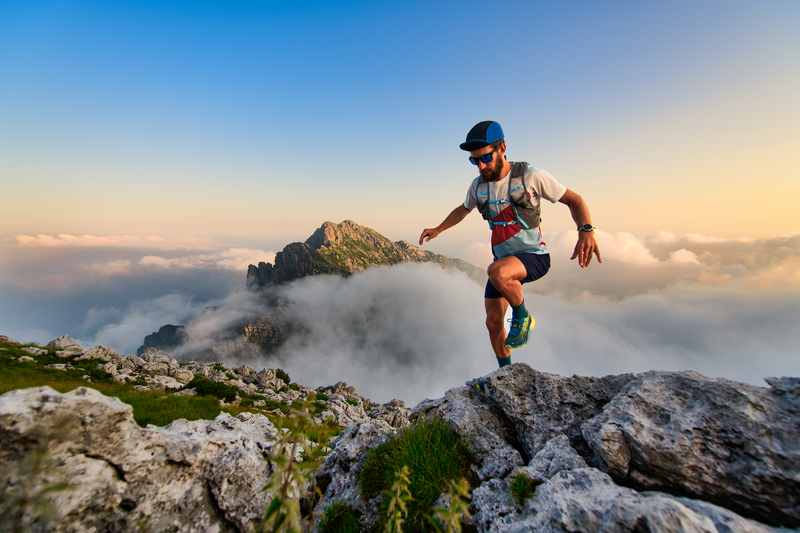
95% of researchers rate our articles as excellent or good
Learn more about the work of our research integrity team to safeguard the quality of each article we publish.
Find out more
ORIGINAL RESEARCH article
Front. Plant Sci. , 10 June 2021
Sec. Plant Pathogen Interactions
Volume 12 - 2021 | https://doi.org/10.3389/fpls.2021.690857
Pine wilt disease (PWD), caused by the plant–parasitic nematode Bursaphelenchus xylophilus, has become a severe environmental problem in the Iberian Peninsula with devastating effects in Pinus pinaster forests. Despite the high levels of this species' susceptibility, previous studies reported heritable resistance in P. pinaster trees. Understanding the basis of this resistance can be of extreme relevance for future programs aiming at reducing the disease impact on P. pinaster forests. In this study, we highlighted the mechanisms possibly involved in P. pinaster resistance to PWD, by comparing the transcriptional changes between resistant and susceptible plants after infection. Our analysis revealed a higher number of differentially expressed genes (DEGs) in resistant plants (1,916) when compared with susceptible plants (1,226). Resistance to PWN is mediated by the induction of the jasmonic acid (JA) defense pathway, secondary metabolism pathways, lignin synthesis, oxidative stress response genes, and resistance genes. Quantification of the acetyl bromide-soluble lignin confirmed a significant increase of cell wall lignification of stem tissues around the inoculation zone in resistant plants. In addition to less lignified cell walls, susceptibility to the pine wood nematode seems associated with the activation of the salicylic acid (SA) defense pathway at 72 hpi, as revealed by the higher SA levels in the tissues of susceptible plants. Cell wall reinforcement and hormone signaling mechanisms seem therefore essential for a resistance response.
Pine wilt disease (PWD) is caused by Bursaphelenchus xylophilus, or pinewood nematode (PWN), which is transmitted by the insect vector Monochamus spp. while feeding on healthy trees. Upon entering the tree stem, PWN spreads through the resin canals, feeds on plant cells or fungi that populate the decaying tree, and breeds (Evans et al., 1996; Vicente et al., 2012; Kim et al., 2020).
During the last century, PWD has become a worldwide threat to conifer forests (Webster and Mota, 2008), being particularly damaging for trees of the genus Pinus. In the Iberian Peninsula, it was first detected in the late 1990's (Mota et al., 1999), spreading rapidly through Portugal and reaching Spain. In this region, it infects mostly Pinus pinaster trees, which are highly susceptible (Evans et al., 1996). Given the high economic and ecological value of P. pinaster in southwestern Europe due to its use in paper, wood, and resin production, its importance for soil protection, and as wildlife habitat, PWD has a huge impact on the local economy and environment (Webster and Mota, 2008; Vicente et al., 2012).
Remarkably, varieties with high resistance levels have been described in susceptible pine species (Toda and Kurinobu, 2002; Xu et al., 2012). In P. pinaster, different levels of resistance were also observed in plants after artificially inoculated with PWN (Menéndez-Gutiérrez et al., 2017a,b; Carrasquinho et al., 2018). Since control measures implemented so far have failed in stopping PWD spreading, breeding resistant varieties may be a highly effective control strategy. Breeding programs have been successfully implemented for Pinus thunbergii, Pinus densiflora, and Pinus massoniana (Toda and Kurinobu, 2002; Xu et al., 2012). For P. pinaster, genetic variation in susceptibility to PWN inoculation was observed in two independent studies (Menéndez-Gutiérrez et al., 2017a; Carrasquinho et al., 2018), in which a moderate family heritability for survival (0.37; Carrasquinho et al., 2018) and mortality (0.59; Menéndez-Gutiérrez et al., 2017a) after inoculation was detected, suggesting that implementation of breeding programs can be valuable. Furthermore, plants without symptoms had very few PWNs when compared to symptomatic plants (Menéndez-Gutiérrez et al., 2017a), suggesting asymptomatic plants were able to control the multiplication of PWN, showing, therefore, true resistance to the parasite (Trudgill, 1991; Woodcock et al., 2018).
The identification of the mechanisms involved in resistance to PWN may inform on effective strategies to fight the disease. In general, plant defense response initiates upon recognition of the pathogen at the cellular level (Couto and Zipfel, 2016). Cell membrane receptor-like kinases (RLKs) or receptor-like proteins (RLPs) recognize pathogen-associated molecular patterns (PAMPs) or damage-associated molecular patterns (DAMPs), initiating a series of signaling events that culminate in transcriptional reprogramming and expression of defense response genes. This pattern-triggered immunity (PTI) represents the first level of plant defense against pathogens (Dodds and Rathjen, 2010; Couto and Zipfel, 2016). However, adapted pathogens release effectors to suppress host immunity. In turn, these effectors may be recognized by intracellular nucleotide-binding/leucine-rich-repeat (NLR) receptors, inducing a more robust defense response, the effector-triggered immunity (ETI) (Cui et al., 2015). Several RLK/RLP and NLR receptors have been implicated in resistance to plant–parasitic nematodes (Sato et al., 2019; Zheng et al., 2021). However, these studies focus on sedentary and biotrophic species, and intracellular NLR receptors may not have a relevant role in resistance to migratory non-biotrophic nematodes such as PWN. The activation of PTI and ETI triggers hormone-dependent plant immune responses, such as salicylic acid (SA), jasmonic acid (JA), and ethylene (ET) pathways (Tsuda and Katagiri, 2010; Buscaill and Rivas, 2014). Other hormones like gibberellins, auxins, cytokinins, and abscisic acid (ABA), although usually associated with development or response to abiotic stresses, have also been shown to play an important role in plant–microbe interactions (De Vleesschauwer et al., 2014).
Although limited knowledge is available about the possible mechanisms involved in resistance to PWD in individuals within susceptible pine species, a few studies have focused on the comparison of transcriptional responses between PWD resistant and susceptible plants. For P. thunbergii (Nose and Shiraishi, 2011; Hirao et al., 2012) and P. massoniana (Liu et al., 2017) resistance was associated with higher expression levels of genes related to the synthesis of secondary metabolites, namely flavonoids (Kuroda et al., 2011) and terpenes (Liu et al., 2017), cell wall reinforcement, including genes related to plant cell wall lignification (Hirao et al., 2012; Liu et al., 2017), and ROS detoxification. Furthermore, higher lignification seemed to limit PWN migration in resistant P. thunbergii plants (Kusumoto et al., 2014). Recently, susceptibility in P. pinaster was associated with the activation of SA and JA pathways, as part of an inefficient trigger of the hypersensitive response (Rodrigues et al., 2021).
The first transcriptomic analysis addressing the PWN response in P. pinaster was based on the comparison to P. pinea, described as less susceptible than P. pinaster (Santos et al., 2012), while more recent reports in P. pinaster described the transcriptional changes after PWN infection during a susceptible interaction (Gaspar et al., 2017, 2020). However, despite the identification of P. pinaster genotypes considered resistant (Menéndez-Gutiérrez et al., 2017a,b; Carrasquinho et al., 2018), the transcriptional response associated with resistance has not been previously analyzed.
Our aim was to identify the molecular mechanisms involved in P. pinaster resistance to PWD. In the absence of available P. pinaster clones showing either susceptibility or resistance toward the PWN, we took advantage of within family variation (Menéndez-Gutiérrez et al., 2017a; Carrasquinho et al., 2018) and used half-siblings from a single family in the transcriptomic analysis. In this way, differences in gene expression resulting from genetic variation in traits other than response to PWN were minimized. While it would be interesting to extend this analysis to other families, the strategy used here contributes to highlight the most relevant genes for the PWN response by exploring the behavior of one of the top-ranking half-sib families regarding genetic effects on survival to PWN infection, previously characterized by Carrasquinho et al. (2018). We hypothesize that differences in survival to PWN infection may be related to different transcriptional responses in the first days after inoculation, as it was observed in other Pinus spp. (Nose and Shiraishi, 2011; Hirao et al., 2012; Xu et al., 2012; Liu et al., 2017). In order to test this, we inoculated several plants within the selected family and analyzed the differential expression (DE) in susceptible and resistant plants at 72 h post-inoculation (72 hpi) (Nose and Shiraishi, 2011; Hirao et al., 2012; Xu et al., 2012). Through a comparative transcriptomic analysis of PWN resistant and susceptible plants, complemented with the investigation of cell wall lignification and hormone signaling, we obtained the first insights into the resistance mechanisms possibly involved and detected candidate resistance genes that can be a valuable resource for future studies.
Bursaphelenchus xylophilus isolate Bx013.003 (Carrasquinho et al., 2018; Rodrigues et al., 2021) was obtained from an infested field tree exhibiting wilting symptoms in central Portugal (39°43′33.8′′N, 9°01′55.7′′W) and was included in the collection of INIAV's Nematology Laboratory, Oeiras, Portugal. The sequence of the ITS region is available at GenBank (NCBI) under the accession number MF611984.1. Nematodes were kept in pure culture at 25 ± 1°C on a non-sporulating Botrytis cinerea strain grown on autoclaved barley grains. Prior to inoculation, nematodes were allowed to grow on sterilized wood. Nematodes were separated from the culture media using the “tray” method (Whitehead and Hemming, 1965) and suspended in water in a concentration of 1,000 PWN/ml.
