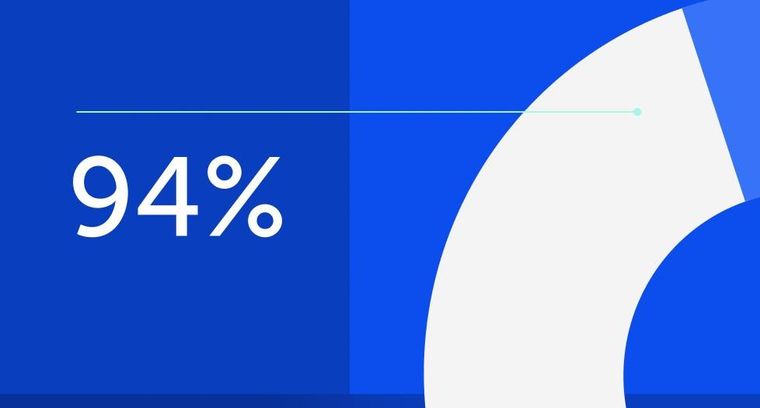
94% of researchers rate our articles as excellent or good
Learn more about the work of our research integrity team to safeguard the quality of each article we publish.
Find out more
ORIGINAL RESEARCH article
Front. Plant Sci., 13 August 2021
Sec. Plant Abiotic Stress
Volume 12 - 2021 | https://doi.org/10.3389/fpls.2021.704983
Phosphorus plays an important role in plant growth and development, and is an important limiting factor for crop yield. Although previous studies have shown that 6-phosphogluconate dehydrogenase (6PGDH) plays an important role in plant resistance to adversity, its response to low phosphorus (P) stress remains unknown. In this study, we reported the cloning and characterization of a cytosolic 6PGDH gene, Gm6PGDH1, which enhanced the tolerance to phosphate (Pi) starvation by improving root system development and modifying the antioxidant system in transgenic plants. Gm6PGDH1 was highly expressed in the root at full bloom stage, and strongly induced by Pi starvation. The results from intact soybean composite plant and soybean plant, both containing a Gm6PGDH1-overexpressing construct, showed that Gm6PGDH1 was involved in root system development, and subsequently affected P uptake under Pi-deficient conditions. Meanwhile, the accumulation of reactive oxygen species (ROS) in the root tip of transgenic soybean was reduced, and the activity of ROS-scavenging enzymes was enhanced compared with those of the wild type under Pi-deficient conditions. Interestingly, we found that the overexpression of Gm6PGDH1 weakened the response of several other important Pi-answer genes to Pi starvation, such as some purple acid phosphatases (PAPs) and redox-related genes. In addition, the results from a virus-induced gene silencing (VIGS) indicated that Gm6PGDH1 might have functional redundancy in soybean, and the results from a heterogeneous transformation system showed that overexpressing Gm6PGDH1 also enhanced tolerance to Pi starvation in transgenic Arabidopsis. Together, these results suggested the great potential of Gm6PGDH1 in crop breeding for low Pi tolerance.
Phosphorus is one of the most important mineral elements that affect plant growth and metabolism, which plays an important role in the regulation of photosynthesis, respiration, and a series of enzymes (Jain et al., 2007). Plant roots obtain essential phosphorus (P) only by absorbing inorganic phosphate (Pi) of soil. Although the overall P content in soil is high, most P is converted to highly insoluble and tightly bound forms, resulting in a large amount of P immobilized in the soil and unusable by plants (Schachtman et al., 1998). Nearly half of the arable land in the world has insufficient availability of Pi, which limits crop productivity (Hinsinger, 2001). Using Pi fertilizer solely to ensure production will not only increase economic costs but also cause environmental pollution (Bennett et al., 2001). Furthermore, the non-renewable nature of Pi fertilizers means that cheap sources of P, such as Pi rocks, will be exhausted within the next 60–90 years (Hammond et al., 2004). Therefore, in the face of increasing P shortage and P pollution, it is undoubtedly one of the most correct and effective means to establish efficient P genotypes in crops (Cong et al., 2020).
To cope with Pi deficiency, plants have developed many adaptive mechanisms to enhance the availability and uptake of P from soil, including modification in root architecture by stimulating the growth of lateral roots and root hairs to maximize root surface area, increased exudation of small molecular organic acids and activities of purple acid phosphatases (PAPs), and symbiosis with mycorrhizal fungi (Veneklaas et al., 2012). Root is the only organ that plants absorb Pi, and its favorable development is the key to adapting to Pi starvation (Chevalier et al., 2003; Péret et al., 2011; Oldroyd and Leyser, 2020). So far, through the unremitting efforts of botanists, hundreds of genes regulating root development in a low-Pi environment have been discovered, which have made important contributions to the cultivation of low-Pi tolerant crops. For example, OsPTF1-overexpressing transgenic rice showed significantly higher total root length and root surface area under Pi-deficient conditions, which results in a higher instantaneous Pi uptake rate over their wild-type counterparts (Yi et al., 2005); overexpression of OsMYB2P-1 in rice led to longer primary roots and adventitious roots than wild-type plants under Pi-deficient conditions, enhancing tolerance to Pi starvation (Dai et al., 2012); the knockout of OsARF16 led to primary root (PR), lateral root (LR), and root hair losing sensitivity to low Pi response (Shen et al., 2013). These studies indicate that root system development is closely related to low Pi resistance of plants. However, the molecular mechanisms of root system development in response to Pi starvation remain unclear.
Reactive oxygen species (ROS) signal molecules mainly include hydrogen peroxide (H2O2), superoxide radicals (O2–), and hydroxide ions (OH–), which play an important role in maintaining the normal growth of plants and improving their stress resistance (Huang et al., 2019). ROS production induced by adverse environmental stimuli is a common phenomenon in plants (Saed-Moucheshi et al., 2014), including Pi starvation (Shin et al., 2005). Plants can respond to stress through ROS to adapt to the environment, but excessive ROS in plants will cause damage to seedlings, cell structure and function, enzyme system, and DNA (Schieber and Chandel, 2014; Waszczak et al., 2018). In plant cells, ROS can be scavenged through several major ROS-scavenging enzymes, such as superoxide dismutase (SOD), ascorbate peroxidase (APX), catalase (CAT), glutathione peroxidase (GPX), and peroxidase (POD), and these ROS-scavenging systems are crucial to plant tolerance under stresses (Foyer and Noctor, 2005; Dvorak et al., 2020). ROS has been shown to be involved in plant redox processes, and the redox state of plant cells is highly correlated with cellular ROS production and processing (Nadarajah, 2020). Studies have shown that the redox balance of root cells was important to sustain the activity of root apical meristem under Pi starvation (Gutierrezalanis et al., 2018). In recent years, many genes related to oxidative stress that control the level of ROS have been found by functional classification of Pi-response genes with the development of gene sequencing technology, suggesting that redox reactions in roots plays an important role in plant adaptation to low Pi (Wu et al., 2003; Misson et al., 2005; Zhang et al., 2019), but few redox-related genes have been functionally validated under low Pi stress conditions.
The oxidative pentose phosphoric pathway (OPPP) is a major metabolic pathway of glucose degradation. OPPP can produce a large amount of nicotinamide adenine dinucleotide phosphate (NADPH, reduced coenzyme II), which provides the main reducing power for biosynthesis, and the intermediate metabolites it produces also provide raw materials for many biosynthesis (And and Emes, 2000; Kruger and Schaewen, 2003). Both glucose-6-phosphate dehydrogenase (G6PDH) and 6-phosphogluconate dehydrogenase (6PGDH) are considered as rate-limiting enzymes in OPPP, and are widely distributed over the cytoplasm and plastids of higher plants (Ap Rees, 1985). For years, G6PDH has been reported to be associated with tolerance responses to abiotic stress in many plants, such as Arabidopsis (Arabidopsis thaliana), rice (Oryza sativa), wheat (Triticum aestivum), and soybean (Glycine max) (Nemoto and Sasakuma, 2000; Liu et al., 2013; Zhang et al., 2013; Yang et al., 2019a). However, compared with G6PDH, there were few studies on the relationship between 6PGDH and plant tolerance response to abiotic stress. Current studies show that 6PGDH plays an important role in regulating biological metabolite resistance to damage to the external environment, and may improve the stress resistance of rice by enhancing the glucose metabolism pathway (Hou et al., 2007).
Soybean is an important grain and oil crop in the world, and the main source of human high-quality protein and feed protein for animal husbandry (Sample et al., 1980). It can interact with rhizobia to form a nodule for symbiotic nitrogen fixation, so Pi deficiency is more likely to be one of the important limiting factors for high yield of soybean (Ohlrogge, 1960). To date, few studies have linked 6PGDH to the response of plants to Pi starvation. In this study, we cloned Gm6PGDH1, a cytosolic 6PGDH gene from soybean, and found that it might enhance tolerance to Pi starvation by improving root system development and modifying the antioxidant system in soybean.
The NCBI database1 was used to search for genetic information. The SMART database2 was used to analyze amino acid sequences. The BioXM 2.6 software was used to predict the molecular weight and isoelectric point of the gene. ClustalX 1.83 was used for multiple alignments. Neighbor-joining phylogenetic trees were generated using the MEGA 5.1 program.
