- 1Institute of Experimental Botany of the Czech Academy of Sciences, Center of the Region Haná for Biotechnological and Agricultural Research, Olomouc, Czechia
- 2Agricultural Research Centre, Field Crops Research Institute, Cairo, Egypt
- 3ELKH Centre for Agricultural Research, Agricultural Institute, Martonvásár, Hungary
- 4Biological and Environmental Science and Engineering Division, Center for Desert Agriculture, King Abdullah University of Science and Technology, Thuwal, Saudi Arabia
- 5NBIS (National Bioinformatics Infrastructure Sweden, Science for Life Laboratory), Division of Cell Biology, Department of Clinical and Experimental Medicine, Faculty of Medicine and Health Sciences, Linköping University, Linköping, Sweden
- 6Wheat Genetics Resource Center, Kansas State University, Manhattan, KS, United States
Breeding of agricultural crops adapted to climate change and resistant to diseases and pests is hindered by a limited gene pool because of domestication and thousands of years of human selection. One way to increase genetic variation is chromosome-mediated gene transfer from wild relatives by cross hybridization. In the case of wheat (Triticum aestivum), the species of genus Aegilops are a particularly attractive source of new genes and alleles. However, during the evolution of the Aegilops and Triticum genera, diversification of the D-genome lineage resulted in the formation of diploid C, M, and U genomes of Aegilops. The extent of structural genome alterations, which accompanied their evolution and speciation, and the shortage of molecular tools to detect Aegilops chromatin hamper gene transfer into wheat. To investigate the chromosome structure and help develop molecular markers with a known physical position that could improve the efficiency of the selection of desired introgressions, we developed single-gene fluorescence in situ hybridization (FISH) maps for M- and U-genome progenitors, Aegilops comosa and Aegilops umbellulata, respectively. Forty-three ortholog genes were located on 47 loci in Ae. comosa and on 52 loci in Ae. umbellulata using wheat cDNA probes. The results obtained showed that M-genome chromosomes preserved collinearity with those of wheat, excluding 2 and 6M containing an intrachromosomal rearrangement and paracentric inversion of 6ML, respectively. While Ae. umbellulata chromosomes 1, 3, and 5U maintained collinearity with wheat, structural reorganizations in 2, 4, 6, and 7U suggested a similarity with the C genome of Aegilops markgrafii. To develop molecular markers with exact physical positions on chromosomes of Aegilops, the single-gene FISH data were validated in silico using DNA sequence assemblies from flow-sorted M- and U-genome chromosomes. The sequence similarity search of cDNA sequences confirmed 44 out of the 47 single-gene loci in Ae. comosa and 40 of the 52 map positions in Ae. umbellulata. Polymorphic regions, thus, identified enabled the development of molecular markers, which were PCR validated using wheat-Aegilops disomic chromosome addition lines. The single-gene FISH-based approach allowed the development of PCR markers specific for cytogenetically mapped positions on Aegilops chromosomes, substituting as yet unavailable segregating map. The new knowledge and resources will support the efforts for the introgression of Aegilops genes into wheat and their cloning.
Introduction
High-yielding and stress-tolerant wheat (Triticum aestivum, L.) cultivars with good quality traits will be needed to feed the nearly 10 billion human population estimated to be present in 2050 under changing global climate. One of the challenges is to increase genetic variation in the wheat gene pool to provide resistance to pests and diseases and tolerance to extreme abiotic conditions without significant yield loss. One way to increase the genetic variation of wheat is chromosome-mediated transfer of new gene variants from wild relatives by interspecific or intergeneric hybridization (Molnár-Láng et al., 2015).
Annual goat grasses (Aegilops L.) are closely related to Triticum and represent a rich source of genes with considerable agronomic value (Friebe et al., 1996; Schneider et al., 2008; Kilian et al., 2011). Within the genus, seven different genomes (C, D, M, N, S, T, and U) were identified in 11 diploid and 12 polyploid species (van Slageren, 1994). Aegilops comosa Sm in Sibth et Sm (2n = 2x = 14, MM) and Aegilops umbellulata Zhuk (2n = 2x = 14, UU) are diploid progenitors of the M- and U- genomes, respectively, which are also detected in 10 allopolyploid Aegilops species (van Slageren, 1994). Ae. comosa and Ae. umbellulata were reported as a source of resistance to wheat rust (Sears, 1956; Riley et al., 1968b; McIntosh et al., 1982; Bansal et al., 2017, 2020; Olivera et al., 2018), powdery mildew, Hessian fly, and greenbug (Riley et al., 1968a,b; Gill et al., 1985; Liu et al., 2019). With the exception of a transfer of several resistance genes from Ae. comosa (Yr8, Sr34) or Ae. umbellulata (Lr9, Lr76, and Yr70) (Sears, 1956; Bansal et al., 2020) to cultivated wheat (Riley et al., 1968a,b; Miller et al., 1988), their genetic diversity is largely underutilized in wheat breeding (Schneider et al., 2008).
Several factors hamper the transfer of wild alleles to wheat by interspecific hybridization. The collinearity between chromosomes of the donor species and wheat is one of them (Friebe et al., 1996). If it is broken down because of evolutionary chromosome rearrangements, homoeologous recombination may result in progenies with non-balanced genomes and poor agronomic performance (Devos et al., 1993; Zhang et al., 1998; Naranjo, 2019). Moreover, the altered structure of the donor chromosomes may interfere with recombination and compromise the attempts to reduce the size of introgressed chromatin and elimination of undesirable traits (Nasuda et al., 1998).
The efforts with chromosome-mediated gene transfer to wheat are also hampered by shortage of high-throughput techniques to screen large pre-breeding populations for the presence of alien chromatin (King et al., 2017). The most popular cytogenetic methods [genomic in situ hybridization (GISH) and fluorescence in situ hybridization (FISH)] allow the identification of M- and U-genome chromosomes or wheat-Aegilops intergeneric translocations (Badaeva et al., 1996a,b; Badaeva et al., 2004; Molnár et al., 2009). However, these methods are laborious and time consuming, and the tandem repeat probes frequently used for chromosome analysis using FISH in Triticum/Aegilops complexes hybridize predominantly in distal regions of chromosome arms (Molnár et al., 2011, 2016). This limits the identification of interstitial wheat-Aegilops translocations.
While cytogenetic analysis remains an important step in the selection procedure, marker-assisted selection would facilitate the identification of M- and U-genome chromatin at much higher throughput. This approach requires a minimum of two markers per chromosome arm, one specific for the telomeric and the other for the centromeric/near-centromeric region, so that the presence of an alien chromosome arm in the wheat background can be detected. To date, only few wheat molecular markers (AFLP, RFLP, SSR) have been tested in Aegilops species, and only some COS and PLUG markers were specific to Ae. comosa or Ae. umbellulata (Peil et al., 1998; Molnár et al., 2011, 2013; Liu et al., 2019). A serious problem is that the precise chromosomal location of these markers is unknown. One possibility is mapping these markers genetically, but, with Aegilops, it is time-consuming to develop a biparental mapping population (Zhang et al., 1998; Edae et al., 2016, 2017). Moreover, the positions of genetic markers do not always correspond to their physical locations on chromosomes, especially in the rarely recombining pericentromeric regions (Saintenac et al., 2009).
DNA sequences of conserved orthologous genes and their order are generally conserved in grass genomes (Gale and Devos, 1998), and the markers designed over the exon–intron boundaries of genes (COS markers) define orthologs regions, thus enabling the comparison of regions on the chromosomes of related species (Burt and Nicholson, 2011). In Aegilops, wheat COS markers were used successfully to map Quantitative trait loci (QTLs) for the B-type starch granule content and resistance genes (Howard et al., 2011). COS markers were also used to study homoeologous relationships between the M- and U-genome chromosomes of Aegilosps and rice, and Brachypodium and wheat (Molnár et al., 2013, 2016). However, the positions and the order of gene-specific markers along the chromosomes of Aegilops have not been determined as segregating mapping population was not available, a fact that hampers the use of the markers in marker-assisted introgression breeding.
Visualization of single-copy genes by FISH on mitotic metaphase chromosomes provides an attractive alternative to determine the exact position and the order of genes and gene-based markers along chromosomes and to investigate cross-species homology. In cereals with large and complex genomes, the use of cDNA-sequences as FISH probes allowed the visualization of single orthologous genes. Danilova et al. (2014, 2017a,b) used a set of wheat cDNA probes to develop a wheat single-gene FISH map, and analyze homoeologous relationships and chromosomal rearrangements in the tertiary gene pool of bread wheat. Single-gene FISH has also been used successfully in barley (Karafiátová et al., 2013; Danilova et al., 2017b), and Agropyron cristatum (Said et al., 2018) for the comparative analysis of chromosome structure and identification of gene order along chromosomes.
This study describes a new design strategy to develop molecular markers with known physical positions on chromosomes for wild gene-source species of wheat where a mapping population is not available. Using cDNA sequences as probes, we visualized and ordered a set of orthologous genes on the chromosomes of Ae. comosa and Ae. umbellulata. Furthermore, we developed draft sequence assemblies for the individual chromosomes of Ae. comosa and Ae. umbellulata and used them for the in silico validation of the cytogenetic positions. Using the aligned sequences of wheat and Aegilops, primers were designed for the mapped single-gene sequences to the regions polymorphic between Aegilops genomes and wheat. Finally, the primers were PCR validated in wheat, Aegilops, and wheat-Aegilops disomic addition lines representing the M- and U-genome chromosomes.
Materials and Methods
Plant Material
Seeds of Ae. comosa (2n = 2x = 14, MM) accession MvGB1039 and Ae. umbellulata (2n = 2x = 14, UU) accession AE740/03 were provided by the Institute of Plant Genetics and Crop Plant Research (Gatersleben, Germany) and were maintained in the Martonvásár Cereal Genebank (Martonvásár, Hungary). Seeds of bread wheat (Triticum aestivum) line Mv9kr1 carrying recessive crossability allele kr1 (Molnár-Láng et al., 1996), Aegilops biuncialis (2n = 4x = 28, MMUU) accession MvGB642 and Mv9kr1/Ae. biuncialis MvGB642 amphiploid were obtained from Martonvásár Genebank (Martonvásár, Hungary). Partial sets of bread wheat (cv. Chinese Spring)/Ae. umbellulata (JIC2010001) chromosome addition lines 1, 2, 6, 7U, and bread wheat (cv. Chinese Spring)/Ae. comosa (JIC2110001) chromosome addition lines 2, 3, 4, 5, 6, and 7M were provided by Dr. Steve Reader (John Innes Centre, Norwich, UK). Bread wheat (cv. Chinese Spring)/Aegilops geniculata (TA2899) chromosome addition lines 3, 4, 5Ug, and 1Mg (Friebe et al., 1999) were provided by Dr. Bernd Friebe (Kansas State University, Manhattan, KS, United States). Seeds of rye (Secale cereale L.; 2n = 2x = 14, RR) cv. Dankovské were obtained from Ms. Jarmila Kvardová (Oseva Agro, Brno, Czech Republic).
Mitotic Chromosome Preparations
Root tip meristem cells were synchronized using hydroxyurea, accumulated in metaphase using amiprohos-methyl, and mildly fixed with formaldehyde as described by Vrána et al. (2016a,b). The synchronized root tips were used to prepare chromosome suspensions for flow cytometric sorting as described below and also for the preparation of chromosome spreads for single-gene FISH using the drop technique demonstrated by Kato et al. (2004, 2006), with modifications as described by Danilova et al. (2012) and Said et al. (2018).
