemosynthesis, biological properties, and toxicology. Food Chem. 320:126617. doi: 10.1016/j.foodchem.2020.126617
Jiang, Y. N., Wang, B., Li, H., Yao, L. M., and Wu, T. L. (2010). Flavonoid production is effectively regulated by RNAi interference of two flavone synthase genes from Glycine max. J. Plant Biol. 53, 425–432. doi: 10.1007/s12374-010-9132-9
Johnson, A. A. T., Kyriacou, B., Callahan, D. L., Carruthers, L., Stangoulis, J., Lombi, E., et al. (2011). Constitutive overexpression of the OsNAS gene family reveals single-gene strategies for effective iron-and zinc-biofortification of rice endosperm. PLoS One 6:e24476. doi: 10.1371/journal.pone.0024476
Jung, Y.-S., Kim, D. H., Hwang, J. Y., Yun, N. Y., Lee, Y.-H., Han, S. B., et al. (2014). Anti-inflammatory effect of tricin 4'-O-(threo-β-guaiacylglyceryl) ether, a novel flavonolignan compound isolated from Njavara on in RAW264. 7 cells and in ear mice edema. Toxicol. Appl. Pharmacol. 277, 67–76. doi: 10.1016/j.taap.2014.03.001
Jung, Y.-J., Park, J.-H., Cho, J.-G., Seo, K.-H., Lee, D.-S., Kim, Y.-C., et al. (2015). Lignan and flavonoids from the stems of Zea mays and their anti-inflammatory and neuroprotective activities. Arch. Pharm. Res. 38, 178–185. doi: 10.1007/s12272-014-0387-4
Katsumoto, Y., Fukuchi-Mizutani, M., Fukui, Y., Brugliera, F., Holton, T. A., Karan, M., et al. (2007). Engineering of the rose flavonoid biosynthetic pathway successfully generated blue-hued flowers accumulating delphinidin. Plant Cell Physiol. 48, 1589–1600. doi: 10.1093/pcp/pcm131
Kerscher, F., and Franz, G. (1987). Biosynthesis of vitexin and isovitexin: enzymatic synthesis of the C-glucosylflavones vitexin and isovitexin with an enzyme preparation from Fagopyrum esculentum M. seedlings. Z. Naturforsch. C. J. Biosci. 42, 519–524. doi: 10.1515/znc-1987-0505
Kerscher, F., and Franz, G. (1988). Isolation and some properties of an UDP-glucose: 2-hydroxyflavanone-6 (or 8)-C-glucosyltransferase from Fagopyrum esculentum M. cotyledons. J. Plant Physiol. 132, 110–115. doi: 10.1016/S0176-1617(88)80193-7
Kim, J. H., Cheon, Y. M., Kim, B.-G., and Ahn, J.-H. (2008). Analysis of flavonoids and characterization of the OsFNS gene involved in flavone biosynthesis in rice. J. Plant Biol. 51, 97–101. doi: 10.1007/BF03030717
Kim, B.-G., Lee, Y., Hur, H.-G., Lim, Y., and Ahn, J.-H. (2006). Flavonoid 3'-O-methyltransferase from rice: cDNA cloning, characterization and functional expression. Phytochemistry 67, 387–394. doi: 10.1016/j.phytochem.2005.11.022
Kim, B. G., Yang, S. M., Kim, S. Y., Cha, M. N., and Ahn, J.-H. (2015). Biosynthesis and production of glycosylated flavonoids in Escherichia coli: current state and perspectives. Appl. Microbiol. Biotechnol. 99, 2979–2988. doi: 10.1007/s00253-015-6504-6
Kitada, C., Gong, Z., Tanaka, Y., Yamazaki, M., and Saito, K. (2001). Differential expression of two cytochrome P450s involved in the biosynthesis of flavones and anthocyanins in chemo-varietal forms of Perilla frutescens. Plant Cell Physiol. 42, 1338–1344. doi: 10.1093/pcp/pce169
Ko, J. H., Kim, B.-G., Hur, H.-G., Lim, Y., and Ahn, J.-H. (2006). Molecular cloning, expression and characterization of a glycosyltransferase from rice. Plant Cell Rep. 25, 741–746. doi: 10.1007/s00299-006-0119-4
Ko, J. H., Kim, B.-G., Kim, J. H., Kim, H., Lim, C. E., Lim, J., et al. (2008). Four glucosyltransferases from rice: cDNA cloning, expression, and characterization. J. Plant Physiol. 165, 435–444. doi: 10.1016/j.jplph.2007.01.006
Kong, C.-H., Xu, X.-H., Zhang, M., and Zhang, S.-Z. (2010). Allelochemical tricin in rice hull and its aurone isomer against rice seedling rot disease. Pest Manag. Sci. 66, 1018–1024. doi: 10.1002/ps.1976
Kong, C., Xu, X., Zhou, B., Hu, F., Zhang, C., and Zhang, M. (2004). Two compounds from allelopathic rice accession and their inhibitory activity on weeds and fungal pathogens. Phytochemistry 65, 1123–1128. doi: 10.1016/j.phytochem.2004.02.017
