- Noble Research Institute, LLC, Ardmore, OK, United States
Virus-induced gene silencing (VIGS) is a rapid and powerful method to evaluate gene function, especially for species like hexaploid wheat that have large, redundant genomes and are difficult and time-consuming to transform. The Brome mosaic virus (BMV)-based VIGS vector is widely used in monocotyledonous species but not wheat. Here we report the establishment of a simple and effective VIGS procedure in bread wheat using BMVCP5, the most recently improved BMV silencing vector, and wheat genes PHYTOENE DESATURASE (TaPDS) and PHOSPHATE2 (TaPHO2) as targets. Time-course experiments revealed that smaller inserts (~100 nucleotides, nt) were more stable in BMVCP5 and conferred higher silencing efficiency and longer silencing duration, compared with larger inserts. When using a 100-nt insert and a novel coleoptile inoculation method, BMVCP5 induced extensive silencing of TaPDS transcript and a visible bleaching phenotype in the 2nd to 5th systemically-infected leaves from nine to at least 28 days post inoculation (dpi). For TaPHO2, the ability of BMVCP5 to simultaneously silence all three homoeologs was demonstrated. To investigate the feasibility of BMV VIGS in wheat roots, ectopically expressed enhanced GREEN FLUORESCENT PROTEIN (eGFP) in a transgenic wheat line was targeted for silencing. Silencing of eGFP fluorescence was observed in both the maturation and elongation zones of roots. BMVCP5 mediated significant silencing of eGFP and TaPHO2 mRNA expression in roots at 14 and 21 dpi, and TaPHO2 silencing led to the doubling of inorganic phosphate concentration in the 2nd through 4th systemic leaves. All 54 wheat cultivars screened were susceptible to BMV infection. BMVCP5-mediated TaPDS silencing resulted in the expected bleaching phenotype in all eight cultivars examined, and decreased TaPDS transcript was detected in all three cultivars examined. This BMVCP5 VIGS technology may serve as a rapid and effective functional genomics tool for high-throughput gene function studies in aerial and root tissues and in many wheat cultivars.
Introduction
Bread wheat (Triticum aestivum L.) is the second largest food crop in the world by acreage (USDA ERS., 2018). Biotic and abiotic stresses, such as rust pathogens and drought (Ellis et al., 2014; Lesk et al., 2016; Yu et al., 2018; Babu et al., 2020), and deficiency or poor use of nutrients, particularly nitrogen and phosphate (López-Arredondo et al., 2014; Chen and Liao, 2017; Hawkesford, 2017), limit wheat production. The dramatic increase in global wheat production during the past several decades was mainly due to the adoption of high-yielding and input-responsive wheat varieties (Tadesse et al., 2016). Continued improvement in wheat production by developing broadly adapted, abiotic and biotic stress tolerant, nutrient and water efficient, and high yielding varieties requires the continued utilization of modern breeding and biotechnological approaches.
Bread wheat has a large and complex genome (~17 Gb, ~129,000 genes, with 80–90% repetitive sequences), and significant genome variation (~36% or ~47,000 genes) exists among cultivars (Wanjugi et al., 2009; Safar et al., 2010; Montenegro et al., 2017). Being an allohexaploid, bread wheat contains three diploid sets of seven chromosomes each from three parents (2n = 6x = 42, genomes AABBDD) (Feldman and Levy, 2012). In the Chinese Spring reference genome, more than half of the high-confidence protein-coding genes are present as exactly three homoeologous copies (one copy per subgenome A, B, and D), and homoeologs on average share over 95% similarity within their coding regions (Adamski et al., 2020). Transcriptional analysis revealed that 70% of the three homoeologous copies show balanced and ubiquitous expression (Ramírez-González et al., 2018). Functional redundancy between homoeologs is commonly observed in wheat due to the high similarity in sequence and expression pattern among homoeologs (Uauy, 2017). The increasing availability of genetic and genomic resources, transcriptomic platforms and novel biotechnology approaches greatly facilitate our understanding of gene function in this species (Jia et al., 2018). However, functional redundancy between homoeologs makes it difficult to perform functional genomics research in wheat using stable genetics or mutant resources. Extended time is required to identify plants with all homoeologs modified even with cultivars having more rapid seeding cycles and new biotechnology techniques such as genome-editing allowing analysis in the first generation of stable transformants (Zhao et al., 2016; Liang et al., 2017, 2018; Zong et al., 2017; Adamski et al., 2020).
Virus-induced gene silencing (VIGS), a transient RNA-silencing technology that exploits the plant antiviral defense system (Baulcombe, 1999; Vance and Vaucheret, 2001; Voinnet, 2001), could overcome many of the difficulties associated with other procedures for studying gene function in this species. To initiate VIGS, a host gene fragment is expressed through the viral vector during infection of the host plant, which triggers the accumulation of virus-derived small interfering RNAs (siRNAs) and consequently leads to sequence-specific degradation of both the viral RNA and the mRNA transcribed from the host target gene (Baulcombe, 2004; Csorba et al., 2009). VIGS is capable of silencing a single gene, a subset of or all homoeologs, or even a gene family. As an alternative to stable transformation to knock down gene expression, VIGS provides a simple, rapid, inexpensive and effective way for gene function characterization in plants. It can be used for high-throughput prescreening of candidate genes identified through genome-wide association studies or RNA-sequencing analysis before using more definitive but also more time-consuming gene function analyses (Scofield and Nelson, 2009; Adamski et al., 2020).
In the past two decades, over 50 plant VIGS vectors have been generated (Dommes et al., 2019). However, most VIGS vectors functioning in eudicots are not effective in monocots (Bekele et al., 2019; Dommes et al., 2019; Kant and Dasgupta, 2019). Among those developed for monocotyledons, three have been applied to silence genes in wheat. Barley stripe mosaic virus (BSMV) has been extensively employed to assess gene function in wheat, as well as other Triticeae species (Dommes et al., 2019; Li et al., 2020; Zhou et al., 2020). The recently developed Foxtail mosaic virus (FoMV)-based VIGS system triggered efficient gene silencing in a broad range of host plants, including wheat (Liu et al., 2016; Mei et al., 2016). A modified Chinese wheat mosaic virus (CWMV) mediated efficient silencing of wheat genes at low temperature (Yang et al., 2018). Selection of a particular VIGS vector for use is influenced by the biosafety laws of the country and institution where the interested researcher resides and by any previous history the researcher may have with a particular vector. Therefore, it is worthwhile to introduce additional vector options that may better fulfill researcher and biosafety requirements.
