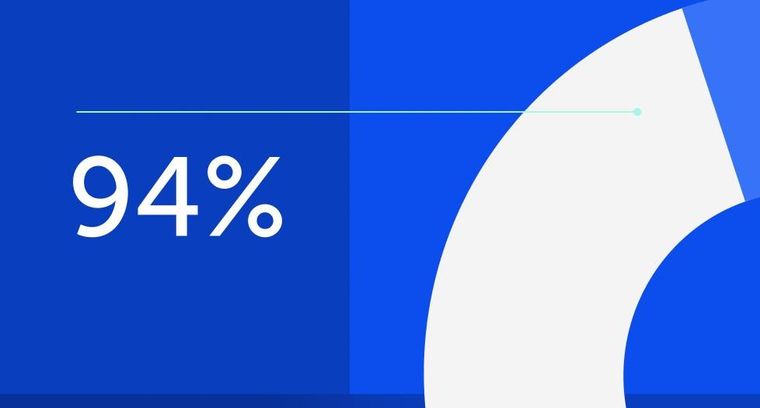
94% of researchers rate our articles as excellent or good
Learn more about the work of our research integrity team to safeguard the quality of each article we publish.
Find out more
MINI REVIEW article
Front. Plant Sci., 05 August 2021
Sec. Plant Metabolism and Chemodiversity
Volume 12 - 2021 | https://doi.org/10.3389/fpls.2021.712022
This article is part of the Research TopicThe Back-and-Forth between Metabolism and Photosystem IView all 7 articles
Plant productivity is determined by the conversion of solar energy into biomass through oxygenic photosynthesis, a process performed by protein-cofactor complexes including photosystems (PS) II and I, and ATP synthase. These complexes are embedded in chloroplast thylakoid membrane lipids, which thus function as structural support of the photosynthetic machinery and provide the lipid matrix to avoid free ion diffusion. The lipid and fatty acid composition of thylakoid membranes are unique in chloroplasts and cyanobacteria, which implies that these molecules are specifically required in oxygenic photosynthesis. Indeed, there is extensive evidence supporting a relevant function of glycerolipids in chloroplast biogenesis and photosynthetic efficiency in response to environmental stimuli, such as light and temperature. The rapid acclimation of higher plants to environmental changes is largely based on thiol-based redox regulation and the disulphide reductase activity thioredoxins (Trxs), which are reduced by ferredoxin (Fdx) via an Fdx-dependent Trx reductase. In addition, chloroplasts harbour an NADPH-dependent Trx reductase C, which allows the use of NADPH to maintain the redox homeostasis of the organelle. Here, we summarise the current knowledge of chloroplast lipid metabolism and the function of these molecules as structural basis of the complex membrane network of the organelle. Furthermore, we discuss evidence supporting the relevant role of lipids in chloroplast biogenesis and photosynthetic performance in response to environmental cues in which the redox state of the organelle plays a relevant role.
In oxygenic photosynthesis, ferredoxin (Fdx), at the stromal side of photosystem I (PSI), functions as a mobile carrier distributing reducing equivalents from the photosynthetic electron transport chain to produce NADPH, via Fdx-NADP-reductase (FNR), or to thioredoxins (Trx), via Fdx-Trx-reductase (FTR; Cejudo et al., 2019). Trxs participate in the light-dependent reductive activation of biosynthetic enzymes, including Calvin-Benson cycle enzymes (Michelet et al., 2013), among other processes. In addition, chloroplasts harbour a NADPH-dependent Trx reductase termed NTRC (Serrato et al., 2004). NTRC is an efficient reductant of 2-Cys peroxiredoxin (Prx; Moon et al., 2006; Pérez-Ruiz et al., 2006; Pérez-Ruiz and Cejudo, 2009), which suggested an antioxidant function for the enzyme. However, the Arabidopsis mutant devoid of NTRC shows impaired light-dependent reduction of enzymes of the Calvin-Benson cycle (Thormählen et al., 2015; Ojeda et al., 2017), indicating a function in redox regulation for the enzyme. More recently, it was proposed that NTRC and 2-Cys Prxs form a redox relay that modulates the reducing capacity of Trxs allowing the light-dependent activation of their downstream targets (Pérez-Ruiz et al., 2017). Thus, a tight functional relationship exists between chloroplast redox regulation and thiol-dependent antioxidant systems (Cejudo et al., 2021).
Chloroplast thylakoid membranes have a characteristic lipid composition and content of unsaturated fatty acids. Lipids have a well-established structural function serving as matrix for the photosynthetic complexes and allowing the compartmentalisation of the organelle, but also affect chloroplast biogenesis and photosynthetic performance. Environmental cues, such as light and temperature changes, affect chloroplast lipids biosynthesis and fatty acids desaturation, a process influenced by the redox state of the organelle (Geigenberger and Fernie, 2014; Yu et al., 2020). In this review, we summarise the lipid biosynthetic pathways in the chloroplast and the current knowledge of the role of lipids in chloroplast biogenesis and performance. The function of lipids in thylakoid membrane biogenesis and the organisation of membrane-associated processes during chloroplast differentiation is discussed. Moreover, the role of the unsaturated fatty acid content of thylakoid membranes on the photosynthetic activity in response to environmental cues is also updated. Finally, we discuss the redox regulatory mechanisms that control lipids biosynthesis and fatty acids desaturation, thus coordinating the redox state with chloroplast performance and plant growth.
De novo plastid fatty acid biosynthesis is initiated by the ATP consuming conversion of acetyl-CoA and CO2 to malonyl-CoA catalysed by acetyl-CoA carboxylase (ACCase). Malonyl-CoA is the substrate of the fatty acid synthase (FAS) complex, which performs the consecutive condensation of acetyl-CoA units to generate palmitoyl-acyl carrier protein (16:0-ACP) and stearoyl-ACP (18:0-ACP) as main products (Ohlrogge and Browse, 1995; Figure 1). Fatty acid desaturation is initiated by the soluble stearoyl-ACP desaturase (SAD) that forms oleoyl-ACP (18:1-ACP), as main product of plastid fatty acid biosynthesis. Fatty acids are then incorporated into the two plant glycerolipids biosynthesis pathways, prokaryotic- and eukaryotic-type (Figure 1). The prokaryotic-type pathway is exclusive of plastids and involves the synthesis of phosphatidylglycerol (PG), and the glycolipids monogalactosyldiacylglycerol (MGDG), digalactosyldiacylglycerol (DGDG) and sulfoquinovosyldiacylglycerol (SQDG). Alternatively, fatty acids can be exported to the cytosol and incorporated into the eukaryotic-type pathway. Part of the glycerolipids assembled in the endoplasmic reticulum return to the plastid to serve as substrates for glycolipids synthesis (Li-Beisson et al., 2013; Figure 1). In both pathways, fatty acids can be further desaturated by the membrane-bound fatty acid desaturases (FAD). Chloroplast FADs use glycerolipids as substrates and reduced Fdx, produced by the photosynthetic electron transport chain in the light or from NADPH in the dark, as electron donor (Shanklin and Cahoon, 1998; Figure 2).
