- 1College of Food Science and Engineering, Gansu Agricultural University, Lanzhou, China
- 2Department of Postharvest Science of Fresh Produce, Agricultural Research Organization, Volcani Center, Rishon LeZion, Israel
The occurrence of reactive oxygen species (ROS) during the colonization of necrotrophic pathogens attacking fruit is critical during the attack, but its importance in Penicillium expansum remains unclear. This study aimed to determine the regulatory effects of NADPH oxidase (Nox) genes on the growth and pathogenicity of P. expansum in apple fruits. Deletion mutants of ΔPeNoxA, ΔPeNoxR, and ΔPeRacA genes were constructed to determine the contribution to the colonization process. The ΔPeRacA strain had a significant effect on the reduction of growth and pathogenicity, the ΔPeNoxA strain negatively regulated the growth and development of P. expansum and did not show any significant effect on the pathogenicity, and the ΔPeNoxR strain showed no effect on the growth or pathogenicity of P. expansum in the apple fruits. However, analysis of the content of O2– and H2O2 in the mycelium of all the Nox mutants showed a significant reduction, confirming the functionality of Nox mutations. Growth under stress conditions in the presence of Congo red, sodium lauryl sulfate, and H2O2 showed a negative effect on the radial growth of ΔPeNoxA, but a positive effect on radial growth reduction by ΔPeNoxR and ΔPeRacA mutants was shown. Interestingly, the host antioxidant activity levels of superoxide dismutase (SOD) andcatalase (CAT) in the fruits after inoculation with ΔPeNoxA, ΔPeNoxR, and ΔPeRacA mutants declined, suggesting reduced ROS accumulation in the colonized region. These results suggest that PeNoxA, PeNoxR, and PeRacA differentially regulate the growth and pathogenicity of P. expansum by producing ROS, and that PeRacA showed the strongest regulatory effect.
Introduction
Penicillium expansum is a necrotrophic pathogen that infects temperate fruits, such as pomes, stones, and berries, through wounds during harvesting and storage (Sun et al., 2018). Evidence of the ability of P. expansum to germinate and temporarily grow in a host tissue producing high levels of H2O2 suggested their role in signaling molecule for the induction of fruit defense (Hadas et al., 2007). Upon initial colonization of a non-host fruit by P. expansum, the host releases a large amount of reactive oxygen species (ROS) at the infection site, inhibiting fungal colonization. However, the addition of exogenous catalase (an H2O2-scavenging enzyme) led to reduced ROS production in the host and enhanced the successful colonization of P. expansum on non-host citrus fruits (Macarisin et al., 2007). Studies on the mechanism underlying the sensitivity of P. Expansum to intracellular accumulation of P. expansum indicate that under high H2O2-induced oxidative stress, intracellular ROS production by the host is mainly located in the mitochondria. Overexposure of host cells to ROS causes impairments in DNA, lipids, and protein, eventually leading to cell death and progressive aging of an organism (Levine et al., 2000; Xu and Tian, 2008). In order to maintain a stable level of ROS, many organisms have evolved ROS scavenging systems that are mainly enzymatic or non-enzymatic. The enzymatic scavenging system includes superoxide dismutase (SOD) and catalase (CAT), ascorbate peroxidase (APX), etc. SOD causes superoxide conversion into H2O2, whereas CAT converts H2O2 into H2O. ROS scavenging systems are essential for maintaining ROS levels both in hosts and in pathogens (Wang et al., 2019).
Pathogenic fungi produce ROS by the catalysis of NADPH oxidase (Nox) and play an essential role in their infection processes, signal transduction, and pathogenicity (Tudzynski et al., 2012). In these cases, proper fungal antioxidative systems are expressed in the pathogens to enable fungal development (Lin et al., 2017). Three Nox catalytic subunits, namely NoxA, NoxB, and NoxC, have been found in filamentous fungi, such as Botrytis cinerea, Neurospora crassa, and Magnaporthe oryzae (Kim, 2014). NoxR is a regulatory factor of the Nox catalytic subunit; its coding structure is similar to that of the p67phox protein in mammals (Takemoto et al., 2006). Other Nox regulatory subunits such as Rho3, Cdc42, and RacA also play regulatory roles in fungi (Minz et al., 2013; Si et al., 2016). In the Nox family, only NoxA has a catalytic core domain. The regulatory subunit NoxR and the small GTPases RacA are necessary to activate fungal NoxA function and sometimes act alone (Li et al., 2019).
Studies have reported that NoxA in Aspergillus nidulans and Podospora anserina had no effect on their asexual development but the affected ROS production and sporophore formation of their hyphae (Lara-Ortíz et al., 2003; Malagnac et al., 2004). In B. cinerea, NoxR is responsible for activating the function of NoxA. NoxR deletion was shown to severely affect the sexual and asexual development and the sensitivity oxidative stress of B. cinerea (Segmüller et al., 2008). The absence of RacA in Epichloë festucae increased hyphal branching, altered the growth of the hyphal tip, and decreased the ROS content. Furthermore, NoxR could activate NoxA synergistically with RacA (Scott et al., 2007). In Aspergillus niger, RacA governs polarity maintenance by controlling actin but not microtubule dynamics, which is consistent with its localization at the hyphal apex. The deletion of RacA caused an actin localization defect, leading to the loss in the polarization tip extension of hyphae. Moreover, NoxR is a specific effector of RacA, which plays a critical role in the asexual development of the pathogen (Kwon et al., 2011).
A report has illustrated the effects of Nox on the asexual and sexual development of filamentous fungi (Tudzynski et al., 2012). However, for P. expansum, no reports have described the regulatory effects of the Nox family. Using gene knockout and complementation methods, we analyzed the ROS contents and stress resistance of NoxA, NoxR, and RacA on the growth and pathogenicity of P. expansum. By studying the production of mutant ROS as well as their growth, morphology and pathogenicity, we determined the effect of PeNoxA, PeNoxR, and PeRacA deletion on the colonization patterns of P. expansum.
Materials and Methods
Fungal Strain and Growth Condition
Penicillium expansum (T01) was obtained from the Institute of Botany, the Chinese Academy of Sciences. A spore suspension was prepared according to Kumar et al. (2017), with minor modifications. Cultures were grown at 25°C in the dark, and maintained on potato dextrose agar (PDA) plates (Beijing Soleibao Technology Co., LTD, China). Then, 10 ml of sterile distilled water supplemented with 0.05% (v/v) Tween 80 (Solarbio, China) by removing the conidia from 7-day-old PDA. A hemocytometer was used to determine the concentrations of the spores. Single-spore cultures were obtained and stored at –80°C until use.
DNA Extraction
DNA from the WT strain grown in a CY liquid medium (3 g/L NaNO3, 1 g/L K2HPO4⋅3H2O, 0.5 g/L KCl, 0.5 g/L MgSO4⋅7H2O, 0.01 g/L FeSO4⋅7H2O, 30 g/L sucrose, 5 g/L yeast extract, pH = 5.2) in the dark at 25°C for 48 h and hyphae were collected and stored at –80°C for DNA extraction. According to the instructions of the manufacturer, The DNA was prepared with Fungal DNA Extraction Kit (No. D3195, OMEGA, Guangzhou Feiyang Biological Engineering Co., LTD, China) as described by Yang et al. (2014). The extracted DNA was analyzed by gel electrophoresis and then stored at –20°C until use.
Gene Knockout and Complementation
Based on the gene sequences of PeNoxA (ID:27678081), PeNoxR (ID:27678342), and PeRacA (ID:27674691) of P. expansum T01 in the NCBI database,1 knockout mutants of PeNoxA, PeNoxR, and PeRacA were generated using a homologous recombination strategy and by Agrobacterium tumefaciens-mediated transformation, as described by Li et al. (2015). The primers used for the amplification of the up-and downstream sequences are listed in Supplementary Table 1, and the results are shown in Supplementary Figure 1. Gene knockout vectors were constructed by inserting the homologous recombination sequences (approximately 1 k beach), flanking the target genes into the upstream and downstream sides of the hygromycin resistance gene in the vector pCHPH. The homologous recombination knockout strategy is shown in Figure 1. The vectors were transformed into A. tumefaciens by freezing-thawing method. During transformation, hygromycin B (Beijing Soleibao Technology Co., LTD, China) was used to select transformants that have undergone resistance screening collection of hyphae and extraction of genomic DNA. Mutants were identified by the difference in the size of amplified fragments between them and the wild type (WT), and the results are shown in Supplementary Figure 2. For the construction of complementation vectors, DNA fragments of PeNoxA, PeNoxR, and PeRacA, namely, the full genomic sequence of the genes and the promoter and terminator regions were cloned into pCNEO-N. G418 (GIBCO, Wolcavi (Beijing) Biological Technology co., LTD, China) was used to select the transformants. The primer sequences used to construct the complementation vectors are shown in Supplementary Table 2.
