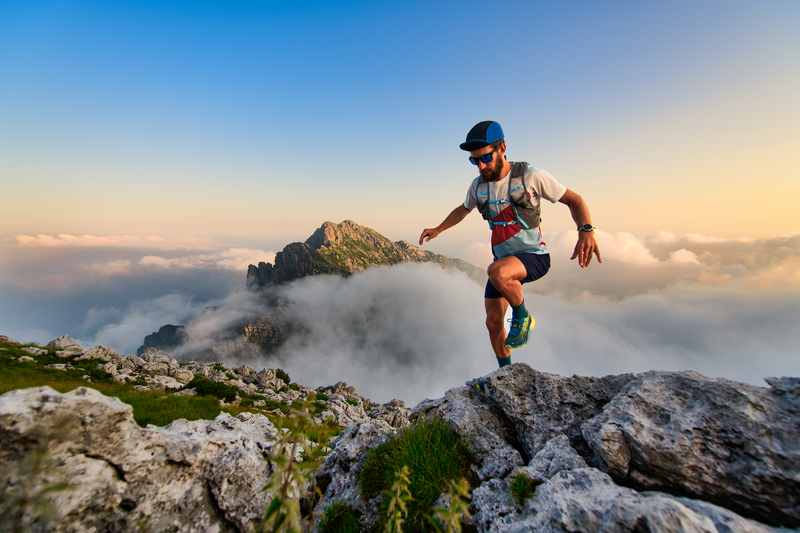
95% of researchers rate our articles as excellent or good
Learn more about the work of our research integrity team to safeguard the quality of each article we publish.
Find out more
ORIGINAL RESEARCH article
Front. Plant Sci. , 28 June 2021
Sec. Plant Symbiotic Interactions
Volume 12 - 2021 | https://doi.org/10.3389/fpls.2021.686075
This article is part of the Research Topic Nutrient Dependent Signaling Pathways Controlling the Symbiotic Nitrogen Fixation Process, Volume II View all 6 articles
Legume plants are able to establish nitrogen-fixing symbiotic relations with Rhizobium bacteria. This symbiosis is, however, affected by a number of abiotic constraints, particularly drought. One of the consequences of drought stress is the overproduction of reactive oxygen (ROS) and nitrogen species (RNS), leading to cellular damage and, ultimately, cell death. Ascorbic acid (AsA), also known as vitamin C, is one of the antioxidant compounds that plants synthesize to counteract this oxidative damage. One promising strategy for the improvement of plant growth and symbiotic performance under drought stress is the overproduction of AsA via the overexpression of enzymes in the Smirnoff-Wheeler biosynthesis pathway. In the current work, we generated Medicago truncatula plants with increased AsA biosynthesis by overexpressing MtVTC2, a gene coding for GDP-L-galactose phosphorylase. We characterized the growth and physiological responses of symbiotic plants both under well-watered conditions and during a progressive water deficit. Results show that increased AsA availability did not provide an advantage in terms of plant growth or symbiotic performance either under well-watered conditions or in response to drought.
Legumes are able to establish nitrogen-fixing symbioses with soil bacteria of the Rhizobiaceae family. This interaction provides a source of nitrogen for plant growth and reproduction, reducing the need for synthetic fertilizers in agricultural systems. However, establishment and functioning of these symbioses is negatively affected by a number of stresses. Among these, water deficit inhibits symbiotic nitrogen fixation (SNF) in both temperate and tropical legumes, although the molecular and physiological bases remain unclear (Serraj et al., 2001; King and Purcell, 2005; Marino et al., 2007; Gil-Quintana et al., 2013a,b). One of the effects of drought stress in plants generally is an overproduction of reactive oxygen (ROS) and nitrogen species (RNS), leading to oxidative damage at the cellular level and, ultimately, cell death (Smirnoff, 1993; Moran et al., 1994; Cruz De Carvalho, 2008). Plants are able to counteract such damage via the production of antioxidant compounds such as ascorbic acid (AsA). AsA, also known as vitamin C, is a water-soluble vitamin essential for plant and animal growth and development. In plants, AsA is involved in a number of physiological processes including photosynthesis, cell wall growth, seed germination, flowering time, and senescence, among others (Foyer and Noctor, 2011; Zechmann, 2011; Mellidou et al., 2012; Tóth et al., 2013). Additionally, it is the substrate of ascorbate peroxidase within the AsA-glutathione (AsA-GSH) or Foyer-Halliwell-Asada pathway (Foyer and Noctor, 2011), which mitigates ROS and RNS damage in plant cells.
The AsA-GSH cycle is important for the maintenance of SNF in root nodules (Matamoros et al., 2003; Becana et al., 2010). Nodule tissue is enriched in the enzymes of the AsA-GSH cycle, and the activities of these enzymes are strongly correlated with nodule effectiveness (Dalton et al., 1993, 1998). A current hypothesis is that increased AsA pools under stressful conditions should lead to improved (or at least less affected) SNF. Indeed, stem infusion of 10 mM AsA has been shown to increase nitrogenase activity 4-fold (based on acetylene reduction measurements) in soybean plants (Bashor and Dalton, 1999). Similarly, in vitro assays using isolated rhizobia, myoglobin/leghemoglobin, 2 mM AsA, and recombinant AsA peroxidase led a significant increase in nitrogenase activity (Ross et al., 1999). Despite these promising results, a formal in planta approach to increase the levels of AsA in nitrogen-fixing legume plants has not been undertaken so far.
Although alternative pathways have been described, the Smirnoff-Wheeler or D-mannose/L-galactose pathway is considered the primary route of AsA biosynthesis in plants (Wheeler et al., 1998; Conklin et al., 1999; Dowdle et al., 2007). The rate-limiting and committed step for AsA biosynthesis is catalyzed by GDP-L-galactose phosphorylase, which is encoded by the genes AtVTC2 and AtVTC5 in the model plant, A. thaliana (Laing et al., 2007; Linster et al., 2007; Smirnoff, 2007). Double mutants in AtVTC2 and AtVTC5 were unable to grow unless supplemented with AsA (Dowdle et al., 2007), demonstrating the key role of these enzymes in the biosynthesis pathway.
In the current work, we tested the hypothesis that increased AsA in nodules can lead to improved symbiotic performance in the model legume, Medicago truncatula. We generated stably-transformed M. truncatula plants with increased AsA biosynthesis by overexpressing the MtVTC2 gene and characterized the growth of plants in symbiosis with Ensifer (Sinorhizobium) meliloti bacteria under well-watered conditions and during progressive water deficit. Results show that higher levels of AsA in nodules did not improve symbiosis establishment/nodule functioning or ameliorate the effects of drought stress on plant performance.
To construct Medicago truncatula lines overexpressing MtVTC2, the coding sequence of the gene Medtr5g093390.1 (including the stop codon) was amplified from a M. truncatula Jemalong A17 leaf cDNA library using Phusion High-Fidelity DNA Polymerase (Thermo Fisher Scientific Inc.). Primers 5′-CACCATGATGCTAAAAATCAAAAGGG-3′ and 5′-TTACTGTAGAACAACACATTCTTGTGAAC-3′ were used for directional cloning into pENTR/D-TOPO (Life Technologies). Sequence-verified constructs were recombined into the destination binary vector pK7RWG2 (https://gatewayvectors.vib.be/) with LR clonase (Invitrogen) and transformed into One Shot TOP10 chemically competent E. coli (Invitrogen). The empty vector pK7RWG2, lacking the MtVTC2 gene, was employed as a control. Sequence-verified binary vectors were transformed into Agrobacterium tumefaciens strain EHA105 and 10 stable transgenic M. truncatula R108 plants per construct were obtained by A. tumefaciens-mediated transformation as previously described (Jiang et al., 2019).