Preparation of cDNA Probes for Fluorescence in situ Hybridization
A total of 44 cDNA probes previously mapped by FISH to chromosomes of bread wheat (Danilova et al., 2014) and A. cristatum (Said et al., 2018) were used. The cDNA clones were developed by the National BioResource Project-Wheat, Japan, and all cDNA clones were provided by Dr. Tatiana V. Danilova (Department of Plant Pathology, Wheat Genetics Resource Center, Kansas State University, United States). cDNA sequences were amplified using PCR with T3/T7 primers and LongAmp DNA Polymerase (New England Biolabs, Ipswich, MA, United States) following the recommendations of the manufacturer. The PCR products were purified with the Invitrogen PCR purification kit (Life Technologies, Carlsbad, CA, United States) according to the instructions of the manufacturer.
The rye 120-bp repeat family (Bedbrook et al., 1980) was amplified by PCR from DNA clone pSc119.2 using M13universal primers as described by Said et al. (2018). GAA microsatellites were PCR amplified from rye (cv. Dankovské) genomic DNA with (GAA)7 and (CCT)7 primers according to Kubaláková et al. (2005), while the Afa family repeat was amplified from genomic DNA of bread wheat (cv. Chinese Spring) using primers AS-A and AS-B (Nagaki et al., 1995). Probes pSc119.2, GAA microsatellites, and Afa family repeat were directly labeled by PCR using aminoallyl-dUTP-CY5 (Jena Biosciences, Jena, Germany). Plasmid pTa71 (45S rDNA) (Gerlach and Bedbrook, 1979) was directly labeled by nick translation with ChromaTide® Fluorescein-12-dUTP (Thermo Fisher Scientific, Waltham, MA, United States).
All cDNA probes were labeled with ChromaTide® Texas Red®-12-dUTP (Thermo Fisher Scientific, Waltham, MA, United States). Direct labeling of cDNA and 45S rDNA probes by nick translation was done following Kato et al. (2004, 2006) and Said et al. (2018). The probe quality was checked on 1.5% agarose gel. To increase probe concentration and remove non-incorporated nucleotides, the probes were precipitated and purified by adding 173 μl 1 × TE buffer (pH 7.5), 20 μl sodium acetate (3M, pH 5.2), and 500 μl 100% ethanol; 3 μg herring sperm DNA (Promega, Madison, WI, USA) was added as blocking DNA. After overnight precipitation at −20°C, the probes were centrifuged for 30 min at 4°C at 14,000 × g, rinsed in 70% ethanol, and air dried for 7–15 min. Subsequently, the probes were dissolved in 20 μl of 2 × SSC and 1 × TE buffer (pH 7.6) in 1:1 ratio at 65°C for 10 min.
Fluorescence in situ Hybridization
All FISH experiments were done with two or more probes, which were hybridized simultaneously. cDNA probes were localized in combination with 45S rDNA and one of the tandem repeats for pSc119.2, Afa family, or GAA microsatellite repeat. The experiments, such as the denaturation of probe and chromosomal DNA, hybridization and post hybridization washes, and the composition of hybridization mixture, were the same as described by Cabrera et al. (2002) and Said et al. (2018). Briefly, 300-ng cDNA probe and 50-ng DNA for each tandem repeat probe in the hybridization mixture (20 μl) were denatured at 80°C for 3 min and hybridized overnight at 37°C. After post-hybridization washes, the slides were mounted and counterstained with 4′,6-diamidino-2-phenylindole (DAPI) in a Vectashield mounting medium (Vector Laboratories, Burlingame, CA, United States).
Microscopy, Software, Signal Capture, and Image Analysis
Chromosome preparations were examined using an Axio Imager Z.2 fluorescence microscope (Zeiss, Oberkochen, Germany) equipped with a Cool Cube 1 camera (Metasystems, Altlussheim, Germany) and appropriate filter sets. Signal capturing and picture processing were performed using the ISIS software (Metasystems, Altlussheim, Germany). Final image adjustment was done with Adobe Photoshop CS5 (Adobe Systems Incorporated, San Jose, CA, United States). Chromosome measurements and determination of the position of FISH signals were measured using the ISIS software (Metasystems).
Chromosome Measurements
Chromosome measurements to determine the relative positions of cDNA sites were carried out as described by Said et al. (2018). Briefly, 10 best images of mitotic metaphase spreads obtained at × 100 magnification were selected and used for calculating the centromeric index, arm ratio (L/S), and relative chromosome length for each chromosome. The distance of cDNA sites from the centromere was measured in micrometers, and the average of 10 measurements was used to calculate the fraction length (FL) value. SDs and confidence intervals with a significance level of 0.05 were calculated using Microsoft Office Excel 2016 functions. The cDNA positions on wheat D-genome chromosomes and Aegilops markgrafii C-genome chromosomes were taken from Danilova et al. (2017a).
Flow Cytometric Analysis and Sorting of Aegilops Chromosomes
The preparation of mitotic metaphase chromosome suspensions of Ae. comosa MvGB1039 and Ae. umbellulata AE740/03 was carried out as described by Vrána et al. (2000) and Kubaláková et al. (2005). Prior to the flow cytometric analysis, the chromosomes were labeled by fluorescence in situ hybridization in suspension (FISHIS) using 5′-FITC-GAA7-FITC-3′ oligonucleotides (Sigma, Saint Louis, MO, United States) according to Giorgi et al. (2013) and stained by DAPI (4′,6-diamidino 2-phenylindole) at 2 μg/ml. Chromosome analysis and sorting were carried out using a FACSAria II SORP flow cytometer and sorter (Becton Dickinson Immunocytometry Systems, San José, CA, United States) as described by Molnár et al. (2016) and Said et al. (2019). Bivariate flow karyotypes FITC vs. DAPI fluorescence were acquired for each sample and two batches of 25,000–76,000 copies of each chromosome were sorted into PCR tubes containing 40 μl sterile deionized water. The chromosome content of the flow-sorted fractions was determined by FISH on chromosomes sorted onto a microscopic slide using the probes for pSc119.2, Afa family repeat, and 45S rDNA according to Molnár et al. (2016). The chromosomes were classified following the karyotype described by Parisod and Badaeva (2020).
Chromosome Sequencing and Assembly
The assembled DNA sequence contigs from flow-sorted chromosomes of Ae. umbellulata AE740/03 obtained previously from Bansal et al. (2020) were used for downstream analysis in this study. Chromosomes 1–7M flow sorted from Ae. comosa were used to prepare sequencing libraries. Samples of chromosomal DNA were purified according to Šimková et al. (2008), and 20 ng DNA was fragmented in 20 μl using Bioruptor Plus (Diagenode, Liège, Belgium) for five times for 30 s at HIGH setting. The sequencing libraries were prepared from sheared DNA using NEBNext® Ultra™ II DNA Library Prep Kit for Illumina® with the following modification: (i) size selection was directed for larger final library size (~1,000 bp) and (ii) PCR enrichment was done in nine cycles. The fragment size distribution of the libraries was checked on Agilent Bioanalyzer 2100 with High Sensitivity DNA kit (Agilent Technologies; Santa Clara, CA, United States). The samples were selected based on size using BluePippin (Sage Science, Beverly, MA, United States) in pre-cast 1.5% agarose gel cassettes. The setting of the selected range was chosen according to size distribution from Agilent Bioanalyzer results to achieve longer fragment sizes and the best recovery yield (TAB1). The recovered libraries were quantified by real-time PCR by KAPA Library Quantification Kit KK4844 (Roche, Pleasanton, CA, United States). The libraries were sequenced on an illuminaNovaSeq 6000 platform, and 2 × 150 bp paired-end reads were produced. The raw data were trimmed for low-quality bases using Trimmomatic (Bolger et al., 2014) and assembled to scaffolds with Meraculous v2.0.5 (Chapman et al., 2011) using 111-bp k-mers. Scaffolds shorter than 1 kb were eliminated. A percent of the genome represented by each chromosome is equivalent to its relative chromosome length, considering the sum of the absolute lengths (in micrometers) of all chromosomes in a haploid chromosome set (n) equal to 100% (Said et al., 2018). Chromosome sizes in Mbp were calculated using chromosome lengths (in micrometers) listed in Supplementary Tables 1, 2. Briefly, 1C value of a species is the amount of DNA in a haploid chromosome complement (n). For the calculation of chromosome size in Mbp, the 1C values of 5.05 and 5.53 pg for Ae. umbellulata and Ae. comosa, respectively (Furuta, 1970; Eilam et al., 2007), were used. Using the relative length of a chromosome (percent of the genome it represents) and 1C value, the amount of DNA in a chromosome can be calculated and then converted to Mbp using formula 1 pg DNA = 978 Mbp (Doležel et al., 2003). The coverage of a chromosome draft assembly is then determined by dividing the “total length of chromosome assembly (Mbp) by the chromosome size (Mbp)” value.
Expressed Sequence Tag Mapping and Functional Annotations
Cytogenetically determined physical positions of cDNA sequences on the M- and U-genome chromosomes were validated in silico using a sequence similarity approach. The publicly available sequences of wheat cDNA probes (https://shigen.nig.ac.jp/wheat/komugi/) were used as queries in BLASTn searches against the reference pseudomolecules of the A, B, and D genomes of hexaploid wheat; Ensembl Plants, release-46 (Appels et al., 2018). The start position of each cDNA sequences was determined on the best hits obtained on the wheat reference sequence by the use of the BLASTn package of Blast Command Line Application 2.9.0 (ftp://ftp.ncbi.nlm.nih.gov/) with the following parameters: -task “BLASTn”; -evalue 1e-5; -max_target_seqs 2; -max_hsps 1. Translated sequences of the identified wheat genes were used for functional annotations. The Gene Ontology information was extracted from the Universal Protein Resource (ftp://ftp.uniprot.org; UniProt release 2019_11) database; the resulting protein collections were subsequently scanned with the Hidden Markov Model (HMM)-based HMMER 3.0 software package (http://eddylab.org/software/hmmer/) (Eddy, 2009) on the Pfam 32.0 entries (ftp://ftp.ebi.ac.uk) (El-Gebali et al., 2019).
Similar BLASTn searches were carried out against the chromosomal scaffolds of Ae. comosa MvGB1039 and Ae. umbellulata AE740/03, and the first two best hits were used for the in silico validation of single-gene FISH positions on the M- and U-genome chromosomes. The results of BLASTn, including the genomic start positions in bp on wheat chromosomes (Groups I–VII), e-value, wheat gene ID, protein domains identified in database (PFAM), and gene ontology (GO) together with the BLASTn results on chromosomal scaffolds of Ae. comosa and Ae. umbellulata, are summarized in Supplementary Data 1.
Polymerase Chain Reaction Marker Design and Validation
To design Aegilops markers specific for the mapped cDNAs, pairwise alignment of wheat cDNA-sequences with the chromosomal scaffolds of Aegilops selected by BLASTn was carried out using UGENE software (v. 1.23). INDELs and polymorphic regions between wheat and Aegilops were determined, and primer pairs were designed for the exon–intron boundary and intronic regions with the Primer3 software (Untergasser et al., 2012) to amplify polymorphic fragments. At least three primer pairs per cDNA-Ae. comosa or cDNA-Ae. umbellulata alignment were designed and tested by PCR on wheat line Mv9kr1, Ae. comosa MvGB1039, Ae. umbellulata AE740/03, and Ae. biuncialis MvGB642. The primer pairs producing Aegilops-specific amplicons were further checked by PCR on Mv9kr1/Ae. biuncialis MvGB 642 amphiploid and partial sets of Chinese spring/Ae. comosa, Chinese spring/Ae. umbellulata, and Chinese spring/Ae. geniculata chromosome addition lines representing complete sets of the M and U-genome chromosomes in wheat genetic background. The reaction mixture (15 μl), containing 0.2 μmol/L of forward and reverse primers, and the thermal reaction profile used for the PCR reactions were described by Molnár et al. (2014). PCR amplicons were detected along with a 75–400 bp Range DNA Ladder using a Fragment Analyzer Automated CE System (Advanced Analytical Technologies, Ames, IA, United States) and analyzed with the PROsize v2.0 software (Advanced Analytical Technologies, Ames, IA, United States). The primer sequences, source cDNA, and Aegilops contigs, and the PCR amplicons obtained on different wheat and Aegilops genotypes are included in Supplementary Data 2.