Koshiba, T., Hirose, N., Mukai, M., Yamamura, M., Hattori, T., Suzuki, S., et al. (2013). Characterization of 5-hydroxyconiferaldehyde O-methyltransferase in Oryza sativa. Plant Biotechnol. 30, 157–167. doi: 10.5511/plantbiotechnology.13.0219a
Kumar, S., Stecher, G., Li, M., Knyaz, C., and Tamura, K. (2018). MEGA X: molecular evolutionary genetics analysis across computing platforms. Mol. Biol. Evol. 35, 1547–1549. doi: 10.1093/molbev/msy096
Kuwabara, H., Mouri, K., Otsuka, H., Kasai, R., and Yamasaki, K. (2003). Tricin from a malagasy connaraceous plant with potent antihistaminic activity. J. Nat. Prod. 66, 1273–1275. doi: 10.1021/np030020p
Lam, P. Y., Liu, H., and Lo, C. (2015). Completion of tricin biosynthesis pathway in rice: cytochrome P450 75B4 is a novel chrysoeriol 5'-hydroxylase. Plant Physiol. 175, 1527–1536. doi: 10.1104/pp.15.00566
Lam, P. Y., Lui, A. C. W., Yamamura, M., Wang, L., Takeda, Y., Suzuki, S., et al. (2019a). Recruitment of specific flavonoid B-ring hydroxylases for two independent biosynthesis pathways of flavone-derived metabolites in grasses. New Phytol. 223, 204–219. doi: 10.1111/nph.15795
Lam, P. Y., Tobimatsu, Y., Matsumoto, N., Suzuki, S., Lan, W., Takeda, Y., et al. (2019b). OsCAldOMT1 is a bifunctional O-methyltransferase involved in the biosynthesis of tricin-lignins in rice cell walls. Sci. Rep. 9:11597. doi: 10.1038/s41598-019-47957-0
Lam, P. Y., Tobimatsu, Y., Takeda, Y., Suzuki, S., Yamamura, M., Umezawa, T., et al. (2017). Disrupting flavone synthase II alters lignin and improves biomass digestibility. Plant Physiol. 174, 972–985. doi: 10.1104/pp.16.01973
Lam, P. Y., Zhu, F.-Y., Chan, W. L., Liu, H., and Lo, C. (2014). Cytochrome P450 93G1 is a flavone synthase II that channels flavanones to the biosynthesis of tricin O-linked conjugates in rice. Plant Physiol. 165, 1315–1327. doi: 10.1104/pp.114.239723
Lan, W., Lu, F., Regner, M., Zhu, Y., Rencoret, J., Ralph, S. A., et al. (2015). Tricin, a flavonoid monomer in monocot lignification. Plant Physiol. 167, 1284–1295. doi: 10.1104/pp.114.253757
Lan, W., Morreel, K., Lu, F., Rencoret, J., del Río, J. C., Voorend, W., et al. (2016a). Maize tricin-oligolignol metabolites and their implications for monocot lignification. Plant Physiol. 171, 810–820. doi: 10.1104/pp.16.02012
Lan, W., Rencoret, J., del Río, J. C., and Ralph, J. (2019). “Tricin in grass lignin: biosynthesis, characterization, and quantitation,” in Lignin: Biosynthesis, Functions and Economic Significance. eds. F. Lu and F. Yue (New York: Nova Science Publishers), 51–78.
Lan, W., Rencoret, J., Lu, F., Karlen, S. D., Smith, B. G., Harris, P. J., et al. (2016b). Tricin-lignins: occurrence and quantitation of tricin in relation to phylogeny. Plant J. 88, 1046–1057. doi: 10.1111/tpj.13315
Lee, S.-S., Baek, Y.-S., Eun, C.-S., Yu, M.-H., Baek, N.-I., Chung, D.-K., et al. (2015). Tricin derivatives as anti-inflammatory and anti-allergic constituents from the aerial part of Zizania latifolia. Biosci. Biotechnol. Biochem. 79, 700–706. doi: 10.1080/09168451.2014.997184
Lee, Y. J., Kim, B. G., Chong, Y., Lim, Y., and Ahn, J.-H. (2008a). Cation dependent O-methyltransferases from rice. Planta 227, 641–647. doi: 10.1007/s00425-007-0646-4
Lee, Y. J., Kim, J. H., Kim, B. G., Lim, Y., and Ahn, J.-H. (2008b). Characterization of flavone synthase I from rice. BMB Rep. 41, 68–71. doi: 10.5483/bmbrep.2008.41.1.068
Li, Y., Kim, J. I., Pysh, L., and Chapple, C. (2015). Four isoforms of Arabidopsis 4-coumarate: CoA ligase have overlapping yet distinct roles in phenylpropanoid metabolism. Plant Physiol. 169, 2409–2421. doi: 10.1104/pp.15.00838
Li, H., Li, D., Yang, Z., Zeng, Q., Luo, Y., and He, N. (2020b). Flavones produced by mulberry flavone synthase type I constitute a defense line against the ultraviolet-B stress. Plan. Theory 9:215. doi: 10.3390/plants9020215
