- State Key Laboratory of Crop Biology, Collaborative Innovation Center of Fruit & Vegetable Quality and Efficient Production in Shandong, College of Horticulture Science and Engineering, Shandong Agricultural University, Tai-an, China
Ozone (O3) stress severely affects the normal growth of grape (Vitis vinifera L.) leaves. Melatonin (MT) plays a significant role in plant response to various abiotic stresses, but its role in O3 stress and related mechanisms are poorly understood. In order to understand the mechanism of MT in alleviate O3 stress in grape leaves, we perform a transcriptome analyses of grapes leaves under O3 stress with or without MT treatment. Transcriptome analysis showed that the processes of ethylene biosynthesis and signaling were clearly changed in “Cabernet Sauvignon” grapes under O3 and MT treatment. O3 stress induced the expression of genes related to ethylene biosynthesis and signal transduction, while MT treatment significantly inhibited the ethylene response mediated by O3 stress. Further experiments showed that both MT and aminoethoxyvinylglycine (AVG, an inhibitor of ethylene biosynthesis) enhanced the photosynthetic and antioxidant capacities of grape leaves under O3 stress, while ethephon inhibited those capacities. The combined treatment effect of MT and ethylene inhibitor was similar to that of MT alone. Exogenous MT reduced ethylene production in grape leaves under O3 stress, while ethephon and ethylene inhibitors had little effect on the MT content of grape leaves after O3 stress. However, overexpression of VvACO2 (1-aminocyclopropane-1-carboxylate oxidase2) in grape leaves endogenously induced ethylene accumulation and aggravated O3 stress. Overexpression of the MT synthesis gene VvASMT1 (acetylserotonin methyltransferase1) in tobacco (Nicotiana tabacum L.) alleviated O3 stress and reduced ethylene biosynthesis after O3 stress. In summary, MT can alleviate O3 stress in grape leaves by inhibiting ethylene biosynthesis.
Introduction
Ozone (O3) in the troposphere is a highly oxidizing atmospheric pollutant. Elevated O3 concentration severely affects the growth and development of plants (Serengil et al., 2011), as well as human health (Karnosky et al., 2007; Borowiak, 2013). At present, the near-surface O3 concentration is increasing at an annual rate of 0.5–∼2.0% (Vingarzan, 2004) and is projected to increase by 40–60% at the end of the 21st century, when the tropospheric O3 concentration will reach 80 nL L–1 (Fiscus et al., 2005). O3 stress induces the release of large amounts of ethylene from leaf stomata, the damage of plant leaves caused by O3 is correlated with the release of ethylene (Tingey et al., 1976; Mehlhorn and Wellburn, 1987). As an important signal molecule, ethylene plays an important role in plant response to abiotic stress (Zhang M. et al., 2016). Ethylene biosynthesis begins with the formation of S-adenosyl-L-methionine (SAM) from methionine by SAM synthetase. Then, 1-aminocyclopropane-1-carboxylic acid (ACC) synthase (ACS) catalyzes SAM to produce ACC, and ACC oxidase (ACO) oxidizes ACC to ethylene (Najeeb et al., 2018).
Grapes (Vitis vinifera L.) are an important fruit crop grown worldwide. Previous investigation suggested that O3 stress adversely affects the photosynthetic system of grape (cultivar Cabernet Sauvignon) leaves, and exogenous MT treatment can alleviate O3 stress (Geng et al., 2016; Chen et al., 2020). Grapes and other plants have evolved various strategies to withstand abiotic stresses, such as regulating interactive hormone networks, including MT and ethylene (Arnao and Hernández-Ruiz, 2015; Ryu and Cho, 2015; Nguyen et al., 2016).
Melatonin is an indole derivative of tryptophan that is ubiquitous in plants and animals and has high efficiency, conservation, and strong antioxidant effects (Galano et al., 2011; Yang et al., 2018). MT synthesis in plants requires the participation of many enzymes, and the last step of the reaction is catalyzed by acetylserotonin o-methyltransferase (ASMT) (Kang et al., 2011). MT is an essential plant growth regulator, and both external application and endogenous induction can improve plant tolerance to drought, salinity, and other abiotic stresses (Zuo et al., 2014; Arnao and Hernández-Ruiz, 2015). For example, exogenous MT treatment can enhance the antioxidant capacity of cabbage (Brassica oleracea L.) (Zhang N. et al., 2016) and tea tree (Camellia sinensis L.) (Li et al., 2021) by increasing their anthocyanin content. In apple (Malus domestica), MT can regulate reactive oxygen species (ROS) signaling and activate the CBL1-CIPK23 (calcineurin B-like 1-interacting protein kinases23) pathway to regulate the expression of potassium channel protein genes, thereby improving salt tolerance (Li et al., 2016). Furthermore, exogenous MT can reduce the ion poisoning of mushrooms (Agaricus campestris) (Gao et al., 2020) and wheat (Triticum aestivum L.) (Al-Huqail et al., 2020). MT can also interact with other plant hormones [abscisic acid (ABA), jasmonic acid, salicylic acid, ethylene, etc.] to form a significant component of the plant immune system (Arnao and Hernández-Ruiz, 2018). For example, MT can increase GA (gibberellin) and reduce ABA content by regulating the expression of GA and ABA synthesis-related genes in cucumber seedlings and alleviating the inhibitory effect of a high salt environment on seedlings (Zhang et al., 2014). In Arabidopsis thaliana, MT reduces root meristem size by inhibiting auxin synthesis and polar transport (Wang Q. et al., 2016) and interacts with ethylene signaling pathways to improve disease resistance (Lee et al., 2014).
So far, the relationship between MT and other signaling molecules under abiotic stress is obscure, especially under O3 stress. Thus, this experiment has explored the key metabolic changes caused by increasing the MT content in grape leaves under O3 stress and its possible action mechanism. This research will promote the application of MT in improving the O3 tolerance of grapes and reveal the potential molecular mechanism of MT in regulating other signal molecules under O3 stress.
Materials and Methods
O3 Fumigation System
Two O3 fumigation systems were set up in the school vineyard (36°11′N, 117°06′E), which were divided into four parts, including open-top air chambers (OTCs), gas supply systems, O3 generation system, and O3 concentration monitoring system (Geng et al., 2017). The mainframe of the OTC is composed of galvanized steel pipes with a 3 cm diameter and is divided into two parts: the lower part is a regular octagonal prism with length and height of 1.1 and 2.2 m. The upper part is a regular octagonal pedestal; the area of the upper base of the pedestal is one in third of the area of the lower base, and the angle between the side and the vertical is 45°. The top is open to the atmosphere, and the sides are covered with particular polyethylene plastic film for the greenhouse; the outside is covered with a sunshade net. The installation height of the LED light source (LED cold light source plant light, SP501-N, 405 W, Shanghai Sanhao Electromechanical Co., Ltd.) is 1.5 m. To ensure the stability of the gas concentration in the OTC, the gap between the exhaust ports is gradually reduced from the center of the OTC to the four sides. The O3 generating system (WJ-HY5, Jinan Sankang) is a high-frequency O3 generator. The oxygen intake of the O3 generator can be modulated by adjusting the rotor flowmeter to control the O3 concentration. The O3 concentration monitor (DR70C-O3 type) in the OTC was used to measure the O3 concentration in real-time and transmits the data to the computer for observation and storage.
Plant Materials, Growth Conditions, and Experimental Treatments
Two-year-old potted seedlings of grapevine cultivar “Cabernet Sauvignon” were used to explore the effects of exogenous MT and ethylene on grape leaves under O3 stress. Cuttings were planted in cylindrical pots with a diameter of 25 cm and a height of 35 cm (substrate:sand:soil = 2:1:1). The potted seedlings were cultivated in a greenhouse. When the new shoot leaves grew to 10–12 pieces, the plants with the same growth potential were selected and treated with water, 50 μM MT (Xu et al., 2019), 250 mg L–1 ethephon (Ma et al., 2021), or 2 μM aminoethoxyvinylglycine (AVG) (Xu et al., 2019) every 2 days at 6 p.m. (three times in total), and each treatment (1.5 L) was replicated in five plants. After that, the plants were exposed to 110 nL L–1 O3 for 3 h at 800 μmol m–2 s–1 light intensity at 8 a.m. (Geng et al., 2016, 2017).
All treatments were as follows: The roots were irrigated with clean water and the leaves sprayed with clean water without O3 treatment (control); the roots were irrigated with clean water, the leaves sprayed with clean water, and then plants were exposed to O3 (O3); The roots were irrigated with 50 μM MT, the leaves sprayed with water, and then plants were exposed to O3 (MT + O3); the leaves were sprayed with 250 mg L–1 ethephon, the roots irrigated with clear water, and then plants were exposed to O3 (Ethephon + O3); The leaves were sprayed with 2 μM AVG, the roots irrigated with clean water, and then plants were exposed to O3 (AVG + O3); the roots were irrigated with 50 μM MT, the leaves sprayed with 2 μM AVG, and then plants were exposed to O3 (MT + AVG + O3). After the treatment, leaves with similar nodes and sizes were selected for RNA-Seq analysis and determination of physiological indexes.
“Cabernet Sauvignon” tissue culture seedlings were used to evaluate the effect of increasing endogenous ethylene content on grape leaves under O3 stress. Healthy apical growth tips of “Cabernet Sauvignon” vines were removed in early summer to establish grapevine in vitro shoot cultures. The plant materials were sterilized (75% alcohol for 2 min, 4% sodium hypochlorite for 15 min) and cultured on MS medium supplemented with 30 g L–1 sucrose, 7.5 g L–1 agar powder, and 0.2 mg L–1 indole-3-butyric acid (IBA). The plants were kept in a growth chamber maintained at 25/20°C, with a photoperiod of 16 h light/8 h dark, and branches with at least one bud and leaf were used for subculture every month. Healthy 2-month-old seedlings with consistent growth were selected for infection treatment. The tissue culture bottle caps were opened one week before treatment adapt the seedlings to the external environment gradually.
