- 1Realizing Increased Photosynthetic Efficiency (RIPE), The Australian National University, Canberra, ACT, Australia
- 2Australian Research Council Centre of Excellence for Translational Photosynthesis, Research School of Biology, The Australian National University, Canberra, ACT, Australia
Heterologous synthesis of a biophysical CO2-concentrating mechanism (CCM) in plant chloroplasts offers significant potential to improve the photosynthetic efficiency of C3 plants and could translate into substantial increases in crop yield. In organisms utilizing a biophysical CCM, this mechanism efficiently surrounds a high turnover rate Rubisco with elevated CO2 concentrations to maximize carboxylation rates. A critical feature of both native biophysical CCMs and one engineered into a C3 plant chloroplast is functional bicarbonate (HCO3−) transporters and vectorial CO2-to-HCO3− converters. Engineering strategies aim to locate these transporters and conversion systems to the C3 chloroplast, enabling elevation of HCO3− concentrations within the chloroplast stroma. Several CCM components have been identified in proteobacteria, cyanobacteria, and microalgae as likely candidates for this approach, yet their successful functional expression in C3 plant chloroplasts remains elusive. Here, we discuss the challenges in expressing and regulating functional HCO3− transporter, and CO2-to-HCO3− converter candidates in chloroplast membranes as an essential step in engineering a biophysical CCM within plant chloroplasts. We highlight the broad technical and physiological concerns which must be considered in proposed engineering strategies, and present our current status of both knowledge and knowledge-gaps which will affect successful engineering outcomes.
Introduction
Crop improvement technologies utilizing synthetic biology approaches have been central to a number of recent advances in photosynthetic output (e.g., Kromdijk et al., 2016; Salesse-Smith et al., 2018; Ermakova et al., 2019; South et al., 2019; Batista-Silva et al., 2020; López-Calcagno et al., 2020). These ambitious aims come at an unprecedented time in human history when agricultural productivity must be rapidly boosted in order to feed future populations (Kromdijk and Long, 2016). In a 2008 review, we discussed the potential of utilizing components of the CO2-concentrating mechanism (CCM) of cyanobacteria as a means to improve crop photosynthetic CO2 fixation (Price et al., 2008), with potential to raise rates of carboxylation at ribulose-1,5-bisphosphate (RuBP) carboxylase/oxygenase (Rubisco) while improving nitrogen and water-use efficiencies (Price et al., 2011a; McGrath and Long, 2014; Rae et al., 2017). In the intervening years great steps forward have been made to address this challenge, yet many uncertainties remain on the path to generating a functional chloroplastic CCM.
The CCMs of proteobacteria, cyanobacteria, and microalgae are comprised of bicarbonate (HCO3−) transporters and vectorial CO2-to-HCO3− conversion complexes which, in concert, accumulate a high concentration of HCO3− in prokaryotic cells and microalgal chloroplasts (Figure 1; Kaplan et al., 1980; Badger and Price, 2003; Moroney and Ynalvez, 2007). As a charged species of inorganic carbon (Ci), HCO3− is not freely diffusible through cell membranes (Tolleter et al., 2017), and allows for the generation of an elevated cellular or stromal HCO3− pool compared with the external environment (Price and Badger, 1989a). The second chief component of these CCMs are specialized Rubisco compartments called carboxysomes (Rae et al., 2013) and pyrenoids (Figure 1; Moroney and Ynalvez, 2007; Mackinder, 2018; Hennacy and Jonikas, 2020) where co-localized carbonic anhydrase (CA) enzymes dehydrate HCO3− into CO2, providing high concentrations of CO2 as substrate for RuBP carboxylation.
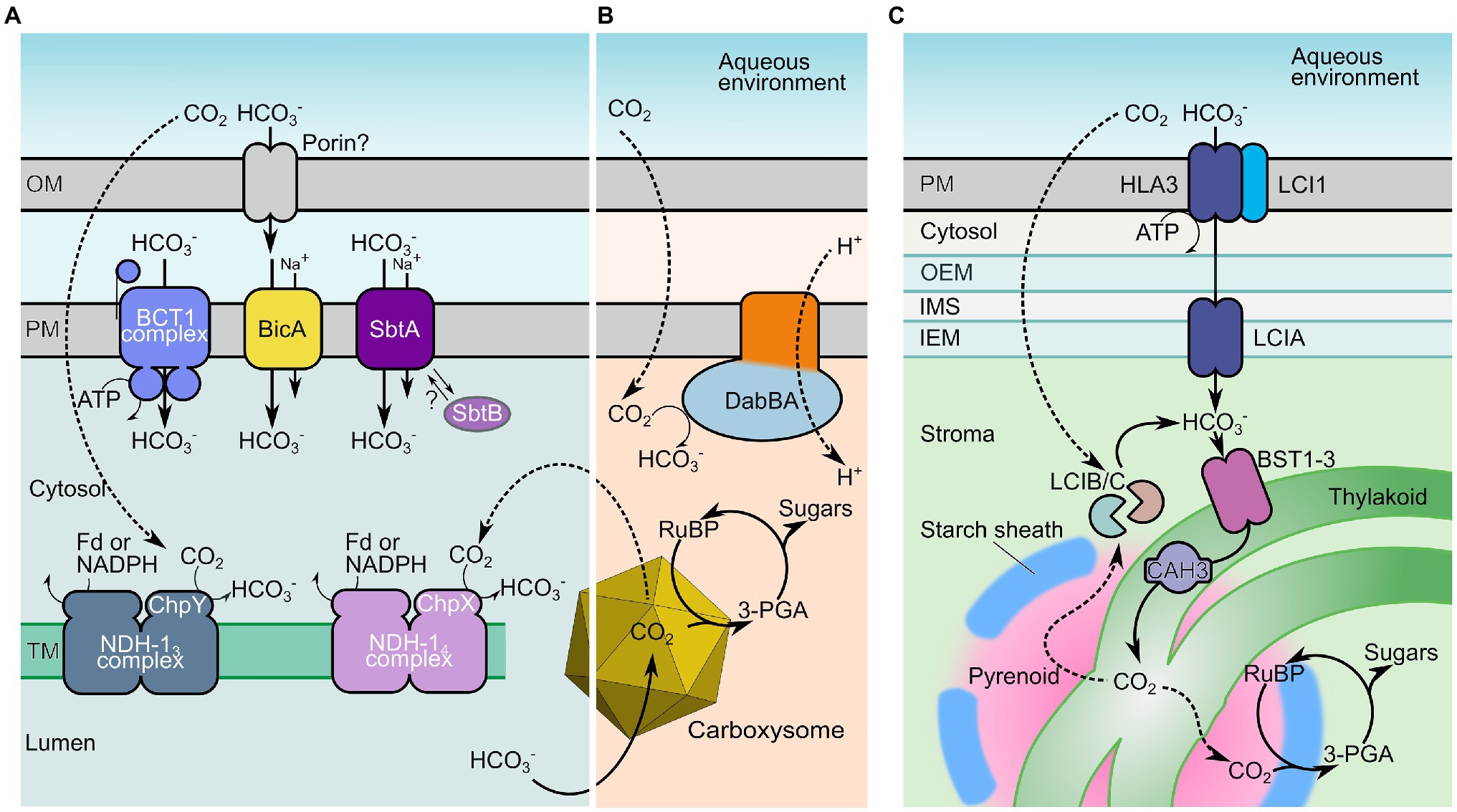
Figure 1. Inorganic carbon uptake components of cyanobacterial, proteobacterial, and microalgal CO2-concentrating mechanisms (CCMs). Key inorganic carbon transport mechanisms of cyanobacteria (A), proteobacteria (B), and microalgae (C) that facilitate elevated cytoplasmic and stromal HCO3− concentrations. The HCO3− pool is utilized to generate localized high concentrations of CO2 in specialized Rubisco-containing compartments knowns as carboxysomes (A,B) or pyrenoids (C), supporting high carboxylation rates. In cyanobacteria (A), HCO3− is potentially supplied to the periplasmic space via an outer-membrane (OM) porin, and is directly transferred across the plasma membrane (PM) by the single-protein Na+-dependent transporters bicarbonate transporter A (BicA) and SbtA, or by the ATP-driven complex BCT1. In addition, cytosolic CO2, acquired by either diffusion, leakage from the carboxysome or spontaneous dehydration of HCO3−, is converted to HCO3− by the energy-coupled, vectorial CO2 pumps NHD-13 and NHD-14 in the thylakoid membranes (TM). In proteobacteria (B), DabBA plays a similar role, taking advantage of relatively high rates of CO2 influx from a low-pH external environment to vectorially generate a cytoplasmic HCO3− pool (Desmarais et al., 2019). In microalgae (C), HCO3− is accumulated via a series of transporters located on the PM (LCI1 and presumably ATP-driven HLA3), the chloroplast inner envelope membrane [inner-envelope membrane (IEM); LCIA] and the TM (bestrophins, BST1-3). Thylakoids traverse the Rubisco-containing pyrenoid where the thylakoid lumen-localized carbonic anhydrase (CA) CAH3 is thought to convert HCO3− supplied to the thylakoid lumen to CO2. Analogous to the cyanobacterial system, the LCIB/C complex constitutes a putative, vectorial CA that may recycle any CO2 arising in the chloroplast stroma back to HCO3−. Fd, ferredoxin; RuBP, ribulose-1,5-bisphosphate; 3-PGA, 3-phosphoglycerate; SbtB, SbtA regulator protein; and ChpX/ChpY, NDH-1 complex vectorial CO2-HCO3− domains. Individual transporter proteins are as listed in Table 1.
Collectively, these systems are often termed biophysical CCMs since their function utilizes the active movement of Ci across cellular compartments to release it as CO2 around Rubisco (Giordano et al., 2005). This is distinct from biochemical CCMs found in C4 and CAM plants, which generally utilize HCO3− for the carboxylation of phosphoenolpyruvate into transportable organic acids, prior to spatial or temporal CO2 re-release and carboxylation by Rubisco.
Modeling has shown that the installation of biophysical CCM HCO3− transporters in the inner-envelope membrane (IEM) of C3 chloroplasts is sufficient to elevate the chloroplastic Ci, leading to a net improvement in CO2 available for Rubisco carboxylation and therefore net carbon gain (Price et al., 2011a; McGrath and Long, 2014). This initial step in the conversion of crop plant chloroplasts to a sub-cellular CCM not only provides potential yield gains but is also necessary to generate the required stromal [HCO3−] needed for carboxysome or pyrenoid function in the engineering trajectory toward a complete chloroplastic CCM (Price et al., 2013). Therefore, the successful engineering of HCO3− accumulation in C3 stroma is a critical step in this process.
While the idea to generate a C3 chloroplastic CCM has been considered for some time (Price et al., 2008), the pace of progress in this field highlights a myriad of conceptual and technical challenges associated with achieving such a complex goal. Progress toward the construction of carboxysomes and pyrenoids in C3 chloroplasts has been made (Lin et al., 2014a; Long et al., 2018; Atkinson et al., 2020), and the transfer of a complete and functional CCM from proteobacteria into Rubisco-dependent Escherichia coli (Flamholz et al., 2020) indicates theoretical potential for successful transfer of CCMs to plants. However, hurdles remain in both understanding and constructing CCM components within eukaryotic organelles where system complexity confounds an already difficult engineering task. This is exemplified by reports of the successful expression of HCO3− transporters into C3 chloroplasts, but their lack of function and/or incorrect targeting (Pengelly et al., 2014; Atkinson et al., 2016; Rolland et al., 2016; Uehara et al., 2016, 2020), or lack of functional characterization in planta (Nölke et al., 2019), highlights the need to further understand the composite interactions of chloroplast protein targeting, membrane energization, and small molecule passage across the chloroplast envelope.
Herein, we discuss some of the known complexities associated with the engineering task of generating functional HCO3− transport systems in C3 chloroplasts and highlight unknown details, which require ongoing research focus to enable a clearer path to successful elevation of chloroplastic HCO3− for increased carboxylation efficiency in crop plants.
Can Hco3− Concentrations Be Elevated In A C3 Chloroplast?
The terrestrial nature of C3 plants and their appearance in geological history during a period of relatively high atmospheric CO2 (Flamholz and Shih, 2020) is a possible contributor to the absence of biophysical CCMs from higher plant chloroplasts (Raven et al., 2017). The efficiency of Rubisco carboxylation is hampered by O2, leading to photorespiratory expenditure of accumulated CO2 and chemical energy (Busch, 2020). It is assumed that factors selecting for maintenance of relatively high rates of carboxylation, as atmospheric concentrations of CO2 decreased while O2 increased approximately 350 million years ago, may have led to a divergence in mechanistic adaptations between aquatic and terrestrial photosynthetic organisms (Flamholz and Shih, 2020; Long et al., 2021). Thus, cyanobacteria and many eukaryotic algae evolved CCMs to overcome these challenges, while emerging terrestrial C3 plants have maintained a larger investment in Rubisco and evolved to maximize beneficial biochemical contributions from photorespiratory nitrogen and sulfur metabolism (Shi and Bloom, 2021). As a result, terrestrial C3 plant lineages have not evolved with a capability to elevate chloroplastic Ci concentrations like many of their aquatic counterparts. Indeed, there is good argument that biochemical CCM evolution (e.g., C4 photosynthesis) would be favored in terrestrial systems over strategies which accumulate HCO3− (Flamholz and Shih, 2020). While horizontal gene transfer may have been involved in the evolution of C4 photosynthesis (Wickell and Li, 2020), there has presumably been very little opportunity or evolutionary pressure for plants to acquire genes from aquatic biophysical CCMs in order to evolve alternative CO2 fixation strategies. In addition, the slower diffusion of Ci species in aquatic environments compared with plant tissue may confine evolutionary trajectories (Raven et al., 2017; Flamholz and Shih, 2020). This underscores the fact that the C3 chloroplast has evolved in a gaseous atmosphere and with alternative solutions to Rubisco promiscuity to its aquatic cousins, highlighting that the concept of an engineered chloroplastic CCM is one in which considerable evolutionary complexity must be considered.