Phenotypic Analysis
Colony growth was determined according to the method of Zong et al. (2015), with minor modifications. PDA plates were inoculated with 3 μl of 1 × 106 conidia/ml of either the WT, mutants or complementation strains and incubated at 25°C in the dark. Growth was determined by measuring the diameter after 7 days. Average surface (cm2) was used to express colony growth. Each strain had three replicates, and nine plates were used for each replicate.
The rate of spore germination and the length of germ tubes were determined based on the method of Long et al. (2017), with minor modifications. Briefly, 3 μl of each conidial suspension (1 × 106 conidia/ml) was inoculated on PDA plates and incubated at 25°C for 9, 10, and 11 h in the dark. The rate of spore germination and the length of germ tubes of each strain were recorded at different time periods with a microscope (Olympus Corporation, Tokyo, Japan). Each strain had three replicates, and nine plates were used for each replicate.
According to the instructions of the manufacturer, the O2– and H2O2 contents were measured with a kit from Suzhou Comin Biotechnology (Suzhou, China) as described by Zhang et al. (2020). The spore suspension of either the WT, mutants, or complementation strains (3 μl, 1 × 106 conidia/ml) was inoculated on PDA plates, cultured for 24 h, and transferred to a CY medium for 48 h. Then, hyphae were collected to determine the contents of O2– and H2O2. All determination was done at least three times.
Stress resistance was assayed based on Siwy et al. (2016), with minor modifications. Congo red (CR) 25 mg/L, 0.02% (w/v) sodium dodecyl sulfate (SDS), and 100 mM H2O2 were added to the PDA plates. Each plate was inoculated with 3 μl of each conidial suspension (1 × 106 conidia/ml) and cultured at 25°C in the dark. The morphology of colony growth was recorded, and radial growth was measured after 7 days. Average surface (cm2) was used to express colony growth. Each strain had three replicates, and nine plates were used for each replicate.
Spore production was determined according to the method of Zhou et al. (2018), with minor modifications. PDA plates containing 1 × 106 conidia/ml of each strain were incubated at 25°C in the dark for 7 days, and then 5 ml of sterile water (containing 0.05% Tween-20) was added to each plate. Conidia were visualized with a microscope (Olympus Corporation, Tokyo, Japan) and counted with a hemocytometer. Each strain had three replicates, and nine plates were used for each replicate.
Pathogenicity Experiments
Pathogenicity was determined based on the method of Levin et al. (2019), with minor modifications. The spore suspension (10 μl, 1 × 105 conidia/ml) was inoculated at the wound of the apple fruits (cv. Fuji) using Nichipet EX (Nichiryo, Nagaoka, Japan). The inoculated fruits were packed in polyethylene bags and then stored under ambient conditions (25 ± 2°C, RH 80–85%) in darkness. We evaluated the decayed fruits after 7 days. Colonized area (cm2) was used to express wound surface. Each strain had three replicates, and nine fruits were used for each replicate.
According to the instructions of the manufacturer, SOD and CAT activities were measured with the kit from Beijing Solarbio Science and Technology (Solarbio, China) as described by Zhang et al. (2020). The configured spore suspension (10 μl, 1 × 105 conidia/ml) was inoculated at the wound of the apple fruits (cv. Fuji). The inoculated fruits were packed in polyethylene bags and then stored under ambient condition (25 ± 2°C, RH 80–85%) in darkness. Disease-health junction tissues of the apple fruits were collected after 7 days. The SOD activity in the fruits was measured with the SOD kit from Beijing Solarbio Science and Technology. The CAT activity in the fruits was measured with the CAT kit from Beijing Solarbio Science and Technology. All determination was done at least three times.
Statistical Analysis
All the experiments were repeated at least three times, and the average and standard error (±SE) of the data were calculated using Microsoft Excel 2010. The significance analysis of Duncan’s multiple differences was performed using SPSS 19.0 (SPSS Inc., Chicago, IL, United States) (P < 0.05).
Results
Effect of PeNoxA, PeNoxR, and PeRacA Knockout on Colony Development and Morphology of the Mutants
Average surface and colony morphology of ΔPeNoxR and their complementation strains indicated that they were similar to those of the WT strain (Figure 2). ΔPeNoxA showed a 12% increase compared with the WT, but the surface of complementation strain was similar to that of the WT. However, the average surface of the ΔPeRacA mutant was inhibited by 34% with that of WT, but the average surface was regained in the ΔPeRacA-C strain (Figure 2A). In terms of colony morphology, the ΔPeNoxA and ΔPeNoxR mutants did not differ from the WT. The morphology of ΔPeRacA showed irregular edges. In terms of appearance, the edges are slightly wrinkled but the morphology was recovered in the ΔPeRacA-C (Figure 2B). These results indicated that the PeNoxA gene showed a negative regulatory effect on P. expansum in surface and colony morphology, while the ΔPeRacA strain showed significant inhibition in surface and colony morphology.
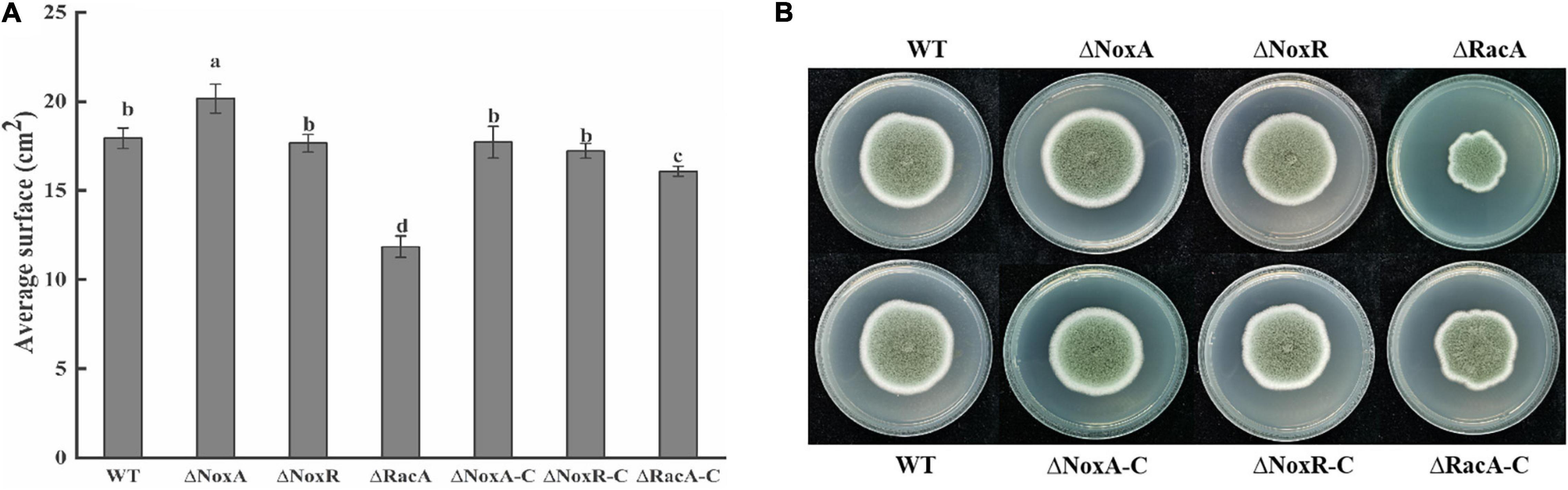
Figure 2. Average surface and morphology of wild type (WT), mutants, and complementation strains. The spore suspension (3 μl) was inoculated on potato dextrose agar (PDA) plates, and cultured at 25°C in the dark. The average surface and morphology were evaluated after 7 days. (A) Average surface of WT, mutants, and complementation strains. (B) Morphology of WT, mutants, and complementation strains. Bars indicate standard error. Different letters indicate significant differences (P < 0.05).
Effect of PeNoxA, PeNoxR, and PeRacA Knockout on Germ Tube Elongation of the Mutants
The rate of germination in the ΔPeNoxA and ΔPeNoxR was similar to that in the WT and complementation strains, with minor changes (Figure 3). However, a delay in germination of 20% was observed in the ΔPeRacA mutant when compared with the WT and the complementation strain (Figure 3). Also, the length of the germinated tube of the ΔPeRacA mutant was inhibited by 61% at 11 h after the initiation of germination, while the complementation strain showed a reduction in inhibition that is not similar to that of the WT strain. The above results also suggested that the spore germination in P. expansum was affected in the ΔPeRacA mutant.
