- 1Faculty of Life and Environmental Sciences, University of Tsukuba, Tsukuba, Japan
- 2Western Highlands Agriculture and Forestry Science Institute, Buon Ma Thuot, Vietnam
Asian soybean rust (ASR) caused by Phakopsora pachyrhizi, an obligate biotrophic fungal pathogen, is the most devastating soybean production disease worldwide. Currently, timely fungicide application is the only means to control ASR in the field. We investigated cellulose nanofiber (CNF) application on ASR disease management. CNF-treated leaves showed reduced lesion number after P. pachyrhizi inoculation compared to control leaves, indicating that covering soybean leaves with CNF confers P. pachyrhizi resistance. We also demonstrated that formation of P. pachyrhizi appressoria, and also gene expression related to these formations, such as chitin synthases (CHSs), were significantly suppressed in CNF-treated soybean leaves compared to control leaves. Moreover, contact angle measurement revealed that CNF converts soybean leaf surface properties from hydrophobic to hydrophilic. These results suggest that CNF can change soybean leaf surface hydrophobicity, conferring resistance against P. pachyrhizi, based on the reduced expression of CHSs, as well as reduced formation of pre-infection structures. This is the first study to investigate CNF application to control field disease.
Introduction
Diseases in important crop plants have a significant negative impact on agricultural productivity. For example, Asian soybean rust (ASR) caused by Phakopsora pachyrhizi, an obligate biotrophic fungal pathogen, is the most devastating soybean production disease worldwide, with an estimated crop yield loss of up to 90%. ASR has impacted the South American economy in recent years. Godoy et al. (2016) reported that the losses caused by ASR were over 2 billion US dollars in Brazil annually between 2003 and 2014. Although most rust fungi have a high host specificity, the P. pachyrhizi host range is broad and can infect diverse leguminous plant leaves in the field (Slaminko et al., 2008). The infection process starts when urediniospores germinate to produce a single germ-tube with an appressorium. Unlike cereal rust fungi that penetrates through stomata (Bolton et al., 2008), P. pachyrhizi directly penetrates into host plant epidermal cells by an appressorial peg. After penetration, P. pachyrhizi extends the infection hyphae and forms haustoria (feeding structures) in the mesophyll cells 24–48 h after infection (Goellner et al., 2010). Five to eight days after infection, P. pachyrhizi then produces urediniospores by asexual reproduction (Goellner et al., 2010). Urediniospores can be dispersed by wind and germinate on other host plants.
There are several ASR control methods for soybean protection against P. pachyrhizi, including chemical control by fungicide application, growing ASR resistant soybean cultivars, and employing cultivation practices. Synthetic fungicides are the primary ASR disease control method. However, fungicide use can cause many problems such as environmental impacts (Maltby et al., 2009), increased production costs (Godoy et al., 2015), and the emergence of fungicide-resistant pathogens (Langenbach et al., 2016; Klosowski et al., 2018). Another major and effective control method is breeding or engineering of ASR resistant soybean cultivars. Analysis of soybean accessions disclosed six dominant R genes conferring resistance to a particular P. pachyrhizi race, and these loci were referred to as the Rpp 1–6 genes (Bromfield and Hartwig, 1980; McLean and Byth, 1980; Hartwig, 1986; Garcia et al., 2008; Li et al., 2012). However, none of the soybean accessions in the world show resistance to all P. pachyrhizi races (Monteros et al., 2007). Due to the limited resistance available in soybean cultivars, heterologous expression of resistance genes from other plant species in soybean has been investigated as an alternative source of ASR resistance. Kawashima et al. (2016) reported that soybean plants expressing Cajanus cajan Resistance against Phakopsora pachyrhizi 1 (CcRpp1) from pigeon pea (Cajanus cajan) showed full resistance against P. pachyrhizi. Conversely, identifying resistance traits from non-host plant species has become an intelligent approach. Uppalapati et al. (2012) screened Medicago truncatula Tnt1 mutant lines and identified an inhibitor of rust germ tube differentiation 1 (irg1) mutant with reduced formation of pre-infection structures, including germ-tubes and appressoria. They demonstrated that the loss of abaxial epicuticular wax accumulation resulting in reduced surface hydrophobicity inhibited formation of pre-infection structures on the irg1 mutant (Uppalapati et al., 2012). Furthermore, Ishiga et al. (2013) reported that gene expression related to pre-infection structure formation was activated on the hydrophobic surface of the M. truncatula wild-type, but not on the irg1 mutant, based on P. pachyrhizi transcriptome analysis, suggesting that leaf surface hydrophobicity can trigger gene expression related to formation of pre-infection structures. Based on these previous studies, we hypothesized that modification of leaf surface hydrophobicity might be a useful strategy to confer resistance against P. pachyrhizi.
Cellulose is an organic polysaccharide consisting of a β-1,4 linked glucopyranose skeleton. Cellulose is an important structural component of plant primary cell walls and is essential in maintaining the plant structural phase. Due to the positive properties, cellulose has been investigated as an application in different research and development fields including energy, environmental, water, and biomedical related fields (Mondal, 2017). Cellulose nanofiber (CNF) can be produced from cellulose, which is one of the most abundant and renewable biomasses in nature (Abe et al., 2007). Because CNF exhibits properties such as low weight, high aspect ratio, high strength, high stiffness, and large surface area, CNF potentially has wide areas of application. There are several CNF isolation methods, e.g., acid hydrolysis, enzymatic hydrolysis, and mechanical processes. The aqueous counter collision (ACC) method can make it possible to cleave interfacial interactions among cellulose molecules without any chemical modification (Kondo et al., 2014). Because of this characteristic, CNF made by the ACC method has higher thermal stability and crystallinity than chemically separated CNF. Both hydrophobic and hydrophilic sites co-exist in a cellulose molecule resulting in amphiphilic properties when CNF is derived from the ACC method. Kose et al. (2011) reported that coating with CNF derived from the ACC method could switch surface hydrophilic and hydrophobic properties, depending on substrate characteristics. They demonstrated that coating a filter paper and polyethylene with CNF changed the surface property into hydrophobic and hydrophilic, respectively (Kose et al., 2011). In addition, Halim et al. (2020) demonstrated that the contact angle of CNF prepared by the ACC method was smaller than that of CNF prepared by chemical treatment, suggesting that CNF made by the ACC method has higher wettability than CNF made by other methods. To investigate the potential application of CNF in agriculture, we examined whether coating with CNF protected soybean plants against P. pachyrhizi. We show that a specific CNF property can change soybean leaf surface hydrophobicity, resulting in reduced formation of pre-infection structures associated with reduced P. pachyrhizi infection.
Materials and Methods
Plant Growth Conditions, Pathogen Inoculation Assay, and CNF Treatment
Susceptible soybean cultivar seeds (Glycine max cv. Enrei) were germinated in a growth chamber at 25/20°C with 16-h-light/8-h-dark cycle (100–150 μmol m–2 s–1) for 3–4 weeks.
An isolate of the ASR pathogen P. pachyrhizi T1–2 (Yamaoka et al., 2014) was maintained on soybean leaves. Fresh urediniospores were collected and suspended in distilled water with 0.001% Tween 20 (FUJIFILM, Tokyo, Japan). The 3-week-old soybean plants were spray-inoculated with 1 × 105 spores/ml using a hand sprayer for uniform spore deposition. The inoculated plants were maintained in a chamber for 24 h with 90–95% humidity at 23°C in the dark. The plants were then transferred to a growth chamber (22/20°C with 16-h-light/8-h-dark cycle) and incubated further to allow symptom development. To quantify ASR lesion number on CNF-treated plants, soybean leaves were spray-inoculated with P. pachyrhizi. At 10 days after inoculation, photographs were taken, and lesions were counted to calculate the lesion number per cm2. Lesions were counted from 54 random fields on three independent leaves.
Cellulose nanofiber (marketed as nanoforest®) was supplied through the courtesy of Chuetsu Pulp & Paper (Takaoka, Japan). CNF suspension was adjusted to a concentration of 0.1% (v/v) in water including 0.02% Tween 20 before treatment. Both adaxial and abaxial sides of soybean leaves were spray-treated with 0.1% CNF till runoff and then the treated soybean plants were dried at room temperature for 3–4 h before inoculation. Scopoletin (TCI, Tokyo, Japan) was pre-solved as 500 mM stock solutions in dimethyl sulfoxide (DMSO; FUJIFILM) and diluted to 500 μM in P. pachyrhizi spore suspensions.
Quantification of Pre-infection Structures Formation
To quantify the formation of pre-infection structures including germ-tubes and appressoria on control, CNF-, and scopletin-treated plants, soybean leaves were spray-inoculated with P. pachyrhizi 1 × 105 spores/ml. At 6 h after inoculation, the leaves were observed with an Olympus BX51 fluorescence microscope after Calcofluor White (Sigma-Aldrich, St. Louis, MO, United States) staining and photographed. The germ-tubes forming differentiated appressoria were counted as appressoria. The differentiated germ-tubes without appressoria that grew on the leaf surface were also counted from at least 100 urediniosopres on three independent leaves.
The formation of pre-infection structures on borosilicate glass slides and polyethylene tape with or without CNF treatment was quantified after dropping P. pachyrhizi spores (2 × 105/ml). Six hours after inoculation, pre-infection structures were observed with a Nikon ECLIPSE 80i phase contrast microscope. The germ-tubes forming differentiated appressoria were counted as appressoria. The differentiated germ-tubes without appressoria that grew on the leaf surface were also counted from at least 500 urediniosopres on three independent leaves.
