- 1Departamento de Ciencias Ecológicas, Facultad de Ciencias, Universidad de Chile, Santiago, Chile
- 2Instituto de Ecología y Biodiversidad, Santiago, Chile
- 3Departamento de Ciencias Biológicas, Departamento de Ecología y Biodiversidad, Facultad de Ciencias de la Vida, Universidad Andrés Bello, Santiago, Chile
Andean uplift and the concomitant formation of the Diagonal Arid of South America is expected to have promoted species diversification through range expansions into this novel environment. We evaluate the evolution of Argylia, a genus belonging to the Bignoniaceae family whose oldest fossil record dates back to 49.4 Ma. Today, Argylia is distributed along the Andean Cordillera, from 15°S to 38.5°S and from sea level up to 4,000 m.a.s.l. We ask whether Argylia’s current distribution is a result of a range expansion along the Andes Cordillera (biological corridor) modulated by climatic niche conservatism, considering the timing of Andean uplift (30 Ma – 5 Ma). To answer this question, we reconstructed the phylogenetic relationships of Argylia species, estimated divergence times, estimated the realized climatic niche of the genus, reconstructed the ancestral climatic niche, evaluated its evolution, and finally, performed an ancestral range reconstruction. We found strong evidence for climatic niche conservatism for moisture variables, and an absence of niche conservatism for most of the temperature variables considered. Exceptions were temperature seasonality and winter temperature. Results imply that Argylia had the capacity to adapt to extreme temperature conditions associated with the Andean uplift and the new climatic corridor produced by uplift. Ancestral range reconstruction for the genus showed that Argylia first diversified in a region where subtropical conditions were already established, and that later episodes of diversification were coeval with the of Andean uplift. We detected a second climatic corridor along the coastal range of Chile-Peru, the coastal lomas, which allowed a northward range expansion of Argylia into the hyperarid Atacama Desert. Dating suggests the current distribution and diversity of Argylia would have been reached during the Late Neogene and Pleistocene.
Introduction
Southern South America exhibits a wide range of climates that results in an extraordinary diversity of biomes including forest, grassland, steppe, alpine, and desert (Ortega et al., 2012). The current distribution of these biomes reflects the interaction of climatic factors and historical processes at different time scales. These include past climate change, plate tectonics and mountain building.
One of the most defining features of South America biogeography is a large arid region with annual rainfall below 500 mm that crosses the western side of the continent diagonally from northwest to southeast, beginning at coastal equatorial latitudes (4°S) and culminating on the Atlantic coast at latitude 55°S. This region has been defined as the South American Arid Diagonal (SAD, Veit and Garleff, 1995; Villagrán and Hinojosa, 1997; Garreaud et al., 2010; Figure 1). SAD developed gradually during the Oligocene and Miocene as a consequence of the Andean uplift and the establishment of the current equatorial-polar temperature gradient (Hinojosa and Villagrán, 1997; Zachos et al., 2001; Dunai et al., 2005; Hinojosa, 2005). Arid conditions have been postulated for latitudes affected by the anticyclonic influence of the South America Subtropical High (24°S) in the absence of the Andes since the Late Jurassic (150 Ma, Hartley et al., 2005; Garreaud et al., 2010). The final uplift of the Andes during the Miocene defined the current pattern of rainfall distribution in the area through the rain shadow effect of the Andes blocking moist air brought by winds from the east in its northern extreme and conversely, from the west in its southern extreme. The coeval emergence of the Humboldt Current during the Neogene further intensified aridity leading to the development of the current hyperarid Atacama Desert on the Pacific coast, a region considered one of the most arid in the world (Hinojosa and Villagrán, 1997; Latorre et al., 1997; Hartley et al., 2005; Garreaud et al., 2010).
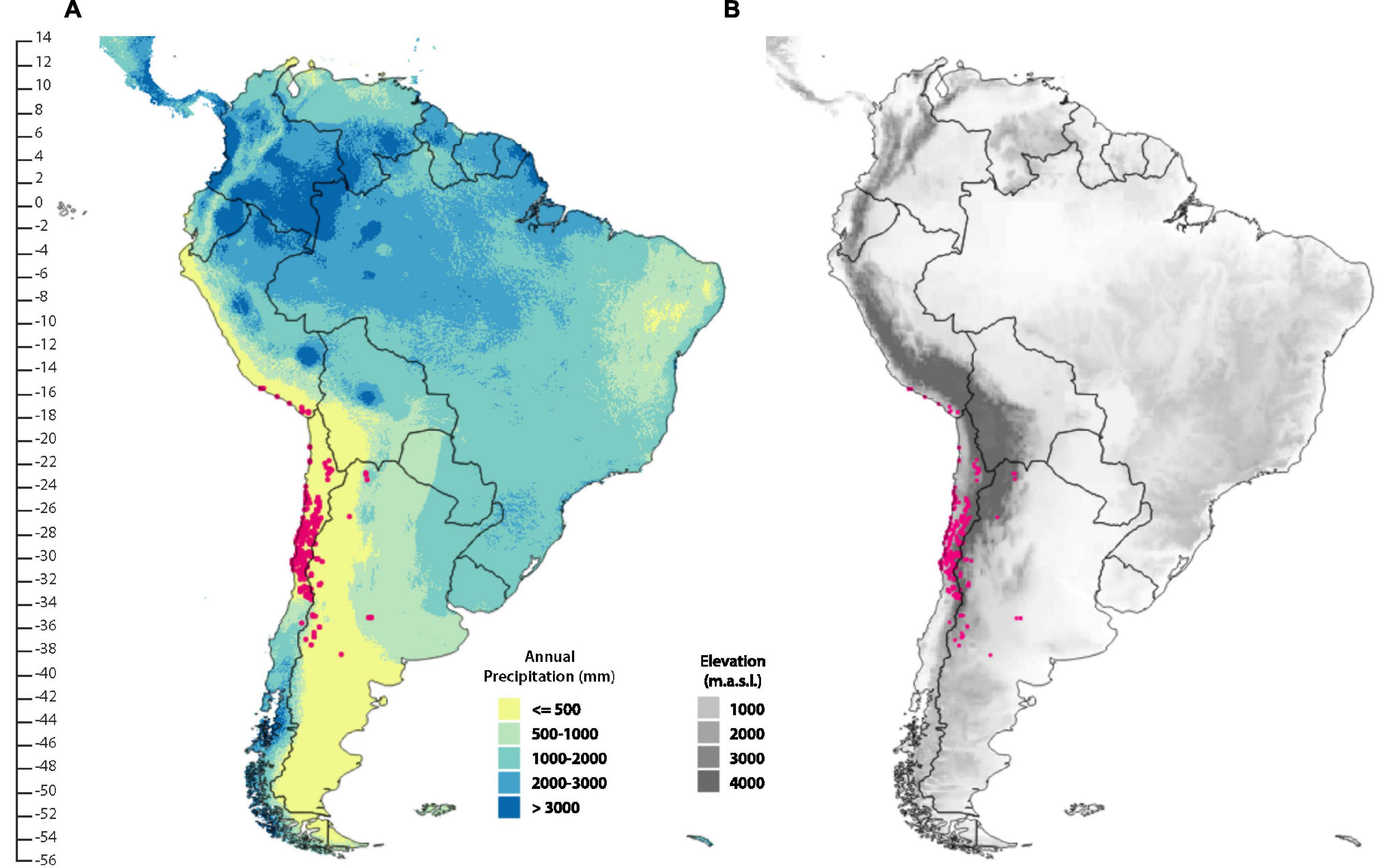
Figure 1. (A) Distribution of the genus Argylia in relation to the South American Arid Diagonal (SAD) (shown in yellow) and elevation (B). The SAD is defined by less than or equal to 500 mm annual precipitation. The red circles are occurrences for all species in the genus.
The Central Andes (4°S–46°S, Ramos, 1999) orogeny is considered to have occurred in different uplift pulses since ca. 30 Ma. The region between 14°S and 16°S reached its current elevation around ca. 5 Ma, Garzione et al. (2017) with the development of a rain shadow blocking off easterly flow today to around 27°S. In contrast, in the southernmost part (from 39°S south), uplift produced a rain shadow effect on the westerlies at 16 Ma (Ramos and Ghiglione, 2008). At a continental scale, the development of SAD led to the disjunction of the forest biome in South America, with several tree/shrub genera distributed today in South American tropical and temperate forests: Azara, Blepharocalyx, Crinodendron, Escallonia, Myrteola, and Myrceugenia (Villagrán and Hinojosa, 1997).
However, the development of SAD associated with Andean uplift would have also promoted diversification of taxa adapted to arid conditions and low temperatures, configuring the development of an arid/semi-arid biogeographic corridor. This corridor would have favored northward expansion and the evolution of Andean-Patagonian taxa. Recognition of a biogeographic corridor necessarily implies that expansion following the SAD would occur when lineages tend to retain their climatic niche requirements (Eldredge et al., 2005; Kozak and Wiens, 2007; Donoghue, 2008; Little et al., 2010; Wiens et al., 2010; Hinojosa et al., 2016). Therefore, when new environments appear, the latter would be occupied by lineages that possess the relevant adaptations for these new conditions and thus manage to colonize these areas by tracking their original climatic niches.
The large family Bignoniaceae Juss. comprises trees, shrubs and vines that are distributed largely in tropical areas and to a lesser extent in temperate zones (Gleisner and Ricardi, 1969; Gentry, 1980; Olmstead, 2013). The oldest fossil record attributed to Bignoniaceae dates to 49.4 Ma (Pigg and Wehr, 2002). Molecular analyses subdivide the family into nine clades made up of 82 genera and 860 species (Lohmann, 2006). The genus Argylia D. Don belongs to the Tecomeae clade (Olmstead, 2013) which together with the Tourrettieae and Jacarandeae clades are the earliest branching clades of the family (Olmstead et al., 2009). Argylia is exclusively distributed from southern Peru (coastal lomas) to the Central Andes of Chile and Argentina. Following the Arid Diagonal of South America, Argylia occurs along the Andes ranges, on the western and eastern slopes but mostly on the western slopes, from 15°S to 38.5°S, from sea level to 4,000 m.a.s.l. Species commonly are found above 1,200 m.a.s.l. A notable exception is Argylia radiata which is distributed mainly along the Pacific coast (Figure 2).