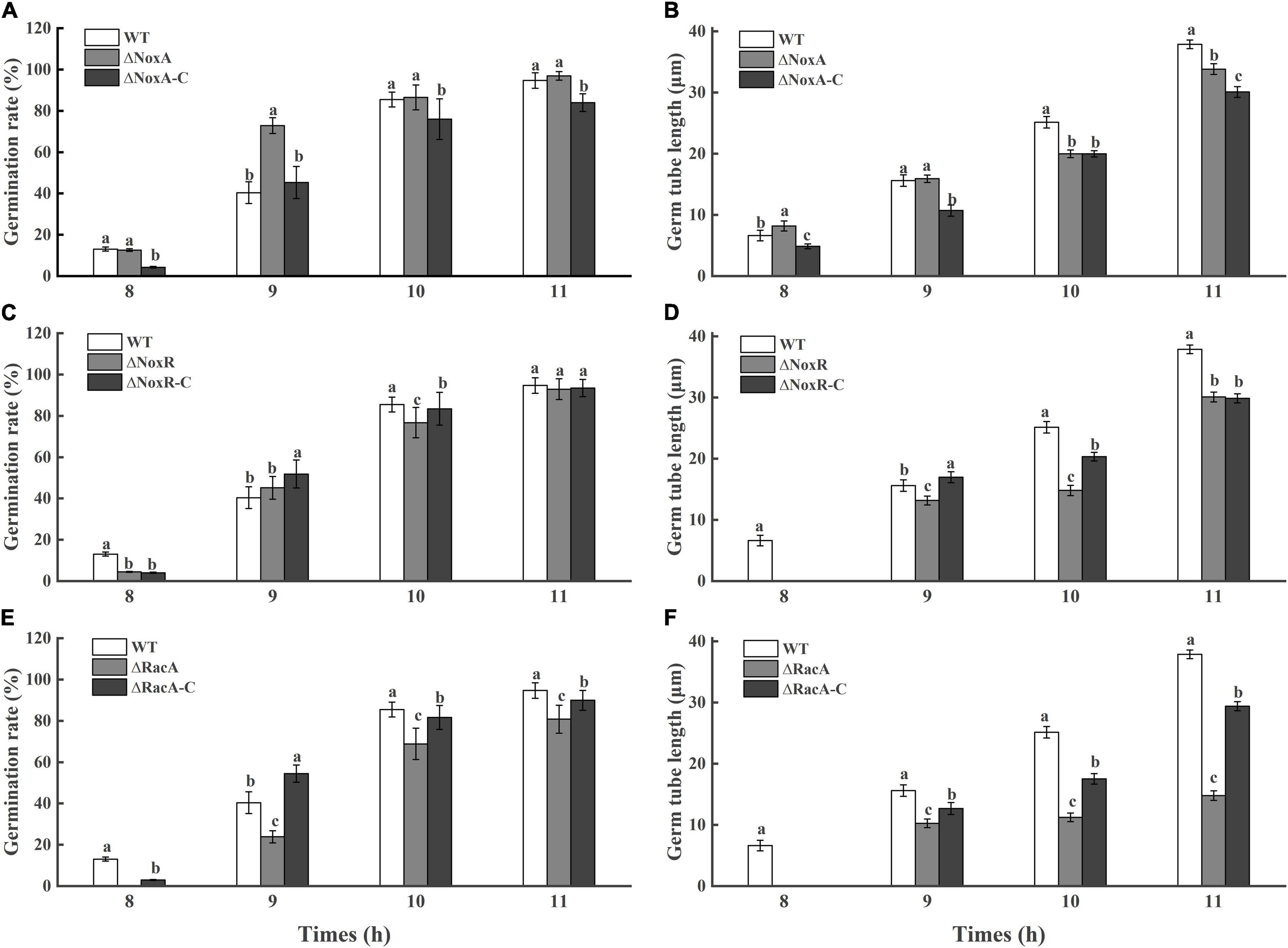
Figure 3. Germination rate and germ tube length of wild type (WT), mutants, and complementation strains. The spore suspension (3 μl) was inoculated on potato dextrose agar (PDA) plates, and cultured for 8, 9, 10, and 11 h at 25°C in the dark. The spore germination and germ tube length were observed under a microscope. Bars indicate standard error. Different letters indicate significant differences (P < 0.05). (A,C,E): Germination rate of WT, mutants, and complementation strains. (B,D,F): Germ tube length of WT, mutants, and complementation strains.
O2– and H2O2 Production in the Nox Mutants
Analysis of the O2– content in the ΔPeNoxA, ΔPeNoxR, and ΔPeRacA mutants were significantly reduced by 36, 17, and 41%, respectively, when compared with that produced by the WT (Figure 4A). Similarly, the content of H2O2 in the ΔPeNoxA and ΔPeRacA mutants were reduced by 56 and 17% compared with that in the WT (Figure 4B). ΔPeNoxR was similar to the WT and was recovered in ΔNoxR-C. The results indicated that PeNoxA, PeNoxR, and PeRacA genes were involved in the production of ROS in P. expansum.
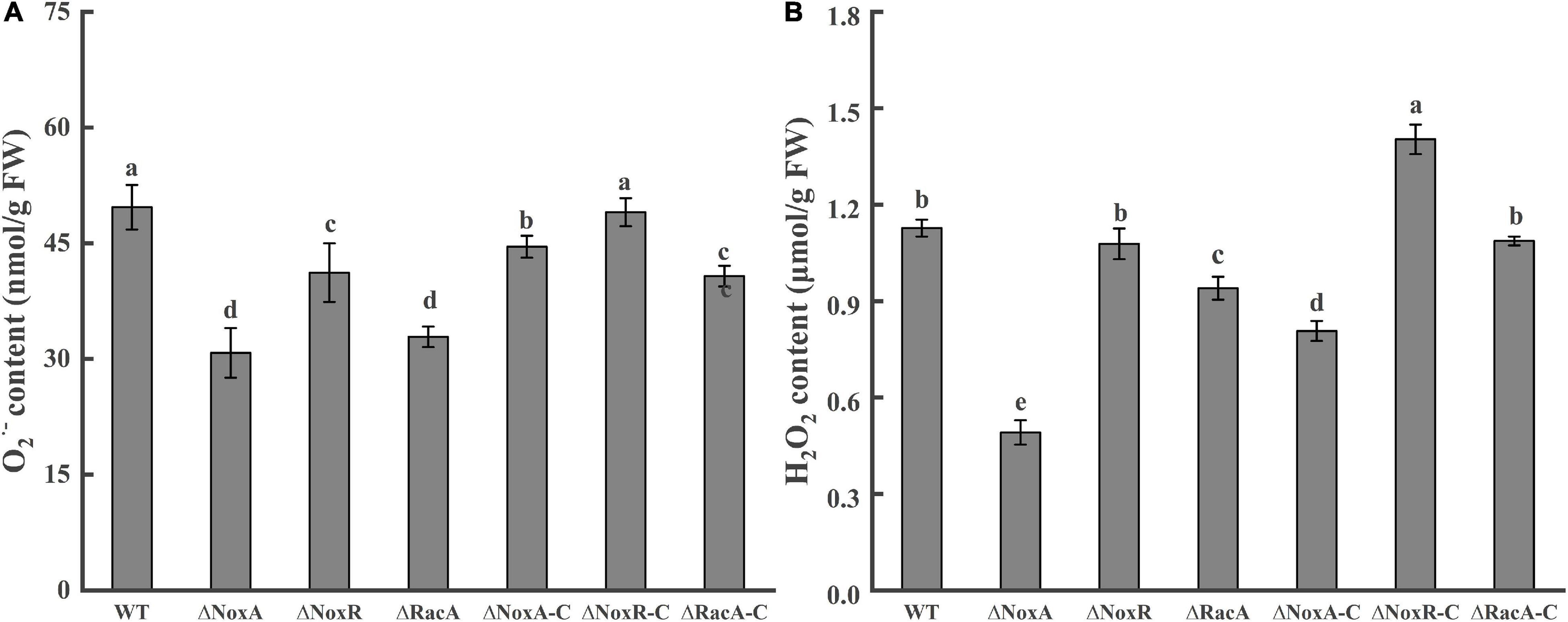
Figure 4. Contents of O2– and H2O2 in the Nox mutants and complementation strains. The spore suspension (3 μl) was inoculated on potato dextrose agar (PDA) plates, cultured for 24 h, and transferred to a CY medium for 48 h. Then, the hyphae were collected to determine the contents of O2– and H2O2. Bars indicate standard error. Different letters indicate significant differences (P < 0.05). (A) Content of O2– of WT, mutants, and complementation strains. (B) Content of H2O2 of WT, mutants, and complementation strains.