Twenty-three potted 4-year-old P. pinaster plants from the half-sib family 440 were maintained in a greenhouse and placed according to a completely randomized experimental design. The plants were derived from seeds obtained from the mother tree 440, which is included in the reference population for PWD resistance from “Herdade da Comporta” (38°21′28.52′′N, 8°45′49.89′′W) in southern Portugal (Ribeiro et al., 2012), resulting from a mass selection program initiated in 2009. Within a half-sib family, part of the individuals may prove resistant while the majority are susceptible. Family 440 was previously characterized by Carrasquinho et al. (2018) as one of the 15 top-ranked half-sib families (among 96 evaluated families) regarding the genetic effects on survival after PWN inoculation. Predicted survival means at 157 days after inoculation ranged from 6 to 23% using 2-year-old plants, having family 440 shown a predicted survival mean of 15% (Carrasquinho et al., 2018). The plants were inoculated in September 2016, following the method of Futai and Furuno (1979). A suspension aliquot with 500 nematodes was pipetted into a small longitudinal wound made in the main stem with a sterile scalpel below the apical shoot region (Figures 1A,B). Inoculated wounds were covered with parafilm to prevent drying of the inoculum. Eighteen plants were inoculated with PWN and five controls were inoculated with sterile water. Stem samples of approximately 5 cm, including the inoculation zone (Figure 1A), were collected 72 hpi (Nose and Shiraishi, 2011; Hirao et al., 2012; Xu et al., 2013) and immediately frozen in liquid nitrogen. After removal of the inoculation zone (and apical stem), the remaining part of each plant was kept in the greenhouse and observed for symptoms weekly for a period of 210 days (Figures 1C–E). Plants were classified according to a scale from 0 (no visible symptoms) to 4 (more than 75% of needles brown/wilted) (Figure 1E). The first symptoms were visible 14 days post-inoculation (dpi) and evolved progressively until the end of the experiment. Plants presenting symptoms (1–4 in the symptoms scale) were considered susceptible, while plants without any symptoms (0) were classified as resistant. As this classification is based on external symptoms and not on nematode counting, plants here considered resistant may in fact be tolerant, maintaining a healthy phenotype despite PWN multiplication (Trudgill, 1991; Woodcock et al., 2018), although true resistance, in which plants were able to inhibit PWN multiplication, was observed in other P. pinaster families (Menéndez-Gutiérrez et al., 2017a). It should be noted that at 72 hpi PWNs are expected to have spread through plant tissues several centimeters away from the inoculation zone (Ichihara et al., 2000; Kusumoto et al., 2014; Son et al., 2015).
Figure 1. Inoculation, sampling, and symptoms observation. Plants were inoculated in the stem, below the apical region (A,B). Samples of the stem, including the inoculation zone, were collected 72 h post-inoculation (hpi). After debarking, these samples were homogenized and total RNA was extracted. The remaining part of the plant, below the cutting region, was maintained for symptoms observations for 210 days post-inoculation (dpi). Symptoms were evaluated weekly and registered according to a five-level scale based on percentage of brown/wilted needles: 0, 0% (D); 1, 1–25%; 2, 26–50%; 3, 51–75%; 4, 76–100% (C). Symptom progression in selected timepoints is represented in (E). Plants without any visible symptom at the end of the experiment were considered resistant.
Height and diameter at the base of the stem were measured before inoculation. A two-sample unpaired t-test was performed using R v3.5.1 (https://www.r-project.org) to evaluate significant differences in these parameters between resistant and susceptible plants.
Total RNA was extracted from each stem sample, after debarking, using the method described in Le Provost et al. (2007). RNA concentrations were measured using Qubit™ 4 Fluorometer (Thermo Fisher Scientific, Waltham, MA USA) with the RNA BR Assay Kit and integrity was verified with LabChip GX (PerkinElmer, Hopkinton, MA USA). Four susceptible and five resistant plants with the most contrasting phenotypes, i.e., plants that died faster (symptoms scale level 4) and plants without symptoms (symptoms scale level 0) during the entire observation period, were selected for library preparation, as well as four control samples. Libraries were prepared with the Illumina TruSeq Stranded mRNA Kit and sequenced on Illumina HiSeq 2500 (Fasteris, Switzerland), providing 125 bp single-end reads. Each sample was run in two independent lanes.
The quality of the RNA-seq data was evaluated with FastQC v0.11.2 (Andrews, 2010). Adapter and quality trimming were performed using clc_adapter_trim and clc_quality_trim, respectively, from CLC Assembly Cell v7.0.4 (Quiagen, Hilden, Germany), with default parameters.
At the moment of our analysis, a reference transcriptome was available for P. pinaster (Cañas et al., 2017). However, this transcriptome did not include samples submitted to any kind of biotic stress. Therefore, in order to include transcripts that may be specific to PWN infection response, we performed a de novo assembly with reads from all inoculated samples using Trinity v2.6.6 (Grabherr et al., 2013) with default parameters. The resulting contigs were compared with the previously available P. pinaster transcriptome and PWN genome (Kikuchi et al., 2011) using BLASTn (DeCypher Tera-BLASTn, TimeLogic, California, USA) and highly similar sequences (e ≤ 10−5) were filtered out. To further exclude contigs originating from PWN, a BLASTx (DeCypher Tera-BLASTx) was performed with the National Center for Biotechnology Information (NCBI) Protein database (accessed January 2019) and all the sequences with blast hits to a nematode species were excluded. In this way, 34,737 new transcripts were added to the 206,574 from the previous P. pinaster reference transcriptome (Supplementary Table 1). For these 34,737 transcripts, Transdecoder v2.1.0 (Haas, 2019) was used to predict protein coding regions.
Reads were mapped to the P. pinaster transcripts containing predicted coding regions (CDS), including both the newly predicted and the ones available in Gymno PLAZA 1.0 database (https://bioinformatics.psb.ugent.be/plaza/versions/gymno-plaza/) (70,870 transcripts). The nematode reference transcriptome (17,704 sequences) (Kikuchi et al., 2011) was obtained from WormBase ParaSite (http://parasite.wormbase.org) and used to filter out the reads corresponding to the pathogen. Reads were mapped using the BWA alignment software v0.7.5a (BWA-MEM) (Li, 2013) with default parameters. The mapping results were filtered and only uniquely mapped reads where kept for read counting using SAMtools v1.3 (Li et al., 2009). Pinus pinaster and PWN transcripts and respective counts were separated in two files, and only P. pinaster data was used for DE analysis.
Protein sequences were obtained from Gymno PLAZA 1.0 for the available transcriptome and Transdecoder predictions were generated for the newly discovered transcripts. To functionally annotate the P. pinaster transcriptome, a similarity search was performed using BLASTp (DeCypher Tera-BLASTp) alignments and the NCBI RefSeq Plant database (accessed February 2019). InterProScan was used to identify protein domains, assign gene ontology (GO) terms, and assign Kyoto Encyclopedia of Genes and Genomes (KEGG) pathways. KEGG annotation was further improved by using KEGG Automatic Annotation Server (KAAS) (Moriya et al., 2007). In the set of differentially expressed genes (DEGs), transcription factors were identified and classified using iTAK (Zheng et al., 2016). Genes potentially involved in disease resistance were identified with DRAGO 2 available from the Plant Resistance Genes database (Osuna-Cruz et al., 2017).
The DE analysis was done using DESeq2 (Love et al., 2014) with a 0.05 false discovery rate (FDR) threshold. Results were filtered for genes with log2 fold change ≥|2|. Venn diagrams were drawn (http://bioinformatics.psb.ugent.be/webtools/Venn/).
Gene set enrichment analysis was performed using BiNGO plugin (Maere et al., 2005) for Cytoscape (Shannon et al., 2003). The hypergeometric statistical test was used, and Benjamini and Hochberg FDR was applied for multi testing correction, with a significance level ≤ 0.05. Gene ontology redundancy was decreased by using Revigo tool (Supek et al., 2011) with a soft trim threshold of 40%. Pathway enrichment analysis was performed using the hypergeometric statistical test implemented in BiNGO with the same parameters.
To validate DE results, 10 genes with different expression patterns in susceptible and resistant plants were selected for quantitative RT-qPCR. Primers were designed using PerlPrimer (Marshall, 2004) (Supplementary Table 2). cDNA synthesis was performed from total RNA samples of three resistant, three susceptible, and three control plants using SuperScript™ IV First-Strand Synthesis System (Invitrogen, USA) and oligo(dT)20 primer. RT-qPCR was run in a LightCycler 480 Instrument II (Roche, Switzerland) using SYBR Green I Master (Roche) and the following conditions: 5 min at 95°C, 40 cycles of 95°C for 10 s, 58–63°C for 15 s (Supplementary Table 2), and 72°C for 12 s. Primer specificity was monitored by analyzing the melting curves. Three technical replicates were performed for each biological replicate. Transcript profiles were normalized using the reference genes actin, 40S rRNA (Pascual et al., 2015), and histone H3 (de Vega-Bartol et al., 2013). Relative expression levels of candidate genes were calculated with the Pfaffl (2001) method.
Powdered stem samples were freeze-dried and 1 mg was used for determining lignin content. Acetyl bromide-soluble lignin was determined according to Foster et al. (2010) and a standard curve was generated with alkali lignin (Sigma-Aldrich, 370959). Five susceptible, five resistant, and two control samples were used for this analysis and three technical replicates were made for each biological replicate. A two-sample unpaired t-test was performed to evaluate significant differences between control and susceptible or resistant plants (R v3.5.1).
Hormone quantification was performed for five susceptible, five resistant, and four control samples. Before extraction, freeze dried powdered stem samples were weighed in 2 ml-microtubes and spiked with 25 μl of an internal standard mixture (containing ABA-d6, DHJA, and C13-SA concentration of 1 mg L−1) to correct for analyte loses. Extraction was carried out in 1 ml ultrapure water for 10 min in a ball mill at room temperature using 2 mm glass beads. After extraction, homogenates were centrifuged at 10,000 rpm for 10 min at 4°C and supernatants recovered. The resulting solutions were partitioned twice against an equal volume of di-ethyl ether after adjusting pH to 3.0 with a 30% acetic acid solution. The combined organic layers were evaporated under vacuum in a centrifuge concentrator (Jouan, Sant Germaine Cedex, France) and the dry residues reconstituted in 0.5 ml of a 10% aqueous methanol solution. Prior to injection, extracts were filtered through 0.20 μm PTFE syringe membrane filters and filtrates recovered in chromatography amber glass vials. Samples were analyzed by tandem LC/MS in an Acquity SDS UPLC system (Waters Corp., USA) coupled to a TQS triple quadrupole mass spectrometer (Micromass Ltd., UK) through an electrospray ionization source. Separations were carried out on a C18 column (Luna Omega Polar C18, 50 × 2.1 mm, 1.6 μm particle size, Phenomenex, USA) using a linear gradient of ultrapure acetonitrile and water, both supplemented with formic acid to a 0.1% (v/v) concentration, at a constant flow rate of 0.3 ml min−1. During analyses, column temperature was maintained at 40°C and samples at 10°C to slow down degradation. Plant hormones were detected in negative electrospray mode following their specific precursor-to-product ion transitions (ABA, 263>153; JA, 209>59; JA-Ile, 322>130; and SA, 137>93) and quantified using an external calibration curve with standards of known amount. To evaluate for significant differences between control and susceptible or control and resistant plants, a two-sample unpaired t-test was performed (R v3.5.1).