The expression vector pET29a-Gm6PGDH1 was constructed using the Gateway Technology with a One Step Cloning Kit (Vazyme Biotech, Nanjing, China) according to the protocol of the manufacturer. The sequences of the primers are provided in Supplementary Table 1. The pET29a-Gm6PGDH1 plasmid was transformed into the Escherichia coli Rosetta (DE3) strain, and the expression of Gm6PGDH1 was induced with 0.5 mM IPTG to generate the putative recombinants. Then, the His-tagged Gm6PGDH1 proteins were extracted and purified under native conditions using Ni Focurose 6FF (IMAC), and the products were detected by 10% Sodium dodecyl sulfate-polyacrylamide gel electrophoresis (SDS-PAGE).
The measurement of Gm6PGDH1 enzyme activity was based on a previous method, with some changes (Šindelář et al., 1999). The recombinants were ultrasonic, broken and centrifuged at 4°C, and the supernatant was enzyme crude extracts. The total reaction volume of the assay was 2 ml containing a 1.8-ml reaction buffer (50 mM Triethanolamine, pH 7.5, 5 mM MgCl2, 0.18 mM NADP+, 1 mM 6PG) and 0.2 ml enzyme crude extracts. Absorbance changes at 340 nm were monitored using a visible spectrophotometer (722N, Shanghai Precision Science Instrument, Shanghai, China). The absorbance value was observed and recorded once every 10 s, and the reaction time was 2 min. A total of three replicates were included in this experiment.
Soybean Williams 82 plants were used in this study. Soybean seeds normally were germinated and grown in a growth chamber under controlled photoperiod and temperature (16 h light; 25°C/8 h dark; 20°C). Five-day-old seedlings (removal of cotyledons) were transferred to a vermiculite medium containing a modified Hoagland solution for 11 days, with 1 mM KH2PO4 (1 mM Pi, Pi-sufficient) or 2.5 μM KH2PO4 (2.5 μM Pi, Pi-deficient) as the P supply. On the 10th day, the Pi-deficient group was re-supplied with 1 mM Pi for 1 day and was called R1d. The nutrient solution was refreshed every 3 days. Soybean tissues were collected at specific times. All the samples were stored at −80°C prior to RNA extraction.
Three biological replicates, each comprising three individual plants, were used for quantitative real-time PCR (RT–qPCR). Total RNA was extracted using the RNA Prep Pure Plant Kit (TIANGEN, Beijing, China). RT–qPCR analysis was performed as described previously (Li et al., 2020). The relative level of expression was calculated using the formula 2–△Ct or 2–△△Ct. Actin (GLYMA_08G146500) or Actin2-8 (AT3G18780) was used as an endogenous control to normalize the samples. All the primers used for RT-qPCR are listed in Supplementary Tables 2, 3.
The coding region of Gm6PGDH1 was amplified with specific primers (Supplementary Table 1), and cloned into the pJIT166-GFP vector to construct the pJIT166-Gm6PGDH1-GFP vector. The fusion (pJIT166-Gm6PGDH1-GFP) and control (pJIT166-GFP) constructs were transformed into Arabidopsis mesophyll protoplast using the polyethylene glycol method (Yoo et al., 2007). The fluorescence of GFP was imaged with a Zeiss LSM780 confocal microscope (Carl Zeiss, SAS, Jena, Germany).
The fused plasmid vector (pCAMBIA3301-Gm6PGDH1) was transformed into wild-type Arabidopsis (Col-0) plants using the Agrobacterium-mediated floral-dip procedure (Clough and Bent, 1998). Transgenic plants were selected on a Basta (20 mgL–1) medium, and the selected plants were identified by PCR and RT-qPCR (Supplementary Figures 6A,B). Homozygous T3 or T4 seeds and wild-type seeds, which were harvested in the same environment, were used for research.
To confirm the function of Gm6PGDH1 in soybean, the full-length Gm6PGDH1 coding sequence was inserted after the CaMV35S promoter, resulting in pCAMBIA3301-Gm6PGDH1 constructs with a GFP label (the original GUS label was replaced by the GFP label). The sequences of the primers are provided in Supplementary Table 1. Then, the overexpression vector and the empty vector were separately introduced into Agrobacterium rhizogenes K599, which was used to infect soybean (Williams 82) seedlings in order to obtain composite soybean plants with transgenic hairy roots (Attila et al., 2007). When transgenic hairy roots grew approximately 8–10 cm long, a small part was harvested for RT-qPCR identification (Supplementary Figure 1). The primary roots of the transgenic composite plants were cut off, and plants were transferred to Hoagland vermiculite medium containing 1 mM Pi or 2.5 mM Pi for 14 days after 2 days of growth with Hoagland nutrient solution. Each transgenic root represented an independent transgenic line; for each Pi treatment, six independent transgenic lines were included. After 14 days of growth, leaves and hairy roots were harvested separately to determine dry weight and total P concentration. Several hairy roots were taken for further RT-qPCR analysis. An independent transgenic line originating from a composite soybean plant with a transgenic hairy root was considered as a semi-biological replicate. A total of three replicates were included in this experiment.
The fused plasmid vector (pCAMBIA3301-Gm6PGDH1) was transformed into soybean (Williams 82) using the Agrobacterium-mediated transformation method (Li et al., 2017b). Positive transgenic plants were screened in a greenhouse by leaf herbicide painting and PCR-based amplification of genomic DNA. T3-generation plants of homozygous independent transgenic lines were used for research.
A 329-bp DNA fragment of the Gm6PGDH1 gene was PCR-amplified using gene-specific primers (Supplementary Table 1) and cloned into the BPMV RNA2 silencing vector to construct BPMV-Gm6PGDH1 vectors (Zhang et al., 2010). As described earlier, DNA-based BPMV constructs were inoculated in V1 stage soybean seedlings by mechanical friction (Pflieger et al., 2015). The empty BPMV vector was used as a control. Two weeks post-virus inoculation, the leaf edge of some of the plants was wrinkled and deformed (Supplementary Figure 5A), indicating successful infection. Then, small leaves of the plants with infection symptoms were harvested to isolate the RNA for verification of gene silencing by RT-qPCR (Supplementary Figure 5B). The infected plants were transferred to vermiculite medium containing 2.5 μM Pi Hoagland nutrient solutions for 7 days. Each infected plant represented one independent gene silencing line. One independent infected plant was considered as one biological replication. A total of three replicates were included in this experiment.
Five-day-old soy bean plants were grown in a Hoagland medium with two Pi levels (1 mM KH2PO4 and 2.5 μM KH2PO4) for 30 days. The nutrient solution was refreshed every 3 days. The chlorophyll content of the second newly unfolded leaves at the top was assayed with SPAD-502Plus (Konicaminolta, Japan).
For the plant total P concentration assay, fresh plant samples were heated at 75°C until completely dry, and then ground into powder separately. Approximately 0.05 g of the plant dry samples were weighed and digested with H2SO4 and H2O2. After cooling, the digested samples were diluted to 100 ml with distilled water. Then, the concentration of P in the solution was determined with 710 ICP-OES (Agilent Technologies, Santa Clara, CA, United States). Each experiment was repeated three times.
For the soluble Pi concentration assay, Tissue Inorganic Phosphorus Content Detection Kit (Solarbio, Beijing, China) was used. Each sample consisted of five seedlings, which were ground into fine powder in liquid nitrogen, repeated three times.
For transgenic soybean root analysis, an Expression 21000XL (Epson, Nagano, Japan) scanner with a root analysis system WinRHIZO 2020 (Regent, Canada) was used. Transgenic Arabidopsis photography and root system analysis were performed as described previously (Li et al., 2020).
Trypan blue was used for the analysis of root activity. The root tips (2 cm) of soybean seedlings were dyed with 0.1% (W/V) trypan blue for 3 min in a boiling water bath, washed with distilled water for 10 min, and the observed under an OLYMPUS CX31 stereo microscope with a SPOT-RT digital camera (Olympus, Melville, NY, United States) attached to a computer.
For ROS accumulation analysis, staining with Diaminobenzidine (DAB) of stress-treated seedlings was performed following the reported protocol (Daudi and O’Brien, 2012). H2O2 content was determined according to the method described previously, with slight modifications (Yang et al., 2019b). For the soybean plants, 0.2 g of the root tips were taken as a sample, and for Arabidopsis, five seedlings were taken as a sample. After overnight reaction, the absorbance was read at 390 nm, and H2O2 content was determined using a standard curve. Each experiment was repeated three times.
For activity of ROS-scavenging enzymes analysis, soybean root tips (0.2 g) were ground with a 1.6 ml ice-cold 50 mM phosphate-buffered saline (PBS) (pH 7.8). The homogenate was centrifuged at 16,000 × g for 20 min at 4°C, and the supernatant was used for the assays. The activities of SOD, POD, and APX were determined according to established protocols (Luo et al., 2020). Nicotinamide adenine dinucleotide phosphate (NADPH) content was determined by following the method described previously, with slight modifications (Matsumura and Miyachi, 1980; Wei et al., 2019). Homogenate plant tissue samples in 2 ml 0.1 M NaOH solution under ice bath conditions were then centrifuged at 10,000 × g for 20 min at 25°C, and 50 μl of the supernatant of the sampling solution was added with 300 μl of a mixture (0.1 M NaOH, 40 mM EDTA, 4.2 mM MTT, 16.6 mM PMS), followed by 450 μl of 0.1 mM NaCl, and the solution was kept for 5 min at 37°C. Then, the solution was mixed with 50 μl G6P dehydrogenase (0.5 KU/ml) and incubated at 37°C for 40 min. The reaction was terminated with 200 μl of saturated NaCl and centrifuged at 10,000 × g for 10 min at 25°C. The supernatant was discarded, and the precipitate was dissolved with 1 ml 95% ethanol (v/v). After the precipitation was completely dissolved, OD value was measured at 570 nm. Five Arabidopsis seedlings were taken as a sample, and three biological replicates were set in each sample. The NADPH content was expressed as OD570/plant FW.