When considering any engineering design for enhanced HCO3− uptake into C3 chloroplasts, a reasonable question to ask is whether HCO3− can be elevated in this organelle, and if so, how? There is sufficient HCO3− in the mesophyll cytoplasm available for transport into chloroplasts (at least 250μM; Evans and von Caemmerer, 1996). However, a CCM engineering strategy must ensure HCO3− can gain passage across both the outer-envelope membrane (OEM) and IEM of the chloroplast. Given that C3 chloroplasts typically access Ci from the external environment (primarily as the more membrane-permeable CO2); chloroplast membranes appear not to have specific HCO3− transport mechanisms (Rolland et al., 2012). Nonetheless, a number of oxyanions, such as phosphate, nitrate, and sulfate evidently do diffuse through the OEM (Bölter et al., 1999). Notably, simple diffusion of CO2 through leaf tissue is insufficient to support the supply rates needed for observed rates of CO2 assimilation by plants (Morison et al., 2005), and it is likely that CO2 entry into the chloroplast is also facilitated by CO2-permeable aquaporins (Flexas et al., 2006; Evans et al., 2009; Tolleter et al., 2017; Ermakova et al., 2021) and CA-driven distribution of Ci between predominant species (HCO3− and CO2; Price et al., 1994). Therefore, the facilitated entry of Ci into C3 chloroplasts is conceptually not counter to contemporary chloroplast function, and on face value would appear beneficial.
In general, solute transport across the chloroplast OEM is considered to be relatively unhindered due to the presence of low-selectivity and large-molecule channel proteins present in this membrane (Bölter et al., 1999; Hemmler et al., 2006; Duy et al., 2007). It is expected that anion passage into the inter-membrane space (IMS), and presumably that of HCO3−, occurs via at least one of the outer envelope protein channels (OEPs; Duy et al., 2007), with OEP21 a potential route for broad anion uptake into the IMS (Figure 2; Hemmler et al., 2006). While inward passage through this specific channel may be hampered by triose-phosphate export in the light (Duy et al., 2007), it and other OEPs offer broad selective import into the IMS. Currently, there is no reason to expect that HCO3− cannot access the IMS. Nonetheless, it is worthy of consideration, and additional transport mechanisms or solutions should be considered for the elevation of IMS [HCO3−] if this becomes a roadblock to the overall strategy. Notably, insertion of an IMS-specific CA would likely generate the requisite HCO3− from diffusion of CO2 in this location (depending on the IMS pH) for utilization by an IEM-localized pump, in the unlikely scenario that insufficient HCO3− is present here. The ΔpH across the chloroplast IEM has been measured to be up to 1 pH unit (Demmig and Gimmler, 1983) suggesting that an IMS pH of 7–7.5 is feasible in the light, ensuring that >80% of Ci species would exist as HCO3− in the presence of CA.
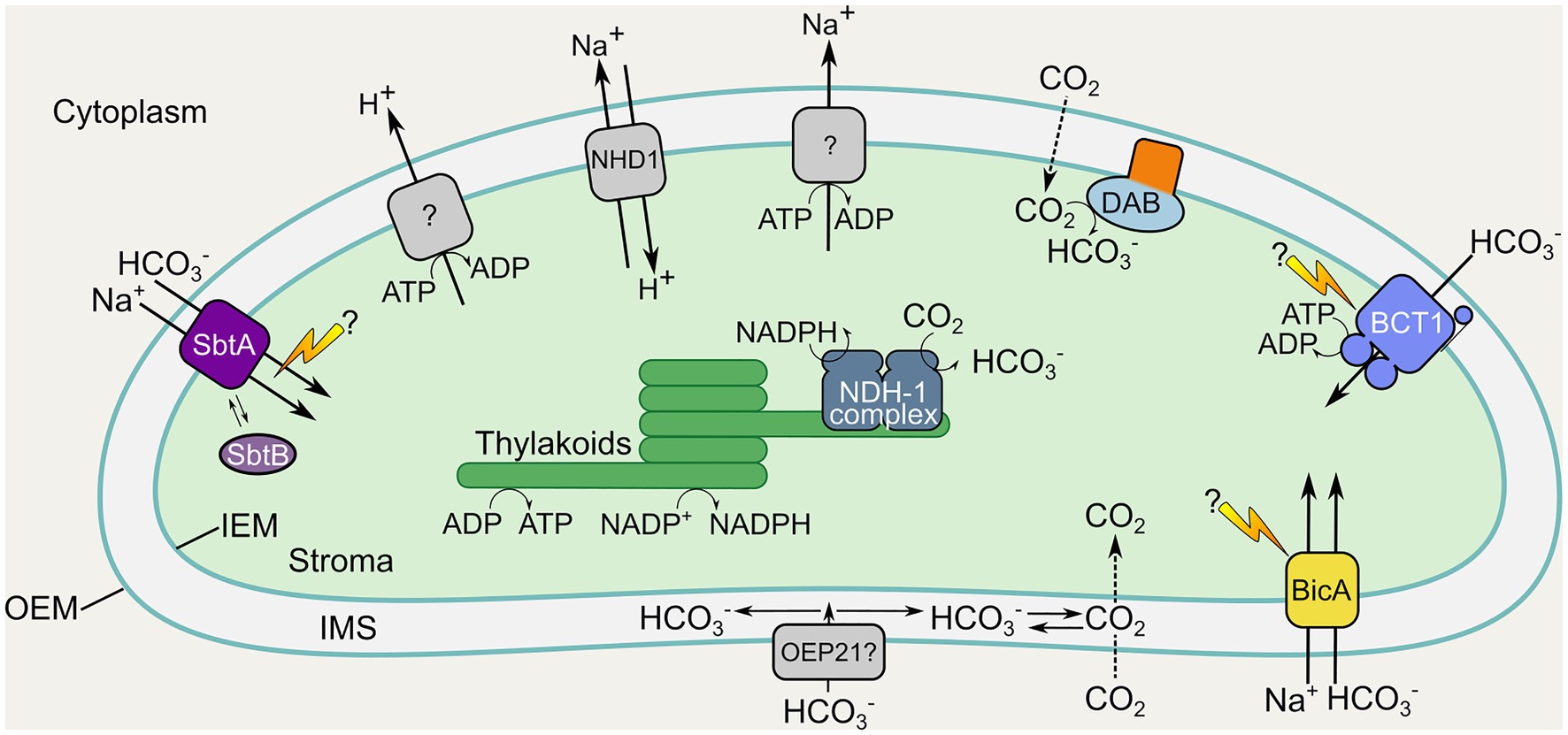
Figure 2. Location and function of inorganic carbon uptake systems in a proposed chloroplastic CCM. Inorganic carbon uptake components from cyanobacteria and proteobacteria proposed for engineering into the chloroplast IEM and thylakoids of terrestrial C3 plants. Membrane transporters and vectorial CO2-HCO3− conversion complexes from cyanobacteria and proteobacteria are shown in color (see Figure 1; Table 1). Additional native chloroplastic systems which may support the required energetics and facilitation of active HCO3− uptake (including Na+ and H+ extrusion systems and the NHD1 Na+/H+ antiporter) are shown in gray. The cyanobacterial HCO3− transporters BicA and SbtA, as well as the ATP-driven BCT1 complex are targeted to the IEM may require unknown activation processes, indicated by lightning bolts. Note the added complexity of the multi-subunit BCT1 complex requiring protein components in the inter-membrane space (IMS), IEM, and stroma. The cyanobacterial vectorial NDH-1 complex has been suggested on the thylakoid membrane as it likely requires energization by components of the photosynthetic electron transport chain (ETC). The proteobacterial vectorial DAB complex is tentatively depicted on the IEM, but, alternatively, co-localization with the ETC on the thylakoid membrane may be favorable for its energization. The broad-specificity channel protein OEP21 on the outer-envelope membrane (OEM) is depicted as a putative access point for cytoplasmic HCO3− uptake into the IMS. Individual transporter proteins are as listed in Table 1.
Assuming sufficient HCO3− is available in the IMS from the cytosolic pool, its transport across the IEM into the chloroplast stroma is predicted to be feasible using either high affinity, low flux transporters [e.g., the cyanobacterial sodium-dependent bicarbonate transporter, SbtA, and the ATP driven bicarbonate transporter, BCT1; Table 1; Figures 1, 2], or low to medium affinity, high flux transporters (e.g., BicA; Table 1; Figures 1, 2). For the most part, the affinity of these HCO3− transporter types falls below the proposed cytosolic [HCO3−] (Table 1), suggesting that sufficient transport is feasible. Either independently, or in concert, modeling suggests that functional forms of these transporter types should provide net import into the stroma and enable increased CO2 supply to Rubisco (Price et al., 2011a).
Once HCO3− concentrations in the chloroplast are elevated, it is acknowledged that stromal CA is likely to prevent the desired function of a complete chloroplastic CCM, since its action in converting HCO3− to CO2 transforms the Ci pool from one with low membrane permeability to one which can rapidly diffuse away from the site of fixation (Price et al., 2013; McGrath and Long, 2014). This would rob an engineered carboxysome (housing a Rubisco with relatively high KMCO2 ) of its primary Ci substrate, and ectopic CA is known to lead to a high-CO2-requiring phenotype in cyanobacteria (Price and Badger, 1989a). However, in the development of a simpler CCM with only functional HCO3− uptake, stromal CA would provide the rapid, pH-driven development of CO2 needed in the chloroplast to supply additional CO2 to Rubisco. The net effect of such a system is the modest elevation of chloroplastic Ci, which leads to enhanced CO2 availability at Rubisco (Price et al., 2011a; McGrath and Long, 2014).
It is relevant to consider what effects elevated stromal HCO3− might have on chloroplast function beyond the capability of supplying increased CO2 to Rubisco. A role for HCO3− as a proton acceptor during water oxidation has been proposed in photosystem II (PSII) function, with HCO3− providing stabilizing and protective effects (Shevela et al., 2012). CO2 formation from HCO3− at PSII occurs at a rate that correlates with O2 evolution at the donor side (somewhat slower at the acceptor side; Shevela et al., 2020). A simplistic viewpoint therefore is that greater quantities of stromal HCO3− may support PSII function rather than having any negative effects, as appears to be the case for cyanobacteria and microalgae. This PSII property highlights potential conversion of HCO3− to CO2 in an engineered chloroplastic CCM, however, and longer-term goals would be to generate systems which recycle stromal CO2 back to HCO3−, whether it is generated through PSII action, anaplerotic reactions, or indeed leakage from an engineered carboxysome or pyrenoid (Price et al., 2013). Nonetheless, CO2 losses via these processes are likely to be minimal within an engineering scheme utilizing a HCO3− transporter and a carboxysome, benefiting only marginally from the addition of vectorial CO2-to-HCO3− conversion complexes (McGrath and Long, 2014).
Which Ci Uptake Systems Could Facilitate Chloroplastic Hco3− Accumulation?
Inorganic carbon acquisition is an essential step in driving a biophysical CCM and for maximizing its efficiency. Acquisition of the predominant Ci species (CO2 and HCO3−) contributes to the accumulation of an intracellular/chloroplastic HCO3− pool well above external Ci levels, with up to 1,000-fold increases observed in cyanobacteria (Price, 2011). This can only be achieved by active Ci uptake against a concentration gradient, requiring energy, as opposed to passive diffusional uptake through protein channels such as CO2 aquaporins (Uehlein et al., 2012; Li et al., 2015). Active Ci uptake systems can be divided into two categories, energy-coupled CAs (also known as vectorial CO2 pumps or CO2-to-HCO3− conversion systems) and active HCO3− transporters. A number of Ci transport systems have been identified through genetic screens of high CO2 requiring mutants in the microalga Chlamydomonas (Spalding, 2008; Fang et al., 2012), several cyanobacteria (Price and Badger, 1989b; Badger and Price, 2003; Price et al., 2008) and, recently non-photosynthetic, CO2-fixing γ-proteobacteria (Scott et al., 2018; Desmarais et al., 2019), summarized in Table 1 and Sui et al. (2020).
Cyanobacterial Ci Uptake Systems
In cyanobacteria, five Ci uptake systems have been verified, subsets of which are present in all species (Figure 1A; Table 1). These transport systems differ in subcellular localization, substrate affinity, flux rates, energization and regulation of gene expression, and transport activity (Price, 2011). These properties somewhat determine their suitability for function in a proposed chloroplastic CCM. Dependent on the species, some Ci uptake systems are constitutively expressed, but in most cases, their expression is controlled by a combination of limiting Ci and light (Badger and Andrews, 1982; Kaplan et al., 1987; McGinn et al., 2003; Price et al., 2011b).