Contact Angle Measurement on Soybean Leaves and Polyethylene Tapes
The surface hydrophobicity on the CNF-treated leaves, borosilicate glass slides, and polyethylene tapes were investigated based on contact angle measurement using an automatic contact angle meter DM-31 (Kyowa Interface Science, Niiza, Japan). The contact angle was measured by dropping 2 μl of water from a syringe attached to the DM-31 automatic contact angle meter. The contact angle was measured on the adaxial and abaxial leaf surfaces, and polyethylene tapes with or without 0.1% CNF treatments. The contact angle was analyzed using the multi-functional integrated analysis software FAMAS (Kyowa Interface Science).
RNA-Spray-Induced Gene Silencing of Chitin synthases
Double-stranded RNA (dsRNA) of green fluorecent protein (GFP), and chitin synthase (CHS) were synthesized using the in vitro Transcription T7 Kit (TaKaRa, Otsu, Japan). Briefly, we designed three primer sets to amplify P. pachyrhizi CHS5-1 fragments (Supplementary Figure 1 and Supplementary Table 1). After RT-PCR amplification, fragments were purified and used as templates for in vitro transcription. The products of RNA transcripts were confirmed by gel electrophoresis (Supplementary Figure 1) and quantified by NanoDrop (Thermo Fisher Scientific, Waltham, MA, United States). We equally mixed three dsRNA fragments and used for spray-induced gene silencing (SIGS) assay on polyethylene tape. The formation of pre-infection structures and expression levels of CHSs were quantified after dropping 1 × 105/ml of P. pachyrhizi spores containing 10 ng/ml dsRNA on polyethylene tape. Six hours after inoculation, pre-infection structures were observed with a Nikon ECLIPSE 80i phase contrast microscope.
Quantitative RT-PCR Analyses
For urediniospores attachment assay, 4-week-old soybean leaves covered with or without 0.1% CNF were spray-inoculated with P. pachyrhizi 1 × 105 spores/ml. The inoculated leaves were immediately fixed, and total RNA was extracted from the leaf areas and purified using RNAiso Plus (TaKaRa). To investigate the SIGS efficacy, expression levels of CHSs were quantified after dropping 1 × 105/ml of P. pachyrhizi spores containing 10 ng/ml dsRNA on polyethylene tape. Six hours after inoculation, total RNA was purified using RNAiso Plus. To investigate the gene expression profiles of P. pachyrhizi CHSs during infection, 4-week-old soybean leaves were spray-inoculated with P. pachyrhizi 1 × 105 spores/ml and incubated in darkness overnight, and then transferred to a growth chamber (22/20°C with a 16-h-light/8-h-dark cycle). At 2, 4, 6, 12, and 24 h after inoculation, total RNA was extracted from the inoculated leaf areas and purified using RNAiso Plus. For gene expression profiles of P. pachyrhizi CHSs and soybean defense-related genes, 4-week-old soybean leaves covered with or without 0.1% CNF were spray-inoculated with P. pachyrhizi 1 × 105 spores/ml and incubated in darkness overnight, and then transferred to a growth chamber (22/20°C with a 16-h-light/8-h-dark cycle). At 6, 12, and 24 h after inoculation, total RNA was extracted from the inoculated leaf areas and purified using RNAiso Plus according to the manufacture’s protocol.
Two micrograms of total RNA were treated with gDNA Remover (TOYOBO, Osaka, Japan) to eliminate genomic DNA, and the DNase-treated RNA was reverse transcribed using the ReverTra Ace qPCR RT Master Mix (TOYOBO). The cDNA (1:10) was then used for quantitative RT-PCR using the primers shown in Supplementary Table 1 with THUNDERBIRD SYBR qPCR Mix (TOYOBO) on a Thermal Cycler Dice Real Time System (TaKaRa). P. pachyrhizi ubiquitin 5 (PpUBQ5) and soybean ubiquitin 3 (GmUBQ3) were used to compare urediniospores attachment on soybean leaves. P. pachyrhizi elongation factor 1α (PpEF1α) and PpUBQ5 were used to normalize P. pachyrhizi gene expression. Soybean GmEF1α and GmUBQ3 were used as internal controls to normalize soybean gene expression.
Results
Covering Soybean Leaves With CNF Confers Resistance Against P. pachyrhizi
To investigate the potential application of CNF in agriculture, especially disease resistance against pathogens, we first treated soybean leaves with CNF. Four hours after spraying with 0.1% CNF, we challenged soybean leaves with P. pachyrhizi and observed lesion formation including uredinia at 10 days after inoculation. CNF-treated leaves showed reduced lesion area compared to control leaves (Figure 1A). CNF-treated leaves showed significantly reduced lesion number compared to control leaves (Figure 1B). These results indicate that covering soybean leaves with CNF confers resistance against P. pachyrhizi. Next, we investigated urediniospores attachment on control and CNF-treated leaves by quantifying the relative ratio of ubiquitin gene transcripts in soybean and P. pachyrhizi. As shown in Figure 1C, we found no significant difference in the relative ratio of ubiquitin transcripts between control and CNF-treated leaves, indicating that urediniospores were uniformly sprayed on control and CNF-treated leaves.
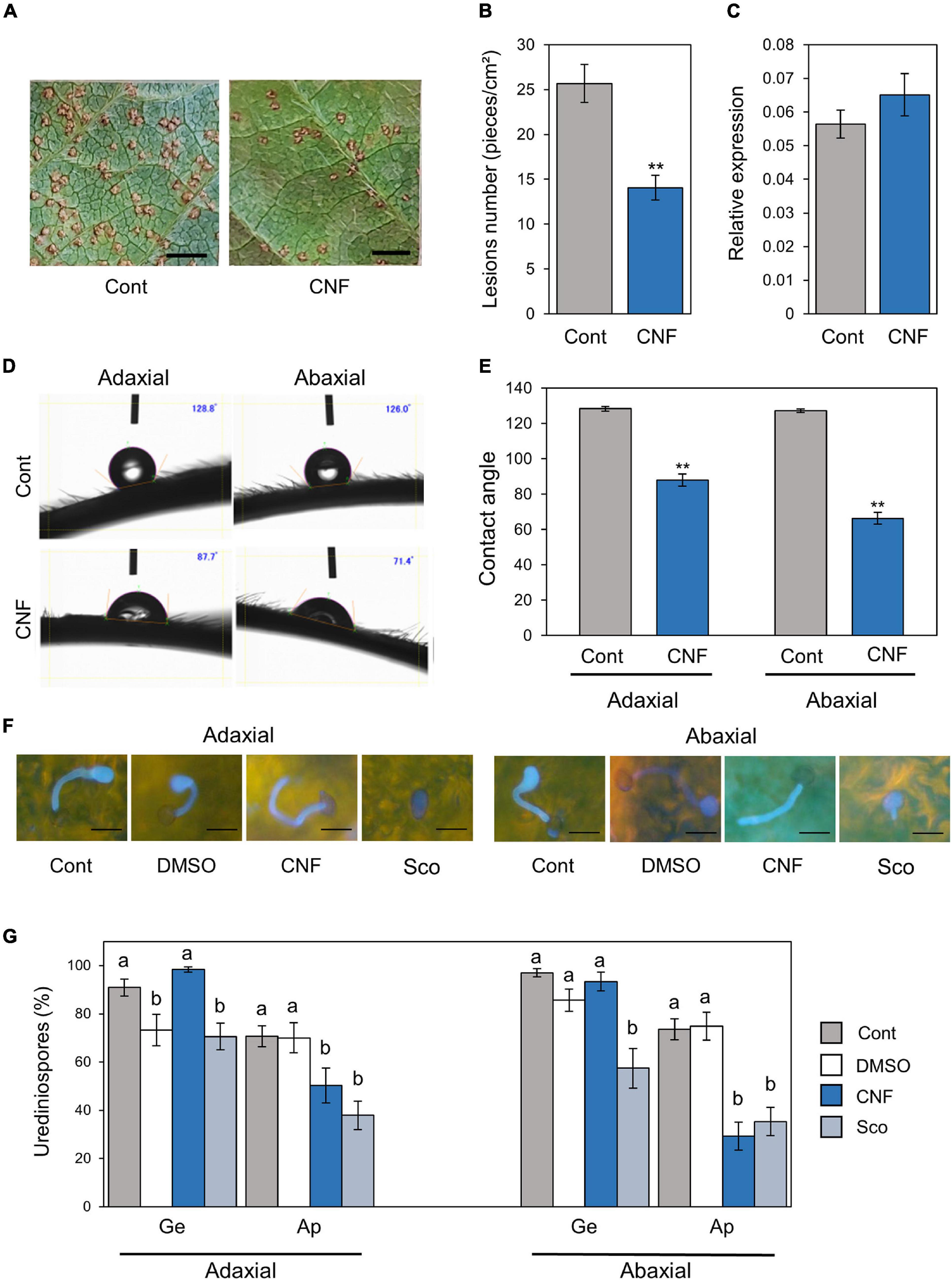
Figure 1. Phakopsora pachyrhizi lesion formation, pre-infection structures formation, and hydrophobicity on CNF-treated soybean leaves. Disease lesions (A) and lesion numbers (B) resulting from P. pachyrhizi infection on the abaxial leaf surface of control, and leaves covered with 0.1% cellulose nanofiber (CNF). Soybean plants were spray-inoculated with P. pachyrhizi (1 × 105 spores/ml). Photographs were taken 10 days after inoculation. Bars indicate 0.2 cm. Lesion numbers were counted to calculate lesion number per cm2. Vertical bars indicate the standard error of the means (n = 54). Asterisks indicate a significant difference between control and CNF-treatments in a t-test (**p < 0.01). (C) Urediniospore attachment quantification on the leaf surface of control and leaves covered with 0.1% CNF derived. Soybean plants were spray-inoculated with P. pachyrhizi (1 × 105 spores/ml) and immediately total RNAs including soybean and P. pachyrhizi were purified. Relative expression of soybean ubiquitin 3 (GmUBQ3) and P. pachyrhizi ubiquitin 5 (PpUBQ5) were evaluated using RT-qPCR. Vertical bars indicate the standard error of the means (n = 4). Droplet profiles (D) and quantification of contact angles (E) on the adaxial and abaxial leaf surface of control, and leaves covered with 0.1% CNF derived. Contact angles were evaluated as described in section “Materials and Methods”. Vertical bars indicate the standard error of the means (n = 60). Asterisks indicate a significant difference between control and CNF-treatments in a t-test (**p < 0.01). P. pachyrhizi pre-infection structure formation (F) and percentage of urediniospores (G) on the adaxial and abaxial surfaces of control, and leaves covered with 0.1% CNF, treated with 0.1% DMSO and 500 mM scopoletin (Sco). Soybean plants were spray-inoculated with P. pachyrhizi (1 × 105 spores/ml). The pre-infection structures were stained with Calcofluor White and photographs were taken 6 h after inoculation. Bars indicate 50 μm. The percentage of germinated (Ge) urediniospores and differentiated germ-tubes with appressoria (Ap) were evaluated as described in section “Materials and Methods.” Vertical bars indicate the standard error of the means (n = 21). Significant differences (p < 0.05) are indicated by different letters based on a Tukey’s honestly significant difference (HSD) test.