Chlorophyll Fluorescence Imaging and Determination of Related Enzyme Activities and Physiological Indexes
Rapid chlorophyll fluorescence imaging of grape leaves was performed using a fluorescence imaging system (PSI, Czechia). Hydrogen peroxide (H2O2) contents in leaves were estimated using the trichloroacetic acid (TCA) method at 390 nm (Velikova et al., 2000). Superoxide radical (O2–) was measured as described by Elstner and Heupel (1976) by monitoring the nitrite formation from hydroxylamine in the presence of⋅O2–. The tissue staining methods of H2O2 and O2– were according to Thordal-Christensen et al. (1997) and Orozco-Cardenas and Ryan (1999), respectively. Reduced ascorbic acid (AsA) content was measured by bipyridine colorimetry, while reduced glutathione (GSH) was determined using 5,5′-Dithio-bis (2-nitrobenzoic acid) (DTNB) (Zhou et al., 2005). The total glutathione content was determined by the method of previous descripted (Kosugi and Kikugawa, 1985). Oxidized glutathione (GSSG) content was calculated by the difference between total glutathione content and GSH content, and then GSH/GSSG value was obtained. Determination of superoxide dismutase (SOD) was by photochemical reduction of nitroblue tetrazolium (NBT) (Giannopolitis and Ries, 1977). The SOD activity unit U was 50% inhibition of NBT photochemical reduction. Peroxidase (POD) activity was determined by monitoring the increase in absorbance at 470 nm, caused by guaiacol oxidation (Scebba et al., 2001). One unit of POD activity was defined as the change of A470 by 0.01 per min. The activity of catalase (CAT) was determined according to the method of Cakmak and Marschner (1992). CAT can decompose H2O2, and H2O2 has a strong absorption peak at 240 nm wavelength, reducing A240 by 0.1 per minute to a unit (U) of CAT enzyme activity. The chlorophyll content was measured by UV (ultraviolet) spectrophotometry (Yang et al., 2009), while the activity of ascorbate peroxidase (APX) was measured as previously reported (Nakano and Asada, 1981). One unit of activity for APX was defined as the amount of enzyme that degraded 1 μmol of AsA per min. The activities of glutathione reductase (GR), monodehydroascorbate reductase (MDHAR), and dehydroascorbate reductase (DHAR) were measured with a kit (Keming Biotechnology Co., Ltd., Suzhou, China). One unit of activity for GR was defined as catalyzing the oxidation of 1 nmol NADPH (nicotinamide adenine dinucleotide phosphate) per gram of sample per min. One unit of MDHAR activity was defined as 1 nmol NADH (nicotinamide adenine dinucleotide) oxidized per min per gram of sample. One unit of DHAR activity was defined as 1 nmol AsA produced per min per gram of sample.
Determination of MT, Ethylene, and ACC Contents
The MT content was determined in reference to the previously reported method (Qianqian et al., 2015), with some modifications. The sample weight was 3.0 g, and the MT was extracted with analytical grade methanol; the final extract was purified by the C18 solid-phase extraction cartridge (ProElutTM, DIKMA, China) with the help of a vacuum pump, and then the volume was adjusted to 1 mL. The ethylene production rate was measured by gas chromatography (Shimadzu GC-16A, Japan) and repeated three times (Farmer et al., 1986). In order to avoid the wounding effect on ethylene production, the wound was wrapped in cotton with water after sampling and then sealed with a sealing film. The leaves were immediately put into a container and sealed, maintained in a light incubator at 25°C for 24 h, and then the gas was extracted into a 1 mL syringe for determination. The extraction and determination of ACC were according to Tucker et al. (2010). After O3 treatment, the samples were stored in liquid nitrogen.
RNA-Seq and Quantitative Real-Time PCR
Transcriptome sequencing was conducted by OE Biotech Co., Ltd. (Shanghai, China). Total RNAs were extracted using TRIzol Reagent (Invitrogen, Carlsbad, CA, United States), and the mRNA was enriched using magnetic beads containing Oligo (dT). The quality of the constructed gene library was checked by the Agilent 2100 Bioanalyzer (Agilent Technologies, Santa Clara, CA, United States). After passing the quality test, the HiSeq X Ten sequencer of Illumina Company was used for sequencing, and the double-terminal data of 150 bp was produced. Raw data (raw reads) were processed using Trimmomatic (Bolger et al., 2014). The reads containing ploy-N and the low quality reads were removed to obtain the clean reads. Then the clean reads were mapped to reference genome1 using hisat2 (Kim et al., 2015). The reads were reassembled using StringTie (Pertea et al., 2015). The protein-coding gene expression was calculated in FPKM (Fragments Per kb Per Million Reads). The default screening difference condition was P < 0.05 and log2 (fold change) >1. FDR (false discovery rate) error control method was used for P-value multiple hypothesis testing and correction. GO (Gene Ontology) enrichment and KEGG (Kyoto Encyclopedia of Genes and Genomes) pathway enrichment analysis of differentially expressed genes (DEGs) were respectively performed using R based on the hypergeometric distribution. The quantitative real-time PCR (qRT-PCR) analysis was carried out with the UltraSYBR Mixture kit (CWBIO, Beijing, China) on a Bio-Rad iQ5 (Hercules, CA, United States) instrument. The reaction mixture was 20 μL: double distilled water (ddH2O) 7.0 μL, forward primer (10 μmol L–1) 1.0 μL, reverse primer (10 μmol L–1) 1.0 μL, 2 × UltraSYBR Mixture 10.0 μL, cDNA 1.0 μL. The Vvactin gene was used as an internal reference. The relative quantitative gene expression values were calculated using the 2–Δ Δ CT method from three replicates. The primer sequences used are shown in Supplementary Table 1.
Genetic Transformation of ACO2 and ASMT1 in Grape and Tobacco
The open reading frames (ORFs) of ACO2 and ASMT1 from “Cabernet Sauvignon” leaves were cloned and then respectively ligated to the pRI101-AN expression vector driven by the 35S promoter. Then, the plasmid was transformed into Agrobacterium tumefaciens strain GV3101 by the heat shock method. The “Cabernet Sauvignon” tissue culture seedlings were immersed in an Agrobacterium suspension adjusted to OD600 = 0.6, placed in a closed container, and then the bacteria solution was completely immersed in grape leaves (with obvious water stains) by vacuum extraction. The bacterial suspension on the leaf surfaces was dried, and the seedlings were cultured in bottles with medium. After 2 days, a qRT-PCR analysis was done to detect the expression level, and the overexpression strain was used for the O3 treatment experiment. The plants infected with an empty carrier were used as a control, and each line was set with three replicates. The tobacco was infected by the leaf disc method (Wang F. et al., 2016), and the T0 tobacco plants overexpressing VvASMT1 were obtained after screening in selection medium. The transgenic lines were further identified by PCR and confirmed by qRT-PCR, after which T2 transgenic lines were obtained for experimental treatment.
Statistical Analysis
All statistical analyses were performed by SPSS 24.0 software. A one-way analysis of variance (ANOVA) followed by Duncan’s multiple range test was employed, standard deviation (SD) was calculated from three replicates. The differences between individual means were deemed to be significant at P < 0.05.
Results
Exogenous MT Inhibits the Ethylene Biosynthesis and Signaling Caused by O3 Stress
To explore the mechanism by which MT alleviates O3 stress in grape leaves, RNA-Seq analysis was performed on “Cabernet Sauvignon” grape leaves treated with control, O3, and MT + O3. DEGs were represented by a Venn diagram (Figure 1A). Compared with the control, O3 significantly (P-value < 0.05) up-regulated and down-regulated 5121 and 2935 genes in grape leaves, respectively (Supplementary Table 3). Compared with O3 treatment, MT + O3 up-regulated and down-regulated 2342 and 3310 genes, respectively (Supplementary Table 4). GO enrichment analysis showed that the DEGs were primarily associated with biological regulation, cellular process, metabolic process, signaling, cell, cell part, membrane, membrane part, binding, catalytic activity, nucleic acid binding transcription factor activity, and transporter activity (Figures 1B,C). KEGG enrichment analysis indicated that all of the annotated DEGs were primarily related to signal transduction, amino acid metabolism, biosynthesis of secondary metabolites, carbohydrate metabolism, and environmental adaptation (Figure 1D). In the classification of signal transduction pathways, the most apparent change in the number of DEGs occurred in the ethylene signal pathway (Supplementary Tables 5, 6). Compared with the control, O3 resulted in significant changes in the expression levels of 71 genes related to ethylene biosynthesis and signaling pathways. Except for the obvious down-regulation of three genes, all the others were up-regulated; the expression of ACO2 was up-regulated by 5.2-fold (Supplementary Table 5). Compared with O3 treatment, MT + O3 caused significant changes in the expression of 38 ethylene biosynthesis and signaling pathway-related genes. Among these 38 genes, 22 were significantly down-regulated, and ACO2 was down-regulated by 1.03-folds (Supplementary Table 6). When the expression levels of these 22 genes in O3 treatment and control were compared, it was found that 19 had higher expression levels than the O3 treatment (Supplementary Table 7). qRT-PCR analysis was done to further determine changes in the expression of ethylene-related genes under O3 and MT + O3 treatments. The results showed that the expression levels of 11 genes increased significantly with the extension of O3 treatment time, among which the expressions of ACO2 and ERF16 (ethylene-responsive transcription factor16) were up-regulated by 12.7 and 12.9-folds, respectively (Figure 1E). During stress, the relative expression levels of all detected genes under the MT + O3 treatment were significantly lower than that under the O3 treatment (Figure 1E), which was similar to the transcriptome analysis results.