Intracellular CO2-to-HCO3− conversion in cyanobacteria is facilitated by two specialized, thylakoid-located NAD(P)H dehydrogenase (NDH1) complexes related to the respiratory complex-I from mitochondria: the low Ci-inducible, high affinity NDH-13, and the constitutive, slightly lower affinity NDH-14 complexes (Maeda et al., 2002; Ohkawa et al., 2002). The CO2 hydration subunits ChpY (CupA) and ChpX (CupB) of NDH-13 and NDH-14, respectively, convert cytoplasmic CO2 to HCO3−, energized by reduced ferredoxin or NADPH that are generated by photosynthetic electron transport, and hence light-dependent (Ogawa et al., 1985; Maeda et al., 2002; Price et al., 2008; Battchikova et al., 2011). Recently, catalytic properties of the cryo-EM structure of the NDH-13 complex have been analyzed applying quantum chemical density modeling to the cryo-EM structure, which has shed light onto putative regulatory mechanisms. CO2 hydration by NDH-13 (and by analogy NDH-14) is energetically linked to plastoquinone oxido-reduction coupled to proton-pumping, which controls the opening and closing of the putative CO2 diffusion channel and lateral removal of H+ generated in the CO2 hydration reaction catalyzed by the ChpY (CupA) subunit. This mechanism ensures that the backward reaction, and unfavorable CO2 release, is prevented (Badger and Price, 2003; Schuller et al., 2020). In plant chloroplasts, we expect such systems would require thylakoid localization for correct function.
Direct transfer of HCO3− from the outside into the cytoplasm is facilitated by three types of plasma membrane-located HCO3− transporters (Figure 1). The high affinity transporters, BCT1 and SbtA, were shown to be newly synthesized upon activation of HCO3− uptake, while constitutively expressed BicA was induced without further de novo protein synthesis (Sültemeyer et al., 1998; McGinn et al., 2003). The heteromeric BCT1 complex (encoded by the cmpABCD operon; Table 1) is a high affinity-low flux HCO3− transporter (Omata et al., 1999) of the ATP binding cassette (ABC) transporter superfamily, strongly suggesting ATP is used for energization. However, ATPase activity has not yet been demonstrated. BCT1 is composed of the membrane-anchored, substrate-binding protein CmpA, the homodimeric, membrane integral CmpB domain, and the cytoplasmic ATPase subunits CmpC and CmpD. CmpC appears to be a fusion protein which contains both the ATPase moiety and a putative regulatory substrate-binding domain homologous to CmpA. CmpA requires Ca2+ as co-ligand for binding of HCO3−, yet it is unclear whether Ca2+ plays a role in HCO3− transport (Koropatkin et al., 2007). The complexity of the proposed subunit localization of BCT1 for chloroplast envelope expression (one subunit in the IMS, one in the IEM, and two in the stroma; see below) provides further plant engineering challenges in addition to correct transporter function.
Both, BicA and SbtA (Table 1) are HCO3−/Na+ symporters that require a cell-inward directed Na+ gradient for HCO3− uptake (Shibata et al., 2002; Price et al., 2004), and as single protein transporters are attractive considerations for chloroplast engineering. BicA, a medium affinity-high flux transporter of the SLC26A solute carrier superfamily, is thought to function as a homodimer (Compton et al., 2011; Price and Howitt, 2014; Wang et al., 2019). The high affinity-low flux SbtA transporter, constitutes its own Na+-dependent HCO3− transporter superfamily, and is likely to be active as a trimer (Du et al., 2014; Fang et al., 2021; Förster et al., 2021). These requirements for Na+ for HCO3− uptake highlight the potential for excessive influx of Na+ in a chloroplast-based CCM which we discuss below.
Non-photosynthetic Bacterial Ci Uptake Systems
The DAB proteins (encoded by the dab1 and dab2 operons) first identified in Halothiobacillus neapolitanus are distributed throughout prokaryotic phyla and have been proposed to function as energy-coupled CAs accumulating HCO3− in the cytoplasm (Desmarais et al., 2019). A heterodimeric functional unit consists of the cytoplasmic exposed β-CA-like DabA protein coupled to the membrane-integral cation antiporter-like membrane subunit DabB (Figure 1B). Vectorial CO2 hydration by DabA has been hypothesized to be driven by a cation (H+ or Na+) gradient but has not yet been proven experimentally (Laughlin et al., 2020). From an engineering standpoint, DAB proteins may represent a viable alternative to NDH13/4 complexes as candidates for CO2 uptake/recapture in chloroplasts as introduction of only two proteins is required for DABs compared to 20–21 different proteins for NDH13/4 (Price et al., 2019). However, the suitability of DABs to function in chloroplasts will be uncertain until mechanisms of energization/regulation are resolved. In addition, we need to consider that DABs or any vectorial CA will only be effective in the final engineering stages once the endogenous stromal CA has been successfully removed (Price et al., 2011a).
Microalgal Ci Uptake Systems
In Chlamydomonas, HCO3− transporter genes induced under low Ci include plasma membrane-located HLA3 and LCI1 (Figure 2; Kono and Spalding, 2020), the chloroplast envelope-located LCIA (Nar1.2; Wang et al., 2011; Atkinson et al., 2016; Kono and Spalding, 2020), thylakoid membrane-integral bestrophin-like proteins BST1, BST2, and BST3 (Mukherjee et al., 2019), and the chloroplast-located CIA8 (Machingura et al., 2017). In addition, stromal LCIB/C complex and the thylakoid lumenal carbonic anhydrase CAH3 have been implied in CO2 recapture (reviewed in Mackinder, 2018; Mallikarjuna et al., 2020). Importantly, neither substrate affinities, net accumulation capacity, and energization nor regulatory mechanisms of individual transporters are sufficiently understood to evaluate their suitability for expression in C3 chloroplasts at this time (Table 1). It is highly likely though that HLA3 (Figure 1C), as a member of the ABC and transporter family, is energized by ATP hydrolysis (Wang et al., 2015), and heterologous expression of HLA3 or LCIA in Xenopus oocytes showed some HCO3− uptake activity but were not characterized further (Atkinson et al., 2016).
What are the Energetic and Functional Requirements of Ci Uptake Systems?
One major challenge for heterologous expression of Ci uptake systems is the regulation of protein function, which encompasses both primary energization and fine-tuning of activity to match dynamic photosynthetic CO2 assimilation capacity of plant leaves (Price et al., 2013; Rae et al., 2017; Mackinder, 2018). Irrespective of the organism, Ci uptake appears to be controlled at the level of gene expression as well as protein function. While our current knowledge allows us to control expression of transgenes quite effectively, control of protein function in a non-native environment is still vastly empirical and, without greater understanding, far from attaining control by rational design.
Regulation of transporter function appears to be as little understood as energization. Most knowledge has been gathered for the cyanobacterial Ci uptake systems (Table 1). In cyanobacteria, as in chloroplasts, elevated HCO3− concentration is only beneficial for photosynthetic carbon gain in the light. For maximum efficiency, Ci uptake activity needs to be in tune with day/night cycles and changes in light intensity. In cyanobacteria, CO2 uptake and HCO3− transport are activated within seconds in the light, with CO2 uptake preceding HCO3− uptake (Badger and Andrews, 1982; Price et al., 2008, 2011b), and both SbtA and BicA are inactivated within seconds in the dark (Price et al., 2013; Förster et al., 2021). While a link between light-activation/dark-inactivation of Ci uptake and the state of photosynthetic electron transport and/or to a redox signal has been suggested by Kaplan et al. (1987), the identity of the light signal, signal transduction pathways and sensory/response mechanisms of the Ci uptake proteins are still elusive. Furthermore, protein phosphorylation may play a role in post-translational modulation of HCO3− transporter activity (Sültemeyer et al., 1998), and it is uncertain whether the native cyanobacterial regulatory kinases/phosphatases could function correctly in plastids when co-expressed with their transporter targets. This level of regulation dependency needs to be addressed to ensure replication of cyanobacterial-like control of Ci uptake mechanisms in a C3 system.
Light/Dark Control of Ci Uptake
There is some evidence for redox-regulation of CO2 uptake by the NDH-1 complexes in cyanobacteria. NDH13/4 function is directly linked to the trans-thylakoid proton motive force and cyclic electron transfer at photosystem I through interaction with ferredoxin and plastoquinone intermediates of the photosynthetic electron transport chain (ETC; Schuller et al., 2020). Light-driven changes in photosynthetic electron transport cause instantaneous changes of the redox state of the ETC which modulates CO2 fixation via changes in NADPH production, ATP synthesis, and the redox-sensitive activation state of the Calvin-Benson-Bassham (CBB) cycle enzymes. In cyanobacteria, oxidizing conditions activate the small, inhibitory CP12 protein and ferredoxin-thioredoxin redox signaling cascades which inhibit the CBB cycle enzymes (via thiol-oxidation of cysteines; McFarlane et al., 2019), thus coordinating CO2 uptake and carboxylation. Given that the ETC and the ferredoxin-thioredoxin-CP12 regulatory system are highly conserved and present in all plant chloroplasts, regulatory features may already be present in chloroplasts if large, multi-gene NDH-1 complexes could be heterologously expressed. However, it is unlikely that this modus of redox-regulation applies to plasma membrane-located HCO3− transporters, which are spatially separated from the ETC and have not been detected among proteins targeted by thioredoxin (Lindahl and Florencio, 2003).
Currently without experimental evidence, other putative redox-sensitive regulatory mechanisms for cyanobacterial Ci uptake, such as eliciting signaling molecules such as Ca2+ (Torrecilla et al., 2004; Domínguez et al., 2015), light-stimulated changes in membrane potential (Murvanidze and Glagolev, 1982), and Ca2+ sensory phosphorylation relays triggered by light-dark transitions (Mata-Cabana et al., 2012) are speculative. However, regardless of the regulatory mechanism, the main concern remains whether an analogous regulatory system exists in the chloroplast and whether it can interact appropriately with the introduced foreign proteins, or, whether such systems need to be transplanted into chloroplasts alongside Ci uptake systems. Importantly, Ca2+ plays a major regulatory role for photosynthesis and related metabolism in chloroplasts and light-dark transitions elicit specific Ca2+ responses (Pottosin and Shabala, 2016). Therefore, chloroplasts harbor an extensive Ca2+ signaling infrastructure and are part of the whole plant signaling network which includes crosstalk between chloroplastic and cytoplasmic Ca2+ signaling responses to environmental stimuli (Navazio et al., 2020). How the incorporation of additional systems, which could have Ca2+ dependencies, might impact on overall inter- and intra-cellular signaling is yet to be seen.
So far, evidence for control of HCO3− uptake involving interaction of the transporter with regulatory proteins and/or additional co-factors has only emerged for SbtA. Heterologous co-expression of SbtA and its cognate PII-like SbtB proteins in E. coli abolished SbtA-mediated HCO3− uptake constitutively and formed SbtA:SbtB containing protein complexes (Du et al., 2014). This suggests activity of SbtA can be modulated through binding its respective SbtB (Fang et al., 2021). Effects on SbtA activity have not been observed in low Ci-acclimated, SbtB-deficient cyanobacterial mutants (Förster et al., 2021), although initial Ci acclimation and growth appeared to be compromised in Synechocystis sp. PCC6803 (Selim et al., 2018). However, so far, in vitro evidence suggest that certain SbtA and SbtB pairs interact in response to adenylate ratios and adenylate energy charge sensed through SbtB (Kaczmarski et al., 2019; Förster et al., 2021), and even though the in vivo role of the SbtA-SbtB interaction is not clear yet, co-expression of SbtA and SbtB may be necessary for appropriate functional control in chloroplasts.
Implications for pH Balance, Ion Homeostasis, and Energetic Requirements
While single gene HCO3− transporters such as the SbtA HCO3−/Na+ symporters are prime candidates for chloroplast expression (Du et al., 2014), accumulation of HCO3− and Na+ in the stroma in the dark could theoretically lead to pH imbalances and high concentrations of Na+ impairing chloroplast biochemistry (Price et al., 2008; Mueller et al., 2014; Myo et al., 2020). Cellular pH is tightly regulated to ensure near optimal conditions for biochemical reactions to occur. The cytoplasmic pH in Arabidopsis is maintained at about 7.3 (Shen et al., 2013), whereas the chloroplast stroma has been reported to vary between pH 7.2 in the dark to about pH 8 in the light (Höhner et al., 2016). All membrane systems in plant cells possess numerous transport systems (comprised of cation/H+ and anion/H+ exchangers) that maintain pH homeostasis in different subcellular compartments, and transmembrane H+ gradients as a proton motive energy source. In the light, the capacity for pH-regulation and buffering in chloroplasts is likely to accommodate the alkalinization caused by continued HCO3− import into the chloroplast. Bicarbonate accumulation in the chloroplast via a single transporter type is unlikely to exceed the pool sizes of up to 50mM measured in CCM-induced and actively photosynthesizing cyanobacteria (Kaplan et al., 1980; Woodger et al., 2005). Moreover, the pH disturbance associated with short-term (~ 5min) exposure of leaves to high CO2, which elevated stromal HCO3− up to 90mM in the dark and 120mM in the light, was counteracted rapidly within seconds (Hauser et al., 1995). However, it is uncertain whether pH buffering is as effective if continued HCO3− uptake in the dark were to accumulate substantial HCO3− pools without consumption by Rubisco. Consideration must therefore be given to this uncertainty in CCM engineering strategies.