Two microsymbionts were used: Ensifer (Sinorhizobium) meliloti strain 2011 constitutively expressing either LacZ (pXLGD4; Ardourel et al., 1994) or GFP, which are classical reporter genes to monitor bacteroids in nodules. Bacteria were grown at 28°C for 48 h in yeast extract mannitol broth containing (g L−1): mannitol (10), yeast extract (0.4), NaCl (0.1), K2HPO4 (0.5), 0.2 MgSO4·7H2O (Vincent, 1970). Seedlings were inoculated twice with 1 mL of the corresponding strain the day of planting and 1 week afterwards (corresponding to ~1 × 109 colony forming units mL−1).
M. truncatula R108 seeds of the empty vector and overexpressing lines were grown in 1-L pots with a mixture of vermiculite:perlite (5:2, v/v) as substrate under controlled environmental conditions (14-h day/10-h night; 600 μmol m−2 s−1 light intensity; 22/16°C day/night temperature; 70–60% relative humidity). Plants were inoculated as described above and watered with nutrient solution (Evans, 1981) containing 0.25 mM ammonium nitrate for the first 2 weeks. The nutrient solution remained N-free afterwards. Two-month-old plants (i.e., 8 weeks post-inoculation) from each line were separated into three sets according to their values of leaf water potential (Ψleaf). One set of plants (control, C) was maintained to field capacity and the two others were subjected to water deprivation for the establishment of drought stress (mild and severe drought, MD and SD, respectively). To establish the effect of water deficit on plants, transpiration rates, stomatal conductance, leaf and nodule water potentials were measured. Plant transpiration rates were gravimetrically determined daily on a whole-plant basis. Stomatal conductance was measured with an AP4 porometer (Delta-T Devices). Ψleaf was estimated using a Scholander pressure chamber (Soil Moisture Equipment). Stomatal conductance, Ψleaf and chlorophyll content were determined in the youngest fully expanded leaf. Nodule water potential (Ψnodule) was analyzed using C52 sample chambers coupled to a Wescor HR-33T Dew Point Microvoltmeter (Wescor). The relative levels of leaf chlorophyll were estimated using a portable SPAD-502 meter (Soil Plant Analysis Development, Konica-Minolta Inc.). Symbiotic nitrogen fixation was estimated as apparent nitrogenase activity (ANA) according to the method described by Witty and Minchin (1998). H2 evolution of intact plants was measured in an open flow-through system under N2/O2 (79/21%) using an electrochemical H2 sensor (Qubit System Inc.). The H2 sensor was calibrated with high purity gases (Praxair) using a gas mixer (Air Liquide) flowing at the same rate as the sampling system (500 mL min−1).
Plants were physiological characterized, and then, leaf, root and nodule samples were collected and frozen in liquid N2 and stored at −80°C for further analysis. Other set of samples were weighed for fresh weight (FW) determinations and, subsequently, oven-dried at 70°C for 48 h before dry weight (DW) determinations.
The putative VTC2 and VTC5 orthologous genes in M. truncatula and other plant species were identified by BLASTP (cutoff E-value <1E−10, identity >60%) using the A. thaliana proteins VTC2 (AT4G26850.1) and VTC5 (AT5G55120.1) as baits. Subsequently, a HMMER analysis was carried out to further validate the selection of candidates. Both analyses were performed in the Ensembl Plants website (http://plants.ensembl.org/) using default parameters, except for the Lotus japonicus sequences, which were analyzed independently using the Lotus Base website (https://lotus.au.dk/). The predicted amino acid sequences were aligned using MAFFT (http://mafft.cbrc.jp/alignment/server) defining G-INS-i as iterative refinement method and BLOSUM30 as scoring matrix. Phylogenetic analyses were conducted in MEGA7 (Kumar et al., 2016) using the Maximum Likelihood method based on the JTT amino acid substitution model and 1,000 bootstrap replications as previously described (Seminario et al., 2017).
Nodule and leaf AsA levels were measured by high-performance capillary electrophoresis, as previously described (Davey et al., 1996; Seminario et al., 2017). To obtain total AsA pools, samples were treated with dithiothreitol, and DHA levels were calculated as the difference between the total AsA pool and the reduced AsA levels.
Nodule extracts were prepared from 0.1 g FW of nodules, as previously described (Marino et al., 2006). Sucrose was determined by ion-exchange chromatography in a 940 Professional IC Vario with amperometric detector (Metrohm AG) equipped with Metrosep Carb 2 Guard/4.0 + Metrosep Carb 2–150/4.0 columns. Samples were eluted with 300 mM NaOH/1 mM C2H3NaO2 at 30°C.
For RNA extraction, nodules were collected from roots of the M. truncatula EV and oxVTC2 lines 6 weeks post-inoculation and total RNA was extracted in 200 μL GTC lysis buffer (Omega Bio-Tek) containing 4 μL of β-mercaptoethanol. The lysate was then passed through a homogenizer column (Omega Bio-Tek) by centrifugation at 15,000 g and the flow-through was mixed with one volume of 100% ethanol. Nucleic acids were recovered following the Clean and Concentrator-5 protocol (Zymo Research) beginning at step 3 and eluted with 50 μL of nuclease-free water. The solution was treated with Turbo DNase (Invitrogen) to yield purified RNA following the manufacturer's instructions. RNA concentration and quality were measured using the Qubit RNA Broad Range assay (LifeTechnologies). RNA samples were further processed with at least RNA Quality Number values above 6.0 and 28S/18S area ratio values above 0.8. Single stranded cDNA was generated by reverse transcription using SuperScript IV Reverse Transcriptase (LifeTechnologies). Real-time quantitative PCR (qPCR) was performed on a Bio-Rad CFX96 System (Bio-Rad). The PCR reaction consisted of 7.5 μL of GoTaq qPCR Master Mix (Promega), 0.5 μM of forward and reverse primers, and 2.0 μl of template cDNA in a total volume of 15 μl. For the reference gene, the polypyrimidine tract-binding-like gene (PTB, Medtr3g090960) was used, a gene shown to present high transcript stability for Medicago truncatula samples at several developmental stages (Kakar et al., 2008). All reactions were set up in duplicate and three biological samples per genotype were analyzed. The PCR program consisted of a polymerase activation step of 2 min at 95°C, followed by 40 cycles of 15 s at 95°C and 30 s at 60°C. A final melting curve was included to confirm the specificity of the reaction. The software LinRegPCR (Ramakers et al., 2003; Ruijter et al., 2009) was employed to calculate the quantification cycles (Cq) and amplification efficiency of each primer pair. Relative quantification of MtVTC2 transcripts was estimated using the 2−ΔΔCq method (Livak and Schmittgen, 2001) since the differences in primer efficiency were <5%. A list of the primers used can be found as Supplementary Table 1.