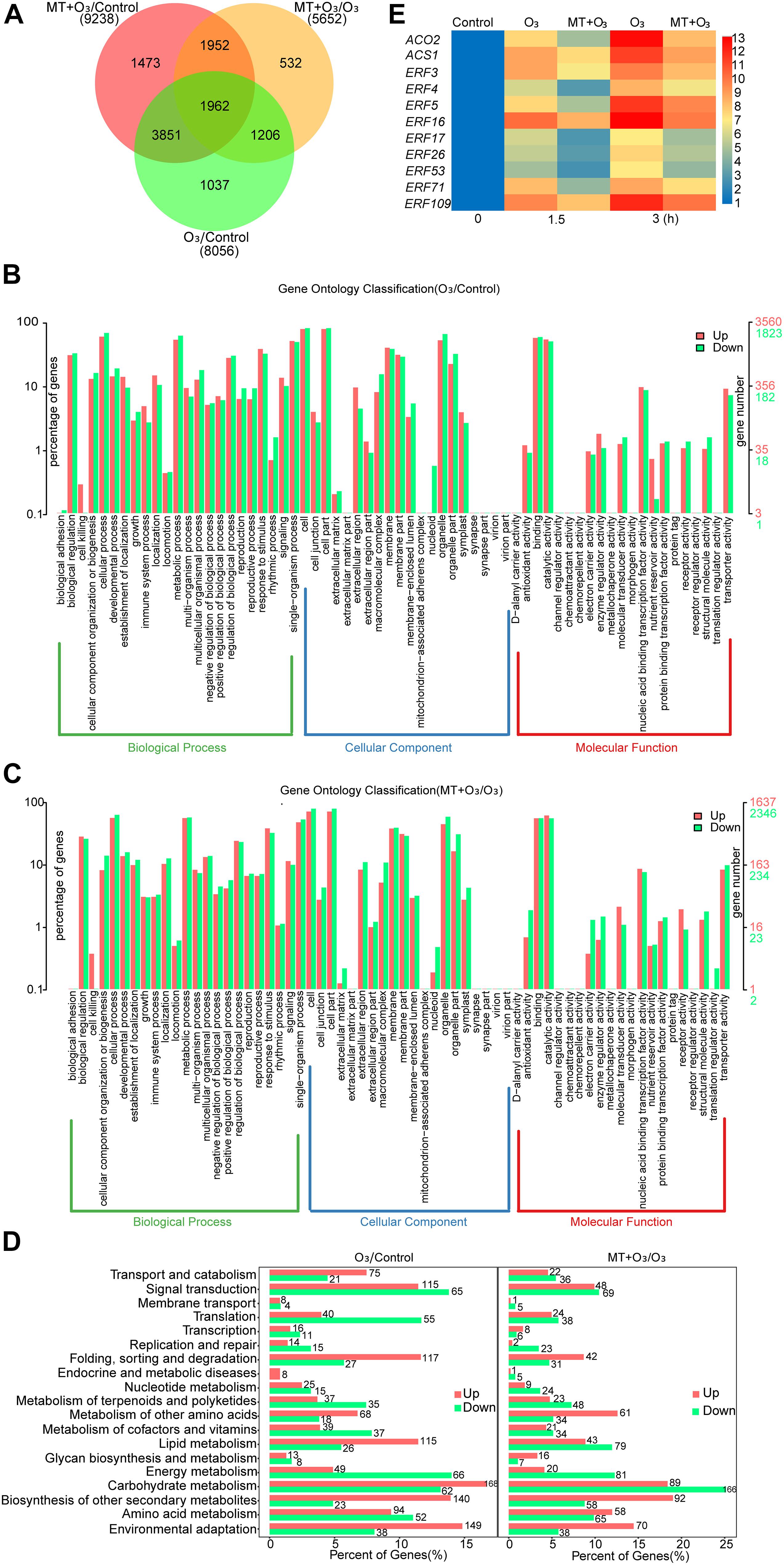
Figure 1. The number (A) and functional category (B–D) of DEGs (differentially expressed genes) and changes in the expression of genes related to ethylene biosynthesis and signal transduction (C) caused by O3 and MT + O3 treatment. (A) The number of common and unique DEGs between different comparison groups was counted to construct a Venn diagram. (B,C) GO (Gene Ontology) enrichment analysis of DEGs. (D) Pathway analyses of DEGs were carried out using the KEGG (Kyoto Encyclopedia of Genes and Genomes) database. (E) qRT-PCR was used to analyze the relative expression of genes related to ethylene biosynthesis and signal transduction after O3 and MT + O3 treatment for different times, and then a heat map was constructed. The normalized gene expression value was displayed as a color scale. MT, melatonin; ACS, 1-aminocyclopropane-1-carboxylate synthase; ACO, 1-aminocyclopropane-1-carboxylate oxidase; ERF, ethylene-responsive transcription factor.
MT Relieves O3 Stress by Regulating the Ethylene Pathway
To determine the effects of MT and ethylene on grape leaves under O3 stress, “Cabernet Sauvignon” grapes were treated differently. O3 stress caused more yellowing spots on grape leaves, and ethephon aggravated leaf damage symptoms under O3 stress, causing obvious chlorosis. In addition, AVG, MT, and a combination of the two treatments significantly reduced the leaf injury symptoms after O3 stress, and the yellowing area was smaller (Figure 2A). To further explore the mutual influence of MT and ethylene under O3 stress, the contents of MT and ethylene under different treatments were determined (Figures 2B–D). The results showed that compared with the control, O3 stress increased the ethylene release rate and the ACC content of “Cabernet Sauvignon” leaves by 100.8 and 82.19%, respectively. Meanwhile, the ethylene production after MT + O3 treatment was significantly less than in the O3 treatment (Figures 2B,C). Compared with the control, the MT content after O3 stress was significantly reduced by 59.25% (Figure 2D). After watering, the MT content in grape leaves increased by 97.64% relative to the control (Figure 2D). Compared with O3 treatment, the MT content after MT + O3 and MT + AVG + O3 treatment increased by 41.48 and 35.7%, respectively; however, there was no significant difference between ethephon + O3, AVG + O3, and the O3 treatment (Figure 2D).
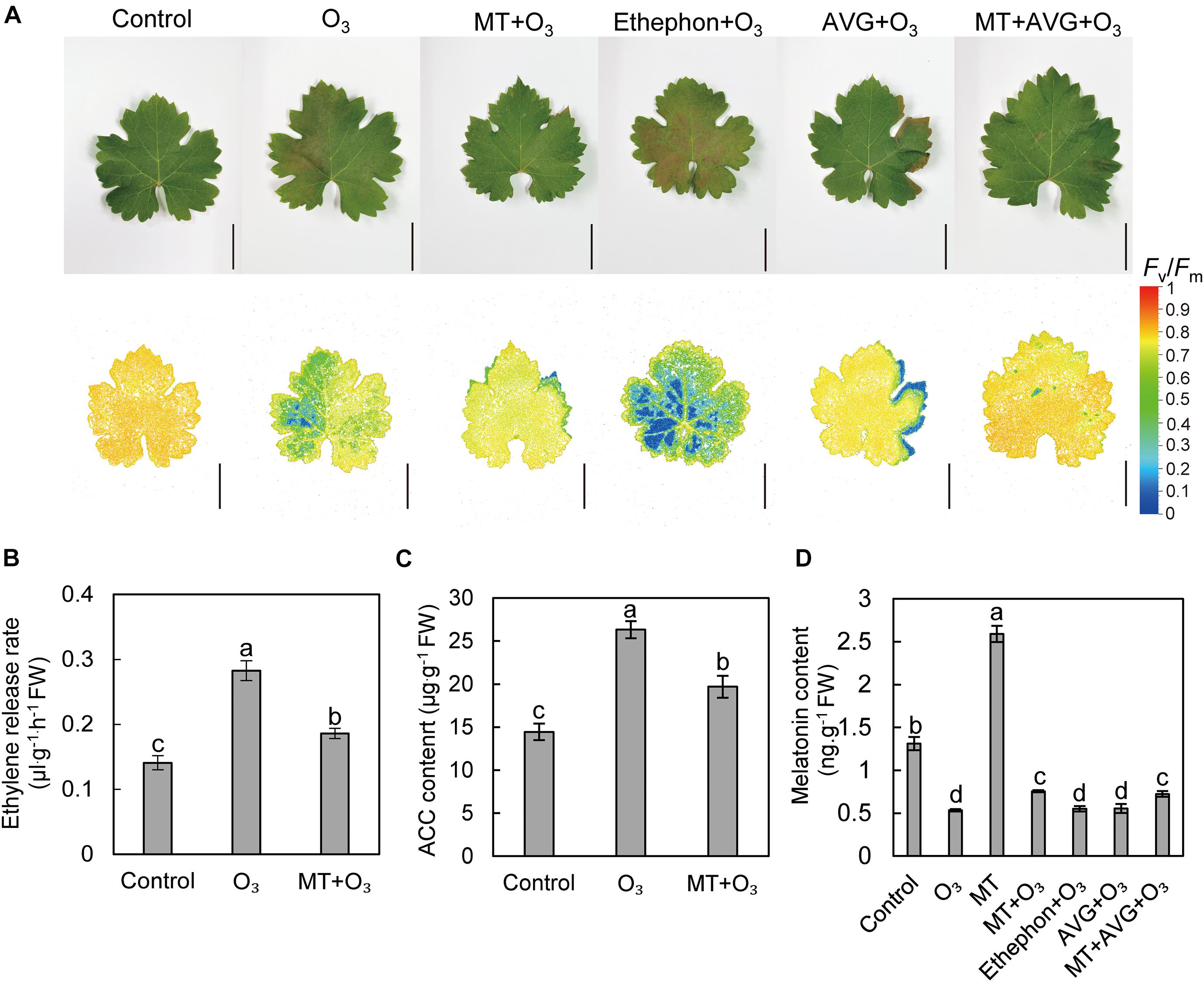
Figure 2. Effects of MT and ethylene on the apparent symptoms and chlorophyll fluorescence (A), ethylene release rate (B), ACC (C), and MT (D) contents in grape leaves after O3 stress. AVG, aminoethoxyvinylglycine; ACC, 1-aminocyclopropane-1-carboxylic acid. Values represent the mean of three replicates ± SD. The difference was not significant at a 5% significance level among values labeled with the same lowercase letter. Bars, 5 cm.
Effects of MT and Ethylene on Fv/Fm and Reactive Oxygen Species in Grape Leaves After O3 Stress
Compared with the control, the Fv/Fm of grape leaves after O3 stress decreased by 28.01% (Figure 3A). Compared with O3 treatment, the Fv/Fm of grape leaves treated with ethephon + O3 decreased by 23.34%, while the Fv/Fm increased by 28.35, 10.25, and 28.17% after MT + O3, AVG + O3, and MT + AVG + O3 treatment, respectively (Figure 3A). After O3 stress, the H2O2 content and O2– production rate increased significantly by 43.55 and 163.30%, respectively, relative to the control. Compared with the O3 treatment, the H2O2 content and O2– production rate of grape leaves increased by 23.37 and 22.30% after treatment with ethephon + O3, while MT + O3, AVG + O3, and AVG + MT + O3 decreased by 24.18 and 42.51, 15.31, and 25.78, 19.29, and 46.70%, respectively (Figures 3B,C).