Brome mosaic virus (BMV) is a popular VIGS vector for monocotyledonous species such as maize, sorghum, rice, barley, and tall fescue (Singh et al., 2018; Dommes et al., 2019; Jiao et al., 2021). Recently, an improved BMV VIGS vector, BMVCP5, displayed greater gene insert stability and enhanced target gene silencing in maize, compared with its older version (Ding et al., 2018). Although wheat is a natural host of BMV (Mise and Pocsai, 2004; Hodge et al., 2019), no BMV VIGS studies have been reported in wheat. To date, most BMV-mediated VIGS studies analyzed gene function in leaves (Pacak et al., 2010a; van der Linde et al., 2011; Sun et al., 2013; Ding et al., 2018), and one study in sorghum showed genes in the inflorescence could be silenced (Singh et al., 2018). The ability of this BMV vector to silence gene expression in other tissues has not been reported. In this study, we chose wheat homologs of PHYTOENE DESATURASE (PDS) and PHOSPHATE2 (PHO2) as targets to evaluate the feasibility of BMV VIGS system in wheat leaves and roots. PDS, a key enzyme for the synthesis of β-carotenoids necessary for photoprotection among other functions (Ruiz-Sola and Rodríguez-Concepción, 2012), has been targeted in many VIGS systems due to the easily observed bleaching phenotype on leaves associated with its silencing (Yuan et al., 2011; Liu et al., 2016; Yang et al., 2018). PHO2, encoding an ubiquitin-conjugating E2 enzyme, negatively regulates inorganic phosphate (Pi) uptake and root-to-shoot translocation (Aung et al., 2006; Bari et al., 2006). In reciprocal grafting experiments with Arabidopsis, a pho2 (PHO2 loss of function mutant) genotype root resulted in increased Pi accumulation in grafted wild-type (normal PHO2) shoot, and a wild-type root is sufficient to restore wild-type levels of Pi in the grafted pho2 genotype shoot (Bari et al., 2006). Findings from that study indicate that PHO2 may serve as a target to study VIGS activity in roots, and indeed, BSMV-mediated silencing of PHO2 homologs in barley resulted in the expected Pi accumulation in leaves (Pacak et al., 2010b).
Here we report that BMVCP5 silencing vector, targeting TaPDS and TaPHO2 or eGFP, functions in leaves, shoots and roots, as well as in multiple hexaploid wheat cultivars. Additionally, we investigated the optimal insert size for this vector to maintain gene silencing in wheat.
Results
A Simple and Efficient Method for BMV-Based VIGS in Wheat
BMV is a tripartite RNA virus with a genome comprising three positive-sense RNAs, RNAs 1–3 (Noueiry and Ahlquist, 2003). The improved BMV-based two-part silencing vector, BMVCP5, is Agrobacterium-based with RNAs 1 and 2 expressed from binary plasmid pC13/F1 + 2, and RNA 3 expressed from binary plasmid pC13/F3CP5 (Ding et al., 2018). Containing modifications from the previous BMV VIGS protocols for maize and sorghum (Zhu et al., 2014; Ding et al., 2018; Singh et al., 2018) and optimized based on the findings from this study (see below and discussion), a simple and effective BMVCP5 VIGS procedure was established in wheat (Figure 1). Major steps of this protocol include: (1) construction of the BMV VIGS vector by inserting a target gene fragment of ~100 base pair (bp) into the NcoI and AvrII sites, located in the 3′ UTR of RNA3 immediately after the stop codon of the coat protein, of pC13/F3CP5 in an antisense orientation; (2) amplification of the VIGS vector in Nicotiana benthamiana leaves via agro-infiltration; (3) rub-inoculation of coleoptiles of 3 day-old or leaf blades of 7–9 day-old wheat seedlings using crude sap from infected N. benthamiana leaves; and (4) growing the inoculated wheat plants at 19–22°C for 2–4 weeks before silencing characterization.
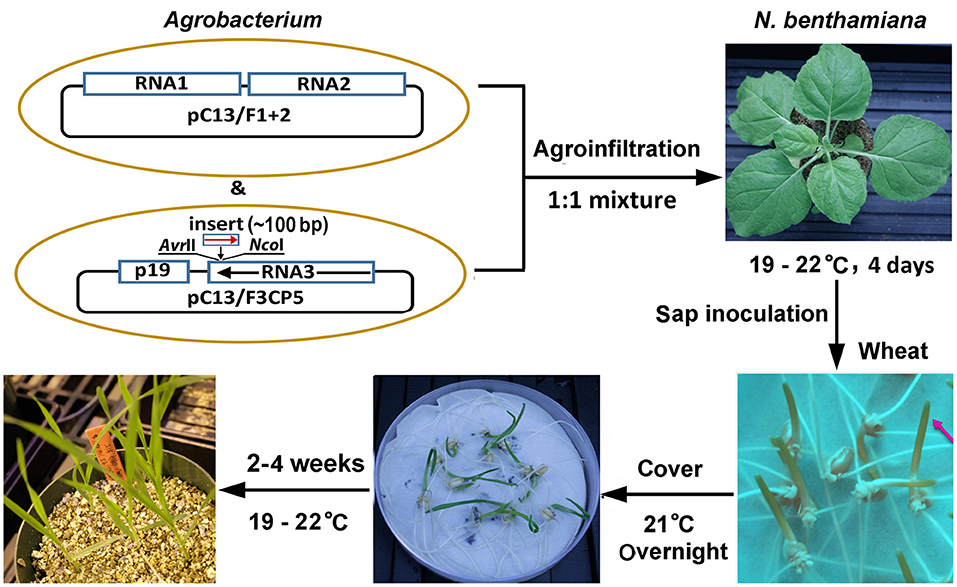
Figure 1. Protocol for BMVCP5-mediated gene silencing in wheat. Binary vectors pC13/F1 + 2 and pC13/F3CP5 contain sequences allowing expression of RNAs 1 and 2 and RNA 3, respectively, of the BMVCP5 silencing vector. For VIGS, a host gene fragment, optimally of ~100-bp, is inserted into the NcoI and AvrII sites in RNA3 sequence of pC13/F3CP5 in an antisense orientation. Agrobacterium containing the binary vectors with BMVCP5 are infiltrated into Nicotiana benthamiana leaves for multiplication of the viral silencing vector. N. benthamiana leaves are harvested at 4 days post infiltration, and crude sap is prepared for mechanical (rub) inoculation to wheat coleoptiles at 3 days post germination (or wheat leaves at 7–9 days post germination). Red arrow points to coleoptile for inoculation. Inoculated wheat seedlings are covered, kept at room temperature (21°C) overnight, and then transferred to solid media (or a hydroponic growth system) and grown in a greenhouse at 19–22°C for 2–4 weeks for target gene silencing.
Determining Optimal Insert Size for BMVCP5 VIGS in Wheat
TaPDS and TaPHO2 were chosen as silencing targets for proof-of-concept studies to determine the feasibility of BMV VIGS system in hexaploid winter wheat cultivar (cv.) Overley. To ensure only the three homoeologs of TaPDS or TaPHO2 were targeted, insert sequences were chosen at a highly specific and conserved region within the target genes. Different size inserts for the same target gene overlapped with each other to share as much common sequence as possible (Supplementary Figure 1). The stability of TaPDS, TaPHO2, and eGFP fragments varying in size from 100 to 252 nt in the BMV vector was evaluated. Vectors carrying eGFP fragments, which have no target in the wheat genome, were used as a non-silencing vector control in VIGS studies targeting TaPDS or TaPHO2. Such a control, as opposed to one without an insert, would produce an infection likely more similar in virus accumulation and disease effect on the host to those induced by the viruses inserted with fragments of target genes (Zhu et al., 2014).