The second potentially confounding issue with expression of the SbtA and BicA transporters on the chloroplast envelope is the influx of Na+. Assuming a stoichiometry of 1:1 for Na+ and HCO3− co-transport, these transporters could increase chloroplast [Na+] by at least as much as the [HCO3−] mentioned above. In contrast to halophytes which tolerate higher chloroplastic Na+ concentrations, photosynthesis in glycophytes (including many C3 crop plants) becomes impaired by subtle elevation of stromal Na+ from 0.21 to 0.38mM in Arabidopsis (Mueller et al., 2014). The NHD1 Na+/H+ antiporter on the chloroplast envelope is active in Na+ extrusion (Figure 2), maintaining a positive Na+ gradient for other Na+-dependent carriers on the chloroplast envelope, regulating stromal pH, and contributing to salt tolerance (Höhner et al., 2016; Tsujii et al., 2020). This suggests that, in particular, light/dark regulated Na+ extrusion and Na+/HCO3− symport need to be synchronized. Thus, boosting Na+ export systems on the chloroplast envelope may be required to restore ion/pH balance, which could involve overexpression of the endogenous NHD1 or expression of foreign Na+/H+ antiporters such as cyanobacterial NhaS proteins (Price et al., 2013).
Unfortunately, regulation of Na+ fluxes between different compartments of plant cells and the characteristics of Na+ carriers are not well understood, therefore making it difficult to predict how active HCO3− uptake might influence Na+ fluxes. In addition to the potential over-accumulation of stromal [Na+], it is not clear whether the cytoplasmic [Na+] and the magnitude of the Na+ gradient across the chloroplast envelope will be sufficient for optimal energization of SbtA or BicA and in varying environments. Estimates of cytoplasmic [Na+] range between 3 and 30mM (Karley et al., 2000; Tester and Davenport, 2003), which exceeds the K 0.5 (Na+ concentration supporting half-maximum HCO3− uptake rates) of 1–2mM Na+ for SbtA and BicA (Price et al., 2004; Du et al., 2014). Stromal Na+ concentrations have been reported between 0.2 and 7mM (Schröppel-Meier and Kaiser, 1988; Mueller et al., 2014). Therefore, dependent on the plant species and/or environmental conditions, cytoplasmic Na+ is in the lower concentration range, and the differential between cytoplasm and stroma, could impose limits on HCO3− uptake rates depending on substrate availability. However, plants under field conditions experience relatively higher salinity in most agricultural soils than in controlled growth environments, which means we can expect their cells operate at slightly elevated cytoplasmic Na+ levels (Tester and Davenport, 2003), which renders Na+ limitation fairly unlikely.
Based on homology to ABC transporters, the cyanobacterial BCT1 and the Chlamydomonas HLA3 (Figures 1A,C) are thought to be energized by ATP hydrolysis, but the ATP required per HCO3− transported has not been determined. Modeled ATP requirements for SbtA and BicA activity, which consume ATP indirectly as costs for proton transport to maintain the Na+ gradient, project 0.5 and 0.25 ATP, respectively, per HCO3− transported (Price et al., 2011a). Particularly at low external Ci, suppression of photorespiration by active HCO3− uptake is more ATP cost-effective than typical C3 photosynthesis, and ATP demand for transporter function should be readily covered by photophosphorylation in the chloroplast. The modeling did not consider additional ATP requirements for synthesis and maintenance of Ci uptake complexes though, since protein accumulation and turnover rates are unknown in both native organisms and chloroplasts, which is a modest pressure onto ATP production compared to the overall daily expenditure in living cells. Recent modeling of proposed pyrenoid-based systems also highlights ATP costs to chloroplastic CCMs; however, these can be limited depending on the engineering strategy (Fei et al., 2021).
How can we get Ci Uptake Systems Into the Chloroplast?
The expression of transgenes from the nuclear genome of terrestrial plants is the favored means to introduce a CCM into crop plants due to current difficulties associated with successful insertion of exogenous genes into the chloroplast genomes of some major crops (Hanson et al., 2013). Nonetheless, many proof-of-concept approaches utilize plastome expression to assess CCM components (Lin et al., 2014b; Pengelly et al., 2014; Long et al., 2018). We focus here on strategies relating to the import of nuclear-encoded proteins into chloroplastic membranes and stroma where broader application to the majority of globally important crops is feasible. This approach introduces many complicating challenges when considering the transfer of systems from a cyanobacterium where proteins are targeted to the membrane from the inside, whereas in chloroplast proteins would come from the outside.
Successful transport of HCO3− into C3 plant chloroplasts requires that a transporter will be pumping solute across the chloroplast IEM, into the chloroplast stroma. This sounds simple in principle but implies several assumptions about the transporter are true. Firstly, that it is successfully expressed and targeted to the chloroplast. Secondly, correct direction of the imported protein to the chloroplast IEM occurs. Thirdly, the protein must fold and orient itself in the appropriate manner such that its intended direction of transport is inward to the stroma. Finally, any processes which ensure activation and energization of the transporter must be met (discussed above). Correct targeting of HCO3− transporters to the chloroplast IEM has been the subject of several reports in recent years (Atkinson et al., 2016; Rolland et al., 2016, 2017; Uehara et al., 2016, 2020; Nölke et al., 2019), however, correct localization, orientation, and activation of these proteins to ensure favorable function remain an engineering challenge.
Foreign Protein Expression and Targeting
The initial step of expressing foreign genes in transgenic plants is a common point of failure due to a myriad of factors relating to gene positional effects (Pérez-González and Caro, 2019) and silencing (Jackson et al., 2014), codon usage (Nakamura and Sugiura, 2009), promotor and terminator combinations (Beyene et al., 2011; de Felippes et al., 2020), and potential degradation of the precursor protein (Lee et al., 2009; Shen et al., 2017; Hristou et al., 2020). This usually requires the analysis of relatively large numbers of plant transformation events and somewhat laborious testing of gene expression cassettes (often in transient expression systems) to ensure appropriate levels of protein expression can be achieved. We do not provide further discussion on this point but highlight that fine-tuning this aspect of CCM engineering in C3 plants is not trivial and can heavily impact on the trajectories of engineering approaches.
Once expressed, nuclear encoded proteins targeted to the chloroplasts are translocated as pre-proteins within the cytosol where chaperones, such as Hsp70, Hsp90, and the 14–3-3 protein complex are involved throughout the translocation process (Figure 3; May and Soll, 2000; Schwenkert et al., 2011). Proteins translated in the cytosol and destined for the chloroplast either remain unfolded with the help of chaperones (Jarvis, 2008), or can be imported to the chloroplast in a fully-formed state (Ganesan et al., 2018), prior to translocation across the chloroplast envelope. These chaperones are crucial to prevent the premature folding of large proteins and aggregation and/or degradation of pre-proteins (Wojcik and Kriechbaumer, 2021).
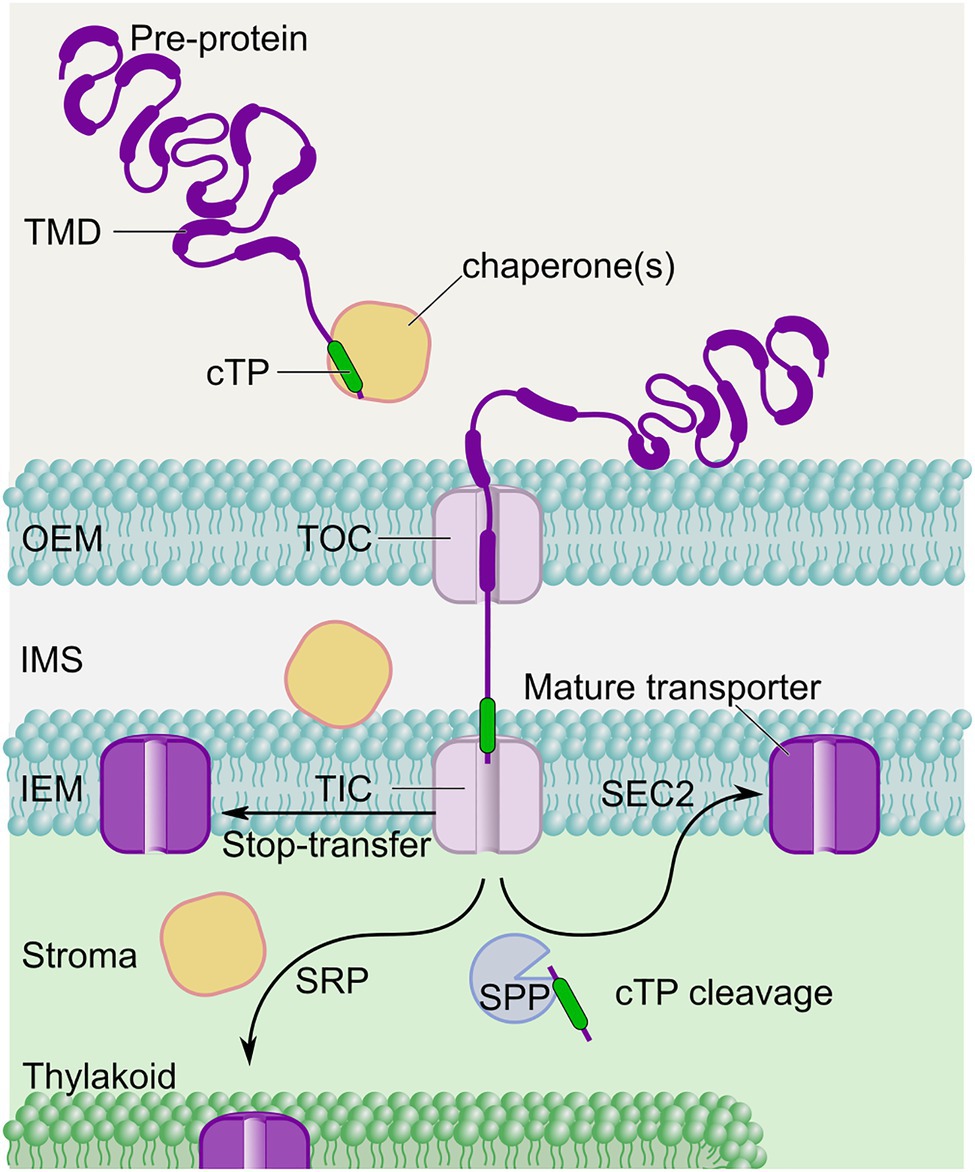
Figure 3. Possible pathways for membrane HCO3− transporter insertion in C3 chloroplasts. For successful targeting to the correct membrane in C3 chloroplasts, candidate inorganic carbon uptake system proteins need to utilize an appropriate chloroplast targeting peptide (cTP) at the N-terminus of the nuclear-encoded pre-protein. Chaperones co-ordinate transit of membrane pre-proteins to the TIC-TOC translocons enabling initial import into the chloroplast. Here, proteins can undergo a number of localization processes, dependent on sequence motifs and interactions. Proteins destined for the IEM are handled by the stop-transfer or post-import (SEC2) pathways, whereas the signal recognition particle (SRP) pathway is utilized by proteins destined for the thylakoid (Lee et al., 2017). Ultimate localization can be partly driven by transmembrane domain (TMD) sequences (Rolland et al., 2017; Singhal and Fernandez, 2017). The stromal processing peptidase (SPP) must recognize and cleave the cTP in such a way that no functionally disruptive scar is left behind on mature transporters when they reach their proper destination. Very little is known about targeting foreign proteins to the IMS.
Upon reaching the chloroplast, pre-proteins enter through the TIC-TOC pathway and are then directed within the chloroplast to their final destination (e.g., IEM, OEM, stroma, thylakoid membrane, or lumen; Figure 3; Oh and Hwang, 2015; Lee et al., 2017; Xu et al., 2020). Noticeably, post-import insertion into the IEM could involve additional processing by the Cpn60/Cpn10 chaperonin complex within the stroma, prior to insertion into the IEM through a membrane bound translocase (SEC2; Li and Schnell, 2006; Li et al., 2017). These various processes are facilitated by the pre-protein chloroplast transit peptide (cTP) which possesses binding sites for chaperones and is crucial for targeting nuclear-encoded proteins into the chloroplast (Ivey et al., 2000; Rial et al., 2000; Lee and Hwang, 2018). Therefore, the types of chaperones that would mediate foreign pre-protein chloroplast import would depend on the cTP used. There is currently no understanding of the cyanobacterial chaperone requirements for CCM-related HCO3− transporters in their native systems, thus we must rely on host system chaperones for correct folding (if required) in heterologous plant expression. However, Atkinson et al. (2016) and Nölke et al. (2019) have shown successful targeting of microalgal chloroplast membrane transporters, suggesting there is a propensity for direct transfer of proteins from homologous systems with chloroplasts. Notably, the common ancestral origin of cyanobacteria and C3 plant chloroplasts is partly identified in shared phylogeny of many of their outer membrane proteins (Day and Theg, 2018), and this might suggest potential for successful transfer of cyanobacterial membrane components to the chloroplast IEM. However, the transfer of genes from the plastome to the nucleus during C3 plant evolution means both the inversion of directional insertion of membrane proteins (Day and Theg, 2018), and the emergence of cTPs to enable protein trafficking through the TIC-TOC complex and to the correct membrane (Figure 3; Knopp et al., 2020; Ramundo et al., 2020).