CNF Converts Leaf Surface Properties From Hydrophobic to Hydrophilic
Cellulose nanofiber has amphipathic properties, and thus can convert material surface properties from hydrophobic to hydrophilic, and vice versa (Kose et al., 2011). To confirm whether CNF-treatment can convert soybean leaf surface properties from hydrophobic to hydrophilic, we quantified the differences in surface hydrophobicity by measuring the contact angle at the interface of a liquid (water) drop with the leaf surface. A greater contact angle (>90°) is indicative of poor wetting or hydrophobicity. Interestingly, significant differences in the contact angle were observed between control and CNF-treated adaxial leaf surfaces (Figures 1D,E). The adaxial leaf surface of control leaves exhibited an average contact angle of 128°, whereas CNF-treated leaves showed a dramatic decrease in the contact angle (around 90°), which is indicative of a hydrophilic surface (Figure 1E). Similarly, significant differences in the contact angle were observed between control and CNF-treated abaxial leaf surfaces (Figures 1D,E). The abaxial leaf surface of control leaves exhibited an average contact angle of 127°, whereas CNF-treated leaves showed a dramatic decrease in contact angle (around 70°; Figure 1E). These results clearly indicate that CNF-treatments can convert leaf surface properties from hydrophobic to hydrophilic.
Covering Soybean Leaves With CNF Suppresses Formation of P. pachyrhizi Pre-infection Structures
Since CNF-treatments suppressed the lesion number, we next investigated the formation of pre-infection structures including germ-tubes and appressoria on CNF-treated leaves. In control leaves, around 90% of urediniospores germinated, and ∼75% formed appressoria on adaxial and abaxial leaves (Figures 1F,G). In CNF-treated leaves, around 90% of urediniospores germinated, and interestingly ∼50 and ∼30% of them formed appressoria on adaxial and abaxial leaves, respectively (Figures 1F,G). Scopoletin is known to protect soybean from soybean rust by suppressing the formation of pre-infection structures (Beyer et al., 2019). Thus, we also investigated the scopoletin application effect. Consistent with a previous study, in scopoletin-treated leaves, ∼70 and ∼60% of urediniospores germinated, and ∼40 and ∼30% of them formed appressoria on adaxial and abaxial leaves, respectively (Figures 1F,G). These results suggest that covering soybean leaves with CNF suppresses formation of pre-infection structures, including germ-tubes and appressoria.
Hydrophobicity With CNF Suppresses Formation of P. pachyrhizi Pre-infection Structures
Since CNF-treatments converted leaf surface properties from hydrophobic to hydrophilic, and suppressed the formation of pre-infection structures, we next investigated the effect of CNF treatment on hydrophobic polyethylene tape. The hydrophilic borosilicate glass slide exhibited an average contact angle of 16.8°, whereas the hydrophobic polyethylene tape showed an average contact angle of 115.1° (Figures 2A,B). Interestingly, CNF-treated polyethylene tape showed a dramatic decrease in contact angle (around 75°), which is indicative of a hydrophilic surface (Figures 2A,B). On control polyethylene tape, around 90% of urediniospores germinated, and ∼50% formed appressoria on hydrophobic surfaces (Figure 2C). On CNF-treated polyethylene tape, around 90% of urediniospores germinated, and interestingly ∼20% of them formed appressoria (Figure 2C). We also investigated the scopoletin application effect, since scopoletin is known to suppress the formation of pre-infection structures (Beyer et al., 2019). Scopoletin suppressed urediniospore germination (Figure 2C). These results suggest that covering hydrophobic surfaces with CNF suppresses formation of appressoria, which resulted from conversion of surface properties from hydrophobic to hydrophilic.
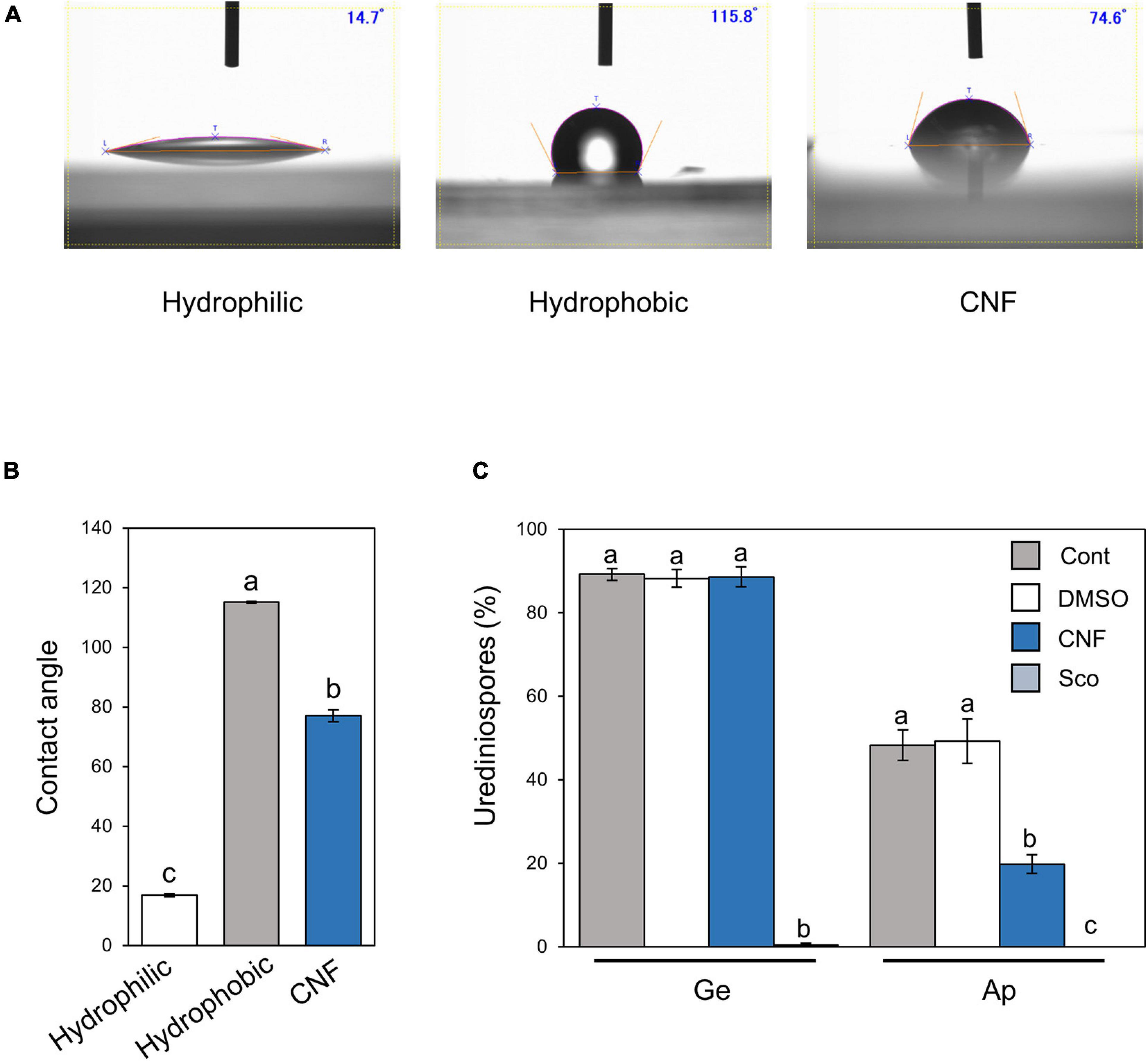
Figure 2. Phakopsora pachyrhizi pre-infection structures formation and hydrophobicity on polyethylene surfaces covered with CNF. Droplet profiles (A) and quantification of contact angles (B) on borosilicate glass slide (hydrophilic), and polyethylene tape (hydrophobic) covered with or without 0.1% cellulose nanofiber (CNF). Contact angles were evaluated as described in section “Materials and Methods.” Significant differences (p < 0.05) are indicated by different letters based on a Tukey’s honestly significant difference (HSD) test. (C) Percentage of urediniospores on polyethylene tape covered with or without 0.1% CNF, treated with 0.1% DMSO and 500 mM scopoletin (Sco). Polyethylene tapes were spray-inoculated with P. pachyrhizi (1 × 105 spores/ml). The photographs were taken 6 h after inoculation and the percentage of germinated (Ge) urediniospores and differentiated germ-tubes with appressoria (Ap) were evaluated as described in section “Materials and Methods.” Vertical bars indicate the standard error of the means (n = 19 ∼ 28). Significant differences (p < 0.05) are indicated by different letters based on a Tukey’s honestly significant difference (HSD) test.