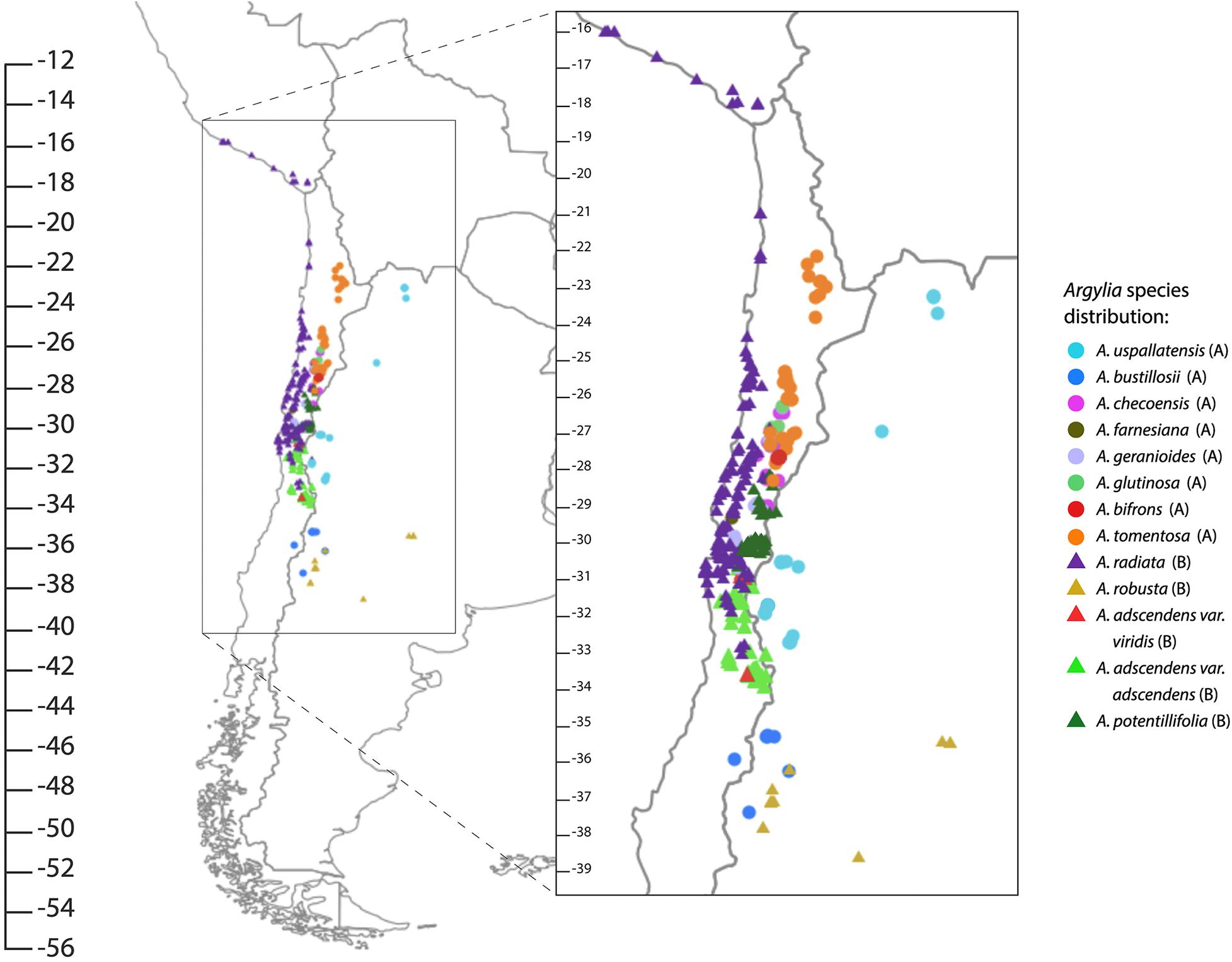
Figure 2. Distribution of species of Argylia. A and B indicate the clade to which a species belongs.
Argylia is unusual in the Bignoniaceae for its perennial herb to subshrub habit and presence of thick woody roots. The palmate leaves with a long petiole are alternate to subopposite. The leaf arrangement also distinguishes Argylia from other genera of Bignoniaceae (Figure 3; Gentry, 1992). The large bell-shaped flowers are cream to brick-red (including within the same species). They appear adapted to bee-pollination. The genus has wind-dispersed seeds.
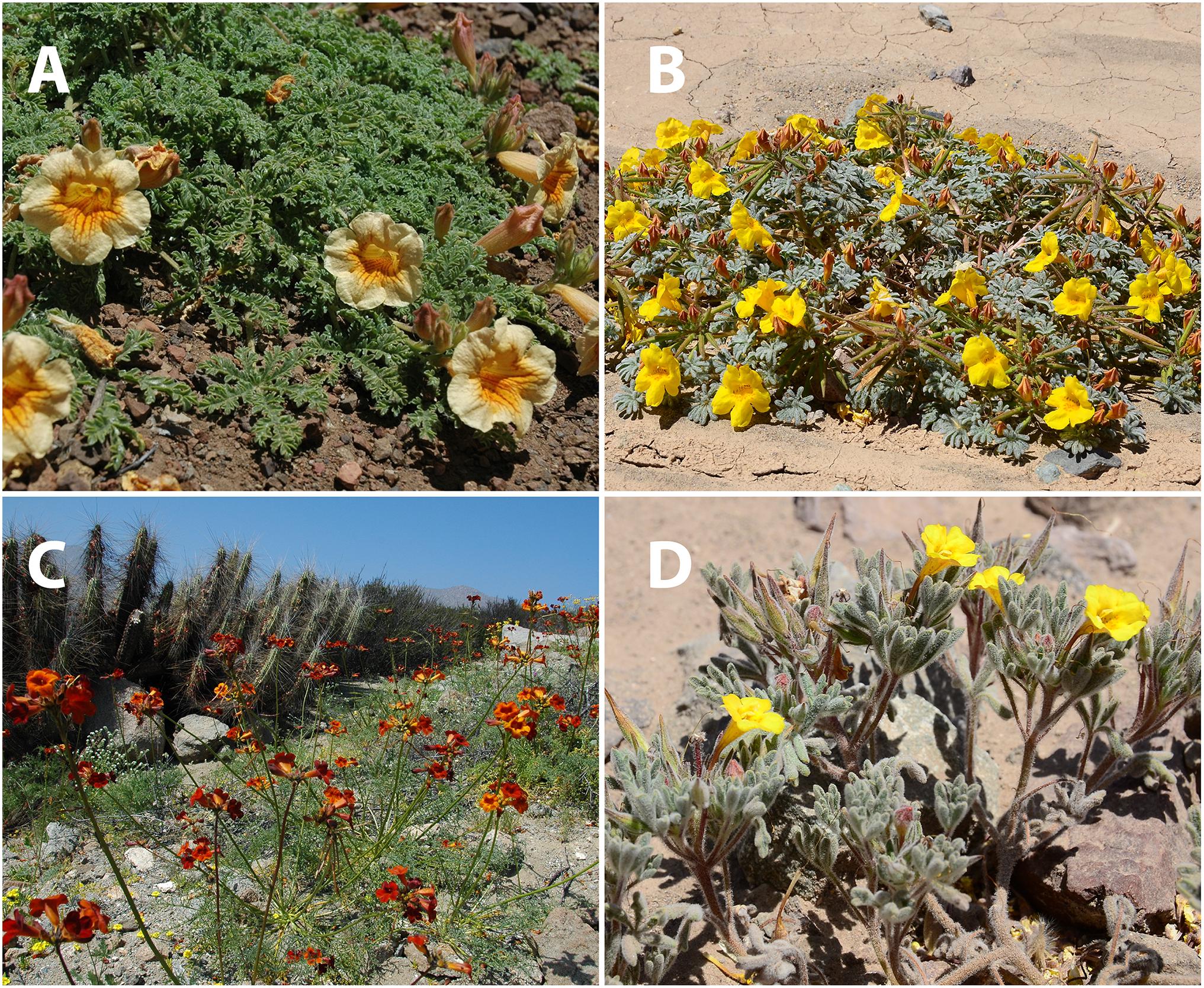
Figure 3. Images of four species of Argylia showing the range of habits found in the genus. (A) A. adscendens van adscendens. (B) A. checoensis. (C) A. radiata. (D) A. tomentosa. Image credits: Maria Teresa Eyzaguirre, Fundación R. A. Philippi.
Argylia is an excellent model for understanding the role of the Andean uplift as promoter of arid environments on plant evolution, and for evaluating the hypothesis of climatic niche conservation in these new environments. In this study we propose: Argylia initially diversified in an area subjected to the South Pacific Subtropical High, the ancestral niche was arid, and the lineage is characterized by niche conservatism in moisture variables. This implies that the current distribution of Argylia was attained over the period of Andean uplift and consequently would have been affected by it. Specifically, we: (1) we establish the phylogenetic relationships of species of the genus Argylia, (2) determine the climatic conditions under which the genus originated, (3) evaluate climatic niche conservatism during its diversification, and finally, (4) evaluate the Andean uplift on the diversification of the genus.
Methodology
Taxon Sampling
DNA for Argylia and other species used in this study was obtained from leaf material of individuals collected in the field and from herbarium material stored CONC (Herbarium of the Department of Botany, University of Concepción), SGO (Herbarium of the National Museum of Natural History), and HULS (Herbarium of de University of La Serena). We obtained DNA of the 13 taxa of the genus Argylia: 10 taxa endemic to Chile [Argylia adscendens DC. var. adscendens; A. adscendens DC. var. viridis (Phil.) Gleisner & Ricardi adscendens; Argylia bifrons Phil.; Argylia checoensis (Meyen) I.M. Johnst.; Argylia farnesiana Gleisner & Ricardi; Argylia geranioides DC.; Argylia glutinosa Phil.; Argylia potentillifolia DC.; A. radiata (L.) D. Don, and Argylia tomentosa Phil.], two species which also occur in Argentina (Argylia bustillosii Phil.; Argylia uspallatensis DC.), and one which is endemic to Argentina (Argylia robusta Sandwith) (Table 1; Gleisner and Ricardi, 1969; Zuloaga et al., 2008). We incorporated several representatives of the family Bignoniaceae as an outgroup; Tecoma capensis (Thunb.) Lindl., Campsidium valdivianum (Phil.) Skottsb., four taxa of the genus Fridericia Mart. and one member of the Verbenaceae [Glandularia laciniata (L.) Schnack & Covas.]. A representative of Verbenaceae was included given that the Verbenaceae is the plant family closest to the Bignoniaceae (Stevens et al., 2001; Table 2). We used more than one individual per taxon initially to verify the positions of Argylia species in the topology.
DNA Extraction, Amplification, and Sequencing
Genomic DNA was extracted with the DNeasy Plant Kit (Qiagen, Valencia, CA, United States). We amplified the DNA using the primers listed in Table 1. PCR used a final volume of 30 μl, which contained 4 μl DNA (25 ng/μl), 8.35 μl distilled water, 3 μl MgCl2 (25 mM), 6 μl buffer, 2.4 μl of dNTP (1 mM), 1.8 μl of each primer (10x ITS4-ITS5 y trnLF), 2.4 μl BSA (25 mM), and 0.25 μl GoTaq (5 U/μl). DNA was denatured at 95°C for 5 min, followed by 35 amplification cycles of 45 s at 94°C, annealing for 1 min at 52°C, elongation for 1.5 min at 72°C and a final extension of 7 min at 72°C. In the case of rpL32-F-trnL, the thermal cycler protocol was modified: DNA was denatured at 80°C for 5 min, followed by 35 amplification cycles of 30 s at 94°C, annealing for 1 min at 56°C, elongation temperature change of 0.3° per second to the elongation temperature for 5 min at 69°C and a final extension of 7 min at 72°C. Samples were sent to Macrogen (Seoul, South Korea) for purification and sequencing. Sequences were loaded, edited and aligned using ChromasPro 2.33 (Technelysium, Brisbane, QLD, Australia) and BioEdit 7.0 (Hall, 1999). All new sequences have been deposited in GenBank (Table 2).
Phylogenetic Analysis and Estimation of Divergence Times
We performed a combined rDNA and cpDNA analysis with a total of 3,149 nuclear characters (913 ITS, 1099 trnF-trnL, and 1495 rpL32-F-trnL, Table 2). Phylogenetic reconstruction was performed with Bayesian inference in the MrBayes version 3.2 program (Ronquist et al., 2012). Three partitions were used for each gene; the evolutionary models for each were: GTR + G in ITS; GTR + I + G in trnL-trnF; and GTR + I in rpL32-F-trnL, which were obtained with the MrModeltest program version 2.2 (Nylander, 2004). Runs appeared stationary prior to 206 generations, and we conservatively excluded the first 2.0 × 106 generations of each run as burn-in. The effective sample size (ESS) value was greater than 200 in a range between 1,174,899 and 1,623,107. Nodes with >0.95 were considered to be supported for posterior probabilities (Ronquist and Huelsenbeck, 2003).
Divergence times were estimated assuming a relaxed molecular clock with evolutionary models for each partition in the Beast program (version 1.4.8; Drummond and Rambaut, 2007). We used the estimated age of the genus Fridericia, sister clade of Argylia, 30.9 Ma (Lohmann et al., 2013) as a calibration point.