Statistical analyses were performed using Microsoft Excel 2010 and the SAS System for Windows V10. Significant differences were evaluated by two-tailed Student’s t-test or one-way ANOVA and Duncan’s test. All test differences at P < 0.05 were significant. All the error bars were SD value.
A 6PGDH gene (GLYMA_08G254500) was obtained through BLAST searching against the soybean genome utilizing the reported Arabidopsis 6PGDH family protein gene PGD2 as query (Hölscher et al., 2016), and it was named Gm6PGDH1. Gm6PGDH1 was located on Gm8 (22503089-22506054) of soybean, and its full-length coding sequence (CDS) had 486 amino acids with a calculated molecular mass of 53.57 kD and an isoelectric point of 6.4. Amino acid sequence analysis showed that Gm6PGDH1 had a C-terminal 6GPD domain and an N-terminal NAD-binding domain (Figure 1A). Phylogenetic analysis was performed to determine the evolutionary relationship between Gm6PGDH1 and the other plant 6PGDH proteins, among which Gm6PGDH1 was most closely related to At6PGDH2 (Figure 1B). The results of amino acid sequence alignment showed that Gm6PGDH1 was highly conserved, and that the homology between Gm6PGDH1 and At6PGDH1, At6PGDH2, At6PGDH3, Os6PGDH1, and Os6PGDH2 was 76.35, 90.12, 76.76, 85.65, and 70.95%, respectively (Figure 1C).
Figure 1. Protein structures, prokaryotic induction, and enzyme activity determination of Gm6PGDH1. (A) Domain analysis of Gm6PGDH1. (B) Phylogenetic tree constructed based on Gm6PGDH1 and the other plant 6PGDH proteins. (C) Protein sequence comparison between Gm6PGDH1 and Arabidopsis (At6PGDH1, At6PGDH2, and At6PGDH3) and rice (Os6PGDH1 and Os6PGDH2) 6PGDH proteins. Identical amino acids are shown with a black background, and analogous amino acids are shaded in gray. (D) Coomassie-stained 10% SDS-PAGE of cell extract. Lane 1: Rosetta (DE3) Escherichia coli strain expressing empty vector protein (PET29a); Lane 2: DE3 expressing His-tagged Gm6PGDH1 fusion protein (PET29a-Gm6PGDH1); Lane 3: Purified PET29aprotein; Lane 4: Purified PET29a-Gm6PGDH1 fusion protein; M: protein maker. The target protein was in the red frame. (E) GPDH enzyme activities from Gm6PGDH1 fusion protein. Data means ± SD (n = 3). Asterisks indicate a significant difference from the PET29a (Student’s t-test, ***P < 0.001).
To further test whether Gm6PGDH1 exhibited an enzymatic activity, the prokaryotic expression vector of Gm6PGDH1 was constructed. The recombinant protein generated by the E. coli Rosetta (DE3) strain expressing plasmid of Histagged Gm6PGDH1 was purified with a Ni-NTA column, and recombinant proteins produced by DE3 strain were detected by SDS-PAGE. A Gm6PGDH1-His fusion protein with an expected size of 54.39 kDa (consisting of target gene and histidine marker) was identified after Coomassie brilliant blue staining (Figure 1D). Meanwhile, the recombinant Gm6PGDH1 protein was assayed for its kinetic properties relative to the substrate NADP+. The recombinant proteins were ultrasonic-broken and centrifuged at 4°C, and the supernatant was taken as enzyme crude extracts. Then, the reaction buffer was added into the crude enzyme extracts of Gm6PGDH1-His, and the crude enzyme extracts of an empty carrier were used as the control. The relative activity of bacterial protein was calculated by the slope of the curve, and the results showed that the relative enzyme activity of the prokaryotic-induced Gm6PGDH1 fusion protein was significantly higher than that of the empty carrier (Figure 1E).
To investigate the transcript levels of Gm6PGDH1 in specific tissues, the total RNA was extracted from root, stem, leaf, flower, pod, or seed of the soybean plants at first trifoliate (V1), full bloom (R2), and full seed (R6) stages. The RT-qPCR analysis showed that Gm6PGDH1 was expressed in all the tissues examined, with the highest expression in root at the R2 stage and lowest in leaf at the R6 stage (Figure 2A). The expression patterns of Gm6PGDH1 in the root was altered significantly that the largest transcription was observed at the R2 stage. A similar expression pattern was observed in the leaf (Figure 2A). Then, RNA samples from the root and leaf were used to evaluate the expression patterns of Gm6PGDH1 to Pi starvation by RT-qPCR. The results showed that the expression levels of Gm6PGDH1 significantly increased over time under low Pi conditions. After 10 days of treatment with Pi starvation, the expression level of Gm6PGDH1 in the root and leaf both rose to the maximum value, but the expression level of Gm6PGDH1 in the root was higher than that in the leaf (Figure 2B). Furthermore, the relative levels of Gm6PGDH1 in both the root and the leaf dropped rapidly after recovery of Pi for a day (Figure 2B). These results suggested that Gm6PGDH1 was involved in transcriptional response during soybean Pi starvation adaptions.
Figure 2. Expression patterns and subcellular localization of Gm6PGDH1. (A) Tissue-specific transcript abundance of Gm6PGDH1 in root, stem, leaf, flower, pod, and seed at different developmental stages, the first trifoliate stage (V1), full bloom stage (R2), and full seed stage (R6). (B) Time course of the Gm6PGDH1 expression in response to Pi starvation. R1d means resume Pi supply for 1 day. Actin was used as an internal control. Data means ± SD (n = 3). Data that are significantly different from the corresponding controls are indicated (Student’s t-test, **P < 0.01; ***P < 0.001). (C) Subcellular localization of the Gm6PGDH1 protein in protoplasts of Arabidopsis. Scale bar = 20 μm.
Previous literature suggested that there were two forms of 6PGDH in plants: cytosolic 6PGDH and plastidic 6PGDH, and that the N-terminal of plastid 6PGDH amino acid sequence had one more signal peptide sequence compared with cytosolic 6PGDH (Tanksley and Kuehn, 1985; Spielbauer et al., 2013). The domain analysis of Gm6PGDH1 showed no signal peptide at the N-terminal (Figure 1A), so it may be cytosolic 6PGDH. To verify the subcellular localization of Gm6PGDH1, a Gm6PGDH1-green fluorescent protein (GFP) fusion construct and a control construct containing only GFP were generated. Both constructs were transformed into the mesophyll protoplasts of Arabidopsis and observed under a confocal laser scanning microscope. The free GFP alone was present throughout the whole cell, whereas Gm6PGDH1-GFP was specifically located in the cytosol, which demonstrated that Gm6PGDH1 was cytosolic 6PGDH (Figure 2C).
Composite plants with transgenic hairy root attached to wild-type (WT) shoot can be obtained by hairy root transformation, which provides a fast and effective way for functional analysis of genes expressed in soybean root (Zhang et al., 2014; Xue et al., 2017). To produce composite soybean plants consisting of a wild-type shoot with transgenic roots, Agrobacterium rhizogenes should be injected into the cotyledonary node and/or the proximal part of the hypocotyl, which would lead to the development of hairy roots at the infection site. In this case, the chimeric plants have a strong root connection based on direct outgrowth from the vasculature (Attila et al., 2007). By RT-qPCR analysis, the expression level of Gm6PGDH1 in transgenic hairy roots was about twofold over empty vector control hairy roots (Supplementary Figure 1). Under Pi-deficient conditions, the control plants showed more obvious symptoms of P deficiency than the Gm6PGDH1-overexprssing composite plants, such as fewer buds and deep color leaves (Figure 3A). Meanwhile, the dry weight and total P concentration of the roots of the composite transgenic plants were significantly higher than those of the control plants under Pi-deficient conditions, while there was no significant difference under Pi-sufficient conditions (Figures 3C,E). These results suggested that the expression of Gm6PGDH1 in soybean root may affect the P efficiency of plant root by regulating root system development under Pi-deficient conditions. In this experiment, the overexpression of Gm6PGDH1 did not lead to changes in dry weight and total P concentration of the composite transgenic plants leaf, regardless under Pi-deficient or Pi-sufficient condition (Figures 3B,D), that part of the reason may be the shorter processing time.
Figure 3. Comparison of control (CK) and Gm6PGDH1-overexpressing composite soybean plants under two Pi level conditions. Composite soybean plants with transgenic hairy roots were grown with a normal nutrient solution for 2 days, and then the plants were switched to a nutrient solution containing 1 mM or 2.5 μM KH2PO4. After 14 more days, the leaves and roots were separately harvested for analysis. (A) Phenotype of composite soybean plants. Scale unit: cm. (B) Dry weight of leaves. (C) Dry weight of roots. (D) Total P concentration of the leaves. (E) Total P concentration of the roots. CK represents the composite plants transformed with the empty vector; OE indicates Gm6PGDH1-overexpressing composite plants. DW means dry weight. Data are means ± SD of three biological replicates. Asterisks indicate significant differences between OE and CK (Student’s t-test, *P < 0.05).