Li, D.-D., Ni, R., Wang, P.-P., Zhang, X.-S., Wang, P.-Y., Zhu, T.-T., et al. (2020a). Molecular basis for chemical evolution of flavones to flavonols and anthocyanins in land plants. Plant Physiol. 184, 1731–1743. doi: 10.1104/pp.20.01185
Li, M., Pu, Y., Yoo, C. G., and Ragauskas, A. J. (2016). The occurrence of tricin and its derivatives in plants. Green Chem. 18, 1439–1454. doi: 10.1039/C5GC03062E
Li, K., Wang, D., Gong, L., Lyu, Y., Guo, H., Chen, W., et al. (2019). Comparative analysis of metabolome of rice seeds at three developmental stages using a recombinant inbred line population. Plant J. 100, 908–922. doi: 10.1111/tpj.14482
Li, H., Zhou, C. X., Pan, Y., Gao, X., Wu, X., Bai, H., et al. (2005). Evaluation of antiviral activity of compounds isolated from Ranunculus sieboldii and Ranunculus sceleratus. Planta Med. 71, 1128–1133. doi: 10.1055/s-2005-873169
Lin, F., Yamano, G., Hasegawa, M., Anzai, H., Kawasaki, S., and Kodama, O. (2006). Cloning and functional analysis of caffeic acid 3-O-methyltransferase from rice (Oryza sativa). J. Pestic. Sci. 31, 47–53. doi: 10.1584/jpestics.31.47
Luang, S., Cho, J.-I., Mahong, B., Opassiri, R., Akiyama, T., Phasai, K., et al. (2013). Rice Os9BGlu31 is a transglucosidase with the capacity to equilibrate phenylpropanoid, flavonoid, and phytohormone glycoconjugates. J. Biol. Chem. 288, 10111–10123. doi: 10.1074/jbc.M112.423533
Lui, A. C. W., Lam, P. Y., Chan, K. H., Wang, L., Tobimatsu, Y., and Lo, C. (2020). Convergent recruitment of 5'-hydroxylase activities by CYP75B flavonoid B-ring hydroxylases for tricin biosynthesis in Medicago legumes. New Phytol. 228, 269–284. doi: 10.1111/nph.16498
Ma, Q.-H., and Xu, Y. (2008). Characterization of a caffeic acid 3-O-methyltransferase from wheat and its function in lignin biosynthesis. Biochimie 90, 515–524. doi: 10.1016/j.biochi.2007.09.016
Mao, J.-Z., Zhang, X., Li, M.-F., and Xu, F. (2013). Effect of biological pretreatment with white-rot fungus Trametes hirsuta C7784 on lignin structure in Carex meyeriana Kunth. Bio Resources 8, 3869–3883. doi: 10.15376/biores.8.3.3869-3883
Markham, K. R., Mues, R., Stoll, M., and Zinsmeister, H. D. (1987). NMR spectra of flavone di-C-glycosides from Apometzgeria pubescens and the detection of rotational isomerism in 8-C-hexosylflavones. Z. Naturforsch. C. J. Biosci. 42, 1039–1042. doi: 10.1515/znc-1987-9-1006
Martens, S., and Forkmann, G. (1999). Cloning and expression of flavone synthase II from gerbera hybrids. Plant J. 20, 611–618. doi: 10.1046/j.1365-313X.1999.00636.x
Martens, S., Forkmann, G., Matern, U., and Lukačin, R. (2001). Cloning of parsley flavone synthase I. Phytochemistry 58, 43–46. doi: 10.1016/S0031-9422(01)00191-1
Martens, S., and Mithöfer, A. (2005). Flavones and flavone synthases. Phytochemistry 66, 2399–2407. doi: 10.1016/j.phytochem.2005.07.013
Miyahisa, I., Funa, N., Ohnishi, Y., Martens, S., Moriguchi, T., and Horinouchi, S. (2006). Combinatorial biosynthesis of flavones and flavonols in Escherichia coli. Appl. Microbiol. Biotechnol. 71, 53–58. doi: 10.1007/s00253-005-0116-5
Miyaichi, Y., Segawa, A., and Tomimori, T. (2006). Studies on Nepalese crude drugs. XXIX. Chemical constituents of Dronapuspi, the whole herb of Leucas cephalotes Spreng. Chem. Pharm. Bull. 54, 1370–1379. doi: 10.1248/cpb.54.1370
Morohashi, K., Casas, M. I., Ferreyra, M. L. F., Mejía-Guerra, M. K., Pourcel, L., Yilmaz, A., et al. (2012). A genome-wide regulatory framework identifies maize pericarp color 1 controlled genes. Plant Cell 24, 2745–2764. doi: 10.1105/tpc.112.098004
Murayama, T., Li, Y., Takahashi, T., Yamada, R., Matsubara, K., Tuchida, Y., et al. (2012). Anti-cytomegalovirus effects of tricin are dependent on CXCL11. Microbes Infect. 14, 1086–1092. doi: 10.1016/j.micinf.2012.05.017
Nakatsubo, T., Kitamura, Y., Sakakibara, N., Mizutani, M., Hattori, T., Sakurai, N., et al. (2008). At5g54160 gene encodes Arabidopsis thaliana 5-hydroxyconiferaldehyde O-methyltransferase. J. Wood Sci. 54, 312–317. doi: 10.1007/s10086-008-0958-4