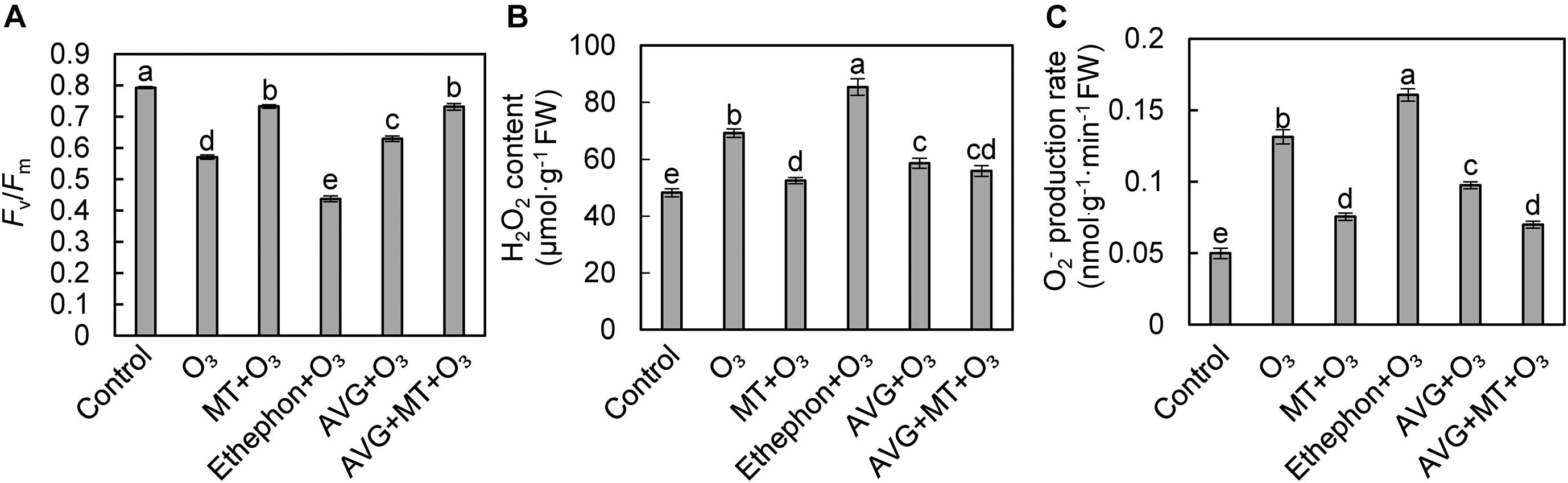
Figure 3. Effects of O3, MT + O3, Ethephon + O3, AVG + O3, and AVG + MT + O3 treatments on Fv/Fm (A) and ROS (B,C) of “Cabernet Sauvignon” grape leaves. Values represent the mean of three replicates ± SD. Different lowercase letters above the bars indicate significant difference (P < 0.05).
Effects of MT and Ethylene on Antioxidant System in Grape Leaves After O3 Stress
Ascorbic acid and GSH are antioxidants involved in scavenging of active oxygen free radicals under stress conditions. Compared with the control, O3 stress significantly reduced GSH, AsA contents, and GSH/GSSG in grape leaves, while increased GSSG content (Figures 4A–D). Compared with O3 treatment, the GSH, AsA content, and GSH/GSSG were increased after MT + O3, AVG + O3, and AVG + MT + O3 treatments. However, ethephon + O3 treatment reduced GSH, AsA content, and GSH/GSSG, but increased the content of GSSG (Figures 4A–D).
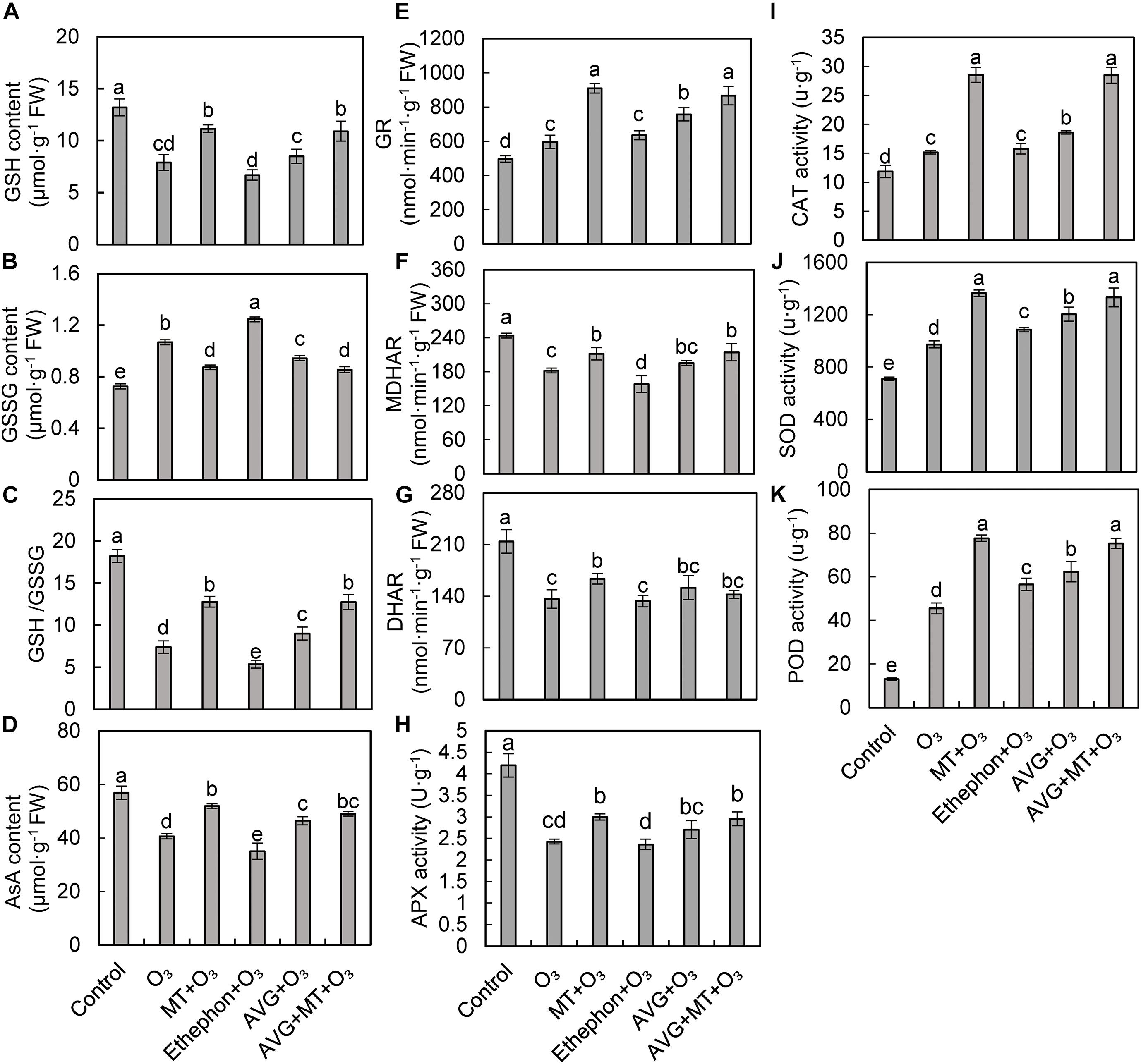
Figure 4. Effects of O3, MT + O3, Ethephon + O3, AVG + O3, and AVG + MT + O3 treatments on reduced glutathione (GSH, A) and oxidized glutathione (GSSG, B) contents, GSH/GSSG (C), reduced ascorbic acid (AsA, D) content, glutathione reductase (GR, E), monodehydroascorbate reductase (MDHAR, F), dehydroascorbate reductase (DHAR, G), ascorbate peroxidase (APX, H), catalase (CAT, I), superoxide dismutase (SOD, J), and peroxidase (POD, K) activities in “Cabernet Sauvignon” grape leaves. Values represent the mean of three replicates ± SD. For the values labeled with the same lowercase letter, the difference was not significant, according to Duncan’s multiple range test at a 5% significance level.
Compared with the control, the GR, CAT, SOD, and POD activities in grape leaves increased significantly after O3 stress. In contrast, the activities of MDHAR, DHAR, and APX were significantly inhibited (Figures 4E–K). Compared with the O3 treatment, MT + O3, AVG + O3, and AVG + MT + O3 treatments increased the GR, MDHAR, DHAR, APX, CAT, SOD, and POD activities of grape leaves, but the difference between AVG + MT + O3 and MT + O3 treatment was not significant (Figures 4E–K). Compared with the O3 treatment, the SOD and POD activities of grape leaves after treatment with ethephon + O3 were significantly increased, the activities of MDHAR and APX were inhibited. In contrast, the activities of GR, CAT, and DHAR did not change (Figures 4E–K). The above results indicate that both MT and AVG can alleviate the damage caused by O3 stress on grape leaves by regulating the antioxidant system.
Overexpression of VvACO2 Intensifies O3 Stress in Grape Leaves
To further verify that ethylene can exacerbate the stress effect of O3 on grape leaves, the VvACO2 gene was transiently overexpressed in grape leaves to promote ethylene biosynthesis. The expression levels of the two plants infected with 35S: VvACO2 carrier were 7.98 and 10.83-folds that of plants infected with the empty carrier, respectively (Figure 5A). These results confirm the successful expression of VvACO2 in grape leaves. The ethylene release rate and ACC content were also significantly higher in the leaves of plants overexpressing VvACO2 than those of the control group (Figures 5B,C). Under normal conditions, the growth of the control and overexpression plants was the same. After 110 nL L–1 O3 treatment for 3 h, the yellowing degree (Figure 5D), H2O2 content (Figure 5E), and O2– production rate (Figure 5F) in the leaves of overexpressed plants were significantly higher than those of the control group. Meanwhile, the chlorophyll content (Figure 5G) of overexpressed plant leaves was significantly lower than that of the control group. These results show that the endogenous induction of ethylene biosynthesis in grape leaves aggravated O3 damage to the leaves.