Phakopsora pachyrhizi Chitin Synthases Are Required for Formation of Pre-infection Structures
Ishiga et al. (2013) reported that gene expression related to formation of pre-infection structures was induced on the hydrophobic surface based on P. pachyrhizi transcriptome analysis. CHSs are key enzymes in the biosynthesis of the fungal cell wall structural component, chitin. Since Ishiga et al. (2013) demonstrated that P. pachyrhizi CHS expression was induced on the hydrophobic leaf surface, we next tested the expression profiles of P. pachyrhizi CHS genes in soybean leaves. Except for CHS2-1 and CHS3-3, all CHS gene transcripts were significantly induced within 2 h after soybean leaf inoculation (Figure 3A and Supplementary Figure 2), suggesting CHSs may be involved in the formation of pre-infection structures, including germ-tubes and appressoria.
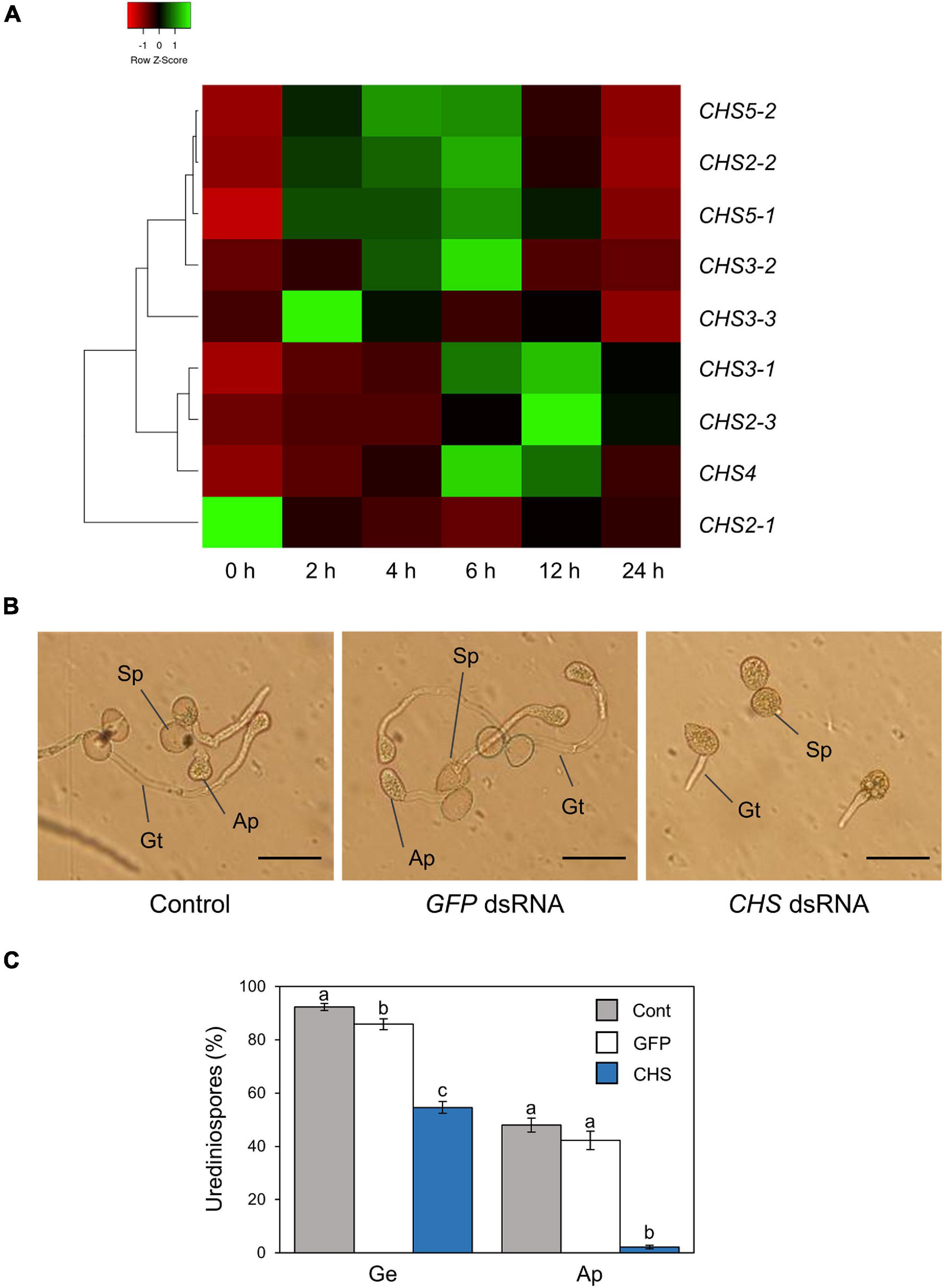
Figure 3. Gene expression profiles and functional analysis of P. pachyrhizi chitin synthase genes. (A) The heatmap created from gene expression profiles of P. pachyrhizi chitin synthases, including CHS2-1, CHS2-2, CHS2-3, CHS3-1, CHS3-2, CHS3-3, CHS4, CHS5-1, and CHS5-2 on soybean leaves. Soybean plants were spray-inoculated with P. pachyrhizi (1 × 105 spores/ml). Total RNAs including soybean and P. pachyrhizi was purified at 0, 2, 4, 6, 12, and 24 h after inoculation, and expression profiles were evaluated using RT-qPCR. P. pachyrhizi elongation factor and ubiquitin 5 were used to normalize the samples. Expression profiles were visualized as a heatmap using Heatmapper (Babicki et al., 2016). P. pachyrhizi pre-infection structure formation (B) and percentage of urediniospores (C) on polyethylene tapes treated with GFP double-stranded RNA (dsRNA) and chitin synthase (CHS) dsRNA. Polyethylene tapes were spray-inoculated with P. pachyrhizi (1 × 105 spores/ml). The photographs were taken 6 h after inoculation. Bars indicate 50 μm. The percentage of germinated (Ge) urediniospores and differentiated germ-tubes with appressoria (Ap) were evaluated as described in section “Materials and Methods.” Vertical bars indicate the standard error of the means (n = 46 ∼ 47). Significant differences (p < 0.05) are indicated by different letters based on a Tukey’s honestly significant difference (HSD) test.
To investigate P. pachyrhizi CHSs function on pre-infection structures formation, we performed RNA-SIGS targeting CHS genes. We designed dsRNA to target all CHS genes, and checked these gene expression levels on a hydrophobic polyethylene surface with or without CHS dsRNA for 6 h. As expected, all CHS transcripts were significantly suppressed by treatment with CHS dsRNA (Supplementary Figure 3). We next investigated the effect of CHS dsRNA on pre-infection structures formation. On control polyethylene tape with GFP dsRNA treatment, around 90% of urediniospores germinated, and ∼50% of them formed appressoria on the hydrophobic surface (Figures 3B,C). Interestingly, with CHS dsRNA treatment, around ∼60% of urediniospores germinated, and less than 5% of them formed appressoria (Figures 3B,C). These results clearly indicate that P. pachyrhizi CHSs are required for formation of pre-infection structures, including germ-tubes and appressoria.
Soybean Defense-Related Gene Expression Analysis
Nanofibers such as chitin nanofibers induce plant immune responses by activating defense-related gene expression (Egusa et al., 2015). Therefore, one could argue that the CNF-induced resistance phenotype in soybean plants may result from defense response activation, rather than from the direct effects of CNF treatments against P. pachyrhizi. To rule out this possibility, we investigated the expression profiles of the defense marker PR genes and defense-related genes, including phenylpropanoid and isoflavonoid pathways leading to phytoalexin production. Except for chalcone reductase (CHR) and isoflavone reductase (IFR), all defense marker PR genes and defense-related genes were clearly induced within 6 h of P. pachyrhizi inoculation, and these transcripts reached high levels at 12 h (Figure 4 and Supplementary Figure 4). Interestingly, the transcript levels of defense marker PR genes and defense-related genes were significantly less at 6 h on CNF-treated soybean leaves compared to control leaves (Figure 4 and Supplementary Figure 4), suggesting that CNF treatment does not induce PR and defense-related genes. These results confirmed that the resistance phenotype against P. pachyrhizi on CNF-treated soybean leaves is a direct effect of CNF treatment.