Correct targeting to the chloroplast membranes is further complicated by the presence of additional organelles in plant cells, and dual targeting between chloroplasts and mitochondria is commonly observed (Peeters and Small, 2001; Sharma et al., 2018). This complexity of organelle targeting (Bruce, 2000; Wojcik and Kriechbaumer, 2021) requires specific choice of cTP in proposed photosynthetic engineering strategies, and we suggest that the direction of foreign proteins to the appropriate cellular compartment is unlikely to be a one-size-fits-all solution (Rolland et al., 2017). There are also likely to be protein cargo-specific requirements which determine the choice of cTP for each heterologous membrane protein directed to the chloroplast IEM, thus identifying the need to test and tailor genetic constructs on an individual basis. This strategy is also required to optimize promotor/terminator requirements and is highly relevant in systems where protein stoichiometry (such as for multi-protein complex transporters such as BCT1) may be essential for function.
Successful incorporation of multi-component membrane transporter complexes such as BCT1 (Figures 1, 2) will require subunits which lie not only in the IEM, but also in the IMS and the stroma of the chloroplast. Targeting to the IMS has not been well investigated, with few examples in the literature investigating the subject (Kouranov et al., 1999; Vojta et al., 2007). At least two pathways to this location are thought to exist, one where proteins mature in the IMS (e.g., the TIC complex subunit Tic22; Kouranov et al., 1999), and one where proteins transit through the stroma and are then re-inserted into the IMS (e.g., MGD1; Vojta et al., 2007). Which may be the most appropriate pathway and whether foreign proteins can utilize either approach is yet to be described. In contrast, targeting to the stroma has been thoroughly studied and might therefore be the easiest to achieve (reviewed in Li and Chiu, 2010). One aspect worth mentioning is the stromal processing peptidase (SPP) which is known to cleave cTPs from several nuclear-encoded proteins imported into the stroma (Figure 3; Richter and Lamppa, 1998, 2002). The complete removal of cTPs is highly desirable in chloroplast engineering, as N-terminal additions to foreign proteins can impede their function. However, successful cTP cleavage may be prevented by cargo protein secondary and tertiary structure. With difficult cargoes, cTPs may need to be extended beyond the cleavage site with a flexible linker which will ultimately leave a scar that might also impede protein function. Notably, however, some novel cTPs have been designed to reduce the proteolytic scars while enhancing targeting of difficult protein cargoes. These engineered cTPs, such as RC2 and PC1 (Shen et al., 2017; Yao et al., 2020) include about 20 residues from its native mature cargo (a spacer to allow translocating factors better access to the cTP) which are followed by a second SPP cleavage site (to allow removal of the additional 20 residues used as spacer). Another approach that has been specifically used for the HCO3− transporters SbtA and BicA included a TEV protease cleavage site after the cTP to enable removal by a heterologously expressed TEV protease (Uehara et al., 2016, 2020).
As mentioned above, the NDH complex may depend on plastoquinone for energization, and if we were to use such a complex for CO2 recapture, the chloroplast thylakoid membrane would be the destination of choice (Figure 2; Long et al., 2016; Price et al., 2019; Hennacy and Jonikas, 2020). While the chloroplast twin arginine translocation, and secretory pathways direct mostly soluble proteins to the thylakoid lumen, it is the chloroplast signal recognition pathway (SRP) that targets membrane proteins to the thylakoid membrane (Figure 3; Smeekens et al., 1985; Schnell, 1998; Aldridge et al., 2009; Ouyang et al., 2020; Xu et al., 2020). Note that dual targeting between thylakoid and IEM was encountered when foreign transporters were targeted to the IEM (Pengelly et al., 2014; Rolland et al., 2017). A study on two closely related Arabidopsis proteins, SCY1 (thylakoids) and SCY2 (IEM), shed light on the sorting mechanism between IEM and thylakoids. In brief, the N-terminal region of SCY2 alone was not sufficient for exclusive targeting to the IEM. Instead, two internal transmembrane domains (TMDs) were required to achieve unambiguous localization to the IEM with no leakage toward the thylakoid membrane (Singhal and Fernandez, 2017). This study demonstrated that targeting is cargo-dependent. Hence, a more complex engineering of cargo TMDs might be required to successfully target foreign HCO3− transporters within the chloroplast (Rolland et al., 2017).
Control of Membrane Protein Orientation
Due to the inverted targeting strategy proposed for cyanobacterial transporters, there is potential for nuclear-encoded membrane proteins to be incorrectly oriented in the chloroplast IEM, even if targeting is successful. Most of the work done to understand membrane protein orientation (i.e., TMD topology) has been carried out in bacteria, establishing the positive-inside rule (Lys and Arg rich loops orient in the cytoplasm; von Heijne, 1986) and the charge-balanced rule (Dowhan et al., 2019). However, little is known about topology determinants in C3 plant chloroplast membranes. Membrane lipid composition is known to influence the orientation of membrane proteins in the OEM (Schleiff et al., 2001). However, since the lipid composition of the C3 chloroplast OEM differs from the IEM (Block et al., 2007), it is difficult to draw parallels between their orientation determinants. Interestingly, specific TMDs also appear to affect membrane protein orientation (Viana et al., 2010; Okawa et al., 2014). While changing lipid composition to control orientation is unrealistic in plants (but was achieved in bacteria; Dowhan et al., 2019), rational design of TMDs, and interconnecting loops (Rapp et al., 2007) from HCO3− uptake systems might be an option. As shown for the secretory pathway in plant endoplasmic reticulum, membrane protein signal peptides may also play a role in the orientation of some proteins (Wojcik and Kriechbaumer, 2021). Hence, it is reasonable to assume that correct targeting and orientation of membrane proteins in the chloroplast IEM are dependent on both the cargo protein and its targeting sequence (Rolland et al., 2016; Uehara et al., 2016, 2020). As a result, broad screening of targeting peptides for candidate cyanobacterial membrane protein cargos is likely required, both on a case-by-case basis and possibly between heterologous hosts. Membrane protein orientation must therefore be considered when addressing CCM component expression in plant systems and will affect predicted outcomes of functional HCO3− uptake assessment in transformed plants.
Perspectives and Conclusion
Application of synthetic biology approaches to elevate HCO3− concentrations in C3 plant chloroplasts, as a means to enhance Rubisco carboxylation, is an ongoing engineering endeavor among plant biologists. It is, however, a complex task which needs to be considered within a broad framework of molecular and physiological complexity. Efforts to heterologously express candidate HCO3− transporters and CO2-to-HCO3− converting complexes in C3 plants must therefore be contemplated within this context. Therefore, it is critically important that researchers addressing this challenge gather evidence of correct targeting, orientation and processing of protein transporters in plant systems. Functionality should be addressed where possible, and techniques which provide evidence of successful HCO3− import (e.g., Tolleter et al., 2017) and elevated leaf-level carboxylation should accompany reports of plant growth and productivity to ensure that predicted physiological outcomes correlate with enhanced growth. In addition to this, greater detail is required on the functional characterization of existing HCO3− uptake systems in their native systems (Table 1), while an understanding of the broader natural variation in HCO3− uptake systems (e.g., Scott et al., 2018; Desmarais et al., 2019) should be accumulated to provide greater options for engineering purposes.
Author Contributions
BF generated the table. BML generated the figures. All authors contributed to the article and approved the submitted version.
Funding
This work was supported by a sub-award from the University of Illinois as part of the research project Realizing Increased Photosynthetic Efficiency (RIPE) that is funded by the Bill & Melinda Gates Foundation, Foundation for Food and Agriculture Research, and the UK Government’s Department for International Development under grant number OPP1172157. We also acknowledge funding support from the Australian Research Council Centre of Excellence for Translational Photosynthesis (CE140100015).
Conflict of Interest
The authors declare that the research was conducted in the absence of any commercial or financial relationships that could be construed as a potential conflict of interest.
Publisher’s Note
All claims expressed in this article are solely those of the authors and do not necessarily represent those of their affiliated organizations, or those of the publisher, the editors and the reviewers. Any product that may be evaluated in this article, or claim that may be made by its manufacturer, is not guaranteed or endorsed by the publisher.
Acknowledgments
The authors thank Suyan Yee and Nghiem Nguyen for proofreading the final manuscript.
References
Aldridge, C., Cain, P., and Robinson, C. (2009). Protein transport in organelles: protein transport into and across the thylakoid membrane. FEBS J. 276, 1177–1186. doi: 10.1111/j.1742-4658.2009.06875.x
Atkinson, N., Feike, D., Mackinder, L. C. M., Meyer, M. T., Griffiths, H., Jonikas, M. C., et al. (2016). Introducing an algal carbon-concentrating mechanism into higher plants: location and incorporation of key components. Plant Biotechnol. J. 14, 1302–1315. doi: 10.1111/pbi.12497
Atkinson, N., Mao, Y., Chan, K. X., and McCormick, A. J. (2020). Condensation of rubisco into a proto-pyrenoid in higher plant chloroplasts. Nat. Commun. 11:6303. doi: 10.1038/s41467-020-20132-0
Badger, M. R., and Andrews, T. J. (1982). Photosynthesis and inorganic carbon usage by the marine cyanobacterium, Synechococcus sp. Plant Physiol. 70, 517–523. doi: 10.1104/pp.70.2.517
Badger, M. R., and Price, G. D. (2003). CO2 concentrating mechanisms in cyanobacteria: molecular components, their diversity and evolution. J. Exp. Bot. 54, 609–622. doi: 10.1093/jxb/erg076
Batista-Silva, W., da Fonseca-Pereira, P., Martins, A. O., Zsögön, A., Nunes-Nesi, A., and Araújo, W. L. (2020). Engineering improved photosynthesis in the era of synthetic biology. Plant Commun. 1:100032. doi: 10.1016/j.xplc.2020.100032
Battchikova, N., Eisenhut, M., and Aro, E. M. (2011). Cyanobacterial NDH-1 complexes: novel insights and remaining puzzles. Biochim. Biophys. Acta Bioenerg. 1807, 935–944. doi: 10.1016/j.bbabio.2010.10.017
Beyene, G., Buenrostro-Nava, M. T., Damaj, M. B., Gao, S.-J., Molina, J., and Mirkov, T. E. (2011). Unprecedented enhancement of transient gene expression from minimal cassettes using a double terminator. Plant Cell Rep. 30, 13–25. doi: 10.1007/s00299-010-0936-3
Block, M. A., Douce, R., Joyard, J., and Rolland, N. (2007). Chloroplast envelope membranes: a dynamic interface between plastids and the cytosol. Photosynth. Res. 92, 225–244. doi: 10.1007/s11120-007-9195-8