Results
Chromosome Structure and Wheat-Aegilops Genome Relationships
In order to physically map single orthologous genes to chromosomes, we used FISH with cDNA-probes in combination with probes for DNA repeats, whose hybridizing patterns on the M- and U-genome chromosomes are known (Molnár et al., 2016; Parisod and Badaeva, 2020). 45S rDNA, Afa family repeat, and GAA microsatellites permitted the identification of the whole chromosome complement of Ae. comosa, while 45S rDNA and pSc119.2 repeats were used to identify the chromosomes of Ae. umbellulata (Figures 1, 2, Supplementary Figures 1–3). The hybridization patterns together with the data on chromosome size and centromere position are summarized in Supplementary Figures 1–3 and Supplementary Tables 1, 2.
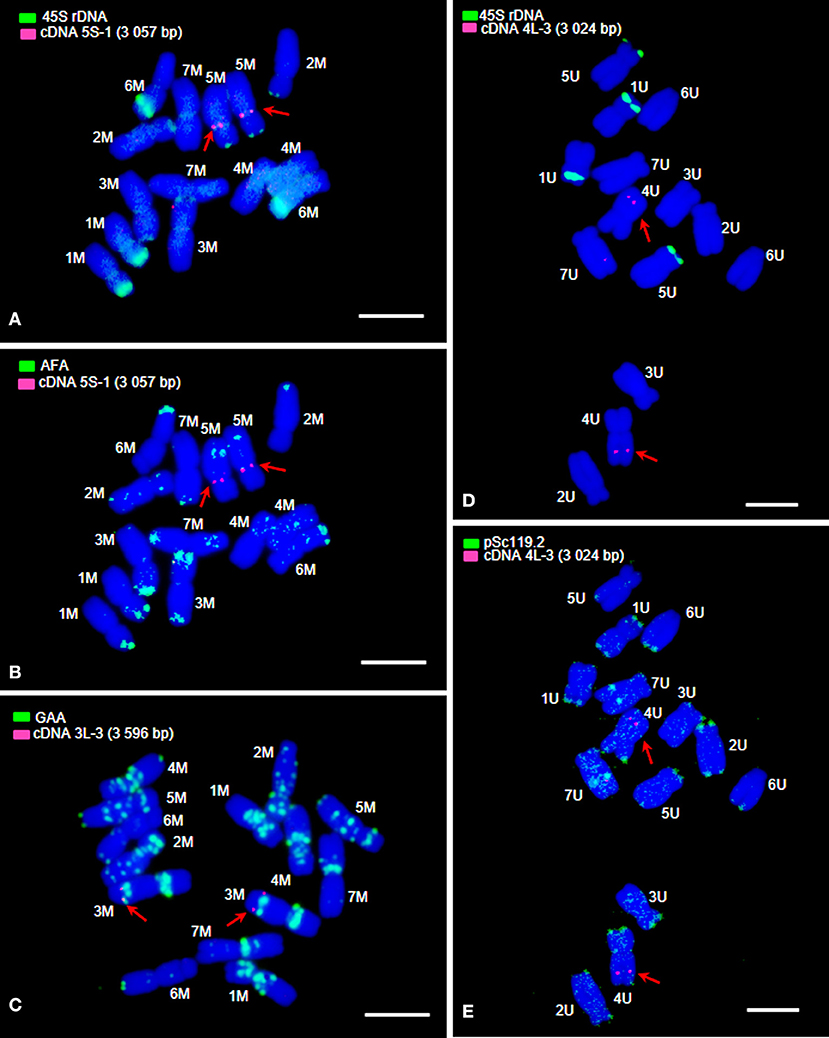
Figure 1. Fluorescence in situ hybridization (FISH) with probes for 45S rDNA, Afa family repeat, GAA microsatellites, pSc119.2 repeat, and cDNAs (red arrows) on mitotic metaphase chromosomes of Ae. umbellulata and Ae. comosa. FISH on chromosomes of Ae. comosa (A) 45S rDNA (green) and cDNA (red); (B) Afa family repeat (green) and cDNA (red); (C) GAA microsatellites (green) and cDNA (red). FISH on chromosomes of Ae. umbellulata (D) 45S rDNA (green) and cDNA (red); and (E) pSc119.2 repeat (green) and cDNA (red). The chromosomes were counterstained with DAPI (blue). Bars = 5 μm.
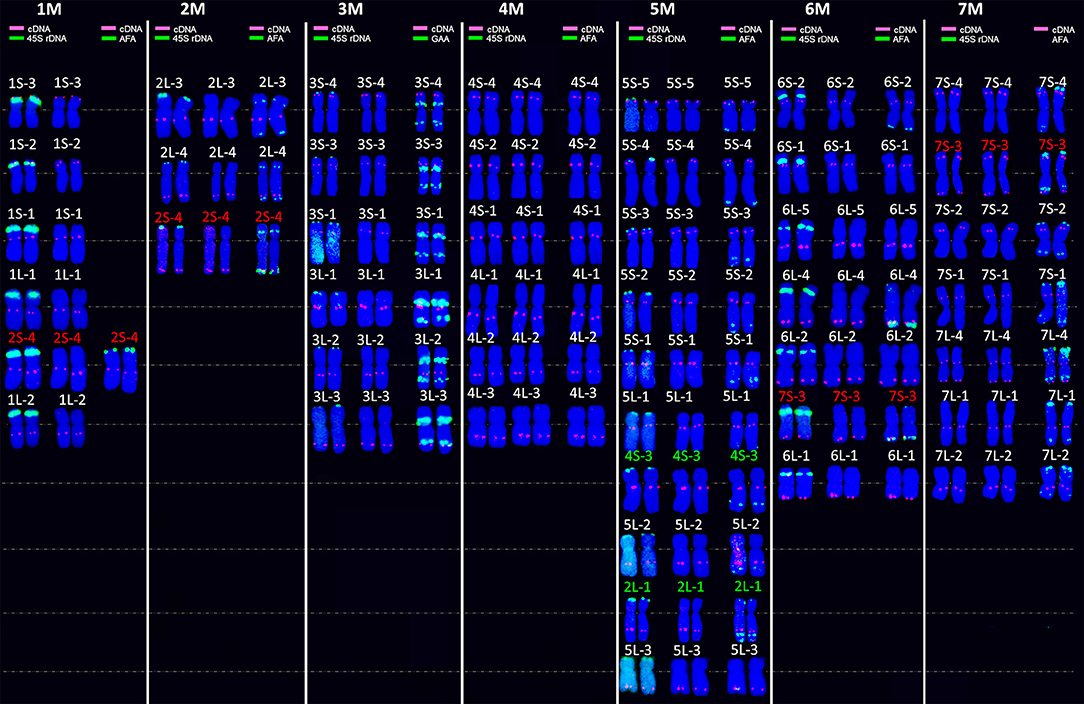
Figure 2. FISH on mitotic metaphase chromosomes of Ae. comosa reveals the distribution of 43 wheat cDNA probes (red dots), in addition to 45S rDNA, Afa family repeat, and GAA microsatellites (all in green). Each chromosome pair (1–7M) is shown three times, with the patterns of the repeats in addition to cDNA (left and right of the column) and with the cDNA-probes only (middle of the column). The names of the cDNA probes are shown above each chromosome pair. The names of cDNA probes that hybridized to more than one chromosome are highlighted in red. The names of cDNA-probes that hybridized only to a non-homoeologous chromosome are highlighted in green.
A set of 44 wheat cDNA-probes covering wheat homoeologous groups 1–7 (4–8 cDNA probes per chromosome) was used for FISH to investigate the long-range organization of the M- and U-genome chromosomes and their homology with those of hexaploid wheat and Ae. markgrafii. One cDNA sequence (5L-4) failed to produce PCR amplicon and was discarded from the FISH experiments. The remaining 43 cDNA-probes produced clear hybridization signals in 47 positions on the chromosomes of Ae. comosa. Thirty-nine probes (90.7%) were hybridized to the same homoeologous chromosome groups as in wheat at a total of 40 loci. Some cDNAs were located at two (2S-4 and 7L-4) or three different positions (7S-3) on chromosomes homoeologous and non-homoeologous with wheat. Finally, two (4.7%) cDNA probes (4S-3 and 2L-1) were detected only on single loci on non-homoeologous M-genome chromosomes relative to wheat (Figures 2, 3, Supplementary Table 3).
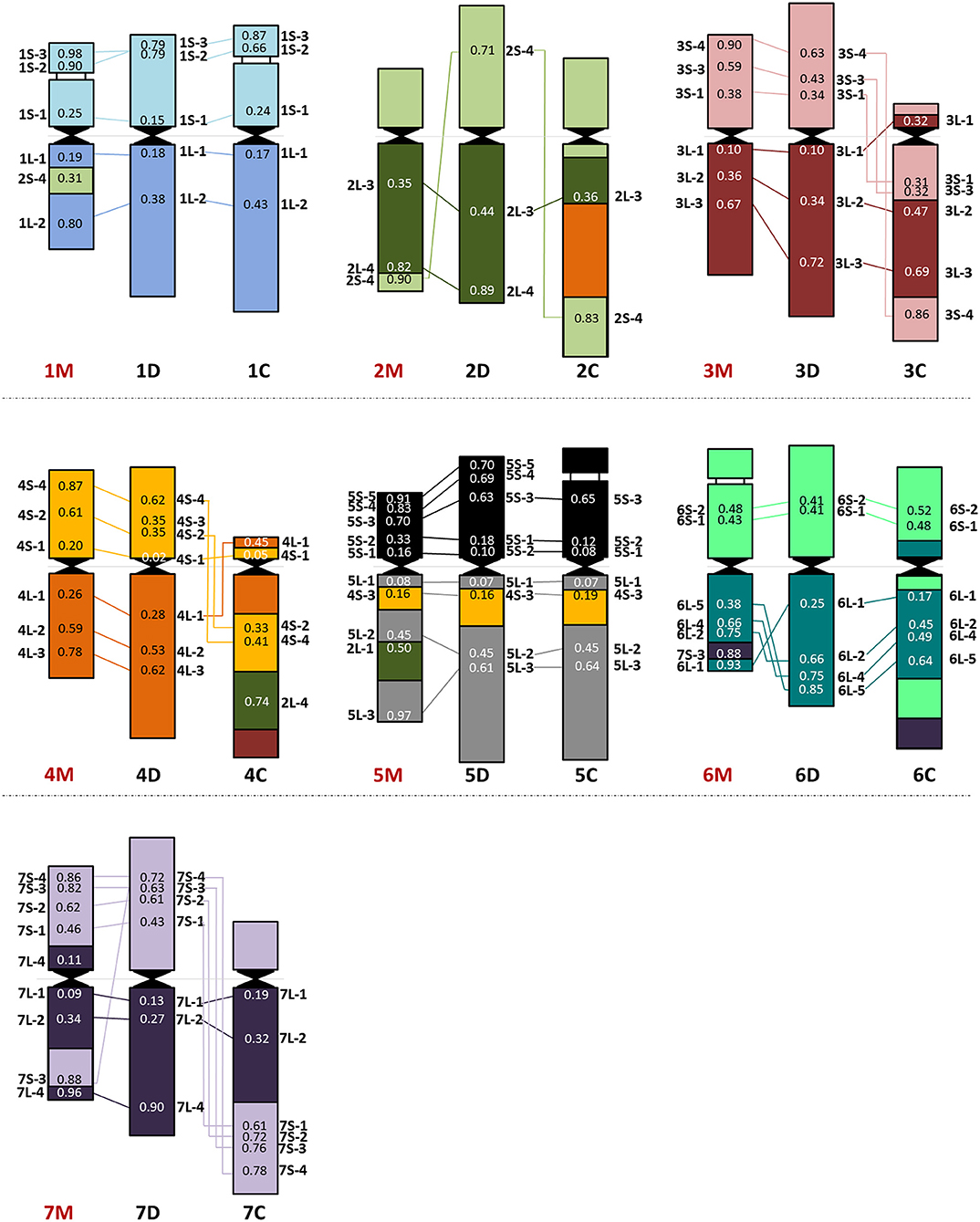
Figure 3. Chromosome organization of the Ae. comosa M genome (left) compared with the D (sub)genome of wheat (middle) and the Ae. markgrafii C genome (right). The average relative positions of cDNAs are shown on the chromosomes.