Figure 1. Pathways of glycerolipids biosynthesis in Arabidopsis. De novo synthesised fatty acids in chloroplasts are incorporated into lipids via two pathways. The prokaryotic-type pathway takes place exclusively in the chloroplast (dotted box), the eukaryotic-type pathway takes place in the endoplasmic reticulum and the chloroplast using the glycerol backbone assembled in the endoplasmic reticulum. The synthesis of polyunsaturated fatty acids is catalysed by membrane-bound fatty acid desaturases (FADs) in both cell compartments. ACCase, Acetyl-CoA carboxylase; DAG, diacylglycerol; DGDG, digalactosyldiacylglycerol; DGD, DGDG synthase; FADs, membrane-bound fatty acid desaturases; FAS, Fatty acid synthase complex; MGDG, monogalactosyldiacylglycerol; MGD, MGDG synthase; PG, phosphatidylglycerol; SAD, stearoyl-ACP desaturase; SQDG, sulfoquinovosyldiacylglycerol; and SQD, SQDG synthase.
Figure 2. Redox control of chloroplast lipid metabolism. Fdx, the final acceptor of the photosynthetic electron transport chain, acts as a hub distributing reducing equivalents for the formation of NADPH via FNR, to enzymes involved in fatty acid desaturation, SAD and FADs and to Trxs via FTR. Initial reactions of lipid biosynthesis catalysed by ACCase and MGDG synthase undergo Trx-dependent redox regulation. The reductive capacity of chloroplast Trxs is balanced by the NTRC-2-Cys Prxs redox relay, which uses NADPH as source of reducing power and hydrogen peroxide as final sink of electrons. An additional chloroplast Prx, Prx Q, affects FAD4 activity at thylakoid membrane, though the molecular basis of this effect is not yet known. ACCase, Acetyl-CoA carboxylase; 2CP, 2-Cys Prx; FAD4, phosphatidylglycerol desaturase (palmitate specific); FAD6, chloroplast omega-6 desaturase; FAD7/8, chloroplast omega-3 desaturases; FAS, Fatty acid synthase complex; FNR, Fdx-NADP reductase; FTR, Fdx-dependent Trx reductase; Fdx, ferredoxin; MGDG, monogalactosyldiacylglycerol; NTRC, NADPH-dependent Trx reductase C; Prx, peroxiredoxin; SAD, stearoyl-ACP desaturase; and Trx, thioredoxin.
The fact that the lipid composition of thylakoid membranes is unique and highly conserved suggests a specific requirement of these lipids for the structure and function of photosynthetic complexes (Petroutsos et al., 2014). The most abundant lipids in thylakoid membranes are galactolipids MGDGs and DGDGs, which account for about 50 and 25% of total thylakoid lipids, respectively (Douce and Joyard, 1996), the sulfolipids SQDGs and the phospholipids PGs. MGDGs are non-bilayer forming lipids with conical shape that participate in thylakoid curvature, whereas DGDGs have cylindrical shape, are bilayer forming and were proposed to stabilise the membrane lipid bilayer (Jouhet, 2013; Garab et al., 2017). Thus, for photosynthetic membranes stability, the MGDG/DGDG ratio is crucial (Demé et al., 2014). SQDGs and PGs are bilayer forming lipids that contain negatively charged head groups. The amount of total anionic lipids in the thylakoid membranes is strictly maintained by compensation between SQDGs and PGs contents. X-ray crystallographic studies identified integral glycolipids specifically bound to the core proteins of photosynthetic complexes that participate in protein-protein and protein-cofactors interactions (Kern et al., 2009). These findings support the notion that, besides providing the structural matrix for photosynthetic complexes, lipids participate in the formation of the photosynthetic machinery during chloroplast biogenesis.
MGDGs, the most abundant glycolipids in thylakoid membranes, are essential for chloroplast structure and photosynthetic performance (Jarvis et al., 2000; Kobayashi et al., 2007). MGDGs synthesis is catalysed by MGDG synthases (MGD); then, DGDGs are synthesised by the galactosylation of MGDGs catalysed by DGDG synthases (DGD; Figure 1; Boudière et al., 2014). Arabidopsis mutants with residual amounts of DGDGs show impaired photosynthetic performance (Kelly et al., 2003) and PSI alteration (Ivanov et al., 2006), while mutants deficient in MGDGs show impaired formation and maintenance of PSI and PSII (Kobayashi et al., 2013; Fujii et al., 2014), indicating their relevant role in chloroplast structure and function. This notion was further confirmed by in vitro studies showing the specific requirement of MGDGs for the ordered oligomerization of light harvesting complex II (LHCII; Schaller et al., 2011), the dimerisation of PSII (Kansy et al., 2014) and the coupling between LHCII and PSII core complexes (Zhou et al., 2009).
An additional effect of thylakoid lipids is exerted on gene expression as shown by the impaired expression of plastid and nuclear photosynthesis-related genes in the Arabidopsis mgd1-2 mutant (Kobayashi et al., 2013). Thus, a transcriptional link between the synthesis of chlorophyll, photosynthetic proteins, and galactolipids seems to be essential for the organisation of the thylakoid membrane networks (Kobayashi et al., 2014). Consistent with these data, the expression of MGD1 gene was downregulated in Arabidopsis PG deficient mutants, which failed to develop functional chloroplasts (Kobayashi et al., 2015). Thus, MGD1 activity might link galactolipid synthesis with chloroplast transcriptional regulation during cotyledon greening (Fujii et al., 2014). Once MGDGs are properly synthesised during chloroplast biogenesis, the development of chloroplast progresses even if MGD1 expression is inhibited afterwards, indicating that MGDGs synthesis is essential at early stages of the process (Fujii et al., 2014).
The etioplast-to-chloroplast differentiation upon illumination involves the transformation of prolamellar bodies (PLBs) and prothylakoids of etioplasts in fully organised thylakoid membranes (Solymosi and Schoefs, 2010; Kowalewska et al., 2016, 2019). Etioplasts lack chlorophyll but accumulate the chlorophyll precursor protochlorophyllide (Pchlide; Lebedev et al., 1985; Schoefs, 2001) and have similar lipid composition than thylakoid membranes (Selstam and Sandelius, 1984). Galactolipids have different functions in chlorophyll biosynthesis and organisation of light-harvesting complexes during the etioplast-to-chloroplast transition (Fujii et al., 2019b). MGDGs are involved in Mg-Proto IX metabolism for Pchlide biosynthesis and are essential for the formation of Pchlide-LPOR-NADPH ternary complexes in PLBs, which are responsible for the Pchlide to Childe conversion upon illumination (Fujii et al., 2017, 2019a). On the other hand, DGDGs are required for the conversion of Mg-Proto IX methyl ester to Pchlide (Fujii et al., 2018). Furthermore, MGDGs, but not DGDGs, enhance the oligomerization of the Pchlide-LPOR complex, whereas DGDGs play a specific role in the dissociation of Childe-LPOR complex and the formation of PLBs structure (Gabruk et al., 2017; Fujii et al., 2018). Galactolipids have also different contributions to the development of the thylakoid membranes during chloroplast differentiation. The Arabidopsis mgd1 knockout mutant shows totally blocked thylakoid biogenesis indicating that MGDGs are necessary for grana formation and stacking (Kobayashi et al., 2007). On the other hand, although DGDGs also contribute to grana stacking, the Arabidopsis dgd1-2 mutant, severely deficient in DGDGs, exhibits a slow development of bent thylakoids with highly stacked membranes (Mazur et al., 2019). Moreover, DGDGs have a critical role as galactolipid zipper during membrane stacking (Demé et al., 2014). It should be noted that the lack of MGD1 affects DGDGs, which are synthesised from MGDGs. Therefore, both galactolipids have essential but different roles during chloroplast biogenesis.