Nakayama, T., Takahashi, S., and Waki, T. (2019). Formation of flavonoid metabolons: functional significance of protein-protein interactions and impact on flavonoid chemodiversity. Front. Plant Sci. 10:821. doi: 10.3389/fpls.2019.00821
Ninomiya, M., Tanaka, K., Tsuchida, Y., Muto, Y., Koketsu, M., and Watanabe, K. (2011). Increased bioavailability of tricin − amino acid derivatives via a prodrug approach. J. Med. Chem. 54, 1529–1536. doi: 10.1021/jm1015457
Noda, N., Aida, R., Kishimoto, S., Ishiguro, K., Fukuchi-Mizutani, M., Tanaka, Y., et al. (2013). Genetic engineering of novel bluer-colored chrysanthemums produced by accumulation of delphinidin-based anthocyanins. Plant Cell Physiol. 54, 1684–1695. doi: 10.1093/pcp/pct111
Ogo, Y., Ozawa, K., Ishimaru, T., Murayama, T., and Takaiwa, F. (2013). Transgenic rice seed synthesizing diverse flavonoids at high levels: a new platform for flavonoid production with associated health benefits. Plant Biotechnol. J. 11, 734–746. doi: 10.1111/pbi.12064
Owens, B. (2018). Golden Rice is safe to eat, says FDA. Nat. Biotechnol. 36, 559–560. doi: 10.1038/nbt0718-559a
Oyama, T., Yasui, Y., Sugie, S., Koketsu, M., Watanabe, K., and Tanaka, T. (2009). Dietary tricin suppresses inflammation-related colon carcinogenesis in male Crj: CD-1 mice. Cancer Prev. Res. 2, 1031–1038. doi: 10.1158/1940-6207.CAPR-09-0061
Paine, J. A., Shipton, C. A., Chaggar, S., Howells, R. M., Kennedy, M. J., Vernon, G., et al. (2005). Improving the nutritional value of Golden Rice through increased pro-vitamin A content. Nat. Biotechnol. 23, 482–487. doi: 10.1038/nbt1082
Paniagua, C., Bilkova, A., Jackson, P., Dabravolski, S., Riber, W., Didi, V., et al. (2017). Dirigent proteins in plants: modulating cell wall metabolism during abiotic and biotic stress exposure. J. Exp. Bot. 68, 3287–3301. doi: 10.1093/jxb/erx141
Park, S., Choi, M. J., Lee, J. Y., Kim, J. K., Ha, S.-H., and Lim, S.-H. (2016). Molecular and biochemical analysis of two rice flavonoid 3'-hydroxylase to evaluate their roles in flavonoid biosynthesis in rice grain. Int. J. Mol. Sci. 17:1549. doi: 10.3390/ijms17091549
Peng, M., Shahzad, R., Gul, A., Subthain, H., Shen, S., Lei, L., et al. (2017). Differentially evolved glucosyltransferases determine natural variation of rice flavone accumulation and UV-tolerance. Nat. Commun. 8:1975. doi: 10.1038/s41467-017-02168-x
Peterson, P. M., and Rieseberg, L. H. (1987). Flavonoids of the annual Muhlenbergia. Biochem. Syst. Ecol. 15, 647–652. doi: 10.1016/0305-1978(87)90038-X
Piquemal, J., Chamayou, S., Nadaud, I., Beckert, M., Barriere, Y., Mila, I., et al. (2002). Down-regulation of caffeic acid O-methyltransferase in maize revisited using a transgenic approach. Plant Physiol. 130, 1675–1685. doi: 10.1104/pp.012237
Poulev, A., Chen, M.-H., Cherravuru, S., Raskin, I., and Belanger, F. C. (2018). Variation in levels of the flavone tricin in bran from rice genotypes varying in pericarp color. J. Cereal Sci. 79, 226–232. doi: 10.1016/j.jcs.2017.11.001
Poulev, A., Heckman, J. R., Raskin, I., and Belanger, F. C. (2019). Tricin levels and expression of flavonoid biosynthetic genes in developing grains of purple and brown pericarp rice. PeerJ 7:e6477. doi: 10.7717/peerj.6477
Qin, J.-C., Zhang, Y.-M., Lang, C.-Y., Yao, Y.-H., Pan, H.-Y., and Li, X. (2012). Cloning and functional characterization of a caffeic acid O-methyltransferase from Trigonella foenum-graecum L. Mol. Biol. Rep. 39, 1601–1608. doi: 10.1007/s11033-011-0899-7
Ragauskas, A. J., Beckham, G. T., Biddy, M. J., Chandra, R., Chen, F., Davis, M. F., et al. (2014). Lignin valorization: improving lignin processing in the biorefinery. Science 344:1246843. doi: 10.1126/science.1246843
Ralph, J. (2020). Tricin and tricin-lignins in Medicago versus in monocots. New Phytol. 228, 11–14. doi: 10.1111/nph.16827