Plant nodules were harvested and cut with a vibratome (Lancer Series 1000) in 75 μm-longitudinal sections. The in-situ detection of H2O2 and in nodules was performed as described in Muglia et al. (2008). In plants inoculated with the LacZ-expressing E. meliloti strain, β-galactosidase activity in nodule sections was measured as previously described (Larrainzar et al., 2015). Nodule preparations were visualized with a Zeiss Axioskop2 optical microscope (Zeiss Microscopy) or a Leica fluorescence microscope DM750 (Leica).
The nodule bacteroid fraction was isolated as described by Saalbach et al. (2002). Briefly, 0.1 g FW of nodules were homogenized using a mortar and pestle in 1 ml of ice-cold buffer containing 50 mM HEPES (pH 7.5), 450 mM mannitol, 7 mM ethylenediaminotetraacetic acid (EDTA), 7 mM CaCl2, 5 mM MgCl2, and 5 mM di-thiothreitol (DTT). The mixture was filtered through Miracloth and centrifuged at 500 g for 10 min to remove cell debris. The supernatant was recovered and centrifuged again at 6000 g for 10 min to pellet the bacteroid fraction. The supernatant was discarded and the pellet was washed twice with extraction buffer and centrifuged at 10000 g for 10 min. β-galactosidase activity was measured based on the assay described by Miller (1972), subsequently adapted to a 96-well plate format (Griffith and Wolf, 2002). The bacteroid-containing pellet was resuspended in 1 mL of buffer Z. To permeabilize the bacteroids, 20 μL of 0.1% SDS and 40 μL of chloroform were added. The assay was initiated by adding 20 μL of orthonitrophenyl-β-D-galactopyranoside (ONPG; 4 mg/ml) and the time was noted. As soon as the yellow chromophore ortho-nitrophenol (ONP) was formed, the reaction was terminated by the addition of 50 μL of 1 M Na2CO3. Microplate readings were then determined at 420 and 550 nm, respectively. The β-galactosidase specific activities in Miller units are calculated based on the protein content of the bacteroid samples (Brito et al., 2008).
Results correspond to two independent experiments with a number of biological replicates for each ranging from 3 to 5. Experiments were arranged in a completely randomized design with 5 plants per treatment. The data were subjected to an analysis of two-way ANOVA with plant line as one factor and drought treatment as the other using the SPSS Statistics for Windows, version 24 (Armonk, NY, IBM corp.). Significant differences between treatments were determined by LSD (p ≤ 0.05) and are represented with different letters.
One of the key steps controlling AsA biosynthesis in plants is the conversion of GDP-L-galactose to L-galactose 1-P by GDP-L-galactose phosphorylase (EC 2.7.7.69). In A. thaliana two genes have been identified coding for this enzyme, AtVTC2 (AT4G26850.1) and AtVTC5 (AT5G55120.1; Dowdle et al., 2007). Using the predicted amino acid sequences of these two enzymes, BLASTp search of the M. truncatula genome retrieved the genes Medtr5g093390 (MtrunA17Chr5g0444591 in v5 of the genome; Pecrix et al., 2018) and Medtr3g053020 (MtrunA17Chr3g0099501) as the putative orthologs for AtVTC2 and AtVTC5, respectively. We also performed reciprocal BLASTP followed by HMMER to retrieve additional orthologs within the legume family and built a phylogenetic tree using Brachypodium distachyon as outgroup (Figure 1A). Subsequently, we designated Medtr5g093390 as MtVTC2 and Medtr3g053020 as MtVTC5.
Figure 1. Phylogenetic analysis (A) and RNA-sequencing (RNA-seq) expression data corresponding to the MtVTC2 and MtVTC5 genes. The Maximum Likelihood method was applied to generate a phylogenetic tree (1,000 bootstrap replications) using the software Mega 7 (Kumar et al., 2016). Putative orthologs in A. thaliana, Brachypodium distachyon, Glycine max, Phaseolus vulgaris, and Vigna angularis were retrieved after BLASTP and HMMER analysis at the Ensembl Plants website (http://plants.ensembl.org/). Sequences corresponding to Lotus japonicus were extracted from the Lotus Base using BLASTP (https://lotus.au.dk/blast/) against the Lotus japonicus MG20 v3.0 Proteins database. Data corresponding to gene expression in different plant organs (B), during nodule development (C), and regions of a nitrogen-fixing nodule (D; FI: apical region; FIId and FIIp: distal and a proximal FII fraction, respectively; IZ: interzone; ZIII: nitrogen-fixation zone) was collected from the M. truncatula Small Secreted Peptide database (MtSSPdb; Boschiero et al., 2020; https://mtsspdb.noble.org/database) and Symbimics website (Roux et al., 2014; https://iant.toulouse.inra.fr/symbimics). Values represent the average ± SE (n = 3 biological replicates). FPKM, Fragments Per Kilobase Million. Asterisks denote significant differences (p ≤ 0.05) using ANOVA test with respect to the sample used as a reference: “Leaf” for panel (B), “Nod0” for panel (C), and FI region for panel (D).
We queried the M. truncatula Small Secreted Peptide database (MtSSPdb; Boschiero et al., 2020; https://mtsspdb.noble.org/database) to obtain expression profiles of these two genes in different M. truncatula organs and upon inoculation with symbiotic bacteria (Figures 1B–D). MtVTC2 showed the highest relative expression in the different plant tissues measured, being particularly abundant in leaves and large pods (Figure 1B). In uninoculated roots, both MtVTC2 and MtVTC5 exhibited similar expression levels and neither showed a symbiosis-related increase in gene expression, even in mature nitrogen-fixing nodules at day 28 after inoculation (Figure 1C). Interestingly, fertilization of plants with nitrate, a classical inhibitor of nitrogen fixing activity, did not significantly alter the expression levels of these genes (data not shown). Looking at gene expression profiles in microdissected nodules in the Symbimics database (Roux et al., 2014; https://iant.toulouse.inra.fr/symbimics), both MtVTC2 and MtVTC5 were present in all the nodule regions, with MtVTC5 expression significantly higher in the nitrogen-fixing zone (ZIII), using the meristematic zone (FI) as a reference (Figure 1D). However, in terms of response to abiotic stresses, the expression profile of MtVTC2 showed the most interesting pattern, with a strong induction in roots of M. truncatula subjected to a progressive drought stress (Seminario et al., 2017). Based on this expression analysis, we selected MtVTC2 as a candidate to be overexpressed in nodules to test for improved nitrogen fixation and drought resilience.
To test if increased AsA in nodules could lead to an improved symbiosis or reduce the impact of drought stress, we generated stably transformed M. truncatula plants expressing MtVTC2 under the control of the 35S promoter. From the several lines generated, we selected two independent lines per construct for further studies; lines oxVTC2#2-1 and oxVTC2#4 for MtVTC2 overexpression, and lines EV#4 and EV#6 transformed with the empty vector (EV) as controls. To test the effect of the overexpression of MtVTC2 in the AsA biosynthesis pathway, the levels of AsA, the total AsA pool and the ratio AsA/total AsA pool were measured in both leaves and nitrogen-fixing root nodules (Table 1). In leaf tissue, the oxVTC2 lines presented an average increase of +31% content of AsA compared to the EV lines. In nodules, results show that oxVTC2 lines presented an average increase of +51% content of AsA and +41% of the total AsA pool relative to the EV lines. Accordingly, qPCR analysis showed that the levels of expression of the MtVTC2 in nodules of the oxVTC2 lines was 3.8-fold this of EV plants (Supplementary Figure 1).