Bölter, B., Soll, J., Hill, K., Hemmler, R., and Wagner, R. (1999). A rectifying ATP-regulated solute channel in the chloroplastic outer envelope from pea. EMBO J. 18, 5505–5516. doi: 10.1093/emboj/18.20.5505
Bruce, B. D. (2000). Chloroplast transit peptides: structure, function and evolution. Trends Cell Biol. 10, 440–447. doi: 10.1016/S0962-8924(00)01833-X
Busch, F. A. (2020). Photorespiration in the context of rubisco biochemistry, CO2 diffusion and metabolism. Plant J. 101, 919–939. doi: 10.1111/tpj.14674
Compton, E. L. R., Karinou, E., Naismith, J. H., Gabel, F., and Javelle, A. (2011). Low resolution structure of a bacterial SLC26 transporter reveals dimeric stoichiometry and mobile intracellular domains. J. Biol. Chem. 286, 27058–27067. doi: 10.1074/jbc.M111.244533
Day, P. M., and Theg, S. M. (2018). Evolution of protein transport to the chloroplast envelope membranes. Photosynth. Res. 138, 315–326. doi: 10.1007/s11120-018-0540-x
de Felippes, F. F., McHale, M., Doran, R. L., Roden, S., Eamens, A. L., Finnegan, E. J., et al. (2020). The key role of terminators on the expression and post-transcriptional gene silencing of transgenes. Plant J. 104, 96–112. doi: 10.1111/tpj.14907
Demmig, B., and Gimmler, H. (1983). Properties of the isolated intact chloroplast at cytoplasmic K+ concentrations: light-induced cation uptake into intact chloroplasts is driven by an electrical potential difference. Plant Physiol. 73, 169–174. doi: 10.1104/pp.73.1.169
Desmarais, J. J., Flamholz, A. I., Blikstad, C., Dugan, E. J., Laughlin, T. G., Oltrogge, L. M., et al. (2019). DABs are inorganic carbon pumps found throughout prokaryotic phyla. Nat. Microbiol. 4, 2204–2215. doi: 10.1038/s41564-019-0520-8
Domínguez, D. C., Guragain, M., and Patrauchan, M. (2015). Calcium binding proteins and calcium signaling in prokaryotes. Cell Calcium 57, 151–165. doi: 10.1016/j.ceca.2014.12.006
Dowhan, W., Vitrac, H., and Bogdanov, M. (2019). Lipid-assisted membrane protein folding and topogenesis. Protein J. 38, 274–288. doi: 10.1007/s10930-019-09826-7
Du, J., Förster, B., Rourke, L., Howitt, S. M., and Price, G. D. (2014). Characterisation of cyanobacterial bicarbonate transporters in E. coli shows that SbtA homologs are functional in this heterologous expression system. PLoS One 9:e115905. doi: 10.1371/journal.pone.0115905
Duanmu, D., Wang, Y., and Spalding, M. H. (2009). Thylakoid lumen carbonic anhydrase (CAH3) mutation suppresses air-dier phenotype of LCIB mutant in Chlamydomonas reinhardtii. Plant Physiol. 149, 929–937. doi: 10.1104/pp.108.132456
Duy, D., Soll, J., and Philippar, K. (2007). Solute channels of the outer membrane: from bacteria to chloroplasts. Biol. Chem. 388, 879–889. doi: 10.1515/BC.2007.120
Ermakova, M., Lopez-Calcagno, P. E., Raines, C. A., Furbank, R. T., and von Caemmerer, S. (2019). Overexpression of the Rieske FeS protein of the cytochrome b6f complex increases C4 photosynthesis in Setaria viridis. Commun. Biol. 2:314. doi: 10.1038/s42003-019-0561-9
Ermakova, M., Osborn, H., Groszmann, M., Bala, S., McGaughey, S., Byrt, C., et al. (2021). Expression of a CO2-permeable aquaporin enhances mesophyll conductance in the C4 species Setaria viridis. bioRxiv [Preprint]. doi: 10.1101/2021.04.28.441895
Evans, J. R., Kaldenhoff, R., Genty, B., and Terashima, I. (2009). Resistances along the CO2 diffusion pathway inside leaves. J. Exp. Bot. 60, 2235–2248. doi: 10.1093/jxb/erp117
Evans, J. R., and von Caemmerer, S. (1996). Carbon dioxide diffusion inside leaves. Plant Physiol. 110, 339–346. doi: 10.1104/pp.110.2.339
Fang, S., Huang, X., Zhang, X., Zhang, M., Hao, Y., Guo, H., et al. (2021). Molecular mechanism underlying transport and allosteric inhibition of bicarbonate transporter SbtA. Proc. Natl. Acad. Sci. U. S. A. 118:e2101632118. doi: 10.1073/pnas.2101632118
Fang, W., Si, Y., Douglass, S., Casero, D., Merchant, S. S., Pellegrini, M., et al. (2012). Transcriptome-wide changes in Chlamydomonas reinhardtii gene expression regulated by carbon dioxide and the CO2-concentrating mechanism regulator CIA5/CCM1. Plant Cell 24, 1876–1893. doi: 10.1105/tpc.112.097949
Fei, C., Wilson, A. T., Mangan, N. M., Wingreen, N. S., and Jonikas, M. C. (2021). Diffusion barriers and adaptive carbon uptake strategies enhance the modeled performance of the algal CO2-concentrating mechanism. bioRxiv [Preprint]. doi: 10.1101/2021.03.04.433933
Flamholz, A. I., Dugan, E., Blikstad, C., Gleizer, S., Ben-Nissan, R., Amram, S., et al. (2020). Functional reconstitution of a bacterial CO2 concentrating mechanism in Escherichia coli. elife 9:e59882. doi: 10.7554/eLife.59882
Flamholz, A., and Shih, P. M. (2020). Cell biology of photosynthesis over geologic time. Curr. Biol. 30, R490–R494. doi: 10.1016/j.cub.2020.01.076
Flexas, J., Ribas-Carbó, M., Hanson, D. T., Bota, J., Otto, B., Cifre, J., et al. (2006). Tobacco aquaporin NtAQP1 is involved in mesophyll conductance to CO2 in vivo. Plant J. 48, 427–439. doi: 10.1111/j.1365-313X.2006.02879.x
Förster, B., Mukherjee, B., Rourke, L., Kaczmarski, J. A., and Jackson, C. J. (2021). Regulatory adenylnucleotide-mediated binding of the PII-like protein SbtB to the cyanobacterial bicarbonate transporter SbtA is controlled by the cellular energy state. bioRxiv [Preprint]. doi: 10.1101/2021.02.14.431189
Ganesan, I., Shi, L. X., Labs, M., and Theg, S. M. (2018). Evaluating the functional pore size of chloroplast toc and tic protein translocons: import of folded proteins. Plant Cell 30, 2161–2173. doi: 10.1105/tpc.18.00427
Gao, H., Wang, Y., Fei, X., Wright, D. A., and Spalding, M. H. (2015). Expression activation and functional analysis of HLA3, a putative inorganic carbon transporter in Chlamydomonas reinhardtii. Plant J. 82, 1–11. doi: 10.1111/tpj.12788
Giordano, M., Beardall, J., and Raven, J. A. (2005). CO2 concentrating mechanisms in algae: mechanisms, environmental modulation, and evolution. Annu. Rev. Plant Biol. 56, 99–131. doi: 10.1146/annurev.arplant.56.032604.144052
Hanson, M. R., Gray, B. N., and Ahner, B. A. (2013). Chloroplast transformation for engineering of photosynthesis. J. Exp. Bot. 64, 731–742. doi: 10.1093/jxb/ers325
Hauser, M., Eichelmann, H., Oja, V., Heber, U., and Laisk, A. (1995). Stimulation by light of rapid pH regulation in the chloroplast stroma in vivo as indicated by CO2 solubilization in leaves. Plant Physiol. 108, 1059–1066. doi: 10.1104/pp.108.3.1059
Hemmler, R., Becker, T., Schleiff, E., Bölter, B., Stahl, T., Soll, J., et al. (2006). Molecular properties of Oep21, an ATP-regulated anion-selective solute channel from the outer chloroplast membrane. J. Biol. Chem. 281, 12020–12029. doi: 10.1074/jbc.M513586200
Hennacy, J. H., and Jonikas, M. C. (2020). Prospects for engineering biophysical CO2 concentrating mechanisms into land plants to enhance yields. Annu. Rev. Plant Biol. 71, 461–485. doi: 10.1146/annurev-arplant-081519-040100
Höhner, R., Aboukila, A., Kunz, H. H., and Venema, K. (2016). Proton gradients and proton-dependent transport processes in the chloroplast. Front. Plant Sci. 7:218. doi: 10.3389/fpls.2016.00218
Hristou, A., Grimmer, J., and Baginsky, S. (2020). The secret life of chloroplast precursor proteins in the cytosol. Mol. Plant 13, 1111–1113. doi: 10.1016/j.molp.2020.07.004
Ivey, R. A., Subramanian, C., and Bruce, B. D. (2000). Identification of a Hsp70 recognition domain within the rubisco small subunit transit peptide. Plant Physiol. 122, 1289–1299. doi: 10.1104/pp.122.4.1289
Jackson, M. A., Sternes, P. R., Mudge, S. R., Graham, M. W., and Birch, R. G. (2014). Design rules for efficient transgene expression in plants. Plant Biotechnol. J. 12, 925–933. doi: 10.1111/pbi.12197
Jarvis, P. (2008). Targeting of nucleus-encoded proteins to chloroplasts in plants. New Phytol. 179, 257–285. doi: 10.1111/j.1469-8137.2008.02452.x
Jin, S., Sun, J., Wunder, T., Tang, D., Cousins, A. B., Sze, S. K., et al. (2016). Structural insights into the LCIB protein family reveals a new group of β-carbonic anhydrases. Proc. Natl. Acad. Sci. U. S. A. 113, 14716–14721. doi: 10.1073/pnas.1616294113
Kaczmarski, J. A., Hong, N. S., Mukherjee, B., Wey, L. T., Rourke, L., Förster, B., et al. (2019). Structural basis for the allosteric regulation of the SbtA bicarbonate transporter by the PII-like protein, SbtB, from Cyanobium sp. PCC7001. Biochemistry 58, 5030–5039. doi: 10.1021/acs.biochem.9b00880
Kaplan, A., Badger, M. R., and Berry, J. A. (1980). Photosynthesis and the intracellular inorganic carbon pool in the bluegreen alga Anabaena variabilis: response to external CO2 concentration. Planta 149, 219–226. doi: 10.1007/BF00384557
Kaplan, A., Zenvirth, D., Marcus, Y., Omata, T., and Ogawa, T. (1987). Energization and activation of inorganic carbon uptake by light in cyanobacteria. Plant Physiol. 84, 210–213. doi: 10.1104/pp.84.2.210
Karley, A. J., Leigh, R. A., and Sanders, D. (2000). Where do all the ions go? The cellular basis of differential ion accumulation in leaf cells. Trends Plant Sci. 5, 465–470. doi: 10.1016/s1360-1385(00)01758-1
Knopp, M., Garg, S. G., Handrich, M., and Gould, S. B. (2020). Major changes in plastid protein import and the origin of the Chloroplastida. iScience 23:100896. doi: 10.1016/j.isci.2020.100896
Kono, A., and Spalding, M. H. (2020). LCI1, a Chlamydomonas reinhardtii plasma membrane protein, functions in active CO2 uptake under low CO2. Plant J. 102, 1127–1141. doi: 10.1111/tpj.14761
Koropatkin, N. M., Koppenaal, D. W., Pakrasi, H. B., and Smith, T. J. (2007). The structure of a cyanobacterial bicarbonate transport protein, CmpA. J. Biol. Chem. 282, 2606–2614. doi: 10.1074/jbc.M610222200
Kouranov, A., Wang, H., and Schnell, D. J. (1999). Tic22 is targeted to the intermembrane space of chloroplasts by a novel pathway. J. Biol. Chem. 274, 25181–25186. doi: 10.1074/jbc.274.35.25181
Kromdijk, J., Głowacka, K., Leonelli, L., Gabilly, S. T., Iwai, M., Niyogi, K. K., et al. (2016). Improving photosynthesis and crop productivity by accelerating recovery from photoprotection. Science 354, 857–861. doi: 10.1126/science.aai8878
Kromdijk, J., and Long, S. P. (2016). One crop breeding cycle from starvation? How engineering crop photosynthesis for rising CO2 and temperature could be one important route to alleviation. Proc. R. Soc. B 283:20152578. doi: 10.1098/rspb.2015.2578
Laughlin, T. G., Savage, D. F., and Davies, K. M. (2020). Recent advances on the structure and function of NDH-1: the complex I of oxygenic photosynthesis. Biochim. Biophys. Acta Bioenerg. 1861:148254. doi: 10.1016/j.bbabio.2020.148254
Lee, D. W., and Hwang, I. (2018). Evolution and design principles of the diverse chloroplast transit peptides. Mol. Cell 41, 161–167. doi: 10.14348/molcells.2018.0033
Lee, D. W., Lee, J., and Hwang, I. (2017). Sorting of nuclear-encoded chloroplast membrane proteins. Curr. Opin. Plant Biol. 40, 1–7. doi: 10.1016/j.pbi.2017.06.011
Lee, S., Lee, D. W., Lee, Y., Mayer, U., Stierhof, Y. D., Lee, S., et al. (2009). Heat shock protein cognate 70-4 and an E3 ubiquitin ligase, CHIP, mediate plastid-destined precursor degradation through the ubiquitin-26S proteasome system in Arabidopsis. Plant Cell 21, 3984–4001. doi: 10.1105/tpc.109.071548
Li, H. M., and Chiu, C. C. (2010). Protein transport into chloroplasts. Annu. Rev. Plant Biol. 61, 157–180. doi: 10.1146/annurev-arplant-042809-112222
Li, Y., Martin, J. R., Aldama, G. A., Fernandez, D. E., and Cline, K. (2017). Identification of putative substrates of SEC2, a chloroplast inner envelope translocase. Plant Physiol. 173, 2121–2137. doi: 10.1104/pp.17.00012
Li, M., and Schnell, D. J. (2006). Reconstitution of protein targeting to the inner envelope membrane of chloroplasts. J. Cell Biol. 175, 249–259. doi: 10.1083/jcb.200605162