Before inoculating the wheat seedlings, the stabilities of the inserts in the virus vector in N. benthamiana sap were analyzed by reverse transcription polymerase chain reaction (RT-PCR), using primers flanking the insert location. All gene fragment inserts were stably maintained in the BMVCP5 silencing vector extracted from N. benthamiana leaves at 4 days post infiltration except for a minor insert loss from the 252-nt TaPHO2 insert (Supplementary Figure 2). N. benthamiana leaf sap containing the BMV vector progeny was then rub-inoculated on the first two leaves of 9 day-old wheat seedlings. Analysis of insert stability in infected wheat demonstrated that longer inserts were more rapidly lost than shorter inserts (Figure 2). For eGFP, the 220-nt insert was partially lost in the 1st inoculated leaf at 4 dpi in 1 out of 5 plants, in the 2nd inoculated leaf at 7 dpi in 2 out of 5 plants, and completely lost in the 3rd systemic leaf above the inoculated leaves at 20 dpi in 2 out of 5 plants (Figure 2A). The 107- and 180-nt fragments were maintained in all leaves analyzed at all dpi. For TaPDS, the 250-nt insert was partially lost in 1 out of 5 plants but shorter inserts remained intact in the 1st inoculated leaf at 4 dpi (Figure 2B). In the 2nd inoculated leaf at 7 dpi, the 100- and 200-nt inserts were stable in all 5 plants, while the 150- and 250-nt inserts were partially or completely lost in over half the plants. Lastly, in the 3rd systemic leaf at 20 dpi, all the 150-, 200-, and 250-nt inserts were completely lost, but the 100-nt insert was still intact in most of the infected plants. Similar results were obtained with TaPHO2, where the 114-nt insert was more stable than the 150-, 204-, and 252-nt inserts (Figure 2C). Taken together, shorter gene fragment inserts (~100 nt) were generally more stable than longer inserts in BMVCP5 during infection of wheat leaves.
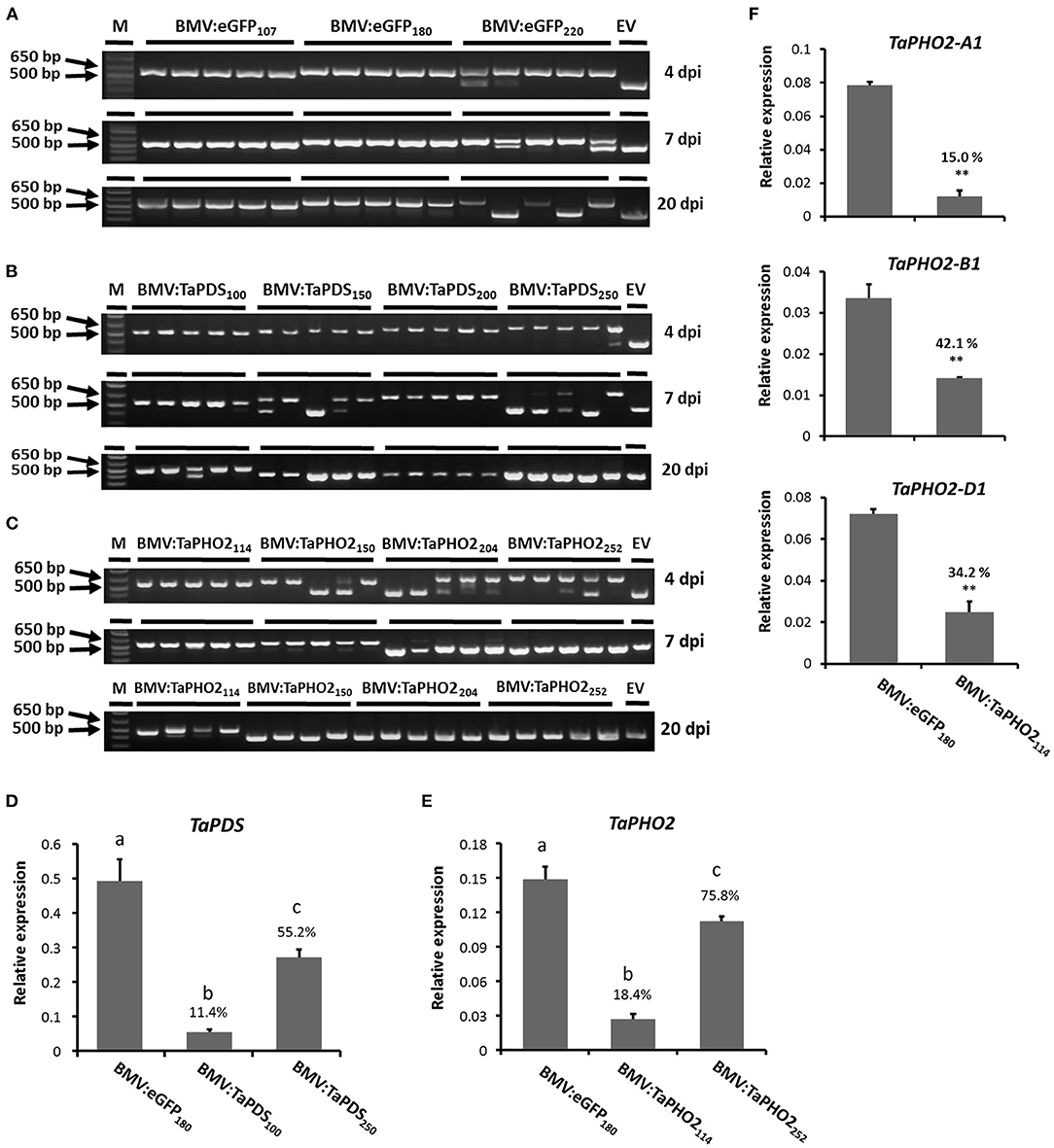
Figure 2. Stability of insert fragments and gene silencing in wheat leaves with BMVCP5. RT-PCR products from wheat leaves of plants inoculated with BMVCP5 harboring gene fragments in length from 100 to 252 nt from eGFP (A), TaPDS (B), and TaPHO2 (C). Subscript numbers after target gene designation indicate the insert size in the BMV vector. The 1st or 2nd inoculated leaf or 3rd systemic leaf were harvested, respectively, at 4, 7, and 20 dpi, and total RNA extracted for RT-PCR amplification using primers flanking the cloning site (P4-F/P4-R). Each lane represents an RT-PCR product from an individual plant. Similar results were obtained in three independent experiments. A 1 kb plus DNA ladder (M, Invitrogen) and a 427-bp PCR product amplified from plasmid pC13/F3CP5 with no insert (EV) serve as size markers. (D–F) Relative expression levels of target gene mRNA in the 3rd systemic leaf at 20 dpi were determined by RT-qPCR using the same cDNA analyzed for insert stability in Panels (A–C) and primers specific for the host target mRNA (but not the relevant insert fragment). PCR product quantities for target genes were normalized against the levels of wheat translation elongation factor subunit EF1α (TaEF1α) mRNA. (D) Relative expression levels of TaPDS mRNA in plants infected with BMV:eGFP180, BMV:TaPDS100 or BMV:TaPDS250. (E) Relative expression levels of TaPHO2 mRNA in plants infected with BMV:eGFP180, BMV:TaPHO2114 or BMV:TaPHO2252. (F) Relative expression levels of TaPHO2-A1, TaPHO2-B1, TaPHO2-D1 mRNA in plants infected with BMV:eGFP180 or BMV:TaPHO2114. Values in panels (D–F) represent means + SE of four or five biological replicates. Significant differences between treatment mean values in panels (D,E) are indicated by different letters above bars for each treatment (P < 0.05, significant ANOVA followed by LSD analysis); treatment values in panel (F) were compared by t-test (**P < 0.01). Percentage values are relative to the BMV:eGFP180 control.