As an important category of genes involved in the Pi starvation rescue system, PAPs play an important role in plant Pi acquisition (Raghothama, 1999; Tomscha et al., 2004; Liang et al., 2010). Previous studies have shown that the transcript level of 66% GmPAP genes were induced or increased by Pi-deprivation treatment in soybean (Li et al., 2012). In order to further understand the regulatory role of Gm6PGDH1 in Pi starvation, the transcription of five GmPAP genes was analyzed in hairy roots of transgenic composite plants. In the hairy roots of the control plants, Pi starvation significantly induced the expression of the five GmPAP genes (Supplementary Figure 2), which was similar to previous reports (Li et al., 2012, 2017a; Kong et al., 2018). In composite plant hairy roots with overexpressing Gm6PGDH1, however, no significant induction of GmPAP14 and GmPAP21 expression was detected (Supplementary Figure 2). In addition, the induction ratio of the GmPAP3, GmPAP15, and GmPAP17 transcripts in the composite plants hairy roots overexpressing Gm6PGDH1 was reduced by 50–80% compared with the control plants under Pi-deficient conditions (Supplementary Figure 2). There was no significant difference in the expression levels of these genes between composite plants hairy roots with overexpressing Gm6PGDH1 and the control under Pi-sufficient conditions (Supplementary Figure 2). These results suggested that the overexpression of Gm6PGDH1 attenuates the response to Pi starvation of several Pi-starvation inducible GmPAP genes in hairy roots of transgenic composite plants.
Then, we generated three stable overexpressing soybean lines (OX-1, OX-2, and OX-3) to better investigate the role of Gm6PGDH1 response and adaptation to Pi starvation (Supplementary Figures 3A,B). The T3 progeny of 5-day-old transgenic soybean lines and WT seedlings was exposed to a nutrient solution containing a high level of Pi (1 mM Pi, Pi sufficient) and a low level of Pi (2.5 μM Pi, Pi deficient) for 30 days (removal of cotyledons). The three Gm6PGDH1-overexpressing lines showed no significant growth difference compared with WT when grown with the Pi-sufficient solution (Figure 4). However, the Gm6PGDH1-overexpressing lines showed better growth than the WT plants when grown with the Pi-deficient solution (Figure 4A). There was a significant difference in plant height and dry weight between WT and Gm6PGDH1-overexpressing plants under Pi-deficient condition (Figures 4B,C). At the same time, the total P concentrations of the Gm6PGDH1-overexpressing lines were higher than in the WT under Pi-deficient conditions (Figure 4D). Since Pi starvation will lead to a relative increase in chlorophyll content (Devaiah et al., 2009), we monitored the chlorophyll content of all the plants and found that the chlorophyll content of transgenic plants under Pi-deficient conditions was significantly lower than that of WT (Figure 4E). These results showed that the overexpression of Gm6PGDH1 increased the resistance of soybean to Pi starvation.
Figure 4. Overexpression of Gm6PGDH1 enhances tolerance to Pi starvation in transgenic soybean. Five-day-old seedlings (removal of cotyledons) were exposed to nutrient solutions containing two levels of Pi, 1 mM Pi (Pi-sufficient), or 2.5 μM Pi (Pi-deficient) for 30 days. (A) Phenotypes of wild type (WT) and Gm6PGDH1-overexpressing lines (OX-1, OX-2, and OX-3) in response to two levels of Pi treatment. Scale unit: cm. (B–G) Plant height, fresh weight, total P concentrations, chlorophyll content, the total root length, and the number of root tips were recorded at the end of the treatment period. Data are means of three replicates with SD. Asterisks show that the values are significantly different between the transgenic lines and the WT at the same time point (Student’s t-test, *P < 0.05, **P < 0.01, ***P < 0.001).
Since Pi starvation could cause changes in plant root architecture (Péret et al., 2011), we further observed the root development of transgenic soybean under Pi starvation. Under Pi-sufficient conditions, no significant differences in root architecture were observed between the WT and Gm6PGDH1-overexpressing lines (Figures 4F,G). However, the total root length in the Gm6PGDH1-overexpressing lines was significantly longer than that in WT under Pi-deficient conditions (Figure 4F). The number of root tips in Gm6PGDH1-overexpressing lines was significantly higher than that in WT under Pi-deficient conditions (Figure 4G). In addition, trypan blue can enter dead cells and appear blue (Zhang et al., 2011), so we tried to use it to explore the difference in root activity between transgenic soybean lines and WT. Under Pi-deficient conditions, the staining degree of soybean root tip (more accurately the meristematic zone) increased compared with that under P-sufficient conditions, indicating that the number of dead cells in the root tip increased (Supplementary Figure 4). However, we found that the staining degree of transgenic lines was lighter than that of WT, indicating higher root activity of Gm6PGDH1-overexpressing lines than WT under Pi-deficient conditions (Supplementary Figure 4). These results suggested that the improved growth of the Gm6PGDH1-overexpressing lines under Pi-deficient conditions may be partly due to better root system development.
As the OPPP is widely involved in plant redox balance, and 6PGDH is its rate-limiting enzyme (Balzergue et al., 2017), we naturally thought that the overexpression of Gm6PGDH1 might affect the redox system of transgenic soybeans (Lehmann et al., 2009). The T3 progeny of 5-day-old Gm6PGDH1-overexpressing transgenic soybean and WT seedlings were exposed to a nutrient solution containing a high level of Pi (1 mM Pi, Pi sufficient) or a low level of Pi (2.5 μM Pi, Pi deficient) for 10 days (removal of cotyledons). As ROS is a major factor causing oxidative stress, we examined the accumulation of one major ROS, H2O2. The root tips of all the treated soybean materials were dyed with DAB. As shown in Figure 5A, Pi starvation accentuates the staining of upper root in the expansion and elongation zones, while the Gm6PGDH1-overexpressing lines were stained to a lighter extent compared with that of WT under Pi-deficient conditions, implying that less ROS was produced in the transgenic soybean lines. Quantitative measurements further demonstrated that H2O2 contents in the root tips of the Gm6PGDH1-overexpressing lines were remarkably lower than that of WT (Figure 5B). These results indicated that transgenic lines had better oxidative stress tolerance in a low Pi environment. The important role of ROS-scavenging enzymes in ROS homeostasis prompted us to assess the enzyme activities of SOD, POD, and APX in the root tips of soybean (Gill and Tuteja, 2010). After 10 days of Pi starvation, it was noticeable that the three enzyme activities of the Gm6PGDH1-overexpressing lines were significantly higher than those in the WT (Figures 5C–E). No similar difference was found under Pi-sufficient conditions.
Figure 5. Changes in levels of H2O2 accumulation and activities of ROS-scavenging enzymes of soybean root tips during Pi starvation. Five-day-old soybean seedlings (removal of cotyledons) were exposed to nutrient solutions containing two levels of Pi, 1 mM Pi (Pi-sufficient) or 2.5 μM Pi (Pi-deficient) for 10 days. (A) Results from DAB staining to detect H2O2. Scale bar = 2 mm. (B) H2O2content. (C) Superoxide dismutase (SOD) activity. (D) Peroxidase (POD) activity. (E) Ascorbate peroxidase (APX) activity. The root tips of wild-type (WT) and Gm6PGDH1-overexpressing lines (OX-1, OX-2, and OX-3) are used as substrates for detection. These data were measured on day 10 of both Pi treatments. Data are means of the three replicates with SD. Asterisks show that the values are significantly different between the transgenic lines and the WT at the same time point (Student’s t-test, *P < 0.05, **P < 0.01, ***P < 0.001).
To further elucidate the mechanisms underlying the regulation of antioxidant system by Gm6PGDH1, RT-qPCR was performed to monitor the expression of six oxidative stress-related genes identified by previous transcriptome to be associated with Pi starvation (Zeng et al., 2016). Under Pi-sufficient conditions, the expression levels of these genes showed no significant difference between Gm6PGDH1-overexpressing lines and WT, except that the expression levels of Gm03g36620 and Gm09g02590 were increased in some transgenic soybean lines compared with the WT (Figures 6A–F). At the same time, under Pi-deficient conditions, the transcription level of Gm06g11720 and Gm08g05680 increased (Figures 6A,B), while the transcription level of Gm03g36620, Gm09g02590, Gm09g02650, and Gm15g13491 decreased (Figures 6C–F), which was similar to the results of previous studies (Zeng et al., 2016). Interestingly, the expression of these genes in response to Pi starvation was attenuated in transgenic lines (Figures 6A–F). For example, the expression of Gm06g11720 under Pi-deficient conditions was 2.32 times than that under Pi-sufficient conditions in WT, while the expression of Gm06g11720 under Pi-deficient conditions was 2.14, 1.49, and 2.28 times more than that under Pi-sufficient conditions in OX-1, OX-2, and OE-3, respectively (Figure 6A).
Figure 6. Changes in transcript levels for genes involved in the antioxidant system of soybean root tips during Pi starvation. Five-day-old soybean seedlings (removal of cotyledons) were exposed to nutrient solutions containing two levels of Pi, 1 mM Pi (Pi-sufficient) or 2.5 μM Pi (Pi-deficient) for 10 days. (A) Gm06g11720, (B) Gm08g05680, (C) Gm03g36620, (D) Gm09g02590, (E) Gm09g02650, (F) Gm15g13491. Actin was used as an internal control. Data are means of the three replicates with SD. Means with different letters are significantly different (one-way ANOVA, Duncan, P < 0.05).