To identify genes that may be involved in resistance to PWD, an artificial PWN inoculation assay was performed with plants from a previously characterized half-sib family (Carrasquinho et al., 2018). After sampling the stem of inoculated plants at 72 hpi, plants were observed and evaluated weekly for PWD symptoms for 210 dpi. In each timepoint, plants were classified on a scale from 0 (absence of symptoms) to 4 (more than 75% of brown/wilted needles) (Figure 1E). The first symptoms were visible at 14 dpi and at 35 dpi the first plants died (level 4). After 210 dpi, 28% of the plants continued showing no symptoms (level 0) and were considered resistant. The remaining plants were considered susceptible. From the susceptible plants, 69% had died (level 4) by the end of the experiment. The first four plants reaching level 4 in the symptoms scale were selected as the susceptible plants to be sequenced by RNA-seq. Resistant and susceptible plants showed no significant differences in height and diameter at the stem base (Supplementary Figure 1).
RNA-seq data from samples of stem tissue from four susceptible, five resistant, and four control plants yielded 17–20 million reads per sample, with sizes ranging between 70 and 125 bp and an average quality score of 36. The de novo transcriptome assembly produced 250,339 transcripts (Supplementary Table 1), from which 215,602 were highly similar to the P. pinaster transcriptome previously available (Cañas et al., 2017), the PWN genome (Kikuchi et al., 2011) or sequences available from other nematode species. From the remaining 34,737 transcripts, 1,445 had a predicted protein coding sequence (CDS) (Data Sheet 1). In combination with the transcripts retrieved from the Gymno PLAZA 1.0 database, a reference transcriptome of 70,870 transcripts with predicted proteins was obtained. From these transcripts, 46,625 were functionally annotated with BLASTp (DeCypher Tera-BLASTx) similarity search. Using InterProScan, at least one protein domain was identified for 44,839 transcripts, of which 31,192 had GO annotations assigned. By joining InterProScan and KAAS annotations, 17,059 transcripts were associated with at least one KEGG pathway.
Read sequences were mapped to the P. pinaster and PWN transcriptomes. On average, a mapping ratio of 93% was obtained, of which 69% were uniquely mapped. The percentage of reads derived from PWN in infected plants varied between samples, from 0.2 to 0.7%. PWN reads mapped to genes previously described as important for pathogenicity (Supplementary Table 3) (Kikuchi et al., 2011; Shinya et al., 2013; Espada et al., 2016), such as genes encoding enzymes involved in plant cell wall degradation (e.g., endo-β-1,4-glucanase, pectate lyase, expansin), peptidases (e.g., cysteine proteinase, aspartic protease), anti-oxidant proteins (e.g., peroxiredoxin, glutathione S-transferase, thioredoxin, superoxide dismutase) (Shinya et al., 2013), and effector protein genes, such as venom-allergen like protein 1 (VAP1) and VAP2, which may cause the suppression of the plant immune response (Lozano-Torres et al., 2014). The expression of several pathogenicity genes during infection found in our dataset is consistent to what was described for other plant–parasitic nematodes (Haegeman et al., 2012; Goverse and Smant, 2014). For the DE analysis, only the reads uniquely mapped to P. pinaster transcriptome were retained.
Differential expression analysis was performed by comparing control plants to either resistant or susceptible ones. From the 40,391 transcripts with mapped reads, 1,916 and 1,226 were differentially expressed in resistant and in susceptible plants, respectively (log2FoldChange ≥ 2, adjusted p-value ≤ 0.05; Supplemetary Tables 4, 5). In resistant plants, 1,182 genes were upregulated and 734 downregulated, while in susceptible plants 720 were upregulated and 506 downregulated. Part of the DEGs was shared (44.6%), while 11.8% were unique to susceptible and 43.6% were unique to resistant plants (Figure 2A). Analysis by RT-qPCR of 10 randomly selected genes show the same expression trends as the RNA-seq results (Figure 3A) with a positive correlation coefficient (R2 = 0.91, Figure 3B).
Figure 2. Venn diagram showing overlap of differentially expressed genes in susceptible (S) and resistant (R) samples (A) and gene set enrichment analysis (B–D). (A) Differential expression was calculated by comparing susceptible (S) or resistant (R) samples with controls (C). (B–D) GO terms overrepresented in the upregulated genes in resistant (dark gray) and susceptible (light gray) samples are displayed, separated by (B) biological process (BP), (C) cellular component (CC), and (D) molecular function (MF). The x-axis represents the significance of GO enrichment (–log10 of corrected p-values).
Figure 3. RT-qPCR analysis of 10 DEGs from the RNA-seq results. (A) Bars represent differential expression levels, in log2(fold change), of susceptible (white) and resistant (gray) plants in comparison with controls. Results from both the RNA-seq analysis (filled colors) and the RT-qPCR analysis (stripes) are displayed. Error bars represent the standard error of the biological replicates used for RNA-seq (4–5) and RT-qPCR (3). (B) Correlation of expression levels between RNA-Seq and RT-qPCR.
After redundancy reduction, 38 and 53 GO terms were enriched for upregulated genes in susceptible (Supplementary Table 6) and resistant plants (Supplementary Table 7), respectively. Several GO terms that are related with biotic stress response, such as DNA-binding transcription factor activity, response to oxidative stress, or defense response to bacterium, were enriched both in susceptible and resistant plants (Figures 2B–D). Gene ontology terms as the MFs chitinase activity and terpene synthase activity (Figure 2B), the BP reactive oxygen species metabolic process (Figure 2C), or the CCs cell wall and exocyst (Figure 2D), were enriched only in resistant plants.
For the upregulated genes, 13 pathways were enriched in resistant plants and 9 in susceptible plants (Figure 4). Pathways commonly associated with biotic stress response were enriched in both resistant and susceptible plants, including alpha-Linolenic acid metabolism, which leads to the synthesis of JA, phenylpropanoid biosynthesis, which leads to the synthesis of several compounds including lignin, plant hormone signal transduction, and flavonoid biosynthesis. Pathways enriched only in resistant plants include amino sugar and nucleotide sugar metabolism and MAPK signaling pathway, while plant–pathogen interaction was enriched only in susceptible plants (Figure 4).
Figure 4. Pathway enrichment analysis. KEGG pathways overrepresented in the upregulated genes in resistant (dark gray) and susceptible (light gray) samples are depicted in the graph. The x-axis represents the significance of KEGG enrichment (–log10 of corrected p-values).
Secondary metabolites play an important role in conifers defense response and have been associated with resistance to insects and pathogens (Keeling and Bohlmann, 2006; Eyles et al., 2010; Ahuja et al., 2012). Although several genes involved in secondary metabolism pathways were differentially expressed after inoculation, a few genes related to the biosynthesis of terpenoids, such as AS (bifunctional abietadiene synthase, unigene128167), LPS (bifunctional levopimaradiene synthase, unigene10412), or GERD [(-)-germacrene D synthase, unigene144607 and unigene8510] had higher expression levels in resistant plants (Supplementary Figure 2). Likewise, a few genes from the flavonoid biosynthesis pathway were more expressed in resistant plants (Supplementary Figure 2), such as CHS4 (chalcone synthase 4, isotig47436), CHS2 (unigene147178), and LDOX (leucoanthocyanidin dioxygenase, unigene210255).
In contrast, a high number of genes in the phenylpropanoid biosynthesis pathway had different expression levels in resistant and susceptible plants (Figure 5). Several genes involved in lignin synthesis, including peroxidase (PER, Figure 5D) and laccase (LAC, Figure 5C) genes (Vogt, 2010; Xie et al., 2018), were more expressed in resistant plants. In addition, genes encoding for aldehyde oxidase (GLOX) enzymes, which produce hydrogen peroxide, a molecule necessary for lignin polymerization by PERs, had considerably higher expression levels in resistant plants (Supplementary Figure 3). These results, suggesting the induction of lignin biosynthesis, were supported by experimental determination of the lignin content in stem tissues, which detected a significantly higher amount of lignin in resistant plants when compared to controls, while susceptible plants were not significantly different from controls (Figure 5E). Hydrogen peroxide may also play an important role in the activation of the plant defense response and is toxic for pests and pathogens (Holbein et al., 2016). The higher production of ROS was reflected by the high number of oxidative stress response genes upregulated (Supplementary Figure 3).
Figure 5. Lignin biosynthesis pathway. Lignin biosynthesis pathway is represented (adapted from Xie et al., 2018), with the differential expressed genes highlighted in gray. Heatmaps represent log10(TPM) values of differentially expressed genes in the general phenylpropanoid pathway (A) and the lignin specific pathway (B–D). The final steps of lignin synthesis are carried out by laccases (LAC, C) and peroxidases (PER, D). The percentage of acetyl bromide soluble lignin of cell wall (ABSL of CW) measured in control (C), susceptible (S), and resistant (R) plants is represented in (E). Error bars represent the standard error of the mean. Significant differences between control and inoculated plants, using Student's t-test, are indicated by an asterisk (*p-value < 0.05).
Several genes involved in the JA biosynthesis pathway were upregulated in inoculated plants, with Lipoxygenase (LOX), phospholipase A2 (PLA2G), and 12-oxophytodienoic acid reductase (OPR) genes showing higher expression levels in resistant plants (Figure 6E). Analysis of hormone levels in the several sample types detected similar JA-Ile levels in resistant, susceptible, and control plants (Figure 6A), while the JA levels were higher only in inoculated plants (Figure 6C), although with no significant differences between resistant and susceptible plants.
Figure 6. Hormone response to PWN inoculation. (A) Levels of jasmonate-Illenine (JA-Ile), (B) abscisic acid (ABA), (C) jasmonic acid (JA), and (D) salicylic acid (SA) (ng per 1 g of plant dry weight) measured in control (C), susceptible (S), and resistant (R) plants. Error bars represent the standard error of the mean. Significant differences between control and inoculated plants, using Student's t-test, are indicated by an asterisk (*p-value < 0.05). (E) Differential expression of hormone responsive genes in resistant (R) and susceptible (S) plants, compared to controls. For each gene annotation, the average of the log2(fold change) is represented. Error bars represent the standard error of the mean. For more details about the genes used and respective functional annotations see Supplementary Table 9.
Consistent with these data, JA induced transcription factors, such as ethylene response factors (ERF), MYC2 and the negative regulators JAZ/Tify were upregulated in all inoculated plants (Supplementary Figure 4). However, 25 chitinase, 3 PR-4, and 16 PR-5 genes, usually associated with JA response (Davis et al., 2002; Piggott et al., 2004), were more strongly upregulated in resistant plants (Figures 7A–C).