Next, to compare the silencing effects induced by the smallest and largest inserts, transcript abundance of TaPDS and TaPHO2 in the 3rd systemic leaf at 20 dpi from plants inoculated with BMVCP5 vectors containing the 100- and 250-nt TaPDS fragments and the 114- and 252-nt TaPHO2 fragments was investigated by quantitative reverse transcription PCR (RT-qPCR). Samples from plants inoculated with BMV:eGFP180 were analyzed as a non-silencing control. Primers simultaneously amplifying all three homoeologs of TaPDS or TaPHO2 were synthesized. Transcripts representing the TaPDS homoeologs were greatly down-regulated in plants infected with BMV:TaPDS100: an 89% decrease on average compared with the control (Figure 2D). Interestingly, even though the BMV:TaPDS250 vector had completely lost its insert in the 3rd systemic leaves (Figure 2B), TaPDS transcripts were knocked down to 55% of the BMV:eGFP180 control. Similarly, a large decrease in TaPHO2 transcripts was observed in the 3rd systemic leaf at 20 dpi infected with BMV:TaPHO2114 (81%), but not with BMV:TaPHO2252 (24%; Figure 2E). In summary, BMVCP5 with a smaller insert, 100-nt for TaPDS or 114-nt for TaPHO2, induced greater silencing of the target gene than virus with a larger insert, 250-nt TaPDS or 252-nt TaPHO2. These data show that insert length was inversely related to the insert stability and target transcript silencing with the BMVCP5 VIGS system in wheat, and an optimal insert size for this vector is around 100 nt.
Although the 114-nt TaPHO2 insert had 100% identity with each of the three target homoeologs (Supplementary Figure 1), we wanted to verify that transcript from each homoeolog was downregulated. RT-qPCR analysis was performed using homoeolog-specific primers (representing TaPHO2-A1, -B1, and -D1) and the same cDNA templates that were used for analysis of the overall TaPHO2 expression in Figure 2E. All three homoeologs were silenced in this tissue but at different levels, with expression of TaPHO2-A1, -B1, and -D1 decreased to 15, 42, and 34%, respectively, relative to the values from BMV:eGFP180-infected control tissue (Figure 2F).
BMVCP5-Mediated TaPDS Silencing Features in Wheat Leaves
Analysis of the target gene silencing efficiency (amount of visible silencing per leaf) and effectiveness (number and position of leaves showing silencing) mediated by the BMVCP5 VIGS system helps determine its usefulness for functional studies in wheat (see Senthil-Kumar and Mysore, 2011 and Dommes et al., 2019 for further description of these silencing characteristic categories). These characteristics were analyzed in systemic leaves during plant development using TaPDS as the target. In wheat seedlings inoculated with BMV:TaPDS100 via the leaf blade rub-inoculation method, longitudinal photo-bleaching patches often appeared on the 2nd through 5th systemic leaves where virus disease symptoms often developed (Supplementary Figure 3A). The white bleaching phenotype associated with TaPDS silencing was clearly distinct from the yellow chlorosis phenotype in plants infected with BMV:eGFP180. Significant silencing of TaPDS genes in the 3rd systemic leaves at 20 dpi (Figure 2D), 4th systemic leaves at 28 dpi and 5th systemic leaves at 35 dpi were detected at the transcript level (Supplementary Figure 3B). Insert stability analysis revealed that the majority of virus vectors maintained their full-length insert, regardless of gene source, at 28 dpi, but by 35 dpi the majority of leaves contained virus that had lost some or all of the respective gene fragment inserts (Supplementary Figure 3C). By contrast, photobleaching caused by the 250-nt insert more often occurred in the 3rd and 4th systemic leaves, appeared more intense (whiter) than that induced by the 100-nt insert, and did not show in succeeding leaves.
Target gene transcript silencing, along with silencing efficiency and effectiveness, were further investigated in wheat leaves using the coleoptile inoculation method and the BMV vector containing TaPDS fragments of various lengths. When coleoptiles were inoculated with BMV:TaPDS100 there was >64% decrease in the level of TaPDS transcript in the 2nd to 5th systemic leaves relative to the BMV:eGFP180 control (Figures 3A–D). Bleaching was observed in the 2nd to 5th systemic leaves infected with BMV:TaPDS100 compared with the sparse green-yellow streaking phenotype associated with leaves infected with BMV:eGFP180 (Figure 3E). Thus, the coleoptile inoculation method induced efficient and effective visible silencing. For the larger inserts (150, 200, and 250 nt), the level of target transcript silencing varied but generally decreased more rapidly than that induced by the 100-nt insert over time (Figures 3A–D; Supplementary Figure 4A shows transcript values for individual plants from each treatment). Consistent with previous observations, the bleaching phenotype looked more intense (whiter) but the silencing frequency (number of plants showing silencing/total plants treated) and effectiveness were decreased for the longer inserts compared with the 100-nt insert (Table 1). This loss in target gene transcript silencing was positively correlated with the relatively quicker loss of the insert in succeeding leaves from the vectors with inserts longer than 100 nt (Supplementary Figure 4B).