Anionic thylakoid glycerolipids, PGs and SQDGs, also play an important role in chloroplast biogenesis acting as allosteric regulators in the formation of Pchlide-LPOR-NADPH complexes (Gabruk et al., 2017). Analysis of Arabidopsis mutants impaired in PGs biosynthesis revealed the essential role of these lipids in thylakoid membrane formation (Hagio et al., 2002; Babiychuk et al., 2003). Moreover, PGs deficiency affects electron transfer from antenna pigments to the PSII reaction centre (Kobayashi et al., 2016). These in vivo data agree with in vitro studies suggesting that depletion of PGs in thylakoid membranes impairs the function of the photosynthetic complexes (Siegenthaler et al., 1987; Kruse et al., 2000). The role of PGs cannot be substituted by glycolipids (Kobayashi et al., 2015, 2016), except under specific environmental conditions, such as phosphate starvation (Benning et al., 1993; Güler et al., 1996). Under standard growth conditions, SQDGs are not essential since the sqd2 mutant showed normal growth and photosynthetic parameters (Yu et al., 2002). However, under phosphate limitation SQDGs substitute anionic phospholipids (PGs) to maintain the negative charge at the lipid-water interface. Under these conditions, the total content of anionic thylakoid lipids becomes limiting for chloroplast structure and function (Yu and Benning, 2003).
Lipids of thylakoid membranes have also an unusual and characteristic fatty acid composition. Trienoic fatty acids (16:3 and 18:3) represent 60–70% of total fatty acids in thylakoids and more than 90% of the fatty acids in MGDGs, the most abundant chloroplast lipids. In addition, the atypical fatty acid Δ3-trans-hexadecenoate (16:1Δ3t) is present as a component of PGs, the major phospholipid in thylakoid membranes (Li-Beisson et al., 2013). Fatty acid unsaturation is determined by the activity of fatty acid desaturases (FADs), which introduce double bonds into specific acyl chain positions. Interestingly, most of the mutants affected in FADs show wild-type phenotype when grown under standard conditions (Wallis and Browse, 2002); in fact, 16:1Δ3t and trienoic fatty acids could be eliminated without any significant effect on photosynthesis and plant growth (Wallis and Browse, 2002). However, some mutant phenotypes that become evident under stressful conditions suggest a role of thylakoid fatty acid composition in photoinhibition.
PSII function requires the fine adjustment between D1 inactivation and replacement at the core of PSII (Baena-González and Aro, 2002). While D1 photo-damage is highly dependent on light intensity (Anderson and Chow, 2002), the recovery process depends on temperature and is highly affected by the level of unsaturation of thylakoid membrane lipids (Los et al., 2013). Temperature affects the fluidity of chloroplast membranes, which can be compensated by changes in the level of the unsaturation of their fatty acids: cold causes membrane rigidity, which can be alleviated by increasing unsaturation, whereas heat causes fluidisation, which can be amended by replacement of unsaturated fatty acids by de novo synthesised saturated ones (Falcone et al., 2004).
The degree of fatty acid unsaturation affects chilling sensitivity in Nicotiana tabacum leaves, the higher the degree of unsaturation, the lower the chilling sensitivity (Murata et al., 1992). These results were further confirmed by the finding that up to 88% of high-melting point fatty acids (16:0+18:0+16:1Δ3t) in PGs does not affect D1 inactivation but is important for its recovery after low-temperature photoinhibition (Moon et al., 1995). Later, Vijayan and Browse (2002) suggested that the level of high-melting point fatty acids is tightly correlated with recovery from photoinhibition at temperatures lower than 27°C. A threshold level of these PG fatty acids may be required for photoinhibition since no detectable differences between fab1 plants, with 69% of high-melting point PG fatty acids, and wild-type plants, with only 55%, were found. Similarly, the level of trienoic fatty acids in chloroplast lipids has been related to photoinhibition recovery at low temperatures (Vijayan and Browse, 2002). Although the Arabidopsis fad3-2fad7-2fad8 mutant, lacking 18:3 and 16:3 fatty acids, has only subtle effects on photosynthetic performance at temperatures as low as 5°C in the short-term, prolonged incubation at low temperature provoked a severe effect, revealing the essential role of trienoic fatty acids in photosynthetic capacity at low temperature (Routaboul and Browse, 2000). Consistent with these results, transgenic tomato plants overexpressing plastid omega-3 desaturase, hence having increased 18:3/18:2 ratio, showed alleviated photoinhibition under chilling conditions and higher tolerance to low temperature (Liu et al., 2008; Domínguez et al., 2010). Interestingly, analysis of the fad3-2fad7-1fad8 triple mutant, with decreased contents of leaf trienoic fatty acids, exhibited wild-type levels of quantum yield of electron transfer (ɸII) at 4°C (Routaboul and Browse, 2000). These results highlight the importance of trienoic fatty acids for chloroplast response to low temperatures, beyond maintenance of membrane fluidity. Moreover, Barkan et al. (2006) described a fab1 suppressor line that could survive after 16 weeks at 2°C. This line was an allele of the fad5 containing 31% of 16:0 compared to 23% in fab1 and 17% in wild type. Thus, the suppressed line does not behave as expected since the increase in saturated fatty acids in the fab1fad5-2 double mutant would increase sensitivity to low temperature. To explain these surprising results, it was suggested that the suppressor phenotype could be caused by a change in lipids molecular shape, which is important for several membrane functions (Wilhelm et al., 2020).
The level of thylakoid lipids unsaturation is also important for plants tolerance to high temperatures as shown by the enhanced thermotolerance of Arabidopsis mutants with reduced contents of polyunsaturated fatty acids in thylakoid membranes (Hugly et al., 1989; Routaboul et al., 2012). Similar results were obtained in FAD7 silenced N. benthamiana leaves containing higher dienoic to trienoic fatty acids ratio, which show better photosynthesis performance at high temperature (Murakami et al., 2000; Hiremath et al., 2017). Routaboul et al. (2012) reported a close correlation of the thermal damage to the O2-evolving complex with the level of 16:3 fatty acid since mutants with lower amount of 16:3 fatty acids in thylakoid membranes lipids showed higher thermotolerance.
