- 1College of Land and Environment, National Key Engineering Laboratory for Efficient Utilization of Soil and Fertilizer Resources, Northeast China Plant Nutrition and Fertilization Scientific Observation and Research Center for Ministry of Agriculture and Rural Affairs, Key Laboratory of Protected Horticulture of Education Ministry and Liaoning Province, Shenyang Agricultural University, Shenyang, China
- 2The UWA Institute of Agriculture, The University of Western Australia, Perth, WA, Australia
- 3School of Biological Sciences, The University of Western Australia, Perth, WA, Australia
- 4School of Agriculture and Environment, The University of Western Australia, Perth, WA, Australia
- 5National Sorghum Improvement Center, Liaoning Academy of Agricultural Sciences, Shenyang, China
- 6Professional Technology Innovation Center of Magnesium Nutrition, Yingkou Magnesite Chemical Ind Group Co., Ltd., Yingkou, China
- 7Department of Biosystems and Technology, Swedish University of Agricultural Sciences, Alnarp, Sweden
The cyclic electron transport (CET), after the linear electron transport (LET), is another important electron transport pathway during the light reactions of photosynthesis. The proton gradient regulation 5 (PGR5)/PRG5-like photosynthetic phenotype 1 (PGRL1) and the NADH dehydrogenase-like complex pathways are linked to the CET. Recently, the regulation of CET around photosystem I (PSI) has been recognized as crucial for photosynthesis and plant growth. Here, we summarized the main biochemical processes of the PGR5/PGRL1-dependent CET pathway and its physiological significance in protecting the photosystem II and PSI, ATP/NADPH ratio maintenance, and regulating the transitions between LET and CET in order to optimize photosynthesis when encountering unfavorable conditions. A better understanding of the PGR5/PGRL1-mediated CET during photosynthesis might provide novel strategies for improving crop yield in a world facing more extreme weather events with multiple stresses affecting the plants.
Introduction
Life on earth depends on energy derived from the sun. Photosynthesis is the pivotal process that could harvest light energy and ultimately generate biomass using water, CO2 and mineral nutrients. The bulk of our earth’s energy resources is derived from global photosynthetic activity in either recent or ancient times (Vass et al., 2007; Liu et al., 2011; Lambers and Oliveira, 2019; Nawrocki et al., 2019). The normal operation of photosynthesis is inseparable from the participation of light energy, but any excessive high light would impact the photosystem II (PSII), photosystem I (PSI) and the other thylakoid membrane proteins and resulting in photoinhibition and the accumulation of reactive oxygen species (ROS; Foyer and Noctor, 1999; Chaux et al., 2017; Liu, 2020). In the real world, multiple stress episodes affecting growth and development are common (Suzuki et al., 2014). Any excessive high light situation could be exacerbated further with a co-occurring high temperature (Sun et al., 2017; Lu et al., 2020), low temperature (Liu et al., 2013; Song et al., 2020; Wu et al., 2020), phosphorus deficiency (Carstensen et al., 2018; Shi et al., 2019) and drought (Wada et al., 2019). Plants have evolved several adaptations to cope with the unfavorable light situation: adjusting leaf orientation, ROS scavenging competence (Gill and Tuteja, 2010), xanthophyll cycle (Kuczynska et al., 2020), state transitions strategy (Hepworth et al., 2021), cyclic electron transport (CET; Yadav et al., 2020) and photorespiration (Storti et al., 2019). This review summarizes the main biochemical processes of PGR5/PGRL1-dependent CET pathway. The significance of the PGR5/PGRL1-dependent CET pathway is discussed to understand how plants optimize photosynthesis under unfavorable conditions by protecting the PSII and PSI, ATP/NADPH ratio maintenance, and regulating the transitions between linear electron transport (LET) and CET.
Photosynthetic Electron Transport
Chloroplasts convert light energy into chemical energy via electron transport (ET), which provides energy for the Calvin cycle and other processes. During the LET, electrons derived from water splitting in PSII are transferred via the cytochrome (Cytb6f) complex, PSI and ferredoxin (Fd) to the ferredoxin-NADP reductase (FNR), which ultimately reduce NADP+ to NADPH, resulting in the production of NADPH (Figure 1A; Lu et al., 2020). The H+ enters the thylakoid lumen by the Q cycle, and the H+ produced by water splitting in OEC together to form the required proton motive force (pmf) across the thylakoid membrane (Wang et al., 2020). The pmf, composed of the transmembrane potential (Δψ) and proton gradient (ΔpH), plays a key role in driving the chloroplast ATP synthase to synthesize ATP (Lapashina and Feniouk, 2018). ATP synthesis coupled with the LET is known as noncyclic photophosphorylation (NCPSP; Arnon et al., 1954, 1958). The energy derived from LET and NCPSP plays an essential role in photosynthesis and other processes. However, there would be insufficient ATP from the LET during certain multiple stress situations. Plants could compensate for the deficiency of ATP/NADPH in the LET by using the CET around the PSI (Sato et al., 2019; Ma et al., 2021), the water-water cycle (Mehler reaction; Asada et al., 1999) and the mitochondrial alternative oxidase respiration (Meng et al., 2012).
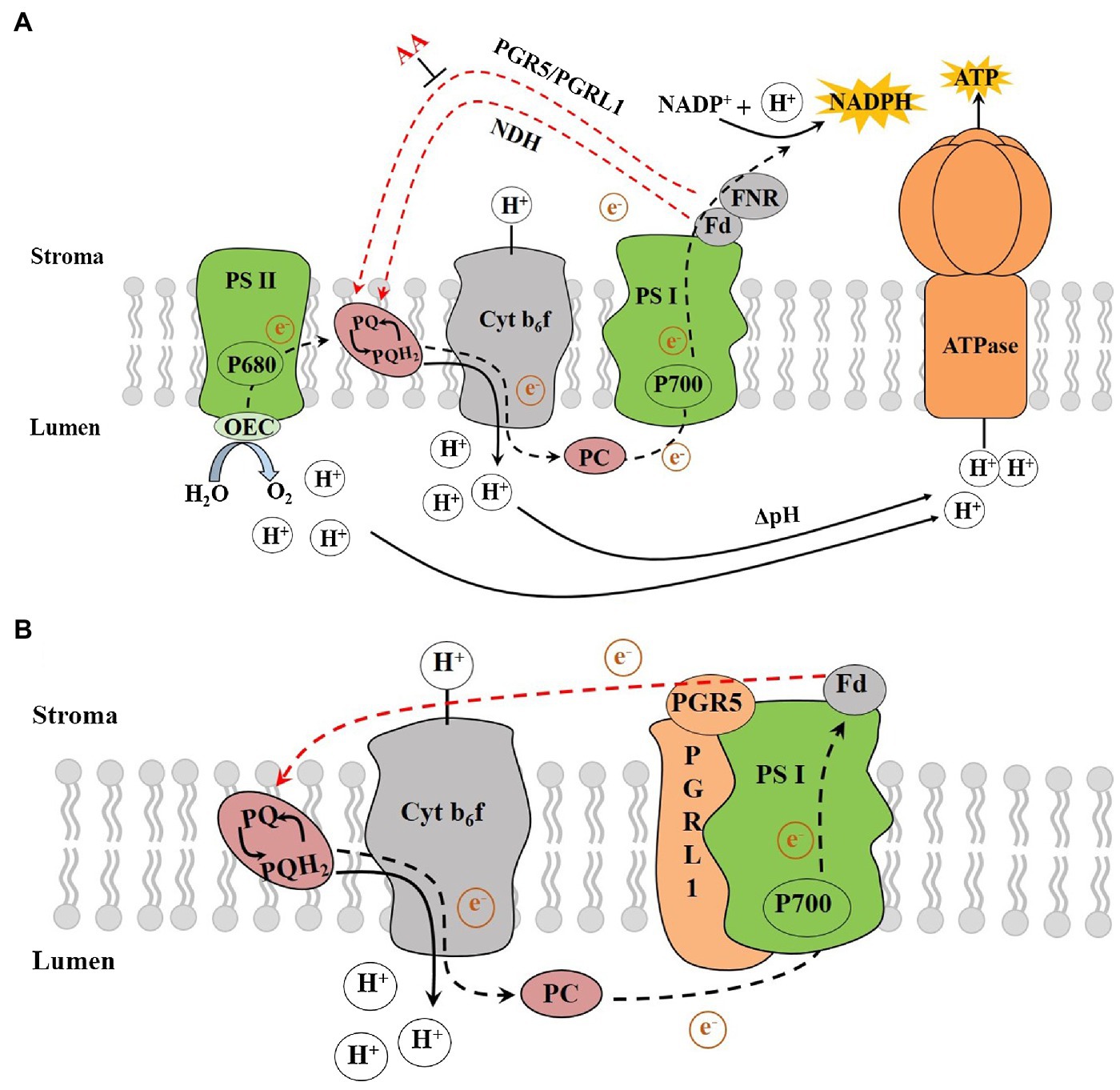
Figure 1. (A) The photosynthetic electron transport chain and (B) The PGR5/PGRL1-dependent CET. AA, antimycin A; ATPase, ATP synthase; Cytb6f, cytochrome b6f complex; Fd, ferredoxin; FNR, ferredoxin-NADP reductase; Lumen, thylakoid lumen; NADPH, reduced nicotinamide adenine dinucleotide phosphate; NDH, NADH dehydrogenase-like; OEC, oxygen-evolving complex; P680, pigment molecule of PSII reaction centre; P700, pigment molecule of PSI reaction centre; PC, plastocyanin; PGR5, proton gradient regulation 5; PGRL1, PRG5-like photosynthetic phenotype 1; PQ, plastoquinone; PQH2, plastoquinol; PSI, photosystem I; PSII, photosystem II, Stroma, thylakoid stroma and ΔpH, proton gradient (adapted from Yamori and Shikanai, 2016).