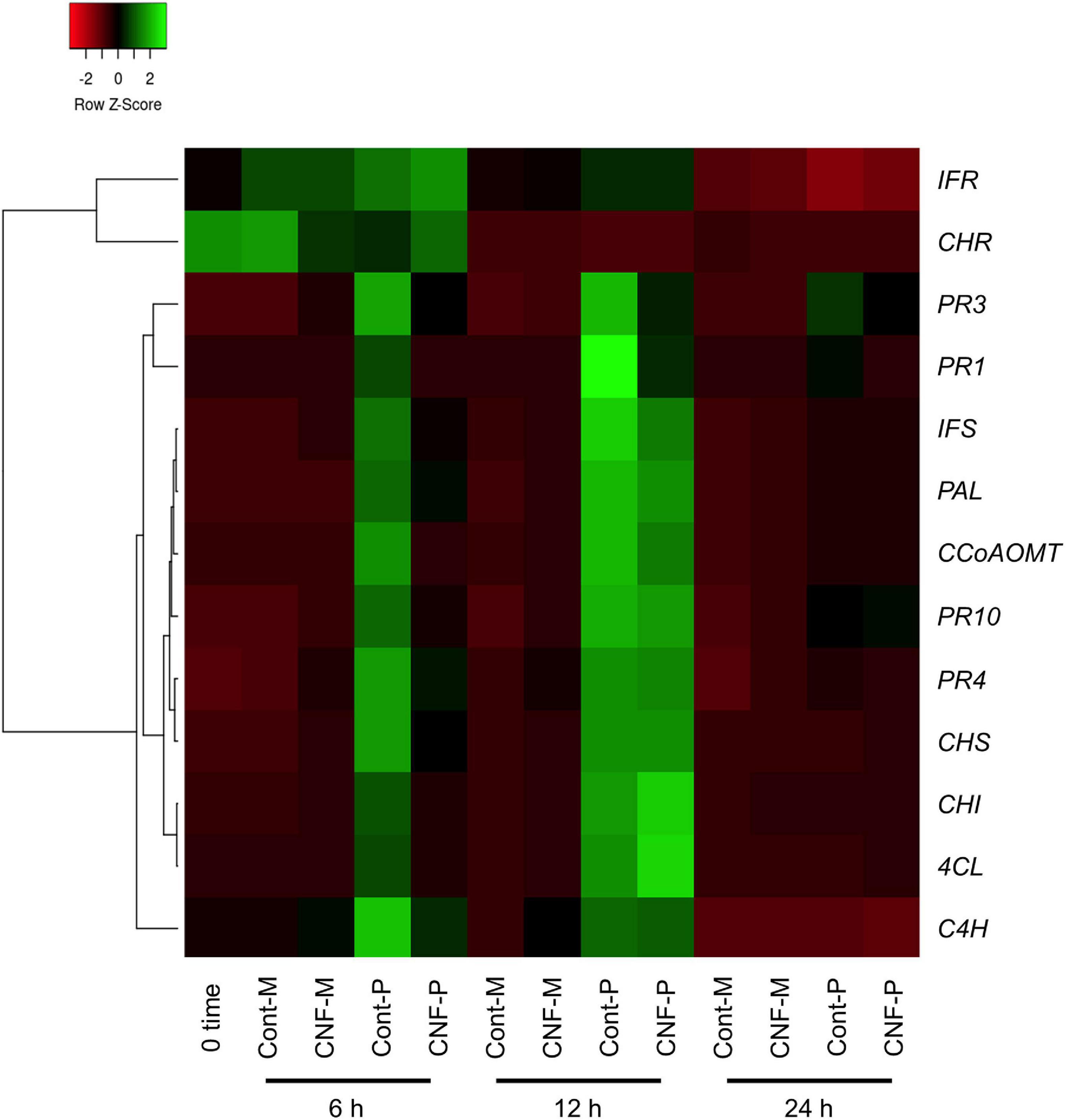
Figure 4. Gene expression profiles of soybean defense marker PR and defense-related genes in response to P. pachyrhizi inoculation on CNF-treated leaves. The heatmap was created from gene expression profiles of soybean defense marker PR and defense-related genes including pathogenesis-related protein 1 (PR1), 2 (PR2), 3 (PR3), 4 (PR4), 10 (PR10), phenylalanine ammonia-lyase (PAL), cinnamate 4-hydroxylase (C4H), 4-coumarate CoA ligase (4CL), caffeoyl coenzyme A O-methyltransferase (CCoAOMT), chalcone synthase (CHS), chalcone reductase (CHR), chalcone isomerase (CHI), isoflavone synthase (IFS), and isoflavone reductase (IFR) in response to P. pachyrhizi inoculation on CNF-treated leaves. Soybean plants were spray-inoculated with P. pachyrhizi (1 × 105 spores/ml). Total RNAs including soybean and P. pachyrhizi were purified at 6, 12, and 24 h after inoculation and expression profiles were evaluated using RT-qPCR. Soybean elongation factor 1α (GmEF1α) and ubiquitin 3 (GmUBQ3) were used to normalize the samples. Expression profiles were visualized as a heatmap using Heatmapper (Babicki et al., 2016). In heatmap, P and M indicate the treatments with or without pachyrhizi inoculation, respectively.
Covering Soybean Leaves With CNF Changes Gene Expression Profiles Related to Formation of Pre-infection Structures
Phakopsora pachyrhizi CHSs are required for formation of pre-infection structures (Figure 3). We next investigated gene expression profiles of CHSs in control and CNF-treated leaves at 6, 12, and 24 h after P. pachyrhizi inoculation. Except for CHS2-1 and CHS3-3, all CHSs gene transcripts were clearly induced within 6 h in control soybean leaves (Figure 5). However, the expression of these genes was clearly suppressed in CNF-treated leaves (Figure 5), indicating that covering soybean leaves with CNF changes gene expression profiles of CHSs. Together, these results suggest that CNF-treatments suppress the expression of CHSs, resulting in reduced chitin biosynthesis activity in the P. pachyrhizi cell wall.
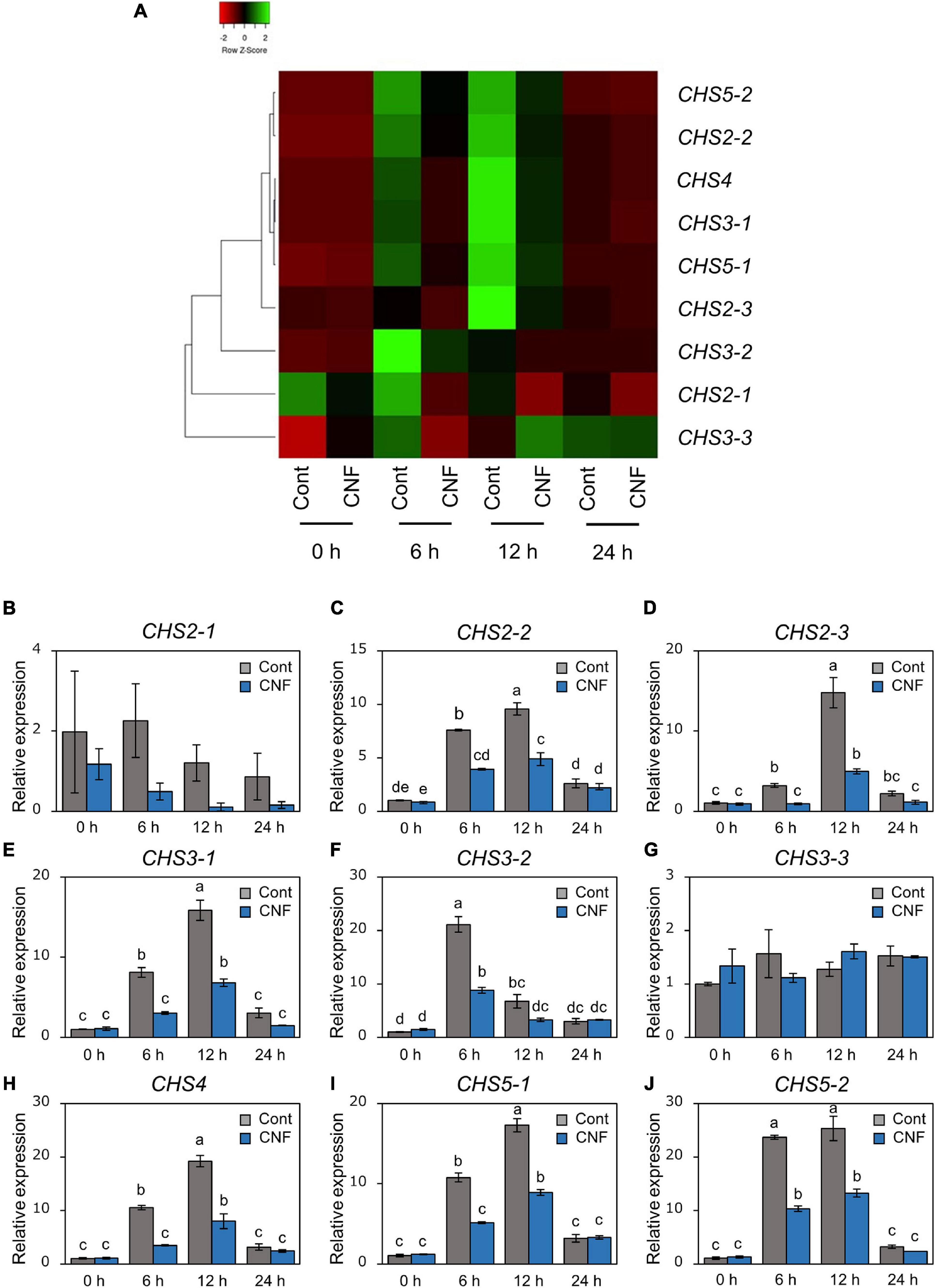
Figure 5. Gene expression profiles of P. pachyrhizi chitin synthase genes on CNF-treated soybean leaves. (A) The heatmap was created from gene expression profiles of P. pachyrhizi chitin synthases, including CHS2-1, CHS2-2, CHS2-3, CHS3-1, CHS3-2, CHS3-3, CHS4, CHS5-1, and CHS5-2 on soybean leaves covered with or without 0.1% CNF. Soybean plants were spray-inoculated with P. pachyrhizi (1 × 105 spores/ml). Total RNAs including soybean and P. pachyrhizi were purified at 0, 6, 12, and 24 h after inoculation, and expression profiles were evaluated using RT-qPCR. P. pachyrhizi elongation factor 1α (PpEF1α) and ubiquitin 5 (PpUBQ5) were used to normalize the samples. Expression profiles were visualized as a heatmap using Heatmapper (Babicki et al., 2016). Gene expression profiles of P. pachyrhizi chitin synthases, including CHS2-1 (B), CHS2-2 (C), CHS2-3 (D), CHS3-1 (E), CHS3-2 (F), CHS3-3 (G), CHS4 (H), CHS5-1 (I), and CHS5-2 (J) on soybean leaves covered with or without 0.1% cellulose nanofiber (CNF). Vertical bars indicate the standard error of the means (n = 4). Significant differences (p < 0.05) are indicated by different letters based on a Tukey’s honestly significant difference (HSD) test.