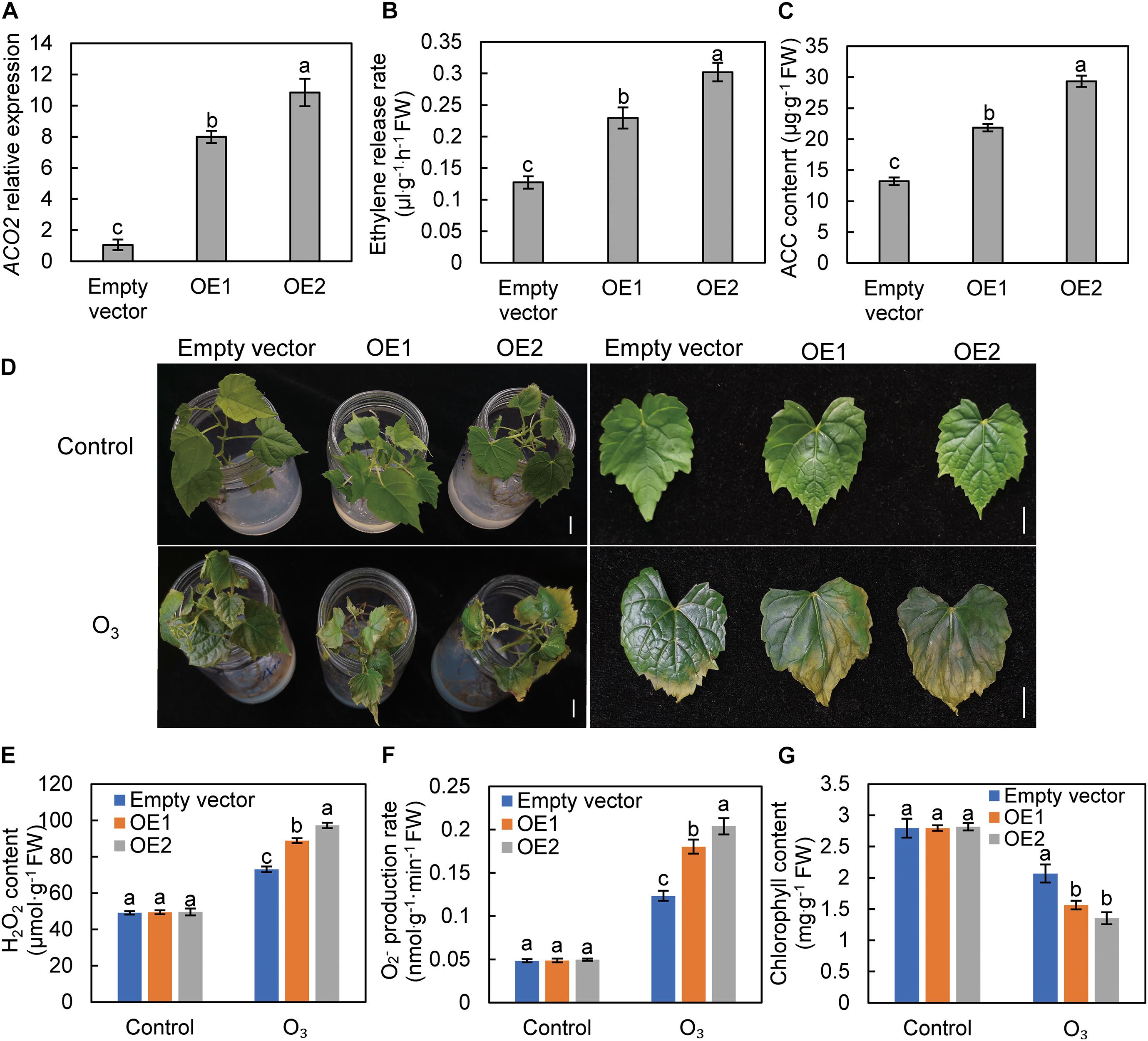
Figure 5. The effect of VvACO2 overexpression on the response of grape leaves to O3 stress. The ACO2 relative expression (A), ethylene release rate (B), and ACC content (C) in over-expressing (OE) and control (empty vector). The leaf appearance (D), ROS (E,F), and chlorophyll content (G) of the tissue culture seedlings of “Cabernet Sauvignon” grape after O3 stress. Letters marked by the same lowercase letters are not significant at P < 0.05 (Duncan’s multiple range test). Bars, 1 cm.
Overexpression of VvASMT1 Enhances Tobacco Tolerance to O3
To analyze the effect of endogenous MT on plant O3 tolerance, the overexpression vector 35S: VvASMT1 was transformed into tobacco to increase the endogenous MT content and test its O3 resistance. The upstream primer of the 35S promoter and the downstream primer for amplifying the ASMT gene were used for PCR amplification. The results showed no specific band in the control group, while specific bands appeared in the five tobacco lines. The band sizes were the sum of the 35S promoter fragment length and the ASMT ORF sequence length (Figure 6A). The results indicated that VvASMT1 was successfully transferred into tobacco. Two lines with moderate expression and high expression, line 1 and line 3, respectively, were selected for functional analysis (Figure 6B). The results showed that the MT content in the leaves of the wild-type and the two transgenic tobacco lines were 0.2299, 0.4957, and 0.5676 ng g–1, respectively (Figure 6C). After treatment with 110 nL L–1 O3 for 3 h, the tobacco showed yellowing and wilting symptoms, and the stress degree of transgenic tobacco was significantly lower than that of wild-type tobacco (Figure 6D). The leaf color in the transgenic lines after H2O2 and O2– staining was significantly lighter than that of the wild-type (Figures 6E,F), indicating that the ROS content was significantly lower than that of the wild-type. Compared with the wild-type, overexpression of VvASMT1 significantly increased GSH, AsA content, and related antioxidant enzymes (GR, SOD, POD, CAT, MDHAR, DHAR, and APX) activities after stress (Supplementary Figure 1). After O3 treatment, the ethylene release rate and ACC content of transgenic tobacco leaves were significantly lower than the wild-type (Figures 6G,H). The above results indicate that overexpression of the VvASMT1 gene in tobacco increased the MT content, alleviated the O3 stress, and reduced the ethylene content after stress.
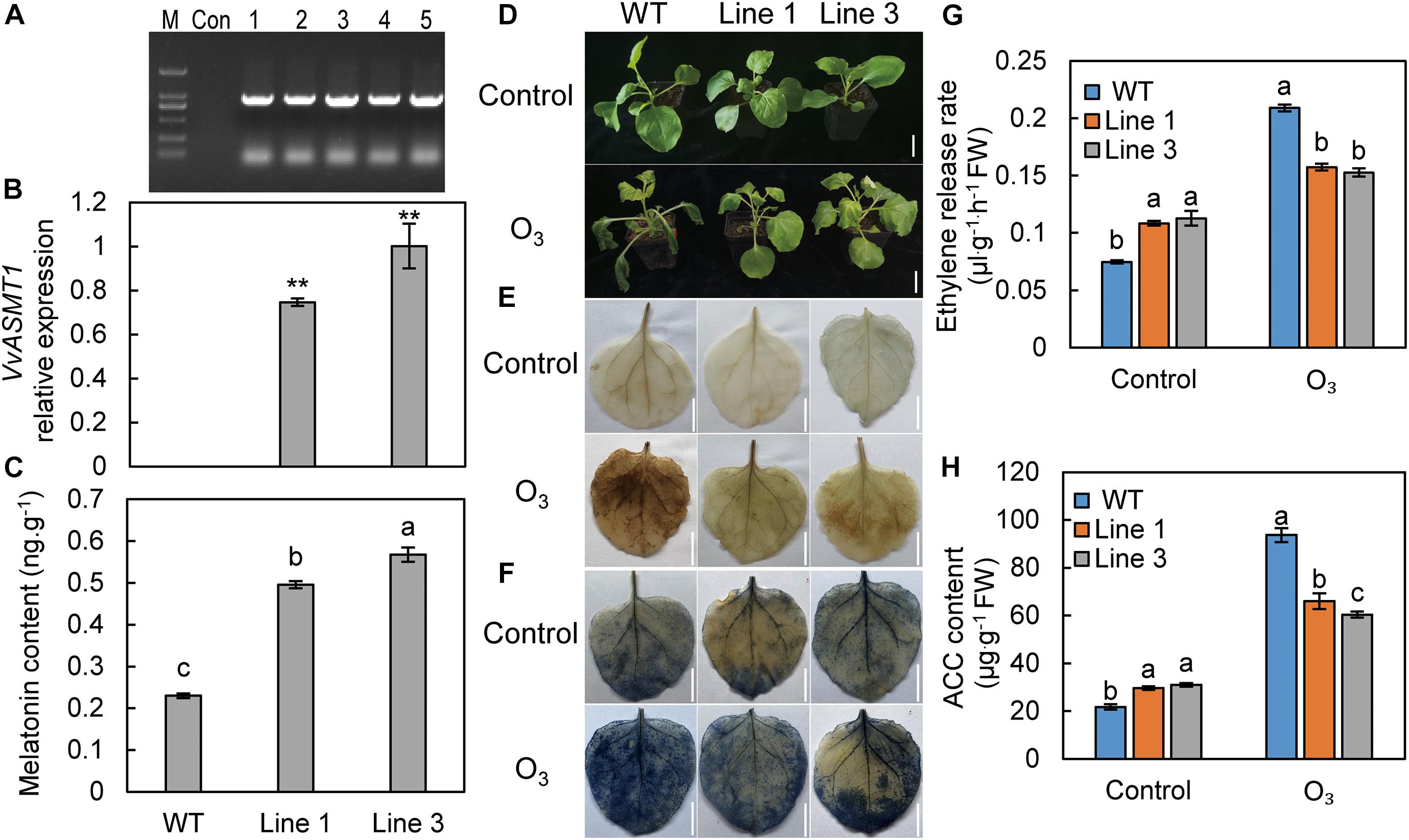
Figure 6. Tobacco plants overexpressing VvASMT1 showed increased tolerance to O3. (A) PCR detection of VvASMT1 overexpression in tobacco. (B) The expression level of VvASMT1 in transgenic and wild-type tobacco. (C) The content of MT in transgenic and wild-type tobacco. Phenotypes (D), H2O2 staining (E), O2– staining (F), ethylene release rate (G), and ACC content (H) of wild-type and transgenic lines after O3 treatment. The numbers 1–5 represent five different tobacco lines; M, DNA marker; Con, control; ASMT, acetylserotonin methyltransferase; WT, wild-type. Values represent the means ± SD of three replicates. **Highly significant difference, P < 0.01. Values indicated by the same lowercase letters are not significant at P < 0.05. Bars, 3 cm in (D), 2 cm in (E,F).