Figure 7. Differential expression of PR and chitinase genes. For each gene family, the average of the log2(fold change) is represented for resistant (R) and susceptible (S) plants, compared to controls. Error bars represent the standard error of the mean. (A) PR-4, 3 genes; (B) chitinase, 25 genes; (C) PR-5, 16 genes; (D) PR-1, 4 genes. For more details about the genes used and respective functional annotations see Supplementary Table 9.
Abscisic acid may act synergistically with JA in the activation of the MYC branch of JA response (Pieterse et al., 2011). In turn, JA induces the expression of PYL4, which encodes for an ABA receptor (Lackman et al., 2011). In our results, we observed the upregulation of PYL4 concomitantly with PP2C (Merlot et al., 2001), an ABA signaling repressor gene (Figure 6E), only in resistant plants. Five transcription factors of the NAC family, implicated in ABA–JA interactions (Pieterse et al., 2011), were upregulated in all inoculated plants, although with higher intensity in resistant ones (Supplementary Figure 4). Despite these differences in gene expression, the amount of ABA measured in the samples was similar between control and inoculated plants, with resistant plants tending to have a smaller amount (Figure 6B).
Genes encoding for proteins that induce the synthesis and accumulation of SA, namely EDS1, PAD4, and SAG101 (Wiermer et al., 2005; Caarls et al., 2015), were more expressed in susceptible plants (Figure 6E). On the other hand, a gene encoding for the SA signaling suppressor MKS1 (Andreasson et al., 2005; Pieterse et al., 2011) was more upregulated in resistant plants (Figure 6E). In accordance with these results, the induction of the SA response in susceptible plants was validated by quantifying SA levels, which was higher in these plants than in controls and resistant plants (Figure 6D).
Several WRKY transcription factors, involved in the SA response pathway (Caarls et al., 2015), were upregulated in both susceptible and resistant plants, with a few showing higher expression in the susceptible ones (e.g., unigene36207-WRKY23, isotig49008-WRKY50) (Supplementary Figure 4). In addition, the SA responsive pathogenesis-related protein 1 (PR-1) genes were also upregulated in all inoculated plants (Figure 7D). Although not significantly different, there seems to be a tendency for higher expression of PR-1 genes in susceptible plants.
The analysis with DRAGO 2 identified a set of genes that encode for the characteristic domains of proteins described in the literature as having a role in resistance to pathogens (Osuna-Cruz et al., 2017), including RLK, RLP, protein kinases, and nucleotide-binding domain leucine-rich repeat (NLR) proteins (Table 1, Supplementary Table 8). The RLP differ from RLK by the presence of a kinase domain and genes in which this domain was not detected were classified as RLPs. Several RLPs and RLKs were more expressed in resistant plants (e.g., unigene148155-IRL6, DN63749_c0_g2_i1-RLK, unigene73543-PIRL3) (Table 1, Supplementary Table 8), while others were more expressed in susceptible plants (e.g., isotig67777-FLS2, unigene102513-RLP30, isotig84710-PXC2). Most genes encoding intracellular receptors NLRs had higher expression levels in resistant plants (Table 1).
A significant reprogramming of gene expression was observed in P. pinaster plants after inoculation with the PWN. This observation is not surprising, and it is in accordance with previous studies on P. pinaster inoculated with PWN (Santos and Vasconcelos, 2012; Gaspar et al., 2017, 2020) where susceptible plants have been analyzed. However, by focusing on both resistant and susceptible interactions in P. pinaster plants, we show here that although part of the transcriptional response to PWN was shared between both resistant and susceptible groups, significant qualitative and quantitative differences exist in gene expression. Importantly, some of these differences were confirmed to translate into relevant functional outcomes.
Several possible mechanisms involved in PWN resistance in P. pinaster are here described. Some clear differences in P. pinaster resistant and susceptible transcriptional responses were visible at 72 hpi, highlighting the activation of different phytohormone pathways, contrasting expression of resistance genes, lignin biosynthesis and, possibly, different levels of synthesis of other secondary metabolites. The induction of JA or SA in resistant and susceptible plants, respectively, can be pivotal to determine if the plant defense response is effective against PWN.
The synthesis and accumulation of SA is induced by EDS1 and its interacting proteins, PAD4 and SAG101, which have also a role in repressing the JA pathway (Wiermer et al., 2005; Pieterse et al., 2011; Zhang and Li, 2019). Genes encoding for these proteins were more upregulated in susceptible plants, suggesting an activation of SA pathway at 72 hpi. At the same time, MKS1, which encodes for a protein that can repress SA signaling (Andreasson et al., 2005), was more expressed in resistant plants. This indicates that the activation of SA immune response at the studied time point may be characteristic of a susceptible response. In fact, levels of SA were higher in susceptible plants compared to resistant and controls, supporting this hypothesis.
Salicylic acid and jasmonic acid immune responses are often antagonistic, with SA pathway being mostly associated with response to biotrophic pathogens and JA pathway with response to necrotrophic pathogens and herbivory (Dar et al., 2015). Although JA and JA-Ile levels were similar in resistant and susceptible plants, SA may still inhibit JA response in susceptible plants, independently of JA biosynthesis (Caarls et al., 2015). The repression of JA pathway in susceptible plants, or activation in resistant plants, is supported by a higher expression of JA responsive genes in the latter. These include chitinase, PR-4, PR-5, JAZ/Tify transcription factors, and JA biosynthesis genes (Wasternack and Hause, 2013). Therefore, SA/JA antagonism may play a role in the outcome to PWN inoculation in P. pinaster, with the activation of SA pathway in susceptible plants and JA pathway in resistant ones, at 72hpi. In a recent study where hormone levels were analyzed for another P. pinaster family described in Carrasquinho et al. (2018), high levels of SA and jasmonic acid methyl ester (JA-ME) were detected in susceptible plants at 72 hpi (Rodrigues et al., 2021), supporting an important role for SA in PWN susceptibility. In P. thurnbergii, the induction of JA and SA responsive genes was also observed in susceptible plants (Hirao et al., 2012). Therefore, this hormonal response seems to be shared not only among P. pinaster families, but also susceptible pine species.
The role of ABA in plant immunity seems to be an ambivalent one (Ton et al., 2009). In some interactions, ABA can inhibit SA and JA/ET response (Lorenzo et al., 2004; Adie et al., 2007; Nahar et al., 2012; Hillwig et al., 2016), while in others it enhances JA response against fungi or herbivory (Ton and Mauch-Mani, 2004; Bodenhausen and Reymond, 2007; Ton et al., 2009; Liu et al., 2020b), activating the MYC branch of JA pathway (Pieterse et al., 2011; Vos et al., 2019). In this work, the similar levels of ABA seen in both inoculated and control plants suggests it does not play a part in defense response to PWN, as it was concluded for P. pinaster response to Fusarium circinatum (Hernandez-Escribano et al., 2020). However, the overexpression of both a positive regulator of ABA response, PYL4, and a repressor of ABA signaling, PP2C (Lackman et al., 2011), in resistant plants seems to indicate some role for the ABA pathway in the early stages of the infection. PYL4 is a receptor that recognizes ABA, activating ABA signaling pathway, and has been implicated in the crosstalk between ABA and JA during stress response (Lackman et al., 2011). Furthermore, PYL4 induces the expression of both ABA signaling pathway genes, such as PP2C, and JA signaling pathway genes, such as MYC2 or JAZ TFs (Liu et al., 2020a). In this way, the upregulation of PYL4 may lead to the activation of ABA pathway independently of ABA accumulation.
The expression of PR and chitinase genes is commonly induced by defense response phytohormones (Van Loon et al., 2006; Pieterse et al., 2011). In this work, a higher expression of several chitin-binding PR-4 and chitinase encoding genes was observed in resistant plants. As chitin is a component of nematode eggshell (Fukushige and Futai, 1985; Holbein et al., 2016), these chitinases may compromise egg integrity and embryo development. In the RKN Meloidogyne hapla, treatments with chitinase plant extracts caused premature egg hatching and increased juvenile mortality (Mercer et al., 1992). It would be interesting to see if chitinase extracts from resistant P. pinaster plants have similar effects in PWM.
Several DEGs were identified as putative resistance genes. Interestingly, for many of these, different patterns of expression were detected in resistant and susceptible plants, emphasizing the differences between resistant and susceptible immune responses. For instance, the upregulation of a FLS2 and a RLP30 only in susceptible plants seem to reflect the activation of the SA pathway in these plants, since these genes have been described as SA responsive (Zhang and Li, 2019). On the other hand, the higher expression of NLR receptors in resistant plants may lead to the recognition of PWN effectors. Several studies have previously shown relevant roles for NRL receptors in plant resistance to parasitic nematodes (Sato et al., 2019; Zheng et al., 2021) and herbivorous insects (Hogenhout and Bos, 2011; Erb and Reymond, 2019). For instance, the NLR receptor encoded by gene Mi-1.2 confers resistance to tomato root-knot nematodes (Meloidogyne spp.) (Milligan et al., 1998), the potato aphid (Macrosiphum euphorbiae) (Rossi et al., 1998), the white fly (Bemisia tabaci) (Nombela et al., 2003), and the tomato psyllid (Bactericerca cockerelli) (Casteel et al., 2006). In the same way, it is plausible that PWN delivers effectors to the plant cell cytoplasm while feeding through the stylet. The recognition of these effectors by NLR receptors could induce a stronger defense response in resistant plants.
Secondary metabolites can be induced both by SA or JA, and their importance in plant defense response is well-established (Dar et al., 2015), particularly in conifer trees (e.g., Martin et al., 2002; Zhao et al., 2004; Zeneli et al., 2006; Moreira et al., 2009; Zulak et al., 2009; Zas et al., 2014). The overexpression of genes involved in the synthesis of secondary metabolites was induced by PWN inoculation in several pine species (Shin et al., 2009; Xu et al., 2013; Gaspar et al., 2017, 2020), particularly in resistant varieties (Kuroda et al., 2011; Hirao et al., 2012; Liu et al., 2017). In this work, similar results were obtained, with the induction of several genes involved in the phenylpropanoid biosynthesis pathway, including flavonoid or lignin biosynthesis, and the induction of a few genes involved in terpenoid biosynthesis.
The synthesis of terpene compounds has been implicated in resistance to several pests in pine species (Keeling and Bohlmann, 2006) and seems to be induced by JAs, including in P. pinaster (Moreira et al., 2009; Sampedro et al., 2011; Zas et al., 2014). For instance, specific diterpenes produced by AS and LPS, encoded by two genes that were here more expressed in P. pinaster resistant plants after PWN inoculation, were associated with Pinus resinosa resistance to bark beetle (Mason et al., 2017). An increased expression of terpene synthase genes was also observed in resistant P. massoniana plants in response to PWN (Liu et al., 2017), and the products of two of these enzymes, α-pinene and longifolene, directly inhibited the survival rate of PWN in vitro (Liu et al., 2020a). Other terpenoid compounds found in the resistant species Pinus strobus and Pinus palustris had nematicidal or repelling effect on PWN (Suga et al., 1993). In P. pinaster, plants can be grouped into several chemotypes according to the constitutive content in terpenoid compounds (Rodrigues et al., 2017; Gonçalves et al., 2020). Furthermore, feeding by the PWN insect vector M. galloprovincialis induced an increased production of these compounds in different patterns for the studied chemotypes, including α-pinene and longifolene (Gonçalves et al., 2020). The impact of these chemotypes on PWN resistance is, however, unknown and it would be relevant to investigate it. As synthesis of terpenes seems to be an effective and conserved response to herbivory, and more precisely to PWN, in several conifer species, distinct levels of specific compounds may have a significant impact on nematode survival in resistant P. pinaster plants.