Renders, T., Van den Bossche, G., Vangeel, T., Van Aelst, K., and Sels, B. (2019). Reductive catalytic fractionation: state of the art of the lignin-first biorefinery. Curr. Opin. Biotechnol. 56, 193–201. doi: 10.1016/j.copbio.2018.12.005
Righini, S., Rodriguez, E. J., Berosich, C., Grotewold, E., Casati, P., and Ferreyra, M. L. F. (2019). Apigenin produced by maize flavone synthase I and II protects plants against UV-B-induced damage. Plant Cell Environ. 42, 495–508. doi: 10.1111/pce.13428
Rinaldi, R., Jastrzebski, R., Clough, M. T., Ralph, J., Kennema, M., Bruijnincx, P. C., et al. (2016). Paving the way for lignin valorisation: recent advances in bioengineering, biorefining and catalysis. Angew. Chem. Int. Ed. 55, 8164–8215. doi: 10.1002/anie.201510351
Rosler, J., Krekel, F., Amrhein, N., and Schmid, J. (1997). Maize phenylalanine ammonia-lyase has tyrosine ammonia-lyase activity. Plant Physiol. 113, 175–179. doi: 10.1104/pp.113.1.175
Russell, D. W. (1971). The metabolism of aromatic compounds in higher plants X. properties of the cinnamic acid 4-hydroxylase of pea seedlings and some aspects of its metabolic and developmental control. J. Biol. Chem. 246, 3870–3878. doi: 10.1016/S0021-9258(18)62115-5
Russell, D. W., and Conn, E. E. (1967). The cinnamic acid 4-hydraxylase of pea seedlings. Arch. Biochem. Biophys. 122, 256–258. doi: 10.1016/0003-9861(67)90150-6
Saltzman, A., Birol, E., Bouis, H. E., Boy, E., De Moura, F. F., Islam, Y., et al. (2013). Biofortification: progress toward a more nourishing future. Glob. Food Sec. 2, 9–17. doi: 10.1016/j.gfs.2012.12.003
Sattler, S. E., Palmer, N. A., Saballos, A., Greene, A. M., Xin, Z., Sarath, G., et al. (2012). Identification and characterization of four missense mutations in brown midrib 12 (Bmr 12), the caffeic O-methyltranferase (COMT) of sorghum. Bioenergy Res. 5, 855–865. doi: 10.1007/s12155-012-9197-z
Schijlen, E. G., De Vos, C. R., van Tunen, A. J., and Bovy, A. G. (2004). Modification of flavonoid biosynthesis in crop plants. Phytochemistry 65, 2631–2648. doi: 10.1016/j.phytochem.2004.07.028
Schilmiller, A. L., Stout, J., Weng, J. K., Humphreys, J., Ruegger, M. O., and Chapple, C. (2009). Mutations in the cinnamate 4-hydroxylase gene impact metabolism, growth and development in Arabidopsis. Plant J. 60, 771–782. doi: 10.1111/j.1365-313X.2009.03996.x
Schutyser, W., Renders, A. T., Van den Bosch, S., Koelewijn, S.-F., Beckham, G., and Sels, B. F. (2018). Chemicals from lignin: an interplay of lignocellulose fractionation, depolymerisation, and upgrading. Chem. Soc. Rev. 47, 852–908. doi: 10.1039/C7CS00566K
Seitz, C., Ameres, S., Schlangen, K., Forkmann, G., and Halbwirth, H. (2015). Multiple evolution of flavonoid 3', 5'-hydroxylase. Planta 242, 561–573. doi: 10.1007/s00425-015-2293-5
Seitz, C., Eder, C., Deiml, B., Kellner, S., Martens, S., and Forkmann, G. (2006). Cloning, functional identification and sequence analysis of flavonoid 3'-hydroxylase and flavonoid 3', 5'-hydroxylase cDNAs reveals independent evolution of flavonoid 3', 5'-hydroxylase in the Asteraceae family. Plant Mol. Biol. 61, 365–381. doi: 10.1007/s11103-006-0012-0
Shalini, V., Bhaskar, S., Kumar, K. S., Mohanlal, S., Jayalekshmy, A., and Helen, A. (2012). Molecular mechanisms of anti-inflammatory action of the flavonoid, tricin from Njavara rice (Oryza sativa L.) in human peripheral blood mononuclear cells: possible role in the inflammatory signaling. Int. Immunopharmacol. 14, 32–38. doi: 10.1016/j.intimp.2012.06.005
Shalini, V., Pushpan, C. K., Sindhu, G., Jayalekshmy, A., and Helen, A. (2016). Tricin, flavonoid from Njavara reduces inflammatory responses in hPBMCs by modulating the p38MAPK and PI3K/Akt pathways and prevents inflammation associated endothelial dysfunction in HUVECs. Immunobiology 221, 137–144. doi: 10.1016/j.imbio.2015.09.016