In general, chromosomes 1, 3, 4, 5, and 7M of Ae. comosa were found collinear with the corresponding wheat chromosomes. However, a collinearity distortion on chromosome 2M was indicated by wheat group 2 short arm subtelomeric-specific cDNA-probe 2S-4, which localized to the telomeric regions of the 2M long arm and interstitial region of 1ML, and by group 2 long arm-specific marker located on 5ML. A paracentric inversion was detected on 6ML relative to wheat (Figure 3). The hybridization sites of two group 7–specific probes were also duplicated (7L-4) or triplicated (7S-3).
The 43 wheat cDNA-probes hybridized at 52 loci in Ae. umbellulata and the positions of these hybridization signals indicated significant genome rearrangements in U-genome relative to wheat (Figure 4, Supplementary Figure 3, Supplementary Table 4). Apart from the signal of 7S-3 on 1UL, chromosomes 1, 3, and 5U (excluding the telomeric part of the 3U short arm which was detected in distal position of 7UL) were generally collinear with the corresponding wheat chromosomes. No probes produced signals on 2US as the cDNA-probes specific for this chromosome arm were detected on telomeres of 2UL and 6UL. The probe specific for the distal part of the wheat group 2 long arm (2L-4) also hybridized on 6UL and 7UL. The most pronounced collinearity distortion relative to wheat was observed for chromosomes 4, 6, and 7U. For example, the signals of group 4-specific cDNA-probes on 6U and group 6 probes on 4U indicated multiple reciprocal translocations between chromosomes 4 and 6U together with inversion on 4US. Chromosome 6U exhibited the most rearranged structure, as its short arm showed homology with wheat group 4, while its long arm contained regions homoeologous with group 2, the short arm of group 4, long arm of group 6, group 7, and pericentric inversion. Finally, an intra-chromosomal translocation on 7U relative to wheat was detected with a putative breakpoint between 7US-1 and 7US-2 on the short arm, and translocations of the distal half of the short arm to the end of the long arm accompanied/followed by inversion of the interstitial region of 7UL and further insertions of fragments from groups 2, 3, and 6.
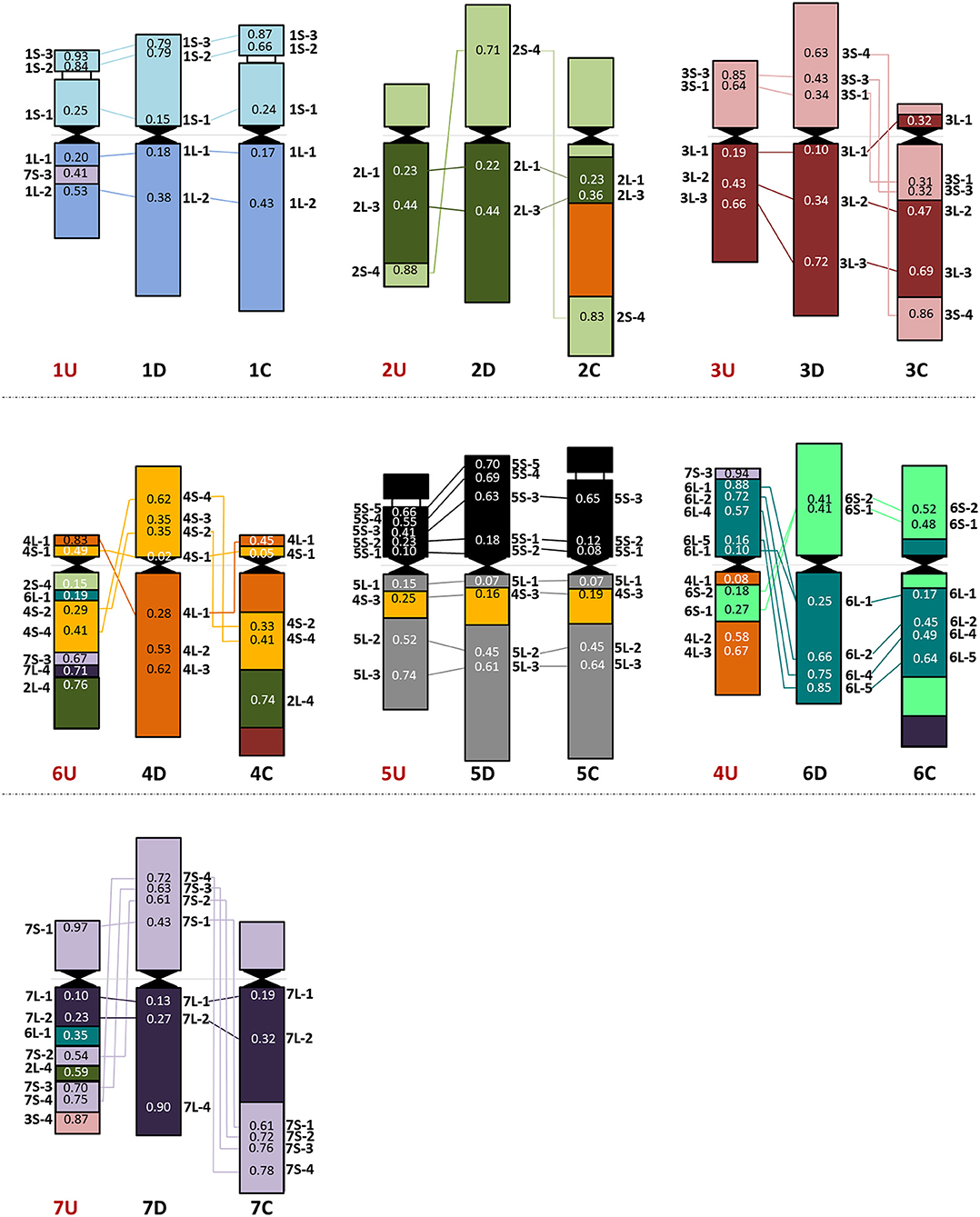
Figure 4. Chromosome organization of the Ae. umbellulata U genome (left) compared with the D (sub)genome of wheat (middle) and the Ae. markgrafii C genome (right). The average relative positions of cDNAs are shown on the chromosomes.
In silico Validation of cDNA Locations Using Sequences From Flow-Sorted Chromosomes
The cytogenetically determined positions of wheat single-gene (cDNA) probes were validated by sequence similarity search of cDNA sequences against the chromosomal assemblies of Ae. comosa and Ae. umbellulata. In the case of Ae. umbellulata, previously developed chromosome assemblies were used for the analysis. In order to obtain chromosome assemblies for Ae. comosa, each of the M-genome chromosomes was sorted by flow cytometry and then shot-gun sequenced. Bivariate flow karyotypes GAA-FITC vs. DAPI fluorescence comprised three clearly separated and two mixed populations (Figure 5). FISH with chromosomes sorted onto a microscope slide (Figure 5) showed that the well-resolved populations corresponded to pure (89–98%) fractions of chromosomes 3, 6, and 7M (Supplementary Table 5), while chromosomes 1 and 4M and 2 and 5M could be sorted from the two mixed populations at 72–86% purities. A total of 67,000–150,000 chromosomes were sorted to prepare 59.5 ng DNA on average from each chromosome (Supplementary Table 5). Shotgun sequencing of chromosomal DNA resulted in 112.44–210.01 Gb output for each sample (Table 1). From 52% (5M) to 89% (4M), the chromosome sizes were represented in the total length of the draft assemblies. The statistical parameters of the chromosome assemblies are summarized in Table 1.
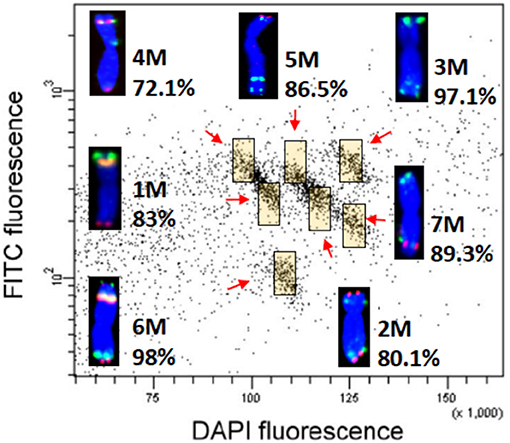
Figure 5. Bivariate flow karyotyping of Ae. comosa chromosomes after fluorescence in situ hybridization in suspension (FISHIS) with probes for (GAA)7 and (ACG)5 resolved all seven M-genome chromosomes, which could be flow-sorted at purities of 72–98%. Chromosomes were assigned to the colored regions (yellow) by fluorescence in situ hybridization using probes for 45S rDNA (yellow), Afa family (green), and pSc119.2 (red). Chromosomes were counterstained by DAPI (blue).
The 44 cDNA query sequences resulted in 44 on the genome assemblies of wheat, while 88 and 86 significant hits on the chromosome assemblies of Ae. comosa and Ae. umbellulata, respectively, as we used the first two best hits for the analysis (Supplementary Data 1). The generally similar alignment length of the cDNA-sequences on wheat and on the two Aegilops species and the high identity of the aligned sequences (mean value: >95%) in the three species reflected high homology in coding regions between wheat and the Aegilops species. Finally, out of the 47 cytogenetically determined single-gene positions on Ae. comosa chromosomes, 44 (93.6%) were confirmed when one of the two best hits of the cDNA-sequences was found in the assembly of the same chromosomes as determined by FISH (Figure 6A). Similarly, 40 (76.9%) out of the 52 single-gene FISH sites of the Ae. umbellulata map were confirmed (Figure 6B).