Table 1. Analysis of the levels of ascorbic acid (AsA), total AsA pool, and AsA to total AsA pool ratios in leaves and nodules of M. truncatula empty vector (EV) and MtVTC2 overexpression lines (oxVTC2) under well-watered conditions.
Regarding plant performance and symbiotic efficiency, under well-watered conditions both the overexpression and EV lines showed similar growth rates, with average total plant biomass of 2.04 ± 0.15 g DW and 2.19 ± 0.25 g DW, respectively (Table 2). Similarly, we did not observe significant differences in terms of nodule formation, and both sets of plants presented similar relative chlorophyll levels. We also analyzed the levels of nitrogen fixation, measured as apparent nitrogenase activity (ANA) in intact plants, and results show no statistical differences compared to the control lines (Table 2).
Table 2. Physiological characterization of M. truncatula empty vector (EV) and MtVTC2 overexpression lines (oxVTC2) under well-watered conditions.
To test if increased biosynthesis of AsA could affect nodule development or the antioxidant status in nodules, we compared bacteroid occupancy in infected cells and accumulation of ROS in the two sets of lines. We used two E. meliloti 2011 strains constitutively expressing either GFP or LacZ and monitored the intensity levels of the fluorescent protein or the product of the X-Gal reaction in infected cells of the nitrogen-fixing zones of nodules (region FIII) using microscopy. Based on image analysis, nodule occupancy in the oxVTC2 lines was found comparable to those observed in EV nodules (Figure 2). These results were supported by the β-galactosidase activity assay in the nodule bacteroid fraction from both plant lines, with average activity of 18.77 ± 1.19 Miller units μg protein−1 in the EV line and 20.16 ± 4.18 Miller units μg protein−1 in the oxVTC2 line, respectively (n = 4 biological replicates per line).
Figure 2. Representative images of M. truncatula root nodule sections of the EV (A,B) and oxVTC2 lines (C,D) upon inoculation with E. meliloti bacteria constitutively expressing GFP (A,C) or LacZ (B,D). Six weeks after inoculation nodules were collected, sectioned (and stained in the case of LacZ), and visualized using a fluorescent or light microscopy. Selected images are representative images corresponding to observations carried out in two independent experiments. Scale bars represent 500 μm.
Similarly, qualitative comparisons of the levels of H2O2 and did not appear to differ in nodules formed in the EV lines compared to the MtVTC2-overexpressing lines (Supplementary Figure 2).
To check whether the effect of increased AsA content in nodules could minimize the impact of water deficit in M. truncatula, we subjected the plants to progressive drought stress by withholding water for 6 days. Water potential in leaves and nodules, evapotranspiration and stomatal conductance were measured in plants following drought treatment. Both sets of plants showed a decline in leaf water potential levels reaching values around −1.5 and −2 MPa under mild drought (MD) and severe drought (SD) conditions, respectively (Figure 3A). A similar trend was observed for nodule water potential in the four lines tested (Figure 3B). Regarding evapotranspiration, under MD stress we observed a progressive decline in the levels of water lost in the EV and oxVTC2 lines (Figure 4A). Likewise, comparison of the two sets of plants revealed no significant differences in terms of stomatal conductance, with drought resulting in 95% decline in conductance regardless of the plant line (Figure 4B). The only exception was overexpressing line oxVTC2#2, which under control conditions showed relatively higher stomatal closure (Figure 4B). Likewise, plant biomass and chlorophyll levels did not show significant differences during the drought experiment regardless of the lines tested (data not shown).
Figure 3. Effects of progressive drought stress on leaf (A) and nodule (B) water potentials in M. truncatula empty vector (EV) and oxVTC2 plants under well-watered control (C), mild drought (MD), and severe drought (SD) conditions. Values represent the average ± SE (n = 5 biological replicates). Mean values represented by the same letter do not differ statistically (p ≤ 0.05 ANOVA test).
Figure 4. Effects of progressive drought stress on evapotranspiration (A) and stomatal conductance (B) in M. truncatula empty vector (EV) and oxVTC2 plants under well-watered control (C), mild drought (MD), and severe drought (SD) conditions. Values represent the average ± SE (n = 5 biological replicates). Mean values represented by the same letter do not differ statistically (p ≤ 0.05 ANOVA test).
Finally, to test if overexpression of MtVTC2 could prevent drought-induced decline in nitrogen fixation, we measured ANA in the four lines. However, both the EV plants and the overexpression lines showed a similar reduction in the levels of ANA, showing no significant differences among genotypes (Figure 5). The inhibition of symbiotic nitrogen fixation before this of photosynthesis has been well-documented in the literature (Durand et al., 1987; González et al., 1995). Several studies in different plant species have shown that the decline in nitrogen fixation is not due to a limitation in carbohydrate transport to the nodule. In fact, the opposite is observed: sucrose, the main C compound transported to sink tissues, is accumulated in nodules during drought stress. To test if the decline in ANA levels in the present study was also associated to this regulatory mechanism, we measured the levels of sucrose in nodules of the EV and overexpressing lines (Supplementary Figure 3). Results show indeed a progressive accumulation of sucrose in nodules of both plant lines when subjected to water deficit conditions.
Figure 5. Effects of drought stress on nitrogen fixation in M. truncatula empty vector (EV) and oxVTC2 plants under well-watered control (C), mild drought (MD), and severe drought (SD) conditions. Values represent the average ± SE (n = 5 biological replicates). Mean values represented by the same letter do not differ statistically (p ≤ 0.05 ANOVA test). NDW, nodule dry weight.
This drought-induced reduction in nitrogen fixation activity correlated well with the variations on the levels of AsA in leaves and nodules throughout the drought experiment (Figures 6A,C, respectively). In the EV lines, MD reduced the content of AsA in nodules by 30% in EV#4 while no significant effect was observed in EV#6 (Figure 6C). In contrast, SD had a similar impact on both EV lines reaching AsA values around 0.31 ± 0.03 mg g−1 NDW. Moderate levels of water deficit led to a 44 and 38% decline in AsA in lines oxVTC2#2-1 and oxVTC2#4, respectively (Figure 6C). As the stress increased, the content of AsA was reduced to 0.54 ± 0.13 and 0.31 ± 0.05 mg g−1 NDW in oxVTC2#2-1 and oxVTC2#4 lines, respectively. Regarding DHA, line oxVTC2#4 presented the highest DHA values in leaf tissue (Figure 6B), while in nodules oxVTC2#2-1 showed the highest DHA content under control conditions (Figure 6D). However, drought stress had a similar impact in all the lines tested, with a progressive decline as the water deficit became more severe in the case of leaves (Figure 6B), while the decline was much sharper in nodule tissue (Figure 6D). Interestingly, the only exception to this trend in nodules was line EV#6, which did not show significant variations in the content of DHA during the water deficit, most likely due to the relatively low levels of DHA detected in nodules from plants under control conditions (Figure 6D).
Figure 6. Levels of ascorbic acid (AsA) and dehydroascorbic acid (DHA) in leaves (A,B) and nodules (C,D) of M. truncatula empty vector (EV) and oxVTC2 plants under well-watered control (C), mild drought (MD), and severe drought (SD) conditions. Values represent the average ± SE (n = 5 biological replicates). Mean values represented by the same letter do not differ statistically (p ≤ 0.05 ANOVA test). LDW, leaf dry weight; NDW, nodule dry weight.