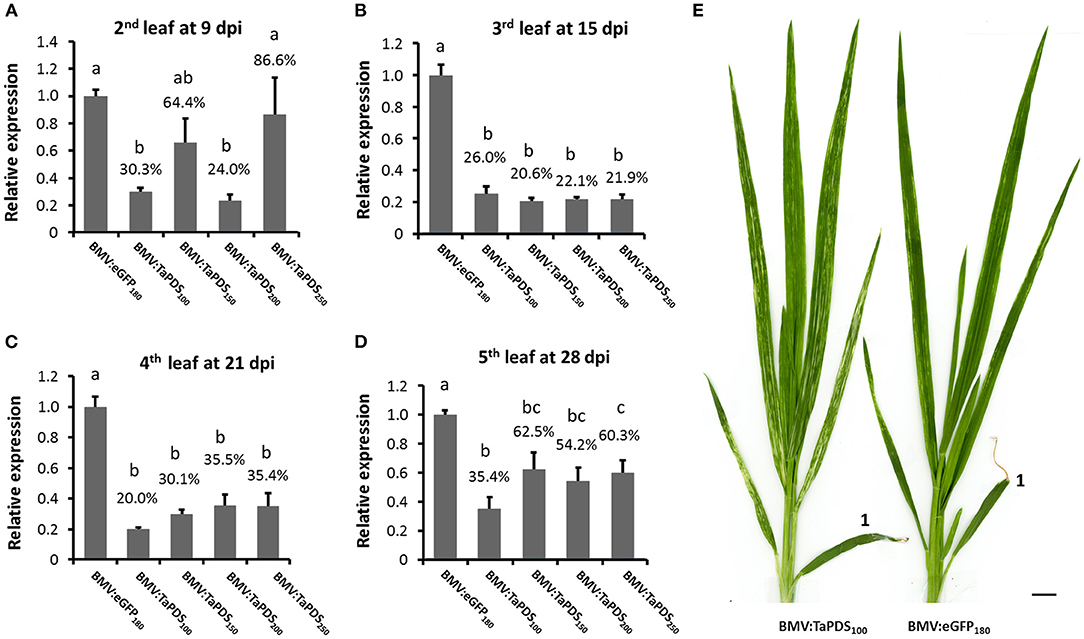
Figure 3. Time-course analysis of BMVCP5-mediated silencing of TaPDS in wheat leaves. (A–D) Groups of five wheat plants were inoculated with BMVCP5 containing TaPDS fragments of 100, 150, 200, or 250 nt, or a 180-nt eGFP fragment using the coleoptile inoculation method. Relative expression levels of TaPDS mRNA in various systemically-infected leaves at various dpi were determined by RT-qPCR with values across treatments normalized against relative TaEF1α mRNA levels. Expression percentages are given relative to the BMV:eGFP180 control. Values represent means + SE of five biological replicates for all treatments except BMV:TaPDS150, in which four biological replicates were used. Different letters denote significant difference between treatments (P < 0.05, significant ANOVA followed by LSD analysis). (E) Plants infected by BMV:PDS100 or BMV:eGFP180 at 28 dpi. The first leaf above the inoculated coleoptile is marked with “1.” Bar = 1 cm. A consistent bleaching phenotype and silencing of TaPDS transcripts were detected in the 2nd to 5th systemic leaves of wheat plants infected with BMV:PDS100. Similar results were obtained in two independent experiments.
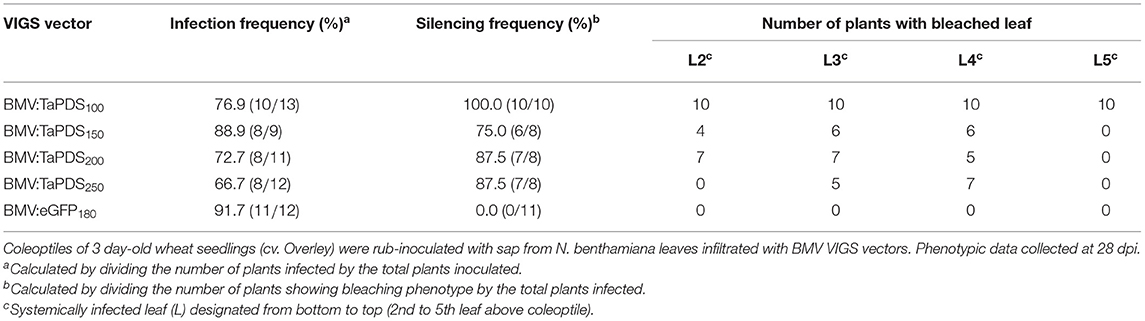
Table 1. Comparison of the infection and silencing features of BMVCP5 containing different TaPDS inserts.
To investigate whether an even shorter insert in BMVCP5 could induce gene silencing, a 52-bp TaPDS fragment, which overlapped with the 100-bp insert tested above, was cloned into BMVCP5 for wheat infection. No bleaching phenotype was observed on any systemic leaves from a total of over 20 infected plants in two independent experiments (Supplementary Figure 5A). However, RT-qPCR analysis revealed knockdown of TaPDS transcripts to 32% of control tissue values in the 4th systemic leaf at 25 dpi (Supplementary Figure 5B), in which the majority of samples maintained a vector with full-length insert (Supplementary Figure 5C).
BMVCP5-Induced Gene Silencing in Wheat Roots
To explore whether BMVCP5 can mediate gene silencing in roots, VIGS of a visual marker gene, eGFP, in homozygous T3 lines of transgenic OsRCg2GFP spring wheat (cv. Fielder) was investigated. Concurrently, the accumulation of BMVCP5 in roots of inoculated plant was detected by an antibody-based tissue-blotting assay (Nelson et al., 1993). Without BMVCP5 infection, GFP fluorescence was primarily detected in the elongation zone and the central stele of the mature region of the root (Xue et al., 2016, Figures 4A,B), and no or very weak background signal of the BMV capsid protein was observed after probing tissue with polyclonal antibody against the BMV capsid protein (Figure 4C). Upon infection with BMV:eGFP180, strong signal representing BMV capsid protein was exhibited in all root regions except the root cap (Figure 4D), indicating the presence of BMVCP5 and the potential to silence gene expression in those root regions. Relative to roots infected with BMVCP5 without an insert (BMV:00), GFP fluorescence was greatly decreased in both the elongation zone and the mature region of roots at 21 dpi with BMV:eGFP180 (Figures 4E,F), with 51 and 66% reduction, respectively, compared to the BMV:00 control (Figures 4G,H), indicating BMVCP5 significantly silenced target gene expression in the host roots.
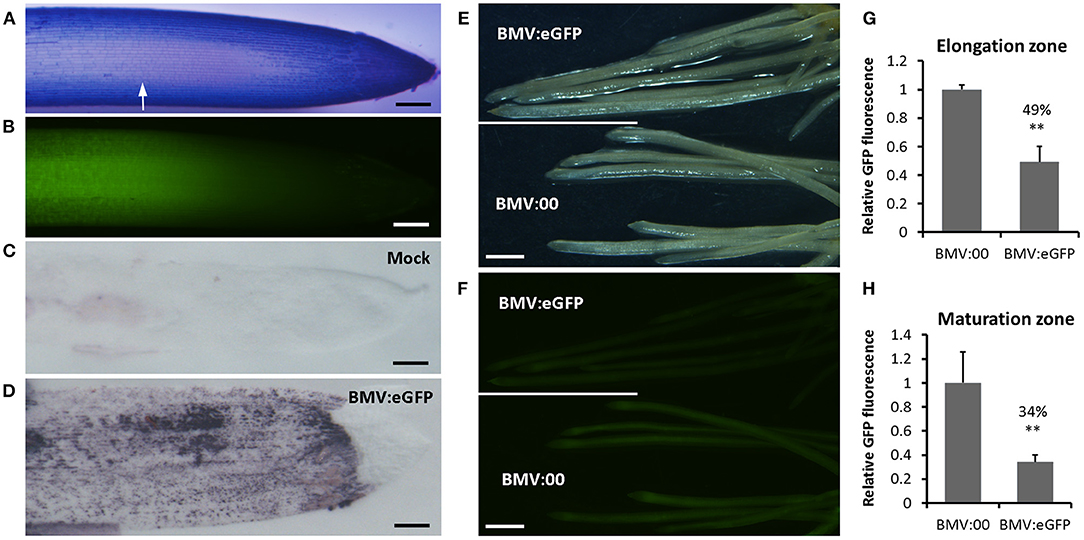
Figure 4. Silencing transgenically-expressed GFP in wheat roots with BMVCP5. (A) Root apical region from transgenic OsRCg2GFP wheat (cv. fielder) seedlings expressing GFP stained with toluidine blue. White arrow indicates the start of the elongation zone. (B) Green fluorescence from GFP detected in the elongation zone of the same root as in panel (A). Accumulation of BMV in a wheat root from plants inoculated with sap of N. benthamiana leaves without virus (Mock, C), or containing BMV:eGFP (180-nt insert; D), detected by tissue-print assay using a polyclonal antibody against the BMV CP. Purple color indicates presence of virus. Bars in (A–D) = 200 μm. (E) Bright-field image of roots at 21 dpi from plants infected with BMV:eGFP or BMV:00 (no insert control). (F) Fluorescence from roots in panel E showing silencing of GFP in transgenic OsRCg2GFP wheat roots after infection with BMV:eGFP, but not BMV:00. Images taken with an Olympus SZX 12 fluorescence microscope. Bars (E,F) = 1 mm. Similar results were obtained in three independent experiments. Quantification of GFP fluorescence in the elongation zone (G) and maturation zone (H) of roots at 21 dpi from plants infected with BMV:00 or BMV:eGFP. Values represent means + SD of six to eight biological replicates, and treatment values compared (t-test; **P < 0.01). Percentage values are relative to the BMV:00 treatment.