Higher induction of the flavonoid biosynthesis pathway has consistently been found in nematode resistant varieties of several plants species (Chin et al., 2018). For instance, chalcone synthase (CHS) encoding genes were frequently more expressed in resistant plants (Chin et al., 2018) and the product of these enzymes, naringenin, caused reduced egg hatching in the burrowing nematode (Wuyts et al., 2006). In turn, LDOX is involved in the synthesis of another compound with a similar effect, kaempferol, and quercetin, which repelled root-knot nematode and burrowing nematode juveniles (Wuyts et al., 2006). As these flavonoids can affect nematode egg development, nematode mobility, and survival (Chin et al., 2018), the higher expression of genes encoding for CHS and LDOX enzymes in resistant P. pinaster plants may impact PWN and contribute to the observed phenotype. In P. densiflora, a higher expression of flavonoid biosynthesis pathway genes was also observed in resistant varieties (Kuroda et al., 2011).
The phenylpropanoid pathway was the secondary metabolism pathway more highly induced by PWN inoculation in P. pinaster, with special emphasis in the lignin biosynthesis pathway. Several genes specific to lignin synthesis, such as PER and LAC genes, had high expression levels in resistant plants and we were able to show that the higher gene expression translated into a significant increase in cell wall lignin content. The upregulation of PER genes and genes involved in cell wall strengthening was also associated with resistance in P. thunbergii (Hirao et al., 2012) and P. massoniana (Liu et al., 2017). Furthermore, higher lignification in regions surrounding plant tissue damaged by PWN has been observed in resistant P. thunbergii plants and associated with limited PWN migration (Kusumoto et al., 2014). Our results support that lignification seems to be an efficient strategy to reduce the spread of PWN, consequent plant tissue damage and likely to interfere with nematode feeding on plant cells (Naoumkina et al., 2010; Holbein et al., 2016).
In our data, it was possible to detect PWN gene expression during the infection process important for the successful infestation of plant tissues. Among these, were genes encoding for antioxidant proteins, which protect PWN from ROS produced by the plant during defense response (Espada et al., 2016). Resistance to oxidative stress has been positively correlated with PWN virulence (Vicente et al., 2015), suggesting that detoxification is essential for successful infection. As above mentioned, the expression of genes encoding the hydrogen peroxide producing enzymes GLOX, possibly involved in increased lignification, was higher in resistant plants. The production of higher amounts of this toxic compound in resistant plants may surpass the PWN capacity for detoxification and negatively influence the nematode performance. In inoculated P. massoniana plants, levels of hydrogen peroxide were slightly higher in resistant plants at 24 hpi (Liu et al., 2017), but no data was collected at 72 hpi. At 15 dpi, levels were reversed, being significantly lower in resistant plants, which was associated with a higher expression of oxidative stress response genes (Liu et al., 2017). In P. pinaster, as well as in P. thunbergii (Hirao et al., 2012), oxidative stress response genes were also more expressed in resistant plants, indicating that a better protection from oxidative damage is important for PWN resistance in several Pinus ssp.
Investigation of P. pinaster defense response to PWN inoculation in resistant plants has not been previously reported. Combining differential gene expression analysis with hormone and lignin quantification, we identified pathways and mechanisms potentially involved in PWN resistance. The induction of different hormone pathways, namely the SA pathway in susceptible plants vs. the JA pathway in resistant plants, and the higher lignification of plant tissues around the inoculation zone in resistant plants seem to be of great relevance for the phenotypic outcome after inoculation. Secondary metabolism pathways, resistance genes, and oxidative stress response genes also seem to play an important role in PWN resistance. The high expression of these groups of genes in resistant plants may interfere with nematode feeding, survival, mobility, and reproduction. This study provides the foundation to understand PWN resistance in P. pinaster, highlighting a set of candidate genes greatly relevant for future functional characterization studies. The use of compounds here associated with resistance, such as JA and secondary metabolites, for pest-management strategies has been previously suggested (e.g., Erbilgin et al., 2006; Goławska et al., 2014; Zas et al., 2014; Scalerandi et al., 2018) and should be explored.
Overall, the implication of several distinct pathways in the resistance of P. pinaster to PWN is in accordance with the quantitative nature of the resistance trait and the observation of intermediate symptoms from susceptibility to complete resistance in this study and in previous reports (Menéndez-Gutiérrez et al., 2017a; Carrasquinho et al., 2018). The search for genetic variation in the candidate genes here identified using high-throughput genotyping technologies, further supported by their possible co-location with quantitative trait loci (QTLs) currently under investigation in a larger number of families, may provide relevant molecular markers for identification of resistant genotypes. These approaches will greatly aid selection of individuals from the most resistant families to be used in current breeding or vegetative propagation programs.
The original contributions presented in the study are publicly available. The sequence data for this study has been submitted to the European Nucleotide Archive (ENA) at EMBL EBI under accession number PRJEB26836 (https://www.ebi.ac.uk/ena/browser/view/PRJEB26836).
IM, IC, and CM designed the experiment and performed the inoculation assay. IM performed the RNA experiments. VA and AG-C performed the hormone quantification experiment. IM performed data analysis, guided and supervised by LS and YV. IM and CM interpreted data and prepared the manuscript. All authors discussed the results and reviewed the manuscript.
This work was supported by Fundação para a Ciência e a Tecnologia (FCT), through grants GREEN-it (UID/Multi/04551/2013), BioISI (UIDB/04046/2020 and UIDP/04046/2020), IF/01168/2013, and the doctoral fellowship SFRH/BD/111687/2015 (to IM). Support was also provided by project PTDC/BAA-MOL/28379/2017—LISBOA-01-0145-FEDER-028379 (FCT/MCTES and FEDER).
The authors declare that the research was conducted in the absence of any commercial or financial relationships that could be construed as a potential conflict of interest.
We thank Dr. Lurdes Inácio (INIAV) for providing the nematode cultures, Hugo Matias (ITQB NOVA) for all the technical support in the greenhouse, and the Biopolymer Analytical Platform at Swedish University of Agricultural Sciences, Sweden for the lignin quantification analysis.
The Supplementary Material for this article can be found online at: https://www.frontiersin.org/articles/10.3389/fpls.2021.690857/full#supplementary-material
Supplementary Figure 1. Boxplots of the height and diameter at the base of the stem of inoculated plants (half-sib family 440) and t-test results for the comparison of these parameters' means between resistant (res) and susceptible (sus) plants. (A) Boxplot of height (cm) measurements. (B) Boxplot of diameter at the base of the stem (mm) measurements. Both measurements were made before inoculations. (C) t-Test results for heights comparison. (D) t-Test results for diameter comparison. N, number of samples. SD, standard deviation.
Supplementary Figure 2. Heatmaps representing the expression patterns of genes involved in secondary metabolism. (A) Flavonoid biosynthesis pathway. (B) Terpenoid biosynthesis pathways, including terpenoid backbone biosynthesis (Terp. Backbone), monoterpenoid biosynthesis, sesquiterpenoid biosynthesis, and diterpenoid biosynthesis pathways. The color gradient represents mean expression levels (logTPM) of each gene for control (C), susceptible (S), and resistant (R) samples.
Supplementary Figure 3. Heatmaps representing the expression patterns of genes involved in the synthesis of hydrogen peroxide (A) and response to oxidative stress (B). The color gradient represents mean expression levels (logTPM) of each gene for control (C), susceptible (S), and resistant (R) samples.
Supplementary Figure 4. Heatmaps representing the expression patterns of hormone responsive transcription factors (TFs). Jasmonate responsive TFs JAZ/Tify (A) and ERF (B), salicylic acid responsive TFs WRKY (C), and abscisic acid responsive TFs NAC (D). The color gradient represents mean expression levels (logTPM) of each gene for control (C), susceptible (S), and resistant (R) samples.
Supplementary Table 1. De novo assembly and P. pinaster reference transcriptome statistics.
Supplementary Table 2. Genes selected for quantitative RT-qPCR, respective primer sequences, amplicon size, and annealing temperatures used.
Supplementary Table 3. Genes expressed by Bursaphelenchus xylophilus in inoculated samples.
Supplementary Table 4. Differential expressed genes in susceptible plants when compared to controls (log2 fold change ≥ |2|, FDR corrected p-value (padj) ≤ 0.05). InterPro, KEGG, and blastx annotations are presented.
Supplementary Table 5. Differential expressed genes in resistant plants when compared to controls (log2 fold change ≥ |2|, FDR corrected p-value (padj) ≤ 0.05). InterPro, KEGG, and blastx annotations are presented.
Supplementary Table 6. GO terms enriched in the upregulated genes in susceptible samples when compared with controls, after trimming for redundancy.
Supplementary Table 7. GO terms enriched in the upregulated genes in resistant samples when compared with controls, after trimming for redundancy.
Supplementary Table 8. Complete list of genes annotated with DRAGO 2 tool.
Supplementary Table 9. Genes used for calculating average log2(fold change) expression levels for Figures 5, 6.
Data Sheet 1. Fasta file with de novo assembled Pinus pinaster transcripts.