Cyclic Electron Transport
The pathway of electron transport around PSI, which recycles electrons from Fd to PQ, is called the CET, while the ATP synthesis coupled with it is called the cyclic photophosphorylation (Bendall and Manasse, 1995). Moss and Bendall (1984) proposed that an antimycin A (AA)-sensitive enzyme is involved in the ET from Fd to PQ termed as the ferredoxin-plastoquinone reductase (FQR) with the following configuration: PSI-Fd-FQR-PQ-Cyt b6f-PSI. The discovery of a protein complex that could receive electrons from Fd and transferring electrons to PQ represented major progress in CET research. Due to the high similarity to complex I within the mitochondrial respiratory chain, it was aptly called NDH (NADH dehydrogenase-like complex; Burrows et al., 1998). The NDH pathway is the main pathway compared with PGR5 one in cyanobacteria (Miller et al., 2021). It has been reported that NDH pathway plays a crucial role at high temperature or low temperature in tobacco (Wang et al., 2006) and low-light intensity in rice (Yamori et al., 2015) and Marchantia polymorpha (Ueda et al., 2012). However, it is not sensitive to AA, which implied that there is possibly another FQR pathway that is sensitive to AA and regulated by Fd in CET.
The PGR5/PGRL1-Mediated CET Pathway
It was suggested that the CET around PSI helps contribute electrons to synthesize ATP: Chlamydomonas (Yadav et al., 2020), Phaeodactylum (Zhou et al., 2020), C3 (Wang et al., 2015) and C4 plants (Munekage et al., 2010). There are at least two CET pathways in vascular plants and Phycophyta: antimycin A-sensitive pathway that involves proton gradient regulation 5 (PGR5) and PGR5-like photosynthetic phenotype 1 (PGRL1), and antimycin A-insensitive NADH dehydrogenase-like (NDH) pathway (Figure 1A; Munekage et al., 2002; Huang et al., 2005; Dalcorso et al., 2008; Taira et al., 2013; Ishikawa et al., 2016). There is a high similarity between the NDH and respiratory chain proteins. Conversely, PGR5 has no homology with the mitochondrial respiratory chain proteins. In a mutant of pgr5, due to less influx of protons that should be from the Q cycle, the ability of non-photochemical quenching (NPQ) PSII is reduced under strong light (Yadav et al., 2020). Although PGR5 plays a key role in CET from Fd to PQ, its molecular characteristics are not sufficient to deliver all the functionality reported for FQR. Specifically, PGR5 does not contain any redox-active cysteine residues that mediates ET nor has any transmembrane domains (Yamori and Shikanai, 2016). Therefore, the role played by PGR5 in the AA-sensitive CET pathway is still unclear. It was suggested that the decrease of CET activity in pgr5 mutants is due to its plausible role in feedback regulation (Nandha et al., 2007), and the postulated function of PGR5 is to regulate LET (Suorsa et al., 2012).
The PGRL1 was identified as another important regulator of the CET process in Arabidopsis. Plants lacking PGRL1 showed a decrease in CET rate and exhibiting a similar performance to the pgr5 mutant (Dalcorso et al., 2008; Yadav et al., 2020). The regulatory role of PGR5/PGRL1-dependent CET under environmental perturbations has been studied (Wang et al., 2014; Yamori et al., 2016; Wolf et al., 2020). PGR5 is a small thylakoid protein without any known motifs that suggest its function (Munekage et al., 2002), while PGRL1 is a transmembrane protein with two transmembrane domains, and its two cysteine residues are involved in an iron cofactor binding (Hertle et al., 2013). The previous studies showed that the PGR5 proteins in Arabidopsis have low similarity to those found in cyanobacteria, excluding the coding genes of PGRL1 (Peltier et al., 2010). The double mutant of Arabidopsis prgl1ab showed a phenotype similar to that of pgr5 (Dalcorso et al., 2008). In rice pgr5 mutants, the PGRL1 protein level decreased by 50% (Nishikawa et al., 2012). Generally, the transport of electrons from Fd to PGRL1 requires the participation of PGR5 proteins, where the loss of any protein would affect the CET activity (Munekage et al., 2002; Dalcorso et al., 2008; Kono et al., 2014). Under in vitro conditions and when reduced Fd is present, an unknown redox reaction would catalyse the formation of disulphide bonds between cysteine residues in PGRL1 and the recombinant PGRL1 thereby reducing the analogue of PQ and quinone 2,6-dimethyl-p-benzoquinone (Hertle et al., 2013). This finding was confirmed by examining the mercaptan group of FQR (Strand et al., 2016). Shikanai (2007) speculated that PGR5 and PGRL1 proteins are important components of FQR. Subsequently, when the molecular features of PGRL1 were found to be similar to the FQR protein, the researchers further proposed that PGRL1 could be the FQR proteins (Figure 1B; Hertle et al., 2013; Labs et al., 2016).
State Transitions and the CET
In plants, the redistribution of excitation energy between the two photosystems is modulated by reversible phosphorylation of light-harvesting complex II (LHCII) in response to light fluctuation (Allen et al., 1981; Bhatti et al., 2020). Generally, these processes are known as state transitions (Bonaventura and Myers, 1969). For Chlamydomonas reinhardtii, when PSII is excited, the LHCII is phosphorylated, separated from PSII and adhering to the PSI. Meanwhile, the absorbed light energy is allocated to PSI, and thereby allowing the CET to dominate; this is called state II. PSI is preferentially excited during state I during which LHCII-P is dephosphorylated, recombined with PSII, and giving priority to facilitate the LET (Finazzi et al., 2002). While it is true that CET is the main pathway of ET during state II, this does not imply that state II is a necessary condition for the CET to operate. It was found in C. reinhardtii and Arabidopsis that the CET is not affected by the state transition (Takahashi et al., 2013). Although the CET is not related to state transition, state II is beneficial for the separation of the CET-PSI complexes (Yamori and Shikanai, 2016). The core mechanism of PGR5/PGRL1-mediated CET is similar in C. reinhardtii and Arabidopsis (Yamori and Shikanai, 2016), except for their supercomplex components related to ET. Iwai et al. (2010) identified the supercomplexes containing FNR, Fd, PGRL1, Cytb6 and PSI in C. reinhardtii. In Arabidopsis, however, it was only confirmed that the PGRL1-PGR5 complex could interact with PSI, thus facilitating the formation of Arabidopsis CET supercomplex (Dalcorso et al., 2008). Although the potential CET supercomplex was not identified clearly, there were more studies to support the association of PGR5 and PGRL1 in the CET (Breyton et al., 2006; Xue et al., 2017).