Altogether, these results indicate that the degree of chloroplast lipid desaturation plays a key role in plant acclimation to temperature changes; however, the relationship between thylakoid lipids, photosynthetic performance and temperature is highly complex and the molecular mechanism underlying this relationship remains to be elucidated. Evidence of chloroplast FAD regulation by temperature has been reported. The fad7 mutant shows unaltered trienoic fatty acids at temperatures below 20°C (Browse et al., 1986), which was proposed to be due to a compensation effect exerted by the induction of the FAD8 gene at low temperature (McConn et al., 1994; Román et al., 2015). Similarly, high temperatures affected FAD8 activity more severely than FAD7 activity (Román et al., 2015), though in this case a destabilisation of FAD8 protein was reported (Matsuda et al., 2005). Therefore, FAD8 regulation at either transcriptional or post-transcriptional levels could play a significant role in plant response to temperature. Interestingly, FAD8 showed higher specificity for PGs (Román et al., 2015), which are essential for photosynthetic complexes arrangement, development of thylakoid membranes and tolerance to chilling (Wada and Murata, 2007).
The first committed step of fatty acid biosynthesis is catalysed by plastid ACCase, a multienzyme complex composed of biotin carboxyl carrier protein (BCPP), biotin carboxylase (BC) and carboxyltransferases (CT)-α and -β subunits (Sasaki et al., 1993). Different mechanisms participate in the control of ACCase activity by light, such as changes in stromal pH and Mg2+ (Sasaki et al., 1997; Ye et al., 2020a), CT-β mRNA editing (Sasaki et al., 2001), ‘envelope-docking’ (Ye et al., 2020b) and redox regulation (Sasaki and Nagaro, 2004). Moreover, ACCase is also regulated in response to long-term changes in light intensity (Yu et al., 2020), evidencing that the first step of fatty acid, which controls carbon flow into the pathway, is highly regulated (Figure 2). In pea leaves, CT activity of ACCase is redox regulated (Sun et al., 1997; Kozaki and Sasaki, 1999) resulting in light-dependent activation of fatty acid synthesis, with Trx f playing a more relevant role that Trx m in this regulatory mechanism (Sasaki et al., 1997). Site-directed mutagenesis revealed the formation of a disulphide linking Cys-267 in CT-α and Cys-442 in CT-β subunits (Kozaki et al., 2001). Interestingly, while Cys-267 is highly conserved, Cys-442 is not conserved in plants, such as spinach (Hunter and Ohlrogge, 1998) and tobacco (Ohlrogge et al., 1993), hence suggesting that an additional Cys is involved in this redox regulatory mechanism. In Arabidopsis, no redox regulation of ACCase has been described so far; however, the recent identification of the ACCase CT-β subunit as partner of NTRC suggested a putative role of the NTRC/2-Cys Prx system in the redox regulation of lipid biosynthesis in this species (González et al., 2019). Redox regulation also extends to other metabolic reactions of lipid metabolism. This is the case of galactolipid biosynthesis since MGDG synthase undergoes Trx-dependent redox regulation (Shijojima et al., 2013; Yamaryo et al., 2006; Figure 2), thus, linking the role of galactolipids in thylakoid structural reorganisations to light (Yu et al., 2020).
Recently, Horn et al. (2020) reported that FAD4 activity requires Prx Q to produce wild-type levels of 16:1Δ3t in Arabidopsis, although redox regulation of the enzyme was discarded. It is unlikely that Prx Q acts as electron donor for FAD4 because it does not contain any FeS cluster as Fdx, the reported electron donor for chloroplast FADs (Shanklin and Cahoon, 1998). An additional possibility is that Prx Q protects FAD4 enzyme from oxidative stress, given the role of PG 16:1Δ3t preventing photoinhibition under temperature stress (Murata et al., 1992; Moon et al., 1995). In fact, the protective role of antioxidant enzymes on photosynthesis under high temperature has been reported (Almeselmani et al., 2006) and, consequently, maintaining a redox homeostasis is an important common regulatory pathway for plant tolerance to temperature stress (Yuan et al., 2019).
The content of lipids and fatty acids in chloroplast thylakoid membranes is unique of this organelle and different from other plant cell compartments, which indicates a major role of lipids in chloroplast structure and function. In addition to the structural function of lipids to maintain the complex membranous network of chloroplasts, in this review, we have summarised evidence supporting an important role of lipids in the formation of photosynthetic complexes, which affects chloroplast biogenesis. Moreover, thylakoid membrane lipids have a deep effect on the photochemical reactions of photosynthesis. As sessile organisms, plant growth is highly affected by environmental cues, such as light and temperature, and there is strong evidence in support of the relationship of the degree of chloroplast fatty acid unsaturation with plant acclimation to temperature changes. Therefore, the complex pathways of chloroplast lipid and fatty acid biosynthesis are tightly regulated both at the transcriptional and post-transcriptional levels. Since Fdx is the electron donor for SAD and FAD activities, the redox state of the organelle directly affects the degree of fatty acid unsaturation. It was recently shown that Prx Q affects FAD4 activity; however, the mechanism of this effect is still poorly understood. Similarly, it has been shown that ACCase and MGDG synthase undergo Trx-mediated redox regulation, suggesting an important role of light in the regulation of initial steps of chloroplast lipid biosynthesis, though the molecular basis of this regulatory mechanism is not yet fully understood. The progress in the understanding of the mechanisms of control of chloroplast redox homeostasis and its relationship with thiol-dependent antioxidant systems, as well as the availability of Arabidopsis mutants affected in chloroplast redox regulation, provides an excellent opportunity to progress in elucidating the mechanisms regulating chloroplast lipid biosynthesis in response to environmental cues.
MLH and FJC contributed to the conception of the study. MLH drafted the manuscript. All authors revised, edited and approved the final submitted version.
Work in our lab is supported by FEDER/Ministerio de Ciencia e Innovación – Agencia Estatal de Investigación/ _Proyecto (BIO2017-85195-C2-1-P). MLH is the recipient of a research contract from the VPPI-US.
The authors declare that the research was conducted in the absence of any commercial or financial relationships that could be construed as a potential conflict of interest.
All claims expressed in this article are solely those of the authors and do not necessarily represent those of their affiliated organizations, or those of the publisher, the editors and the reviewers. Any product that may be evaluated in this article, or claim that may be made by its manufacturer, is not guaranteed or endorsed by the publisher.