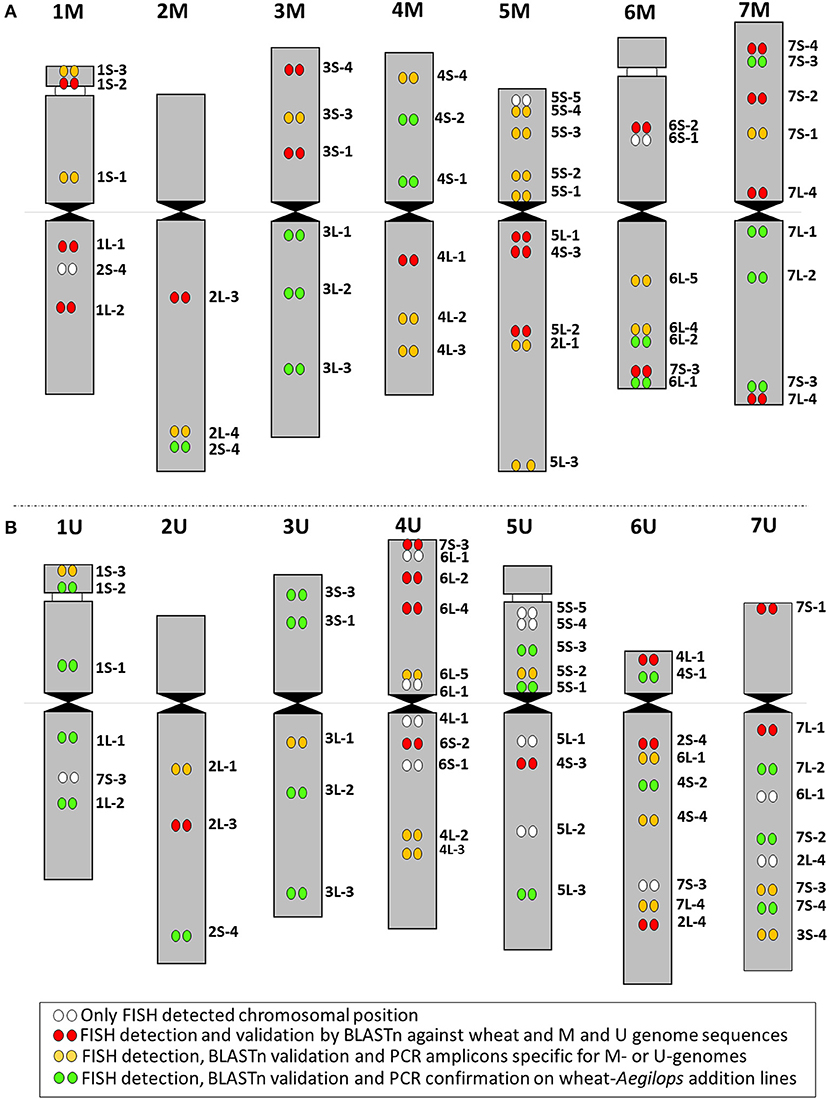
Figure 6. Idiogram summarizing the results of FISH mapping of cDNAs on (A) M- and (B) U-genome chromosomes. The color scheme (bottom) indicates detection of the cDNAs as FISH probes on M and U genomes (white dots), detection as FISH probes and in silico validation by BLASTn against bread wheat and M- and U-genome sequences (red dots); detection as FISH probes, validation by BLASTn and PCR amplicons specific for M or U genomes (yellow dots); detection as FISH probes, validation by BLASTn, and PCR markers validated on wheat-Aegilops addition lines (green dots).
Marker Design and Validation
In order to design PCR markers specific for the cytogenetically mapped single genes, 44 full-length cDNA sequences were aligned to the corresponding Aegilops contigs (Supplementary Data 1) and 274 primer pairs (136 and 138 primer pairs using M- and U-contigs, respectively) were designed specifically for the exon–intron boundaries and the intronic region (Supplementary Table 6, Supplementary Data 2). The markers polymorphic between the M- and U- genomes of Aegilops and wheat were validated by PCR using genomic DNA from hexaploid wheat (Mv9kr1), Ae. comosa (MvGB1039), Ae. umbellulata (AE740/03), and allotetraploid (MbMbUbUb) Ae. biuncialis (MvGB642) as the template (Figure 7, Supplementary Data 2).
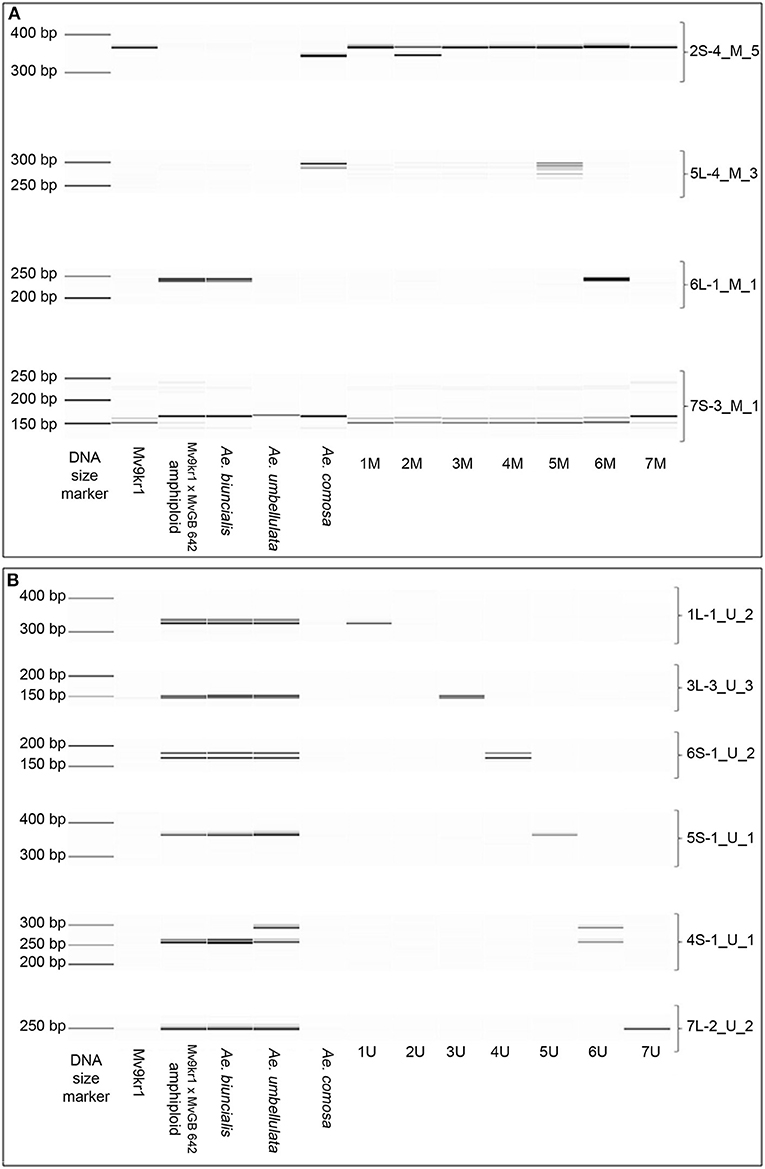
Figure 7. Digital capillary electrophoretic pattern of the cDNA-derived markers designed by the use of corresponding (A) M or (B) U chromosomal sequences determined by the BLASTn search. The markers were tested on hexaploid wheat line Mv9kr1, on Ae. biuncialis MvGB642 accession, wheat-Ae. biuncialis MvGB 642 amphiploid, and Ae. comosa MvGB1039 and Ae. umbellulata AE740/03. The markers were also tested on wheat-Ae. comosa (2M−7M), wheat-Ae. umbellulata (1U, 2U, 6U, 7U), and wheat- Ae. geniculata (1M, 3U, 4U, 5U) disomic chromosome addition lines representing the whole set of M- or U-genome chromosomes. A 35–500 bp DNA ladder was used as a molecular-weight size marker to estimate the fragment size.
Out of the 136M-genome markers, 68 were polymorphic between Aegilops and wheat (16 and 52 showed presence/absence and size polymorphism, respectively) (Figure 7A, Supplementary Table 6, Supplementary Data 2). In the case of the 138 U-genome markers, 74 were polymorphic between Aegilops and wheat (37 and 37 were presence/absence and size polymorphic, respectively) (Figure 7B, Supplementary Table 6, Supplementary Data 2). Independent of which Aegilops genomic contig was used for marker design, 32 markers amplified a specific PCR product from both the M- and U-genomes (Supplementary Data 2). Finally, 34 markers were M-genome specific (produced amplicons only from Ae. comosa and Ae. biuncialis which were polymorphic for wheat), and 48 were U-genome specific (produced amplicons only from Ae. umbellulata and Ae. biuncialis which were polymorphic relative to wheat) (Supplementary Data 2).
The 66 markers producing amplicons from the M genome (34 M-genome specific and 32 M-/U-genome specific) were related to 27 BLAST validated single-gene positions on chromosomes 1–7M of Ae. comosa. In the case of Ae. umbellulata, the 80 markers producing amplicons from the U-genome (48 U-genome specific and 32 M-/U-genome specific) were related to 28 BLAST-validated cDNA sites on all of the chromosomes, excluding chromosome arms 2US and 7US. The marker coverage of the chromosomes ranged from three (2U) to six (7U) (Figures 6B, 7B).
The PCR markers (at least one marker per cDNA site) were further validated on wheat-Aegilops addition lines carrying chromosomes 1–7M and 1–7U. To cover the 27 cDNA sites of M-genome, 28 randomly selected markers were tested. In total, 11 markers confirmed 12 single-gene positions (44.4). Moreover, cDNA-probe 5L-4, whose location by FISH failed, was also located on chromosome 5M. In total, PCR markers were validated on the addition lines for one gene position on chromosome 2M, for two on 4 and 6M, for three on 3M, and for four positions on 7M (Figure 6A, Supplementary Data 2).
We tested a total of 34 randomly selected markers for the 28 cDNA sites of the U-genome. Out of the 30 markers with Aegilops-specific amplicons covering 24 cDNA sites, 21 markers confirmed 17 single gene positions (60.7%), as they were assigned to the same U-genome chromosomes, as determined by single-gene FISH and by BLASTn. The PCR markers were validated on the addition lines for one cDNA position on 2U, for two on 6U, for three on 5U and 7U, and for four gene positions on 1U and 3U (Figure 6B, Supplementary Data 2). A PCR marker for cDNA-sequence 5L-4 was located on chromosome 4U.
Discussion
The single-gene FISH maps developed during the course of this study significantly improve the knowledge on the long-range chromosome organization in Ae. comosa and Ae. umbellulata. The new results and molecular tools will make the alien gene transfer from these species into wheat more efficient.
As the 44 orthologous genes used in this study were also mapped on the A, B, and D subgenomes of hexaploid wheat (Danilova et al., 2014), on C-genome chromosomes of Ae. markgrafii (Danilova et al., 2017a), the single-gene FISH maps of Ae. comosa and Ae. umbellulata provide a unique opportunity to investigate the karyotype evolution in the Triticum/Aegilops complex. The results support the previous observations obtained by molecular markers specific for conserved orthologous genes, suggesting a more similar organization of the M genome of Ae. comosa than those of the U-genome chromosomes of Ae. umbellulata relative to wheat (Molnár et al., 2016; Liu et al., 2019). However, as shown in the previous studies, the marker positions were not determined along the chromosomes of Aegilops, and the identification of intrachromosomal rearrangements was not possible. We observed a well-preserved wheat-Ae. comosa collinearity for chromosomes 1, 3, and 4M and, to a lesser extent, for chromosomes 5 and 7M (Figures 2, 3, Supplementary Table 7), while conserved wheat-Aegilops synteny was observed only for chromosomes 1, 3, and 5U in Ae. umbellulata (Figure 4, Supplementary Figure 3, Supplementary Table 7).
In agreement with Nasuda et al. (1998), who identified terminal intra-chromosomal translocation in chromosome 2M relative to wheat group 2, we detected a distal part of the short arm on the distal end of group 2 long arms in Ae. comosa as well as in Ae. umbellulata. This translocation was also detected in Ae. umbellulata by genetic mapping (Zhang et al., 1998; Edae et al., 2017) and in Ae. markgrafii by single-gene FISH (Danilova et al., 2017a). However, the break points on chromosome group 2 long arms were different in the three species as most distal cDNA-probe 2L-4 was present on 2ML, while this region was translocated to the distal part of 6UL, or to 4CL. Similar organization of chromosomes 6U and 4C was also highlighted by the pericentric inversion of group 4 short and long arm regions. Another example of a similar genome structure of Ae. umbellulata and Ae. markgrafii was the intrachromosomal translocation detected in chromosomes 7U and 7C, where the chromosome region homoeologous to the group 7 short arm of wheat was translocated to the long arm in Ae. umbellulata and Ae. markgrafii accompanied/followed by paracentric inversions on 7UL and 7CL. However, this rearrangement was not detected in Ae. comosa (Figures 2–4, Supplementary Figure 3).