The Function of PGR5/PGRL1-Mediated CET in Plants
Regulating the Level of ATP and Maintaining the Balance of ATP/NADPH
The ‘Proton Gradient Regulation 5’ or PGR5 plays a pivotal role in proton gradient regulation. In the chloroplasts, the regulation of pmf must satisfy two competing physiological demands: (1) ensuring the requirements of carbon fixation for ATP and (2) decreasing the ET rate to avoid light damage under certain situations. Under relatively low light, the proportion of Δψ is equal to that of ΔpH. With increasing light, the proportion of ΔpH in pmf increases gradually. In Arabidopsis pgr5 mutants, ΔpH accounts for about 90% of the total pmf at a light intensity greater than 312 μmol·m−2 s−1 (Yamamoto et al., 2016). Therefore, the size of ΔpH may be partly compensated by increasing the partitioning of ΔpH in pmf in pgr5 (Yamamoto et al., 2016). To move from Δψ to ΔpH, some cations (mainly Mg2+ and K+) have to be transported to the stroma via the thylakoid membrane (Kramer et al., 2003). Hind et al. (1974) indicated that the outflow of these cations could facilitate pmf adjusting into the ΔpH form. Besides, AtVCCN1, a voltage-dependent chloride channel, located in Arabidopsis thylakoid membrane can make Cl− influx into the lumen during illumination and partially dissipate the Δψ in the lumen, thereby increasing the ΔpH/Δψ ratio (Herdean et al., 2016).
Plants regulate the proportion of ATP/NADPH to meet the competing demands of metabolism and photoprotection. Thus, the regulation of the electron distribution between LET and CET is essential to maintaining optimal photosynthesis under prevailing conditions. Particularly, the PGR5/PGRL1-dependent CET plays a central role in the regulation of LET via the downregulation of the Cyt b6f complex (Shikanai, 2014; Yamori et al., 2016). The function of CET is more relevant under conditions when the LET cannot produce sufficient ΔpH; consequently, it is necessary to improve the ratio of ATP/NADPH by increasing the ΔpH to promote the synthesis for more ATP (Figure 2; Yamori and Shikanai, 2016). Besides, sufficient ΔpH of thylakoid lumen contributes to the downregulation of electron transport through NPQ, preventing photodamage (Niyogi, 1999; Shikanai, 2007). Both PGR5/PGRL1 and NDH-mediated CET play a role in low light and facilitating CO2 assimilation by providing additional ATP (Nishikawa et al., 2012). In contrast, the regulatory effect of PGR5/PGRL1 and NDH-mediated CET on ATP/NADPH is negligible in rice-growing under strong light (Yamori et al., 2015). However, strong light led to the decrease of pmf formation in pgr5 mutant in Arabidopsis and the concomitant decrease of ATP yield, thereby disrupting the optimal ATP/NADPH balance (Kawashima et al., 2017). The stimulation of ATP/NADPH homeostasis in primary metabolism demonstrated that the energy requirement under high light is not less than that under low light (Walker et al., 2014). Hence, one might envisage that the CET should be beneficial to regulating the balance of ATP/NADPH under different light conditions. Recent studies have shown that the CET was needed to achieve a balanced ATP/NADPH ratio even under non-stress conditions in C3 plants (Wang et al., 2015).
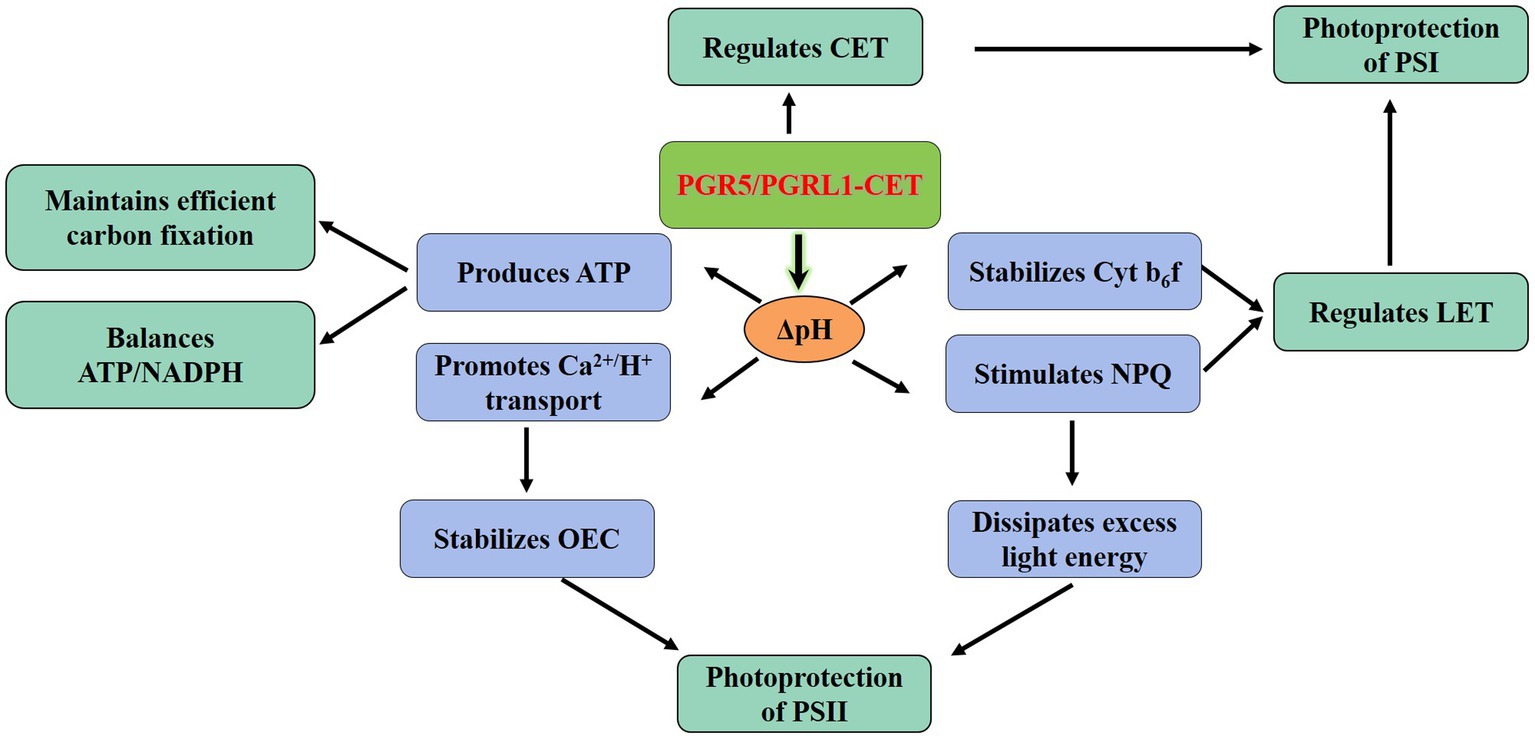
Figure 2. Physiological functionality of the PGR5/PGRL1-dependent CET. The PGR5/PGRL1-mediated CET produces more ATP by increasing ΔpH to balance ATP/NADPH and meeting the requirements for efficient C fixation. Meanwhile, the increase of ΔpH would protect the PSII from photoinhibition via stimulating the NPQ and facilitating the transport of Ca2+/H+. ATP, adenosine triphosphate; CET, cyclic electron transport; Cytb6f, cytochrome b6f complex; LET, linear electron transport; NADPH, reduced nicotinamide adenine dinucleotide phosphate; NPQ, non-photochemical quenching; OEC, oxygen-evolving complex; PGR5, proton gradient regulation 5; PGRL1, PRG5-like photosynthetic phenotype 1; PSI, photosystem I and PSII, photosystem II.