Almeselmani, M., Deshmukh, P. S., Sairam, R. K., Kushwaha, S. R., and Singh, T. P. (2006). Protective role of antioxidant enzymes under high temperature stress. Plant Sci. 171, 382–388. doi: 10.1016/j.plantsci.2006.04.009
Anderson, J. M., and Chow, W. S. (2002). Structural and functional dynamics of plant photosystem II. Philos. Trans. R. Soc. Lond. Ser. B Biol. Sci. 357, 1421–1430. doi: 10.1098/rstb.2002.1138
Babiychuk, E., Müller, F., Eubel, H., Braun, H.-P., Frentzen, M., and Kushnir, S. (2003). Arabidopsis phosphatidylglycerophosphatesynthase1 is essential for chloroplast differentiation, but is dispensable for mitochondrial function. Plant J. 33, 899–909. doi: 10.1046/j.1365-313X.2003.01680.x
Baena-González, E., and Aro, E. M. (2002). Biogenesis, assembly and turn-over of photosystem II units. Philos. Trans. R. Soc. Lond. Ser. B Biol. Sci. 357, 1451–1460. doi: 10.1098/rstb.2002.1141
Barkan, L., Vijayan, P., Carlsson, A. S., Mekhedov, S., and Browse, J. (2006). A suppressor of fab1 challenges hypotheses on the role of thylakoid unsaturation in photosynthetic function. Plant Physiol. 141, 1012–1020. doi: 10.1104/pp.106.080481
Benning, C., Beatty, J. T., Prince, R. C., and Somerville, C. R. (1993). The sulfolipid SQDG is not required for photosynthetic electron transport in Rhodobacter sphaeroides but enhances growth under phosphate limitation. Proc. Natl. Acad. Sci. 90, 1561–1565. doi: 10.1073/pnas.90.4.1561
Boudière, L., Michaud, M., Petroutsos, D., Rébeillé, F., Falconet, D., Bastien, O., et al. (2014). Glycerolipids in photosynthesis: composition, synthesis and trafficking. Biochim. Biophys. Acta 1837, 470–480. doi: 10.1016/j.bbabio.2013.09.007
Browse, J., McCourt, P., and Somerville, C. R. (1986). A mutant of Arabidopsis deficient in C18:3 and C16:3 leaf lipids. Plant Physiol. 81, 859–864. doi: 10.1104/pp.81.3.859
Cejudo, F. J., González, M.-C., and Pérez-Ruiz, J. M. (2021). Redox regulation of chloroplast metabolism. Plant Physiol. 186, 9–21. doi: 10.1093/plphys/kiaa062
Cejudo, F. J., Ojeda, V., Delgado-Requerey, V., González, M., and Pérez-Ruiz, J. M. (2019). Chloroplast redox regulatory mechanisms in plant adaptation to light and darkness. Front. Plant Sci. 10:380. doi: 10.3389/fpls.2019.00380
Demé, B., Cataye, C., Block, M. A., Maréchal, E., and Jouhet, J. (2014). Contribution of galactoglycerolipids to the 3-dimensional architecture of thylakoids. FASEB J. 28, 3373–3383. doi: 10.1096/fj.13-247395
Domínguez, T., Hernández, M. L., Pennycooke, J. C., Jiménez, P., Martínez-Rivas, J. M., Sanz, C., et al. (2010). Increasing ω-3 desaturase expression in tomato results in altered aroma profile and enhanced resistance to cold stress. Plant Physiol. 153, 655–665. doi: 10.1104/pp.110.154815
Douce, R., and Joyard, J. (1996). “Biosynthesis of thylakoid membrane lipids,” in Advances in Photosynthesis/Oxygenic Photosynthesis: the Light Reactions. eds. D. R. Ort and C. F. Yocum (Springer, Dordrecht), 69–101.
Falcone, D. L., Ogas, J. P., and Somerville, C. R. (2004). Regulation of membrane fatty acid composition by temperature in mutants of Arabidopsis with alterations in membrane lipid composition. BMC Plant Biol. 4:17. doi: 10.1186/1471-2229-4-17
Fujii, S., Kobayashi, K., Nagata, N., Masuda, T., and Wada, H. (2017). Monogalactosyldiacylglycerol facilitates synthesis of photoactive protochlorophyllide in etioplasts. Plant Physiol. 174, 2183–2198. doi: 10.1104/pp.17.00304
Fujii, S., Kobayashi, K., Nagata, N., Masuda, T., and Wada, H. (2018). Digalactosyldiacylglycerol is essential for organization of the membrane structure in etioplasts. Plant Physiol. 177, 1487–1497. doi: 10.1104/pp.18.00227
Fujii, S., Kobayashi, K., Nakamura, Y., and Wada, H. (2014). Inducible knockdown of MONOGALACTOSYLDIACYLGLYCEROL SYNTHASE1 reveals roles of galactolipids in organelle differentiation in Arabidopsis cotyledons. Plant Physiol. 166, 1436–1449. doi: 10.1104/pp.114.250050
Fujii, S., Nagat, N., Masuda, T., Wada, H., and Kobayashi, K. (2019a). Galactolipids are essential for internal membrane transformation during etioplat-to-chloroplast differentiation. Plant Cell Physiol. 60, 1224–1238. doi: 10.1093/pcp/pcz041
Fujii, S., Wada, H., and Kobayashi, K. (2019b). Role of galactolipids in plastid differentiation before and after light exposure. Plan. Theory 8:357. doi: 10.3390/plants8100357
Gabruk, M., Mysliwa-Kurdziel, B., and Kruk, J. (2017). MGDG, PG and SQDG regulate the activity of light-dependent protochlorophyllide oxidoreductase. Biochem. J. 474, 1307–1320. doi: 10.1042/BCJ20170047
Garab, G., Ughy, B., de Waard, P., Javornik, U., Kotakis, C., Sket, P., et al. (2017). Lipid polymorphism in chloroplast thylakoid membranes – as revealed by 31P-NMR and time-resolved merocyanine fluorescence spectroscopy. Sci. Rep. 7, 13343–13356. doi: 10.1038/s41598-017-13574-y
Geigenberger, P., and Fernie, A. R. (2014). Metabolic control of redox and redox control of metabolism in plants. Antiox. Redox Signal. 21, 1389–1421. doi: 10.1089/ars.2014.6018
González, M., Delgado-Requerey, V., Ferrández, J., Serna, A., and Cejudo, F. J. (2019). Insights into the function of NADPH thioredoxin reductase C (NTRC) based on identification of NTRC-interacting proteins in vivo. J. Exp. Bot. 70, 5787–5798. doi: 10.1093/jxb/erz326
Güler, S., Seeliger, A., Härtel, H., Renger, G., and Benning, C. (1996). A null mutant of Synechococcus sp. PCC7942 deficient in the sulfolipid sulfoquinovosyl diacylglycerol. J. Biol. Chem. 271, 7501–7507. doi: 10.1074/jbc.271.13.7501
Hagio, M., Sakurai, I., Sato, S., Kato, T., Tabata, S., and Wada, H. (2002). Phosphatidylglycerol is essential for the development of thylakoid membranes in Arabidopsis thaliana. Plant Cell Physiol. 43, 1456–1464. doi: 10.1093/pcp/pcf185
Hiremath, S. S., Sajeevan, R. S., Nataraja, K. N., Chaturvedi, A. K., Chinnusamy, V., and Pal, M. (2017). Silencing of fatty acid desaturase (FAD7) gene enhances membrane stability and photosynthetic efficiency under heat stress in tobacco (Nicotiana benthamiana). Indian J. Exp. Bot. 55, 532–541.