The wheat-Aegilops collinearity was also interrupted on the chromosome arm of 6ML because of paracentric inversion relative to wheat (Danilova et al., 2014). In fact, a similar inversion of the wheat group 6 long arm was observed in Ae. umbellulata, where the inverted region was translocated to 4US, in diploid and tetraploid A. cristatum (Han et al., 2014; Said et al., 2018), but it was not found in Ae. markgrafii (Danilova et al., 2017a). Chromosomes 4U and 6U showed the most rearranged structure in Ae. umbellulata. The localization of four out of the seven group 4-specific cDNA-probes on 6U and all of the six group 6-specific probes on 4U indicated multiple reciprocal translocations between 4U and 6U, confirming a need to rename these chromosomes as recommended by Zhang et al. (1998). Finally, the detection of a cDNA probe from the wheat group 3 short arm on 7UL indicated another interchromosomal translocation in Ae. umbellulata (Figures 2–4, Supplementary Figure 3).
The comparative single-gene FISH analysis highlighted the role of karyotype changes in the evolution of closely related C-, M- and U-genomes of Aegilops. Recent phylogenomic studies on diploid Triticum/Aegilops species using 38 nuclear low copy loci (Huynh et al., 2019) and by genome-wide RNA-seq-based polymorphic analysis (Glémin et al., 2019; Tanaka et al., 2020) have shown that the ancestor of the D-genome lineage went through intensive diversification ~3 Mya, leading to the formation of the current Aegilops species with C, D, M, N, S, and U genomes in the area of Fertile Crescent and their subsequent radiation to the Mediterranean landscapes (Kilian et al., 2011). Glémin et al. (2019) and Tanaka et al. (2020) suggested that C-, M-, N-, and U-genome species formed a separated group from those containing the D and S genomes. The C genome of Ae. markgrafii and U-genome of Ae. umbellulata were found to be closely related, forming a clade separated from the species containing the M genome of Ae. comosa and the N genome of Ae. uniaristata (Glémin et al., 2019; Tanaka et al., 2020). Consistent with these results, the single-gene FISH map of Ae. comosa and Ae. umbellulata together with those of Ae. markgrafii (Danilova et al., 2017a) showed that the organization of the M genome remained relatively similar to the three subgenomes of bread wheat (Figures 2, 3). On the contrary, highly rearranged C and U genomes, which are similar to each other, are significantly different from Ae. comosa as reflected by the similar alterations of the structure of chromosomes 4C and 6U (pericentric inversion of group 4 short and long arm regions, the presence of group 2 long arm region on the long arms) and 7C and 7U (intra-chromosomal translocation of the distal half of short arm to the long arm accompanied/followed by paracentric inversions on 7CL and 7UL) (Figure 4, Supplementary Figure 3).
Chromosome rearrangements could be the outcome of introgressive hybridization with an unknown species, as it was suggested to be involved in the speciation of Ae. markgrafii (Danilova et al., 2017a). The action of gametocidal genes, which induce chromosome breakage in the gametes lacking them during meiosis (Tsujimoto, 1995), cannot be excluded. Interestingly, gametocidal genes were identified in Aegilops species with the C and M genomes on chromosome 3C of Ae. markgrafii (Endo and Katayama, 1978) and Ae. triuncialis (Endo and Tsunewaki, 1975), on 2C of Ae. cylindrica (Endo, 1996) and on chromosome 4Mg of Ae. geniculata (Kynast et al., 2000).
During the speciation of Ae. umbellulata, Ae. caudata, and, to a lesser extent, Ae. comosa, the formation of submeta- or acrocentric chromosomes may result in the accumulation of non-recombining regions with loci for adaptive traits (Parisod and Badaeva, 2020). The large non-recombining haplotype blocks were identified recently in sunflower and were associated with adaptive traits, such as seed size, flowering time, and soil fertility (Todesco et al., 2020). The hypothesis on the involvement of rearranged chromosome structure in adaptive radiation of Ae. markgrafii and Ae. umbellulata relative to Ae. comosa seems to be supported by the geographical distribution of these species, with an east-west shift from Ae. comosa (occurring mainly in Albania, Balkan Peninsula, and Greece) through Ae. markgrafii (abundant in Aegean and Western Turkey) to Ae. umbellulata (abundant in Asia Minor, Anatolia, Transcaucasia, and Iran) (Kilian et al., 2011).
Efficient alien gene transfer to wheat requires the ability to screen large pre-breeding populations for the presence of desirable chromosome segments. While recent improvements in the genotyping platforms based on SNP arrays (King et al., 2017) and genotyping-by-sequencing (GBS)-identified SNPs (Edae et al., 2017) are capable to detect alien chromatin transferred into wheat, their use remains expensive for applied research and breeding to genotype thousands of individuals. Moreover, specific SNPs have to be converted to uniplex (KASP) markers for routine work.
In this study, we propose a new approach for developing molecular markers using the sequences of orthologous genes whose physical positions on chromosomes are determined by single-gene FISH. The advantage of this approach is that the determination of marker position does not rely on genetic mapping, which is challenging in Aegilops (Edae et al., 2017). Moreover, the positions of markers determined by recombination frequency may differ from the actual physical position (Saintenac et al., 2009). The conservative sequences of cDNA probes can be used for comparative cytogenetics in related species, such as wheat, Ae. markgrafii and A. cristatum (Danilova et al., 2014, 2017a; Said et al., 2018), as well as in Ae. comosa and Ae. umbellulata. The results of the sequence similarity search showed that 87.2 and 75% of the cDNAs were located on the same chromosomes in Ae. comosa and Ae. umbellulata, respectively, as determined by single-gene FISH (Figure 6, Supplementary Data 1).
If DNA sequence data are available for the species of interest, the appropriate level of polymorphism in intronic regions permits the development of molecular markers for defined physical positions on alien chromosomes. In this study, we developed 66 PCR-validated markers for 27 single-gene positions BLAST validated on Ae. comosa and 80 markers for 28 cDNA positions BLAST-validated on Ae. umbellulata, which were polymorphic to wheat. The fact that most of the markers produced amplicons in Ae. biuncialis and in wheat-Ae. geniculata chromosome addition lines (Figures 6, 7, Supplementary Data 2) indicates that they will facilitate the introgression of M- and U-genome chromosome segments also from allopolyploid Aegilops species into wheat.
Some chromosome regions remain uncovered with markers, such as chromosome arms 1ML, 2MS, and 6MS, or 2US and 7US. After the ordering of chromosomal scaffolds of Ae. comosa and Ae. umbellulata using the GenomeZipper approach (Mayer et al., 2009), these genomic resources will facilitate the development of additional single-gene FISH probes and molecular markers for targeted chromosomal regions. Moreover, the sequence assemblies obtained from the flow-sorted chromosomes of Ae. comosa and Ae. umbellulata will facilitate the comparative analysis of repeat landscape and gene content of the M- and U-genome chromosomes, as demonstrated for wheat and for the group 5 chromosomes of Ae. tauschii or Ae. geniculata (Lucas et al., 2014; Akpinar et al., 2015; Tiwari et al., 2015). These genomic resources will also open the way for visualization, introgression, and cloning of agronomically important Aegilops genes in wheat.
The single-gene FISH maps obtained in this study uncovered the long-range chromosome organization of Ae. comosa and Ae. umbellulata. The comparative cytogenetic analysis demonstrated that most of the M-genome chromosomes are collinear with those of wheat. The U genome is characterized by significant chromosome rearrangements, and several of them are similar to those in Ae. markgrafii, indicating that the U genome is more similar to the C genome than to the M genome, not only at the sequence level but at the level of chromosome organization. The physically mapped cDNA probes together with the sequence assemblies of flow-sorted chromosomes permitted the development of PCR markers with precisely determined physical positions on the M- and U-genome chromosomes. The chromosome-specific genomic resources, molecular tools, and single-gene FISH maps will support the introgression of Aegilops genes into wheat and their cloning.
Data Availability Statement
The original contributions presented in the study are publicly available. The generated sequence data of Ae. comosa and Ae. umbellulata for this study can be found in DRYAD repository at https://doi.org/10.5061/dryad.wpzgmsbk9 and https://doi.org/10.5061/dryad.70rxwdbwc. The cDNA-sequences were developed by the National BioResource Project-Wheat, Japan and were derived from the following resources available in the public domain https://shigen.nig.ac.jp/wheat/komugi/. The cDNA clones and Ae. comosa accession MvGB1039 and Ae. umbellulata accession AE740/03 together with the other plant genotypes used in the present study are available from the corresponding author upon reasonable request.
Author Contributions
The work involved collaboration between all authors. BF, JD, IM, and MS conceived the research theme. IM and MS designed the experiments and wrote the first draft of the manuscript. MS carried out the single-gene FISH experiments and analyzed the data. PC carried out flow sorting the chromosomes. IM carried out the purity check. KH and JB sequenced the chromosomes. MA and MM-T assembled the chromosomal contigs. BK carried out the BLASTn search and functional annotation of cDNA-sequences. AF, EG, and LI designed and PCR validated the molecular markers. All authors have contributed to read and approved the manuscript.
Funding
This work has been supported by ERDF project Plants as a Tool for Sustainable Global Development (No. CZ.02.1.01/0.0/0.0/16_019/0000827), the Hungarian National Research, Development and Innovation Office (K135057), and the Marie Curie Fellowship Grant award AEGILWHEAT (H2020-MSCA-IF-2016-746253). Computational resources were provided by the ELIXIR-CZ project (LM2015047), a component of the international ELIXIR infrastructure that is part of the project e-Infrastruktura CZ (LM2018140) within the program Projects of Large Research, Development and Innovations Infrastructures.
Conflict of Interest
The authors declare that the research was conducted in the absence of any commercial or financial relationships that could be construed as a potential conflict of interest.
Acknowledgments
The authors would like to thank Dr. Shuhei Nasuda from the Graduate School of Agriculture, Kyoto University, Japan, and Dr. Tatiana Danilova from Kansas State University, USA, for providing the cDNA clones. Z. Dubská, R. Šperková, and J. Weiserová are acknowledged for their assistance with chromosome sorting and preparation of DNA for sequencing.