Inducing the NPQ and Protecting the PSII
Any excess light during photosynthesis would lead to photo-oxidative damage and reducing carbon fixation (Paredes and Quiles, 2017). The non-photochemical quenching mechanism (NPQ) plays an essential role in the photoprotection mechanism (Erickson et al., 2015). Inducing the qE component of NPQ to dissipate excessive absorbed light energy is dependent on the thylakoid lumen acidification modulated by the CET (Müller et al., 2001; Johnson et al., 2014). Under multiple stresses, electrons will preferentially reduce Fd and NADP+ and not O2, thereby avoiding oxidative damage to the photosystems caused by the excess light energy (Chow and Hope, 2004; Kukuczka et al., 2014). The previous studies have shown that NDH-mediated CET plays a significant role in rice and M. polymorpha under low light (Ueda et al., 2012; Yamori et al., 2015). However, in Arabidopsis, the deletion of the NDH gene did not alter photosynthesis significantly (Hashimoto et al., 2003). It was only when both PGR5 and NDH were mutated that the seedling has an altered phenotype (Munekage et al., 2004). Therefore, for C3 plants, the PGR5/PGRL1 pathway is the major pathway of CET (Munekage et al., 2004; Okegawa et al., 2008; Wang et al., 2015; Okegawa and Motohashi, 2020). Recent studies have indicated that the role of CET probably varies with light intensity (Huang et al., 2015). The generation of CET-dependent pmf is for the synthesis of ATP under lower light (Avenson et al., 2005; Walker et al., 2014). With higher light, the acidification of thylakoid lumen is beneficial to protect PSI and PSII from photoinhibition (Takahashi et al., 2009; Tikkanen et al., 2014).
Both pgr5 and pgrl1 mutants in Arabidopsis were sensitive to abiotic stress, such as high light and extreme temperature (Munekage et al., 2002; Jin et al., 2017; Kawashima et al., 2017). When the PSII repair function of wild-type and pgr5 mutants was inhibited, compared with wild-type plants, PSII in pgr5 mutants was still more sensitive to strong light (Takahashi et al., 2009), indicating that PGR5 deficiency caused photodamage to photosystems (Okegawa et al., 2010). The PSI is the likely primary target of photoinhibition, and the dynamic balance between photodamage and restoration in PSII maintains its stability (Pospsil and Tyystjarvi, 1999). Generally, the protection of PSII by CET mediated by PGR5/PGRL1 under adverse environments involves at least two different mechanisms. Firstly, the acidification of the thylakoid lumen activates NPQ to dissipate excess light energy, thereby reducing ROS production in the PSII complex (Munekage et al., 2008). Secondly, the formation of ΔpH promotes the reversed Ca2+/H+ transport to increase the concentration of Ca2+ in the thylakoid lumen (Ettinger et al., 1999). As the stability of OEC depended on the level of lumen Ca2+ (Krieger and Weis, 1993), the acidification in the lumen would avoid the photodamage of PSII by increasing the stability of OEC (Figure 2; Takahashi et al., 2009; Huang et al., 2016). Notably, the effects of PGR5 overexpression were strikingly pleiotropic. The accumulation of PGR5 could enhance the high-light resistance of the plants, but it also markedly delayed the greening of cotyledons, thereby causing the slower seedling growth in the initial growth stage (Okegawa et al., 2007; Long et al., 2008; Sugimoto et al., 2013).
Regulating the LET and Protecting the PSI
The Fe-S clusters within the PSI complex are vulnerable to ROS when exposed to fluctuating light. In particular, PSI photodamage occurred before PSII in pgr5 mutants (Sonoike, 2011; Suorsa et al., 2012; Kono et al., 2014, 2017). Unlike the effective and fast repair of PSII, the restoration of PSI is slower and consequently. In general, most PSI damages are considered to be almost irreversible (Zivcak et al., 2014). Gollan et al. (2017) found that PSI damage inhibited carbon fixation and other processes after high-light exposure.
Similar to its role in PSII, the protective effect of PGR5/PGRL1-mediated CET on PSI is related to the formation of ΔpH (Yamamoto and Shikanai, 2019). The CET-dependent ΔpH formation not only contributes to the synthesis of ATP but also regulates the ET via acidifying the thylakoid lumen (Shikanai, 2014, 2016; Yamori and Shikanai, 2016). The PSI acceptor-side regulation by CET sustains electron sinks downstream of PSI and preventing the over-reduction of the PSI (Munekage et al., 2002). The acidification of the thylakoid lumen downregulates the Cyt b6f complex thereby slowing down the ET from PSII towards PSI and induces the thermal dissipation of absorbed excess photon energy from the PSII antennae (Shikanai, 2016; Yamamoto and Shikanai, 2019). This is the PSI donor-side regulation by CET for PSI photoprotection (Suorsa et al., 2012). It has been reported that exogenous calcium alleviates nocturnal chilling-induced photo damage by facilitating CET, thereby enhancing the photosynthesis and biomass accumulation of peanut under low nocturnal temperature stress (Song et al., 2020; Wu et al., 2020). Additionally, plant dry weight was significantly lowered in the rice PGR5-knockdown line compared to that of WT, especially under fluctuating light (Yamori et al., 2016). Therefore, stimulating CET via artificial growth regulation might be a novel strategy to maintain sufficient photosynthetic carbon fixation and enhance yield under unfavorable conditions. Most notably, Rantala et al. (2020) indicated that both PGR5 and NDH-1 systems do not function as protective electron acceptors but mitigate the consequences of PSI inhibition and protected the remaining PSI centres by enhancing pH-dependent regulation of electron transfer from PSII to PSI.
Functional analysis showed the PSI remained fully reduced under high light in pgr5 mutants. Interestingly, in the wild type under high light, the PSI complex was oxidized; the damage of PSI in pgr5 was later mitigated by exogenous application of 3-(3,4-dichlorophenyl)-1,1-dimethylurea (DCMU: inhibitor of the PSII to PSI ET; Tikkanen et al., 2010). These observations implied that the PGR5/PGRL1-mediated CET could reduce PSI damage from excessive electron flow under strong light. PGR5 and PGRL1 play crucial roles in the efficient operation of CET, whereas the maximum rate of CET is only slightly affected in pgr5 mutants (Nandha et al., 2007). Although the CET varies slightly, the change of ATP/NADPH ratio would be sufficient to have a substantial impact on the levels of ADP, phosphatidylinositol (Pi) and NADP+, thus reducing the activity of PSI electron acceptor and modulating the rate of LET (Kramer et al., 2004; Avenson et al., 2005; Suorsa et al., 2016). This evidence highlighted the important role of PGR5 in regulating the LET to CET transition (Figure 2; Suorsa et al., 2016).
Future Outlook
Optimizing photosynthesis is an effective way to improve plant productivity. However, the variation of light and environmental conditions would often lower photosynthetic capacity and hampering electron transmission. The PGR5/PGRL1-dependent CET around PSI plays an important homeostatic role in electron transfers and thereby alleviating photoinhibition. With the recent advent of molecular techniques and sensitive analytical tools, scientists have achieved a better understanding of the PGR5/PGRL1-CET putative structure and functionality. Thus, the biological significance of the PGR5/PGRL1 pathway is better understood now although there exist several unsolved questions: pathway initiation and interactions leading to better efficiency; the relationship between PGR5/PGRL1 and FQR and the effect of PGR5/PGRL1 expression on the PSII. Most published studies have focused on Arabidopsis and rice, and with less emphasis on other crops. With the availability of novel research tools, it is possible to elucidate the complex regulatory network of the PGR5/PGRL1 pathway and its role in optimizing photosynthesis under unfavorable conditions.
Author Contributions
YL, MM, XH, and JY are responsible for the general overview of the opinions stated in the manuscript. YL, MM, CB, YY, ZS, XL, SZ, and JY wrote and modified the manuscript. All authors reviewed and approved the final version of the submitted manuscript.