To further characterize the role of Gm6PGDH1 in soybean response to Pi starvation, we attempted to knock down Gm6PGDH1 by virus-induced gene silencing (VIGS). VIGS mediated by Beanpod mottle virus (BPMV) has been successfully performed to study the function of soybean genes involved in defense and other processes, such as GmMPK4s, GmWRKY58, and GmWRKY76 (Liu et al., 2011; Yang et al., 2016). Two weeks post virus inoculation, some plants showed fold deformation of leaf margin (Supplementary Figure 5A), and RT–qPCR analysis showed that the plants inoculated with BPMV-Gm6PGDH1 vectors displayed a reduction of approximately 60% in the transcript levels of Gm6PGDH1 when compared with control plants (Supplementary Figure 5B). The identified plants were subjected to Pi starvation for 7 days (Supplementary Figure 5C). There was no significant difference in the dry weight and total P concentration of the leaves and roots between BPMV-Gm6PGDH1-silenced plants and control plants (Supplementary Figures 5D,E). These results showed that the VIGS of Gm6PGDH1 did not affect dry weight and total P concentration of soybean leaves and roots under low Pi conditions.
In order to further verify the role of Gm6PGDH1 in plant resistance to Pi starvation, Gm6PGDH1-overexpressing Arabidopsis plants were constructed. Seeds of T3 transgenic Arabidopsis and the WT were vertically cultured in a plant gel medium with high Pi (1 mM Pi) or low Pi (62.5 μM Pi) for 14 days. Under high Pi conditions, there was no significant difference in the growth status between the transgenic Arabidopsis lines and WT (Figures 7A,B). However, under low Pi conditions, the transgenic Arabidopsis lines grew much better than WT (Figure 7A). The fresh weight of the transgenic Arabidopsis lines was greater than that of WT under low Pi conditions (Figure 7B). At the same time, we found that the short-term low Pi supply significantly inhibited the growth of Arabidopsis, especially the root growth (Figure 7A), indicating that the length of primary and lateral roots length of plants grown with low Pi was shorter (Figures 7A,C,D). However, the primary and lateral roots of the transgenic Arabidopsis lines were significantly longer than those of the WT after 14 days of treatment with low Pi (Figures 7C,D). Furthermore, the soluble Pi concentration of the transgenic plants was significantly higher than that of WT under low Pi conditions (Figure 7E). These results indicated that the overexpression of Gm6PGDH1 significantly promoted root system development and Pi accumulation in transgenic Arabidopsis under low Pi conditions.
Figure 7. Overexpression of Gm6PGDH1 improved root system development and Pi accumulation, and affected the oxidative damage in Arabidopsis under low Pi conditions. (A) Wild-type (WT) and Gm6PGDH1-overexpressing transgenic Arabidopsis (OE-1, OE-2, and OE-3) were grown in a Hoagland medium with 1 mM Pi or 62.5 μM Pi on vertically oriented Petri dishes for 14 days. Bars = 1 cm. (B–E) The fresh weight, primary root length, total lateral root length and soluble Pi concentration were determined in WT and transgenic Arabidopsis plants treated as above. Three independent experiments were performed. (F) Photographs showing DAB staining of 7-day-old WT and transgenic Arabidopsis seedlings treated with a medium with 1 mM Pi and 0 mM Pi for 36 h, respectively. Bars = 1 mm. H2O2 levels (G) and enzyme activity of nicotinamide adenine dinucleotide phosphate (NADPH) (H) in all lines treated as (F). Data are means ± SD (n = 3). Data significantly different from the corresponding controls are indicated (Student’s t-test, *P < 0.05; **P < 0.01; ***P < 0.001).
Then, the ROS levels in the Gm6PGDH1-overexpressing Arabidopsis lines and WT were explored. We treated 7-day-old WT and transgenic Arabidopsis lines in an agar medium with high Pi (1 mM Pi) and low Pi (0 mM Pi) for 36h, then used DAB to stain the root tips of all the plants. Under low Pi conditions, the color of plant root was heavier, reflecting the elevated level of ROS (Figure 7F). However, the slighter coloration of DAB staining was observed in transgenic Arabidopsis lines root tip compared with WT under low Pi conditions (Figure 7F). Meanwhile, quantitative measurements showed that the content of H2O2 in the transgenic Arabidopsis lines was markedly lower than that in the WT under low Pi conditions (Figure 7G). Furthermore, we measured the NADPH content of the plants, since the OPPP contributes to the production of NADPH to support antioxidant enzyme activity (Valderrama et al., 2006; Lehmann et al., 2009). We found that the content of NADPH in the transgenic Arabidopsis lines was higher than that in WT under low Pi conditions (Figure 7H). These results suggested that the overexpression of Gm6PGDH1 might alleviate the root tip ROS accumulation by improving the content of NADPH under low Pi conditions.
The plant OPPP has been shown to be related to plant growth and development, and to have responded to a variety of environmental stresses (Šindelář et al., 1999; Nemoto and Sasakuma, 2000; Spielbauer et al., 2013; Long et al., 2016). However, as one of the two key enzymes of the OPPP, compared with G6PDH, botanists pay less attention to 6PGDH. In this study, we cloned a cytosolic 6PGDH gene from soybean and named it Gm6PGDH1. Prokaryotic induction and enzyme activity determination of Gm6PGDH1 indicated that it was a protein with 6PGDH activity, and that it was like the previously reported 6PGDH (Redinbaugh and Campbell, 1998). The RT-qPCR results showed that Gm6PGDH1 was widely expressed in various tissues of soybean, and that the expression level was highest in roots at the R2 stage, which was different from the expression pattern of Os6PGDH1 in rice. The Os6PGDH1 expression was high in inflorescence, low in the root and embryos, and almost absent in the leaf (Huang et al., 2003). Furthermore, the Gm6PGDH1 expression in the root and leaf at the R2 stage has higher levels than at other stages, which may be related to the increased dependence of soybean on OPPP. The reason is that soybean grows vigorous during this period, and OPPP can promote cell division and biosynthesis (Wu et al., 2015). It is worth noting that the phylogenetic analysis and amino acid sequence alignment indicate that Gm6PGDH1 was most closely related to At6PGDH2 (Figures 1B,C); however, they might not be functionally similar. Previous studies have shown that At6PGDH2 was related to fertility (Hölscher et al., 2016), and the conclusion of this research was that Gm6PGDH1 was involved in Pi starvation tolerance. Therefore, we speculated that 6PGDH of the different plants might have different expression modes and functions, even if they were relatively conservative.
Previous studies have shown that the transcription level of Os6PGDH1 was induced by abiotic stresses such as high salt, low temperature, drought, and ABA (Hou et al., 2007), and we have found that Gm6PGDH1 was strongly induced by Pi starvation. These results suggested that 6PGDH might be widely involved in plant resistance to abiotic stress, but we still did not know exactly how 6PGDH works. In this study, the overall data clearly demonstrated that Gm6PGDH1 enhanced the tolerance of soybean to Pi starvation by increasing the root length and the number of root tips, as well as enhancing the activity of the ROS-scavenging enzymes to reduce ROS (H2O2) accumulation. A recent study showed that a low-Pi tolerant genotype (NN94156) soybean had a larger root system at low Pi levels compared with a low-Pi sensitive genotype (Bogao) soybean, and transcriptome analysis results suggested that NN94156 had more oxidation-reduction processes than Bogao under low Pi conditions (Zhang et al., 2020), which was similar to the results of this study. However, interestingly, the expression of several other important redox-related genes appeared to be inhibited in the root tip of the overexpressed Gm6PGDH1 soybean lines under low Pi conditions. This seemed to contradict the data that the root tips of Gm6PGDH1-overexpressing transgenic soybean lines had a higher activity of ROS-scavenging enzymes and less ROS level compared with those of WT. The possible explanation was that the positive effect of Gm6PGDH1 on root development and the antioxidant system promoted Pi uptake, thus improving the Pi balance in the root system, and leading to interference in the response of some redox-related genes to Pi starvation, which was like performance of the low phosphorus insensitive mutants (Sanchezcalderon et al., 2006). In addition, in the hair root of the transgenic soybean composite plants, some Pi-starvation inducible GmPAP genes were also inhibited in response to low Pi, which further proved that the expression change in Gm6PGDH1 would produce crosstalk to the other Pi-answer genes.
It is generally believed that low Pi will change root structure, leading to suppressed primary roots and enhanced lateral root development (Péret et al., 2011). However, in this study, the overexpression of Gm6PGDH1 had a positive effect on the development of both primary and lateral roots in transgenic Arabidopsis under low Pi conditions, suggesting that the regulation of Gm6PGDH1 in the root system might be independent under low Pi conditions. Further studies have shown that the accumulation of ROS (H2O2) at the root tip of transgenic soybeans and Arabidopsis decreased compared with WT under low Pi conditions, so we speculated that the positive effect of Gm6PGDH1 on plant root might be related to the ROS homeostatic state of the root tip (Tsukagoshi et al., 2010). Low Pi has been demonstrated to promote ROS accumulation, which stiffens the cell wall and represses root growth (Balzergue et al., 2017). Recent evidence demonstrated that H2O2, low pH, malate, iron accumulation, and blue light are all indispensable for primary root response under Pi starvation conditions in Arabidopsis (Zheng et al., 2019). This study showed that the overexpression of the Gm6PGDH1 gene in Arabidopsis decreases the H2O2 content in the root tip, and this may explain why the transgenic lines show longer primary roots compare with WT under phosphate starvation conditions according to the former results. In plant cells, ROS can be scavenged through several major ROS-scavenging enzymes, such as SOD, POD, and APX (Foyer and Noctor, 2005), and NADPH is also a key component in regulating the balance of ROS in cells (Ying, 2008; Jiao et al., 2013; Yang et al., 2019b). The results of enzyme activity determination showed that the activities of SOD, POD, and APX were enhanced in the root tip of transgenic soybeans compared with WT, and that NADPH content was enhanced in transgenic Arabidopsis compared with WT under low Pi conditions. In addition, the Gm6PGDH1-overexpressing transgenic soybean lines have a higher root activity than WT under low Pi conditions, which further supports the opinion of the authors.