Realized Climatic Niche of Extant Argylia Species
We used 371 occurrences for 13 specimens of Argylia obtained from herbarium species stored at CONC (Universidad de Concepción) and from records in the Global Biodiversity Information Facility (GBIF). These occurrences were verified with each species distribution reported in the bibliography (Gentry, 1992; Rodriguez et al., 2018) eliminating doubtful georeferences that did not correspond to what was established or, that corresponded to the location of the herbarium and not to the area where species were sampled. From the CHELSA data base, we obtained 19 bioclimatic variables with approximately 1 km2 resolution (Karger et al., 2017). For niche modeling, we performed a principal component analysis on the 19 bioclimatic variables selecting 11 which retained 78% of the total variance (Supplementary Figure 1): Mean Annual Temperature (MAT), Temperature Seasonality (TS), max. Temperature of the Warmest Month (maxTWaM), min. Temperature of the Coldest Month, mean Temperature of the Warmest Quarter (minTCQ), mean Temperature of the Coldest Quarter (mTCQ), Annual Precipitation (AP), Precipitation of the Driest Month (PDM), Precipitation Seasonality (PS), Precipitation of the wettest Quarter (PWeQ), and Precipitation of the Driest Quarter (PDQ).
The climatic niche for each species was modeled using the maximum entropy algorithm Maxent (Phillips et al., 2006). We used a total of 50 replicates, 25% of the data as a training set and, in order to avoid model overfitting, we defined a species-specific setting selected for Maxent using the “ENMeval” (Muscarella et al., 2014) R package. The approach implemented in ENMeval runs successively several MAXENT models using different combinations of parameters to select the settings that optimize the trade-off between goodness of fit and overfitting. We selected the parameters with the lowest AIC values (Supplementary Table 1).
Climatic Niche Evolution
The predicted niche occupancy (PNO) profiles were used to calculate the maximum likelihood estimate and 95% confidence intervals (95% CI) for each climate variable at each interior node of the phylogeny, assuming Brownian motion evolution (Evans et al., 2009). To obtain PNO profiles with respect to the bioclimatic variables selected, we used the raw probability (RP) distribution of each species derived from Maxent. Confidence intervals were calculated using an unbiased estimate of the variance of the Brownian motion. Analyses were conducted using the R packages Phyloclim (Heibl, 2011), Ape (Paradis et al., 2004), and Phytools (Revell, 2012).
To evaluate the evolution of the climatic niche in Argylia, we tested phylogenetic signal estimating Pagel’s lambda (Pagel, 1994), which ranges from zero to one. Lambda (λ) is a scaling parameter for correlations between the phylogenetic similarity matrix and the trait matrix. λ = 0 signifies that trait correlations between species are independent of phylogeny. Conversely, λ = 1 indicates similarity between species equal to the Brownian motion model of evolution expectation in which case trait evolution is strongly influenced by phylogeny (Pagel, 1999). The λ parameter was estimated for each bioclimatic variable using the phylosig function from the R-package Phytools (Revell, 2012). To evaluate the fit of climatic niche we used the Akaike Information Criterion for three evolutionary models: (a) a Brownian motion (BM) model of gradual and continuous drift, (b) an Ornstein-Uhlenbeck (OU) model which can be thought of as a stabilizing selection model of evolution with one optimum, and (c) a White Noise (WN) model of random variation, in which climatic niche variation is independent of phylogenetic relationships (Hansen et al., 2008; Hawkins et al., 2014). Fits to these alternative models was made using the fitContinuous function from the R package Geiger (Harmon et al., 2008). All the analyses were conducted with R v. 3.3.3 (R Core Team, 2021).
To infer the climatic history of Argylia, we used the projection of our phylogenetic tree in environmental (each bioclimatic variable) and temporal space assuming Brownian motion evolution (BM; Schluter et al., 1997; Evans et al., 2009), using the R package Phytools (Revell, 2012).
Ancestral Range Distribution Reconstruction
We inferred ancestral distributions across the Argylia phylogeny by comparing models that considered anagenetic evolutionary process (i.e., dispersal, extinction), with cladogenetic process (i.e., sympatry and vicariance). BioGeoBEARS R package (Matzke, 2014; R Core Team, 2021) implements widely used models of range evolution (e.g., Ree and Smith, 2008), but it includes an additional parameter of cladogenetic speciation mediated by founder events: the jump parameter “j.” This parameter allows daughter species to instantaneously “jump” outside the geographical range of parental species by long distance dispersal or dispersalism. A likelihood ratio test was performed to reject the null hypothesis that the incorporation of the parameter “J” in each model confer equal likelihoods on data. Finally, the Akaike Information Criterion (AIC) was used to compare the relative fit of the six models and choose the best model of ancestral range reconstruction (Matzke, 2014). The models compared were: Dispersal extinction cladogenesis (DEC) and DEC + J; a likelihood dispersal-vicariance analysis (DIVAlike) and DIVALIKE + J and Bayesian biogeographical inference model (BAYAREALIKE) and BAYAREALIKE + J, a likelihood version where no particular cladogenetic events occurred. Our analysis considered four areas for ancestral range reconstruction, taking into account the altitudinal and latitudinal characteristics of the present distribution of Argylia species: Pacific Coast (A), Central Andes between 20°S and 33°S (B), Central Andes south 33°S, southern limit of Central Andes flat slab (C), Patagonia (D). These areas were defined according to a geological definition for Central Andes (Ramos, 1999) and according to current distribution of the genus. This region was divided into north of 33°S and south of 33°S. The latter corresponds to the Central Andes Flat Slab region, segment where there is an absence of volcanic activity (Charrier et al., 2007). Central Andes, the main area of our study, also corresponds to the Ecoregion of Central Andes according to Olson et al. (2001). On the other hand, South of 33°S is an area where precipitation occurs during winter and is associated to the Southern Andean Steppe Ecoregion (Olson et al., 2001). Finally, we defined the area “Pacific coast” to represents the distribution of the Atacama-Sechura desert Ecoregion (Olson et al., 2001). And finally, we defined the “Patagonia” area, to describe the distribution along the Patagonian Pampa Ecoregion (Olson et al., 2001).
We constrained our analysis to the Andean uplift. For this we divided our multiplier matrix (Supplementary Figure 2) into four time periods: (1) 50-35 My defined by the global cooling event; (2) 35-15 My defined by the glaciation of east Antarctica; (3) 15-5 MY defined by Andean uplift prior to modern elevation; 5–0 My defined by the culmination of Andean uplift.
Finally, we estimated the number and type of biogeographical events that account for the present distribution of Argylia. For this we used biogeographical stochastic mapping (BSM) implemented in “BioGeoBEARS” (Matzke, 2015).
Results
Phylogenetic Analysis and Divergence Times
The results show that Argylia is a monophyletic group with an estimated minimum date of origin of ∼38.21 Ma (Figure 4). It is composed of two main clades and four subclades. Clade A comprises eight taxa. It diverged ∼28.04 Ma to further subdivide into two subclades: SC I, formed by the two Chilean-Argentinean taxa A. uspallatensis and A. bustillosii, with a minimum age of ∼9.76 Ma, and SC II, composed of six Chilean taxa: A. tomentosa, A. bifrons, A. glutinosa, A. geranioides, A. checoensis, A. farnesiana, with a minimum age of ∼19.25 Ma. Clade B, with a minimum age of ∼25.77 Ma, comprises five taxa. This clade is separated into A. robusta, which occurs exclusively in Argentina (Mendoza, Neuquén, and Río Negro) is sister to SC III subclade, which is formed by the Chilean species A. potentillifolia, A. radiata, A. adscendens var. adscendens and A. adscendens var. viridis, with a minimum age of ∼16.33 Ma.
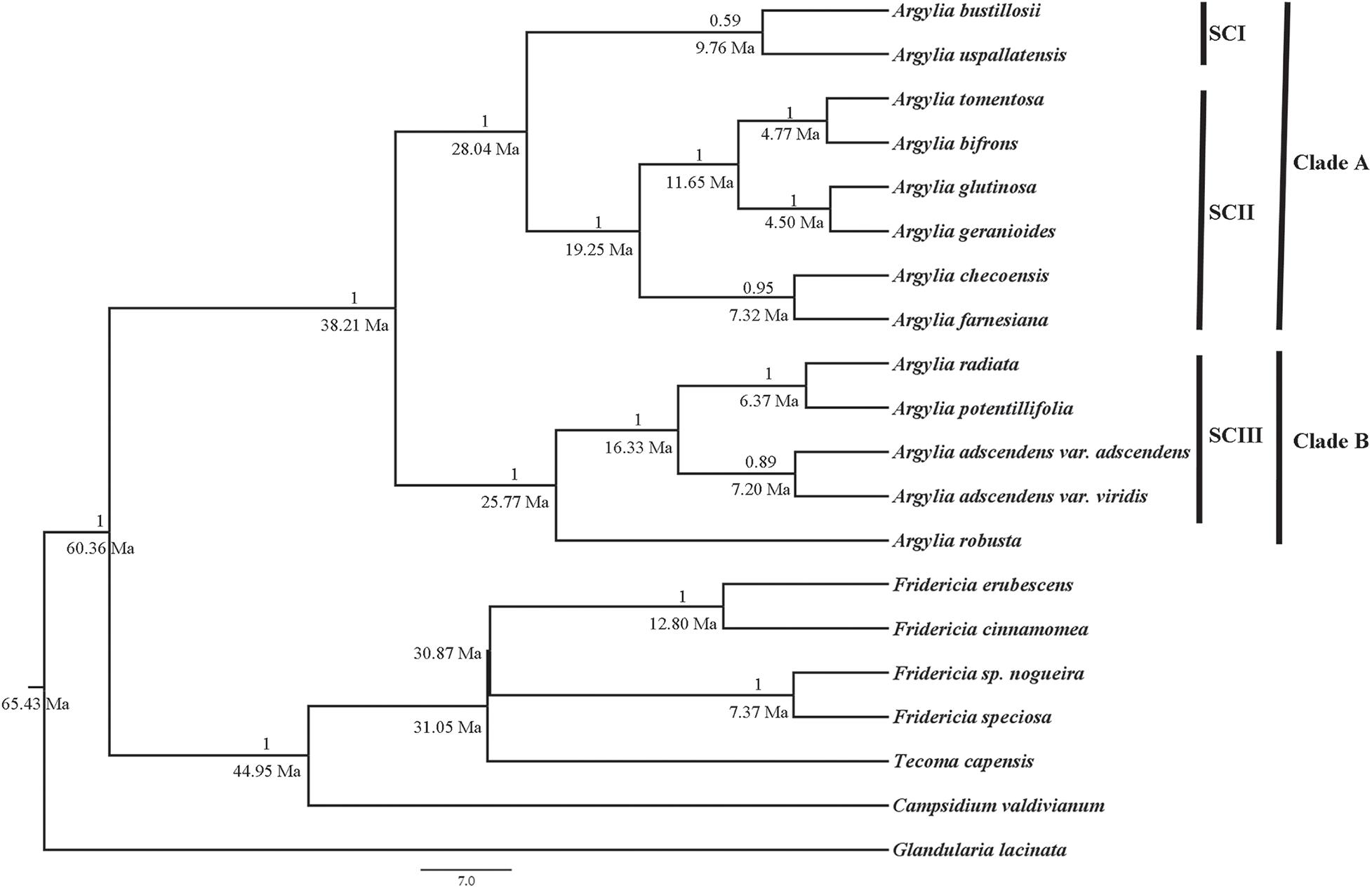
Figure 4. Bayesian inference phylogenetic tree based on combined analysis (rDNA and cpDNA) for 13 taxa of the genus Argylia and seven taxa belong to the Bignoniaceae and Verbenaceae. Posterior probability values are found above the branches. Estimation of divergence times are found below the branches. The principal lineages are indicated by clade A and clade B, and subclades SCI, SCII, and SCIII.