To further understand the silencing characteristics of BMVCP5 VIGS in wheat roots and compare them with those in aerial tissues, time-course experiments were performed targeting eGFP in transgenic OsRCg2GFP spring wheat and TaPHO2 in winter wheat (cv. Overley). After an initial lack of effect at 7 dpi with BMV:eGFP180, eGFP mRNA in roots was significantly decreased, exhibiting ~37 and 44% of the level in roots infected with BMV:00 at 14 and 21 dpi, respectively (Figure 5A). By contrast, higher percentages of eGFP silencing were detected in shoots of plants infected with BMV:eGFP180 at 7, 14, and 21 dpi than in the root tissue (Figure 5A). Transcript silencing of TaPHO2 in root tissue was observed at all dpi with BMV:TaPHO2114: ~69, 46, and 41% relative to transcript level present in root tissue infected with BMV:eGFP107 at 10, 14, and 21 dpi, respectively (Figure 5B). Interestingly, as observed for eGFP silencing in shoots vs. roots, higher percentages of TaPHO2 mRNA silencing were detected in leaves vs. roots at all time-points (Figure 5B).
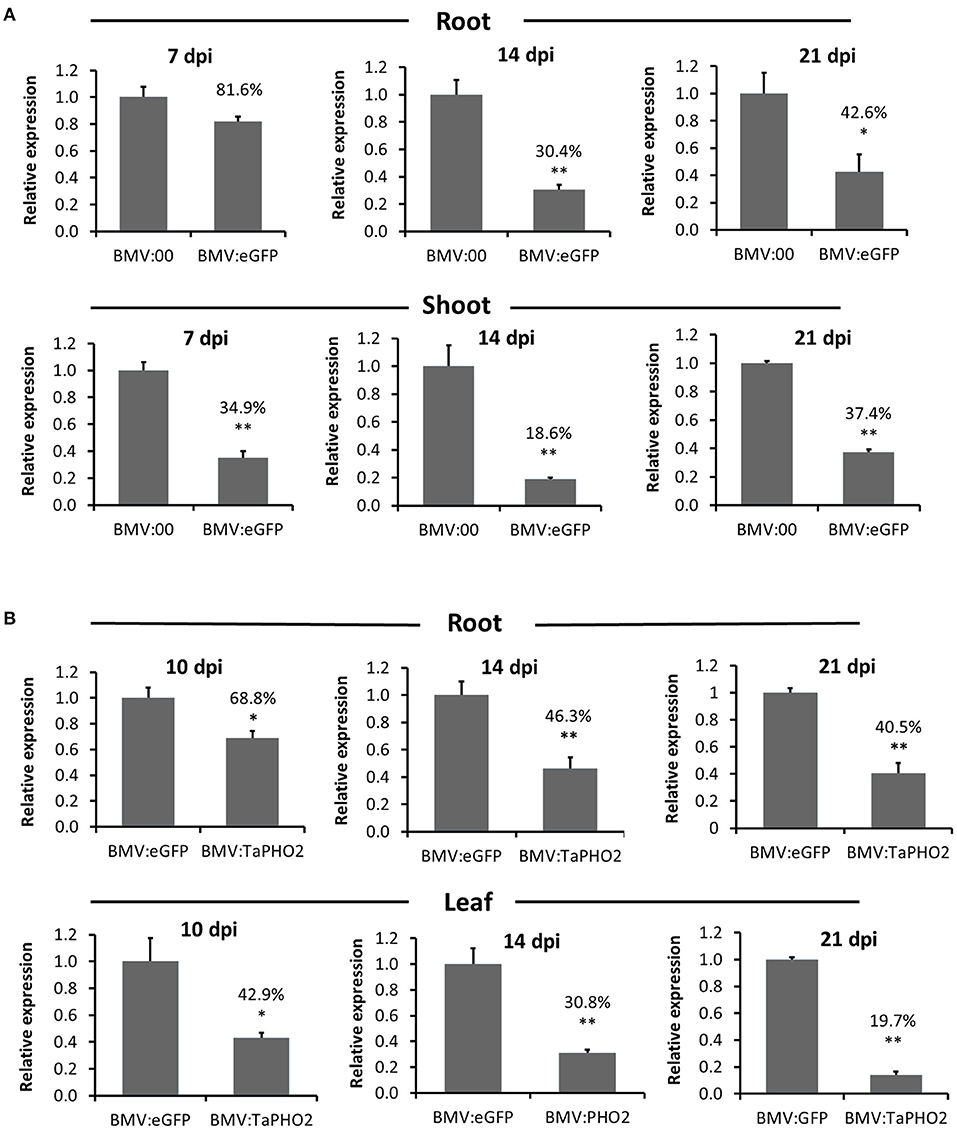
Figure 5. Comparison over time of BMVCP5-mediated silencing of eGFP and TaPHO2 in wheat root and aerial tissues. (A) Relative expression levels of eGFP mRNA in roots and shoots from transgenic OsRCg2GFP wheat (cv. Fielder) plants infected with BMV:eGFP (180-nt insert) or BMV:00 (no insert control) at 7, 14, and 21 dpi. Percentage values are relative to the BMV:00 control. Values represent means + SE of four to seven biological replicates, and treatment values compared (t-test: *P < 0.05; **P < 0.01). (B) Relative expression levels of TaPHO2 mRNA in roots and leaves from wheat plants (cv. Overley) inoculated with BMV:TaPHO2 (114-nt insert) or BMV:eGFP (107-nt insert) at 10, 14, and 21 dpi. Target gene mRNA levels were analyzed by RT-qPCR, and values normalized against relative TaEF1α mRNA levels. Percentage values are relative to the BMV:eGFP (107-nt insert) control. Values represent means + SE from three to six biological replicates, and treatment values compared (t test; *P < 0.05; **P < 0.01). Similar results were obtained in two independent experiments.