Funding
This research was funded by the National Natural Science Foundation of China (project nos. 31772391 and 31301842), National Peanut Research System (project no. CARS-13-Nutrient Management), National Key Research and Development Plan (project no. 2018YFD0201001), Sheng Jing Talents Project (project no. RC170338) and China Scholarship Council Project.
Conflict of Interest
YY was employed by the company Yingkou Magnesite Chemical Ind Group Co. Ltd.
The remaining authors declare that the research was conducted in the absence of any commercial or financial relationships that could be construed as a potential conflict of interest.
References
Allen, J. F., Bennett, J., Steinback, K. E., and Arntzen, C. J. (1981). Chloroplast protein phosphorylation couples plastoquinone redox state to distribution of excitation energy between photosystems. Nature 291, 25–29. doi: 10.1038/291025a0
Arnon, D. I., Allen, M. B., and Whatley, F. R. (1954). Photosynthesis by isolated chloroplasts. Nature 174, 394–396. doi: 10.1038/174394a0
Arnon, D. I., Whatley, F. R., and Allen, M. B. (1958). Assimilatory power in photosynthesis. Science 127, 1026–1034. doi: 10.1126/Science.127.3305.1026
Asada, Y., Miyake, M., Miyake, J., Kurane, R., and Tokiwa, Y. (1999). Photosynthetic accumulation of poly-(hydroxybutyrate) by cyanobacteria—the metabolism and potential for CO2 recycling. Int. J. Biol. Macromol. 25, 37–42. doi: 10.1016/s0141-8130(99)00013-6
Avenson, T. J., Cruz, J. A., Kanazawa, A., and Kramere, D. M. (2005). Regulating the proton budget of higher plant photosynthesis. Proc. Natl. Acad. Sci. U. S. A. 102, 9709–9713. doi: 10.1073/pnas.0503952102
Bendall, D. S., and Manasse, R. S. (1995). Cyclic photophosphorylation and electron transport. Biochim. Biophys. Acta. Bioenerg. 1229, 23–38. doi: 10.1016/0005-2728(94)00195-B
Bhatti, A. F., Choubeh, R. R., Kirilovsky, D., Wientjes, E., and Amerongen, H. V. (2020). State transitions in cyanobacteria studied with picosecond fluorescence at room temperature. Biochim. Biophys. Acta. Bioenerg. 2020:148255. doi: 10.1016/j.bbabio.2020.148255
Bonaventura, C., and Myers, J. (1969). Fluorescence and oxygen evolution from Chlorella pyrenoidosa. Biochim. Biophys. Acta. Bioenerg. 189, 366–383. doi: 10.1016/0005-2728(69)90168-6
Breyton, C., Nandha, B., Johnson, G. N., Joliot, P., and Finazzi, G. (2006). Redox modulation of cyclic electron flow around photosystem I in C3 plants. Biochemistry 45, 13465–13475. doi: 10.1021/bi061439s
Burrows, P. A., Sazanov, A., Svab, Z., Maliga, P., and Nixon, P. J. (1998). Identification of a functional respiratory complex in chloroplasts through analysis of tobacco mutants containing disrupted plastid ndh genes. EMBO J. 17, 868–876. doi: 10.1093/emboj/17.4.868
Carstensen, A., Herdean, A., Schmidt, S. B., Sharma, A., Spetea, C., Pribil, M., et al. (2018). The impacts of phosphorus deficiency on the photosynthetic electron transport chain. Plant Physiol. 177, 271–284. doi: 10.1104/pp.17.01624
Chaux, F., Johnson, X., Auroy, P., Beyly-Adriano, A., Te, L., Cuiné, S., et al. (2017). PGRL1 and LHCSR3 compensate for each other in controlling photosynthesis and avoiding photosystem I photoinhibition during high light acclimation of Chlamydomonas cells. Mol. Plant 10, 216–218. doi: 10.1016/j.molp.2016.09.005
Chow, W. S., and Hope, A. B. (2004). Electron fluxes through photosystem I in cucumber leaf discs probed by far-red light. Photosynth. Res. 81, 77–89. doi: 10.1023/B:PRES.0000028396.83954.36
Dalcorso, G., Pesaresi, P., Masiero, S., Aseeva, E., Danja, S., Finazzi, G., et al. (2008). A complex containing PGRL1 and PGR5 is involved in the switch between linear and cyclic electron flow in Arabidopsis. Cell 132, 273–285. doi: 10.1016/j.cell.2007.12.028
Erickson, E., Wakao, S., and Niyogi, K. K. (2015). Light stress and photoprotection in Chlamydomonas reinhardtii. Plant J. 82, 449–465. doi: 10.1111/tpj.12825
Ettinger, W. F., Clear, A. M., Fanning, K. J., and Peck, M. L. (1999). Identification of a Ca2+/H+ antiport in the plant chloroplast thylakoid membrane. Plant Physiol. 119, 1379–1386. doi: 10.1104/pp.119.4.1379
Finazzi, G., Rappaport, F., Furia, A., Fleischmann, M., Rochaix, J. D., Zito, F., et al. (2002). Involvement of state transitions in the switch between linear and cyclic electron flow in Chlamydomonas reinhardtii. EMBO Rep. 3, 280–285. doi: 10.1093/embo-reports/kvf047
Foyer, C. H., and Noctor, G. (1999). Leaves in the dark see the light. Science 284, 599–601. doi: 10.1126/Science.284.5414.599
Gill, S. S., and Tuteja, N. (2010). Reactive oxygen species and antioxidant machinery in abiotic stress tolerance in crop plants. Plant Physiol. Biochem. 48, 909–930. doi: 10.1016/j.plaphy.2010.08.016
Gollan, P. J., Lima-Melo, Y., Tiwari, A., Tikkanen, M., and Aro, E. M. (2017). Interaction between photosynthetic electron transport and chloroplast sinks triggers protection and signalling important for plant productivity. Philos. Trans. R. Soc. B. Biol. Sci. 372:20160390. doi: 10.1098/rstb.2016.0390
Hashimoto, M., Endo, T., Peltier, G., Tasaka, M., and Shikanai, T. (2003). A nucleus-encoded factor, CRR2, is essential for the expression of chloroplast ndhB in Arabidopsis. Plant J. 36, 541–549. doi: 10.1046/j.1365-313X.2003.01900.x
Hepworth, C., Wood, W. H. J., Emrich-Mills, T. Z., Proctor, M. S., Casson, S., and Johnson, M. P. (2021). Dynamic thylakoid stacking and state transitions work synergistically to avoid acceptor-side limitation of photosystem I. Nat. Plants. 7, 87–98. doi: 10.1038/s41477-020-00828-3
Herdean, A., Teardo, E., Nilsson, A. K., Pfeil, B. E., Johansson, O. N., Ünnep, R., et al. (2016). A voltage-dependent chloride channel fine-tunes photosynthesis in plants. Nat. Commun. 7:11654. doi: 10.1038/ncomms11654
Hertle, A. P., Blunder, T., Wunder, T., Pesaresi, P., Pribil, M., Armbruster, U., et al. (2013). PGRL1 is the elusive ferredoxin-plastoquinone reductase in photosynthetic cyclic electron flow. Mol. Cell 49, 511–523. doi: 10.1016/j.molcel.2012.11.030
Hind, G., Nakatani, H. Y., and Izawa, S. (1974). Light-dependent redistribution of ions in suspensions of chloroplast thylakoid membranes. Proc. Natl. Acad. Sci. U. S. A. 71, 1484–1488. doi: 10.1073/pnas.71.4.1484
Huang, L. S., Cobessi, D., Tung, E. Y., and Berry, E. A. (2005). Binding of the respiratory chain inhibitor antimycin to the mitochondrial bc1 complex: a new crystal structure reveals an altered intramolecular hydrogen-bonding pattern. J. Mol. Biol. 351, 573–597. doi: 10.1016/j.jmb.2005.05.053