Adie, B., Chico, J. M., Rubio-Somoza, I., and Solano, R. (2007). Modulation of plant defenses by ethylene. J. Plant Growth Regul. 26, 160–177. doi: 10.1007/s00344-007-0012-6
Ahuja, I., Kissen, R., and Bones, A. M. (2012). Phytoalexins in defense against pathogens. Trends Plant Sci. 17, 73–90. doi: 10.1016/j.tplants.2011.11.002
Andreasson, E., Jenkins, T., Brodersen, P., Thorgrimsen, S., Petersen, N. H. T., Zhu, S., et al. (2005). The MAP kinase substrate MKS1 is a regulator of plant defense responses. EMBO J. 24, 2579–2589. doi: 10.1038/sj.emboj.7600737
Andrews, S. (2010). FastQC: A Quality Control Tool for High Throughput Sequence Data. Available online at: http://www.bioinformatics.babraham.ac.uk/projects/fastqc
Bodenhausen, N., and Reymond, P. (2007). Signaling pathways controlling induced resistance to insect herbivores in Arabidopsis. Mol. Plant Microbe Interact. 20, 1406–1420. doi: 10.1094/MPMI-20-11-1406
Buscaill, P., and Rivas, S. (2014). Transcriptional control of plant defence responses. Curr. Opin. Plant Biol. 20, 35–46. doi: 10.1016/j.pbi.2014.04.004
Caarls, L., Pieterse, C. M. J., and Van Wees, S. C. M. (2015). How salicylic acid takes transcriptional control over jasmonic acid signaling. Front. Plant Sci. 6:170. doi: 10.3389/fpls.2015.00170
Cañas, R. A., Li, Z., Pascual, M. B., Castro-Rodríguez, V., Ávila, C., Sterck, L., et al. (2017). The gene expression landscape of pine seedling tissues. Plant J. 91, 1064–1087. doi: 10.1111/tpj.13617
Carrasquinho, I., Lisboa, A., Inácio, M. L., and Gonçalves, E. (2018). Genetic variation in susceptibility to pine wilt disease of maritime pine half-sib families. Ann. For. Sci. 75:85. doi: 10.1007/s13595-018-0759-x
Casteel, C. L., Walling, L. L., and Paine, T. D. (2006). Behavior and biology of the tomato psyllid, Bactericerca cockerelli, in response to the Mi-1.2 gene. Entomol. Exp. Appl. 121, 67–72. doi: 10.1111/j.1570-8703.2006.00458.x
Chin, S., Behm, C., and Mathesius, U. (2018). Functions of flavonoids in plant–nematode interactions. Plants 7:85. doi: 10.3390/plants7040085
Couto, D., and Zipfel, C. (2016). Regulation of pattern recognition receptor signalling in plants. Nat. Rev. Immunol. 16, 537–552. doi: 10.1038/nri.2016.77
Cui, H., Tsuda, K., and Parker, J. E. (2015). Effector-triggered immunity: from pathogen perception to robust defense. Annu. Rev. Plant Biol. 66, 487–511. doi: 10.1146/annurev-arplant-050213-040012
Dar, T. A., Uddin, M., Khan, M. M. A., Hakeem, K. R., and Jaleel, H. (2015). Jasmonates counter plant stress: a review. Environ. Exp. Bot. 115, 49–57. doi: 10.1016/j.envexpbot.2015.02.010
Davis, J. M., Wu, H., Cooke, J. E. K., Reed, J. M., Luce, K. S., and Michler, C. H. (2002). Pathogen challenge, salicylic acid, and jasmonic acid regulate expression of chitinase gene homologs in pine. Mol. Plant. Microbe. Interact. 15, 380–387. doi: 10.1094/MPMI.2002.15.4.380
de Vega-Bartol, J. J., Santos, R. R., Simões, M., and Miguel, C. M. (2013). Normalizing gene expression by quantitative PCR during somatic embryogenesis in two representative conifer species: Pinus pinaster and Picea abies. Plant Cell Rep. 32, 715–729. doi: 10.1007/s00299-013-1407-4
De Vleesschauwer, D., Xu, J., and Höfte, M. (2014). Making sense of hormone-mediated defense networking: from rice to Arabidopsis. Front. Plant Sci. 5:611. doi: 10.3389/fpls.2014.00611
Dodds, P. N., and Rathjen, J. P. (2010). Plant immunity: towards an integrated view of plant–pathogen interactions. Nat. Rev. Genet. 11, 539–548. doi: 10.1038/nrg2812
Erb, M., and Reymond, P. (2019). Molecular interactions between plants and insect herbivores. Annu. Rev. Plant Biol. 70, 527–557. doi: 10.1146/annurev-arplant-050718-095910
Erbilgin, N., Krokene, P., Christiansen, E., Zeneli, G., and Gershenzon, J. (2006). Exogenous application of methyl jasmonate elicits defenses in Norway spruce (Picea abies) and reduces host colonization by the bark beetle Ips typographus. Oecologia 148, 426–436. doi: 10.1007/s00442-006-0394-3
Espada, M., Silva, A. C., Eves Van Den Akker, S., Cock, P. J. A., Mota, M., and Jones, J. T. (2016). Identification and characterization of parasitism genes from the pinewood nematode Bursaphelenchus xylophilus reveals a multilayered detoxification strategy. Mol. Plant Pathol. 17, 286–295. doi: 10.1111/mpp.12280
Evans, H. F., McNamara, D. G., Braasch, H., Chadoeuf, J., and Magnusson, C. (1996). Pest Risk Analysis (PRA) for the territories of the European Union (as PRA area) on Bursaphelenchus xylophilus and its vectors in the genus Monochamus. EPPO Bull. 26, 199–249. doi: 10.1111/j.1365-2338.1996.tb00594.x
Eyles, A., Bonello, P., Ganley, R., and Mohammed, C. (2010). Induced resistance to pests and pathogens in trees. New Phytol. 185, 893–908. doi: 10.1111/j.1469-8137.2009.03127.x
Foster, C. E., Martin, T. M., and Pauly, M. (2010). Comprehensive compositional analysis of plant cell walls (Lignocellulosic biomass) part I: lignin. J. Vis. Exp. 2010:1745. doi: 10.3791/1745
Fukushige, H., and Futai, K. (1985). Characteristics of egg shells and the morphology of female tail-tips of Bursaphelenchus xylophilus, B. mucronatus and some strains of related species from France. Japanese J. Nematol. 15, 49–54.
Futai, K., and Furuno, T. (1979). The variety of resistances among pine species to pine wood nematode, Bursaphelenchus lignicolus. Bull. Kyoto Univ. 51, 23–36.
Gaspar, D., Trindade, C., Usié, A., Meireles, B., Barbosa, P., Fortes, A., et al. (2017). Expression profiling in Pinus pinaster in response to infection with the pine wood nematode Bursaphelenchus xylophilus. Forests 8:279. doi: 10.3390/f8080279
Gaspar, D., Trindade, C., Usié, A., Meireles, B., Fortes, A. M., Guimarães, J. B., et al. (2020). Comparative transcriptomic response of two pinus species to infection with the pine wood nematode Bursaphelenchus xylophilus. Forests 11:204. doi: 10.3390/f11020204
Goławska, S., Sprawka, I., Łukasik, I., and Goławski, A. (2014). Are naringenin and quercetin useful chemicals in pest-management strategies? J. Pest Sci. (2004). 87, 173–180. doi: 10.1007/s10340-013-0535-5
Gonçalves, E., Figueiredo, A. C., Barroso, J. G., Henriques, J., Sousa, E., and Bonifácio, L. (2020). Effect of Monochamus galloprovincialis feeding on Pinus pinaster and Pinus pinea, oleoresin and insect volatiles. Phytochemistry 169:112159. doi: 10.1016/j.phytochem.2019.112159
Goverse, A., and Smant, G. (2014). The activation and suppression of plant innate immunity by parasitic nematodes. Annu. Rev. Phytopathol. 52, 243–265. doi: 10.1146/annurev-phyto-102313-050118
Grabherr, M. G., Haas, B. J., Yassour, M., Levin, J. Z., Thompson, D. A., Amit, I., et al. (2013). Trinity: reconstructing a full-length transcriptome without a genome from RNA-Seq data. Nat. Biotechnol. 29, 644–652. doi: 10.1038/nbt.1883.Trinity
Haas, B. J. (2019). Transdecoder. Available online at: https://transdecoder.github.io
Haegeman, A., Mantelin, S., Jones, J. T., and Gheysen, G. (2012). Functional roles of effectors of plant-parasitic nematodes. Gene 492, 19–31. doi: 10.1016/j.gene.2011.10.040
Hernandez-Escribano, L., Visser, E. A., Iturritxa, E., Raposo, R., and Naidoo, S. (2020). The transcriptome of Pinus pinaster under Fusarium circinatum challenge. BMC Genomics 21:28. doi: 10.1186/s12864-019-6444-0
Hillwig, M. S., Chiozza, M., Casteel, C. L., Lau, S. T., Hohenstein, J., Hernández, E., et al. (2016). Abscisic acid deficiency increases defence responses against Myzus persicae in Arabidopsis. Mol. Plant Pathol. 17, 225–235. doi: 10.1111/mpp.12274
Hirao, T., Fukatsu, E., and Watanabe, A. (2012). Characterization of resistance to pine wood nematode infection in Pinus thunbergii using suppression subtractive hybridization. BMC Plant Biol. 12:13. doi: 10.1186/1471-2229-12-13
Hogenhout, S. A., and Bos, J. I. B. (2011). Effector proteins that modulate plant-insect interactions. Curr. Opin. Plant Biol. 14, 422–428. doi: 10.1016/j.pbi.2011.05.003
Holbein, J., Grundler, F. M. W., and Siddique, S. (2016). Plant basal resistance to nematodes: an update. J. Exp. Bot. 67:2049–61. doi: 10.1093/jxb/erw005
Ichihara, Y., Fukuda, K., and Suzuki, K. (2000). Early symptom development and histological changes associated with migration of Bursaphelenchus xylophilus in seedling tissues of Pinus thunbergii. Plant Dis. 84, 675–680. doi: 10.1094/PDIS.2000.84.6.675
Keeling, C. I., and Bohlmann, J. (2006). Genes, enzymes and chemicals of terpenoid diversity in the constitutive and induced defence of conifers against insects and pathogens. New Phytol. 170, 657–675. doi: 10.1111/j.1469-8137.2006.01716.x
Kikuchi, T., Cotton, J. A., Dalzell, J. J., Hasegawa, K., Kanzaki, N., McVeigh, P., et al. (2011). Genomic insights into the origin of parasitism in the emerging plant pathogen Bursaphelenchus xylophilus. PLoS Pathog. 7:e1002219. doi: 10.1371/journal.ppat.1002219
Kim, B. N., Kim, J. H., Ahn, J. Y., Kim, S., Cho, B. K., Kim, Y. H., et al. (2020). A short review of the pinewood nematode, Bursaphelenchus xylophilus. Toxicol. Environ. Health Sci. 12:1–8 doi: 10.1007/s13530-020-00068-0
Kuroda, H., Goto, S., Kazumi, E., and Kuroda, K. (2011). The expressed genes of Japanese red pine (Pinus densiflora) involved in the pine wilt disease severity. BMC Proc. 5:P92. doi: 10.1186/1753-6561-5-S7-P92
Kusumoto, D., Yonemichi, T., Inoue, H., Hirao, T., Watanabe, A., and Yamada, T. (2014). Comparison of histological responses and tissue damage expansion between resistant and susceptible Pinus thunbergii infected with pine wood nematode Bursaphelenchus xylophilus. J. For. Res. 19, 285–294. doi: 10.1007/s10310-013-0417-y
Lackman, P., González-Guzmán, M., Tilleman, S., Carqueijeiro, I., Pérez, A. C., Moses, T., et al. (2011). Jasmonate signaling involves the abscisic acid receptor PYL4 to regulate metabolic reprogramming in Arabidopsis and tobacco. Proc. Natl. Acad. Sci. U.S.A. 108, 5891–5896. doi: 10.1073/pnas.1103010108
Le Provost, G., Herrera, R., Paiva, J. A., Chaumeil, P., Salin, F., and Plomion, C. (2007). A micromethod for high throughput RNA extraction in forest trees. Biol. Res. 40, 291–297. doi: 10.4067/S0716-97602007000400003
Li, H. (2013). Aligning sequence reads, clone sequences and assembly contigs with BWA-MEM. arXiv:1303.3997v2 [q-bio.GN], 1–3.