Horn, P. J., Smith, M. D., Clark, T. R., Froehlich, J. E., and Benning, C. (2020). PEROXIREDOXIN Q stimulates the activity of the chloroplast 16: 1Δ3trans FATTY ACID DESATURASE4. Plant J. 102, 718–729. doi: 10.1111/tpj.14657
Hugly, S., Kunst, L., Browse, J., and Somerville, C. (1989). Enhanced thermal tolerance of photosynthesis and altered chloroplast ultrastructure in a mutant of Arabidopsis deficient in lipid desaturation. Plant Physiol. 90, 1134–1142. doi: 10.1104/pp.90.3.1134
Hunter, S. C., and Ohlrogge, J. B. (1998). Regulation of spinach chloroplast acetyl-CoA carboxylase. Arch. Biochem. Biophys. 359, 170–178. doi: 10.1006/abbi.1998.0900
Ivanov, A. G., Hendrickson, L., Krol, M., Selstam, E., Öquist, G., Hurry, V., et al. (2006). Digalactosyl-diacylglycerol deficiency impairs the capacity for photosynthetic intersystem electron transport and state transitions in Arabidopsis thaliana due to photosystem I acceptor-side limitations. Plant Cell Physiol. 47, 1146–1157. doi: 10.1093/pcp/pcj089
Jarvis, P., Dörmann, P., Peto, C. A., et al. (2000). Galactolipid deficiency and abnormal chloroplast development in the Arabidopsis MGD synthase 1 mutant. Proc. Natl. Acad. Sci. 97, 8175–8179. doi: 10.1073/pnas.100132197
Jouhet, J. (2013). Importance of the hexagonal lipid phase in biological membrane organization. Front. Plant Sci. 4:494. doi: 10.3389/fpls.2013.00494
Kansy, M., Wilhelm, C., and Goss, R. (2014). Influence of thylakoid membrane lipids on the structure and function of the plant photosystem II core complex. Planta 240, 781–796. doi: 10.1007/s00425-014-2130-2
Kelly, A. A., Froehlich, J. E., and Dörmann, P. (2003). Disruption of the two digalactosyldiacylglycerol synthase genes DGD1 and DGD2 in Arabidopsis reveals the existence of an additional enzyme of galactolipid synthesis. Plant Cell 15, 2694–2706. doi: 10.1105/tpc.016675
Kern, J., Zouni, A., Guskova, A., and Krauβ, N. (2009). “Lipid in the structure of photosystem I, photosystem II and the cytochrome b6f complex,” in Lipids in Photosynthesis. eds. H. Wada and N. Murata (Dordrecht: Springer), 203–242.
Kobayashi, K., Endo, K., and Wada, H. (2016). Multiple impacts of loss of plastidic phosphatidylglycerol biosynthesis on photosynthesis during seedling growth of Arabidopsis. Front. Plant Sci. 7:336. doi: 10.3389/fpls.2016.00336
Kobayashi, K., Fujii, S., Mayuko, S., Toyooka, K., and Wada, H. (2015). Specific role of phosphatidylglycerol and functional overlaps with other thylakoid lipids in Arabidopsis chloroplast biogenesis. Plant Cell Rep. 34, 631–642. doi: 10.1007/s00299-014-1719-z
Kobayashi, K., Fujii, S., Sasaki, D., Baba, S., Ohta, H., Masuda, T., et al. (2014). Transcriptional regulation of thylakoid galactolipid biosynthesis coordinated with chlorophyll biosynthesis during the development of chloroplasts in Arabidopsis. Front. Plant Sci. 5:272. doi: 10.3389/fpls.2014.00272
Kobayashi, K., Kondo, M., Fukuda, H., et al. (2007). Galactolipid synthesis in chloroplast inner envelope is essential for proper thylakoid biogenesis, photosynthesis, and embryogenesis. Proc. Natl. Acad. Sci. 104, 17216–17221. doi: 10.1073/pnas.0704680104
Kobayashi, K., Narise, T., Sonoike, K., et al. (2013). Role of galactolipid biosynthesis in coordinated development of photosynthetic complexes and thylakoid membranes during chloroplast biogenesis in Arabidopsis. Plant J. 73, 250–261. doi: 10.1111/tpj.12028
Kowalewska, Ł., Bykowski, M., and Mostowska, A. (2019). Spatial organization of thylakoid network in higher plants. Bot. Lett. 166, 326–343. doi: 10.1080/23818107.2019.1619195
Kowalewska, L. M., Mazur, R., Suski, S., Garstka, M., and Mostowska, A. (2016). Three-dimensional visualization of the internal plastid membrane network during runner bean chloroplast biogenesis. Plant Cell 28, 875–891. doi: 10.1105/tpc.15.01053
Kozaki, A., Mayumi, K., and Sasaki, Y. (2001). Thiol-disulfide exchange between nuclear-encoded and chloroplast encoded subunits of pea acetyl-CoA carboxylase. J. Biol. Chem. 276, 39919–39925. doi: 10.1074/jbc.M103525200
Kozaki, A., and Sasaki, Y. (1999). Light-dependent changes in redox status of the plastidic acetyl-CoA carboxylase and its regulatory component. Biochem. J. 339, 541–546. doi: 10.1042/bj3390541
Kruse, O., Hankamer, B., Konczak, C., Gerle, C., Morris, E., Radunz, A., et al. (2000). Phosphatidylglycerol is involved in the dimerization of photosystem II. J. Biol. Chem. 275, 6509–6514. doi: 10.1074/jbc.275.9.6509
Lebedev, N. N., Siffel, P., and Krasnovsky, A. A. (1985). Detection of protochlorophyllide forms in illuminated green leaves and chloroplasts by difference fluorescence spectroscopy at 77 K. Photosynthetica 19, 183–187.