The enzyme VTC2, or GDP-L-galactose phosphorylase, is considered a control point of ascorbate biosynthesis in plants (Laing et al., 2007; Wolucka and Van Montagu, 2007). Increased expression of the genes coding for this enzyme in different plant species provide evidence for the critical role of this step in the pathway as a way to increase AsA content in plants (Bulley et al., 2009, 2012; Zhang et al., 2015).
Here we characterized the physiological responses of M. truncatula plants overexpressing MtVTC2 under optimal water supply and when subjected to a progressive water deficit under symbiotic conditions. As AsA plays a significant role in ROS scavenging in nodules (Ross et al., 1999; Matamoros et al., 2006; Becana et al., 2010), it might be expected that increasing AsA biosynthesis in symbiotic root nodules would enhance nitrogen fixation and, as a result, increase plant growth. Both MtVTC2 and MtVTC5, the two genes coding for GDP-L-galactose phosphorylases in the M. truncatula genome, are expressed in all plant organs analyzed, including nitrogen-fixing root nodules (Figure 1). Constitutive overexpression of MtVTC2 indeed led to increased AsA content in nodules and this result correlated well with the levels of expression of the corresponding gene (Medtr5g093390), which showed a 3.8-fold increase in nodules when compared with EV plants as measured by qPCR analysis (Supplementary Figure 1). In contrast, the higher content of AsA in nodules did not lead to an increase in nodulation nor improved symbiotic nitrogen fixation rates, with both EV and oxVTC2 lines exhibiting similar values (Table 2). Using E. meliloti bacteria constitutively expressing LacZ, β-galactosidase activity assays in bacteroids isolated from mature nitrogen-fixing nodules did not show significant differences at the level of bacteroid occupancy between the lines (Figure 2). Accordingly, plants did not exhibit higher biomass when grown under exclusively symbiotic conditions (Table 2).
To explore whether the M. truncatula oxVTC2 lines might be more tolerant of stress, which generates ROS, plant responses to drought were measured. Previous work on the VTC2 mutant of A. thaliana, vtc1, showed that it was more sensitive than the wild type to a number of abiotic stresses, including ozone and UV-B radiation (Conklin et al., 1996). Since then, a number of reports have described the potential advantage of bioengineering plants with increased AsA as a strategy to build drought tolerance (e.g., Bao et al., 2016 or Ma et al., 2014). Nevertheless, the experimental estimation of the stress itself and the level of tolerance is often not optimally determined in these works. Experiments aimed at the analysis of the effect of drought stress require the monitoring of physiological parameters such as the plant water potential in order to adequately gauge the degree of water deficit. Such physiological characterization of plants is, however, scarce in the literature, and reports on drought tolerance are often based on morphological aspects such as plant wilting (Bao et al., 2016), without taking into consideration variations in leaf area index or differential plant transpiration rates. Furthermore, the application of compounds such as polyethylene glycol is often equated with drought stress (as in Ma et al., 2014), whereas it should be rather seen as an osmotic contribution to the total water potential. As an example of this variability in experimental approaches, contrasting results regarding the effect of overexpressing VTC2 on plant growth and stress tolerance have been reported. For instance, Zhang et al. (2015) described improved seedling biomass in salt-stressed rice plants overexpressing A. thaliana VTC2, while subsequent studies in rice did not show significant variations in terms of shoot biomass when overexpressing the kiwifruit ortholog (Ali et al., 2019) or the OsVTC2 counterpart (Broad et al., 2020). Again, one important limitation of the conclusions reached by Zhang et al. (2015) is that biomass results were expressed on a fresh-weight basis instead of on a dry-weight basis, as mandatory when working with water-deficit stresses.
In the current work, the physiological characterization of plants showed that both the EV and oxVTC2 lines experienced a similar level of progressive water deficit based on the observed declines in leaf and nodule water potentials (Figure 3). Accordingly, plants rapidly showed a reduction in stomatal conductance, regardless of the overexpression of MtVTC2, despite the involvement of the AsA redox state in guard cell signaling (Chen and Gallie, 2004). Likewise, plant growth was arrested and SNF inhibited in both sets of plants as a consequence of the stress, suggesting that oxVTC2 plants did not present improved drought tolerance.
The fact that nitrogen-fixing legumes with increased antioxidant levels did not show improved plant performance or stress tolerance is disappointing, but not unusual. Previous studies in our laboratory showed that the exogenous application of galactono-1,4-lactone, the precursor for AsA biosynthesis, to nodulated pea plants did not alleviate the negative effect of drought stress either (Zabalza et al., 2008). Furthermore, Medicago sativa plants overexpressing mitochondrial Mn superoxide dismutase also failed to show improved nitrogenase activity or growth either under well-watered conditions or when plants were subjected to drought stress (Rubio et al., 2002). In contrast, positive results were obtained by El Msehli et al. (2011) by overexpressing the enzyme responsible for the biosynthesis of (homo)glutathione, gamma-glutamylcysteine synthetase, in M. truncatula nodules. This overexpression led to improved nitrogen fixation rates (estimated as ARA) and increased expression of a number of symbiotic markers in nodules such as leghemoglobin, although data on plant biomass was not provided. One of the reasons behind the relatively low success of such approaches is the fact that antioxidant compounds such as AsA are key metabolites subjected to a complex regulation and associated to a variety of metabolic pathways, even having regulatory roles on their own. Other factors such as the specific site of production, including subcellular localization, may also play a role in the success of this type of experiments. Additionally, when plants are forced to increase the levels of any regulatory metabolite, this often leads to the unwanted negative effects as a consequence of an impaired metabolism, including stunted plant growth and developmental issues.
The use of constitutive promoters such as the CaMV35S is a matter of debate in the symbiotic scientific community. Although constructs using this promoter are widely used for symbiotic functional analysis in legumes, Auriac and Timmers (2007) presented data suggesting that this promoter was inactive in the nodule meristem and infected cells of Medicago truncatula root nodules. Taking this observation into consideration and even if in the current work the CaMV35S may not drive homogeneous expression through the nodule, the promoter did lead to increased MtVTC2 expression and AsA levels in the tissue. It would be of interest to test whether the use of alternative promoters that are specifically expressed in infected cells of the nitrogen-fixing zone of the nodule leads to a different symbiotic outcome.
In summary, our results show that M. truncatula plants with elevated AsA content can be generated through the overexpression of MtVTC2. However, this increased AsA availability does not provide an advantage in terms of plant growth or symbiotic performance either under well-watered conditions or in response to drought stress.
The original contributions presented in the study are included in the article/Supplementary Material, further inquiries can be directed to the corresponding author.
RH and MU generated the transgenic lines and performed initial experiments. DK performed preliminary screening of transgenic plants and provided qPCR data. RH, DD, and MU conceived the project and supervised preliminary screening of transgenic plants. LC-P performed drought experiments and carried out the physiological characterization of the lines. MR performed microscopy analysis and contributed to the interpretation of the data. EL and CA-I supervised the drought experiments. EL wrote the manuscript with input from all authors.
This work had been funded by the Spanish Ministry of Science and Innovation-European Regional Development Fund (grant RTI2018-094623-B-C22) and the Government of Navarra (project PC112-113 LEGUSI). EL was a Ramón y Cajal fellow (RYC2018-023867-I) and LC-P and MR were Formación de Personal Investigador fellows from the Spanish Ministry of Economy and Competitiveness (BES-2015-074411 and BES-2012-059972, respectively).