Discussion
We investigated the potential application of CNF in agriculture, especially disease protection, and found that CNF-treated soybean leaves conferred resistance against the rust pathogen P. pachyrhizi (Figures 1A,B). CNF-treatments convert soybean leaf surface properties from hydrophobic to hydrophilic (Figures 1D,E), resulting in suppression of P. pachyrhizi CHSs genes involved in the formation of pre-infection structures, including germ-tubes and appressoria (Figure 5) associated with reduced appressoria formation (Figures 1F,G). These results provide new insights into CNF application on P. pachyrhizi disease management strategies.
Cellulose nanofiber-treatments conferred soybean resistance against P. pachyrhizi associated with reduced lesion formation (Figures 1A,B). The application of chitin nanofibers for plant protection against pathogens has been investigated. Egusa et al. (2015) reported that chitin nanofibers effectively reduced fungal and bacterial pathogen infections in Arabidopsis thaliana by activating plant defense responses, including reactive oxygen species (ROS) production and defense-related gene expression. Furthermore, chitin nanofiber treatment can reduce the occurrence of Fusarium wilt disease in tomato plants (Egusa et al., 2019). These results suggest that chitin nanofibers activate plant immunity, resulting in reduced pathogen infection. However, we showed no CNF elicitor activity based on defense gene expression profiles (Figure 4 and Supplementary Figure 4). Although there is no similarity to the mechanism by which nanofibers, including cellulose and chitin, function to protect plants against pathogens, both nanofibers will be able to provide eco-friendly disease control strategies in sustainable agriculture.
Formation of appressoria was significantly suppressed in CNF-treated leaves compared to control leaves (Figures 1F,G). Consistent with our results, Uppalapati et al. (2012) reported the reduced formation of pre-infection structures on a M. truncatula irg1 mutant, in which the epicuticular waxes were completely defective and the surface property was changed to hydrophilic. These results indicate that properties such as hydrophobicity are important to form P. pachyrhizi pre-infection structures during early infection stages. The importance of hydrophobicity and/or epicuticular waxes on the formation of germ-tubes and appressoria has also been reported for other fungal pathogens (Mendoza-Mendoza et al., 2009; Hansjakob et al., 2010; Weidenbach et al., 2014). Further characterization of the mechanisms by which fungal pathogens recognize plant surface properties and initiate infection behavior will be needed to develop effective and sustainable disease control methods.
We demonstrated that ASR was suppressed by CNF made from the ACC method (Figures 1A,B). Various preparation methods have been proposed including mechanical and chemical processes (Tsuji et al., 2021). CNF made from the ACC method has both hydrophobic and hydrophilic sites resulting in amphiphilic properties (Kondo et al., 2014). Halim et al. (2020) demonstrated that CNF made from the ACC method was more hydrophilic than that made from the chemical method based on the contact angle measurements. Therefore, it is necessary to investigate whether the same protective effect is observed not only for the CNF made from the ACC method but also for the CNF treatment made from other methods.
Cellulose nanofiber-treatments suppressed P. pachyrhizi CHSs expression related to chitin formation, which are associated with reduced formation of pre-infection structures (Figures 1F,G, 5). CHSs are important in cell wall formation in most filamentous fungi (Takeshita et al., 2005; Lenardon et al., 2010). Treitschke et al. (2010) reported that an Ustilago maydis CHS5 mutant Δmsc1 showed reduced virulence associated with abnormal hyphal morphology. Madrid et al. (2003) also demonstrated that CHS5 in Fusarium oxysporum, a causal agent of tomato vascular wilt, has a crucial role in virulence and mediates the tomato protective response. A F. oxysporum CHS5 mutant could not infect tomato, and exhibited abnormal morphologies such as hyphal swelling, due to changes in the cell wall properties (Madrid et al., 2003). These results suggest that CHS5 gene deficiency or mutation causes morphological abnormalities in fungal cell wall formation, leading to virulence suppression. Together, it is tempting to speculate that suppression of P. pachyrhizi CHS5 in CNF-treated leaves may result in changes in the cell wall properties of P. pachyrhizi pre-infection structures. Further characterization of CHSs, especially CHS5 based on dsRNA-mediated silencing such as SIGS and host-induced gene silencing (HIGS), in conjunction with analysis of P. pachyrhizi cell wall properties on CNF-treated leaves, will be necessary to understand CHSs molecular function during formation of pre-infection structures.
We demonstrated that CNF-treatments suppressed ASR caused by P. pachyrhizi, one of the most important soybean diseases (Figures 1A,B) associated with reduced formation of pre-infection structures (Figures 1F,G). Because numerous rust and filamentous fungal pathogens form pre-infection structures during early infection stages, these results imply that CNF might be an additional disease management tool to prevent crop diseases against these pathogens. However, we tested the ability of CNF to protect plants against an obligate biotrophic pathogen, but not other pathogen types, including hemibiotrophs and necrotrophs. Therefore, further characterization of CNF effects on disease suppression not only against fungal pathogens, but also against bacterial pathogens will be needed.
Our results demonstrated that SIGS targeting P. pachyrhizi CHSs functioned successfully in reducing pre-infection structures formation on hydrophobic polyethylene surfaces (Figures 3B,C and Supplementary Figure 3). SIGS is a technology that promotes silencing by spraying the target dsRNA on the plant surface. Therefore, it is possible to silence a specific phytopathogen gene and protect the plant without the need for plant gene recombination (Cagliari et al., 2019; Wytinck et al., 2020). Hu et al. (2020) demonstrated that SIGS targeting P. pachyrhizi genes encoding an acetyl-CoA acyltransferase, a 40S ribosomal protein S16, and glycine cleavage system H protein reduced pustule numbers over 70%. SIGS against filamentous fungi threating agronomically important crops has also been studied, including head blight caused by Fusarium graminearum and gray mold caused by Botrytis cinerea (Koch et al., 2016; Wang et al., 2016; Nerva et al., 2020). Koch et al. (2016) demonstrated that SIGS targeting F. graminearum cytochrome P450 genes, which are required for fungal ergosterol biosynthesis, successfully inhibited fungal growth in barley. Although further precise studies for SIGS targeting P. pachyrhizi virulence genes will be needed, SIGS is a powerful tool to develop sustainable disease management strategies.
Expression profiles of soybean leaves revealed that gene transcripts related to the phenylpropanoid and isoflavonoid pathways were upregulated within 6 h of P. pachyrhizi inoculation, and reached high levels at 12 h (Figure 4 and Supplementary Figure 4). Consistent with our results, previous studies reported that the expression of these genes was upregulated within 12 h after P. pachyrhizi inoculation (Schneider et al., 2011; Ishiga et al., 2015; Hossain et al., 2018). We demonstrated that the transcript levels of defense marker PR genes and defense-related genes were significantly less at 6 h in CNF-treated soybean leaves compared to control leaves (Figure 4 and Supplementary Figure 4). Further, appressoria formation was significantly reduced in CNF-treated leaves compared to controls (Figures 1F,G). Therefore, it is tempting to speculate that reduced transcripts of defense marker PR genes and defense-related genes in CNF-treated leaves is the result of the decreased penetration rate associated with reduced appressoria formation. However, since the transcripts of defense marker PR genes and defense-related genes were suppressed even though P. pachyrhizi infection was not completely prevented in CNF-treated soybean leaves, further investigation of CNF effects on plant defense responses will be necessary.