Li, L., Wang, H., Gago, J., Cui, H., Qian, Z., Kodama, N., et al. (2015). Harpin Hpa1 interacts with aquaporin PIP1;4 to promote the substrate transport and photosynthesis in Arabidopsis. Sci. Rep. 5:17207. doi: 10.1038/srep17207
Lin, M. T., Occhialini, A., Andralojc, P. J., Devonshire, J., Hines, K. M., Parry, M. A. J., et al. (2014a). β-Carboxysomal proteins assemble into highly organized structures in Nicotiana chloroplasts. Plant J. 79, 1–12. doi: 10.1111/tpj.12536
Lin, M. T., Occhialini, A., Andralojc, P. J., Parry, M. A. J., and Hanson, M. R. (2014b). A faster rubisco with potential to increase photosynthesis in crops. Nature 513, 547–550. doi: 10.1038/nature13776
Lindahl, M., and Florencio, F. J. (2003). Thioredoxin-linked processes in cyanobacteria are as numerous as in chloroplasts, but targets are different. Proc. Natl. Acad. Sci. U. S. A. 100, 16107–16112. doi: 10.1073/pnas.2534397100
Long, B. M., Förster, B., Pulsford, S. B., Price, G. D., and Badger, M. R. (2021). Rubisco proton production can drive the elevation of CO2 within condensates and carboxysomes. Proc. Natl. Acad. Sci. U. S. A. 118:e2014406118. doi: 10.1073/pnas.2014406118
Long, B. M., Hee, W. Y., Sharwood, R. E., Rae, B. D., Kaines, S., Lim, Y. L., et al. (2018). Carboxysome encapsulation of the CO2-fixing enzyme rubisco in tobacco chloroplasts. Nat. Commun. 9:3570. doi: 10.1038/s41467-018-06044-0
Long, B. M., Rae, B. D., Rolland, V., Förster, B., and Price, G. D. (2016). Cyanobacterial CO2-concentrating mechanism components: function and prospects for plant metabolic engineering. Curr. Opin. Plant Biol. 31, 1–8. doi: 10.1016/j.pbi.2016.03.002
López-Calcagno, P. E., Brown, K. L., Simkin, A. J., Fisk, S. J., Vialet-Chabrand, S., Lawson, T., et al. (2020). Stimulating photosynthetic processes increases productivity and water-use efficiency in the field. Nat. Plants 6, 1054–1063. doi: 10.1038/s41477-020-0740-1
Machingura, M. C., Bajsa-Hirschel, J., Laborde, S. M., Schwartzenburg, J. B., Mukherjee, B., Mukherjee, A., et al. (2017). Identification and characterization of a solute carrier, CIA8, involved in inorganic carbon acclimation in Chlamydomonas reinhardtii. J. Exp. Bot. 68, 3879–3890. doi: 10.1093/jxb/erx189
Mackinder, L. C. M. (2018). The Chlamydomonas CO2 -concentrating mechanism and its potential for engineering photosynthesis in plants. New Phytol. 217, 54–61. doi: 10.1111/nph.14749
Maeda, S. I., Badger, M. R., and Price, G. D. (2002). Novel gene products associated with NdhD3/D4-containing NDH-1 complexes are involved in photosynthetic CO2 hydration in the cyanobacterium, Synechococcus sp. PCC7942. Mol. Microbiol. 43, 425–435. doi: 10.1046/j.1365-2958.2002.02753.x
Mallikarjuna, K., Narendra, K., Ragalatha, R., and Rao, B. J. (2020). Elucidation and genetic intervention of CO2 concentration mechanism in Chlamydomonas reinhardtii for increased plant primary productivity. J. Biosci. 45, 1–18. doi: 10.1007/s12038-020-00080-z
Mata-Cabana, A., García-Domínguez, M., Florencio, F. J., and Lindahl, M. (2012). Thiol-based redox modulation of a cyanobacterial eukaryotic-type serine/threonine kinase required for oxidative stress tolerance. Antioxid. Redox Signal. 17, 521–533. doi: 10.1089/ars.2011.4483
May, T., and Soll, J. (2000). 14-3-3 proteins form a guidance complex with chloroplast precursor proteins in plants. Plant Cell 12, 53–63. doi: 10.2307/3871029
McFarlane, C. R., Shah, N. R., Kabasakal, B. V., Echeverria, B., Cotton, C. A. R., Bubeck, D., et al. (2019). Structural basis of light-induced redox regulation in the Calvin–Benson cycle in cyanobacteria. Proc. Natl. Acad. Sci. U. S. A. 116, 20984–20990. doi: 10.1073/pnas.1906722116
McGinn, P. J., Price, G. D., Maleszka, R., and Badger, M. R. (2003). Inorganic carbon limitation and light control the expression of transcripts related to the CO2-concentrating mechanism in the cyanobacterium Synechocystis sp. strain PCC6803. Plant Physiol. 132, 218–229. doi: 10.1104/pp.019349
McGrath, J. M., and Long, S. P. (2014). Can the cyanobacterial carbon-concentrating mechanism increase photosynthesis in crop species? A theoretical analysis. Plant Physiol. 164, 2247–2261. doi: 10.1104/pp.113.232611
Morison, J. I. L., Gallouët, E., Lawson, T., Cornic, G., Herbin, R., and Baker, N. R. (2005). Lateral diffusion of CO2 in leaves is not sufficient to support photosynthesis. Plant Physiol. 139, 254–266. doi: 10.1104/pp.105.062950
Moroney, J. V., and Ynalvez, R. A. (2007). Proposed carbon dioxide concentrating mechanism in Chlamydomonas reinhardtii. Eukaryot. Cell 6, 1251–1259. doi: 10.1128/EC.00064-07
Mueller, M., Kunz, H.-H., Schroeder, J. I., Kemp, G., Young, H. S., and Neuhaus, H. E. (2014). Decreased capacity for sodium export out of Arabidopsis chloroplasts impairs salt tolerance, photosynthesis and plant performance. Plant J. 78, 646–658. doi: 10.1111/tpj.12501
Mukherjee, A., Lau, C. S., Walker, C. E., Rai, A. K., Prejean, C. I., Yates, G., et al. (2019). Thylakoid localized bestrophin-like proteins are essential for the CO2 concentrating mechanism of Chlamydomonas reinhardtii. Proc. Natl. Acad. Sci. U. S. A. 116, 16915–16920. doi: 10.1073/pnas.1909706116
Murvanidze, G. V., and Glagolev, A. N. (1982). Electrical nature of the taxis signal in cyanobacteria. J. Bacteriol. 150, 239–244. doi: 10.1128/jb.150.1.239-244.1982
Myo, T., Tian, B., Zhang, Q., Niu, S., Liu, Z., Shi, Y., et al. (2020). Ectopic overexpression of a cotton plastidial Na+ transporter GhBASS5 impairs salt tolerance in Arabidopsis via increasing Na+ loading and accumulation. Planta 252:41. doi: 10.1007/s00425-020-03445-8
Nakamura, M., and Sugiura, M. (2009). Selection of synonymous codons for better expression of recombinant proteins in tobacco chloroplasts. Plant Biotechnol. 26, 53–56. doi: 10.5511/plantbiotechnology.26.53
Navazio, L., Formentin, E., Cendron, L., and Szabò, I. (2020). Chloroplast calcium signaling in the spotlight. Front. Plant Sci. 11:186. doi: 10.3389/fpls.2020.00186
Nölke, G., Barsoum, M., Houdelet, M., Arcalís, E., Kreuzaler, F., Fischer, R., et al. (2019). The integration of algal carbon concentration mechanism components into tobacco chloroplasts increases photosynthetic efficiency and biomass. Biotechnol. J. 14:e1800170. doi: 10.1002/biot.201800170
Ogawa, T., Miyano, A., and Inoue, Y. (1985). Photosystem-I-driven inorganic carbon transport in the cyanobacterium, Anacystis nidulans. BBA Bioenerg. 808, 77–84. doi: 10.1016/0005-2728(85)90029-5
Oh, Y. J., and Hwang, I. (2015). Targeting and biogenesis of transporters and channels in chloroplast envelope membranes: unsolved questions. Cell Calcium 58, 122–130. doi: 10.1016/j.ceca.2014.10.012
Ohkawa, H., Sonoda, M., Hagino, N., Shibata, M., Pakrasi, H. B., and Ogawa, T. (2002). Functionally distinct NAD(P)H dehydrogenases and their membrane localization in Synechocystis sp. PCC6803. Funct. Plant Biol. 29, 195–200. doi: 10.1071/pp01180
Okawa, K., Inoue, H., Adachi, F., Nakayama, K., Ito-Inaba, Y., Schnell, D. J., et al. (2014). Targeting of a polytopic membrane protein to the inner envelope membrane of chloroplasts in vivo involves multiple transmembrane segments. J. Exp. Bot. 65, 5257–5265. doi: 10.1093/jxb/eru290
Omata, T., Price, G. D., Badger, M. R., Okamura, M., Gohta, S., and Ogawa, T. (1999). Identification of an ATP-binding cassette transporter involved in bicarbonate uptake in the cyanobacterium Synechococcus sp. strain PCC 7942. Proc. Natl. Acad. Sci. 96, 13571–13576. doi: 10.1073/pnas.96.23.13571
Ouyang, M., Li, X., Zhang, J., Feng, P., Pu, H., Kong, L., et al. (2020). Liquid-liquid phase transition drives intra-chloroplast cargo sorting. Cell 180, 1144–1159.e20. doi: 10.1016/j.cell.2020.02.045
Peeters, N., and Small, I. (2001). Dual targeting to mitochondria and chloroplasts. Biochim. Biophys. Acta 1541, 54–63. doi: 10.1016/S0167-4889(01)00146-X
Pengelly, J. J. L., Föster, B., von Caemmerer, S., Badger, M. R., Price, G. D., and Whitney, S. M. (2014). Transplastomic integration of a cyanobacterial bicarbonate transporter into tobacco chloroplasts. J. Exp. Bot. 65, 3071–3080. doi: 10.1093/jxb/eru156
Pérez-González, A., and Caro, E. (2019). Benefits of using genomic insulators flanking transgenes to increase expression and avoid positional effects. Sci. Rep. 9:8474. doi: 10.1038/s41598-019-44836-6
Pottosin, I., and Shabala, S. (2016). Transport across chloroplast membranes: optimizing photosynthesis for adverse environmental conditions. Mol. Plant 9, 356–370. doi: 10.1016/j.molp.2015.10.006
Price, G. D. (2011). Inorganic carbon transporters of the cyanobacterial CO2-concentrating mechanism. Photosynth. Res. 109, 47–57. doi: 10.1007/s11120-010-9608-y
Price, G. D., and Badger, M. R. (1989a). Expression of human carbonic anhydrase in the cyanobacterium Synechococcus PCC7942 creates a high CO2-requiring phenotype. Plant Physiol. 91, 505–513. doi: 10.1104/pp.91.2.505
Price, G. D., and Badger, M. R. (1989b). Isolation and characterization of high CO2-requiring-mutants of the cyanobacterium Synechococcus PCC7942. Plant Physiol. 91, 514–525. doi: 10.1104/pp.91.2.514
Price, G. D., Badger, M. R., and von Caemmerer, S. (2011a). The prospect of using cyanobacterial bicarbonate transporters to improve leaf photosynthesis in C3 crop plants. Plant Physiol. 155, 20–26. doi: 10.1104/pp.110.164681
Price, G. D., Badger, M. R., Woodger, F. J., and Long, B. M. (2008). Advances in understanding the cyanobacterial CO2-concentrating- mechanism (CCM): functional components, ci transporters, diversity, genetic regulation and prospects for engineering into plants. J. Exp. Bot. 59, 1441–1461. doi: 10.1093/jxb/erm112
Price, G. D., and Howitt, S. M. (2011). The cyanobacterial bicarbonate transporter BicA: its physiological role and the implications of structural similarities with human SLC26 transporters. Biochem. Cell Biol. 89, 178–188. doi: 10.1139/O10-136
Price, G. D., and Howitt, S. M. (2014). Topology mapping to characterize cyanobacterial bicarbonate transporters: BicA (SulP/SLC26 family) and SbtA. Mol. Membr. Biol. 31, 177–182. doi: 10.3109/09687688.2014.953222
Price, G. D., Long, B. M., and Förster, B. (2019). DABs accumulate bicarbonate. Nat. Microbiol. 4, 2029–2030. doi: 10.1038/s41564-019-0629-9
Price, G. D., Pengelly, J. J. L., Förster, B., Du, J., Whitney, S. M., von Caemmerer, S., et al. (2013). The cyanobacterial CCM as a source of genes for improving photosynthetic CO2 fixation in crop species. J. Exp. Bot. 64, 753–768. doi: 10.1093/jxb/ers257
Price, G. D., Shelden, M. C., and Howitt, S. M. (2011b). Membrane topology of the cyanobacterial bicarbonate transporter, SbtA, and identification of potential regulatory loops. Mol. Membr. Biol. 28, 265–275. doi: 10.3109/09687688.2011.593049
Price, G. D., von Caemmerer, S., Evans, J. R., Yu, J. W., Lloyd, J., Oja, V., et al. (1994). Specific reduction of chloroplast carbonic anhydrase activity by antisense RNA in transgenic tobacco plants has a minor effect on photosynthetic CO2 assimilation. Planta 193, 331–340. doi: 10.1007/BF00201810
Price, G. D., Woodger, F. J., Badger, M. R., Howitt, S. M., and Tucker, L. (2004). Identification of a SulP-type bicarbonate transporter in marine cyanobacteria. Proc. Natl. Acad. Sci. U. S. A. 101, 18228–18233. doi: 10.1073/pnas.0405211101
Rae, B. D., Long, B. M., Badger, M. R., and Price, G. D. (2013). Functions, compositions, and evolution of the two types of carboxysomes: polyhedral microcompartments that facilitate CO2 fixation in cyanobacteria and some proteobacteria. Microbiol. Mol. Biol. Rev. 77, 357–379. doi: 10.1128/MMBR.00061-12
Rae, B. D., Long, B. M., Förster, B., Nguyen, N. D., Velanis, C. N., Atkinson, N., et al. (2017). Progress and challenges of engineering a biophysical CO2-concentrating mechanism into higher plants. J. Exp. Bot. 68, 3717–3737. doi: 10.1093/jxb/erx133