The authors declare that the research was conducted in the absence of any commercial or financial relationships that could be construed as a potential conflict of interest.
We acknowledge Lorena Felipe for initial drought experiments and Dr. Jeremy Coate at Reed College for providing qPCR advice, as well as the technical assistance provided by Gustavo Garijo at the Public University of Navarra. We would like to thank Prof. Jose Manuel Palacios for providing the bacteroid beta-galactosidase assay protocol.
The Supplementary Material for this article can be found online at: https://www.frontiersin.org/articles/10.3389/fpls.2021.686075/full#supplementary-material
Supplementary Figure 1. Relative expression of MtVTC2 in nodules of the empty vector (EV) and oxVTC2 lines measured by qPCR using the reference gene PTB (Kakar et al., 2008) for normalization. Values represent the average ± SD fold-change of relative expression in the oxVTC2 lines compared to the EV plants calculated using the 2−ΔΔCq method (Livak and Schmittgen, 2001) (n = 6, three biological and two technical replicates).
Supplementary Figure 2. Representative images of M. truncatula root nodule sections of the empty vector (EV, A,B) and oxVTC2 lines (C,D) after staining for the detection of superoxide using nitrobluetetrazolium (A,C) or H2O2 using 3,3′-diaminobenzidine-HCl (B,D). Scale bars represent 500 μm.
Supplementary Figure 3. Levels of sucrose in nodules of M. truncatula empty vector (EV) and oxVTC2 plants under well-watered control (C), mild drought (MD), and severe drought (SD) conditions. Values represent the average ± SE (n = 5 biological replicates). Mean values represented by the same letter do not differ statistically (p ≤ 0.05 ANOVA test). NDW, nodule dry weight.
Supplementary Table 1. List of primers for used for qPCR analysis.
AsA, ascorbic acid; DHA, dehydroascorbate; Ψw, water potential; WC, water content; FW, fresh weight; DW, dry weight; C, control; SNF, symbiotic nitrogen fixation; ANA, apparent nitrogenase activity.
Ali, B., Pantha, S., Acharya, R., Ueda, Y., Wu, L. B., Ashrafuzzaman, M., et al. (2019). Enhanced ascorbate level improves multi-stress tolerance in a widely grown indica rice variety without compromising its agronomic characteristics. J. Plant Physiol. 240:152998. doi: 10.1016/j.jplph.2019.152998
Ardourel, M., Demont, N., Debelle, F., Maillet, F., de Billy, F., Prome, J. C., et al. (1994). Rhizobium meliloti lipooligosaccharide nodulation factors: different structural requirements for bacterial entry into target root hair cells and induction of plant symbiotic developmental responses. Plant Cell 6, 1357–1374. doi: 10.1105/tpc.6.10.1357
Auriac, M. C., and Timmers, A. C. J. (2007). Nodulation studies in the model legume Medicago truncatula: Advantages of using the constitutive EF1? promoter and limitations in detecting fluorescent reporter proteins in nodule tissues. Mol. Plant-Microbe Interact. 20, 1040–1047. doi: 10.1094/MPMI-20-9-1040
Bao, G., Zhuo, C., Qian, C., Xiao, T., Guo, Z., and Lu, S. (2016). Co-expression of NCED and ALO improves vitamin C level and tolerance to drought and chilling in transgenic tobacco and stylo plants. Plant Biotechnol. J. 14, 206–214. doi: 10.1111/pbi.12374
Bashor, C. J., and Dalton, D. A. (1999). Effects of exogenous application and stem infusion of ascorbate on soybean (Glycine max) root nodules. New Phytol. 142, 19–26. doi: 10.1046/j.1469-8137.1999.00370.x
Becana, M., Matamoros, M. A., Udvardi, M., and Dalton, D. A. (2010). Recent insights into antioxidant defenses of legume root nodules. New Phytol. 188, 960–976. doi: 10.1111/j.1469-8137.2010.03512.x
Boschiero, C., Dai, X., Lundquist, P. K., Roy, S., de Bang, T. C., Zhang, S., et al. (2020). MtSSPdb: the Medicago truncatula small secreted peptide database. Plant Physiol. 183, 399–413. doi: 10.1104/pp.19.01088
Brito, B., Toffanin, A., Prieto, R. I., Imperial, J., Ruiz-Argüeso, T., and Palacios, J. M. (2008). Host-dependent expression of Rhizobium leguminosarum bv. viciae hydrogenase is controlled at transcriptional and post-transcriptional levels in legume nodules. Mol. Plant Microbe Interact. 21, 597–604. doi: 10.1094/MPMI-21-5-0597
Broad, R. C., Bonneau, J. P., Beasley, J. T., Roden, S., Sadowski, P., Jewell, N., et al. (2020). Effect of rice GDP-L-galactose phosphorylase constitutive overexpression on ascorbate concentration, stress tolerance, and iron bioavailability in rice. Front. Plant Sci. 11:595439. doi: 10.3389/fpls.2020.595439
Bulley, S., Rassam, M., Hoser, D., Otto, W., Schünemann, N., Wright, M., et al. (2009). Gene expression studies in kiwifruit and gene over-expression in Arabidopsis indicates that GDP-L-galactose guanyltransferase is a major control point of vitamin C biosynthesis. J. Exp. Bot. 60, 765–778. doi: 10.1093/jxb/ern327
Bulley, S., Wright, M., Rommens, C., Yan, H., Rassam, M., Lin-Wang, K., et al. (2012). Enhancing ascorbate in fruits and tubers through over-expression of the L-galactose pathway gene GDP-L-galactose phosphorylase. Plant Biotechnol. J. 10, 390–397. doi: 10.1111/j.1467-7652.2011.00668.x
Chen, Z., and Gallie, D. R. (2004). The ascorbic acid redox state controls guard cell signaling and stomatal movement. Plant Cell 16, 1143–1162. doi: 10.1105/tpc.021584
Conklin, P. L., Norris, S. R., Wheeler, G. L., Williams, E. H., Smirnoff, N., and Last, R. L. (1999). Genetic evidence for the role of GDP-mannose in plant ascorbic acid (vitamin C) biosynthesis. Proc. Natl. Acad. Sci. U.S.A. 96, 4198–4203. doi: 10.1073/pnas.96.7.4198
Conklin, P. L., Williams, E. H., and Last, R. L. (1996). Environmental stress sensitivity of an ascorbic acid-deficient Arabidopsis mutant. Proc. Natl. Acad. Sci. U.S.A. 93, 9970–9974. doi: 10.1073/pnas.93.18.9970
Cruz De Carvalho, M. H. (2008). Drought stress and reactive oxygen species: production, scavenging, and signaling. Plant Signal. Behav. 3, 156–165. doi: 10.4161/psb.3.3.5536
Dalton, D. A., Joyner, S. L., Becana, M., Iturbe-Ormaetxe, I., and Chatfield, J. M. (1998). Antioxidant defenses in the peripheral cell layers of legume root nodules. Plant Physiol. 116, 37–43. doi: 10.1104/pp.116.1.37
Dalton, D. A., Langeberg, L., and Treneman, N. C. (1993). Correlations between the ascorbate-glutathione pathway and effectiveness in legume root nodules. Physiol. Plant 87, 365–370. doi: 10.1111/j.1399-3054.1993.tb01743.x
Davey, M. W., Bauw, G., and Van Montagu, M. (1996). Analysis of ascorbate in plant tissues by high-performance capillary zone electrophoresis. Anal. Biochem. 239, 8–19. doi: 10.1006/abio.1996.0284
Dowdle, J., Ishikawa, T., Gatzek, S., Rolinski, S., and Smirnoff, N. (2007). Two genes in Arabidopsis thaliana encoding GDP-L-galactose phosphorylase are required for ascorbate biosynthesis and seedling viability. Plant J. 52, 673–689. doi: 10.1111/j.1365-313X.2007.03266.x
Durand, J. L., Sheehy, J. E., and Minchin, F. R. (1987). Nitrogenase activity, photosynthesis and nodule water potential in soyabean plants experiencing water deprivation. J. Exp. Bot. 38, 311–321. doi: 10.1093/jxb/38.2.311
El Msehli, S., Lambert, A., Baldacci-Cresp, F., Hopkins, J., Boncompagni, E., Smiti, S. A., et al. (2011). Crucial role of (homo)glutathione in nitrogen fixation in Medicago truncatula nodules. New Phytol. 192, 496–506. doi: 10.1111/j.1469-8137.2011.03810.x
Evans, H. (1981). “Symbiotic nitrogen fixation in legume nodules,” in Research Experiences in Plant Physiology, ed T. Moore (New York, NY: Springer Berlin Heidelberg), 294–311.