In summary, CNF-treatments confer resistance against P. pachyrhizi, a causal agent of ASR. Moreover, CNF-treatments can change leaf surface hydrophobicity, resulting in CHSs gene suppression related to CHS, which is associated with reduced formation of pre-infection structures including P. pachyrhizi germ-tubes and appressoria (Figure 6). Since CNF is an abundant and renewable biomass in nature, CNF application for plant protection will provide a new avenue into eco-friendly and sustainable disease management.
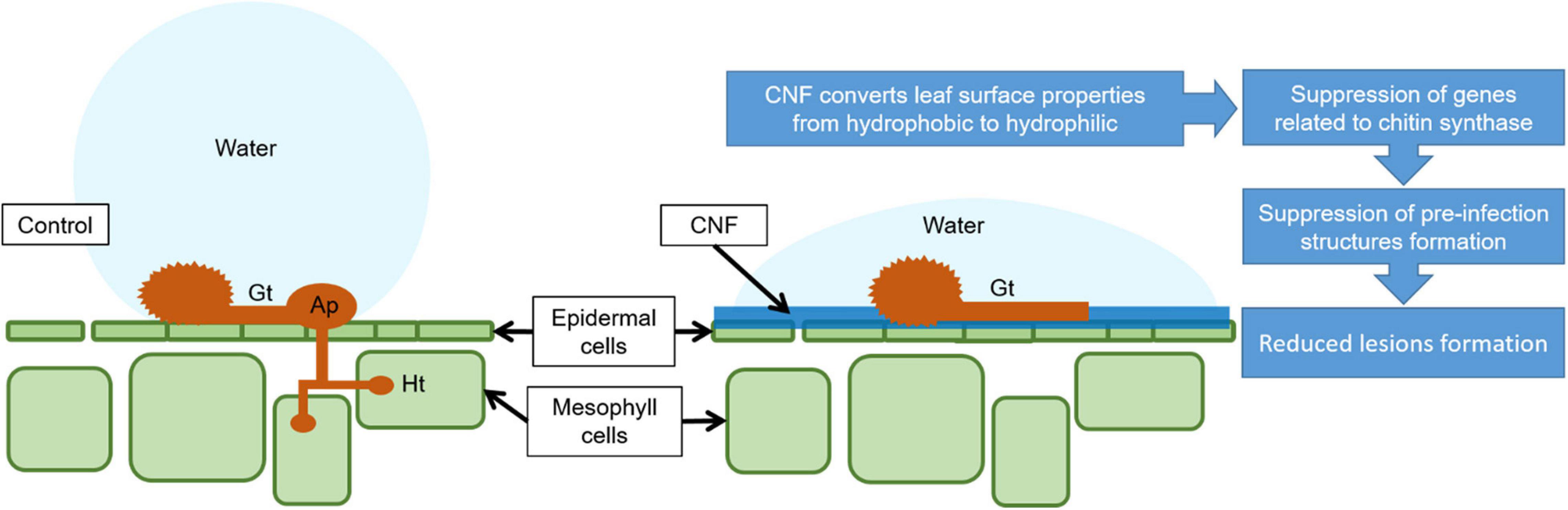
Figure 6. Proposed mechanism model by which CNF-treatments confer resistance against P. pachyrhizi. CNF-treatments convert leaf surface properties from hydrophobic to hydrophilic. The formation of pre-infection structures, and the associated gene expressions related to these formations are suppressed on CNF-treated leaves, resulting in reduced P. pachyrhizi infection. Gt, Ap, and Ht show germ-tubes, appressoria, and haustoria, respectively.
Data Availability Statement
The original contributions presented in the study are included in the article/Supplementary Material, further inquiries can be directed to the corresponding author.
Author Contributions
HS, NSa, and YI contributed to conception and design of the study and performed the statistical analysis. HS, YY, NSa, TI, NSh, GU, VN, EY, and YI performed the experiments. HS wrote the first draft of the manuscript. NSa, YY, and YI wrote sections of the manuscript. All authors contributed to manuscript revision, read, and approved the submitted version.
Funding
This work was supported in part by the JST ERATO NOMURA Microbial Community Control Project, JST, Japan.
Conflict of Interest
The authors declare that the research was conducted in the absence of any commercial or financial relationships that could be construed as a potential conflict of interest.
Publisher’s Note
All claims expressed in this article are solely those of the authors and do not necessarily represent those of their affiliated organizations, or those of the publisher, the editors and the reviewers. Any product that may be evaluated in this article, or claim that may be made by its manufacturer, is not guaranteed or endorsed by the publisher.
Acknowledgments
We thank Christina Baker for editing the manuscript. Cellulose nanofiber (CNF, marketed as nanoforest®) was supplied through the courtesy of Chuetsu Pulp & Paper (Takaoka, Japan).
Supplementary Material
The Supplementary Material for this article can be found online at: https://www.frontiersin.org/articles/10.3389/fpls.2021.726565/full#supplementary-material
References
Abe, K., Iwamoto, S., and Yano, H. (2007). Obtaining cellulose nanofibers with a uniform width of 15 nm from wood. Biomacromolecules 8, 3276–3278. doi: 10.1021/bm700624p
Babicki, S., Arndt, D., Marcu, A., Liang, Y., Grant, J. R., Maciejewski, A., et al. (2016). Heatmapper: web-enabled heat mapping for all. Nucleic Acids Res. 44, W147–W153. doi: 10.1093/nar/gkw419
Beyer, S. F., Beesley, A., Rohmann, P. F. W., Schultheiss, H., Conrath, U., and Langenbach, C. J. G. (2019). The Arabidopsis non-host defense-associated coumarin scopoletin protects soybean from Asian soybean rust. Plant J. 99, 397–413. doi: 10.1111/tpj.14426
Bolton, M. D., Kolmer, J. A., and Garvin, D. F. (2008). Wheat leaf rust caused by Puccinia triticina. Mol. Plant Pathol. 9, 563–575. doi: 10.1111/j.1364-3703.2008.00487.x
Bromfield, K. R., and Hartwig, E. E. (1980). Resistance to soybean rust and mode of inheritance. Crop Sci. 20, 254–255. doi: 10.2135/CROPSCI1980.0011183X002000020026X
Cagliari, D., Dias, N. P., Galdeano, D. M., Dos Santos, E., Smagghe, G., and Zotti, M. J. (2019). Management of pest insects and plant diseases by non-transformative RNAi. Front. Plant Sci. 10:1319. doi: 10.3389/fpls.2019.01319
Egusa, M., Matsui, H., Urakami, T., Okuda, S., Ifuku, S., Nakagami, H., et al. (2015). Chitin nanofiber elucidates the elicitor activity of polymeric chitin in plants. Front. Plant Sci. 6:1098. doi: 10.3389/fpls.2015.01098
Egusa, M., Parada, R., Aklog, Y. F., Ifuku, S., and Kaminaka, H. (2019). Nanofibrillation enhances the protective effect of crab shells against Fusarium wilt disease in tomato. Int. J. Biol. Macromol. 128, 22–27. doi: 10.1016/j.ijbiomac.2019.01.088
Garcia, A., Calvo, E. S., de Souza Kiihl, R. A., Harada, A., Hiromoto, D. M., and Vieira, L. G. (2008). Molecular mapping of soybean rust (Phakopsora pachyrhizi) resistance genes: discovery of a novel locus and alleles. Theor. Appl. Genet. 117, 545–553. doi: 10.1007/s00122-008-0798-z
Godoy, C. V., Bueno, A. D. F., and Gazziero, D. L. P. (2015). Brazilian soybean pest management and threats to its sustainability. Outlooks on Pest Manage. 26:3.
Godoy, C. V., Seixas, C. D. S., Soares, R. M., Mercelino-Gumaraes, F. C., Meyer, M. C., and Costamilan, L. M. (2016). Asian soybean rust in Braizil: past, present and future. Pesquisa Agropecuària Brasileira. 51, 407–421.