Realized Climatic Niche and Niche Evolution
Climatic niche models obtained with Maxent performed consistently well. The average training AUC for 50-replicate runs were all above 0.9 (Table 3). Extant species of Argylia are primarily found in microthermal climatic conditions with a Mean Annual Temperature ranging from 7°C to 13.4°C and an Annual Precipitation ranging from 20 to 477 mm (Figure 5 and Table 3). Exceptionally, A. radiata and A. farnesiana are found in marginally mesothermal conditions, as shown by Mean Annual Temperature above 14°C.
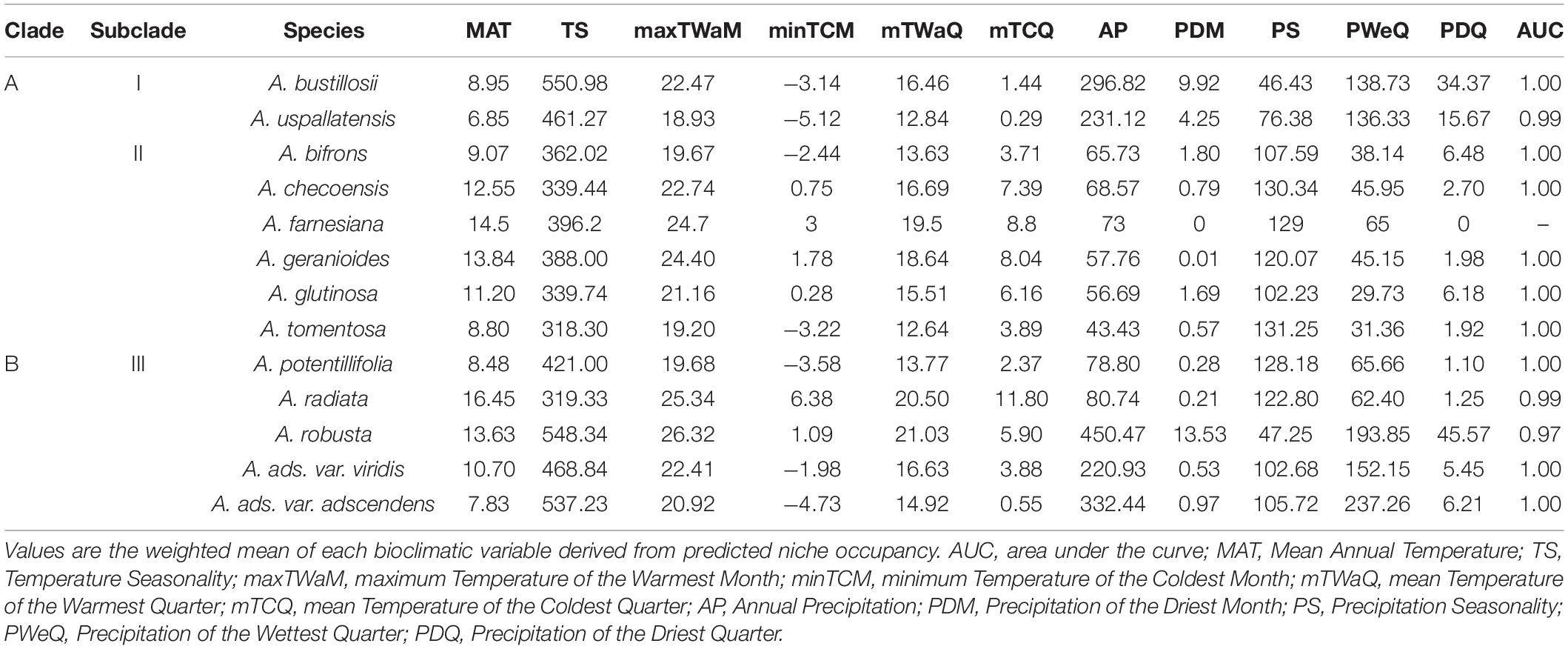
Table 3. Bioclimatic variables for species of the genus Argylia, along with the corresponding phylogenetic clade and subclade (see Figure 4).
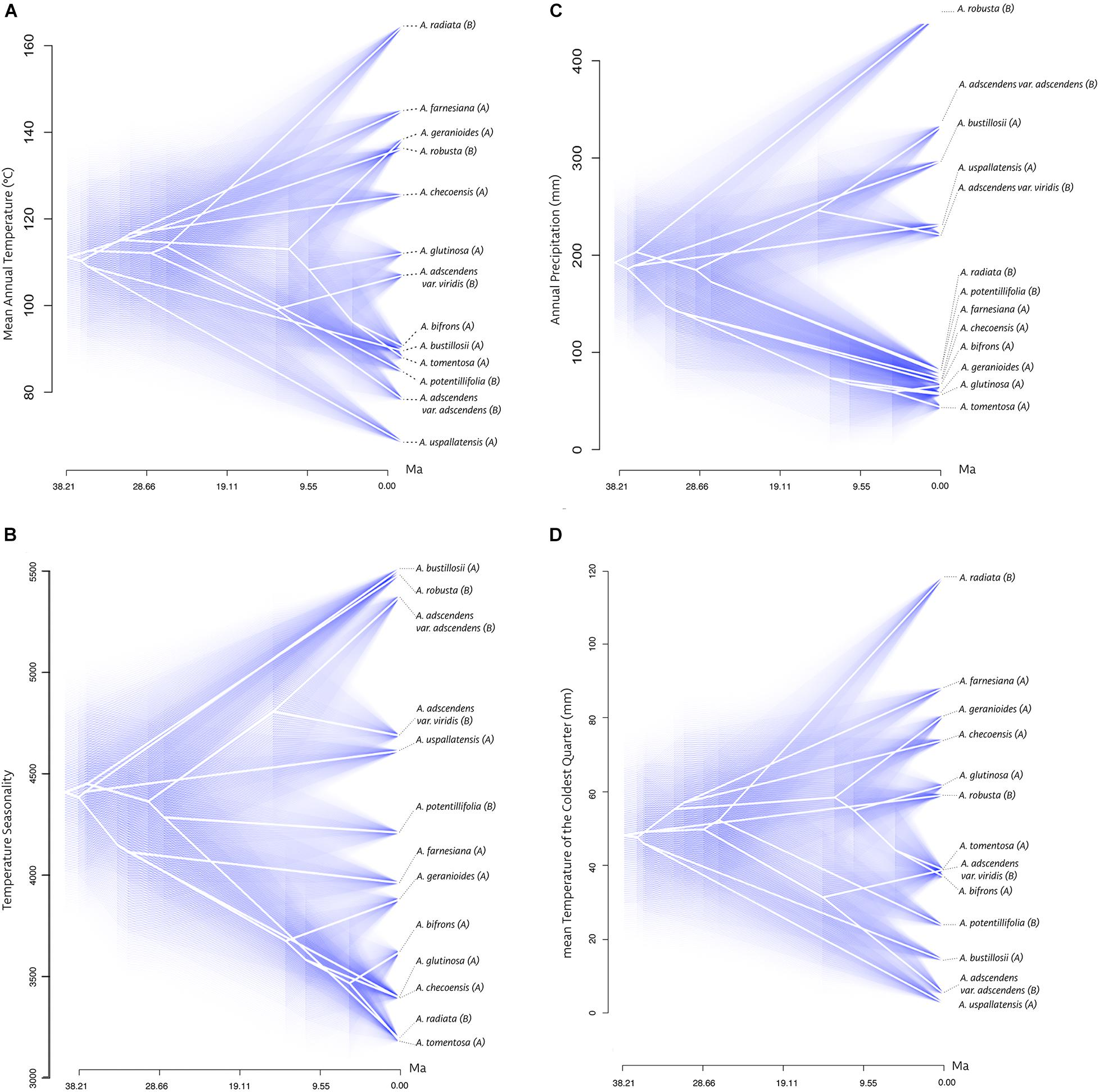
Figure 5. Traitgram of ancestral states of Argylia climatic niche. White lines correspond to a projection of the phylogenetic tree in a space defined by each bioclimatic variable. (A) Mean annual temperature (°C); (B) Temperature Seasonality; (C) Annual Precipitation (mm); (D) mean Temperature of de Coldest Quarter (°C). Blue shade areas correspond to the 95% of confidence interval. Dashed lines to phylogenetic tree tips link the names of Argylia species. Clades of species are shown in parenthesis: (A) and (B) as in Figure 4.
Traitgrams associated with precipitation in Argylia show that sister species are more similar to one another than to their more distant relatives, suggesting phylogenetic niche conservatism of these variables (Figure 5C and Supplementary Figures 3A–D). On the contrary, bioclimatic variables associated with temperature generally show no phylogenetic niche conservatism. In this case, species grouping along the temperature axes show multiple crossing between the two clades (Figure 5A and Supplementary Figures 3E–G). Exceptionally, traitgrams for Temperature Seasonality and winter temperature (mean Temperature of the Coldest Quarter) shows niche conservatism (Figures 5B,D).
Pagel’s phylogenetic signal test shows the same pattern. Precipitation variables presented strong phylogenetic signal, indicated by λ significantly different from zero and equal to 1 (p ≤ 0.05, Table 4). The rest of the temperature bioclimatic variables fit better to the null model of evolution (White Noise), suggesting phylogenetic independence in the evolutionary history of these traits. Exceptionally, Temperature Seasonality (Bio4) and mean Temperature of the Coldest Quarter (Bio11), shows phylogenetic niche conservatism. Winter temperature (mean Temperature of the Coldest Quarter) showed marginally significant niche conservatism (p = 0.055, Table 4).
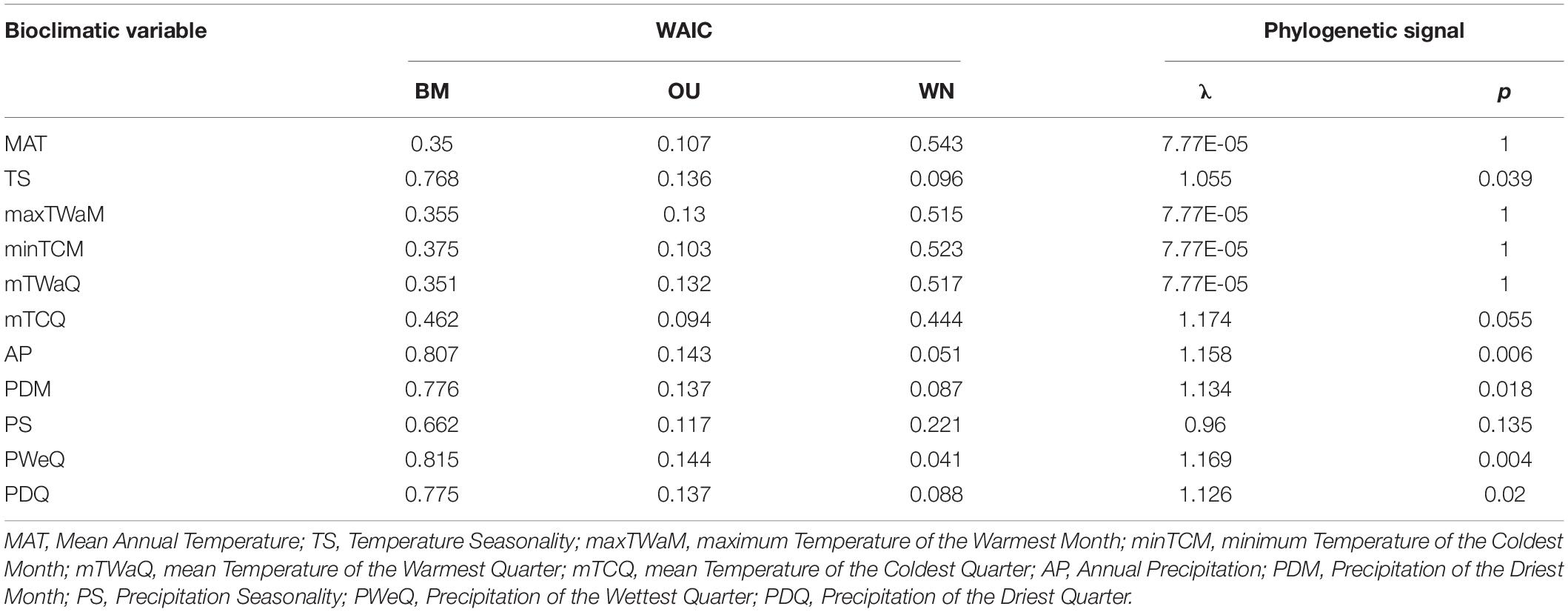
Table 4. Results of phylogenetic signal (Pagel’s Lambda) and Weighted Akaike, based on exp (−0.5 × ΔAIC) to compare the best fit between a Brownian Motion (BM) model, an Ornstein-Uhlenbeck (OU) model and a White Noise (WN) null model of evolution.