To determine whether the decreased silencing in roots also was correlated with more rapid loss of the inserts in the BMVCP5 vector in this tissue vs. the aerial tissue, insert stability was analyzed for the samples described in the previous paragraphs. The 180-nt eGFP insert showed similar stability in shoots and roots at each of the three time points (Supplementary Figure 6A). The 114-nt TaPHO2 insert was more stable in leaf samples than in the root samples at each time point (Supplementary Figure 6B). In a separate experiment analyzing silencing of TaPDS after inoculation with BMV:TaPDS100, TaPDS mRNA expression was silenced to a higher degree in the 5th systemic leaf than in the root at 28 dpi, 86.5% reduction in leaves vs. 56.3% reduction in roots (Supplementary Figure 7A). However, as for eGFP silencing, the 100-nt TaPDS insert showed similar stability in the 5th systemic leaf and roots (Supplementary Figure 7B). Therefore, the decreased level of transcript silencing in the root by BMVCP5 may be due to other factors than just the stability of the insert in the virus vector in that tissue.
BMVCP5-Mediated TaPHO2 Silencing Led to Increased Phosphate Accumulation in Leaves
To determine whether BMV VIGS resulted in a modified biochemical phenotype expected due to down-regulated expression of a target gene, the effect of BMVCP5-mediated TaPHO2 silencing on Pi accumulation in wheat plants was analyzed. In wheat plants infected with BMV:TaPHO2114, TaPHO2 transcripts were decreased to ~23% in the 4th systemic leaf and 32% in roots at 25 dpi compared with similar tissue infected with BMV:eGFP107 (Figure 6A). Pi concentrations in the 2nd, 3rd, and 4th systemic leaves from plants with decreased TaPHO2 mRNA expression were over twice the level of Pi in tissues infected with BMV:eGFP107 (Figure 6B). However, in roots of the TaPHO2-silenced plants Pi concentration was reduced by 22% compared with the BMV:eGFP107 control (Figure 6B). The TaPHO2-silenced plants also had a lower shoot biomass but similar root biomass (Figure 6C), which led to an increased root/shoot ratio in comparison to the control (Figure 6C).
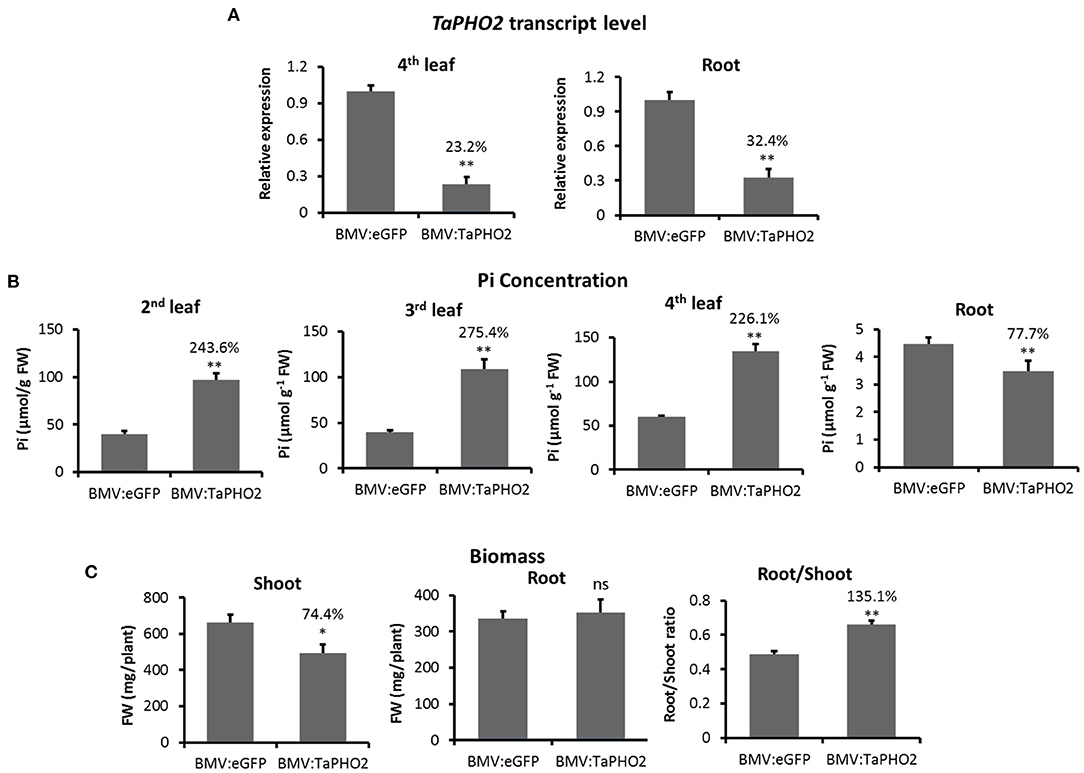
Figure 6. Inorganic phosphate concentration and biomass of wheat plants silenced for TaPHO2 expression with BMV:TaPHO2114 infection. Wheat (cv. Overley) plants inoculated with BMV:TaPHO2 (114-nt insert) or BMV:eGFP (107-nt insert; control) were grown under a low phosphate condition (20 μM Pi). Samples were collected at 24 dpi. (A) TaPHO2 expression levels in the 4th systemic leaf or roots, determined by RT-qPCR with values across treatments normalized against relative TaEF1α mRNA levels. (B) Pi concentrations in the 2nd, 3rd, or 4th systemic leaves or roots of plants inoculated with BMV:TaPHO2 or BMV:eGFP. (C) Shoot biomass, root biomass and Root/Shoot ratio of plants inoculated with viruses. Percentage values are relative to the BMV:eGFP control. Values represent means + SE of five biological replicates and treatment values compared (t-test; *P < 0.05; **P < 0.01). Similar results were obtained in two independent experiments. ns, not significant.
BMVCP5 as a Potential VIGS Tool in Various Wheat Cultivars
In order to determine the range of wheat varieties to which the BMV VIGS system could be applied, a total of 54 wheat cultivars were screened for susceptibility to virus infection by BMV:GFPuv, a vector containing a fragment from a variant GFP (Ding et al., 2018), using the leaf blade inoculation method. All wheat cultivars were susceptible to BMV:GFPuv infection, displaying diverse disease symptoms in systemically-infected leaves. The disease symptoms from the 54 wheat cultivars were divided into three categories according to the severity: (1) mild (mild light green mottling or streaking; 10 cultivars), (2) moderate (mild or moderate yellow mosaic; 17 cultivars), and (3) severe (yellowing or severe yellow mosaic; 27 cultivars). Plant height reduction was related to the severity of virus symptoms (Supplementary Table 1).