It is worth noting that The VIGS of Gm6PGDH1 had no effect on dry weight and total P concentration of the plants under low Pi conditions, suggesting that function redundancy might be present for Gm6PGDH1 in soybean. In previous studies, double mutants of the maizecytosolic isozymes, PGD1 and PGD2, had no obvious phenotype, and the roots of the mutants still had 32% of the catalytic capacity of 6PGDH of the wild-type plants (Bailey-Serres et al., 1992; Averill et al., 1998), so we guess that, partly, soybean plastidic 6PGDH may complement some functions of Gm6PGDH1, but this needs further verification in the later stage. In addition, it is worth investigating whether any other genes in this family were involved in regulating phosphorus deficiency in plants. In addition, results from a heterogeneous transformation system showed that overexpression of Gm6PGDH1 enhanced tolerance to Pi starvation in transgenic Arabidopsis by improving root system development and reducing ROS accumulation, which proved that the positive effect of Gm6PGDH1 for plant to Pi starvation was not limited to soybean. Therefore, it will be interesting for a study to use Gm6PGDH1 to enhance the Pi starvation resistance of other plants and to explore whether the 6PGDH of other plants has a similar function (Ding et al., 2020).
In conclusion, we report the cloning and functional characterization of a cytosolic 6PGDH gene, Gm6PGDH1, and show that this gene may play an important role in the internal regulation of root development and antioxidant system under low Pi stress conditions. Meanwhile, this study provides new evidence for the role of ROS homeostasis balance in root system development under low Pi environment conditions, and a new idea for the development of soybean materials with low Pi tolerance. In addition, due to differences in soil environment and laboratory conditions, further studies to see if the advantage conferred by the altered expression of Gm6PGDH1 is maintained in soil-based systems are being carried out in the laboratory.
The original contributions presented in the study are included in the article/Supplementary Material, further inquiries can be directed to the corresponding author/s.
CL and SY designed the experiments and drafted and edited the manuscript. CL, KL, MZ, XL, and XD performed the experiments. CL processed the data and analyzed the corresponding results. JG reviewed the manuscript. All authors approved the final version of the study.
This study was supported by the National Transgene Science and Technology Major Program of China (2016ZX08004-005), the Fundamental Research Funds for the Central Universities (KYT201801), and the Program for Changjiang Scholars and Innovative Research Team in University (PCSIRT_17R55).
The authors declare that the research was conducted in the absence of any commercial or financial relationships that could be construed as a potential conflict of interest.
All claims expressed in this article are solely those of the authors and do not necessarily represent those of their affiliated organizations, or those of the publisher, the editors and the reviewers. Any product that may be evaluated in this article, or claim that may be made by its manufacturer, is not guaranteed or endorsed by the publisher.
We thank Jianzhong Liu and Steven A. Whitham for the BPMVVIGS vectors.
The Supplementary Material for this article can be found online at: https://www.frontiersin.org/articles/10.3389/fpls.2021.704983/full#supplementary-material
Supplementary Figure 1 | Expression levels of Gm6PGDH1 in hairy roots of soybean composite plants.
Supplementary Figure 2 | Expression levels of several GmPAP genes in Gm6PGDH1-overexpressing composite soybean plants.
Supplementary Figure 3 | The Gm6PGDH1-overexpressing transgenic soybean was identified by PCR and quantitative real-time PCR (RT-qPCR).
Supplementary Figure 4 | Root activity was detected by trypan blue staining.
Supplementary Figure 5 | Silencing Gm6PGDH1 had no effect on tolerance to phosphate starvation in soybean.
Supplementary Figure 6 | The Gm6PGDH1-overexpressing transgenic Arabidopsis was identified by PCR and RT-qPCR.
Supplementary Table 1 | Primer sequences were used for vector construction and detection.
Supplementary Table 2 | Primer sequences for qRT-PCR analysis in soybean.
Supplementary Table 3 | Primer sequences for qRT-PCR analysis in Arabidopsis.
And, H. E. N., and Emes, M. J. (2000). Nonphotosynthetic metabolism in plastids. Annu. Rev. Plant. Physiol. Plant. Mol. Biol. 51, 111–140. doi: 10.1146/annurev.arplant.51.1.111
Ap Rees, T. (1985). ”The organization of glycolysis and the oxidative pentose phosphate pathway in plants,” in Higher plant cell respiration. Cham: Springer, 391–417.
Attila, K., Dongxue, L., Arief, I., Nguyen, C. D. T., Sureeporn, N., Mark, K., et al. (2007). Agrobacterium rhizogenes-mediated transformation of soybean to study root biology. Nat. Protocols 2, 948–952. doi: 10.1038/nprot.2007.141
Averill, R. H., Bailey-Serres, J., and Kruger, N. J. (1998). Co-operation between cytosolic and plastidic oxidative pentose phosphate pathways revealed by 6-phosphogluconate dehydrogenase-deficient genotypes of maize. Plant J. 14, 449–457. doi: 10.1046/j.1365-313X.1998.00143.x
Bailey-Serres, J., Tom, J., and Freeling, M. (1992). Expression and distribution of cytosolic 6-phosphogluconate dehydrogenase isozymes in maize. Biochem. Genet. 30, 233–246. doi: 10.1007/BF02396214
Balzergue, C., Dartevelle, T., Godon, C., Laugier, E., Meisrimler, C., Teulon, J., et al. (2017). Low phosphate activates STOP1-ALMT1 to rapidly inhibit root cell elongation. Nat. Commun. 8:15300. doi: 10.1038/ncomms15300
Bennett, E. M., Carpenter, S. R., and Caraco, N. F. (2001). Human impact on erodable phosphorus and eutrophication: a global perspective: increasing accumulation of phosphorus in soil threatens rivers, lakes, and coastal oceans with eutrophication. BioScience 51, 227–234.
Chevalier, F., Pata, M., Nacry, P., Doumas, P., and Rossignol, M. (2003). Effects of phosphate availability on the root system architecture: large-scale analysis of the natural variation between Arabidopsis accessions. Plant Cell Environ. 26, 1839–1850. doi: 10.1046/j.1365-3040.2003.01100.x
Clough, S. J., and Bent, A. F. (1998). Floral dip: a simplified method for Agrobacterium-mediated transformation of Arabidopsis thaliana. Plant J. 16, 735–743. doi: 10.1046/j.1365-313x.1998.00343.x
Cong, W.-F., Suriyagoda, L. D., and Lambers, H. (2020). Tightening the phosphorus cycle through phosphorus-efficient crop genotypes. Trends Plant Sci. 25, 967–975. doi: 10.1016/j.tplants.2020.04.013
Dai, X., Wang, Y., Yang, A., and Zhang, W.-H. (2012). OsMYB2P-1, an R2R3 MYB transcription factor, is involved in the regulation of phosphate-starvation responses and root architecture in rice. Plant Physiol. 159, 169–183. doi: 10.1104/pp.112.194217
Daudi, A., and O’Brien, J. A. (2012). Detection of hydrogen peroxide by DAB staining in Arabidopsis leaves. Bio. Protoc. 2, 1–4. doi: 10.21769/BioProtoc.263
Devaiah, B. N., Madhuvanthi, R., Karthikeyan, A. S., and Raghothama, K. G. (2009). Phosphate starvation responses and gibberellic acid biosynthesis are regulated by the MYB62 transcription factor in Arabidopsis. Mol. Plant 2, 43–58. doi: 10.1093/mp/ssn081
Ding, L. N., Li, M., Guo, X. J., Tang, M. Q., Cao, J., Wang, Z., et al. (2020). Arabidopsis GDSL1 overexpression enhances rapeseed Sclerotinia sclerotiorum resistance and the functional identification of its homolog in Brassica napus. Plant Biotechnol. J. 18, 1255–1270. doi: 10.1111/pbi.13289
Dvorak, P., Krasylenko, Y., Zeiner, A., Samaj, J., and Takac, T. (2020). Signaling toward ROS-scavenging enzymes in plants. Front. Plant Sci. 11:618835. doi: 10.3389/fpls.2020.618835
Foyer, C. H., and Noctor, G. (2005). Redox homeostasis and antioxidant signaling: a metabolic interface between stress perception and physiological responses. Plant Cell. 17, 1866–1875. doi: 10.1105/tpc.105.033589
Gill, S. S., and Tuteja, N. (2010). Reactive oxygen species and antioxidant machinery in abiotic stress tolerance in crop plants. Plant Physiol. Biochem. 48, 909–930. doi: 10.1016/j.plaphy.2010.08.016
Gutierrezalanis, D., Ojedarivera, J. O., Yongvillalobos, L., Cardenastorres, L., and Herreraestrella, L. (2018). Adaptation to phosphate scarcity: tips from arabidopsis roots. Trends Plant Sci. 23, 721–730. doi: 10.1016/j.tplants.2018.04.006
Hammond, J. P., Broadley, M. R., and White, P. J. (2004). Genetic responses to phosphorus deficiency. Ann. Bot. 94, 323–332.