Oxygen Stress Responses by the Nox Mutants Detected When Grown With Congo Red, Sodium Lauryl Sulfate, and H2O2
The average surface of ΔPeNoxA mutants in the presence of SDS was increased by 25% compared with that of the WT, while in the presence of CR and H2O2 it did not differ from that of the WT. The average surface of the ΔPeNoxR and ΔPeRacA mutants in the presence of SDS was inhibited by almost 28 and 39%, respectively. Also, the average surface of the ΔPeRacA strain in the presence of CR and H2O2 was inhibited by 58 and 78% compared with the WT (Figure 5). The average surface of the complementation stains reverted, in most cases, the effect of the stresses on their fungal growth (results not shown). The colony morphology of the ΔPeNoxA and ΔPeNoxA-C strains were not different compared with the WT, while the ΔPeRacA mutant was wrinkled with irregular colony edge depressions that become flat in the colony edge of the ΔPeRacA-C strain. ΔPeNoxR and ΔPeNoxR-C showed irregular edges when grown with SDS (Figure 5B). These results indicated that the PeRacA gene showed the highest sensitivity to cell wall integrity stress and oxidative stress, followed by the PeNoxR and PeNoxA genes.
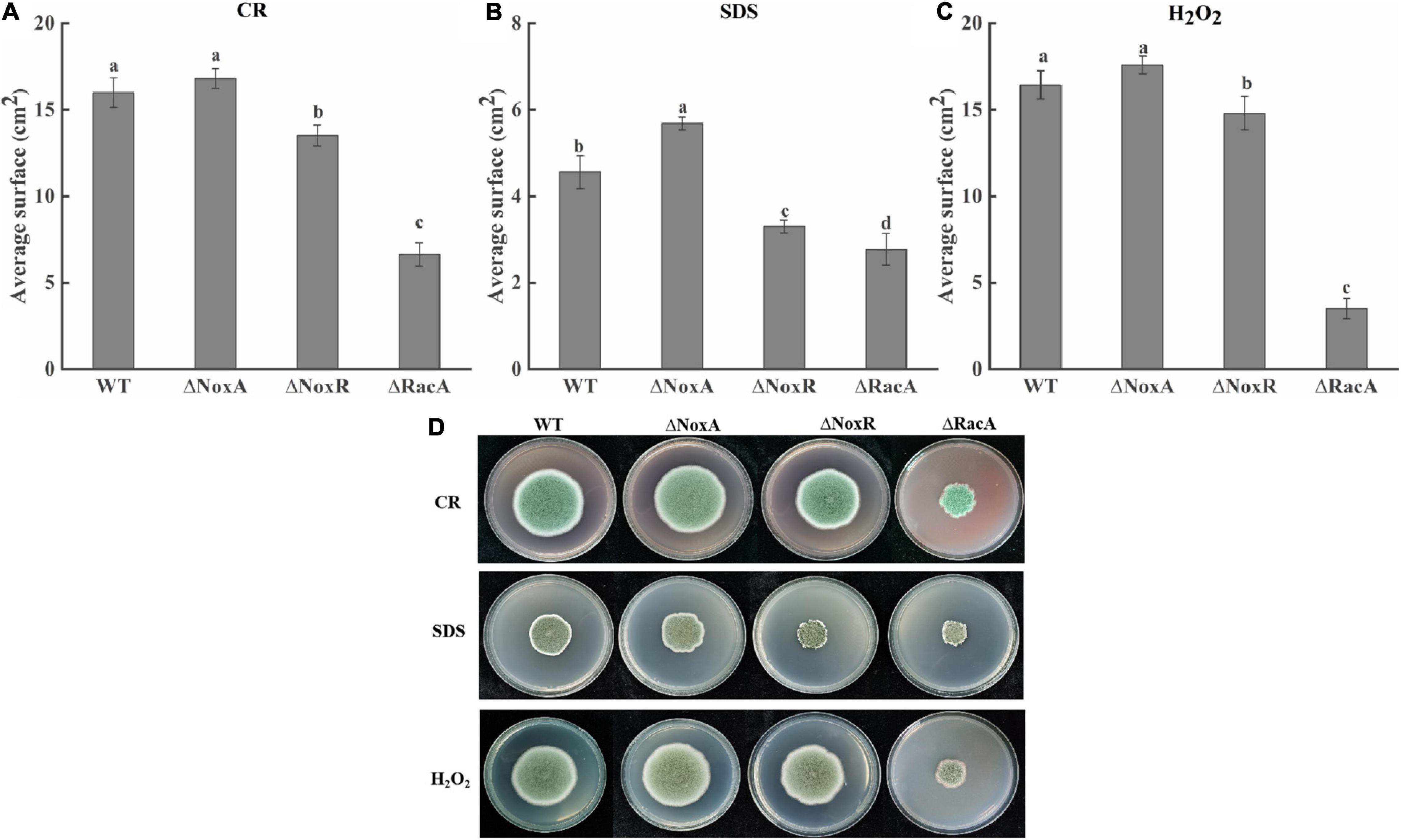
Figure 5. Effect of Congo red (CR), sodium dodecyl sulfate (SDS), and H2O2 on the colony diameter and colony morphology of wild type (WT) and mutants. CR (25 mg/l), SDS (0.02%), and H2O2 (100 mM) were added to the potato dextrose agar (PDA) medium. The spore suspension (3 μl) of P. expansum was inoculated and cultured at 25°C in the dark for 7 days, and the colony morphology and the average surface was recorded. (A) Growth of mutants in the presence of CR. (B) Growth of mutants in the presence of SDS. (C) Growth of mutants in the presence of H2O2. Bars indicate standard error. Different letters indicate significant differences (P < 0.05). (D) The colony morphology of WT and mutants.
Effect of PeNoxA, PeNoxR, and PeRacA Knockout on Sporulation and Pathogenicity of the Mutants
The level of sporulation of the ΔPeNoxA and ΔPeNoxR mutants increased by 60 and 30%, respectively, compared with that of the WT, while the sporulation of the ΔPeRacA mutant was inhibited by 30%. The sporulation of ΔPeRacA-C showed minor differences with the WT strain (Figure 6A). These results indicated that the PeNoxA and PeNoxR genes showed a negative regulation of the sporulation of P. expansum, while the deletion of the PeRacA gene enhanced a significant inhibition on sporulation. The colonized area of the ΔPeNoxA and ΔPeNoxR mutants showed an area similar to that of the WT (Figure 6C), while that of the colonized area of ΔPeRacA was inhibited by 43% compared the WT and the ΔPeRacA-C strain (Figure 6B). These results indicate that the ΔPeRacA mutant is the only one showing a significant contribution to pathogenicity, in comparison with ΔPeNoxA and ΔPeNoxR.