Li, H., Handsaker, B., Wysoker, A., Fennell, T., Ruan, J., Homer, N., et al. (2009). The sequence alignment/map format and SAMtools. Bioinformatics 25, 2078–2079. doi: 10.1093/bioinformatics/btp352
Liu, B., Liu, Q., Zhou, Z., Yin, H., Xie, Y., and Wei, Y. (2020a). Two terpene synthases in resistant Pinus massoniana contribute to defence against Bursaphelenchus xylophilus. Plant Cell Environ. 44, 257–274. doi: 10.1111/pce.13873
Liu, L., Liu, C. Y., Wang, H., Yu, S. Y., Guan, T. S., Huang, Y. F., et al. (2020b). The abscisic acid receptor gene VvPYL4 positively regulates grapevine resistance to Plasmopara viticola. Plant Cell. Tissue Organ Cult. 142, 483–492. doi: 10.1007/s11240-020-01872-9
Liu, Q., Wei, Y., Xu, L., Hao, Y., Chen, X., and Zhou, Z. (2017). Transcriptomic profiling reveals differentially expressed genes associated with pine wood nematode resistance in masson pine (Pinus massoniana Lamb.). Sci. Rep. 7, 1–14. doi: 10.1038/s41598-017-04944-7
Lorenzo, O., Chico, J. M., Sánchez-Serrano, J. J., and Solano, R. (2004). JASMONATE-INSENSITIVE1 encodes a MYC transcription factor essential to discriminate between different jasmonate-regulated defense responses in Arabidopsis. Plant Cell 16, 1938–1950. doi: 10.1105/tpc.022319
Love, M. I., Huber, W., and Anders, S. (2014). Moderated estimation of fold change and dispersion for RNA-seq data with DESeq2. Genome Biol. 15:550. doi: 10.1186/s13059-014-0550-8
Lozano-Torres, J. L., Wilbers, R. H. P., Warmerdam, S., Finkers-Tomczak, A., Diaz-Granados, A., van Schaik, C. C., et al. (2014). Apoplastic venom allergen-like proteins of cyst nematodes modulate the activation of basal plant innate immunity by cell surface receptors. PLoS Pathog. 10:e1004569. doi: 10.1371/journal.ppat.1004569
Maere, S., Heymans, K., and Kuiper, M. (2005). BiNGO: a cytoscape plugin to assess overrepresentation of gene ontology categories in biological networks. Bioinformatics 21, 3448–3449. doi: 10.1093/bioinformatics/bti551
Marshall, O. J. (2004). PerlPrimer: cross-platform, graphical primer design for standard, bisulphite and real-time PCR. Bioinformatics 20, 2471–2472. doi: 10.1093/bioinformatics/bth254
Martin, D., Tholl, D., Gershenzon, J., and Bohlmann, J. (2002). Methyl jasmonate induces traumatic resin ducts, terpenoid resin biosynthesis, and terpenoid accumulation in developing xylem of norway spruce stems. Plant Physiol. 129, 1003–1018. doi: 10.1104/pp.011001
Mason, C. J., Villari, C., Keefover-Ring, K., Jagemann, S., Zhu, J., Bonello, P., et al. (2017). Spatial and temporal components of induced plant responses in the context of herbivore life history and impact on host. Funct. Ecol. 31, 2034–2050. doi: 10.1111/1365-2435.12911
Menéndez-Gutiérrez, M., Alonso, M., Toval, G., and Díaz, R. (2017a). Testing of selected Pinus pinaster half-sib families for tolerance to pinewood nematode (Bursaphelenchus xylophilus). Forestry 91, 38–48. doi: 10.1093/forestry/cpx030
Menéndez-Gutiérrez, M., Alonso, M., Toval, G., and Díaz, R. (2017b). Variation in pinewood nematode susceptibility among Pinus pinaster Ait. provenances from the Iberian Peninsula and France. Ann. For. Sci. 74:76. doi: 10.1007/s13595-017-0677-3
Mercer, C. F., Greenwood, D. R., and Grant, J. L. (1992). Effect of plant and microbial chitinases on the eggs and juveniles of Meloidogyne hapla Chitwood (Nematoda: Tylenchida). Nematologica 38, 227–236. doi: 10.1163/187529292X00199
Merlot, S., Gosti, F., Guerrier, D., Vavasseur, A., and Giraudat, J. (2001). The ABI1 and ABI2 protein phosphatases 2C act in a negative feedback regulatory loop of the abscisic acid signalling pathway. Plant J. 25, 295–303. doi: 10.1046/j.1365-313X.2001.00965.x
Milligan, S. B., Bodeau, J., Yaghoobi, J., Kaloshian, I., Zabel, P., and Williamson, V. M. (1998). The root knot nematode resistance gene Mi from tomato is a member of the leucine zipper, nucleotide binding, leucine-rich repeat family of plant genes. Plant Cell 10, 1307–1319. doi: 10.1105/tpc.10.8.1307
Moreira, X., Sampedro, L., and Zas, R. (2009). Defensive responses of Pinus pinaster seedlings to exogenous application of methyl jasmonate: concentration effect and systemic response. Environ. Exp. Bot. 67, 94–100. doi: 10.1016/j.envexpbot.2009.05.015
Moriya, Y., Itoh, M., Okuda, S., Yoshizawa, A. C., and Kanehisa, M. (2007). KAAS: an automatic genome annotation and pathway reconstruction server. Nucleic Acids Res. 35, W182–W185. doi: 10.1093/nar/gkm321
Mota, M., Braasch, H., Bravo, M. A., Penas, A. C., Burgermeister, W., Metge, K., et al. (1999). First report of Bursaphelenchus xylophilus in Portugal and in Europe. Nematology 1, 727–734. doi: 10.1163/156854199508757
Nahar, K., Kyndt, T., Nzogela, Y. B., and Gheysen, G. (2012). Abscisic acid interacts antagonistically with classical defense pathways in rice – migratory nematode interaction. New Phytol. 196, 901–913. doi: 10.1111/j.1469-8137.2012.04310.x
Naoumkina, M. A., Zhao, Q., Gallego-Giraldo, L., Dai, X., Zhao, P. X., and Dixon, R. A. (2010). Genome-wide analysis of phenylpropanoid defence pathways. Mol. Plant Pathol. 11, 829–846. doi: 10.1111/j.1364-3703.2010.00648.x
Nombela, G., Williamson, V. M., and Muñiz, M. (2003). The root-knot nematode resistance gene Mi-1.2 of tomato is responsible for resistance against the whitefly Bemisia tabaci. Mol. Plant Microbe Interact. 16, 645–649. doi: 10.1094/MPMI.2003.16.7.645
Nose, M., and Shiraishi, S. (2011). Comparison of the gene expression profiles of resistant and non-resistant Japanese black pine inoculated with pine wood nematode using a modified LongSAGE technique. For. Pathol. 41, 143–155. doi: 10.1111/j.1439-0329.2010.00646.x
Osuna-Cruz, C. M., Paytuvi-Gallart, A., Di Donato, A., Sundesha, V., Andolfo, G., Aiese Cigliano, R., et al. (2017). PRGdb 3.0: a comprehensive platform for prediction and analysis of plant disease resistance genes. Nucleic Acids Res. 46, D1197–D1201. doi: 10.1093/nar/gkx1119
Pascual, M. B., Cánovas, F. M., and Ávila, C. (2015). The NAC transcription factor family in maritime pine (Pinus pinaster): molecular regulation of two genes involved in stress responses. BMC Plant Biol. 15:254. doi: 10.1186/s12870-015-0640-0
Pfaffl, M. W. (2001). A new mathematical model for relative quantification in real-time RT-PCR. Nucleic Acids Res. 29:45. doi: 10.1093/nar/29.9.e45
Pieterse, C. M. J., Van der Does, D., Zamioudis, C., Leon-Reyes, A., and Van Wees, S. C. M. (2011). Hormonal modulation of plant immunity. Annu. Rev. Cell Dev. Biol. 28, 489–521. doi: 10.1146/annurev-cellbio-092910-154055
Piggott, N., Ekramoddoullah, A. K. M., Liu, J. J., and Yu, X. (2004). Gene cloning of a thaumatin-like (PR-5) protein of western white pine (Pinus monticola D. Don) and expression studies of members of the PR-5 group. Physiol. Mol. Plant Pathol. 64, 1–8. doi: 10.1016/j.pmpp.2004.05.004
Ribeiro, B., Espada, M., Vu, T., Nóbrega, F., Mota, M., and Carrasquinho, I. (2012). Pine wilt disease: detection of the pinewood nematode (Bursaphelenchus xylophilus) as a tool for a pine breeding programme. For. Pathol. 42, 521–525. doi: 10.1111/efp.12010
Rodrigues, A. M., Langer, S., Carrasquinho, I., Bergström, E., Larson, T., Thomas-Oates, J., et al. (2021). Pinus pinaster early hormonal defence responses to pinewood nematode (Bursaphelenchus xylophilus) infection. Metabolites 11:111. doi: 10.3390/metabo11040227
Rodrigues, A. M., Mendes, M. D., Lima, A. S., Barbosa, P. M., Ascens, L., Pedro, L. G., et al. (2017). Pinus halepensis, Pinus pinaster, Pinus pinea and Pinus sylvestris essential oils chemotypes and monoterpene hydrocarbon enantiomers, before and after inoculation with the pinewood nematode Bursaphelenchus xylophilus. Chem. Biodivers. 14:e1600153. doi: 10.1002/cbdv.201600153
Rossi, M., Goggin, F. L., Milligan, S. B., Kaloshian, I., Ullman, D. E., and Williamson, V. M. (1998). The nematode resistance gene Mi of tomato confers resistance against the potato aphid. Proc. Natl. Acad. Sci. U.S.A. 95, 9750–9754. doi: 10.1073/pnas.95.17.9750
Sampedro, L., Moreira, X., and Zas, R. (2011). Resistance and response of Pinus pinaster seedlings to Hylobius abietis after induction with methyl jasmonate. Plant Ecol. 212, 397–401. doi: 10.1007/s11258-010-9830-x
Santos, C. S., Pinheiro, M., Silva, A. I., Egas, C., and Vasconcelos, M. W. (2012). Searching for resistance genes to Bursaphelenchus xylophilus using high throughput screening. BMC Genomics 13:599. doi: 10.1186/1471-2164-13-599
Santos, C. S. S., and Vasconcelos, M. W. (2012). Identification of genes differentially expressed in Pinus pinaster and Pinus pinea after infection with the pine wood nematode. Eur. J. Plant Pathol. 132, 407–418. doi: 10.1007/s10658-011-9886-z
Sato, K., Kadota, Y., and Shirasu, K. (2019). Plant immune responses to parasitic nematodes. Front. Plant Sci. 10:1165. doi: 10.3389/fpls.2019.01165
Scalerandi, E., Flores, G. A., Palacio, M., Defag,ó, M. T., Carpinella, M. C., Valladares, G., et al. (2018). Understanding synergistic toxicity of terpenes as insecticides: contribution of metabolic detoxification in Musca domestica. Front. Plant Sci. 9:1579. doi: 10.3389/fpls.2018.01579
Shannon, P., Markiel, A., Owen, O.zier 2, Baliga, N. S., Wang, J. T., Ramage, D., et al. (2003). Cytoscape: a software environment for integrated models of biomolecular interaction networks. Genome Res., 2498–2504. doi: 10.1101/gr.1239303
Shin, H., Lee, H., Woo, K. S., Noh, E. W., Koo, Y. B., and Lee, K. J. (2009). Identification of genes upregulated by pinewood nematode inoculation in Japanese red pine. Tree Physiol. 29, 411–421. doi: 10.1093/treephys/tpn034
Shinya, R., Morisaka, H., Kikuchi, T., Takeuchi, Y., Ueda, M., and Futai, K. (2013). Secretome analysis of the pine wood nematode Bursaphelenchus xylophilus reveals the tangled roots of parasitism and its potential for molecular mimicry. PLoS ONE 8:e67377. doi: 10.1371/journal.pone.0067377
Son, J. A., Matsushita, N., and Hogetsu, T. (2015). Migration of Bursaphelenchus xylophilus in cortical and xylem axial resin canals of resistant pines. For. Pathol. 45, 246–253. doi: 10.1111/efp.12164
Suga, T., Ohta, S., Munesada, K., Ide, N., Kurokawa, M., Shimizu, M., et al. (1993). Endogenous pine wood nematicidal substances in pines, Pinus massoniana, P. strobus and P. palustris. Phytochemistry 33, 1395–1401. doi: 10.1016/0031-9422(93)85098-C
Supek, F., Bošnjak, M., Škunca, N., and Šmuc, T. (2011). REVIGO summarizes and visualizes long lists of gene ontology terms. PLoS ONE 6:e21800. doi: 10.1371/journal.pone.0021800
Toda, T., and Kurinobu, S. (2002). Realized genetic gains observed in progeny tolerance of selected red pine (Pinus densiflora) and black pine (P. thunbergii) to pine wilt disease. Silvae Genet. 51, 42–44.