Shi, T., Zhu, A., Jia, J., Hu, X., Chen, J., Liu, W., et al. (2020). Metabolomics analysis and metabolite-agronomic trait associations using kernels of wheat (Triticum aestivum) recombinant inbred lines. Plant J. 103, 279–292. doi: 10.1111/tpj.14727
Shih, C. H., Chu, H., Tang, L. K., Sakamoto, W., Maekawa, M., Chu, I. K., et al. (2008). Functional characterization of key structural genes in rice flavonoid biosynthesis. Planta 228, 1043–1054. doi: 10.1007/s00425-008-0806-1
Singh, S. P., Gruissem, W., and Bhullar, N. K. (2017). Single genetic locus improvement of iron, zinc and β-carotene content in rice grains. Sci. Rep. 7:6883. doi: 10.1038/s41598-017-07198-5
Sun, Z., Fridrich, B., de Santi, A., Elangovan, S., and Barta, K. (2018). Bright side of lignin depolymerization: toward new platform chemicals. Chem. Rev. 118, 614–678. doi: 10.1021/acs.chemrev.7b00588
Sun, H., Li, Y., Feng, S., Zou, W., Guo, K., Fan, C., et al. (2013a). Analysis of five rice 4-coumarate: coenzyme A ligase enzyme activity and stress response for potential roles in lignin and flavonoid biosynthesis in rice. Biochem. Biophys. Res. Commun. 430, 1151–1156. doi: 10.1016/j.bbrc.2012.12.019
Sun, J., Yue, Y.-D., Tang, F., Guo, X.-F., Wang, J., and Yao, X. (2013b). Flavonoids from the leaves of Neosinocalamus affinis. Chem. Nat. Compd. 49, 822–825. doi: 10.1007/s10600-013-0756-0
Tanaka, Y., and Brugliera, F. (2013). Flower colour and cytochromes P450. Philos. Trans. R. Soc. Lond. Ser. B Biol. Sci. 368:20120432. doi: 10.1098/rstb.2012.0432
Tanaka, T., Oyama, T., and Sugie, S. (2019). Dietary tricin suppresses inflammation-related colon carcinogenesis in mice. J. Nutr. Sci. Vitaminol. 65, S100–S103. doi: 10.3177/jnsv.65.S100
Theodor, R., Zinsmeister, H. D., Mues, R., and Markham, K. R. (1981). Flavone C-glycosides of two Metzgeria species. Phytochemistry 20, 1851–1852. doi: 10.1016/0031-9422(81)84019-8
Tobimatsu, Y., and Schuetz, M. (2019). Lignin polymerization: how do plants manage the chemistry so well? Curr. Opin. Biotechnol. 56, 75–81. doi: 10.1016/j.copbio.2018.10.001
Tohge, T., de Souza, L. P., and Fernie, A. R. (2017). Current understanding of the pathways of flavonoid biosynthesis in model and crop plants. J. Exp. Bot. 68, 4013–4028. doi: 10.1093/jxb/erx177
Tohge, T., Yonekura-Sakakibara, K., Niida, R., Watanabe-Takahashi, A., and Saito, K. (2007). Phytochemical genomics in Arabidopsis thaliana: A case study for functional identification of flavonoid biosynthesis genes. Pure Appl. Chem. 79, 811–823. doi: 10.1351/pac200779040811
Tye, Y. Y., Lee, K. T., Abdullah, W. N. W., and Leh, C. P. (2016). The world availability of non-wood lignocellulosic biomass for the production of cellulosic ethanol and potential pretreatments for the enhancement of enzymatic saccharification. Renew. Sust. Energ. Rev. 60, 155–172. doi: 10.1016/j.rser.2016.01.072
Umezawa, T. (2003). Diversity in lignan biosynthesis. Phytochem. Rev. 2, 371–390. doi: 10.1023/B:PHYT.0000045487.02836.32
Umezawa, T. (2018). Lignin modification in planta for valorization. Phytochem. Rev. 17, 1305–1327. doi: 10.1007/s11101-017-9545-x
Umezawa, T., Tobimatsu, Y., Yamamura, M., Miyamoto, T., Takeda, Y., Koshiba, T., et al. (2020). Lignin metabolic engineering in grasses for primary lignin valorization. Lignin 1, 30–41. doi: 10.2524/jtappij.74.1067
Verschoyle, R. D., Greaves, P., Cai, H., Borkhardt, A., Broggini, M., D’Incalci, M., et al. (2006). Preliminary safety evaluation of the putative cancer chemopreventive agent tricin, a naturally occurring flavone. Cancer Chemother. Pharmacol. 57, 1–6. doi: 10.1007/s00280-005-0039-y
Wallace, J. W., and Grisebach, H. (1973). The in vivo incorporation of a flavanone into C-glycosylflavones. Biochim. Biophys. Acta Gen. Subj. 304, 837–841. doi: 10.1016/0304-4165(73)90230-4
Wallace, J., Mabry, T., and Alston, R. (1969). On the biogenesis of flavone O-glycosides and C-glycosides in the Lemnaceae. Phytochemistry 8, 93–99. doi: 10.1016/S0031-9422(00)85800-8