Hinsinger, P. (2001). Bioavailability of soil inorganic P in the rhizosphere as affected by root-induced chemical changes: a review. Plant Soil 237, 173–195. doi: 10.1023/A:1013351617532
Hölscher, C., Lutterbey, M.-C., Lansing, H., Meyer, T., Fischer, K., and von Schaewen, A. (2016). Defects in peroxisomal 6-phosphogluconate dehydrogenase isoform PGD2 prevent gametophytic interaction in Arabidopsis thaliana. Plant Physiol. 171, 192–205. doi: 10.1104/pp.15.01301
Hou, F. Y., Huang, J., Yu, S. L., and Zhang, H. S. (2007). The 6-phosphogluconate dehydrogenase genes are responsive to abiotic stresses in rice. J. Integr. Plant Biol. 49, 655–663. doi: 10.1111/j.1744-7909.2007.00460.x
Huang, H., Ullah, F., Zhou, D.-X., Yi, M., and Zhao, Y. (2019). Mechanisms of ROS regulation of plant development and stress responses. Front. Plant Sci. 10:800. doi: 10.3389/fpls.2019.00800
Huang, J., Zhang, H., Wang, J., and Yang, J. (2003). Molecular cloning and characterization of rice 6-phosphogluconate dehydrogenase gene that is up-regulated by salt stress. Mol. Biol. Rep. 30, 223–227. doi: 10.1023/A:1026392422995
Jain, A., Vasconcelos, M. J., Raghothama, K., and Sahi, S. V. (2007). Molecular mechanisms of plant adaptation to phosphate deficiency. Plant Breed. Rev. 29:359. doi: 10.1002/9780470168035.ch7
Jiao, Y., Sun, L., Song, Y., Wang, L., Liu, L., Zhang, L., et al. (2013). AtrbohD and AtrbohF positively regulate abscisic acid-inhibited primary root growth by affecting Ca2+ signalling and auxin response of roots in Arabidopsis. J. Exper. Bot. 64, 4183–4192. doi: 10.1093/jxb/ert228
Kong, Y., Li, X., Wang, B., Li, W., Du, H., and Zhang, C. (2018). The soybean purple acid phosphatase GmPAP14 predominantly enhances external phytate utilization in plants. Front. Plant Sci. 9:292. doi: 10.3389/fpls.2018.00292
Kruger, N. J., and Schaewen, A. V. (2003). The oxidative pentose phosphate pathway: structure and organisation. Curr. Opin. Plant Biol. 6, 236–246. doi: 10.1016/S1369-5266(03)00039-6
Lehmann, M., Schwarzländer, M., Obata, T., Sirikantaramas, S., Burow, M., Olsen, C. E., et al. (2009). The metabolic response of Arabidopsis roots to oxidative stress is distinct from that of heterotrophic cells in culture and highlights a complex relationship between the levels of transcripts, metabolites, and flux. Mol. Plant 2, 390–406. doi: 10.1093/mp/ssn080
Li, C., Gui, S., Yang, T., Walk, T., Wang, X., and Liao, H. (2012). Identification of soybean purple acid phosphatase genes and their expression responses to phosphorus availability and symbiosis. Ann. Bot. 109, 275–285. doi: 10.1093/aob/mcr246
Li, C., Li, C., Zhang, H., Liao, H., and Wang, X. (2017a). The purple acid phosphatase GmPAP21 enhances internal phosphorus utilization and possibly plays a role in symbiosis with rhizobia in soybean. Physiol. Plantarum 159, 215–227. doi: 10.1111/ppl.12524
Li, S., Cong, Y., Liu, Y., Wang, T., Shuai, Q., Chen, N., et al. (2017b). Optimization of Agrobacterium-mediated transformation in soybean. Front. Plant Sci. 8:246. doi: 10.3389/fpls.2017.00246
Li, C., Liu, X., Ruan, H., Zhang, J., Xie, F., Gai, J., et al. (2020). GmWRKY45 enhances tolerance to phosphate starvation and salt stress, and changes fertility in transgenic arabidopsis. Front. Plant Sci. 10:1714. doi: 10.3389/fpls.2019.01714
Liang, C., Tian, J., Lam, H.-M., Lim, B. L., Yan, X., and Liao, H. (2010). Biochemical and molecular characterization of PvPAP3, a novel purple acid phosphatase isolated from common bean enhancing extracellular ATP utilization. Plant Physiol. 152, 854–865. doi: 10.2307/25680699
Liu, J., Wang, X., Hu, Y., Hu, W., and Bi, Y. (2013). Glucose-6-phosphate dehydrogenase plays a pivotal role in tolerance to drought stress in soybean roots. Plant Cell. Rep. 32, 415–429. doi: 10.1007/s00299-012-1374-1
Liu, J.-Z., Horstman, H. D., Braun, E., Graham, M. A., Zhang, C., Navarre, D., et al. (2011). Soybean homologs of MPK4 negatively regulate defense responses and positively regulate growth and development. Plant Physiol. 157, 1363–1378. doi: 10.1104/pp.111.185686
Long, X., He, B., Fang, Y., and Tang, C. (2016). Identification and characterization of the glucose-6-phosphate dehydrogenase gene family in the para rubber tree, Hevea brasiliensis. Front. Plant Sci. 7:215. doi: 10.3389/fpls.2016.00215
Luo, S., Tang, Z., Yu, J., Liao, W., Xie, J., Lv, J., et al. (2020). Hydrogen sulfide negatively regulates cd-induced cell death in cucumber (Cucumis sativus L) root tip cells. BMC Plant Biol. 20:480. doi: 10.1186/s12870-020-02687-8
Matsumura, H., and Miyachi, S. (1980). ”[43] Cycling assay for nicotinamide adenine dinucleotides,” in Methods in enzymology. Amsterdam: Elsevier, 465–470.
Misson, J., Raghothama, K. G., Jain, A., Jouhet, J., Block, M. A., Bligny, R., et al. (2005). A genome-wide transcriptional analysis using Arabidopsis thaliana Affymetrix gene chips determined plant responses to phosphate deprivation. Proc. Natl. Acad. Sci. 102, 11934–11939. doi: 10.1073/pnas.0505266102
Nadarajah, K. K. (2020). ROS homeostasis in abiotic stress tolerance in plants. Int. J. Mol. Sci. 21:5208. doi: 10.3390/ijms21155208
Nemoto, Y., and Sasakuma, T. (2000). Specific expression of glucose-6-phosphate dehydrogenase (G6PDH) gene by salt stress in wheat (Triticum aestivum L.). Plant Sci. 158, 53–60. doi: 10.1016/S0168-9452(00)00305-8
Ohlrogge, A. J. (1960). Mineral nutrition of soybeans. Adv. Agron. 12, 229–263. doi: 10.1016/S0065-2113(08)60084-X
Oldroyd, G. E., and Leyser, O. (2020). A plant’s diet, surviving in a variable nutrient environment. Science 368:eaba0196. doi: 10.1126/science.aba0196
Péret, B., Clément, M., Nussaume, L., and Desnos, T. (2011). Root developmental adaptation to phosphate starvation: better safe than sorry. Trends Plant Sci. 16, 442–450. doi: 10.1016/j.tplants.2011.05.006
Pflieger, S., Blanchet, S., Meziadi, C., Richard, M. M., and Geffroy, V. (2015). Bean pod mottle virus (BPMV) viral inoculation procedure in common bean (Phaseolus vulgaris L.). Bio-Protocol 5:e1524. doi: 10.21769/BioProtoc.1524
Raghothama, K. G. (1999). PHOSPHATE ACQUISITION. Annu. Rev. Plant Biol. 50, 665–693. doi: 10.1007/s11104-004-2005-6
Redinbaugh, M. G., and Campbell, W. H. (1998). Nitrate regulation of the oxidative pentose phosphate pathway in maize (Zea mays L.) root plastids: induction of 6-phosphogluconate dehydrogenase activity, protein and transcript levels. Plant Sci. 134, 129–140. doi: 10.1016/S0168-9452(98)00048-X
Saed-Moucheshi, A., Shekoofa, A., and Pessarakli, M. (2014). Reactive Oxygen Species (ROS) generation and detoxifying in plants. J. Plant Nutr. 37, 1573–1585. doi: 10.1080/01904167.2013.868483
Sample, E., Soper, R., and Racz, G. (1980). Reactions of phosphate fertilizers in soils. Role Phosph. Agric. 1980, 263–310. doi: 10.2134/1980.roleofphosphorus.c12
Sanchezcalderon, L., Lopezbucio, J., Chaconlopez, A., Gutierrezortega, A., Hernandezabreu, E., and Herreraestrella, L. (2006). Characterization of low phosphorus insensitive mutants reveals a crosstalk between low phosphorus-induced determinate root development and the activation of genes involved in the adaptation of arabidopsis to phosphorus deficiency. Plant Physiol. 140, 879–889. doi: 10.1104/pp.105.073825
Schachtman, D. P., Reid, R. J., and Ayling, S. M. (1998). Phosphorus uptake by plants: from soil to cell. Plant Physiol. 116, 447–453. doi: 10.1104/pp.116.2.447
Schieber, M., and Chandel, N. S. (2014). ROS function in redox signaling and oxidative stress. Curr. Biol. 24, 453–462. doi: 10.1016/j.cub.2014.03.034
Shen, C., Wang, S., Zhang, S., Xu, Y., Qian, Q., Qi, Y., et al. (2013). OsARF16, a transcription factor, is required for auxin and phosphate starvation response in rice (Oryza sativa L.). Plant Cell. Environ. 36, 607–620. doi: 10.1111/pce.12001