Huang, W., Yang, Y. J., Hu, H., and Zhang, S. B. (2015). Different roles of cyclic electron flow around photosystem I under sub-saturating and saturating light intensities in tobacco leaves. Front. Plant Sci. 6:923. doi: 10.3389/fpls.2015.00923
Huang, W., Yang, Y. J., Hu, H., Zhang, S. B., and Cao, K. F. (2016). Evidence for the role of cyclic electron flow in photoprotection for oxygen-evolving complex. J. Plant Physiol. 194, 54–60. doi: 10.1016/j.jplph.2016.02.016
Ishikawa, N., Takabayashia, A., Sato, F., and Endo, T. (2016). Accumulation of the components of cyclic electron flow around photosystem I in C4 plants, with respect to the requirements for ATP. Photosynth. Res. 129, 261–277. doi: 10.1007/s11120-016-0251-0
Iwai, M., Takizawa, K., Tokutsu, R., Okamuro, A., Takahashi, Y., and Minagawa, J. (2010). Isolation of the elusive supercomplex that drives cyclic electron flow in photosynthesis. Nature 464, 1210–1213. doi: 10.1038/nature08885
Jin, Y. J., Chen, S., Fan, X. J., Song, H., Li, X. X., Xu, J. H., et al. (2017). Diuron treatment reveals the different roles of two cyclic electron transfer pathways in photosystem II in Arabidopsis thaliana. Pestic. Biochem. Physiol. 137, 15–20. doi: 10.1016/j.pestbp.2016.09.002
Johnson, X., Steinbeck, J., Dent, R. M., Takahashi, H., Richaud, P., Ozawa, S. I., et al. (2014). Proton gradient regulation 5-mediated cyclic electron flow under ATP- or redox-limited con-ditions: a study of ΔATPase pgr5 and ΔrbcL pgr5; mutants in the green alga Chlamydomonas reinhardtii. Plant Physiol. 165, 438–452. doi: 10.1104/pp.113.233593
Kawashima, R., Sato, R., Harada, K., and Masuda, S. (2017). Relative contributions of PGR5- and NDH-dependent photosystem I cyclic electron flow in the generation of a proton gradient in Arabidopsis chloroplasts. Planta 246, 1045–1050. doi: 10.1007/s00425-017-2761-1
Kono, M., Noguchi, K., and Terashikma, I. (2014). Roles of the cyclic electron flow around PSI (CET-PSI) and O2-dependent alternative pathways in regulation of the photosynthetic electron flow in short-term fluctuating light in Arabidopsis thaliana. Plant Cell Physiol. 55, 990–1004. doi: 10.1093/pcp/pcu033
Kono, M., Yamori, W., Suzuki, Y., and Terashima, I. (2017). Photoprotection of PSI by far-red light against the fluctuating light-induced photoinhibition in Arabidopsis thaliana and field-grown plants. Plant Cell Physiol. 58, 35–45. doi: 10.1093/pcp/pcw215
Kramer, D. M., Avenson, T. J., and Edwards, G. E. (2004). Dynamic flexibility in the light reactions of photosynthesis governed by both electron and proton transfer reactions. Trends Plant Sci. 9, 349–357. doi: 10.1016/j.tplants.2004.05.001
Kramer, D. M., Cruz, J. A., and Kanazawa, A. (2003). Balancing the central roles of the thylakoid proton gradient. Trends Plant Sci. 8, 27–32. doi: 10.1016/S1360-1385(02)00010-9
Krieger, A., and Weis, E. (1993). The role of calcium in the pH-dependent control of photosystem II. Photosynth. Res. 37, 117–130. doi: 10.1007/BF02187470
Kuczynska, P., Jemiola-Rzeminska, M., Nowicka, B., Jakubowska, A., Strzalka, W., Burda, K., et al. (2020). The xanthophyll cycle in diatom Phaeodactylum tricornutum in response to light stress. Plant Physiol. Biochem. 152, 125–137. doi: 10.1016/j.plaphy.2020.04.043
Kukuczka, B., Magneschi, L., Petroutsos, D., Steinbeck, J., Bald, T., Powikrowska, M., et al. (2014). Proton gradient regulation5-like1-mediated cyclic electron flow is crucial for acclimation to anoxia and complementary to non-photochemical quenching in stress adaptation. Plant Physiol. 165, 1604–1617. doi: 10.1104/pp.114.240648
Labs, M., Rühle, T., and Leister, D. (2016). The antimycin A-sensitive pathway of cyclic electron flow: from 1963 to 2015. Photosynth. Res. 129, 231–238. doi: 10.1007/s11120-016-0217-2
Lambers, H., and Oliveira, R. S. (2019). “Photosynthesis, respiration, and long-distance transport: photosynthesis,” in Plant Physiological Ecology (Cham: Springer), 111–114.
Lapashina, A. S., and Feniouk, B. A. (2018). ADP-inhibition of H+-FOF1-ATP synthase. Biochemistry 83, 1141–1160. doi: 10.1134/S0006297918100012
Liu, Y. F. (2020). Calcium chemical priming might play a significant role in relieving overnight chilling-dependent inhibition of photosynthesis in crops: a review. Basic Clin. Pharmacol. Toxicol. 126, 109–110. doi: 10.3389/fpls.2020.607029
Liu, Y. F., Han, X. R., Zhan, X. M., Yang, J. F., Wang, Y. Z., Song, Q. B., et al. (2013). Regulation of calcium on peanut photosynthesis under low night temperature stress. J. Integr. Agric. 12, 2172–2178. doi: 10.1016/S2095-3119(13)60411-6
Liu, Y. F., Qi, H. Y., Bai, C. M., Qi, M. F., Xu, C. Q., Hao, J. H., et al. (2011). Grafting helps improve photosynthesis and carbohydrate metabolism in leaves of muskmelon. Int. J. Biol. Sci. 7, 1161–1170. doi: 10.7150/ijbs.7.1161
Long, T. A., Okegawa, Y., Shikanai, T., Schmidt, G. W., and Covert, S. F. (2008). Conserved role of proton gradient regulation 5 in the regulation of PSI cyclic electron transport. Planta 228, 907–918. doi: 10.1007/s00425-008-0789-y
Lu, J., Yin, Z., Lu, T., Yang, X., Wang, F., Qi, M., et al. (2020). Cyclic electron flow modulates the linear electron flow and reactive oxygen species in tomato leaves under high temperature. Plant Sci. 292:110387. doi: 10.1016/j.plantsci.2019.110387
Ma, M., Liu, Y., Bai, C., and Yong, J. W. H. (2021). The significance of chloroplast NAD(P)H dehydrogenase complex and its dependent cyclic electron transport in photosynthesis. Front. Plant Sci. 12:661863. doi: 10.3389/fpls.2021.661863
Meng, X. L., Zhang, L. T., Zhang, Z. S., Gao, H. Y., and Meng, Q. W. (2012). Role of mitochondrial alternative oxidase (AOX) pathway in photoprotection in Rumex K-1 leaves. Chin. J. Appl. Ecol. 23, 1803–1808. doi: 10.13287/j.1001-9332.2012.0235
Miller, N. T., Vaughn, M. D., and Burnap, R. L. (2021). Electron flow through NDH-1 complexes is the major driver of cyclic electron flow-dependent proton pumping in cyanobacteria. Biochim. Biophys. Acta. Bioenerg. 1862:148354. doi: 10.1016/j.bbabio.2020.148354
Moss, D. A., and Bendall, D. S. (1984). Cyclic electron transport in chloroplasts. The Q-cycle and the site of action of antimycin. Biochim. Biophys. Acta. Bioenerg. 767, 389–395. doi: 10.1016/0005-2728(84)90036-7
Müller, P., Li, X. P., and Niyogi, K. K. (2001). Non-photochemical quenching. A response to excess light energy. Plant Physiol. 125, 1558–1566. doi: 10.1104/pp.125.4.1558
Munekage, Y. N., Eymery, F., Rumeau, D., Cuiné, S., Oguri, M., Nakamura, N., et al. (2010). Elevated expression of PGR5 and NDH-H in bundle sheath chloroplasts in C4 flaveria species. Plant Cell Physiol. 51, 664–668. doi: 10.1093/pcp/pcq030