Ramundo, S., Asakura, Y., Salome, P. A., Strenkert, D., Boone, M., Mackinder, L., et al. (2020). Co-expressed subunits of dual genetic origin define a conserved supercomplex mediating essential protein import into chloroplasts. Proc. Natl. Acad. Sci. U. S. A. 117, 32739–32749. doi: 10.1073/pnas.2014294117
Rapp, M., Seppälä, S., Granseth, E., and von Heijne, G. (2007). Emulating membrane protein evolution by rational design. Science 315, 1282–1284. doi: 10.1126/science.1135406
Raven, J. A., Beardall, J., and Sánchez-Baracaldo, P. (2017). The possible evolution and future of CO2-concentrating mechanisms. J. Exp. Bot. 68, 3701–3716. doi: 10.1093/jxb/erx110
Rial, D. V., Arakaki, A. K., and Ceccarelli, E. A. (2000). Interaction of the targeting sequence of chloroplast precursors with Hsp70 molecular chaperones. Eur. J. Biochem. 267, 6239–6248. doi: 10.1046/j.1432-1327.2000.01707.x
Richter, S., and Lamppa, G. K. (1998). A chloroplast processing enzyme functions as the general stromal processing peptidase. Proc. Natl. Acad. Sci. U. S. A. 95, 7463–7468. doi: 10.1073/pnas.95.13.7463
Richter, S., and Lamppa, G. K. (2002). Determinants for removal and degradation of transit peptides of chloroplast precursor proteins. J. Biol. Chem. 277, 43888–43894. doi: 10.1074/jbc.M206020200
Rolland, V., Badger, M. R., and Price, G. D. (2016). Redirecting the cyanobacterial bicarbonate transporters BicA and SbtA to the chloroplast envelope: soluble and membrane cargos need different chloroplast targeting signals in plants. Front. Plant Sci. 7:185. doi: 10.3389/fpls.2016.00185
Rolland, N., Curien, G., Finazzi, G., Kuntz, M., Maréchal, E., Matringe, M., et al. (2012). The biosynthetic capacities of the plastids and integration between cytoplasmic and chloroplast processes. Annu. Rev. Genet. 46, 233–264. doi: 10.1146/annurev-genet-110410-132544
Rolland, V., Rae, B. D., and Long, B. M. (2017). Setting sub-organellar sights: accurate targeting of multi-transmembrane-domain proteins to specific chloroplast membranes. J. Exp. Bot. 68, 5013–5016. doi: 10.1093/jxb/erx351
Salesse-Smith, C. E., Sharwood, R. E., Busch, F. A., Kromdijk, J., Bardal, V., and Stern, D. B. (2018). Overexpression of rubisco subunits with RAF1 increases rubisco content in maize. Nat. Plants 4, 802–810. doi: 10.1038/s41477-018-0252-4
Schleiff, E., Tien, R., Salomon, M., and Soll, J. (2001). Lipid composition of outer leaflet of chloroplast outer envelope determines topology of OEP7. Mol. Biol. Cell 12, 4090–4102. doi: 10.1091/mbc.12.12.4090
Schnell, D. J. (1998). Protein targeting to the thylakoid membrane. Annu. Rev. Plant Biol. 49, 97–126. doi: 10.1146/annurev.arplant.49.1.97
Schröppel-Meier, G., and Kaiser, W. M. (1988). Ion homeostasis in chloroplasts under salinity and mineral deficiency. Plant Physiol. 87, 828–832. doi: 10.1104/pp.87.4.828
Schuller, J. M., Saura, P., Thiemann, J., Schuller, S. K., Gamiz-Hernandez, A. P., Kurisu, G., et al. (2020). Redox-coupled proton pumping drives carbon concentration in the photosynthetic complex I. Nat. Commun. 11:494. doi: 10.1038/s41467-020-14347-4
Schwenkert, S., Soll, J., and Bölter, B. (2011). Protein import into chloroplasts—how chaperones feature into the game. Biochim. Biophys. Acta Biomembr. 1808, 901–911. doi: 10.1016/j.bbamem.2010.07.021
Scott, K. M., Leonard, J. M., Boden, R., Chaput, D., Dennison, C., Haller, E., et al. (2018). Diversity in CO2-concentrating mechanisms among chemolithoautotrophs from the genera Hydrogenovibrio, Thiomicrorhabdus, and Thiomicrospira, ubiquitous in sulfidic habitats worldwide. Appl. Environ. Microbiol. 85, e02096–e02018. doi: 10.1128/AEM.02096-18
Selim, K. A., Haase, F., Hartmann, M. D., Hagemann, M., and Forchhammer, K. (2018). PII-like signaling protein SbtB links cAMP sensing with cyanobacterial inorganic carbon response. Proc. Natl. Acad. Sci. U. S. A. 115, E4861–E4869. doi: 10.1073/pnas.1803790115
Sharma, M., Bennewitz, B., and Klösgen, R. B. (2018). Rather rule than exception? How to evaluate the relevance of dual protein targeting to mitochondria and chloroplasts. Photosynth. Res. 138, 335–343. doi: 10.1007/s11120-018-0543-7
Shelden, M. C., Howitt, S. M., and Price, G. D. (2010). Membrane topology of the cyanobacterial bicarbonate transporter, BicA, a member of the SulP (SLC26A) family. Mol. Membr. Biol. 27, 12–23. doi: 10.3109/09687680903400120
Shen, J., Zeng, Y., Zhuang, X., Sun, L., Yao, X., Pimpl, P., et al. (2013). Organelle pH in the Arabidopsis endomembrane system. Mol. Plant 6, 1419–1437. doi: 10.1093/mp/sst079
Shen, B. R., Zhu, C. H., Yao, Z., Cui, L. L., Zhang, J. J., Yang, C. W., et al. (2017). An optimized transit peptide for effective targeting of diverse foreign proteins into chloroplasts in rice. Sci. Rep. 7:46231. doi: 10.1038/srep46231
Shevela, D., Do, H. N., Fantuzzi, A., Rutherford, A. W., and Messinger, J. (2020). Bicarbonate-mediated CO2 formation on both sides of photosystem II. Biochemistry 59, 2442–2449. doi: 10.1021/acs.biochem.0c00208
Shevela, D., Eaton-Rye, J. J., Shen, J. R., and Govindjee, (2012). Photosystem II and the unique role of bicarbonate: a historical perspective. Biochim. Biophys. Acta Bioenerg. 1817, 1134–1151. doi: 10.1016/j.bbabio.2012.04.003
Shi, X., and Bloom, A. (2021). Photorespiration: the futile cycle? Plan. Theory 10:908. doi: 10.3390/plants10050908
Shibata, M., Katoh, H., Sonoda, M., Ohkawa, H., Shimoyama, M., Fukuzawa, H., et al. (2002). Genes essential to sodium-dependent bicarbonate transport in cyanobacteria: function and phylogenetic analysis. J. Biol. Chem. 277, 18658–18664. doi: 10.1074/jbc.M112468200
Singhal, R., and Fernandez, D. E. (2017). Sorting of SEC translocase SCY components to different membranes in chloroplasts. J. Exp. Bot. 68, 5029–5043. doi: 10.1093/jxb/erx318
Smeekens, S., De Groot, M., Van Binsbergen, J., and Weisbeek, P. (1985). Sequence of the precursor of the chloroplast thylakoid lumen protein plastocyanin. Nature 317, 456–458. doi: 10.1038/317456a0
South, P. F., Cavanagh, A. P., Liu, H. W., and Ort, D. R. (2019). Synthetic glycolate metabolism pathways stimulate crop growth and productivity in the field. Science 363:eaat9077. doi: 10.1126/science.aat9077
Spalding, M. H. (2008). Microalgal carbon-dioxide-concentrating mechanisms: Chlamydomonas inorganic carbon transporters. J. Exp. Bot. 59, 1463–1473. doi: 10.1093/jxb/erm128
Sui, N., Huang, F., and Liu, L. N. (2020). Photosynthesis in phytoplankton: insights from the newly discovered biological inorganic carbon pumps. Mol. Plant 13, 949–951. doi: 10.1016/j.molp.2020.05.003
Sültemeyer, D., Klughammer, B., Badger, M. R., and Price, G. D. (1998). Fast induction of high-affinity HCO3− transport in cyanobacteria. Plant Physiol. 116, 183–192. doi: 10.1104/pp.116.1.183
Tester, M., and Davenport, R. (2003). Na+ tolerance and Na+ transport in higher plants. Ann. Bot. 91, 503–527. doi: 10.1093/aob/mcg058
Tolleter, D., Chochois, V., Poiré, R., Price, G. D., and Badger, M. R. (2017). Measuring CO2 and HCO3− permeabilities of isolated chloroplasts using a MIMS-18O approach. J. Exp. Bot. 68, 3915–3924. doi: 10.1093/jxb/erx188
Torrecilla, I., Leganés, F., Bonilla, I., and Fernández-Piñas, F. (2004). Light-to-dark transitions trigger a transient increase in intracellular Ca2+ modulated by the redox state of the photosynthetic electron transport chain in the cyanobacterium anabaena sp. PCC7120. Plant Cell Environ. 27, 810–819. doi: 10.1111/j.1365-3040.2004.01187.x
Tsujii, M., Tanudjaja, E., and Uozumi, N. (2020). Diverse physiological functions of cation proton antiporters across bacteria and plant cells. Int. J. Mol. Sci. 21:4566. doi: 10.3390/ijms21124566
Uehara, S., Adachi, F., Ito-Inaba, Y., and Inaba, T. (2016). Specific and efficient targeting of cyanobacterial bicarbonate transporters to the inner envelope membrane of chloroplasts in Arabidopsis. Front. Plant Sci. 7:16. doi: 10.3389/fpls.2016.00016
Uehara, S., Sei, A., Sada, M., Ito-Inaba, Y., and Inaba, T. (2020). Installation of authentic BicA and SbtA proteins to the chloroplast envelope membrane is achieved by the proteolytic cleavage of chimeric proteins in Arabidopsis. Sci. Rep. 10:2353. doi: 10.1038/s41598-020-59190-1
Uehlein, N., Sperling, H., Heckwolf, M., and Kaldenhoff, R. (2012). The Arabidopsis aquaporin PIP1;2 rules cellular CO2 uptake. Plant Cell Environ. 35, 1077–1083. doi: 10.1111/j.1365-3040.2011.02473.x
Viana, A. A. B., Li, M., and Schnell, D. J. (2010). Determinants for stop-transfer and post-import pathways for protein targeting to the chloroplast inner envelope membrane. J. Biol. Chem. 285, 12948–12960. doi: 10.1074/jbc.M110.109744
Vojta, L., Soll, J., and Bölter, B. (2007). Protein transport in chloroplasts—targeting to the intermembrane space. FEBS J. 274, 5043–5054. doi: 10.1111/j.1742-4658.2007.06023.x
von Heijne, G. (1986). The distribution of positively charged residues in bacterial inner membrane proteins correlates with the trans-membrane topology. EMBO J. 5, 3021–3027. doi: 10.1002/j.1460-2075.1986.tb04601.x
Wang, Y., Duanmu, D., and Spalding, M. H. (2011). Carbon dioxide concentrating mechanism in Chlamydomonas reinhardtii: inorganic carbon transport and CO2 recapture. Photosynth. Res. 109, 115–122. doi: 10.1007/s11120-011-9643-3
Wang, Y., Stessman, D. J., and Spalding, M. H. (2015). The CO2 concentrating mechanism and photosynthetic carbon assimilation in limiting CO2: how Chlamydomonas works against the gradient. Plant J. 82, 429–448. doi: 10.1111/tpj.12829
Wang, C., Sun, B., Zhang, X., Huang, X., Zhang, M., Guo, H., et al. (2019). Structural mechanism of the active bicarbonate transporter from cyanobacteria. Nat. Plants 5, 1184–1193. doi: 10.1038/s41477-019-0538-1
Wickell, D. A., and Li, F. W. (2020). On the evolutionary significance of horizontal gene transfers in plants. New Phytol. 225, 113–117. doi: 10.1111/nph.16022
Wojcik, S., and Kriechbaumer, V. (2021). Go your own way: membrane-targeting sequences. Plant Physiol. 185, 608–618. doi: 10.1093/plphys/kiaa058
Woodger, F. J., Badger, M. R., and Price, G. D. (2005). Sensing of inorganic carbon limitation in Synechococcus PCC7942 is correlated with the size of the internal inorganic carbon pool and involves oxygen. Plant Physiol. 139, 1959–1969. doi: 10.1104/pp.105.069146
Xu, X., Ouyang, M., Lu, D., Zheng, C., and Zhang, L. (2020). Protein sorting within chloroplasts. Trends Cell Biol. 31, 9–16. doi: 10.1016/j.tcb.2020.09.011
Keywords: CO2-concentrating mechanism, bicarbonate transport, chloroplast envelope, improving photosynthesis, chloroplast engineering
Citation: Rottet S, Förster B, Hee WY, Rourke LM, Price GD and Long BM (2021) Engineered Accumulation of Bicarbonate in Plant Chloroplasts: Known Knowns and Known Unknowns. Front. Plant Sci. 12:727118. doi: 10.3389/fpls.2021.727118
Edited by:
Patricia León, National Autonomous University of Mexico, MexicoReviewed by:
Suresh Kumar, Indian Agricultural Research Institute (ICAR), IndiaDinakar Challabathula, Central University of Tamil Nadu, India
Copyright © 2021 Rottet, Förster, Hee, Rourke, Price and Long. This is an open-access article distributed under the terms of the Creative Commons Attribution License (CC BY). The use, distribution or reproduction in other forums is permitted, provided the original author(s) and the copyright owner(s) are credited and that the original publication in this journal is cited, in accordance with accepted academic practice. No use, distribution or reproduction is permitted which does not comply with these terms.
*Correspondence: G. Dean Price, ZGVhbi5wcmljZUBhbnUuZWR1LmF1
†These authors have contributed equally to this work