Foyer, C. H., and Noctor, G. (2011). Ascorbate and glutathione: the heart of the redox hub. Plant Physiol. 155, 2–18. doi: 10.1104/pp.110.167569
Gil-Quintana, E., Larrainzar, E., Arrese-Igor, C., and González, E. M. (2013a). Is N-feedback involved in the inhibition of nitrogen fixation in drought-stressed Medicago truncatula? J. Exp. Bot. 64, 281–292. doi: 10.1093/jxb/ers334
Gil-Quintana, E., Larrainzar, E., Seminario, A., Díaz-Leal, J. L., Alamillo, J. M., Pineda, M., et al. (2013b). Local inhibition of nitrogen fixation and nodule metabolism in drought-stressed soybean. J. Exp. Bot. 64, 2171–2182. doi: 10.1093/jxb/ert074
González, E. M., Gordon, A. J., James, C. L., and Arrese-lgor, C. (1995). The role of sucrose synthase in the response of soybean nodules to drought. J. Exp. Bot. 46, 1515–1523. doi: 10.1093/jxb/46.10.1515
Griffith, K. L., and Wolf, R. E. (2002). Measuring β-galactosidase activity in bacteria: cell growth, permeabilization, and enzyme assays in 96-well arrays. Biochem. Biophys. Res. Commun. 290, 397–402. doi: 10.1006/bbrc.2001.6152
Jiang, Q., Fu, C., and Wang, Z. Y. (2019). A unified Agrobacterium-mediated transformation protocol for alfalfa (Medicago sativa L.) and Medicago truncatula. Methods Mol. Biol. 1864, 153–163. doi: 10.1007/978-1-4939-8778-8_11
Kakar, K., Wandrey, M., Czechowski, T., Gaertner, T., Scheible, W. R., Stitt, M., et al. (2008). A community resource for high-throughput quantitative RT-PCR analysis of transcription factor gene expression in Medicago truncatula. Plant Methods 4:18. doi: 10.1186/1746-4811-4-18
King, C., and Purcell, L. (2005). Inhibition of N2 fixation in soybean is associated with elevated ureides and amino acids. Plant Physiol. 137, 1389–1396. doi: 10.1104/pp.104.056317
Kumar, S., Stecher, G., and Tamura, K. (2016). MEGA7: molecular evolutionary genetics analysis version 7.0 for bigger datasets. Mol. Biol. Evol. 33, 1870–1874. doi: 10.1093/molbev/msw054
Laing, W. A., Wright, M. A., Cooney, J., and Bulley, S. M. (2007). The missing step of the L-galactose pathway of ascorbate biosynthesis in plants, an L-galactose guanyltransferase, increases leaf ascorbate content. Proc. Natl. Acad. Sci. U.S.A. 104, 9534–9539. doi: 10.1073/pnas.0701625104
Larrainzar, E., Riely, B. K., Kim, S. C., Carrasquilla-Garcia, N., Yu, H. J., Hwang, H. J., et al. (2015). Deep sequencing of the Medicago truncatula root transcriptome reveals a massive and early interaction between nodulation factor and ethylene signals. Plant Physiol. 169, 233–265. doi: 10.1104/pp.15.00350
Linster, C. L., Gomez, T. A., Christensen, K. C., Adler, L. N., Young, B. D., Brenner, C., et al. (2007). Arabidopsis VTC2 encodes a GDP-L-galactose phosphorylase, the last unknown enzyme in the Smirnoff-Wheeler pathway to ascorbic acid in plants. J. Biol. Chem. 282, 18879–18885. doi: 10.1074/jbc.M702094200
Livak, K. J., and Schmittgen, T. D. (2001). Analysis of relative gene expression data using real-time quantitative PCR and the 2−ΔΔCT method. Methods 25, 402–408. doi: 10.1006/meth.2001.1262
Ma, L., Wang, Y., Liu, W., and Liu, Z. (2014). Overexpression of an alfalfa GDP-mannose 3, 5-epimerase gene enhances acid, drought and salt tolerance in transgenic Arabidopsis by increasing ascorbate accumulation. Biotechnol. Lett. 36, 2331–2341. doi: 10.1007/s10529-014-1598-y
Marino, D., Frendo, P., Ladrera, R., Zabalza, A., Puppo, A., Arrese-Igor, C., et al. (2007). Nitrogen fixation control under drought stress. Localized or systemic? Plant Physiol. 143, 1968–1974. doi: 10.1104/pp.107.097139
Marino, D., González, E. M., and Arrese-Igor, C. (2006). Drought effects on carbon and nitrogen metabolism of pea nodules can be mimicked by paraquat: evidence for the occurrence of two regulation pathways under oxidative stresses. J. Exp. Bot. 57, 665–673. doi: 10.1093/jxb/erj056
Matamoros, M. A., Dalton, D. A., Ramos, J., Clemente, M. R., Rubio, M. C., and Becana, M. (2003). Biochemistry and molecular biology of antioxidants in the rhizobia-legume symbiosis. Plant Physiol. 133, 499–509. doi: 10.1104/pp.103.025619
Matamoros, M. A., Loscos, J., Coronado, M. J., Ramos, J., Sato, S., Testillano, P. S., et al. (2006). Biosynthesis of ascorbic acid in legume root nodules. Plant Physiol. 141, 1068–1077. doi: 10.1104/pp.106.081463
Mellidou, I., Keulemans, J., Kanellis, A. K., and Davey, M. W. (2012). Regulation of fruit ascorbic acid concentrations during ripening in high and low vitamin C tomato cultivars. BMC Plant Biol. 12:239. doi: 10.1186/1471-2229-12-239
Miller, J. (1972). Experiments in Molecular Genetics. New York, NY: Cold Spring Harbor Laboratory Press, Cold Spring Harbor.