Ancestral Range Distribution Reconstruction
Ancestral range estimations under the best fit model (BAYAREALIKE + j, Table 5) showed that the most probable ancestral area for extant species of Argylia is the Central Andes (B, Figure 6). A summary of Biogeographical Stochastic Mappings (BSMs) revealed that most biogeographical events comprise within-area speciation (64.2%) and cladogenetic dispersal (21.4%), with few areal expansions (14.4%) (Table 6 and Supplementary Figure 4).

Table 5. Comparative statistical analyses to compare the best fit between biogeographical models: DEC, dispersal-extinction-cladogenetic model; DIVALIKE, likelihood dispersal-vicariance model; BAYAREALIKE: Bayesian biogeographical inference model.
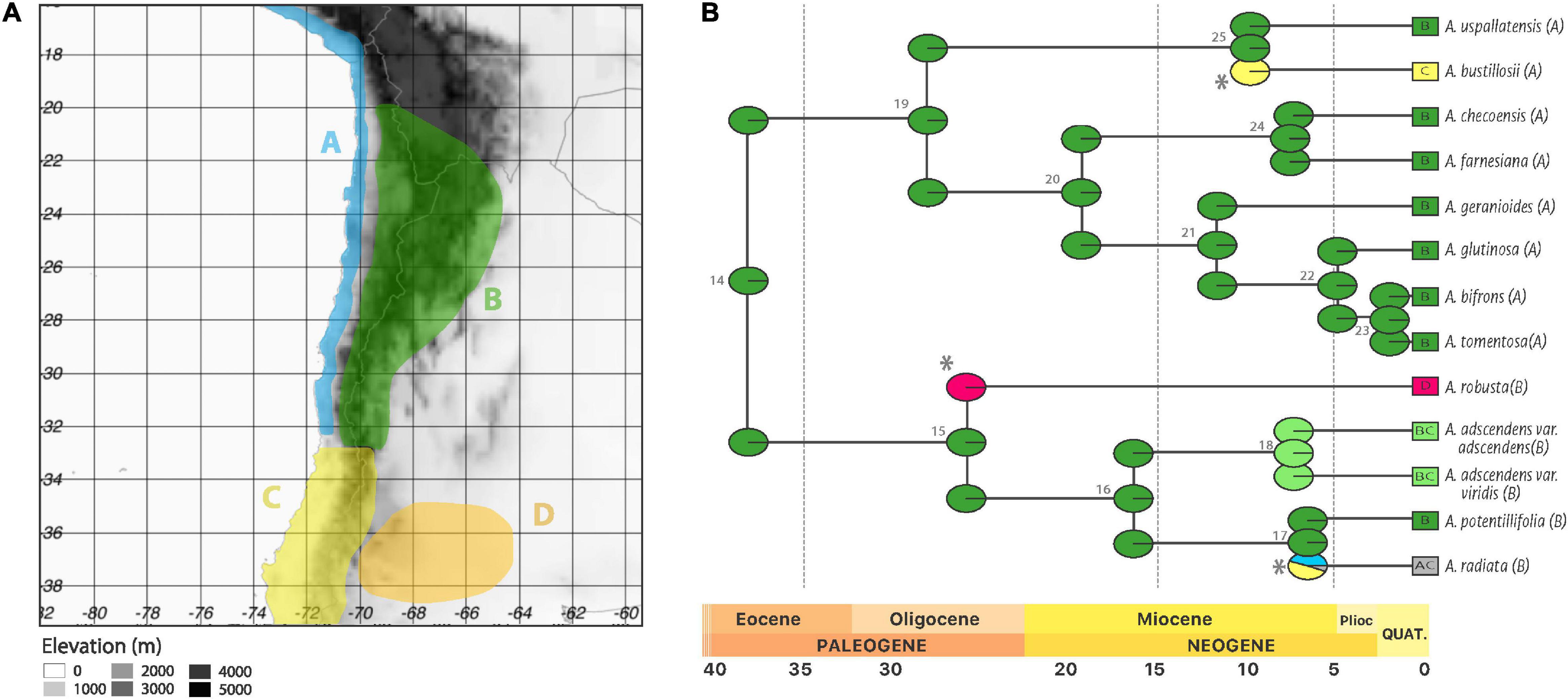
Figure 6. Results for the Ancestral Range Reconstruction. (A) Range distribution of the four areas defined by the ancestral range reconstruction A: Pacific Coast, B: Central Andes 20°S–33°S, C: Central Andes 33°S south and D: Patagonia. (B) Maximum likelihood ancestral range estimation, using the best model BAYAREALIKE+j. The pie diagrams at nodes show the relative probability of the possible states (areas or combinations of areas). The boxes on the right show the native ranges of taxa within these clades, as in (A). The asterisks mark founder events and the small numbers in gray indicate node numbers.
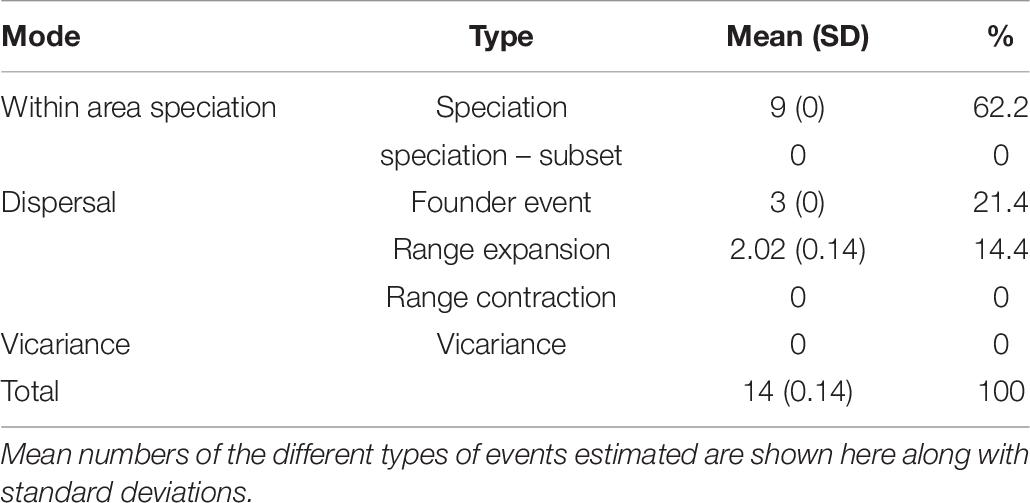
Table 6. Summary of biogeographical stochastic mapping counts for Argylia using the BAYAREALIKE + j model.
The inclusion of the “j” parameter consistently improved model fit, suggesting that range expansions alone are not sufficient to account for movement into new areas (Table 5). Three founder events were identified. The first was defined by dispersal from the Central Andes 20°S to 33°S (B) to Patagonia (D), indicated in red (Figure 6). The second founder event developed from Central Andes 20°S to 33°S (B) to Central Andes 33°S south (C), indicated with yellow in A. bustillosii (Figure 6). A range expansion was detected, from Central Andes 22°S to 33°S to Central Andes 33°S south, defining the current distribution of A. adscendens clade (BC, Figure 6), between Middle and Upper Miocene. The last founder event involved the Central Andes 22°S–33°S (B) to the Central Andes 33°S south (C) with later range expansion toward the Pacific Coast (A), defining the current distribution of A. radiata (indicated in gray in Figure 6).
Discussion
Phylogeny and Climatic Niche Evolution
According to Lohmann et al. (2013), the Bignoniaceae node where Argylia occurs in the family Bignoniaceae has a date of origin of ∼49.8 Ma, while Olmstead et al. (2009) indicated that Argylia is one of the earliest-branching clades of the family. This agrees with the ages we obtained in this study, which show a minimum origin time for Argylia of ∼38.2 Ma (Late Eocene). Two main clades are distinguished in the Argylia phylogeny (Figure 4). Clade A diversified during the Oligocene at ∼28.0 Ma, while clade B diversified around 25.8 Ma.
Extant species of Argylia are distributed mainly on microthermal (11°C in average) and arid conditions (below 500 mm of annual precipitation), as shown by our climatic niche model, apart from A. radiata which inhabits mesothermal conditions (16°C, Table 3). This species has a very large latitudinal distribution (15°S–33°S).
Lack of phylogenetic signal in most of the temperature variables indicates that evolution in Argylia is mediated by temperature adaptation associated. The broad current altitudinal distribution of the genus, where most of Argylia species are distributed between 2,000 and 4,500 m.a.s.l., reflects adaptation to progressively lower temperatures over steep elevational gradients. Exceptionally, Temperature Seasonality had a strong phylogenetic signal (λ = 1, Table 4), indicating that throughout its evolutionary history, Argylia species tracked annual contrasting climatic conditions. Winter temperature (mean Temperature of the Coldest Quarter), which strongly determine temperature variation (or seasonality), also showed phylogenetic signal, although it was only marginally significant (see Table 4).
Ancestral reconstruction for temperature seasonality shows that Argylia must have been distributed in regions with a significant thermal amplitude (Supplementary Table 2). This condition is associated with continentally, where annual temperature fluctuation increases from coastal to inland areas (Driscoll and Fong, 1992). The current distribution of Argylia seems to correlate broadly with this pattern (Supplementary Figure 5), reinforcing the idea that Argylia’s evolution was driven by the emergence of the Arid Diagonal, as we hypothesized.
The requirement of seasonality condition for Argylia’s climatic niche can also be observed with precipitation variables that presented a strong phylogenetic signal, such as winter and summer precipitation (Precipitation Seasonality, Precipitation of the Driest Month, Precipitation of the Wettest Quarter and Precipitation of the Driest Quarter, Figure 5, Table 4, and Supplementary Figure 3). Even though, climatic seasonality is not the only determinant requirement for the genus, but also the availability of moisture seems to have a strong effect over Argylia’s evolution and diversification, given by the strong phylogenetic conservatism of Annual Precipitation (Figure 5A and Table 4).
These results are especially important under current climate change scenarios. Especially in northern and central Chile, where there is a 30% of precipitation deficit and a historic increase in temperatures (Center for Climate and Resilience Research [CR2], 2015). Considering the projection of a decrease in precipitation for the 2100 year (Qin et al., 2014), high Andean genera with strong precipitation conservation, such as Argylia, could be at risk. Indeed, a reduction in precipitation is likely to have much more severe consequences that an increase in temperature.