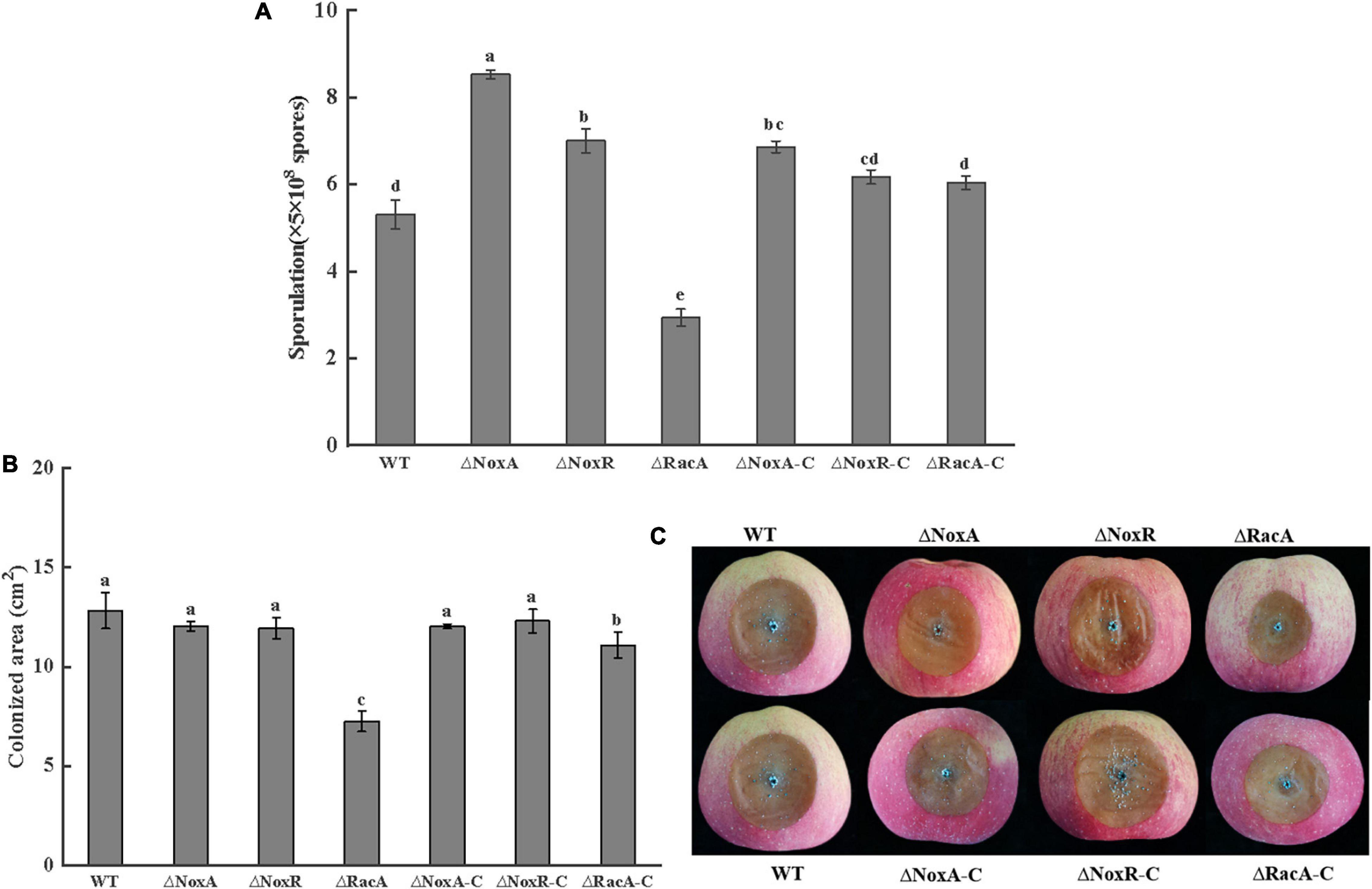
Figure 6. Sporulation and pathogenicity of wild type (WT), mutants, and complementation strains. The spore suspension (3 μl) was inoculated on potato dextrose agar (PDA) plates and cultured for 7 days at 25°C in the dark. The sporulation was recovered by adding sterile distilled water using a bacteriological loop. (A) Sporulation of WT, mutants, and complementation strains. (B) Colonized area of WT, mutants, and complementation strains. Bars indicate standard error. Different letters indicate significant differences (P < 0.05). (C) The disease spot form of WT, mutants, and complementation strains.
Activities of SOD and CAT in Disease-Health Junction Tissues of Apple Fruits of Mutants
The colonization of the Nox mutants raised the question if the level of antioxidant activities of the host fruit will be affected by the colonization of the Nox mutant. Evaluation SOD activity responses in the leading edge of the colonized tissue showed that the ΔPeNoxA, ΔPeNoxR, and ΔPeRacA mutants strongly reduced the SOD activity by 36, 60, and 53%, respectively (Figure 7A). A similar pattern was observed when the CAT activity was evaluated at the leading edge of the colonized tissue by the ΔPeNoxA, ΔPeNoxR, and ΔPeRacA mutants showing a reduced CAT activity by 47, 51, and 26%, respectively (Figure 7B). The SOD and CAT activities of the complementation stains reverted in most cases (results not shown). The results indicate that the deletion of the PeNoxA, PeNoxR, and PeRacA genes are modulating the host antioxidant activities as a result of the mutant colonization.
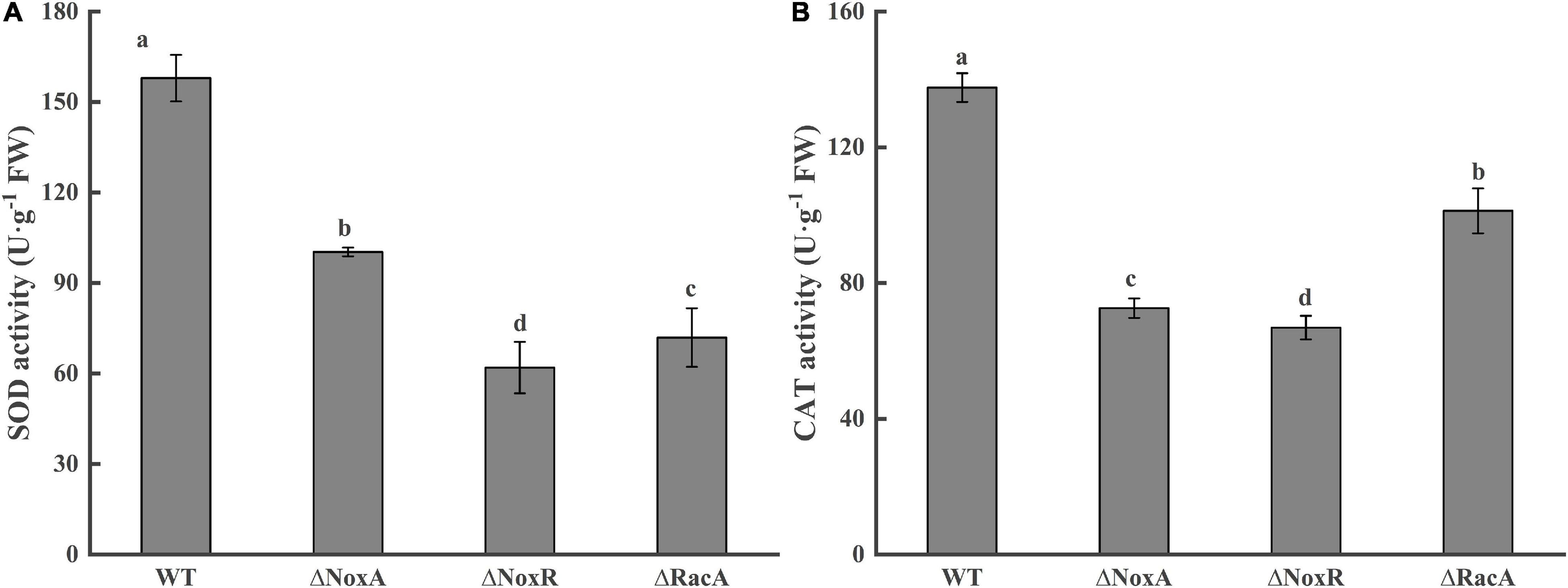
Figure 7. Activities of superoxide dismutase (SOD) and catalase (CAT) at disease-health junction tissues of apple fruits. Bars indicate standard error. Different letters indicate significant differences (P < 0.05). (A) Activitiy of superoxide dismutase (SOD). (B) Activitiy of catalase (CAT).
Discussion
Nox mediates multiple reactions, such as growth of vegetative hyphae, apoptosis, spore fusion, and differentiation of infective structures (Sumimoto, 2008). According to different growth stages, ROS are produced in various intercellular substances, such as peroxidase in vacuoles, and flavin and xanthine oxidase in peroxidase (Susumu et al., 2001; Río et al., 2006). In this study, the colony growth and germination rate of the ΔPeNoxA mutant increased when compared with the WT, while there was no significant change in the ΔPeNoxR mutant. The colony growth and germination of the ΔPeRacA mutant was significantly reduced. Similar results are found in Epichloë festucae, noxA negatively regulates the asexual development, and the deletion of noxR has no effect on growth, while the deletion of RacA shows growth defects (Kayano et al., 2013). In A. nidulans, NoxA is required for sexual development, RacA activates Nox, and NoxR functions in a parallel pathway that regulates Nox localization (Semighini and Harris, 2008). However, in B. cinerea neither NoxA nor NoxB is required for ascospore germination but both are essential for the formation of sclerotia, multicellular sexual structures (Segmüller et al., 2008). These results indicate diverse functions for Nox in different filamentous fungi. Nox catalyzes the ROS production in cells, which can directly act on the cell wall or indirectly act as a second messenger to trigger the hydrolysis and flow of nutrients, thereby weakening nutrients and re-establishing differentiated growth (Malagnac et al., 2004). In A. nidulans, NoxA mainly regulates sexual development. However, it would not affect the asexual development of pathogens, which involved ROS production via the regulation of mitogen activated protein kinases (MAPK) signals (Eaton et al., 2008). Marschall et al. (2016) reported that Nox activity was strictly regulated involving other membrane proteins, such as protein disulfide isomerase (PDI), specific oxidoreductase (ERO1) and scaffold protein (Iqg1), cytoglobin, and different regulatory domains. Therefore, it is hypothesized that NoxA, NoxR and RacA regulated the growth and development in P. expansum. However, whether there is a potential interaction with other regulatory factors requires further research.