Supplementary Material
The Supplementary Material for this article can be found online at: https://www.frontiersin.org/articles/10.3389/fpls.2021.689031/full#supplementary-material
References
Akpinar, B. A., Lucas, S. J., Vrána, J., Doležel, J., and Budak, H. (2015). Sequencing chromosome 5D of Aegilops tauschii and comparison with its allopolyploid descendant bread wheat (Triticum aestivum). Plant Biotechnol. J. 13, 740–752. doi: 10.1111/pbi.12302
Appels, R., Eversole, K., Feuillet, C., Keller, B., Rogers, J., Stein, N., et al. (2018). Shifting the limits in wheat research and breeding using a fully annotated reference genome. Science 361:eaar7191. doi: 10.1126/science.aar7191
Badaeva, E. D., Amosova, A. V., Samatadze, T. E., Zoshchuk, S. A., Shostak, N. G., Chikida, N. N., et al. (2004). Genome differentiation in Aegilops. 4. Evolution of the U-genome cluster. Plant Syst. Evol. 246, 45–76. doi: 10.1007/s00606-003-0072-4
Badaeva, E. D., Friebe, B., and Gill, B. S. (1996a). Genome differentiation in Aegilops. 1. distribution of highly repetitive DNA sequences on chromosomes of diploid species. Genome 39, 293–306. doi: 10.1139/g96-040
Badaeva, E. D., Friebe, B., and Gill, B. S. (1996b). Genome differentiation in Aegilops. 2. physical mapping of 5S and 18S-26S ribosomal RNA gene families in diploid species. Genome 39, 1150–1158. doi: 10.1139/g96-145
Bansal, M., Adamski, N. M., Toor, P. I., Kaur, S., Molnár, I., Holušová, K., et al. (2020). Aegilops umbellulata introgression carrying leaf rust and stripe rust resistance genes Lr76 and Yr70 located to 9.47-Mb region on 5DS telomeric end through a combination of chromosome sorting and sequencing. Theor. Appl. Genet. 133, 903–915. doi: 10.1007/s00122-019-03514-x
Bansal, M., Kaur, S., Dhaliwal, H. S., Bains, N. S., Bariana, H. S., Chhuneja, P., et al. (2017). Mapping of Aegilops umbellulata-derived leaf rust and stripe rust resistance loci in wheat. Plant Pathol. 66, 38–44. doi: 10.1111/ppa.12549
Bedbrook, J. R., Jones, J., O'Dell, M., Thompson, R. D., and Flavell, R. B. (1980). A molecular description of telomeric heterochromatin in Secale species. Cell 19, 545–560. doi: 10.1016/0092-8674(80)90529-2
Bolger, A. M., Lohse, M., and Usadel, B. (2014). Trimmomatic: a flexible trimmer for Illumina sequence data. Bioinformatics 30, 2114–2120. doi: 10.1093/bioinformatics/btu170
Burt, C., and Nicholson, P. (2011). Exploiting co-linearity among grass species to map the Aegilops ventricosa-derived Pch1 eyespot resistance in wheat and establish its relationship to Pch2. Theor. Appl. Genet. 123, 1387–1400. doi: 10.1007/s00122-011-1674-9
Cabrera, A., Martin, A., and Barro, F. (2002). In-situ comparative mapping (ISCM) of Glu-1 loci in Triticum and Hordeum. Chrom. Res. 10, 49–54. doi: 10.1023/A:1014270227360
Chapman, J. A., Ho, I., Sunkara, S., Luo, S., Schroth, G. P., and Rokhsar, D. S. (2011). Meraculous: de novo genome assembly with short paired-end reads. PLoS ONE 6:e23501. doi: 10.1371/journal.pone.0023501
Danilova, T. V., Akhunova, A. R., Akhunov, E. D., Friebe, B., and Gill, B. S. (2017a). Major structural genomic alterations can be associated with hybrid speciation in Aegilops markgrafii (Triticeae). Plant J. 92, 317–330. doi: 10.1111/tpj.13657
Danilova, T. V., Friebe, B., and Gill, B. S. (2012). Single-copy gene fluorescence in situ hybridization and genome analysis: Acc-2 loci mark evolutionary chromosomal rearrangements in wheat. Chromosoma 121, 597–611. doi: 10.1007/s00412-012-0384-7
Danilova, T. V., Friebe, B., and Gill, B. S. (2014). Development of a wheat single gene FISH map for analyzing homoeologous relationship and chromosomal rearrangements within the Triticeae. Theor. Appl. Genet. 127, 715–730. doi: 10.1007/s00122-013-2253-z
Danilova, T. V., Friebe, B., Gill, B. S., Poland, J. A., and Jackson, E. G. (2017b). Development of a complete set of wheat–barley group-7 Robertsonian translocation chromosomes conferring an increased content of β-glucan. Theor Appl Genet 131, 377–388. doi: 10.1007/s00122-017-3008-z
Devos, K. M., Atkinson, M. D., Chinoy, C. N., Francis, H. A., Harcourt, R. L., Koebner, R. M. D., et al. (1993). Chromosomal rearrangements in the rye genome relative to that of wheat. Theor. Appl. Genet. 85, 673–680. doi: 10.1007/BF00225004
Doležel, J., Bartoš, J., Voglmayr, H., and Greilhuber, J. (2003). Nuclear DNA content and genome size of trout and human. Cytometry A 51, 127–128; author reply 129. doi: 10.1002/cyto.a.10013
Edae, E. A., Olivera, P. D., Jin, Y., Poland, J. A., and Rouse, M. N. (2016). Genotype-by-sequencing facilitates genetic mapping of a stem rust resistance locus in Aegilops umbellulata, a wild relative of cultivated wheat. BMC Genom. 17:1039. doi: 10.1186/s12864-016-3370-2
Edae, E. A., Olivera, P. D., Jin, Y., and Rouse, M. N. (2017). Genotyping-by-sequencing facilitates a high-density consensus linkage map for Aegilops umbellulata, a wild relative of cultivated wheat. G3 7, 1551–1561. doi: 10.1534/g3.117.039966
Eddy, S. R. (2009). A new generation of homology search tools based on probabilistic inference. Genome Inform. 23, 205–211. doi: 10.1142/9781848165632_0019
Eilam, T., Anikster, Y., Millet, E., Manisterski, J., Sagi-Assif, O., and Feldman, M. (2007). Genome size and genome evolution in diploid Triticeae species. Genome 50, 1029–1037. doi: 10.1139/G07-083
El-Gebali, S., Mistry, J., Bateman, A., Eddy, S. R., Luciani, A., Potter, S. C., et al. (2019). The Pfam protein families database in 2019. Nucleic Acids Res. 47, D427–D432. doi: 10.1093/nar/gky995
Endo, T. R. (1996). Allocation of a gametocidal chromosome of Aegilops cylindrica to wheat homoeologous group 2. Genes Genet. Syst. 71, 243–246. doi: 10.1266/ggs.71.243
Endo, T. R., and Katayama, Y. (1978). Finding of a selectively retained chromosome of Aegilops caudata L. in common wheat. Wheat Inf. Serv. 47/48, 32–35.
Endo, T. R., and Tsunewaki, K. (1975). Sterility of common wheat with Aegilops triuncialis cytoplasm. J Hered 66, 13–18. doi: 10.1093/oxfordjournals.jhered.a108562
Friebe, B., Jiang, J., Raupp, W. J., McIntosh, R. A., and Gill, B. S. (1996). Characterization of wheat-alien translocations conferring resistance to diseases and pests: current status. Euphytica 91, 59–87. doi: 10.1007/BF00035277
Friebe, B. R., Tuleen, N. A., and Gill, B. S. (1999). Development and identification of a complete set of Triticum aestivum- Aegilops geniculata chromosome addition lines. Genome 42, 374–380. doi: 10.1139/g99-011
Gale, M. D., and Devos, K. M. (1998). Plant comparative genetics after 10 years. Science 282, 656–659. doi: 10.1126/science.282.5389.656
Gerlach, W. L., and Bedbrook, J. R. (1979). Cloning and characterization of ribosomal RNA genes from wheat and barley. Nucleic Acids Res. 7, 1869–1885. doi: 10.1093/nar/7.7.1869
Gill, B. S., Sharma, H. C., Raupp, W. J., Browder, L. E., Hatchett, J. H., Harvey, T. L., et al. (1985). Evaluation of Aegilops species for resistance to wheat powdery mildew, wheat leaf rust, hessian fly, and greenbug. Plant Dis. 69, 314–316.
Giorgi, D., Farina, A., Grosso, V., Gennaro, A., Ceoloni, C., and Lucretti, S. (2013). FISHIS: fluorescence in situ hybridization in suspension and chromosome flow sorting made easy. PLOS ONE 8:e57994. doi: 10.1371/journal.pone.0057994
Glémin, S., Scornavacca, C., Dainat, J., Burgarella, C., Viader, V., Ardisson, M., et al. (2019). Pervasive hybridizations in the history of wheat relatives. Sci. Adv. 5:eaav9188. doi: 10.1126/sciadv.aav9188
Han, H., Bai, L., Su, J., Zhang, J., Song, L., Gao, A., et al. (2014). Genetic rearrangements of six wheat-Agropyron cristatum 6P addition lines revealed by molecular markers. PLoS ONE 9:e91066. doi: 10.1371/journal.pone.0091066
Howard, T., Rejab, N. A., Griffiths, S., Leigh, F., Leverington-Waite, M., Simmonds, J., et al. (2011). Identification of a major QTL controlling the content of B-type starch granules in Aegilops. J. Exp. Bot. 62, 2217–2228. doi: 10.1093/jxb/erq423
Huynh, S., Marcussen, T., Felber, F., and Parisod, C. (2019). Hybridization preceded radiation in diploid wheats. Mol. Phylogenet. Evol. 139:106554. doi: 10.1016/j.ympev.2019.106554
Karafiátová, M., Bartoš, J., Kopecký, D., Ma, L., Sato, K., Houben, A., et al. (2013). Mapping nonrecombining regions in barley using multicolor FISH. Chromosome Res. 21, 739–751. doi: 10.1007/s10577-013-9380-x
Kato, A., Albert, P. S., Vega, J. M., and Birchler, J. A. (2006). Sensitive fluorescence in situ hybridization signal detection in maize using directly labeled probes produced by high concentration DNA polymerase nick translation. Biotech. Histochem. 81, 71–78. doi: 10.1080/10520290600643677
Kato, A., Lamb, J. C., and Birchler, J. A. (2004). Chromosome painting using repetitive DNA sequences as probes for somatic chromosome identification in maize. Proc. Natl. Acad. Sci. U.S.A. 101, 13554–13559. doi: 10.1073/pnas.0403659101
Kilian, B., Mammen, K., Millet, E., Sharma, R., Graner, A., Salamini, F., et al. (2011). “Aegilops,” in Wild Crop Relatives: Genomic and Breeding Resources (New York, NY: Spring-Verlag), 1–76. Available online at: https://www.academia.edu/19236227/Aegilops (accessed August 20, 2020).
King, J., Grewal, S., Yang, C.-Y., Hubbart, S., Scholefield, D., Ashling, S., et al. (2017). A step change in the transfer of interspecific variation into wheat from Amblyopyrum muticum. Plant Biotechnol. J. 15, 217–226. doi: 10.1111/pbi.12606
Kubaláková, M., Kovářová, P., Suchánková, P., Číhalíková, J., Bartoš, J., Lucretti, S., et al. (2005). Chromosome sorting in tetraploid wheat and its potential for genome analysis. Genetics 170, 823–829. doi: 10.1534/genetics.104.039180
Kynast, R. G., Friebe, B., and Gill, B. S. (2000). Fate of multicentric and ring chromosomes induced by a new gametocidal factor located on chromosome 4Mg of Aegilops geniculata. Chromosome Res. 8, 133–139. doi: 10.1023/A:1009294519798
Liu, C., Gong, W., Han, R., Guo, J., Li, G., Li, H., et al. (2019). Characterization, identification and evaluation of a set of wheat-Aegilops comosa chromosome lines. Sci. Rep. 9:4773. doi: 10.1038/s41598-019-41219-9
Lucas, S. J., Akpinar, B. A., Šimková, H., Kubaláková, M., Doležel, J., and Budak, H. (2014). Next-generation sequencing of flow-sorted wheat chromosome 5D reveals lineage-specific translocations and widespread gene duplications. BMC Genom. 15:1080. doi: 10.1186/1471-2164-15-1080
Mayer, K. F. X., Taudien, S., Martis, M., Simková, H., Suchánková, P., Gundlach, H., et al. (2009). Gene content and virtual gene order of barley chromosome 1H. Plant Physiol. 151, 496–505. doi: 10.1104/pp.109.142612
McIntosh, R. A., Miller, T. E., and Chapman, V. (1982). Cytogenetical studies in wheat XII. Lr28 for resistance to Puccinia recondita and Sr34 for resistance to P. graminis tritici. Z Pflanzenzüht 89, 295–306.