Goellner, K., Loehrer, M., Langenbach, C., Conrath, U., Koch, E., and Schaffrath, U. (2010). Phakopsora pachyrhizi, the causal agent of Asian soybean rust. Mol. Plant Pathol. 11, 169–177. doi: 10.1111/j.1364-3703.2009.00589.x
Halim, A., Lin, K. H., and Enomae, T. (2020). Biomimicking properties of cellulose nanofiber under ethanol/water mixture. Sci. Rep. 10:21070. doi: 10.1038/s41598-020-78100-z
Hansjakob, A., Bischof, S., Bringmann, G., Riederer, M., and Hildebrandt, U. (2010). Very-long-chain aldehydes promote in vitro prepenetration processes of Blumeria graminis in a dose- and chain length-dependent manner. New Phytol. 188, 1039–1054. doi: 10.1111/j.1469-8137.2010.03419.x
Hartwig, E. E. (1986). Identification of a fourth major gene conferring resistance to soybean rust. Crop. Sci. 26, 1135–1136. doi: 10.2135/cropsci1986.0011183X002600060010x
Hossain, M. Z., Ishiga, Y., Yamanaka, N., Ogiso-Tanaka, E., and Yamaoka, Y. (2018). Soybean leaves transcriptomic data dissects the phenylpropanoid pathway genes as a defence response against Phakopsora pachyrhizi. Plant Physiol. Biochem. 132, 424–433. doi: 10.1016/j.plaphy.2018.09.020
Hu, D., Chen, Z. Y., Zhang, C., and Ganiger, M. (2020). Reduction of Phakopsora pachyrhizi infection on soybean through host- and spray-induced gene silencing. Mol. Plant Pathol. 21, 794–807. doi: 10.1111/mpp.12931
Ishiga, Y., Uppalapati, S. R., Gill, U. S., Huhman, D., Tang, Y., and Mysore, K. S. (2015). Transcriptomic and metabolomic analyses identify a role for chlorophyll catabolism and phytoalexin during Medicago nonhost resistance against Asian soybean rust. Sci. Rep. 5:13061. doi: 10.1038/srep13061
Ishiga, Y., Upplapapti, S., and Mysore, K. S. (2013). Expression analysis reveals a role for hydrophobic or epicuticular wax signals in pre-penetration structure formation of Phakopsora pachyrhizi. Plant Signal Behav. 8:e26959. doi: 10.4161/psb.26959
Kawashima, C. G., Guimarães, G. A., Nogueira, S. R., MacLean, D., Cook, D. R., Steuernagel, B., et al. (2016). A pigeonpea gene confers resistance to Asian soybean rust in soybean. Nat. Biotechnol. 34, 661–665. doi: 10.1038/nbt.3554
Klosowski, A. C., Castellar, C., Stammler, G., and May De Mio, L. L. (2018). Fungicide sensitivity and monocyclic parameters related to the Phakopsora pachyrhizi–soybean pathosystem from organic and conventional soybean production systems. Plant Pathol. 67, 1697–1705. doi: 10.1111/ppa.12883
Koch, A., Biedenkopf, D., Furch, A., Weber, L., Rossbach, O., Abdellatef, E., et al. (2016). An RNAi-based control of Fusarium graminearum infections through spraying of long dsRNAs involves a plant passage and is controlled by the fungal silencing machinery. PLoS Pathog. 12:e1005901. doi: 10.1371/journal.ppat.1005901
Kondo, T., Kose, R., Naito, H., and Kasai, W. (2014). Aqueous counter collision using paired water jets as a novel means of preparing bio-nanofibers. Carbohyd. Polym. 112, 284–290. doi: 10.1016/j.carbpol.2014.05.064
Kose, R., Kasai, W., and Kondo, T. (2011). Switching surface properties of substrates by coating with a cellulose nanofiber having a high adsorbability. Sen’i Gakkaishi 67, 163–168. doi: 10.2115/fiber.67.163
Langenbach, C., Campe, R., Beyer, S. F., Mueller, A. N., and Conrath, U. (2016). Figurehting Asian soybean rust. Front. Plant Sci. 7:797. doi: 10.3389/fpls.2016.00797
Lenardon, M. D., Munro, C. A., and Gow, N. A. (2010). Chitin synthesis and fungal pathogenesis. Curr. Opin. Microbiol. 13, 416–423. doi: 10.1016/j.mib.2010.05.002
Li, S., Smith, J. R., Ray, J. D., and Frederick, R. D. (2012). Identification of a new soybean rust resistance gene in PI 567102B. Theor. Appl. Genet. 125, 133–142. doi: 10.1007/s00122-012-1821-y
Madrid, M. P., Di Pietro, A., and Roncero, M. I. (2003). Class V chitin synthase determines pathogenesis in the vascular wilt fungus Fusarium oxysporum and mediates resistance to plant defence compounds. Mol. Microbiol. 47, 257–266. doi: 10.1046/j.1365-2958.2003.03299.x
Maltby, L., Brock, T. C., and Van den Brink, P. J. (2009). Fungicide risk assessment for aquatic ecosystems: importance of interspecific variation, toxic mode of action, and exposure regime. Environ. Sci. Technol. 43, 7556–7563. doi: 10.1021/es901461c
McLean, R., and Byth, D. (1980). Inheritance of resistance to rust Phakopsora pachyrhizi in soybeans. Aust. J. Agric. Res. 31, 951–956. doi: 10.1071/AR9800951
Mendoza-Mendoza, A., Berndt, P., Djamei, A., Weise, C., Linne, U., Marahiel, M., et al. (2009). Physical-chemical plant-derived signals induce differentiation in Ustilago maydis. Mol. Microbiol. 71, 895–911.
Mondal, S. (2017). Preparation, properties and applications of nanocellulosic materials. Carbohydr. Polym. 163, 301–316.
Monteros, M. J., Missaoui, A. M., Phillips, D. V., Walker, D. R., and Boerma, H. R. (2007). Mapping and confirmation of the ‘Hyuuga’ red-brown lesion resistance gene for Asian soybean rust. Crop Sci. 47, 829–834.
Nerva, L., Sandrini, M., Gambino, G., and Chitarra, W. (2020). Double-stranded RNAs (dsRNAs) as a sustainable tool against gray mold (Botrytis cinerea) in grapevine: effectiveness of different application methods in an open-air environment. Biomolecules 10:200.
Schneider, K. T., van de Mortel, M., Bancroft, T. J., Braun, E., Nettleton, D., Nelson, R. T., et al. (2011). Biphasic gene expression changes elicited by Phakopsora pachyrhizi in soybean correlate with fungal penetration and haustoria formation. Plant Physiol. 157, 355–371. doi: 10.1104/pp.111.181149
Slaminko, T. L., Miles, M. R., Frederick, R. D., Bonde, M. R., and Hartman, G. L. (2008). New legume hosts of Phakopsora pachyrhizi based on greenhouse evaluations. Plant Dis. 92, 767–771. doi: 10.1094/PDIS-92-5-0767
Takeshita, N., Ohta, A., and Horiuchi, H. (2005). CsmA, a class V chitin synthase with a myosin motor-like domain, is localized through direct interaction with the actin cytoskeleton in Aspergillus nidulans. Mol. Biol. Cell 16, 1961–1970. doi: 10.1091/mbc.e04-09-0761
Treitschke, S., Doehlemann, G., Schuster, M., and Steinberg, G. (2010). The myosin motor domain of fungal chitin synthase V is dispensable for vesicle motility but required for virulence of the maize pathogen Ustilago maydis. Plant Cell 22, 2476–2494. doi: 10.1105/tpc.110.075028
Tsuji, T., Tsuboi, K., Yokota, S., Tagawa, S., and Kondo, T. (2021). Characterization of an amphiphilic janus-type surface in the cellulose nanofibril prepared by aqueous counter collision. Biomacromolecules 22, 620–628.
Uppalapati, S. R., Ishiga, Y., Doraiswamy, V., Bedair, M., Mittal, S., Chen, J., et al. (2012). Loss of abaxial leaf epicuticular wax in Medicago truncatula irg1/palm1 mutants results in reduced spore differentiation of anthracnose and nonhost rust pathogens. Plant Cell 24, 353–370. doi: 10.1105/tpc.111.093104
Wang, M., Weiberg, A., Lin, F. M., Thomma, B. P., Huang, H. D., and Jin, H. (2016). Bidirectional cross-kingdom RNAi and fungal uptake of external RNAs confer plant protection. Nat. Plants 2:16151. doi: 10.1038/nplants.2016.151
Weidenbach, D., Jansen, M., Franke, R. B., Hensel, G., Weissgerber, W., Ulferts, S., et al. (2014). Evolutionary conserved function of barley and Arabidopsis 3-KETOACYL-CoA SYNTHASES in providing wax signals for germination of powdery mildew fungi. Plant Physiol. 166, 1621–1633. doi: 10.1104/pp.114.246348
Wytinck, N., Manchur, C. L., Li, V. H., Whyard, S., and Belmonte, M. F. (2020). dsRNA uptake in plant pests and pathogens: insights into RNAi-based insect and fungal control technology. Plants (Basel) 9:1780.
Keywords: chitin synthase, Asian soybean rust, cellulose nanofiber, hydrophobicity, Phakopsora pachyrhizi, pre-infection structure
Citation: Saito H, Yamashita Y, Sakata N, Ishiga T, Shiraishi N, Usuki G, Nguyen VT, Yamamura E and Ishiga Y (2021) Covering Soybean Leaves With Cellulose Nanofiber Changes Leaf Surface Hydrophobicity and Confers Resistance Against Phakopsora pachyrhizi. Front. Plant Sci. 12:726565. doi: 10.3389/fpls.2021.726565
Received: 17 June 2021; Accepted: 03 August 2021;
Published: 03 September 2021.
Edited by:
Wen-Ming Wang, Sichuan Agricultural University, ChinaReviewed by:
Hironori Kaminaka, Tottori University, JapanJing Shang, Sichuan Agricultural University, China
Copyright © 2021 Saito, Yamashita, Sakata, Ishiga, Shiraishi, Usuki, Nguyen, Yamamura and Ishiga. This is an open-access article distributed under the terms of the Creative Commons Attribution License (CC BY). The use, distribution or reproduction in other forums is permitted, provided the original author(s) and the copyright owner(s) are credited and that the original publication in this journal is cited, in accordance with accepted academic practice. No use, distribution or reproduction is permitted which does not comply with these terms.
*Correspondence: Yasuhiro Ishiga, ishiga.yasuhiro.km@u.tsukuba.ac.jp