Nox is the main enzyme source of ROS in fungi and can use FADH2 and two heme molecules as cofactors. It acts as an electron donor to produce superoxide through electron transfer between membranes. In most cases, oxygen is an electron acceptor, and superoxide is the main product (Rossi et al., 2017). In this study, the contents of O2– and H2O2 in fungi were significantly reduced, indicating that the NoxA, NoxR, and RacA genes affected ROS production in P. expansum. Similar results have been reported when deletion of racA resulted in reduced ROS production in E. festucae (Tanaka et al., 2008). Segal and Wilson (2018) Nox enzymes produce ROS by transferring electrons from NADPH to molecular oxygen to produce superoxide and other ROS. In E. festucae, ROS accumulation was observed in the extracellular matrix of the wild type but not in noxA mutants (Tanaka et al., 2006). These observations suggest that the ROS produced by Nox is dispensable for the establishment of fungi growth. In the process of cell differentiation, Nox catalytic subunits respond to internal or external signals, and RacA and NoxR are transferred to the plasma membrane through electrons to form a multi-enzyme complex with complete membrane catalysis, and convert O2 to O2–. The O2– can be rapidly converted into H2O2 quickly by dismutase and diffuse through the membrane as a second messenger and play their roles on fungal cell walls, plasma membrane receptors, or ion channels to activate internal signaling pathways (Scott and Eaton, 2008). Therefore, it is hypothesized that PeNoxA, PeNoxR, and PeRacA, which are primary ROS sources in P. expansum, are responsible for intracellular signal transduction and activation of metabolic pathways.
Cell differentiation in fungi has multiple manifestations. It is related to various modes of reproduction and differentiation and various forms of resistance to adverse environmental conditions. Various physiological signals and stresses may cause fungal tissues to undertake specific differentiation processes (Georgiou et al., 2006). NoxA, NoxR, and RacA are the core components of the Nox system; however, there are differences among the Nox mutants, and each of them may have unique functions for different environmental stimuli (Marschall and Tudzynski, 2017). Therefore, to study the effects of the NoxA, NoxR, and RacA genes on various stress responses in P. expansum, stress tests were performed. The ΔPeNoxA mutant, with the treatment of SDS, CR, and H2O2, showed an increase in the exogenous stress conditions, whereas the growth of the ΔPeNoxR and ΔPeRacA mutants showed a reduced stress response. Similar results have been reported for the noxA and noxR mutants, which displayed cellular sensitivity to H2O2 and SDS of A. alternata (Yang and Chung, 2013). The regulatory functions of NoxA conferring ROS resistance are modulated partially through the activation of the YAP1- and HOG1 MAP kinase-mediated signaling pathways in A. alternata (Yang and Chung, 2012). Cellular stress transcription factor YAP1 plays a global regulatory role in oxidative stress response. Under oxidative stress, YAP1 enters the nucleus through conformational changes through the formation of disulfide bonds, and activates the expression of Nox (Wood et al., 2003; Lin et al., 2009). The MAPK signaling pathway and Nox complex showed mutual activation effects. The MAPK kinase Hog1 has also been proven to be resistant to high osmotic pressure and to regulate oxidative stress, and the Hog1 gene has shown the flexibility and uniqueness of different signaling pathways in response to different stress (Lin and Chung, 2010). Therefore, it is hypothesized that NADPH oxidase may have a regulatory effect on the integrity of the cell wall and oxidative stress response in P. expansum, but whether it involves specific interactions with other signaling pathways requires further research.
Reactive oxygen species (ROS) play a significant role during host-pathogen communication. The infection process can be categorized broadly as the recognition phase, host-pathogen communication stage, and the final penetration and infection stage. According to different lifestyles and different infection methods, there are also differences in pathogenicity (Marschall and Tudzynski, 2016). The present results indicate that the pathogenicity of the ΔPeNoxA and ΔPeNoxR mutants is similar to that of WT, and that the colonization pattern of ΔPeRacA is significantly reduced. Similar results were obtained by Chen et al. (2008) during the colonization of rice by Magnaporthe grisea. Once the fungal spores are attached to the fruit, they can absorb nutrients from the host for their growth. The chemotropic sensing of nutrients and fungal signals influenced the fungal growth in the fruits (Turrà and Di, 2015). The pathogen counteracts by producing its own ROS to weaken the defense barrier and facilitates the penetration of fruit tissues by specialized infection structures. ROS production plays an important role during infection, and the effects of pathogenicity are often correlated with altered ROS production (Wang et al., 2019). Tanaka et al. (2006) reported that plants infected with the noxA mutant lose apical dominance and eventually die in a fungus-perennial Rye grass mutualistic interaction. Chen et al. (2008) also observed that RacA and PAK kinases Chm1 and Nox1 had mutual activation effects. Furthermore, Ygor et al. (2015) found that the pathogenicity of Colletotrichum gloeosporioides was directly correlated to H2O2 production in cowpea. These results demonstrate that fungal ROS production is critical in maintaining a mutualistic fungus-plant interaction. Therefore, we hypothesize that NADPH oxidase may regulate the pathogenicity of P. expansum.
Plant cells have an evolved set of defense systems that can effectively eliminate excessive reactive oxygen and maintain stable homeostasis. SOD and CAT are important active oxygen-scavenging enzymes (Chen et al., 2016). We found that SOD and CAT activities decline in fruits during colonization by the ΔPeNoxA, ΔPeNoxR, and ΔPeRacA strains. ROS accumulation is of considerable importance for pathogenic interactions between plants and microorganism (Jones and Dangl, 2006). In the early stages of plant-microorganism interactions, there is a rapid and transient production of ROS (superoxide anion, hydrogen peroxide, and hydroxyl radical) (Buron-Moles et al., 2015). In fact, NOX enzymes often partner with SOD in signaling processes, whereby SOD converts the cell-impermeable superoxide to the diffusible hydrogen peroxide-signaling molecule (Jin et al., 2010). In Candida albicans, the deletion of SOD enhanced ROS production during morphogenesis (Rossi et al., 2017). Therefore, it is hypothesized that the Nox of P. expansum activates various antioxidant activities in the host.
Conclusion
Development of the Nox mutants in P. expansum showed specific morphological, growth, and colonization responses by PeNoxA, PeNoxR, and PeRacA. The ΔPeNoxA mutant negatively regulated the growth and development of P. expansum, and showed no effect on pathogenicity. Similarly, the ΔPeNoxR mutant showed no significant effect either in pathogenicity or in growth development. On the contrary, the growth development and pathogenicity of ΔPeRacA were reduced. ΔPeRacA showed the most sensitive to cell wall integrity stress and oxidative stress. Interestingly, the host antioxidant response determined by the analysis of SOD and CAT in fruits showed a reduce response to all the ΔPeNoxA, ΔPeNoxR, and ΔPeRacA mutants, suggesting that ROS play an important role in the interaction between the pathogen and the fruit.
Data Availability Statement
The original contributions presented in the study are included in the article/Supplementary Material, further inquiries can be directed to the corresponding author/s.
Author Contributions
XZ and YZ conceived and designed the experiments with the help of DP. YB wrote the manuscript. XZ, DG, and LY performed the experiments. YB, DP, ES, and YZ reviewed and edited the manuscript. All authors contributed to the article and approved the submitted version.
Funding
This study was supported by the National Natural Science Foundation of China-Israel International Cooperation Project (31861143046).
Conflict of Interest
The authors declare that the research was conducted in the absence of any commercial or financial relationships that could be construed as a potential conflict of interest.
Publisher’s Note
All claims expressed in this article are solely those of the authors and do not necessarily represent those of their affiliated organizations, or those of the publisher, the editors and the reviewers. Any product that may be evaluated in this article, or claim that may be made by its manufacturer, is not guaranteed or endorsed by the publisher.
Acknowledgments
We would like to thank the Qingdao DingDing Translation Co., Ltd. (www.dingdingfanyi.com) for revising the language of this article.