Discussion
At present, only a few studies have examined the molecular mechanism of O3 stress on fruit trees, especially grapes (Valletta et al., 2016). O3 enters plant leaves mainly through the stomata and increases ROS production (Langebartels et al., 2002). Thus, ROS level can be an essential indicator to determine the degree of cellular oxidative stress (Collén et al., 2003). When large amounts of ROS are produced under stress, its clearance system gets damaged, resulting in membrane lipid peroxidation, massive chlorophyll degradation, hindrance of photosynthetic electron transfer, inactivation of antioxidant enzymes, and inhibiting plant growth development (Degl’Innocenti et al., 2007; Iriti and Faoro, 2008; Bose et al., 2014). PSII is the most sensitive component of the photosynthetic electron transport chain under O3 stress (Tran et al., 2013). Similarly, our research showed that O3 stress could damage grape PSII and significantly reduce the chlorophyll content in grape leaves. Stressed leaves also produced large amounts of ROS and changed the activity of antioxidant enzymes, resulting in yellowing and wilting of grape leaves. Further, O3 stress significantly induced the upregulation of genes related to ethylene biosynthesis and some ethylene responsive transcription factors, as well as the increase of ethylene release rate and ACC content. In our study, increase in exogenous and/or endogenous ethylene content aggravated O3 stress. Therefore, it was speculated that O3 destroys the photosynthetic and antioxidant grapes systems by increasing the ethylene content.
Many studies have shown that MT can alleviate various abiotic stresses; plants with higher MT content are more tolerant to O3 (Dubbels et al., 1995). The present study also proved that MT could relieve O3 stress in grape leaves and improve various physiological indexes. Exogenous MT application significantly increased the Fv/Fm of “Cabernet Sauvignon” grape leaves under O3 stress and alleviated leaf chlorosis. Similarly, MT can reduce the damage of barley leaf photosystem II (PSII) under stress and maintain the chlorophyll content (Arnao and Hernández-Ruiz, 2009). These results may be related to the protection of chloroplast structure by MT (Zhao et al., 2016). Additionally, this experiment found that watering the roots of “Cabernet Sauvignon” with MT could increase the leaf MT content. Similarly, treating grapes with MT at the rhizosphere increased the MT levels of the roots, but also in the leaves, thus enhancing salt tolerance in “Crimson seedless” grapevines (Xu et al., 2019). This result suggests that the MT provided through external sources is absorbed in plants (Tan et al., 2007) and can accumulate in distant organs through long-distance transportation to tolerate abiotic stress. In addition, our experiment for the first time verified that overexpression of VvASMT in tobacco could alleviate O3 stress by increasing the MT content. This result is similar to that obtained following overexpression of the key enzyme gene caffeic acid-O-methyltransferase (COMT) for MT synthesis, which increased the endogenous MT content of tomatoes and enhanced salt resistance (Sun et al., 2019).
Plants can resist ROS damage through a defense system composed of enzymatic and non-enzymatic ROS scavenging systems (Apel and Hirt, 2004). Antioxidant enzyme scavenging systems mainly include SOD, POD, CAT, etc., while non-enzymatic systems include the AsA-GSH cycle. GSH can catalyze the degradation of excess H2O2 and activate various defense mechanisms by participating in redox signal transduction (Szalai et al., 2009). The increase in GR activity reduces the cellular glutathione pool, providing sufficient GSH for DHAR to reduce dehydroascorbate (DHA) to AsA (Noctor and Foyer, 1998). MT can enhance the scavenging ability of the ROS system to improve stress tolerance. For example, the application of 0.5 μmol L–1 MT can improve salt tolerance in tomatoes by increasing the antioxidant enzyme activity and the accumulation of AsA and GSH (Liu et al., 2015). Moreover, the leaves of O3-tolerant soybean varieties maintain higher AsA levels than susceptible varieties (Chutteang et al., 2015). Thus, varieties with high antioxidant contents in the leaves are more resistant to the O3 damage. This experiment also found that MT could protect antioxidant enzymes and the AsA-GSH signaling system under O3 stress, while ethylene had the opposite effect. MT may act as an antioxidant to antagonize ethylene and remove excessive ROS, thereby sharing the pressure of other antioxidants.
Melatonin has opposite regulatory effects on ethylene in different crop species. For example, MT can promote the ripening of grape berries by increasing the ethylene content (Xu et al., 2018) and enhancing the salt tolerance of grapes by promoting VviMYB108A-mediated ethylene biosynthesis (Xu et al., 2019). On the contrary, MT treatment reduces ethylene release and improves fruit quality by inhibiting the expression of genes related to ethylene biosynthesis during apple storage (Onik et al., 2020). In our experiment, MT treatment of O3 stressed plants down-regulated the expression of ethylene biosynthesis and signal transduction genes in grape leaves. MT treatment also reduced the ethylene release rate and ACC content in O3 stressed leaves, thus alleviating O3 stress. The increase of endogenous MT in tobacco also reduced ethylene biosynthesis after stress and alleviated O3 stress. However, MT treatment of ‘Crimson seedless’ grapevines roots increased the ethylene release rate of leaves (Xu et al., 2019). These contrasting effects of MT may be due to the multi-pathway characteristics of MT synthesis, making it functionally specific in the developmental stage, tissues, and organs. In addition, the physiological effects of MT are pleiotropic (Byeon and Back, 2014; Zhang et al., 2014), and its regulation of ethylene could be indirect. The different inducing effects could also change the mutual regulation with other hormones; thus, the specific mechanism needs to be further explored. Although both MT and ethylene inhibitor significantly alleviated O3 stress in grapes, the effect of MT treatment was better than treatment with ethylene inhibitor treatment. In addition, the combined treatment effect of MT and ethylene inhibitor was similar to that of MT alone, and the treatment of ethylene and ethylene inhibitor did not affect the MT content under O3 stress. It can be seen that MT and ethylene may play upstream and downstream roles, respectively, in the signal pathway under O3 stress.
Finally, as depicted in Figure 7, O3 stress increased ROS content and decreased the photosynthetic and antioxidant capacities of grape leaves by inducing ethylene biosynthesis. Melatonin pretreatment or overexpression of ASMT1 can enhance the in vivo melatonin level, reduce ethylene production in grape leaves under O3 stress and increase plant O3 tolerance. In addition, overexpression of ACO2 in grape leaves decreased O3 tolerance by increasing endogenous ethylene content. Taken together, MT can alleviate O3 damage to grape leaves by inhibiting ethylene biosynthesis.
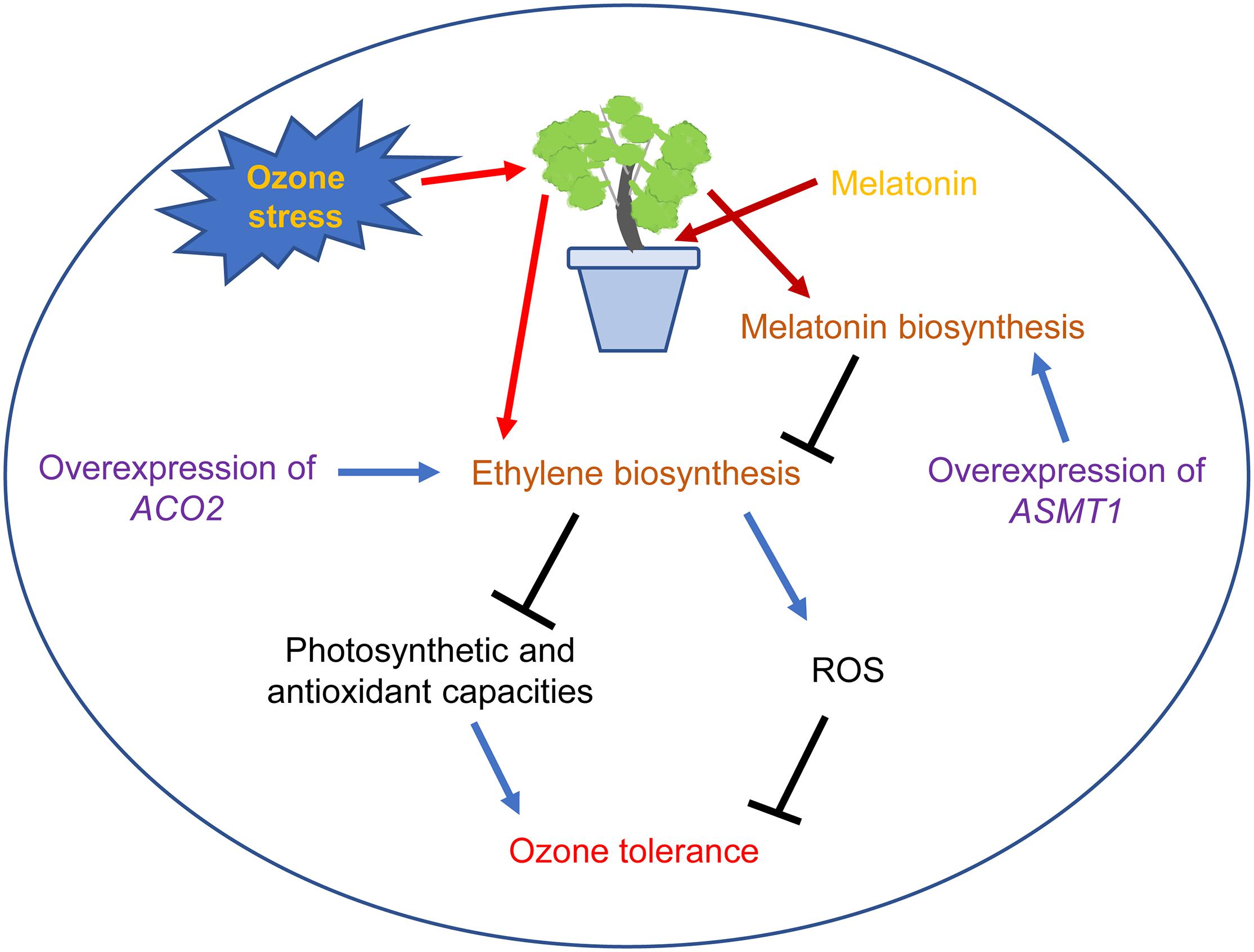
Figure 7. A schematic model showing the mechanisms of MT alleviating O3 stress in grape leaves. The arrow denotes increases and bar denotes decreases.
Data Availability Statement
The original contributions presented in the study are publicly available. This data can be found here: NCBI repository, accession number: PRJNA733572.
Author Contributions
CLCL performed most parts of the experiment, analyzed the data, and wrote the manuscript. HK and YW participated in performing the experiments. ZG participated in the manuscript writing and revison. YD and YY designed the research. All authors have read and approved the final manuscript.
Funding
This work was supported by the National Key Research and Development Program of China (2019YFD1000101), National Natural Sciences Foundation of China in 2016 (31572084), and China Agriculture Research System of MOF and MARA.