Ton, J., Flors, V., and Mauch-Mani, B. (2009). The multifaceted role of ABA in disease resistance. Trends Plant Sci. 14, 310–317. doi: 10.1016/j.tplants.2009.03.006
Ton, J., and Mauch-Mani, B. (2004). β-amino-butyric acid-induced resistance against necrotrophic pathogens is based on ABA-dependent priming for callose. Plant J. 38, 119–130. doi: 10.1111/j.1365-313X.2004.02028.x
Trudgill, D. L. (1991). Resistance to and tolerance of plant parasitic nematodes in plants. Annu. Rev. Phytopathol. 29, 167–192.
Tsuda, K., and Katagiri, F. (2010). Comparing signaling mechanisms engaged in pattern-triggered and effector-triggered immunity. Curr. Opin. Plant Biol. 13, 459–465. doi: 10.1016/j.pbi.2010.04.006
Van Loon, L. C., Rep, M., and Pieterse, C. M. J. (2006). Significance of inducible defense-related proteins in infected plants. Annu. Rev. Phytopathol. 44, 135–162. doi: 10.1146/annurev.phyto.44.070505.143425
Vicente, C., Espada, M., Vieira, P., and Mota, M. (2012). Pine wilt disease: a threat to european forestry. Eur. J. Plant Pathol. 133, 89–99. doi: 10.1007/s10658-011-9924-x
Vicente, C. S. L., Ikuyo, Y., Shinya, R., Mota, M., and Hasegawa, K. (2015). Catalases induction in high virulence pinewood nematode Bursaphelenchus xylophilus under hydrogen peroxide-induced stress. PLoS ONE 10:e0123839. doi: 10.1371/journal.pone.0123839
Vos, I. A., Verhage, A., Watt, L. G., Vlaardingerbroek, I., Schuurink, R. C., Pieterse, C. M. J., et al. (2019). Abscisic acid is essential for rewiring of jasmonic acid-dependent defenses during herbivory. bioRxiv 2019:1–41. doi: 10.1101/747345
Wasternack, C., and Hause, B. (2013). Jasmonates: biosynthesis, perception, signal transduction and action in plant stress response, growth and development. An update to the 2007 review in Annals of Botany. Ann. Bot. 111, 1021–1058. doi: 10.1093/aob/mct067
Webster, J., and Mota, M. (2008). “Pinewilt disease: global issues, trade and economic impact,” in Pine Wilt Disease: A Worldwide Threat to Forest Ecosystems, eds. M. Mota and P. Vieira (Dordrecht: Springer), 1–4.
Whitehead, A. G., and Hemming, J. R. (1965). A comparison of some quantitative methods of extracting small vermiform nematodes from soil. Ann. Appl. Biol. 55, 25–38. doi: 10.1111/j.1744-7348.1965.tb07864.x
Wiermer, M., Feys, B. J., and Parker, J. E. (2005). Plant immunity : the EDS1 regulatory node. Curr. Opin. Plant Biol. 1, 383–389. doi: 10.1016/j.pbi.2005.05.010
Woodcock, P., Cottrell, J. E., Buggs, R. J. A., and Quine, C. P. (2018). Mitigating pest and pathogen impacts using resistant trees: a framework and overview to inform development and deployment in Europe and North America. Forestry 91, 1–16. doi: 10.1093/forestry/cpx031
Wuyts, N., Swennen, R., and De Waele, D. (2006). Effects of plant phenylpropanoid pathway products and selected terpenoids and alkaloids on the behaviour of the plant-parasitic nematodes Radopholus similis, Pratylenchus penetrans and Meloidogyne incognita. Nematology 8, 89–101. doi: 10.1163/156854106776179953
Xie, M., Zhang, J., Tschaplinski, T. J., Tuskan, G. A., Chen, J. G., and Muchero, W. (2018). Regulation of lignin biosynthesis and its role in growth-defense tradeoffs. Front. Plant Sci. 9:1427. doi: 10.3389/fpls.2018.01427
Xu, L., Liu, Z. Y., Zhang, K., Lu, Q., Liang, J., and Zhang, X. Y. (2013). Characterization of the Pinus massoniana transcriptional response to Bursaphelenchus xylophilus infection using suppression subtractive hybridization. Int. J. Mol. Sci. 14, 11356–11375. doi: 10.3390/ijms140611356
Xu, L.-Y., Zhang, J., Gao, J.-B., Chen, X.-L., Jiang, C.-W., and Hao, Y.-P. (2012). Study on the disease resistance of candidate clones in Pinus massoniana to Bursaphelenchus xylophilus. China For. Sci. Technol. 26, 27–30.
Zas, R., Björklund, N., Nordlander, G., Cendán, C., Hellqvist, C., and Sampedro, L. (2014). Exploiting jasmonate-induced responses for field protection of conifer seedlings against a major forest pest, Hylobius abietis. For. Ecol. Manage. 313, 212–223. doi: 10.1016/j.foreco.2013.11.014
Zeneli, G., Krokene, P., Christiansen, E., Krekling, T., and Gershenzon, J. (2006). Methyl jasmonate treatment of mature Norway spruce (Picea abies) protects trees against Ceratocystis polonica infection and increases the accumulation of terpenoids resin components. Tree Physiol. 26, 977–988. doi: 10.1093/treephys/26.8.977
Zhang, Y., and Li, X. (2019). Salicylic acid: biosynthesis, perception, and contributions to plant immunity. Curr. Opin. Plant Biol. 50, 29–36. doi: 10.1016/j.pbi.2019.02.004
Zhao, J., Zheng, S. H., Fujita, K., and Sakai, K. (2004). Jasmonate and ethylene signalling and their interaction are integral parts of the elicitor signalling pathway leading to β-thujaplicin biosynthesis in Cupressus lusitanica cell cultures. J. Exp. Bot. 55, 1003–1012. doi: 10.1093/jxb/erh127
Zheng, Q., Putker, V., and Goverse, A. (2021). Molecular and cellular mechanisms involved in host-specific resistance to cyst nematodes in crops. Front. Plant Sci. 12:641582. doi: 10.3389/fpls.2021.641582
Zheng, Y., Jiao, C., Sun, H., Rosli, H. G., Pombo, M. A., Zhang, P., et al. (2016). iTAK: a program for genome-wide prediction and classification of plant transcription factors, transcriptional regulators, and protein kinases. Mol. Plant 9, 1667–1670. doi: 10.1016/j.molp.2016.09.014
Zulak, K. G., Lippert, D. N., Kuzyk, M. A., Domanski, D., Chou, T., Borchers, C. H., et al. (2009). Targeted proteomics using selected reaction monitoring reveals the induction of specific terpene synthases in a multi-level study of methyl jasmonate-treated Norway spruce (Picea abies). Plant J. 60, 1015–1030. doi: 10.1111/j.1365-313X.2009.04020.x
Keywords: cell wall lignification, jasmonate, maritime pine, pine wilt disease, resistance genes, secondary metabolism, transcriptome, Bursaphelenchus xylophilus
Citation: Modesto I, Sterck L, Arbona V, Gómez-Cadenas A, Carrasquinho I, Van de Peer Y and Miguel CM (2021) Insights Into the Mechanisms Implicated in Pinus pinaster Resistance to Pinewood Nematode. Front. Plant Sci. 12:690857. doi: 10.3389/fpls.2021.690857
Received: 04 April 2021; Accepted: 17 May 2021;
Published: 10 June 2021.
Edited by:
Carolina Escobar, University of Castilla-La Mancha, SpainReviewed by:
John Jones, The James Hutton Institute, United KingdomCopyright © 2021 Modesto, Sterck, Arbona, Gómez-Cadenas, Carrasquinho, Van de Peer and Miguel. This is an open-access article distributed under the terms of the Creative Commons Attribution License (CC BY). The use, distribution or reproduction in other forums is permitted, provided the original author(s) and the copyright owner(s) are credited and that the original publication in this journal is cited, in accordance with accepted academic practice. No use, distribution or reproduction is permitted which does not comply with these terms.
*Correspondence: Célia M. Miguel, Y21taWd1ZWxAZmMudWwucHQ=
Disclaimer: All claims expressed in this article are solely those of the authors and do not necessarily represent those of their affiliated organizations, or those of the publisher, the editors and the reviewers. Any product that may be evaluated in this article or claim that may be made by its manufacturer is not guaranteed or endorsed by the publisher.
Research integrity at Frontiers
Learn more about the work of our research integrity team to safeguard the quality of each article we publish.