Wang, L., Lam, P. Y., Lui, A. C. W., Zhu, F.-Y., Chen, M.-X., Liu, H., et al. (2020a). Flavonoids are indispensable for complete male fertility in rice. J. Exp. Bot. 71, 4715–4728. doi: 10.1093/jxb/eraa204
Wang, L., Lui, A. C. W., Lam, P. Y., Liu, G., Godwin, I. D., and Lo, C. (2020b). Transgenic expression of flavanone 3-hydroxylase redirects flavonoid biosynthesis and alleviates anthracnose susceptibility in sorghum. Plant Biotechnol. J. 18, 2170–2172. doi: 10.1111/pbi.13397
Weng, J.-K., Akiyama, T., Bonawitz, N. D., Li, X., Ralph, J., and Chapple, C. (2010). Convergent evolution of syringyl lignin biosynthesis via distinct pathways in the lycophyte Selaginella and flowering plants. Plant Cell 22, 1033–1045. doi: 10.1105/tpc.109.073528
Weng, J.-K., Li, X., Stout, J., and Chapple, C. (2008). Independent origins of syringyl lignin in vascular plants. Proc. Natl. Acad. Sci. U. S. A. 105, 7887–7892. doi: 10.1073/pnas.0801696105
Wenzig, E., Kunert, O., Ferreira, D., Schmid, M., Schühly, W., Bauer, R., et al. (2005). Flavonolignans from Avena sativa. J. Nat. Prod. 68, 289–292. doi: 10.1021/np049636k
Wirth, J., Poletti, S., Aeschlimann, B., Yakandawala, N., Drosse, B., Osorio, S., et al. (2009). Rice endosperm iron biofortification by targeted and synergistic action of nicotianamine synthase and ferritin. Plant Biotechnol. J. 7, 631–644. doi: 10.1111/j.1467-7652.2009.00430.x
Wollenweber, E., and Dörr, M. (2008). Occurrence and distribution of the flavone tricetin and its methyl derivatives as free aglycones. Nat. Prod. Commun. 3:300812. doi: 10.1177/1934578X0800300812
Wu, S., and Tian, L. (2019). A new flavone glucoside together with known ellagitannins and flavones with anti-diabetic and anti-obesity activities from the flowers of pomegranate (Punica granatum). Nat. Prod. Res. 33, 252–257. doi: 10.1080/14786419.2018.1446009
Wu, J., Wang, X.-C., Liu, Y., Du, H., Shu, Q.-Y., Su, S., et al. (2016). Flavone Synthases from Lonicera japonica and L. macranthoides reveal differential flavone accumulation. Sci. Rep. 6:19245. doi: 10.1038/srep19245
Xiong, L., Zhu, C., Li, Y., Tian, Y., Lin, S., Yuan, S., et al. (2011). Lignans and neolignans from Sinocalamus affinis and their absolute configurations. J. Nat. Prod. 74, 1188–1200. doi: 10.1021/np200117y
Yang, B., Liu, H., Yang, J., Gupta, V. K., and Jiang, Y. (2018). New insights on bioactivities and biosynthesis of flavonoid glycosides. Trends Food Sci. Technol. 79, 116–124. doi: 10.1016/j.tifs.2018.07.006
Yazawa, K., Kurokawa, M., Obuchi, M., Li, Y., Yamada, R., Sadanari, H., et al. (2011). Anti-influenza virus activity of tricin, 4',5, 7-trihydroxy-3',5'-dimethoxyflavone. Antivir. Chem. Chemother. 22, 1–11. doi: 10.3851/IMP1782
Yazawa, K., Tsuchida, Y., Yamada, R., Sadanari, H., and Murayama, T. (2010). Anti-influenza virus activity by tricin, isolated from Sasa albo-marginata in Japan. Antivir. Res. 86:A49. doi: 10.1016/j.antiviral.2010.02.418
Ye, X., Al-Babili, S., Klöti, A., Zhang, J., Lucca, P., Beyer, P., et al. (2000). Engineering the provitamin A (β-carotene) biosynthetic pathway into (carotenoid-free) rice endosperm. Science 287, 303–305. doi: 10.1126/science.287.5451.303
Yonekura-Sakakibara, K., and Hanada, K. (2011). An evolutionary view of functional diversity in family 1 glycosyltransferases. Plant J. 66, 182–193. doi: 10.1111/j.1365-313X.2011.04493.x
Yonekura-Sakakibara, K., Higashi, Y., and Nakabayashi, R. (2019). The origin and evolution of plant flavonoid metabolism. Front. Plant Sci. 10:943. doi: 10.3389/fpls.2019.00943
Yonekura-Sakakibara, K., Yamamura, M., Matsuda, F., Ono, E., Nakabayashi, R., Sugawara, S., et al. (2021). Seed-coat protective neolignans are produced by the dirigent protein AtDP1 and the laccase AtLAC5 in Arabidopsis. Plant Cell 33, 129–152. doi: 10.1093/plcell/koaa014