Conflict of Interest
The authors declare that the research was conducted in the absence of any commercial or financial relationships that could be construed as a potential conflict of interest.
Publisher’s Note
All claims expressed in this article are solely those of the authors and do not necessarily represent those of their affiliated organizations, or those of the publisher, the editors and the reviewers. Any product that may be evaluated in this article, or claim that may be made by its manufacturer, is not guaranteed or endorsed by the publisher.
Supplementary Material
The Supplementary Material for this article can be found online at: https://www.frontiersin.org/articles/10.3389/fpls.2021.702874/full#supplementary-material
Supplementary Figure 1 | Effects of ozone stress on GSH (A), AsA (B), and related antioxidant enzyme activities (C–I) of wild-type and transgenic tobacco.
Supplementary Table 1 | Primers used in this study.
Supplementary Table 2 | RNA-Seq profiles of grape leaves after different treatments, including control, O3, and MT + O3. The default condition for screening differences is P < 0.05 and fold change >2.
Footnotes
References
Al-Huqail, A. A., Khan, M. N., Ali, H. M., Siddiqui, M. H., and Al-Humaid, L. A. (2020). Exogenous melatonin mitigates boron toxicity in wheat. Ecotoxicol. Environ. Saf. 201:110822. doi: 10.1016/j.ecoenv.2020.110822
Apel, K., and Hirt, H. (2004). Reactive oxygen species: metabolism, oxidative stress, and signal transduction. Annu. Rev. Plant Biol. 55, 373–399. doi: 10.1146/annurev.arplant.55.031903.141701
Arnao, M. B., and Hernández-Ruiz, J. (2018). Melatonin and its relationship to plant hormones. Ann. Bot. 121, 195–207. doi: 10.1093/aob/mcx114
Arnao, M. B., and Hernández-Ruiz, J. (2009). Protective effect of melatonin against chlorophyll degradation during the senescence of barley leaves. J. Pineal Res. 46, 58–63. doi: 10.1111/j.1600-079X.2008.00625.x
Arnao, M. B., and Hernández-Ruiz, J. (2015). Functions of melatonin in plants: a review. J. Pineal Res. 59, 133–150. doi: 10.1111/jpi.12253
Bolger, A. M., Lohse, M., and Usadel, B. (2014). Trimmomatic: a flexible trimmer for Illumina sequence data. Bioinformatics 30, 2114–2120. doi: 10.1093/bioinformatics/btu170
Borowiak, K. (2013). Morphological changes in two tobacco and petunia cultivars under exposure to tropospheric ozone. Acta Biol. Cracov. Ser. Bot. 55, 58–66. doi: 10.2478/abcsb-2013-0002
Bose, J., Rodrigo-Moreno, A., and Shabala, S. (2014). ROS homeostasis in halophytes in the context of salinity stress tolerance. J. Exp. Bot. 65, 1241–1257. doi: 10.1093/jxb/ert430
Byeon, Y., and Back, K. (2014). Melatonin synthesis in rice seedlings in vivo is enhanced at high temperatures and under dark conditions due to increased serotonin N−acetyltransferase and N−acetylserotonin methyltransferase activities. J. Pineal Res. 56, 189–195. doi: 10.1111/jpi.12111
Cakmak, I., and Marschner, H. (1992). Magnesium deficiency and high light intensity enhance activities of superoxide dismutase, ascorbate peroxidase, and glutathione reductase in bean leaves. Plant Physiol. 98, 1222–1227. doi: 10.1104/pp.98.4.1222
Chen, Z., Gao, Z., Sun, Y., Wang, Y., Yao, Y., Zhai, H., et al. (2020). Analyzing the grape leaf proteome and photosynthetic process provides insights into the injury mechanisms of ozone stress. Plant Growth Regul. 91, 143–155. doi: 10.1007/s10725-020-00593-5
Chutteang, C., Booker, F. L., Na-Ngern, P., Burton, A., Aoki, M., and Burkey, K. O. (2015). Biochemical and physiological processes associated with the differential ozone response in ozone-tolerant and sensitive soybean genotypes. Plant Biol. 2015:12347. doi: 10.1111/plb.12347
Collén, J., Pinto, E., Pedersén, M., and Colepicolo, P. (2003). Induction of oxidative stress in the red macroalga Gracilaria tenuistipitata by pollutant metals. Arch. Environ. Contam. Toxicol. 45, 337–342. doi: 10.1007/s00244-003-0196-0
Degl’Innocenti, E., Guidi, L., and Soldatini, G. (2007). Effects of elevated ozone on chlorophyll a fluorescence in symptomatic and asymptomatic leaves of two tomato genotypes. Biol. Plant. 51, 313–321. doi: 10.1007/s10535-007-0061-5
Dubbels, R., Reiter, R., Klenke, E., Goebel, A., Schnakenberg, E., Ehlers, C., et al. (1995). Melatonin in edible plants identified by radioimmunoassay and by high performance liquid chromatography−mass spectrometry. J. Pineal Res. 18, 28–31. doi: 10.1111/j.1600-079X.1995.tb00136.x
Elstner, E. F., and Heupel, A. (1976). Inhibition of nitrite formation from hydroxylammonium chloride: a simple assay for superoxide dismutase. Anal. Biochem. 70, 616–620. doi: 10.1016/0003-2697(76)90488-7
Farmer, P., Bailey, E., Gorf, S., Törnqvist, M., Osterman-Golkar, S., Kautiainen, A., et al. (1986). Monitoring human exposure to ethylene oxide by the determination of haemoglobin adducts using gas chromatography—mass spectrometry. Carcinogenesis 7, 637–640. doi: 10.1093/carcin/7.4.637
Fiscus, E. L., Booker, F. L., and Burkey, K. O. (2005). Crop responses to ozone: uptake, modes of action, carbon assimilation and partitioning. Plant Cell Environ. 28, 997–1011. doi: 10.1111/j.1365-3040.2005.01349.x
Galano, A., Tan, D. X., and Reiter, R. J. (2011). Melatonin as a natural ally against oxidative stress: a physicochemical examination. J. Pineal Res. 51, 1–16. doi: 10.1111/j.1600-079X.2011.00916.x
Gao, Y., Wang, Y., Qian, J., Si, W., Tan, Q., Xu, J., et al. (2020). Melatonin enhances the cadmium tolerance of mushrooms through antioxidant-related metabolites and enzymes. Food Chem. 2020:127263. doi: 10.1016/j.foodchem.2020.127263
Geng, Q. W., Xing, H., Sun, Y. J., Hao, G. M., Zhai, H., and Du, Y. P. (2017). Analysis of the interaction effects of light and O3 on fluorescence properties of ‘Cabernet Sauvignon’grapes based on response surface methodology. Sci. Hortic. 225, 599–606. doi: 10.1016/j.scienta.2017.07.030
Geng, Q. W., Xing, H., Hao, G. M., Sun, Y. J., Zhai, H., and Du, Y. P. (2016). Effect of exogenous melatonin on photosynthesis of ‘cabernet sauvigon’ grape leaves under ozone stress. Acta Hortic. Sin. 43, 1463–1472. doi: 10.16420/j.issn.0513-353x.2016-0123
Giannopolitis, C. N., and Ries, S. K. (1977). Superoxide dismutases: I. Occurrence in higher plants. Plant Physiol. 59, 309–314. doi: 10.1104/pp.59.2.309
Iriti, M., and Faoro, F. (2008). Oxidative stress, the paradigm of ozone toxicitye in plants and animals. Water Air Soil Pollut. 187, 285–301. doi: 10.1007/s11270-007-9517-7
Kang, K., Kong, K., Park, S., Natsagdorj, U., Kim, Y. S., and Back, K. (2011). Molecular cloning of a plant N−acetylserotonin methyltransferase and its expression characteristics in rice. J. Pineal Res. 50, 304–309. doi: 10.1111/j.1600-079X.2010.00841.x
Karnosky, D. F., Skelly, J. M., Percy, K. E., and Chappelka, A. H. (2007). Perspectives regarding 50 years of research on effects of tropospheric ozone air pollution on US forests. Environ. Pollut. 147, 489–506. doi: 10.1016/j.envpol.2006.08.043
Kim, D., Langmead, B., and Salzberg, S. L. (2015). HISAT: a fast spliced aligner with low memoryrequirements. Nat. Methods 12, 357–360. doi: 10.1038/nmeth.3317
Kosugi, H., and Kikugawa, K. (1985). Thiobarbituric acid reaction of aldehydes and oxidized lipids in glacial acetic acid. Lipids 20, 915–921. doi: 10.1007/BF02534777
Langebartels, C., Wohlgemuth, H., Kschieschan, S., Grün, S., and Sandermann, H. (2002). Oxidative burst and cell death in ozone-exposed plants. Plant Physiol. Biochem. 40, 567–575. doi: 10.1016/S0981-9428(02)01416-X
Lee, H. Y., Byeon, Y., and Back, K. (2014). Melatonin as a signal molecule triggering defense responses against pathogen attack in Arabidopsis and tobacco. J. Pineal Res. 57, 262–268. doi: 10.1111/jpi.12165
Li, C., Liang, B., Chang, C., Wei, Z., Zhou, S., and Ma, F. (2016). Exogenous melatonin improved potassium content in Malus under different stress conditions. J. Pineal Res. 61, 218–229. doi: 10.1111/jpi.12342
Li, X., Ahammed, G. J., Zhang, X. N., Zhang, L., Yan, P., Zhang, L. P., et al. (2021). Melatonin-mediated regulation of anthocyanin biosynthesis and antioxidant defense confer tolerance to arsenic stress in Camellia sinensis L. J. Hazard. Mater. 403:123922. doi: 10.1016/j.jhazmat.2020.123922
Liu, N., Gong, B., Jin, Z., Wang, X., Wei, M., Yang, F., et al. (2015). Sodic alkaline stress mitigation by exogenous melatonin in tomato needs nitric oxide as a downstream signal. J. Plant Physiol. 2015, 68–77. doi: 10.1016/j.jplph.2015.07.012
Ma, W., Xu, L., Gao, S., Lyu, X., Cao, X., and Yao, Y. (2021). Melatonin alters the secondary metabolite profile of grape berry skin by promoting VvMYB14-mediated ethylene biosynthesis. Hortic. Res. 8, 1–15. doi: 10.1038/s41438-021-00478-2