Ancestral Range Reconstruction
The timing of Andean uplift and late Cenozoic global climate change can be observed in the biogeographical reconstruction of Argylia (Figure 6 and Ramos and Ghiglione, 2008; Giambiagi et al., 2016; Garzione et al., 2017). Our result suggests that the Central Andes between 20°S and 33°S would be the area were Argylia initially diversified. Three founder effects were found in our biogeographical model (Table 6, Figure 6, and Supplementary Figure 4).
The first, allowed the colonization of the Extra Andean area, located south of 32°S at 25.77 Ma. This limit has been previously recognized as a distributional boundary for some Argylia species (Gentry, 1992) and has also been recognized as the southern part of the Central Andean Flat Slab (Ramos and Folguera, 2009). During the Oligocene, both isotopic and fossil evidence show a drop of temperature and precipitation (Zachos et al., 2001; Hinojosa, 2005), coeval with the uplift of Patagonian Andes (Ramos and Ghiglione, 2008; Figure 7), suggesting semi-arid conditions on Patagonia by the time of this colonization. Aridity would also be reinforced by the effect of the anticyclonic subsidence and global temperature decline, which in turned increased equator-pole climatic gradient (Zachos et al., 2001; Hartley et al., 2005).
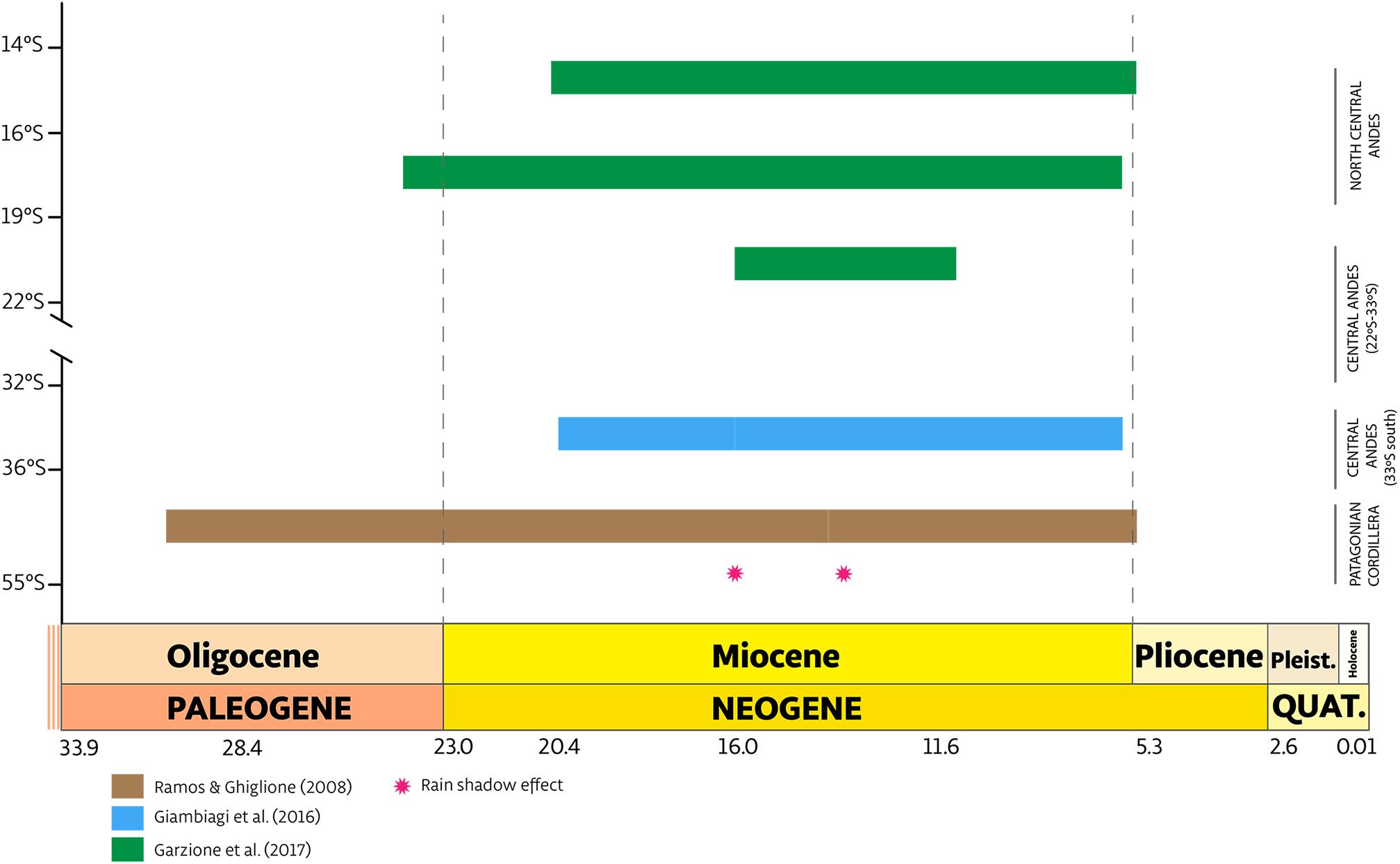
Figure 7. Schematic diagram of the time of uplift along the Northern Central Andes, Central Andes (20°S–33°S and 33°S south) and Patagonian Andes based on Ramos and Ghiglione (2008), Garzione et al. (2017), and Giambiagi et al. (2016). The geological timeline is shown in Ma. Red stars provide an estimation of when the rain shadow associated with elevation came into force.
The last two founder events occurred independently in the evolutionary history of Argylia, resulting in colonization of Central Andes 33°S south from the ancestral area (Central Andes 22°S–33°S, Figure 6). These dispersal events occurred between 15 and 5 Ma, by which time the Andean uplift pulses had already begun (Figures 6, 7; Ramos and Ghiglione, 2008; Giambiagi et al., 2016; Garzione et al., 2017). This period corresponds to the modern climate in South America stemming from the establishment of the Antarctic circumpolar current, the modern atmospheric circulation pattern and reinforcement of the Humboldt Current in the Pacific Ocean (Hinojosa and Villagrán, 1997; Hartley et al., 2005; Garreaud et al., 2010). These events are a sign of the birth of the Andean corridor, allowing north-south dispersal of species (Luebert and Weigend, 2014). This corridor has been considered as an explanation for plant diversification patterns in South America, such as in: Azorella, Chuquiraga; Laretia, Leucheria, Mulinum, and Perezia (Ezcurra, 2002; Simpson et al., 2009; Nicolas and Plunkett, 2012, 2014; Pérez et al., 2020). Apparently, this holds true also for Argylia, where dispersal and cladogenetic events occurred mainly during a period when arid and semi-arid altitudinal belts were formed. However, formal climatic niche analyses, as performed here, are lacking for the above-mentioned genera.
Two range expansion were detected within B clade (Figure 6). The first expansion occurred from Central Andes 22°S to 33°S (ancestral area, B) to Central Andes 33°S south (C), between Middle and Upper Miocene during the Andes Mountain building (Figure 7; Giambiagi et al., 2016), allowing a climatic corridor between both areas and range expansion of A. adscendens Clade (var. adscendens and var. viridis). The second expansion occurred from Central Andes 33°S south to Pacific Coast (A), which allowed A. radiata to colonize the coastal area as far north as southern Peru (Figure 2; Gentry, 1992; Rodriguez et al., 2018). Argylia’s northward expansion (Figure 6) suggest a second corridor along the Pacific Coast at the end of Cenozoic and during the Quaternary as suggested by its distribution. Today, this species is distributed from 33°S to 15.8°S, occupying the coastal range along the subtropical West of South America (Figure 2), where moisture comes mainly from the interception of low coastal clouds, a phenomenon known as the “Camanchaca.” Coastal topography (Rundel et al., 1991; Garreaud et al., 2008) associated with the Camanchaca has allowed the development of high species richness in the coastal Atacama and Sechura Deserts comprising the Lomas Formation (Hueck and Seibert, 1972; Rundel et al., 1991). The main drivers of the Camanchaca are the strong air subsidence associated with the South American Subtropical High (SASH), the annual variability of SASH, the constant temperature inversion by the cool Humboldt Current and the uplift of the coastal topography (Rundel et al., 1991; Garreaud et al., 2008, 2010). The age of the Humboldt Current and elevated coastal topography dates to Middle/Upper Neogene and Pleistocene, respectively (Hinojosa and Villagrán, 1997; Villagrán et al., 2004; Hartley et al., 2005; Garreaud et al., 2010; Regard et al., 2021). These ages are consistent with our ancestral range reconstruction and the strong phylogenetic signal on moisture variables found in this study. It would be worthwhile testing this phylogeographic hypothesis at the population level over the entire distributional range A. radiata.
All the above expansions were mediated by the last pulse of Andean uplift (5 Ma, Figures 6, 7), as well by the emergence of hyper arid conditions along with the birth of the Atacama Desert (Villagrán and Hinojosa, 1997; Hartley et al., 2005), as clearly shown by the current pattern of the northernmost distribution of the genus, which presents an east-west disjunction among the species distributed in this region.
Diversification and development of novel adaptations promoted by arid conditions have been widely established in other South American plant genera: Chaetanthera, Malesherbia, Cristaria, Heliotropium sect. cochranea, Leucheria, and Leucocoryne (Gengler-Nowak, 2002; Guerrero et al., 2013; Jara-Arancio et al., 2014, 2017; Böhnert et al., 2019; Pérez et al., 2020), in accordance with the idea that arid regions are strong drivers of lineage diversification (Stebbins, 1952).
According to Luebert and Weigend (2014), high elevation taxa showed divergence times that correspond mainly with Andean uplift in the late Miocene and early Pliocene, with some taxa also diversifying in Quaternary times. The authors also highlight a north to south trend for high-Andean lineages, with lineages with more recent origin in the north and older lineages in the south. Additionally, in Chile from 18°S to 35°S, phylogenetic diversity increases from north to south, following a precipitation gradient. Both patterns could be explained by the presence of a younger arid environment that favored the diversification in this new biome (Scherson et al., 2017; Böhnert et al., 2019).
Finally, the proposition of range expansion of A. radiata through a corridor from Central Andes south 33°S to Pacific Coast up to 15°S in Peru, by the Late Miocene (Figure 6), would be associated with what Guerrero et al. (2013) described as a widespread temporal lag between the establishment of arid and hyperarid climates and a later diversification through Atacama-Sechura Desert, recorded in genera Nolana, Chaetanthera, and Malesherbia. This temporary lag may be a consequence of the later establishment of the second climatic corridor that we propose in this study, the coastal lomas, between the Upper Miocene and early Quaternary (Regard et al., 2021).
Conclusion
In this work we evaluated the role of the Andean uplift and consequently the development of the Arid Diagonal in the evolution of the Argylia lineage. We postulated that: (a) an early diversification of Argylia in an ancestral area under arid conditions (given by the presence of the high-pressure system of the South Pacific Subtropical High), (b) Phylogenetic niche conservatism of climatic requirements, and (c) dispersal and later diversification of Argylia modulated by the Andean uplift and its role as a biological corridor.
That all precipitations variables considered were highly conserved indicates that the distribution of Argylia is strongly determined by moisture availability. Our results also showed that Argylia’s climatic niche is determined by annual temperature fluctuations, indicating that the ancestral range of Argylia must have been in a region where the Andes was uplifting.
The striking difference between temperature and precipitation variables is a novel finding and one that should be looked for in other Andean genera. Is this a general tendency, or variable among taxa? If it is a general condition, it requires an intrinsic physiological explanation.