Miller, T. E., Reader, S. M., and Singh, D. (1988). Spontaneous non-robertsonian translocations between wheat chromosomes and an alien chromosome,” in Proceedings of the Seventh International Wheat Genetics Symposium, held at Cambridge, UK, 13-19 July 1988 (Cambridge: Institute of Plant Sciences Research), 387–390. Available online at: https://www.cabdirect.org/cabdirect/abstract/19891606463 (accessed August 20, 2020).
Molnár, I., Benavente, E., and Molnár-Láng, M. (2009). Detection of intergenomic chromosome rearrangements in irradiated Triticum aestivum-Aegilops biuncialis amphiploids by multicolour genomic in situ hybridization. Genome 52, 156–165. doi: 10.1139/G08-114
Molnár, I., Cifuentes, M., Schneider, A., Benavente, E., and Molnár-Láng, M. (2011). Association between simple sequence repeat-rich chromosome regions and intergenomic translocation breakpoints in natural populations of allopolyploid wild wheats. Ann. Bot. 107, 65–76. doi: 10.1093/aob/mcq215
Molnár, I., Kubaláková, M., Šimková, H., Farkas, A., Cseh, A., Megyeri, M., et al. (2014). Flow cytometric chromosome sorting from diploid progenitors of bread wheat, T. urartu, Ae. speltoides and Ae. tauschii. Theor. Appl. Genet. 127, 1091–1104. doi: 10.1007/s00122-014-2282-2
Molnár, I., Šimková, H., Leverington-Waite, M., Goram, R., Cseh, A., Vrána, J., et al. (2013). Syntenic relationships between the U and M genomes of Aegilops, wheat and the model species brachypodium and rice as revealed by COS markers. PLOS ONE 8:e70844. doi: 10.1371/journal.pone.0070844
Molnár, I., Vrána, J., Burešová, V., Cápal, P., Farkas, A., Darkó, É., et al. (2016). Dissecting the U, M, S and C genomes of wild relatives of bread wheat (Aegilops spp.) into chromosomes and exploring their synteny with wheat. Plant J. 88, 452–467. doi: 10.1111/tpj.13266
Molnár-Láng, M., Ceoloni, C., and Doležel, J., eds. (2015). Alien Introgression in Wheat: Cytogenetics, Molecular Biology, and Genomics. Cham: Springer International Publishing. Available online at: https://www.springer.com/gp/book/9783319234939 (accessed January 30, 2019).
Molnár-Láng, M., Linc, G., and Sutka, J. (1996). Transfer of the recessive crossability allele kr1 from Chinese Spring into the winter wheat variety Martonvásári 9. Euphytica 90, 301–305. doi: 10.1007/BF00027480
Nagaki, K., Tsujimoto, H., Isono, K., and Sasakuma, T. (1995). Molecular characterization of a tandem repeat, Afa family, and its distribution among Triticeae. Genome 38, 479–486. doi: 10.1139/g95-063
Naranjo, T. (2019). The effect of chromosome structure upon meiotic homologous and homoeologous recombinations in Triticeae. Agronomy 9:552. doi: 10.3390/agronomy9090552
Nasuda, S., Friebe, B., and Gill, B. S. (1998). Gametocidal genes induce chromosome breakage in the interphase prior to the first mitotic cell division of the male gametophyte in wheat. Genetics 149, 1115–1124. doi: 10.1093/genetics/149.2.1115
Olivera, P. D., Rouse, M. N., and Jin, Y. (2018). Identification of new sources of resistance to wheat stem rust in Aegilops spp. in the tertiary genepool of wheat. Front. Plant Sci. 9:1719. doi: 10.3389/fpls.2018.01719
Parisod, C., and Badaeva, E. D. (2020). Chromosome restructuring among hybridizing wild wheats. N. Phytol. 226, 1263–1273. doi: 10.1111/nph.16415
Peil, A., Korzun, V., Schubert, V., Schumann, E., Weber, W. E., and Röder, M. S. (1998). The application of wheat microsatellites to identify disomic Triticum aestivum-Aegilops markgrafii addition lines. Theor. Appl. Genet. 96, 138–146. doi: 10.1007/s001220050720
Riley, R., Chapman, V., and Johnson, R. (1968a). Introduction of yellow rust resistance of Aegilops comosa into wheat by genetically induced homoeologous recombination. Nature 217, 383–384. doi: 10.1038/217383a0
Riley, R., Chapman, V., and Johnson, R. (1968b). The incorporation of alien disease resistance in wheat by genetic interference with the regulation of meiotic chromosome synapsis. Genet. Res. 12, 199–219. doi: 10.1017/S0016672300011800
Said, M., Hřibová, E., Danilova, T. V., Karafiátová, M., Čížková, J., Friebe, B., et al. (2018). The Agropyron cristatum karyotype, chromosome structure and cross-genome homoeology as revealed by fluorescence in situ hybridization with tandem repeats and wheat single-gene probes. Theor. Appl. Genet. 131, 2213–2227. doi: 10.1007/s00122-018-3148-9
Said, M., Kubaláková, M., Karafiátová, M., Molnár, I., Doležel, J., and Vrána, J. (2019). Dissecting the complex genome of crested wheatgrass by chromosome flow sorting. Plant Genome 12:180096. doi: 10.3835/plantgenome2018.12.0096
Saintenac, C., Falque, M., Martin, O. C., Paux, E., Feuillet, C., and Sourdille, P. (2009). Detailed recombination studies along chromosome 3B provide new insights on crossover distribution in wheat (Triticum aestivum L.). Genetics 181, 393–403. doi: 10.1534/genetics.108.097469
Schneider, A., Molnár, I., and Molnár-Láng, M. (2008). Utilisation of Aegilops (goatgrass) species to widen the genetic diversity of cultivated wheat. Euphytica 163, 1–19. doi: 10.1007/s10681-007-9624-y
Sears, E. R. (1956). “The transfer of leaf-rust resistance from Aegilops umbellulata to wheat,” in Brook-Haven Symposia in Biology 1956. Available online at: https://www.cabdirect.org/cabdirect/abstract/19581600184 (accessed September 22, 2020).
Šimková, H., Svensson, J. T., Condamine, P., Hřibová, E., Suchánková, P., Bhat, P. R., et al. (2008). Coupling amplified DNA from flow-sorted chromosomes to high-density SNP mapping in barley. BMC Genom. 9:294. doi: 10.1186/1471-2164-9-294
Tanaka, S., Yoshida, K., Sato, K., and Takumi, S. (2020). Diploid genome differentiation conferred by RNA sequencing-based survey of genome-wide polymorphisms throughout homoeologous loci in Triticum and Aegilops. BMC Genomics 21, 246. doi: 10.1186/s12864-020-6664-3
Tiwari, V. K., Wang, S., Danilova, T., Koo, D. H., Vrána, J., Kubaláková, M., et al. (2015). Exploring the tertiary gene pool of bread wheat: sequence assembly and analysis of chromosome 5Mg of Aegilops geniculata. Plant J. 84, 733–746. doi: 10.1111/tpj.13036
Todesco, M., Owens, G. L., Bercovich, N., Légaré, J.-S., Soudi, S., Burge, D. O., et al. (2020). Massive haplotypes underlie ecotypic differentiation in sunflowers. Nature 584, 602–607. doi: 10.1038/s41586-020-2467-6
Tsujimoto, H. (1995). Gametocidal genes in wheat and its relatives. IV. functional relationships between six gametocidal genes. Genome 38, 283–289. doi: 10.1139/g95-035
Untergasser, A., Cutcutache, I., Koressaar, T., Ye, J., Faircloth, B. C., Remm, M., et al. (2012). Primer3–new capabilities and interfaces. Nucleic Acids Res. 40:e115. doi: 10.1093/nar/gks596
van Slageren, M. W. S. J. M. (1994). Wild Wheats: A Monograph of Aegilops L. and Amblyopyrum (Jaub. & Spach) Eig (Poaceae) : A Revision of All Taxa Closely Related to Wheat, Excluding Wild Triticum Species, With Notes on Other Genera in the Tribe Triticcae, Especially Triticum. Wageningen; Aleppo: Wageningen Agricultural University; International Center for Agricultural Research in the Dry Areas.
Vrána, J., Cápal, P., Cíhalíková, J., Kubaláková, M., and Doležel, J. (2016a). Flow sorting plant chromosomes. Methods Mol. Biol. 1429, 119–134. doi: 10.1007/978-1-4939-3622-9_10
Vrána, J., Čápal, P., Šimková, H., Karafiátová, M., Cížková, J., and Doležel, J. (2016b). Flow analysis and sorting of plant chromosomes. Curr. Protoc. Cytometry 78, 5.3.1–5.3.43. doi: 10.1002/cpcy.9
Vrána, J., Kubaláková, M., Šimková, H., Číhalíková, J., Lysák, M. A., and Doležel, J. (2000). Flow sorting of mitotic chromosomes in common wheat (Triticum aestivum L.). Genetics 156, 2033–2041. doi: 10.1093/genetics/156.4.2033
Keywords: goat grasses, Aegilops comosa, Aegilops umbellulata, single-gene FISH, chromosome flow sorting and sequencing, chromosome rearrangements, homoeologous relationships, molecular markers
Citation: Said M, Holušová K, Farkas A, Ivanizs L, Gaál E, Cápal P, Abrouk M, Martis-Thiele MM, Kalapos B, Bartoš J, Friebe B, Doležel J and Molnár I (2021) Development of DNA Markers From Physically Mapped Loci in Aegilops comosa and Aegilops umbellulata Using Single-Gene FISH and Chromosome Sequences. Front. Plant Sci. 12:689031. doi: 10.3389/fpls.2021.689031
Received: 31 March 2021; Accepted: 19 May 2021;
Published: 15 June 2021.
Edited by:
Luigi Cattivelli, Council for Agricultural and Economics Research, ItalyReviewed by:
Bao Liu, Northeast Normal University, ChinaWei Zhang, North Dakota State University, United States
Surbhi Grewal, University of Nottingham, United Kingdom
Copyright © 2021 Said, Holušová, Farkas, Ivanizs, Gaál, Cápal, Abrouk, Martis-Thiele, Kalapos, Bartoš, Friebe, Doležel and Molnár. This is an open-access article distributed under the terms of the Creative Commons Attribution License (CC BY). The use, distribution or reproduction in other forums is permitted, provided the original author(s) and the copyright owner(s) are credited and that the original publication in this journal is cited, in accordance with accepted academic practice. No use, distribution or reproduction is permitted which does not comply with these terms.
*Correspondence: Mahmoud Said, c2FpZEB1ZWIuY2FzLmN6
†ORCID: Mahmoud Said orcid.org/0000-0002-6169-8655
Katerina Holušová orcid.org/0000-0002-4531-7170
András Farkas orcid.org/0000-0002-9545-0332
László Ivanizs orcid.org/0000-0003-0887-9729
Eszter Gaál orcid.org/0000-0002-1081-9656
Petr Cápal orcid.org/0000-0002-8888-9101
Michael Abrouk orcid.org/0000-0001-9082-1432
Mihaela M. Martis-Thiele orcid.org/0000-0001-7509-2446
Balázs Kalapos orcid.org/0000-0003-2340-4270
Jan Bartoš orcid.org/0000-0002-4154-8895
Bernd Friebe orcid.org/0000-0002-5419-1565
Jaroslav Doležel orcid.org/0000-0002-6263-0492
István Molnár orcid.org/0000-0002-7167-9319