Supplementary Material
The Supplementary Material for this article can be found online at: https://www.frontiersin.org/articles/10.3389/fpls.2021.696210/full#supplementary-material
Supplementary Figure 1 | Construction of gene knockout vector.
Supplementary Figure 2 | Polymerase chain reaction (PCR) results of gene knockout mutants of NADPH oxidase in P. expansum.
Supplementary Table 1 | Primers used to amplify upstream and downstream sequences of the target.
Supplementary Table 2 | Primer sequences used for complementation vectors.
Footnotes
References
Buron-Moles, G., Torres, R., Teixidó, N., Usall, J., Vilanova, L., and Viñas, I. (2015). Characterisation of H2O2 production to study compatible and non-host pathogen interactions in orange and apple fruit at different maturity stages. Postharvest Biol. Technol. 99, 27–36. doi: 10.1016/j.postharvbio.2014.07.013
Chen, H., Li, J., and An, X. (2016). Potential role of reactive oxygen species and antioxidant genes in the regulation of peach fruit development and ripening. Plant Physiol. Biochem. 104, 294–303. doi: 10.1016/j.plaphy.2016.05.013
Chen, J., Zheng, W., Zheng, S., Zhang, D., Sang, W., Chen, X., et al. (2008). Rac1 is required for pathogenicity and Chm1-dependent conidiogenesis in rice fungal pathogen Magnaporthe grisea. PLoS Pathog. 4:e1000202. doi: 10.1371/journal.ppat.1000202
Eaton, C. J., Jourdain, I., Foster, S. J., Hyams, J. S., and Scott, B. (2008). Functional analysis of a fungal endophyte stress-activated MAP kinase.Curr. Genet 53, 163–174. doi: 10.1007/s00294-007-0174-6
Georgiou, C., Patsoukis, N., and Zervoudakis, G. (2006). Sclerotial metamorphosis in filamentous fungis induced by oxidative stress. Integr. Comp. Biol. 46, 691–712. doi: 10.1093/icb/icj034
Hadas, Y., Goldberg, I., Pines, O., and Prusky, D. (2007). Involvement of gluconic acid and glucose oxidase in the pathogenicity of Penicillium expansum in apples. Phytopathology 97, 384–390. doi: 10.1094/PHYTO-97-3-0384
Jin, O., Urao, N., Kim, H. W., Kaplan, N., and Razvi, M. (2010). Extracellular sod-derived H2O2 promotes VEGF signaling in caveolae/lipid rafts and post-ischemicangiogenesis in mice. PLoS One 5:e10189. doi: 10.1371/journal.pone.0010189
Jones, J. D. G., and Dangl, J. L. (2006). The plant immune system. Nature 444, 323–329. doi: 10.1038/nature05286
Kayano, Y., Tanaka, A., Akano, F., Scott, B., and Takemoto, D. (2013). Differential roles of NADPH oxidases and associated regulators in polarized growth, conidiation and hyphal fusion in the symbiotic fungus Epichloë festucae. Fungal Genet. Biol. 56, 87–97. doi: 10.1016/j.fgb.2013.05.001
Kim, H. J. (2014). Exploitation of reactive oxygen species by fungi: roles in host-fungus interaction and fungal development. J. Microbiol. Biotechnol. 24, 1455–1463. doi: 10.4014/jmb.1407.07072
Kumar, D., Barad, S., Yong, C., Luo, X., and Prusky, D. (2017). LaeA regulation of secondary metabolism modulates virulence in Penicillium expansum and is mediated by sucrose. Mol. Plant Pathol. 18, 1150–1163. doi: 10.1111/mpp.12469
Kwon, M. J., Arentshorst, M., Roos, E. D., Hondel, C., Meyer, V., and Ram, A. (2011). Functional characterization of Rho GTPases in Aspergillus niger uncovers conserved and diverged roles of Rho proteins within filamentous fungi. Mol. Microbiol. 79, 1151–1167. doi: 10.1111/j.1365-2958.2010.07524.x
Lara-Ortíz, T., Riveros-Rosas, H., and Aguirre, J. (2003). Reactive oxygen species generated by microbial NADPH oxidase NoxA regulate sexual development in Aspergillus nidulans. Mol. Microbiol. 50, 1241–1255. doi: 10.1046/j.1365-2958.2003.03800.x
Levin, E., Kishore, A., Ballester, A. R., Raphael, G., and Droby, S. (2019). Identification of pathogenicity-related genes and the role of a subtilisin-related peptidase s8 (peprt) in authophagy and virulence of Penicillium expansum on apples. Postharvest Biol. Technol. 149, 209–220. doi: 10.1016/j.postharvbio.2018.10.011
Levine, R. L., Wehr, N., Williams, J. A., Stadtman, E. R., and Shacter, E. (2000). Determination of carbonyl groups in oxidized proteins. Methods Mol. Biol. 99, 15–24. doi: 10.1385/1-59259-054-3:15
Li, B., Zong, Y., Du, Z., Chen, Y., Zhang, Z., Qin, G., et al. (2015). Genomic characterization reveals insights into patulin biosynthesis and pathogenicity in Penicillium species. Mol. Plant Microbe Interact. 28, 635–647. doi: 10.1094/MPMI-12-14-0398-FI
Li, H., Tian, S., and Qin, G. (2019). NADPH oxidase is crucial for the cellular redox homeostasis in fungal pathogen Botrytis cinerea. Mol. Plant Microbe Interact. 32, 1508–1516. doi: 10.1094/MPMI-05-19-0124-R
Lin, C. H., and Chung, K. R. (2010). Specialized and shared functions of the histidine kinase- and HOG1 MAP kinase-mediated signaling pathways in Alternaria alternata, a filamentous fungal pathogen of citrus. Fungal Genet. Biol. 47, 818–827. doi: 10.1016/j.fgb.2010.06.009
Lin, C. H., Yang, S. L., and Chung, K. R. (2009). The YAP1 homolog-mediated oxidative stress tolerance is crucial for pathogenicity of the necrotrophic fungus Alternaria alternata in citrus. Mol. Plant Microbe Interact. 22, 942–952. doi: 10.1094/MPMI-22-8-0942
Lin, Y., Chen, M., Lin, H., Hung, Y. C., Lin, Y., and Chen, Y. (2017). DNP and ATP induced alteration in disease development of Phomopsis longanae chi-inoculated longan fruit by acting on energy status and reactive oxygen species production-scavenging system. Food Chem. 228, 497–505. doi: 10.1016/j.foodchem.2017.02.045
Long, N., Valérie, V., Coroller, L., Dantigny, P., and Rigalma, K. (2017). Temperature, water activity and pH during conidia production affect the physiological state and germination time of Penicillium species. Int. J. Food Microbiol. 241, 151–160. doi: 10.1016/j.ijfoodmicro.2016.10.022
Macarisin, D., Cohen, L., Eick, A., Rafael, G., Belausov, E., and Wisniewski, M. (2007). Penicillium digitatumsuppresses production of hydrogen peroxide in host tissue infection of citrus fruit. Phytopathology 97, 1491–1500.
Malagnac, F., Lalucque, H., Lepère, G., and Silar, P. (2004). Two NADPH oxidase isoforms are required for sexual reproduction and ascospore germination in the filamentous fungus Podospora anserina. Fungal Genet. Biol. 41, 982–997. doi: 10.1016/j.fgb.2004.07.008
Marschall, R., Schumacher, J., Siegmund, U., and Tudzynski, P. (2016). Chasing stress signals-exposure to extracellular stimuli differentially affects the redox state of cell compartments in the wild type and signaling mutants of Botrytis cinerea. Fungal Genet. Biol. 90, 12–22. doi: 10.1016/j.fgb.2016.03.002
Marschall, R., and Tudzynski, P. (2016). Reactive oxygen species in development and infectionprocesses. Semin. Cell Dev. Biol. 57, 138–146. doi: 10.1016/j.semcdb.2016.03.020
Marschall, R., and Tudzynski, P. (2017). The protein disulfide isomerase of Botrytis cinerea: an ER protein involved in protein folding and redox homeostasis influences NADPH oxidase signaling processes. Front. Microbiol. 8:960. doi: 10.3389/fmicb.2017.00960
Minz, D. A., Kokkelink, L., Tudzynski, B., Tudzynski, P., and Sharon, A. (2013). Involvement of Botrytis cinerea small GTPases BcRAS1 and BcRAC in differentiation, virulenceand the cell cycle. Eukaryot. Cell 12, 1609–1618. doi: 10.1128/EC.00160-13
Río, L. A., Sandalio, L. M., Corpas, F. J., Palma, J. M., and Barroso, J. B. (2006). Reactive oxygen species and reactive nitrogen species in peroxisomes. production, scavenging, and role in cell signaling. Plant Physiol. 141, 330–335. doi: 10.1104/pp.106.078204
Rossi, D. C., Gleason, J. E., Hiram, S., Schatzman, S. S., Culbertson, E. M., Johnson, C. J., et al. (2017). Candida albicans FRE8 encodes a member of the NADPH oxidase family that produces a burst of ROS during fungal morphogenesis. PLoS Pathog. 13:e1006763. doi: 10.1371/journal.ppat.1006763
Scott, B., and Eaton, C. J. (2008). Role of reactive oxygen species in fungal cellular differentiations. Curr. Opin. Microbiol. 11, 488–493. doi: 10.1016/j.mib.2008.10.008
Scott, B., Takemoto, D., and Tanaka, A. (2007). Fungal endophyte production of reactive oxygen species is critical for maintaining the mutualistic symbiotic interaction between Epichloë festucae and perennial ryegrass. Plant Signal. Behav. 2, 171–173.