Li-Beisson, Y., Shorrosh, B., Beisson, F., Andersson, M. X., et al. (2013). Acyl-lipid metabolism. Arabidopsis Book 11:e0161. doi: 10.1199/tab.0161
Liu, X.-Y., Li, B., Yang, J.-H., Sui, N., Yang, X.-M., and Meng, Q.-W. (2008). Overexpression of tomato chloroplast omega-3 fatty acid desaturase gene alleviates the photoinhibition of phosystems 2 and 1 under chilling stress. Photosynthetica 42:185. doi: 10.1007/s11120-013-9823-4
Los, D. A., Mironov, K. S., and Allakhverdiev, S. I. (2013). Regulatory role of membrane fluidity in gene expression and physiological functions. Photosynth. Res. 116, 489–509. doi: 10.1007/s11099-008-0030-z
Matsuda, O., Sakamoto, H., Hashimoto, T., and Iba, K. (2005). A temperature-sensitive mechanism that regulates post-translational stability of a plastidial ω-3 fatty-acid desaturase (FAD8) in Arabidopsis leaf tissues. J. Biol. Chem. 280, 3597–3604. doi: 10.1074/jbc.M407226200
Mazur, R., Mostowska, A., Szach, J., Gieczewska, K., Wójtowicz, J., Bednarska, K., et al. (2019). Galactolipid deficiency disturbs spatial arrangement of the thylakoid network in Arabidopsis thaliana plants. J. Exp. Bot. 70, 4689–4704. doi: 10.1093/jxb/erz219
McConn, M., Hugly, S., Browse, J., and Somerville, C. (1994). A mutation at the fad8 locus of Arabidopsis identifies a second chloroplast ω-3 desaturase. Plant Physiol. 106, 1609–1614. doi: 10.1104/pp.106.4.1609
Michelet, L., Zaffagnini, M., Morisse, S., Sparla, F., Pérez-Peérez, M. E., Francia, F., et al. (2013). Redox regulation of the Calvin-Benson cycle: something old, something new. Front. Plant Sci. 4:470. doi: 10.3389/fpls.2013.00470
Moon, B. Y., Higashi, S., Gombos, Z., and Murata, N. (1995). Unsaturation of the membrane lipids of chloroplasts stabilizes the photosynthetic machinery against low-temperature photoinhibition in transgenic tobacco plants. Proc. Natl. Acad. Sci. 92, 6219–6223. doi: 10.1073/pnas.92.14.6219
Moon, J. C., Jang, H. H., Chae, H. B., Lee, J. R., Lee, S. Y., Jung, Y. J., et al. (2006). The C-type Arabidopsis thioredoxin reductase ANTR-C acts as an electron donor to 2-Cys peroxiredoxins in chloroplasts. Biochem. Biophys. Res. Commun. 348, 478–484. doi: 10.1016/j.bbrc.2006.07.088
Murakami, Y., Tsuyama, M., Kobayashi, Y., Kodama, H., and Iba, K. (2000). Trienoic fatty acids and plant tolerance of high temperature. Science 287, 476–479. doi: 10.1126/science.287.5452.476
Murata, N., Ishizaki-Nishizawa, O., Higashi, S., Hayashi, H., Tasaka, Y., and Nishida, I. (1992). Genetically engineered alteration in the chilling sensitivity of plants. Nature 356, 710–713. doi: 10.1038/356710a0
Ohlrogge, J., and Browse, J. (1995). Lipid biosynthesis. Plant Cell 7, 957–970. doi: 10.1105/tpc.7.7.957
Ohlrogge, J., Jaworskl, J., Post-Beittenmlller, D., Roughan, G., Roessler, P., and Nakahlra, K. (1993). “Regulation of flux through the fatty acid biosynthesis pathway,” in Biochemistry and Molecular Biology of Membrane and Storage Lipids of Plants. eds. N. Murata and C. Somerville (Rockville, MD: American Society of Plant Physiologists), 102–112.
Ojeda, V., Pérez-Ruiz, J. M., González, M., Nájera, V. A., Sahrawy, M., Serrato, A. J., et al. (2017). NADPH thioredoxin reductase C and thioredoxins act concertedly in seedling development. Plant Physiol. 174, 1436–1448. doi: 10.1104/pp.17.00481
Pérez-Ruiz, J. M., and Cejudo, F. J. (2009). A proposed reaction mechanism for rice NADPH thioredoxin reductase C, an enzyme with protein disulfide reductase activity. FEBS Lett. 583, 1399–1402. doi: 10.1016/j.febslet.2009.03.067
Pérez-Ruiz, J. M., Naranjo, B., Ojeda, V., Guinea, M., and Cejudo, F. J. (2017). NTRC-dependent redox balance of 2-Cys peroxiredoxins is needed for optimal function of the photosynthetic apparatus. Proc. Natl. Acad. Sci. 114, 12069–12074. doi: 10.1073/pnas.1706003114
Pérez-Ruiz, J. M., Spínola, M. C., Kirchsteiger, K., Moreno, J., Sahrawy, M., and Cejudo, F. J. (2006). Rice NTRC is a high-efficiency redox system for chloroplast protection against oxidative damage. Plant Cell 18, 2356–2368. doi: 10.1105/tpc.106.041541
Petroutsos, D., Amiar, S., Abida, H., Dolch, L.-J., Bastien, O., Rébeillé, F., et al. (2014). Evolution of galactoglycerolipid biosynthetic pathways from cyanobacteria to primary plastids and from primary to secondary plastids. Prog. Lipid Res. 54, 68–85. doi: 10.1016/j.plipres.2014.02.001
Román, A., Hernández, M. L., Soria-García, A., López-Gomollón, S., Lagunas, B., Picorel, R., et al. (2015). Non-redundant contribution of the plastidial FAD8 ω-3 desaturase to glycerolipid unsaturation at different temperaturas in Arabidopsis. Mol. Plant 8, 1599–1611. doi: 10.1016/j.molp.2015.06.004
Routaboul, J.-M., Fischer, S. F., and Browse, J. (2000). Trienoic fatty acids are required to maintain chloroplast function at low temperatures. Plant Physiol. 124, 1697–1705. doi: 10.1104/pp.124.4.1697
Routaboul, J.-M., Sidmore, C., Wallis, J. G., and Browse, J. (2012). Arabidopsis mutants reveal that short- and long-term thermotolerance have different requirements for trienoic fatty acids. J. Exp. Bot. 63, 1435–1443. doi: 10.1093/jxb/err381
Sasaki, Y., Hakamada, K., Suama, Y., Nagano, Y., Furusawa, I., and Matsuno, R. (1993). Chloroplast-encoded protein as a subunit of acetyl-CoA carboxylase in pea plant. J. Biol. Chem. 268, 25118–25123. doi: 10.1016/S0021-9258(19)74577-3
Sasaki, Y., Kozaki, A., and Hatano, M. (1997). Link between light and fatty acid synthesis: Thioredoxin-linked reductive activation of plastidic acetyl-CoA carboxylase. Proc. Natl. Acad. Sci. 94, 11096–11101.