Munekage, Y. N., Genty, B., and Peitier, G. (2008). Effect of PGR5 impairment on photosynthesis and growth in Arabidopsis thaliana. Plant Cell Physiol. 49, 1688–1698. doi: 10.1093/pcp/pcn140
Munekage, Y., Hashimoto, M., Miyake, C., Tomizawa, K. I., Endo, T., Tasaka, M., et al. (2004). Cyclic electron flow around photosystem I is essential for photosynthesis. Nature 429, 579–582. doi: 10.1038/nature02598
Munekage, Y., Hojo, M., Meurer, J., Endo, T., Tasaka, M., and Shikanai, T. (2002). PGR5 is involved in cyclic electron flow around photosystem I and is essential for photoprotection in Arabidopsis. Cell 110, 361–371. doi: 10.1016/S0092-8674(02)00867-X
Nandha, B., Finazzi, G., Joliot, P., Hald, S., and Johnson, G. N. (2007). The role of PGR5 in the redox poising of photosynthetic electron transport. Biochim. Biophys. Acta. Bioenerg. 1767, 1252–1259. doi: 10.1016/j.bbabio.2007.07.007
Nawrocki, W. J., Bailleul, B., Picot, D., Cardol, P., Rappaport, F., Wollman, F. A., et al. (2019). The mechanism of cyclic electron flow. Biochim. Biophys. Acta. Bioenerg. 1860, 433–438. doi: 10.1016/j.bbabio.2018.12.005
Nishikawa, Y., Yamamoto, H., Okegawa, Y., Shinya, W., Nozomi, S., Yoshichika, T., et al. (2012). PGR5-dependent cyclic electron transport around PSI contributes to the redox homeostasis in chloroplasts rather than CO2 fixation and biomass production in rice. Plant Cell Physiol. 53, 2117–2126. doi: 10.1093/pcp/pcs153
Niyogi, K. K. (1999). Photoprotection revisited: genetic and molecular approaches. Annu. Rev. Plant Biol. 50, 333–359. doi: 10.1146/annurev.arplant.50.1.333
Okegawa, Y., Kagawa, Y., Kobayashi, Y., and Shikanai, T. (2008). Characterization of factors affecting the activity of photosystem I cyclic electron transport in chloroplasts. Plant Cell Physiol. 49, 825–834. doi: 10.1093/pcp/pcn055
Okegawa, Y., Kobayashi, Y., and Shikkanai, T. (2010). Physiological links among alternative electron transport pathways that reduce and oxidize plastoquinone in Arabidopsis. Plant J. 63, 458–468. doi: 10.1111/j.1365-313X.2010.04252.x
Okegawa, Y., Long, T. A., Iwano, M., Takayama, S., Kobayashi, Y., Covert, S. F., et al. (2007). A balanced PGR5 level is required for chloroplast development and optimum operation of cyclic electron transport around photosystem I. Plant Cell Physiol. 48, 462–471. doi: 10.1093/pcp/pcm116
Okegawa, Y., and Motohashi, K. (2020). M-type thioredoxins regulate the PGR5/PGRL1-dependent pathway by forming a disulfide-linked complex with PGRL1. Plant Cell 32, 3866–3883. doi: 10.1105/tpc.20.00304
Paredes, M., and Quiles, M. J. (2017). Chilling stress and hydrogen peroxide accumulation in Chrysanthemum morifolium and Spathiphyllum lanceifolium. Involvement of chlororespiration. J. Plant Physiol. 211, 36–41. doi: 10.1016/j.jplph.2016.11.015
Peltier, G., Tolleter, D., Billon, E., and Cournac, L. (2010). Auxiliary electron transport pathways in chloroplasts of microalgae. Photosynth. Res. 106, 19–31. doi: 10.1007/s11120-010-9575-3
Pospsil, P., and Tyystjarvi, E. (1999). Molecular mechanism of high temperature induced inhibition of acceptor side of photosystem II. Photosynth. Res. 62, 55–66. doi: 10.1023/A:1006369009170
Rantala, S., Lempiinen, T., Gerotto, C., Tiwari, A., Aro, E. M., and Tikkanen, M. (2020). PGR5 and NDH-1 systems do not function as protective electron acceptors but mitigate the consequences of PSI inhibition. BBA-Bioenerg. 1861:148154. doi: 10.1016/j.bbabio.2020.148154
Sato, R., Kawashima, R., Trinh, M. D. L., Nakano, M., Nagai, T., and Masuda, S. (2019). Significance of PGR5-dependent cyclic electron flow for optimizing the rate of ATP synthesis and consumption in Arabidopsis chloroplasts. Photosynth. Res. 139, 359–365. doi: 10.1007/s11120-018-0533-9
Shi, Q., Pang, J., Yong, J. W. H., Bai, C., Pereira, C. G., Song, Q., et al. (2019). Phosphorus-fertilisation has differential effects on leaf growth and photosynthetic capacity of Arachis hypogaea L. Plant Soil 447, 99–116. doi: 10.1007/s11104-019-04041-w
Shikanai, T. (2007). Cyclic electron transport around photosystem I: genetic approaches. Annu. Rev. Plant Biol. 58, 199–217. doi: 10.1146/annurev.arplant.58.091406.110525
Shikanai, T. (2014). Central role of cyclic electron transport around photosystem I in the regulation of photosynthesis. Curr. Opin. Biotechnol. 26, 25–30. doi: 10.1016/j.copbio.2013.08.012
Shikanai, T. (2016). Regulatory network of proton motive force: contribution of cyclic electron transport around photosystem I. Photosynth. Res. 129, 253–260. doi: 10.1007/s11120-016-0227-0
Song, Q. B., Liu, Y. F., Pang, J. Y., Yong, J. W. H., Chen, Y. L., Bai, C. M., et al. (2020). Supplementary calcium restores peanut (Arachis hypogaea) growth and photosynthetic capacity under low nocturnal temperature. Front. Plant Sci. 10:01637. doi: 10.3389/fpls.2019.01637
Sonoike, K. (2011). Photoinhibition of photosystem I. Physiol. Plant. 142, 56–64. doi: 10.1111/j.1399-3054.2010.01437.x
Storti, M., Alboresi, A., Gerotto, C., Aro, E. M., Finazzi, G., and Morosinotto, T. (2019). Role of cyclic and pseudo-cyclic electron transport in response to dynamic light changes in Physcomitrella patens. Plant Cell Environ. 42, 1590–1602. doi: 10.1111/pce.13493
Strand, D. D., Fisher, N., Davis, G. A., and Kramer, D. M. (2016). Redox regulation of the antimycin A sensitive pathway of cyclic electron flow around photosystem I in higher plant thylakoids. Biochim. Biophys. Acta. Bioenerg. 1857, 1–6. doi: 10.1016/j.bbabio.2015.07.012
Sugimoto, K., Okegawa, Y., Tohri, A., Long, T. A., Covert, S. F., Hisabori, T., et al. (2013). A single amino acid alteration in PGR5 confers resistance to antimycin A in cyclic electron transport around PSI. Plant Cell Physiol. 54, 1525–1534. doi: 10.1093/pcp/pct098
Sun, Y., Geng, Q., Du, Y., Yang, X., and Zhail, H. (2017). Induction of cyclic electron flow around photosystem I during heat stress in grape leaves. Plant Sci. 256, 65–71. doi: 10.1016/j.plantsci.2016.12.004
Suorsa, M., Järvi, S., Grieco, M., and Nurmi, M. (2012). PROTON GRADIENT REGULATION5 is essential for proper acclimation of Arabidopsis photosystem I to naturally and artificially fluctuating light conditions. Plant Cell 24, 2934–2948. doi: 10.2307/23264750
Suorsa, M., Rossi, F., Tadini, L., Labs, M., Colombo, M., Jahns, P., et al. (2016). PGR5-PGRL1-dependent cyclic electron transport modulates linear electron transport rate in Arabidopsis thaliana. Mol. Plant 9, 271–288. doi: 10.1016/j.molp.2015.12.001
Suzuki, N., Riveri, R. M., Shulaev, V., Blumvald, E., and Mittler, R. (2014). Abiotic and biotic stress combinations. New Phytol. 203, 32–43. doi: 10.1111/nph.12797