Mehlhorn, H., and Wellburn, A. R. (1987). Stress ethylene formation determines plant sensitivity to ozone. Nature 327, 417–418. doi: 10.1038/327417a0
Najeeb, U., Tan, D. K., Bange, M. P., and Atwell, B. J. (2018). Protecting cotton crops under elevated CO2 from waterlogging by managing ethylene. Funct. Plant Biol. 45, 340–349. doi: 10.1071/FP17184
Nakano, Y., and Asada, K. (1981). Hydrogen peroxide is scavenged by ascorbate-specific peroxidase in spinach chloroplasts. Plant Cell Physiol. 22, 867–880. doi: 10.1093/oxfordjournals.pcp.a076232
Nguyen, D., Rieu, I., Mariani, C., and van Dam, N. M. (2016). How plants handle multiple stresses: hormonal interactions underlying responses to abiotic stress and insect herbivory. Plant Mol. Biol. 91, 727–740. doi: 10.1007/s11103-016-0481-8
Noctor, G., and Foyer, C. H. (1998). Ascorbate and glutathione: Keeping active oxygen under control. Annu. Rev. Plant Physiol. Plant Mol. Biol. 49, 249–279. doi: 10.1146/annurev.arplant.49.1.249
Onik, J. C., Wai, S. C., Li, A., Lin, Q., Sun, Q., Wang, Z., et al. (2020). Melatonin treatment reduces ethylene production and maintains fruit quality in apple during postharvest storage. Food Chem. 337:127753. doi: 10.1016/j.foodchem.2020.127753
Orozco-Cardenas, M., and Ryan, C. A. (1999). Hydrogen peroxide is generated systemically in plant leaves by wounding and systemin via the octadecanoid pathway. Proc. Natl. Acad. Sci. 96, 6553–6557. doi: 10.1073/pnas.96.11.6553
Pertea, M., Pertea, G. M., Antonescu, C. M., Chang, T. C., Mendell, J. T., and Salzberg, S. L. (2015). StringTie enables improved reconstruction of a transcriptome from RNA-seq reads. Nat. Biotechnol. 33, 290–295. doi: 10.1038/nbt.3122
Qianqian, S., Na, Z., Jinfang, W., Haijun, Z., Dianbo, L., Jin, S., et al. (2015). Melatonin promotes ripening and improves quality of tomato fruit during postharvest life. J. Exp. Bot. 66, 657–668. doi: 10.1093/jxb/eru332
Ryu, H., and Cho, Y. G. (2015). Plant hormones in salt stress tolerance. J. Plant Biol. 58, 147–155. doi: 10.1007/s12374-015-0103-z
Scebba, F., Sebastiani, L., and Vitagliano, C. (2001). Activities of antioxidant enzymes during senescence of Prunus armeniaca leaves. Biol. Plant. 44, 41–46. doi: 10.1023/A:1017962102950
Serengil, Y., Augustaitis, A., Bytnerowicz, A., Grulke, N., Kozovitz, A., Matyssek, R., et al. (2011). Adaptation of forest ecosystems to air pollution and climate change: a global assessment on research priorities. iForest 4, 44–48. doi: 10.3832/ifor0566-004
Sun, S., Wen, D., Yang, W., Meng, Q., Shi, Q., and Gong, B. (2019). Overexpression of caffeic acid o-methyltransferase 1 (COMT1) increases melatonin level and salt stress tolerance in tomato plant. J. Plant Growth Regul. 2019, 1–15. doi: 10.1007/s00344-019-10058-3
Szalai, G., Kells, T., Galiba, G., and Kocsy, G. (2009). Glutathione as an antioxidant and regulatory molecule in plants under abiotic stress conditions. J. Plant Growth Regul. 28, 66–80. doi: 10.1007/s00344-008-9075-2
Tan, D. X., Manchester, L. C., Di Mascio, P., Martinez, G. R., Prado, F. M., and Reiter, R. J. (2007). Novel rhythms of N1−acetyl−N2−formyl−5−methoxykynuramine and its precursor melatonin in water hyacinth: importance for phytoremediation. Faseb J. 21, 1724–1729. doi: 10.1096/fj.06-7745com
Thordal-Christensen, H., Zhang, Z., Wei, Y., and Collinge, D. B. (1997). Subcellular localization of H2O2 in plants. H2O2 accumulation in papillae and hypersensitive response during the barley—powdery mildew interaction. Plant J. 11, 1187–1194. doi: 10.1046/j.1365-313X.1997.11061187.x
Tingey, D. T., Standley, C., and Field, R. W. (1976). Stress ethylene evolution: A measure of ozone effects on plants. Atmos. Environ. 10, 969–974. doi: 10.1016/0004-6981(76)90204-3
Tran, T. A., Vassileva, V., Petrov, P., and Popova, L. P. (2013). Cadmium-induced structural disturbances in Pisum sativum leaves are alleviated by nitric oxide. Turk. J. Bot. 37, 698–707. doi: 10.3906/bot-1209-8
Tucker, M. L., Xue, P., and Yang, R. (2010). 1-Aminocyclopropane-1-carboxylic acid (ACC) concentration and ACC synthase expression in soybean roots, root tips, and soybean cyst nematode (Heterodera glycines)-infected roots. J. Exp. Bot. 61, 463–472. doi: 10.1093/jxb/erp317
Valletta, A., Salvatori, E., Rita Santamaria, A., Nicoletti, M., Toniolo, C., Caboni, E., et al. (2016). Ecophysiological and phytochemical response to ozone of wine grape cultivars of Vitis vinifera L. Nat. Prod. Res. 30, 2514–2522. doi: 10.1080/14786419.2015.1118631
Velikova, V., Yordanov, I., and Edreva, A. (2000). Oxidative stress and some antioxidant systems in acid rain-treated bean plants: protective role of exogenous polyamines. Plant Sci. 151, 59–66. doi: 10.1016/S0168-9452(99)00197-1
Vingarzan, R. (2004). A review of surface ozone background levels and trends. Atmos. Environ. 38, 3431–3442. doi: 10.1016/j.atmosenv.2004.03.030
Wang, F., Wang, C., Yan, Y., Jia, H., and Guo, X. (2016). Overexpression of cotton GhMPK11 decreases disease resistance through the gibberellin signaling pathway in transgenic Nicotiana benthamiana. Front. Plant Sci. 7:689. doi: 10.3389/fpls.2016.00689
Wang, Q., An, B., Wei, Y., Reiter, R. J., Shi, H., Luo, H., et al. (2016). Melatonin regulates root meristem by repressing auxin synthesis and polar auxin transport in Arabidopsis. Front. Plant Sci. 7:1882. doi: 10.3389/fpls.2016.01882
Xu, L., Xiang, G., Sun, Q., Ni, Y., Jin, Z., Gao, S., et al. (2019). Melatonin enhances salt tolerance by promoting MYB108A-mediated ethylene biosynthesis in grapevines. Hortic. Res. 6, 1–14. doi: 10.1038/s41438-019-0197-4
Xu, L., Yue, Q., Xiang, G., Bian, F., and Yao, Y. (2018). Melatonin promotes ripening of grape berry via increasing the levels of ABA, H2O2, and particularly ethylene. Hortic. Res. 5:41. doi: 10.1038/s41438-018-0045-y
Yang, Q., Chen, Z. Z., Zhou, X. F., Yin, H. B., Li, X., Xin, X. F., et al. (2009). Overexpression of SOS (Salt Overly Sensitive) genes increases salt tolerance in transgenic Arabidopsis. Mol. Plant 2, 22–31. doi: 10.1093/mp/ssn058
Yang, Y., Yan, L., Yana, S., Tao, L., Yanchun, C., Dake, Z., et al. (2018). The role of phyto-melatonin and related metabolites in response to stress. Molecules 23:1887. doi: 10.3390/molecules23081887
Zhang, H. J., Zhang, N., Yang, R. C., Wang, L., Sun, Q. Q., Li, D. B., et al. (2014). Melatonin promotes seed germination under high salinity by regulating antioxidant systems, ABA and GA4 interaction in cucumber (Cucumis sativus L.). J. Pineal Res. 57, 269–279. doi: 10.1111/jpi.12167
Zhang, M., Smith, J. A. C., Harberd, N. P., and Jiang, C. (2016). The regulatory roles of ethylene and reactive oxygen species (ROS) in plant salt stress responses. Plant Mol. Biol. 91, 651–659. doi: 10.1007/s11103-016-0488-1
Zhang, N., Sun, Q., Li, H., Li, X., Cao, Y., Zhang, H., et al. (2016). Melatonin improved anthocyanin accumulation by regulating gene expressions and resulted in high reactive oxygen species scavenging capacity in cabbage. Front. Plant Sci. 7:197. doi: 10.3389/fpls.2016.00197
Zhao, H., Ye, L., Wang, Y., Zhou, X., Yang, J., Wang, J., et al. (2016). Melatonin increases the chilling tolerance of chloroplast in cucumber seedlings by regulating photosynthetic electron flux and the ascorbate-glutathione cycle. Front. Plant Sci. 7:1814. doi: 10.3389/fpls.2016.01814
Zhou, B., Guo, Z., and Liu, Z. (2005). Effects of abscisic acid on antioxidant systems of Stylosanthes guianensis (Aublet) Sw. under chilling stress. Crop Sci. 45, 599–605. doi: 10.2135/cropsci2005.0599
Keywords: grape leaves, melatonin, ozone stress, ethylene, antioxidant capacity
Citation: Liu C, Kang H, Wang Y, Yao Y, Gao Z and Du Y (2021) Melatonin Relieves Ozone Stress in Grape Leaves by Inhibiting Ethylene Biosynthesis. Front. Plant Sci. 12:702874. doi: 10.3389/fpls.2021.702874
Received: 30 April 2021; Accepted: 30 June 2021;
Published: 28 July 2021.
Edited by:
Chris Winefield, Lincoln University, New ZealandCopyright © 2021 Liu, Kang, Wang, Yao, Gao and Du. This is an open-access article distributed under the terms of the Creative Commons Attribution License (CC BY). The use, distribution or reproduction in other forums is permitted, provided the original author(s) and the copyright owner(s) are credited and that the original publication in this journal is cited, in accordance with accepted academic practice. No use, distribution or reproduction is permitted which does not comply with these terms.
*Correspondence: Yuanpeng Du, ZHV5dWFucGVuZzAwMUAxNjMuY29t; Zhen Gao, Z2Fvejg5QHNkYXUuZWR1LmNu
†These authors have contributed equally to this work