Sasaki, Y., Kozaki, A., Ohori, A., Iguchi, H., and Nagano, Y. (2001). Chloroplast RNA editing required for functional acetyl-CoA carboxylase in plants. J. Biol. Chem. 276, 3937–3940. doi: 10.1074/jbc.M008166200
Sasaki, Y., and Nagaro, Y. (2004). Plant acetyl-CoA carboxylase: structure, biosynthesis, regulation and gene manipulation for plant breeding. Biosci. Biotechnol. Biochem. 68, 1175–1184. doi: 10.1271/bbb.68.1175
Schaller, S., Latowski, D., Jemioła-Rzeminska, M., Dawood, A., Wilhelm, C., Strzałka, K., et al. (2011). Regulation of LHCII aggregation by different thylakoid membrane lipids. Biochim. Biophys. Acta 1807, 326–335. doi: 10.1016/j.bbabio.2010.12.017
Schoefs, B. (2001). The protochlorophyllide–chlorophyllide cycle. Photosynth. Res. 70, 257–271. doi: 10.1023/A:1014769707404
Selstam, E., and Sandelius, A. S. (1984). A comparison between prolamellar bodies and prothylakoid membranes of etioplasts of dark-grown wheat concerning lipid and polypeptide composition. Plant Physiol. 76, 1036–1040. doi: 10.1104/pp.76.4.1036
Serrato, A. J., Pérez-Ruiz, J. M., Spínola, M. C., and Cejudo, F. J. (2004). A novel NADPH thioredoxin reductase, localized in the chloroplast, which deficiency causes hypersensitivity to abiotic stress in Arabidopsis thaliana. J. Biol. Chem. 279, 43821–43827. doi: 10.1074/jbc.M404696200
Shanklin, J., and Cahoon, E. B. (1998). Desaturation and related modifications of fatty acids. Annu. Rev. Plant Physiol. Plant Mol. Biol. 49, 611–641. doi: 10.1146/annurev.arplant.49.1.611
Shimojima, M., Watanabe, T., Madoka, Y., Koizumi, R., Yamamoto, M. P., Masuda, K., et al. (2013). Differential regulation of two types of monogalactosyldiacylglycerol synthase in membrane lipid remodeling under phosphate-limited conditions in sesame plants. Front. Plant Sci. 4:469. doi: 10.3389/fpls.2013.00469
Siegenthaler, P.-A., Smutny, J., and Rawyler, A. (1987). Involvement of distinct populations of phosphatidylglycerol and phosphatidylcholine molecules in photosynthetic electron-flow activities. Biochim. Biophys. Acta 891, 85–93. doi: 10.1016/0005-2728(87)90086-7
Solymosi, K., and Schoefs, B. (2010). Etioplast and etio-chloroplast formation under natural conditions: the dark side of chlorophyll biosynthesis in angiosperms. Photosynth. Res. 105, 143–166. doi: 10.1007/s11120-010-9568-2
Sun, J., Ke, J., Johnson, J. L., Nikolau, B. J., and Wurtele, E. S. (1997). Biochemical and molecular biological characterization of CAC2, the Arabidopsis thaliana gene coding for the biotin carboxylase subunit of the plastidic acetyl-coenzyme A carboxylase. Plant Physiol. 115, 1371–1384. doi: 10.1104/pp.115.4.1371
Thormählen, I., Meitzel, T., Groysman, J., Öchsner, A. B., von Roepenack-Lahaye, E., Naranjo, B., et al. (2015). Thioredoxin f1 and NADPH-dependent thioredoxin reductase C have overlapping functions in regulating photosynthetic metabolism and plant growth in response to varying light conditions. Plant Physiol. 169, 1766–1786. doi: 10.1104/pp.15.01122
Vijayan, P., and Browse, J. (2002). Photoinhibition in mutants of Arabidopsis deficient in thylakoid unsaturation. Plant Physiol. 129, 876–885. doi: 10.1104/pp.004341
Wada, H., and Murata, N. (2007). The essential role of phosphatidylglycerol in photosynthesis. Photosynth. Res. 92, 205–215. doi: 10.1007/s11120-007-9203-z
Wallis, J., and Browse, J. (2002). Mutants of Arabidopsis reveal many roles for membrane lipids. Prog. Lip. Res. 41, 254–278. doi: 10.1016/S0163-7827(01)00027-3
Wilhelm, C., Goss, R., and Garab, G. (2020). The fluid-mosaic membrane theory in the context of photosynthetic membranes: is the thylakoid membrane more like a mixed crystal or like a fluid? J. Plant Physiol. 252:153246. doi: 10.1016/j.jplph.2020.153246
Yamaryo, Y., Motohashi, K., Takamiya, K., Hisabori, T., and Ohta, H. (2006). In vitro reconstitution of monogalactosyldiacylglycerol (MGDG) synthase regulation by thioredoxin. FEBS Lett. 580, 4086–4090. doi: 10.1016/j.febslet.2006.06.050
Ye, Y., Fulcher, Y. G., Sliman, D. J., Day, M. T., Schroeder, M. J., Koppisetti, R. K., et al. (2020a). The BADC and BCCP subunits of chloroplast acetyl-CoA carboxylase sense the pH changes of the light-dark cycle. J. Biol. Chem. 295, 9901–9916. doi: 10.1074/jbc.RA120.012877
Ye, Y., Nikovics, K., To, A., Lepiniec, L., Fedosejevs, E. T., Van Doren, S. R., et al. (2020b). Docking of acety-CoA carboxylase to the plastid envelope membrane attenuates fatty acid production in plants. Nat. Commun. 11:6191. doi: 10.1038/s41467-020-20014-5
Yu, B., and Benning, C. (2003). Anionic lipids are required for chloroplast structure and function in Arabidopsis. Plant J. 36, 762–770. doi: 10.1046/j.1365-313X.2003.01918.x
Yu, L., Fan, J., Zhou, C., and Xu, C. (2020). Chloroplast lipid biosynthesis is fine-tuned to thylakoid membrane remodeling during light acclimation. Plant Physiol. 185, 94–107. doi: 10.1093/plphys/kiaa013
Yu, B., Xu, C., and Benning, C. (2002). Arabidopsis disrupted in SQD2 encoding sulfolipid synthase is impaired in phosphate-limited growth. Proc. Natl. Acad. 99, 5732–5737. doi: 10.1073/pnas.082696499
Yuan, L., Wang, J., Xie, S., Zhao, M., Nie, L., Zheng, Y., et al. (2019). Comparative proteomics indicates that redox homeostasis is involved in high-and low-temperature stress tolerance in a novel Wucai (Brassica campestris L.) genotype. Int. J. Mol. Sci. 20:3760. doi: 10.3390/ijms20153760
Keywords: chloroplast, fatty acid, ferredoxin, lipid, membrane, redox regulation
Citation: Hernández ML and Cejudo FJ (2021) Chloroplast Lipids Metabolism and Function. A Redox Perspective. Front. Plant Sci. 12:712022. doi: 10.3389/fpls.2021.712022
Received: 19 May 2021; Accepted: 14 July 2021;
Published: 05 August 2021.
Edited by:
Nicolás Ernesto Blanco, Centro de Estudios Fotosintéticos y Bioquímicos (CEFOBI), ArgentinaReviewed by:
Koichi Kobayashi, Osaka Prefecture University, JapanCopyright © 2021 Hernández and Cejudo. This is an open-access article distributed under the terms of the Creative Commons Attribution License (CC BY). The use, distribution or reproduction in other forums is permitted, provided the original author(s) and the copyright owner(s) are credited and that the original publication in this journal is cited, in accordance with accepted academic practice. No use, distribution or reproduction is permitted which does not comply with these terms.
*Correspondence: M. Luisa Hernández, bWhqaW1lbmV6QHVzLmVz; Francisco Javier Cejudo, ZmpjZWp1ZG9AdXMuZXM=
Disclaimer: All claims expressed in this article are solely those of the authors and do not necessarily represent those of their affiliated organizations, or those of the publisher, the editors and the reviewers. Any product that may be evaluated in this article or claim that may be made by its manufacturer is not guaranteed or endorsed by the publisher.
Research integrity at Frontiers
Learn more about the work of our research integrity team to safeguard the quality of each article we publish.