Moran, J. F., Becana, M., Iturbe-Ormaetxe, I., Frechilla, S., Klucas, R. V., and Aparicio-Tejo, P. (1994). Drought induces oxidative stress in pea plants. Planta 194, 346–352. doi: 10.1007/BF00197534
Muglia, C., Comai, G., Spegazzini, E., Riccillo, P. M., and Aguilar, O. M. (2008). Glutathione produced by Rhizobium tropici is important to prevent early senescence in common bean nodules. FEMS Microbiol. Lett. 286, 191–198. doi: 10.1111/j.1574-6968.2008.01285.x
Pecrix, Y., Staton, S. E., Sallet, E., Lelandais-Brière, C., Moreau, S., Carrère, S., et al. (2018). Whole-genome landscape of Medicago truncatula symbiotic genes. Nat. Plants 4, 1017–1025. doi: 10.1038/s41477-018-0286-7
Ramakers, C., Ruijter, J. M., Lekanne Deprez, R. H., and Moorman, A. F. M. (2003). Assumption-free analysis of quantitative real-time polymerase chain reaction (PCR) data. Neurosci. Lett. 339, 62–66. doi: 10.1016/S0304-3940(02)01423-4
Ross, E. J. H., Kramer, S. B., and Dalton, D. A. (1999). Effectiveness of ascorbate and ascorbate peroxidase in promoting nitrogen fixation in model systems. Phytochemistry 52, 1203–1210. doi: 10.1016/S0031-9422(99)00407-0
Roux, B., Rodde, N., Jardinaud, M. F., Timmers, T., Sauviac, L., Cottret, L., et al. (2014). An integrated analysis of plant and bacterial gene expression in symbiotic root nodules using laser-capture microdissection coupled to RNA sequencing. Plant J. 77, 817–837. doi: 10.1111/tpj.12442
Rubio, M. C., González, E. M., Minchin, F. R., Webb, K. J., Arrese-Igor, C., Ramos, J., et al. (2002). Effects of water stress on antioxidant enzymes of leaves and nodules of transgenic alfalfa overexpressing superoxide dismutases. Physiol. Plant. 115, 531–540. doi: 10.1034/j.1399-3054.2002.1150407.x
Ruijter, J. M., Ramakers, C., Hoogaars, W. M. H., Karlen, Y., Bakker, O., van den hoff, M. J. B., et al. (2009). Amplification efficiency: linking baseline and bias in the analysis of quantitative PCR data. Nucleic Acids Res. 37:e45. doi: 10.1093/nar/gkp045
Saalbach, G., Erik, P., and Wienkoop, S. (2002). Characterisation by proteomics of peribacteroid space and peribacteroid membrane preparations from pea (Pisum sativum) symbiosomes. Proteomics 2, 325–337. doi: 10.1002/1615-9861(200203)2:3<325::aid-prot325>3.0.co;2-w
Seminario, A., Song, L., Zulet, A., Nguyen, H. T., González, E. M., and Larrainzar, E. (2017). Drought stress causes a reduction in the biosynthesis of ascorbic acid in soybean plants. Front. Plant Sci. 8:1042. doi: 10.3389/fpls.2017.01042
Serraj, R., Vadez, V., and Sinclair, T. R. (2001). Feedback regulation of symbiotic N2 fixation under drought stress. Agronomie 21, 621–626. doi: 10.1051/agro:2001153
Smirnoff, N. (1993). The role of active oxygen in the response of plants to water deficit and desiccation. New Phytol. 125, 27–58. doi: 10.1111/j.1469-8137.1993.tb03863.x
Smirnoff, N. (2007). Antioxidants and Reactive Oxygen Species in Plants. Hoboken, NJ: Blackwell Publishing Ltd.
Tóth, S. Z., Schansker, G., and Garab, G. (2013). The physiological roles and metabolism of ascorbate in chloroplasts. Physiol. Plant 148, 161–175. doi: 10.1111/ppl.12006
Vincent, J. M. (1970). A manual for the practical study of the root-nodule bacteria. J. Appl. Ecol. 8:977. doi: 10.2307/2402718
Wheeler, G. L., Jones, M. A., and Smirnoff, N. (1998). The biosynthetic pathway of vitamin C in higher plants. Nature 393, 365–369. doi: 10.1038/30728
Witty, J. F., and Minchin, F. R. (1998). Hydrogen measurements provide direct evidence for a variable physical barrier to gas diffusion in legume nodules. J. Exp. Bot. 49, 1015–1020. doi: 10.1093/jxb/49.323.1015
Wolucka, B. A., and Van Montagu, M. (2007). The VTC2 cycle and the de novo biosynthesis pathways for vitamin C in plants: An opinion. Phytochemistry 68, 2602–2613. doi: 10.1016/j.phytochem.2007.08.034
Zabalza, A., Gálvez, L., Marino, D., Royuela, M., Arrese-Igor, C., and González, E. M. (2008). The application of ascorbate or its immediate precursor, galactono-1,4-lactone, does not affect the response of nitrogen-fixing pea nodules to water stress. J. Plant Physiol. 165, 805–812. doi: 10.1016/j.jplph.2007.08.005
Zechmann, B. (2011). Subcellular distribution of ascorbate in plants. Plant Signal. Behav. 6, 360–363. doi: 10.4161/psb.6.3.14342
Zhang, G. Y., Liu, R. R., Zhang, C. Q., Tang, K. X., Sun, M. F., Yan, G. H., et al. (2015). Manipulation of the rice L-galactose pathway: evaluation of the effects of transgene overexpression on ascorbate accumulation and abiotic stress tolerance. PLoS ONE 10:e0125870. doi: 10.1371/journal.pone.0125870
Keywords: ascorbic acid, legume, symbiosis, water deficit, antioxidant
Citation: Cobos-Porras L, Rubia MI, Huertas R, Kum D, Dalton DA, Udvardi MK, Arrese-Igor C and Larrainzar E (2021) Increased Ascorbate Biosynthesis Does Not Improve Nitrogen Fixation Nor Alleviate the Effect of Drought Stress in Nodulated Medicago truncatula Plants. Front. Plant Sci. 12:686075. doi: 10.3389/fpls.2021.686075
Received: 26 March 2021; Accepted: 03 June 2021;
Published: 28 June 2021.
Edited by:
Maurizio Chiurazzi, National Research Council (CNR), ItalyReviewed by:
William Laing, The New Zealand Institute for Plant and Food Research Ltd, New ZealandCopyright © 2021 Cobos-Porras, Rubia, Huertas, Kum, Dalton, Udvardi, Arrese-Igor and Larrainzar. This is an open-access article distributed under the terms of the Creative Commons Attribution License (CC BY). The use, distribution or reproduction in other forums is permitted, provided the original author(s) and the copyright owner(s) are credited and that the original publication in this journal is cited, in accordance with accepted academic practice. No use, distribution or reproduction is permitted which does not comply with these terms.
*Correspondence: Estíbaliz Larrainzar, ZXN0aWJhbGl6LmxhcnJhaW56YXJAdW5hdmFycmEuZXM=
†Present address: Raúl Huertas, The James Hutton Institute, Dundee, United Kingdom
Disclaimer: All claims expressed in this article are solely those of the authors and do not necessarily represent those of their affiliated organizations, or those of the publisher, the editors and the reviewers. Any product that may be evaluated in this article or claim that may be made by its manufacturer is not guaranteed or endorsed by the publisher.
Research integrity at Frontiers
Learn more about the work of our research integrity team to safeguard the quality of each article we publish.