On the other hand, the ancestral area of distribution for the genus was north of 32°S along the Andean range. At the time of the origin of Argylia, this would have been a region where subtropical conditions were already present. Later dispersal and diversifications occurred coeval with Andean uplift acting as a “corridor.” A second climatic corridor is postulated by our analysis, where the northward expansion into the hyperarid zone in the coast is associate with an extra moisture supply by the marine low clouds Camanchaca. Thus, Argylia tracked its moisture requirements as suggested by the strong phylogenetic signal in these environmental variables. At the end of the Neogene and during the Pleistocene, the Argylia lineage would have reached its modern distribution and associated species diversity.
Finally, Argylia’s biogeographic history highlights the value of niche conservatism studies where the availability of climatic corridors and moisture environmental filters are essential for species range expansions in the context of modern climate change.
Data Availability Statement
The original contributions presented in the study are publicly available. This data can be found here: https://www.ncbi.nlm.nih.gov/genbank/. Accession number(s) can be found in the article/Supplementary Material.
Author Contributions
NG-V, MA, LH, PJ-A, and CR: conception and design of the study. NG-V, PJ-A, PV, and CR: acquisition of the data. NG-V, LH, and PJ-A: analysis and/or interpretation of the data. NG-V, LH, and MA: drafting and revising the manuscript. All authors contributed to the article and approved the submitted version.
Funding
This research was funded by the ANID PIA APOYO CCTE AFB170008 and ACE210006 to the Instituto de Ecología y Biodiversidad (IEB), FONDECYT 1140541, 1180454 and 1211765, and Anillo ACT172099 (PIA, ANID).
Conflict of Interest
The authors declare that the research was conducted in the absence of any commercial or financial relationships that could be construed as a potential conflict of interest.
Publisher’s Note
All claims expressed in this article are solely those of the authors and do not necessarily represent those of their affiliated organizations, or those of the publisher, the editors and the reviewers. Any product that may be evaluated in this article, or claim that may be made by its manufacturer, is not guaranteed or endorsed by the publisher.
Acknowledgments
We are very grateful to the Fundación R. A. Philippi, Santiago, Chile for permission to use their images of the four illustrated Argylia species.
Supplementary Material
The Supplementary Material for this article can be found online at: https://www.frontiersin.org/articles/10.3389/fpls.2021.724057/full#supplementary-material
References
Böhnert, T., Luebert, F., Ritter, B., Merklinger, F. F., Stoll, A., Schneider, J. V., et al. (2019). Origin and diversification of Cristaria (Malvaceae) parallel Andean orogeny and onset of hyperaridity in the Atacama Desert. Glob. Planet. Change 181:102992. doi: 10.1016/j.gloplacha.2019.102992
Center for Climate and Resilience Research [CR2] (2015). The 2010-2015 Mega-Drought: A Lesson for the Future. Santiago: Center for climate and resilience research.
Charrier, R., Pinto, L., and Rodríguez, M. P. (2007). “Tectonostratigraphic evolution of the Andean Orogen in Chile,” in The geology of Chile, eds T. Moreno and W. Gibbons (London: Geological Society Special Publication), 21–114. doi: 10.1144/goch.3
Donoghue, M. J. (2008). A phylogenetic perspective on the distribution of plant diversity. Proc. Natl. Acad. Sci. U.S.A. 105, 11549–11555. doi: 10.1073/pnas.0801962105
Driscoll, D. M., and Fong, J. M. Y. (1992). Continentality: a basic climatic parameter re-examined. Int. J. Climatol. 12, 185–192. doi: 10.1002/joc.3370120207
Drummond, A. J., and Rambaut, A. (2007). BEAST: Bayesian evolutionary analysis by sampling tress. Evol. Biol. 7, 214–221. doi: 10.1186/1471-2148-7-214
Dunai, T. J., López, G. A. G., and Juez-Larré, J. (2005). Oligocene–Miocene age of aridity in the Atacama Desert revealed by exposure dating of erosion-sensitive landforms. Geology 33, 321–324. doi: 10.1130/g21184.1
Eldredge, N., Thompson, J. N., Brakefield, P. M., Gavrilets, S., Jablonski, D., Jackson, J. B., et al. (2005). The dynamics of evolutionary stasis. Paleobiology 31, 133–145.
Evans, M. E., Smith, S. A., Flynn, R. S., and Donoghue, M. J. (2009). Climate, niche evolution, and diversification of the “bird-cage” evening primroses (Oenothera, sections Anogra and Kleinia). Am. Nat. 173, 225–240. doi: 10.1086/595757
Ezcurra, C. (2002). Phylogeny, morphology, and biogeography of Chuquiraga, an Andean-Patagonian genus of Asteraceae-Barnadesioideae. Bot. Rev. 68:153. doi: 10.1663/0006-8101(2002)068[0153:pmaboc]2.0.co;2
Garreaud, R., Barichivich, J., Christie, D. A., and Maldonado, A. (2008). Interannual variability of the coastal fog at Fray Jorge relict forests in semiarid Chile. J. Geophys. Res. Biogeosci. 113:G04011.
Garreaud, R. D., Molina, A., and Farias, M. (2010). Andean uplift, ocean cooling and Atacama hyperaridity: a climate modeling perspective. Earth Planet. Sci. Lett. 292, 39–50. doi: 10.1016/j.epsl.2010.01.017
Garzione, C. N., McQuarrie, N., Perez, N. D., Ehlers, T. A., Beck, S. L., Kar, N., et al. (2017). Tectonic evolution of the Central Andean plateau and implications for the growth of plateaus. Ann. Rev. Earth Planet. Sci. 45, 529–559. doi: 10.1146/annurev-earth-063016-020612
Gengler-Nowak, K. (2002). Reconstruction of the biogeographical history of Malesherbiaceae. Bot. Rev. 68, 171–188. doi: 10.1663/0006-8101(2002)068[0171:rotbho]2.0.co;2
Gentry, A. H. (1980). Bignoniaceae: part I (Crescentieae and tourrettieae). Flora Neotrop. 25, 1–130.
Gentry, A. H. (1992). Bignoniaceae: part II (tribe Tecomeae). Flora Neotrop. Monogr. 25, 1–370. doi: 10.5479/si.00810282.399
Giambiagi, L., Mescua, J., Bechis, F., Hoke, G., Suriano, J., Spagnotto, S., et al. (2016). “Cenozoic orogenic evolution of the Southern Central Andes (32–36°S),” in Growth of the Southern Andes, eds A. Folguera, M. Naipauer, L. Sagripanti, M. Ghiglione, D. Orts, and L. Giambiagi (Cham: Springer International Publishing).
Gleisner, G., and Ricardi, M. (1969). Revisión del genero Argylia (Bignoniaceae). Gayana Bot. 19, 3–62.
Guerrero, P. C., Rosas, M., Arroyo, M. T., and Wiens, J. J. (2013). Evolutionary lag times and recent origin of the biota of an ancient desert (Atacama–Sechura). Proc. Natl. Acad. Sci. 110, 11469–11474. doi: 10.1073/pnas.1308721110
Hall, T. A. (1999). BioEdit: a user-friendly biological sequence alignment editor and analysis program for windows 95/98/NT. Nucleic Acids Symp. 41, 95–98.
Hansen, T. F., Pienaar, J., and Orzack, S. H. (2008). A comparative method for studying adaptation to a randomly evolving environment. Evolution 62, 1965–1977.
Harmon, L. J., Weir, J. T., Brock, C. D., Glor, R. E., and Challenger, W. (2008). GEIGER: investigating evolutionary radiations. Bioinformatics 24, 129–131. doi: 10.1093/bioinformatics/btm538
Hartley, A., Chong, G., Houston, J., and Mather, A. E. (2005). 150 million years of climatic stability: evidence from the Atacama Desert, Northern Chile. J. Geol. Soc. 162, 421–424. doi: 10.1144/0016-764904-071
Hawkins, B. A., Rueda, M., Rangel, T. F., Field, R., and Diniz-Filho, J. A. F. (2014). Community phylogenetics at the biogeographical scale: cold tolerance, niche conservatism and the structure of North A merican forests. J. Biogeogr. 41, 23–38. doi: 10.1111/jbi.12171
Heibl, C. (2011). PHYLOCLIM: Integrating Phylogenetics and Climatic Niche Modelling. R Package Version 0.9-4 (online). Available online at: https://cran.rproject.org/web/packages/phyloclim/phyloclim.pdf (accessed: May 2016, 20).
Hinojosa, L. F. (2005). Cambios climaticos y vegetacionales inferidos a partir de paleofloras cenozoicas del sur de Sudamerica. Rev. Geol. Chile 32, 95–115.
Hinojosa, L. F., Gaxiola, A., Pérez, M. F., Carvajal, F., Campano, M. F., Quattrocchio, M., et al. (2016). Non-congruent fossil and phylogenetic evidence on the evolution of climatic niche in the Gondwana genus Nothofagus. J. Biogeogr. 43, 555–567. doi: 10.1111/jbi.12650
Hinojosa, L. F., and Villagrán, C. (1997). Historia de los bosques del sur de Sudamérica, I: antecedentes paleobotánicos, geológicos y climáticos del Terciario del cono sur de América. Rev. Chil. Hist. Nat. 70, 225–239.
Hueck, K., and Seibert, P. (1972). Vegetationskarte von Südamerika: Erläuterung zur Karte 1:8.000.000. Stuttgart: Gustav Fischer, 71.
Jara-Arancio, P., Arroyo, M. T. K., Guerrero, P. C., Hinojosa, L. F., Arancio, G., and Méndez, M. A. (2014). Phylogenetic perspectives on biome shifts in Leucocoryne (Alliaceae) in relation to climatic niche evolution in western South America. J. Biogeogr. 41, 328–338. doi: 10.1111/jbi.12186
Jara-Arancio, P., Vidal, P. M., Panero, J. L., Marticorena, A., Arancio, G., and Arroyo, M. T. (2017). Phylogenetic reconstruction of the South American genus Leucheria Lag. (Asteraceae, Nassauvieae) based on nuclear and chloroplast DNA sequences. Plant Syst. Evol. 303, 221–232. doi: 10.1007/s00606-016-1366-7
Karger, D. N., Conrad, O., Böhner, J., Kawohl, T., Kreft, H., Soria-Auza, R. W., et al. (2017). Climatologies at high resolution for the earth’s land surface areas. Sci. Data 4, 1–20. doi: 10.1080/17538947.2021.1966526
Kozak, K. H., and Wiens, J. J. (2007). Climatic zonation drives latitudinal variation in speciation mechanisms. Proc. R. Soc. Lond. B: Biol. Sci. 274, 2995–3003.
Latorre, C., Quade, J., and McIntosh, W. C. (1997). The expansion of C4 grasses and global change in the late Miocene: stable isotope evidence from the Americas. Earth Planet. Sci. Lett. 146, 83–96. doi: 10.1016/S0012-821X(96)00231-2
Little, S. A., Kembel, S. W., and Wilf, P. (2010). Paleotemperature proxies from leaf fossils reinterpreted in light of evolutionary history. PLoS One 5:e15161. doi: 10.1371/journal.pone.0015161
Lohmann, L. G. (2006). Untangling the phylogeny of neotropical lianas (Bignonieae, Bignoniaceae). Am. J. Bot. 93, 304–318. doi: 10.3732/ajb.93.2.304
Lohmann, L. G., Bell, C. D., Calió, M. F., and Winkworth, R. C. (2013). Pattern and timing of biogeographical history in the Neotropical tribe Bignonieae (Bignoniaceae). Bot. J. Linn. Soc. 171, 154–170. doi: 10.1111/j.1095-8339.2012.01311.x
Luebert, F., and Weigend, M. (2014). Phylogenetic insights into Andean plant diversification. Front. Ecol. Evol. 2:27. doi: 10.3389/fevo.2014.00027
Matzke, N. (2015). “Bayesian stochastic mapping for estimating biogeographic history on phylogenies,” in Proceedings of the IBS2015 International Biogeography Society 7th Biennial Meeting, (Bayreuth: University of Bayreuth).