Taira, Y., Okegawa, Y., Sugimoto, K., Abe, M., Miyoshi, H., and Shikanai, T. (2013). Antimycin A-like molecules inhibit cyclic electron transport around photosystem I in ruptured chloroplasts. FEBS Open Bio. 3, 406–410. doi: 10.1016/j.fob.2013.09.007
Takahashi, H., Clowez, S., Wollman, F. A., Vallon, O., and Rappaport, F. (2013). Cyclic electron flow is redox-controlled but independent of state transition. Nat. Commun. 4:1954. doi: 10.1038/ncomms2954
Takahashi, S., Milward, S. E., Fan, D. Y., Chow, W. S., and Badger, M. R. (2009). How does cyclic electron flow alleviate photoinhibition in Arabidopsis? Plant Physiol. 149, 1560–1567. doi: 10.1104/pp.108.134122
Tikkanen, M., Grieco, M., Kangasjärvi, S., and Aro, E. M. (2010). Thylakoid protein phosphorylation in higher plant chloroplasts optimizes electron transfer under fluctuating light. Plant Physiol. 152, 723–735. doi: 10.1104/pp.109.150250
Tikkanen, M., Mekala, N. R., and Aro, E. M. (2014). Photosystem II photoinhibition-repair cycle protects photosystem I from irreversible damage. Biochim. Biophys. Acta. Bioenerg. 1837, 210–215. doi: 10.1016/j.bbabio.2013.10.001
Ueda, M., Kuniyoshi, T., Yamamoto, H., Sugimoto, K., Ishizaki, K., Kohchi, T., et al. (2012). Composition and physiological function of the chloroplast NADH dehydrogenase-like complex in Marchantia polymorpha. Plant J. 72, 683–693. doi: 10.1111/j.1365-313x.2012.05115.x
Vass, I., Cser, K., and Cheregi, O. (2007). Molecular mechanisms of light stress of photosynthesis. Ann. N. Y. Acad. Sci. 1113, 114–122. doi: 10.1196/annals.1391.017
Wada, S., Takagi, D., Miyake, C., Makino, A., and Suzuki, Y. (2019). Responses of the photosynthetic electron transport reactions stimulate the oxidation of the reaction center chlorophyll of photosystem I, P700, under drought and high temperatures in rice. Int. J. Mol. Sci. 20:2068. doi: 10.3390/ijms20092068
Walker, B. J., Strand, D. D., Kramer, D. M., and Cousins, A. B. (2014). The response of cyclic electron flow around photosystem I to changes in photorespiration and nitrate assimilation. Plant Physiol. 165, 453–462. doi: 10.1104/pp.114.238238
Wang, P., Duan, W., Takabayashi, A., Endo, T., and Mi, H. (2006). Chloroplastic NAD(P)H dehydrogenase in tobacco leaves functions in alleviation of oxidative damage caused by temperature stress. Plant Physiol. 141, 465–474. doi: 10.1104/pp.105.070490
Wang, Y., He, X., Ma, W., Zhao, X., Li, B., and Tong, Y. (2014). Wheat PROTON GRADIENT REGULATION 5 is involved in tolerance to photoinhibition. J. Integr. Agric. 13, 1206–1215. doi: 10.1016/S2095-3119(13)60604-8
Wang, C., Yamamoto, H., and Shikanai, T. (2015). Role of cyclic electron transport around photosystem I in regulating proton motive force. Biochim. Biophys. Acta. Bioenerg. 1847, 931–938. doi: 10.1016/j.bbabio.2014.11.013
Wang, F., Yan, J., Ahammed, G. J., Wang, X., Bu, X., Xiang, H., et al. (2020). PGR5/PGRL1 and NDH mediate far-red light-induced photoprotection in response to chilling stress in tomato. Front. Plant Sci. 11:669. doi: 10.3389/fpls.2020.00669
Wolf, B., Isaacson, T., Tiwari, V., Dangoor, I., Mufkadi, S., and Danon, A. (2020). Redox regulation of pgrl1 at the onset of low light intensity. Plant J. 103, 715–725. doi: 10.1111/tpj.14764
Wu, D., Liu, Y., Pang, J., Yong, J. W. H., Chen, Y., Bai, C., et al. (2020). Exogenous calcium alleviates nocturnal chilling-induced feedback inhibition of photosynthesis by improving sink demand in peanut (Arachis hypogaea). Front. Plant Sci. 11:607029. doi: 10.3389/fpls.2020.607029
Xue, X., Xu, H. M., Wu, H. Y., Shen, Y. B., Xiao, J. W., and Wan, Y. L. (2017). Research progress of cyclic electron transport in plant photosynthesis. Plant Physiol. J. 53, 145–158. doi: 10.13592/j.cnki.ppj.2016.0432
Yadav, R. M., Aslam, S. M., Madireddi, S. K., Chouhan, N., and Subramanyam, R. (2020). Role of cyclic electron transport mutations pgrl1 and pgr5 in acclimation process to high light in Chlamydomonas reinhardtii. Photosynth. Res. 146, 247–258. doi: 10.1007/s11120-020-00751-w
Yamamoto, H., and Shikanai, T. (2019). PGR5-dependent cyclic electron flow protects photosystem I under fluctuating light at donor and acceptor sides. Plant Physiol. 179, 588–600. doi: 10.1104/pp.18.01343
Yamamoto, H., Takahashi, S., Badger, M. R., and Shikanai, T. (2016). Artificial remodelling of alternative electron flow by flavodiiron proteins in Arabidopsis. Nat. Plants. 2:16012. doi: 10.1038/nplants.2016.12
Yamori, W., Makino, A., and Shikanai, T. (2016). A physiological role of cyclic electron transport around photosystem I in sustaining photosynthesis under fluctuating light in rice. Sci. Rep. 6:20147. doi: 10.1038/srep20147
Yamori, W., and Shikanai, T. (2016). Physiological functions of cyclic electron transport around photosystem I in sustaining photosynthesis and plant growth. Annu. Rev. Plant Biol. 67, 81–106. doi: 10.1146/annurev-arplant-043015-112002
Yamori, W., Shikanai, T., and Makino, A. (2015). Photosystem I cyclic electron flow via chloroplast NADH dehydrogenase-like complex performs a physiological role for photosynthesis at low light. Sci. Rep. 5:13908. doi: 10.1038/srep13908
Zhou, L., Gao, S., Wu, S., Han, D., Wang, H., Gu, W., et al. (2020). PGRL1 overexpression in Phaeodactylum tricornutum inhibits growth and reduces apparent PSII activity. Plant J. 103, 1850–1857. doi: 10.1111/tpj.14872
Keywords: photosynthesis, cyclic electron transport, proton gradient regulation 5, PRG5-like photosynthetic phenotype 1, photoinhibition
Citation: Ma M, Liu Y, Bai C, Yang Y, Sun Z, Liu X, Zhang S, Han X and Yong JWH (2021) The Physiological Functionality of PGR5/PGRL1-Dependent Cyclic Electron Transport in Sustaining Photosynthesis. Front. Plant Sci. 12:702196. doi: 10.3389/fpls.2021.702196
Edited by:
Rebecca L. Roston, University of Nebraska-Lincoln, United StatesReviewed by:
Hualing Mi, Chinese Academy of Sciences, ChinaToshiharu Shikanai, Kyoto University, Japan
Copyright © 2021 Ma, Liu, Bai, Yang, Sun, Liu, Zhang, Han and Yong. This is an open-access article distributed under the terms of the Creative Commons Attribution License (CC BY). The use, distribution or reproduction in other forums is permitted, provided the original author(s) and the copyright owner(s) are credited and that the original publication in this journal is cited, in accordance with accepted academic practice. No use, distribution or reproduction is permitted which does not comply with these terms.
*Correspondence: Yifei Liu, eWlmZWlsaXU2QGhvdG1haWwuY29t; Xiaori Han, aGFueGlhb3JpQDE2My5jb20=