Segal, L. M., and Wilson, R. A. (2018). Reactive oxygen species metabolism and plant-fungal interactions. Fungal Genet. Biol. 110, 1–9. doi: 10.1016/j.fgb.2017.12.003
Segmüller, N., Kokkelink, L., Giesbert, S., Odinius, D., Kan, V. J., and Tudzynski, P. (2008). NADPH oxidases are involved in differentiation and pathogenicity in Botrytis cinerea. Mol. Plant Microbe Interact. 21, 808–819. doi: 10.1094/MPMI-21-6-0808
Semighini, C. P., and Harris, S. D. (2008). Regulation of apical dominance in Aspergillus nidulans hyphae by reactive oxygen species. Genetics 179, 1919–1932. doi: 10.1534/genetics.108.089318
Si, H., Rittenour, W. R., and Harris, S. D. (2016). Roles of Aspergillus nidulans Cdc42/Rho GTPase regulators in hyphal morphogenesis and development. Mycologia 108, 543–555. doi: 10.3852/15-232
Siwy, L. Y., Yu, P., and Chung, K. (2016). The glutathione peroxidase-mediated reactive oxygen species resistance, fungicide sensitivity and cell wall construction in the citrus fungal pathogen Alternaria alternata. Environ. Microbiol. 18, 923–935. doi: 10.1111/1462-2920.13125
Sumimoto, H. (2008). Structure, regulation and evolution of Nox-family NADPH oxidases that produce reactive oxygen species. FEBS J. 275, 3249–3277. doi: 10.1111/j.1742-4658.2008.06488.x
Sun, C., Fu, D., Lu, H., Zhang, J., Zheng, X., and Yu, T. (2018). Autoclaved yeast enhances the resistance against Penicillium expansum in postharvest pear fruit and its possible mechanisms of action. Biol. Control 119, 51–58. doi: 10.1016/j.biocontrol.2018.01.010
Susumu, H., Katsutomo, S., Hiroyuki, I., Yuko, O., and Hirokazu, M. (2001). A large family of class III plant peroxidases. Plant Cell Physiol. 42, 462–468. doi: 10.1016/j.jhep.2008.08.025
Takemoto, D., Tanaka, A., and Scott, B. (2006). A p67Phox-Like regulator is recruited to control hyphal branching in a fungal-grass mutualistic symbiosis. Plant Cell 18, 2807–2821. doi: 10.1105/tpc.106.046169
Tanaka, A., Christensen, M. J., Takemoto, D., Park, P., and Scott, B. (2006). Reactive oxygen species play a role in regulating a fungus-perennial ryegrass mutualistic interaction. Plant Cell 18, 1052–1066. doi: 10.4161/psb.2.3.3725
Tanaka, A., Takemoto, D., Hyon, G. S., Park, P., and Scott, B. (2008). NoxA activation by the small GTPase RacA is required to maintain a mutualistic symbiotic association between Epichloë festucae and perennial ryegrass. Mol. Microbiol. 68, 1165–1178. doi: 10.1111/j.1365-2958.2008.06217.x
Tudzynski, P., Heller, J., and Siegmund, U. (2012). Reactive oxygen species generation in fungal development and pathogenesis. Curr. Opin. Microbiol. 15, 653–659. doi: 10.1016/j.mib.2012.10.002
Turrà, D., and Di, P. A. (2015). Chemotropic sensing in fungus-plant interactions. Curr. Opin. Plant Biol. 26, 135–140. doi: 10.1016/j.pbi.2015.07.004
Wang, Y., Ji, D., Chen, T., Li, B., and Tian, S. (2019). Production, signaling, and scavenging mechanisms of reactive oxygen species in fruit-pathogen interactions. Int. J. Mol. Sci. 20, 2994–3006. doi: 10.3390/ijms20122994
Wood, M. J., Andrade, E. C., and Storz, G. (2003). The redox domain of the Yap1p transcription factor contains two disulfide bonds. Biochemistry 42, 11982–11991. doi: 10.1021/bi035003d
Xu, X., and Tian, S. (2008). Reducing oxidative stress in sweet cherry fruit by Pichia membranaefaciens: a possible mode of action against Penicillium expansum. J. Appl. Microbiol. 105, 1170–1177. doi: 10.1111/j.1365-2672.2008.03846.x
Yang, S. L., and Chung, K. R. (2012). The nadph oxidase-mediated production of hydrogen peroxide (H2O2) and resistance to oxidative stress in the necrotrophic pathogen Alternaria alternata of citrus. Mol. Plant Pathol. 13, 900–914. doi: 10.1111/j.1364-3703.2012.00799.x
Yang, S. L., and Chung, K. R. (2013). Similar and distinct roles of NADPH oxidase componentsin the tangerine pathotype of Alternaria alternata. Mol. Plant Pathol. 14, 543–556. doi: 10.1111/mpp.12026
Yang, Y., Du, X. J., Li, P., Liang, B., and Wang, S. (2014). An optimized method for the preparation of monascus purpureus dna for genome sequencing. Appl. Mech. Materials 563, 379–383. doi: 10.4028/www.scientific.net/AMM.563.379
Ygor, R. G., Eloy, I. M., Vasconcelos, A., Barreto, A., Freire-Filho, F. R., and Oliveira, J. (2015). H2O2 plays an important role in the lifestyle of Colletotrichum gloeosporioides during interaction with cowpea [Vigna unguiculata (L.) Walp.]. Fungal Biol. 119, 745–757. doi: 10.1016/j.funbio.2015.05.001
Zhang, X., Zong, Y., Li, Z., Yang, R., Bi, Y., and Prusky, D. (2020). Postharvest Pichia guilliermondii treatment promotes wound healing of apple fruits. Postharvest Biol. Technol. 167, 111228–111235. doi: 10.1016/j.postharvbio.2020.111228
Zhou, T., Wang, X., Luo, J., Ye, B., Zhou, Y., and Zhou, L. (2018). Identification of differentially expressed genes involved in spore germination of Penicillium expansum by comparative transcriptome and proteome approaches. MicrobiologyOpen 7, 562–575. doi: 10.1002/mbo3.562
Keywords: Penicillium expansum, NADPH oxidases, reactive oxygen species, growth, pathogenicity
Citation: Zhang X, Zong Y, Gong D, Yu L, Sionov E, Bi Y and Prusky D (2021) NADPH Oxidase Regulates the Growth and Pathogenicity of Penicillium expansum. Front. Plant Sci. 12:696210. doi: 10.3389/fpls.2021.696210
Received: 16 April 2021; Accepted: 19 July 2021;
Published: 12 August 2021.
Edited by:
Antonieta De Cal, Instituto Nacional de Investigación y Tecnología Agroalimentaria (INIA), SpainReviewed by:
Lise Korsten, University of Pretoria, South AfricaRosario Torres, Institute of Agrifood Research and Technology (IRTA), Spain
Copyright © 2021 Zhang, Zong, Gong, Yu, Sionov, Bi and Prusky. This is an open-access article distributed under the terms of the Creative Commons Attribution License (CC BY). The use, distribution or reproduction in other forums is permitted, provided the original author(s) and the copyright owner(s) are credited and that the original publication in this journal is cited, in accordance with accepted academic practice. No use, distribution or reproduction is permitted which does not comply with these terms.
*Correspondence: Yang Bi, Yml5YW5nQGdzYXUuZWR1LmNu
†These authors have contributed equally to this work and share first authorship