You, T.-T., Mao, J.-Z., Yuan, T.-Q., Wen, J.-L., and Xu, F. (2013). Structural elucidation of the lignins from stems and foliage of Arundo donax Linn. J. Agric. Food Chem. 61, 5361–5370. doi: 10.1021/jf401277v
Yue, G. G.-L., Gao, S., Lee, J. K.-M., Chan, Y.-Y., Wong, E. C.-W., Zheng, T., et al. (2020). A natural flavone tricin from grains can alleviate tumor growth and lung metastasis in colorectal tumor mice. Molecules 25:3730. doi: 10.3390/molecules25163730
Yun, C.-S., Yamamoto, T., Nozawa, A., and Tozawa, Y. (2008). Expression of parsley flavone synthase I establishes the flavone biosynthetic pathway in Arabidopsis thaliana. Biosci. Biotechnol. Biochem. 72, 968–973. doi: 10.1271/bbb.70692
Zeng, Y., Pu, X., Yang, J., Du, J., Yang, X., Li, X., et al. (2018). Preventive and therapeutic role of functional ingredients of barley grass for chronic diseases in human beings. Oxidative Med. Cell. Longev. 2018:3232080. doi: 10.1155/2018/3232080
Zhang, J., Subramanian, S., Zhang, Y., and Yu, O. (2007). Flavone synthases from Medicago truncatula are flavanone-2-hydroxylases and are important for nodulation. Plant Physiol. 144, 741–751. doi: 10.1104/pp.106.095018
Zhao, Q., Zhang, Y., Wang, G., Hill, L., Weng, J.-K., Chen, X.-Y., et al. (2016). A specialized flavone biosynthetic pathway has evolved in the medicinal plant, Scutellaria baicalensis. Sci. Adv. 2:e1501780. doi: 10.1126/sciadv.1501780
Zhou, J.-M., Fukushi, Y., Wang, X.-F., and Ibrahim, R. K. (2006). Characterization of a novel flavone O-methyltransferase gene in rice. Nat. Prod. Commun. 1, 981–984. doi: 10.1080/13880200701729745
Zhou, J.-M., Fukushi, Y., Wollenweber, E., and Ibrahim, R. K. (2008). Characterization of two O-methyltransferase-like genes in barley and maize. Pharm. Biol. 46, 26–34. doi: 10.1080/13880200701729745
Zhou, J.-M., and Ibrahim, R. K. (2010). Tricin—a potential multifunctional nutraceutical. Phytochem. Rev. 9, 413–424. doi: 10.1007/s11101-009-9161-5
Zhou, J.-M., Seo, Y. W., and Ibrahim, R. K. (2009). Biochemical characterization of a putative wheat caffeic acid O-methyltransferase. Plant Physiol. Biochem. 47, 322–326. doi: 10.1016/j.plaphy.2008.11.011
Zhu, Q., Yu, S., Zeng, D., Liu, H., Wang, H., Yang, Z., et al. (2017). Development of “purple endosperm rice” by engineering anthocyanin biosynthesis in the endosperm with a high-efficiency transgene stacking system. Mol. Plant 10, 918–929. doi: 10.1016/j.molp.2017.05.008
Keywords: tricin, biosynthetic pathways, flavonoids, lignin, bioengineering, biorefinery
Citation: Lam PY, Lui ACW, Wang L, Liu H, Umezawa T, Tobimatsu Y and Lo C (2021) Tricin Biosynthesis and Bioengineering. Front. Plant Sci. 12:733198. doi: 10.3389/fpls.2021.733198
Received: 30 June 2021; Accepted: 28 July 2021;
Published: 26 August 2021.
Edited by:
Igor Cesarino, University of São Paulo, BrazilReviewed by:
Clint Chapple, Purdue University, United StatesCopyright © 2021 Lam, Lui, Wang, Liu, Umezawa, Tobimatsu and Lo. This is an open-access article distributed under the terms of the Creative Commons Attribution License (CC BY). The use, distribution or reproduction in other forums is permitted, provided the original author(s) and the copyright owner(s) are credited and that the original publication in this journal is cited, in accordance with accepted academic practice. No use, distribution or reproduction is permitted which does not comply with these terms.
*Correspondence: Clive Lo, Y2xpdmVsb0Boa3UuaGs=
Disclaimer: All claims expressed in this article are solely those of the authors and do not necessarily represent those of their affiliated organizations, or those of the publisher, the editors and the reviewers. Any product that may be evaluated in this article or claim that may be made by its manufacturer is not guaranteed or endorsed by the publisher.
Research integrity at Frontiers
Learn more about the work of our research integrity team to safeguard the quality of each article we publish.