Shin, R., Berg, R. H., and Schachtman, D. P. (2005). Reactive oxygen species and root hairs in Arabidopsis root response to nitrogen, phosphorus and potassium deficiency. Plant Cell Physiol. 46, 1350–1357. doi: 10.1093/pcp/pci145
Šindelář, L., Šindelářová, M., and Burketová, L. (1999). Changes in activity of glucose-6-phosphate and 6-phosphogluconate dehydrogenase isozymes upon potato virus Y infection in tobacco leaf tissues and protoplasts. Plant Physiol. Biochem. 37, 195–201. doi: 10.1016/S0981-9428(99)80034-5
Spielbauer, G., Li, L., Römisch-Margl, L., Do, P. T., Fouquet, R., Fernie, A. R., et al. (2013). Chloroplast-localized 6-phosphogluconate dehydrogenase is critical for maize endosperm starch accumulation. J. Exper. Bot. 64, 2231–2242. doi: 10.1093/jxb/ert082
Tanksley, S. D., and Kuehn, G. D. (1985). Genetics, subcellular localization, and molecular characterization of 6-phosphogluconate dehydrogenase isozymes in tomato. Biochem. Genet. 23, 441–454. doi: 10.1007/BF00499085
Tomscha, J. L., Trull, M. C., Deikman, J., Lynch, J. P., and Guiltinan, M. J. (2004). Phosphatase under-producer mutants have altered phosphorus relations. Plant Physiol. 135, 334–345. doi: 10.1104/pp.103.036459
Tsukagoshi, H., Busch, W., and Benfey, P. N. (2010). Transcriptional regulation of ROS controls transition from proliferation to differentiation in the root. Cell 143, 606–616. doi: 10.1016/j.cell.2010.10.020
Valderrama, R., Corpas, F. J., Carreras, A., Gomezrodriguez, M. V., Chaki, M., Pedrajas, J. R., et al. (2006). The dehydrogenase-mediated recycling of NADPH is a key antioxidant system against salt-induced oxidative stress in olive plants. Plant Cell. Environ. 29, 1449–1459. doi: 10.1111/j.1365-3040.2006.01530.x
Veneklaas, E. J., Lambers, H., Bragg, J., Finnegan, P. M., Lovelock, C. E., Plaxton, W. C., et al. (2012). Opportunities for improving phosphorus-use efficiency in crop plants. New Phytol. 195, 306–320. doi: 10.1111/j.1469-8137.2012.04190.x
Waszczak, C., Carmody, M., and Kangasjärvi, J. (2018). Reactive oxygen species in plant signaling. Annu. Rev. Plant Biol. 69, 209–236. doi: 10.1146/annurev-arplant-042817-040322
Wei, M., Ge, Y., Li, C., Han, X., Qin, S., Chen, Y., et al. (2019). G6PDH regulated NADPH production and reactive oxygen species metabolism to enhance disease resistance against blue mold in apple fruit by acibenzolar-S-methyl. Postharvest Biol. Technol. 148, 228–235. doi: 10.1016/j.postharvbio.2018.05.017
Wu, P., Ma, L., Hou, X., Wang, M., Wu, Y., Liu, F., et al. (2003). Phosphate starvation triggers distinct alterations of genome expression in Arabidopsis roots and leaves. Plant Physiol. 132, 1260–1271. doi: 10.1104/pp.103.021022
Wu, S., Huang, A., Zhang, B., Huan, L., Zhao, P., Lin, A., et al. (2015). Enzyme activity highlights the importance of the oxidative pentose phosphate pathway in lipid accumulation and growth of Phaeodactylum tricornutum under CO2 concentration. Biotechnol. Biofuels 8, 1–11. doi: 10.1186/s13068-015-0262-7
Xue, Y.-B., Xiao, B.-X., Zhu, S.-N., Mo, X.-H., Liang, C.-Y., Tian, J., et al. (2017). GmPHR25, a GmPHR member up-regulated by phosphate starvation, controls phosphate homeostasis in soybean. J. Exper. Bot. 68, 4951–4967. doi: 10.1093/jxb/erx292
Yang, L., Wang, S., Sun, L., Ruan, M., Li, S., He, R., et al. (2019a). Involvement of G6PD5 in ABA response during seed germination and root growth in Arabidopsis. BMC Plant Biol. 19:44. doi: 10.1186/s12870-019-1647-8
Yang, L., Wang, X., Chang, N., Nan, W., Wang, S., Ruan, M., et al. (2019b). Cytosolic glucose-6-phosphate dehydrogenase is involved in seed germination and root growth under salinity in Arabidopsis. Front. Plant Sci. 10:182. doi: 10.3389/fpls.2019.00182
Yang, Y., Chi, Y., Wang, Z., Zhou, Y., Fan, B., and Chen, Z. (2016). Functional analysis of structurally related soybean GmWRKY58 and GmWRKY76 in plant growth and development. J. Exper. Bot. 67, 4727–4742. doi: 10.1093/jxb/erw252
Yi, K., Wu, Z., Zhou, J., Du, L., Guo, L., Wu, Y., et al. (2005). OsPTF1, a novel transcription factor involved in tolerance to phosphate starvation in rice. Plant Physiol. 138, 2087–2096. doi: 10.1104/pp.105.063115
Ying, W. (2008). NAD+/NADH and NADP+/NADPH in cellular functions and cell death: regulation and biological consequences. Antioxidants Redox Signal. 10, 179–206. doi: 10.1089/ars.2007.1672
Yoo, S.-D., Cho, Y.-H., and Sheen, J. (2007). Arabidopsis mesophyll protoplasts: a versatile cell system for transient gene expression analysis. Nat. Protocols 2:1565. doi: 10.1038/nprot.2007.199
Zeng, H., Wang, G., Zhang, Y., Hu, X., Pi, E., Zhu, Y., et al. (2016). Genome-wide identification of phosphate-deficiency-responsive genes in soybean roots by high-throughput sequencing. Plant Soil 398, 207–227. doi: 10.1007/s11104-015-2657-4
Zhang, C., Bradshaw, J. D., Whitham, S. A., and Hill, J. H. (2010). The development of an efficient multipurpose bean pod mottle virus viral vector set for foreign gene expression and RNA silencing. Plant Physiol. 153, 52–65. doi: 10.1104/pp.109.151639
Zhang, D., Song, H., Cheng, H., Hao, D., Wang, H., Kan, G., et al. (2014). The acid phosphatase-encoding gene GmACP1 contributes to soybean tolerance to low-phosphorus stress. PLoS Genet. 10:e04061. doi: 10.1371/journal.pgen.1004061
Zhang, H., Cao, Y., Zhao, J., Li, X., Xiao, J., and Wang, S. (2011). A pair of orthologs of a leucine-rich repeat receptor kinase-like disease resistance gene family regulates rice response to raised temperature. BMC Plant Biol. 11:160. doi: 10.1186/1471-2229-11-160
Zhang, H., Yang, Y., Sun, C., Liu, X., Lv, L., Hu, Z., et al. (2020). Up-regulating GmETO1 improves phosphorus uptake and use efficiency by promoting root growth in soybean. Plant Cell. Environ. 43, 2080–2094. doi: 10.1111/pce.13816
Zhang, J., Jiang, F., Shen, Y., Zhan, Q., Bai, B., Chen, W., et al. (2019). Transcriptome analysis reveals candidate genes related to phosphorus starvation tolerance in sorghum. BMC Plant Biol. 19:306. doi: 10.1186/s12870-019-1914-8
Zhang, L., Liu, J., Wang, X., and Bi, Y. (2013). Glucose-6-phosphate dehydrogenase acts as a regulator of cell redox balance in rice suspension cells under salt stress. Plant Growth Regul. 69, 139–148. doi: 10.1007/s10725-012-9757-4
Keywords: soybean (Glycine max), Gm6PGDH1, phosphate starvation, root system development, reactive oxygen species
Citation: Li C, Li K, Zheng M, Liu X, Ding X, Gai J and Yang S (2021) Gm6PGDH1, a Cytosolic 6-Phosphogluconate Dehydrogenase, Enhanced Tolerance to Phosphate Starvation by Improving Root System Development and Modifying the Antioxidant System in Soybean. Front. Plant Sci. 12:704983. doi: 10.3389/fpls.2021.704983
Received: 04 May 2021; Accepted: 22 July 2021;
Published: 13 August 2021.
Edited by:
Xiaojuan Li, Beijing Forestry University, ChinaReviewed by:
Zheng Zai, Tsinghua University, ChinaCopyright © 2021 Li, Li, Zheng, Liu, Ding, Gai and Yang. This is an open-access article distributed under the terms of the Creative Commons Attribution License (CC BY). The use, distribution or reproduction in other forums is permitted, provided the original author(s) and the copyright owner(s) are credited and that the original publication in this journal is cited, in accordance with accepted academic practice. No use, distribution or reproduction is permitted which does not comply with these terms.
*Correspondence: Shouping Yang, c3B5dW5nQDEyNi5jb20=
Disclaimer: All claims expressed in this article are solely those of the authors and do not necessarily represent those of their affiliated organizations, or those of the publisher, the editors and the reviewers. Any product that may be evaluated in this article or claim that may be made by its manufacturer is not guaranteed or endorsed by the publisher.
Research integrity at Frontiers
Learn more about the work of our research integrity team to safeguard the quality of each article we publish.