Matzke, N. J. (2014). Model selection in historical biogeography reveals that founder-event speciation is a crucial process in island clades. Syst. Biol. 63, 951–970. doi: 10.1093/sysbio/syu056
Muscarella, R., Galante, P. J., Soley-Guardia, M., Boria, R. A., Kass, J. M., Uriarte, M., et al. (2014). ENM eval: an R package for conducting spatially independent evaluations and estimating optimal model complexity for Maxent ecological niche models. Methods Ecol. Evol. 5, 1198–1205. doi: 10.1111/2041-210X.12261
Nicolas, A. N., and Plunkett, G. M. (2012). Untangling generic limits in Azorella, Laretia, and Mulinum (Apiaceae: Azorelloideae): insights from phylogenetics and biogeography. Taxon 61, 826–840. doi: 10.1002/tax.614008
Nicolas, A. N., and Plunkett, G. M. (2014). Diversification times and biogeographic patterns in Apiales. Bot. Rev. 80, 30–58. doi: 10.1007/s12229-014-9132-4
Nylander, J. A. A. (2004). Mr Modeltest v2. Program Distributed by the Author. Uppsala: Evolutionary Biology Centre, Uppsala University.
Olmstead, R. G. (2013). Phylogeny and biogeography in Sola- naceae, Verbenaceae and Bignoniaceae: a comparison of continental and intercontinental diversification patterns. Bot. J. Linn. Soc. 171, 80–102. doi: 10.1111/j.1095-8339.2012.01306.x
Olmstead, R. G., Zjhra, M. L., Lohmann, L. G., Grose, S. O., and Eckert, A. J. (2009). A molecular phylogeny and classification of Bignoniaceae. Am. J. Bot. 96, 1731–1743. doi: 10.3732/ajb.0900004
Olson, D. M., Dinerstein, E., Wikramanayake, E. D., Burgess, N. D., Powell, G. V., Underwood, E. C., et al. (2001). Terrestrial ecoregions of the world: a new map of life on earth: a new global map of terrestrial ecoregions provides an innovative tool for conserving biodiversity. BioScience 51, 933–938. doi: 10.1641/0006-3568(2001)051[0933:TEOTWA]2.0.CO;2
Ortega, C., Vargas, G., Rutllant, J. A., Jackson, D., and Méndez, C. (2012). Major hydrological regime changes along the semiarid western coast of South America during the early Holocene. Quat. Res. 78, 513–527. doi: 10.1016/j.yqres.2012.08.002
Pagel, M. (1994). Detecting correlated evolution on phylogenies: a general method for the comparative analysis of discrete characters. R. Soc. Lond. B Biol. Sci. 255, 37–45. doi: 10.1098/rspb.1994.0006
Pagel, M. (1999). Inferring the historical patterns of biological evolution. Nature 401:877. doi: 10.1038/44766
Paradis, E., Claude, J., and Strimmer, K. (2004). APE: analyses of phylogenetics and evolution in R language. Bioinformatics 20, 289–290. doi: 10.1093/bioinformatics/btg412
Pérez, F., Lavandero, N., Ossa, C. G., Hinojosa, L. F., Jara-Arancio, P., and Arroyo, M. T. K. (2020). Divergence in plant traits and increased modularity underlie repeated transitions between low and high elevations in the Andean Genus Leucheria. Front. Plant Sci. 11:714. doi: 10.3389/fpls.2020.00714
Phillips, S. J., Anderson, R. P., and Schapire, R. E. (2006). Maximum entropy modeling of species geographic distributions. Ecol. Model. 190, 231–259. doi: 10.1016/j.ecolmodel.2005.03.026
Pigg, K. B., and Wehr, W. C. (2002). Tertiary flowers, fruits, and seeds of Washington state and adjacent areas-part III. Washington Geol. 30, 3–16.
Qin, D., Plattner, G. K., Tignor, M., Allen, S. K., Boschung, J., Nauels, A., et al. (2014). Climate Change 2013: The Physical Science Basis. Contribution of Working Group I to the Fifth Assessment Report of the Intergovernmental Panel on Climate Change, eds T. F. Stocker, D. Qin, G.-K. Plattner, M. Tignor, S. K. Allen, J. Boschung, et al. (Cambridge: Cambridge University Press), 5–14.
R Core Team (2021). R: A Language and Environment for Statistical Computing. Vienna: R Foundation for Statistical Computing.
Ramos, V. A., and Folguera, A. (2009). Andean flat-slab subduction through time. 327, 31–54. doi: 10.1144/SP327.3
Ramos, V. A., and Ghiglione, M. C. (2008). Tectonic evolution of the Patagonian Andes. Dev. Quat. Sci. 11, 57–71. doi: 10.1016/S1571-0866(07)10004-X
Ree, R. H., and Smith, S. A. (2008). Maximum likelihood inference of geographic range evolution by dispersal, local extinction, and cladogenesis. Syst. Biol. 57, 4–14. doi: 10.1080/10635150701883881
Regard, V., Martinod, J., Saillard, M., Carretier, S., Leanni, L., Hérail, G., et al. (2021). Late Miocene-quaternary forearc uplift in southern Peru: new insights from 10Be dates and rocky coastal sequences. J. South Am. Earth Sci. 109:103261. doi: 10.1016/j.jsames.2021.103261
Revell, L. J. (2012). phytools: an R package for phylogenetic comparative biology (and other things). Methods Ecol. Evol. 3, 217–223. doi: 10.1111/j.2041-210X.2011.00169.x
Rodriguez, R., Marticorena, C., Alarcón, D., Baeza, C., Cavieres, L., Finot, V., et al. (2018). Catálogo de las plantas vasculares de Chile. Gayana Bot. 75, 1–430. doi: 10.4067/S0717-66432018000100001
Ronquist, F., and Huelsenbeck, J. P. (2003). MrBayes 3: Bayesian phylogenetic inference under mixed models. Bioinformatics 19, 1572–1574. doi: 10.1093/bioinformatics/btg180
Ronquist, F., Teslenko, M., Van der Mark, P., Ayres, D. L., Darling, A., Hohna, S., et al. (2012). MrBayes 3.2: efficient Bayesian phylogenetic inference and model choice across a large model space. Syst. Biol. 61, 539–542. doi: 10.1093/sysbio/sys029
Rundel, P. W., Dillon, M. O., Palma, B., Mooney, H. A., Gulmon, S. L., and Ehleringer, J. R. (1991). The phytogeography and ecology of the coastal Atacama and Peruvian deserts. Aliso 13, 1–49. doi: 10.5642/aliso.19911301.02
Scherson, R. A., Thornhill, A. H., Urbina-Casanova, R., Freyman, W. A., Pliscoff, P. A., and Mishler, B. D. (2017). Spatial phylogenetics of the vascular flora of Chile. Mol. Phylogenet. Evol. 112, 88–95. doi: 10.1016/j.ympev.2017.04.021
Schluter, D., Price, T., Mooers, A. Ø, and Ludwig, D. (1997). Likelihood of ancestor states in adaptive radiation. Evolution 51, 1699–1711. doi: 10.1111/j.1558-5646.1997.tb05095.x
Shaw, J., Lickey, E. B., Schilling, E. E., and Small, R. L. (2007). Comparison of whole chloroplast genome sequences to choose noncoding regions for phylogenetic studies in angiosperms: the tortoise and the hare III. Am. J. Bot. 94, 275–288. doi: 10.3732/ajb.94.3.275
Simpson, B. B., Arroyo, M. T., Sipe, S., Dias de Moraes, M., and McDILL, J. (2009). Phylogeny and evolution of Perezia (Asteraceae: mutisieae: nassauviinae). J. Syst. Evol. 47, 431–443. doi: 10.1111/j.1759-6831.2009.00039.x
Stebbins, G. L. Jr. (1952). Aridity as a stimulus to plant evolution. Am. Nat. 86, 33–44. doi: 10.1086/281699
Stevens, W. D., Ulloa, U. C., Pool, A., and Montiel, O. M. (2001). “Verbenaceae,” in Flora de Nicaragua. Monographs in Systematic Botany from the Missouri Botanical Garden, Vol. 85, eds W. D. Stevens, C. U. Ulloa, A. Pool, and O. M. Montiel (St. Louis, MO: Missouri Botanical Garden), 2497–2525.
Taberlet, P., Gielly, L., Pautou, G., and Bouvet, J. (1991). Universal primers for amplification of three non-coding regions of chloroplast DNA. Plant Mol. Biol. 17, 1105–1109. doi: 10.1007/BF00037152
Veit, H., and Garleff, K. (1995). “Evolución del paisaje cuaternario y los suelos en Chile central-sur,” in Ecologia de los Bosques nativos de Chile, eds J. J. Armesto, C. Villagran, and M. K. Arroyo (Santiago: Santiago de Chile).
Villagrán, C., and Hinojosa, L. F. (1997). Historia de los bosques del sur de Sudamérica, II: análisis fitogeográfico. Rev. Chil. Hist. Nat. 70, 1–267.
Villagrán, C., León, A., and Roig, F. A. (2004). Paleodistribución del alerce y ciprés de las Guaitecas durante períodos interestadiales de la Glaciación Llanquihue: provincias de Llanquihue y Chiloé, Región de Los Lagos, Chile. Rev. Geol. Chile 31, 133–151. doi: 10.4067/S0716-02082004000100008
White, T. J., Bruns, T., Lee, S. J. W. T., and Taylor, J. (1990). “Amplification and direct sequencing of fungal ribosomal RNA genes for phylogenetics,” in PCR Protocols: A Guide to Methods and Applications, Vol. 18, eds M. A. Innis, D. H. Gelfand, J. J. Sninsky, and T. J. White (New York, NY: Academic Press), 315–322. doi: 10.1016/B978-0-12-372180-8.50042-1
Wiens, J. J., Ackerly, D. D., Allen, A. P., Anacker, B. L., Buckley, L. B., Cornell, H. V., et al. (2010). Niche conservatism as an emerging principle in ecology and conservation biology. Ecol. Lett. 13, 1310–1324. doi: 10.1111/j.1461-0248.2010.01515.x
Zachos, J., Pagani, M., Sloan, L., Thomas, E., and Billups, K. (2001). Trends, rhythms, and aberrations in global climate 65 Ma to present. Science 292, 686–693. doi: 10.1126/science.1059412
Keywords: Andes, diversity, phylogeny, niche conservatism, Bignoniaceae
Citation: Glade-Vargas NS, Rojas C, Jara-Arancio P, Vidal P, Arroyo MTK and Hinojosa LF (2021) Biogeography of Argylia D. Don (Bignoniaceae): Diversification, Andean Uplift and Niche Conservatism. Front. Plant Sci. 12:724057. doi: 10.3389/fpls.2021.724057
Received: 11 June 2021; Accepted: 20 September 2021;
Published: 19 October 2021.
Edited by:
Stefan Wanke, Technische Universität Dresden, GermanyReviewed by:
Maximilian Weigend, University of Bonn, GermanyMarie-Stéphanie Samain, Instituto de Ecología (INECOL), Mexico
Copyright © 2021 Glade-Vargas, Rojas, Jara-Arancio, Vidal, Arroyo and Hinojosa. This is an open-access article distributed under the terms of the Creative Commons Attribution License (CC BY). The use, distribution or reproduction in other forums is permitted, provided the original author(s) and the copyright owner(s) are credited and that the original publication in this journal is cited, in accordance with accepted academic practice. No use, distribution or reproduction is permitted which does not comply with these terms.
*Correspondence: Nataly S. Glade-Vargas, TmF0YWx5LmdsYWRlQHVnLnVjaGlsZS5jbA==; Luis Felipe Hinojosa, bGZoaW5vam9zYUB1Y2hpbGUuY2w=