Next, the effect of BMVCP5-induced TaPDS silencing was analyzed in eight wheat cultivars, including four with mild disease symptoms (Overley, OK09520, Pete, and RonL), three with moderate disease symptoms (Bentley, NF97117, and Robidoux) and one with severe disease symptoms (Big Sky). Seventeen to twenty plants from each cultivar were infected with BMV:eGFP107 or BMV:TaPDS100 by the leaf inoculation method. In the eight cultivars, infection frequencies ranged from 95 to 100% for BMV:eGFP107 and 74 to 100% for BMV:TaPDS100. Streaking or mottling symptoms of varying degrees were observed in all cultivars inoculated with BMV:eGFP107. The frequency of visible VIGS phenotype induced by BMV:TaPDS100 infection, characterized by bleaching in infected leaves (Figure 7A), varied from 74 to 100% among cultivars (Table 2). Bleaching usually developed from the 2nd to 5th systemic leaves in the eight cultivars, with the strongest bleaching occurring on the 3rd and 4th leaves.
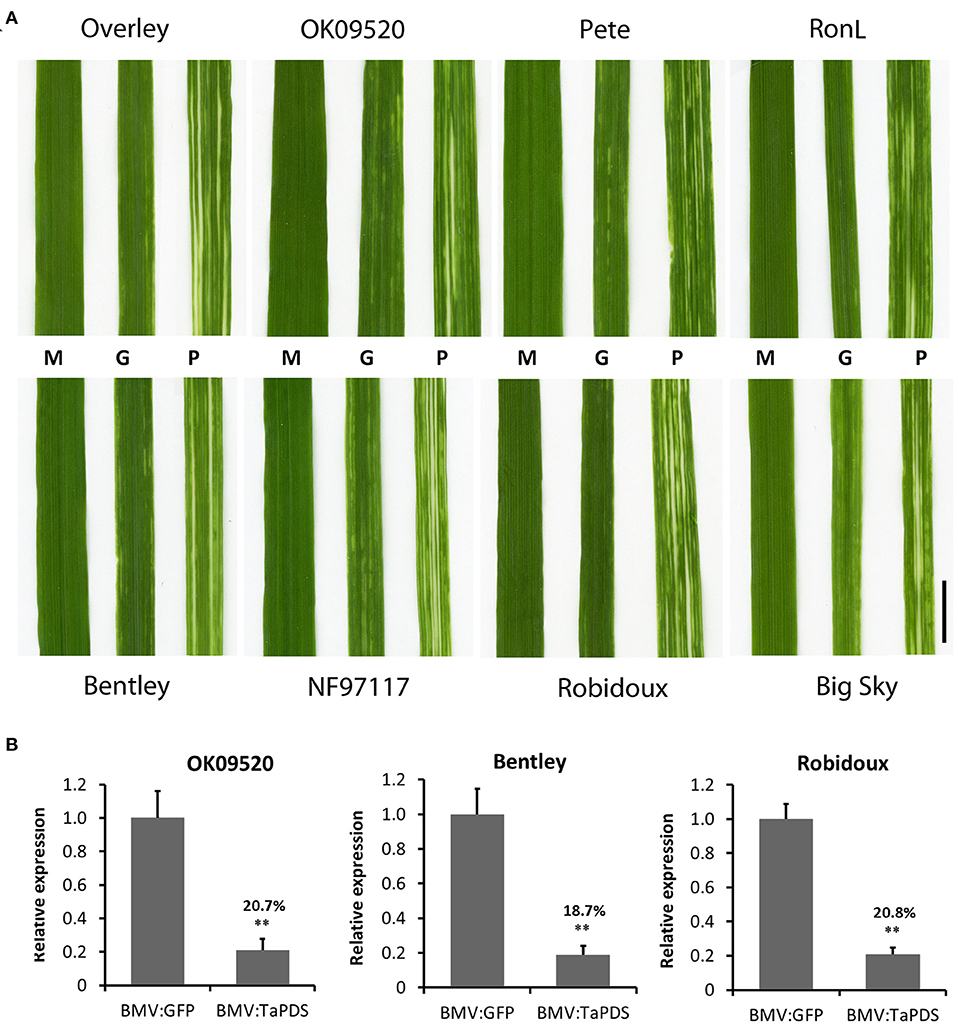
Figure 7. BMVCP5-induced TaPDS silencing in eight winter wheat cultivars. (A) Images show a representative phenotype of the 3rd systemic leaf at 22 dpi from plants infected with mock (M), BMV:eGFP107 (G) or BMV:TaPDS100 (P). Bar = 1 cm. (B) TaPDS mRNA expression levels in the 3rd systemic leaf at 22 dpi from plants infected with BMV:eGFP (107-nt insert) or BMV:TaPDS (100–nt insert) in cultivars OK09520, Bentley and Robidoux, analyzed by RT-qPCR with values across treatments normalized against the transcript level of TaEF1α. Expression percentages are given relative to the BMV:eGFP control. Values represent means + SD of five biological replicates, and treatments compared (t-test; **P < 0.01). Similar results were obtained in two independent experiments.
TaPDS mRNA silencing was verified in the 3rd systemic leaves of OK09520, Bentley and Rubidoux. An ~80% decrease in TaPDS transcript levels was detected in leaf extracts from all the three cultivars compared with the BMV:eGFP controls (Figure 7B). These results demonstrate the efficacy of BMVCP5-mediated VIGS in various wheat genotypes.
Discussion
Factors Affecting BMVCP5-Mediated VIGS
VIGS studies have found that insert stability in a VIGS vector is positively correlated with the ability of the VIGS system to silence expression of the target gene (Ramanna et al., 2013). Therefore, improving the stability of foreign inserts in the virus vector during infection appears to be critically important for a VIGS system. Using our silencing protocol for wheat, the BMVCP5 silencing vector was amplified in an intermediate host, N. benthamiana, and then extract from the inoculated leaves was inoculated to wheat. Therefore, insert stability in both hosts was important to obtain maximum silencing in wheat. For the three target genes there was no loss of insert at 180 nt or less from the BMVCP5 vector in N. benthamiana extract at 4 days post infiltration (Supplementary Figure 2). Full insert stability was observed previously with this vector expressing a maize 250-nt PDS gene fragment in N. benthamiana at 3 days post infiltration, with minor loss at 6 days post infiltration (Ding et al., 2018). We previously recommended analyzing insert stability in N. benthamiana extracts to select extract with the most intact insert for inoculation to the monocotyledonous host (e.g., Zhu et al., 2014; Ding et al., 2018). Our current findings suggest that when using inserts of optimal length (~100 nt) it is not necessary to check insert stability in the N. benthamiana inoculum. This will shorten the time and lessen the associated costs when using BMV-mediated VIGS in all monocotyledonous species where N. benthamiana is an intermediate host.
In wheat leaves, small inserts around 100 nt were much more stable than larger inserts (150–252 nt) and resulted in consistent and substantial silencing of the target gene mRNA (Figure 3, Supplementary Figure 4); loss of the larger inserts was correlated with higher target gene mRNA expression. A negative correlation between insert length and insert stability and/or silencing efficiency also was reported for other VIGS systems, such as Potato virus X-VIGS in N. benthamiana (Avesani et al., 2007), BSMV-VIGS in barley (Bruun-Rasmussen et al., 2007) or N. benthamiana (Yuan et al., 2011) and CWMV-VIGS in wheat (Yang et al., 2018). In maize, stability of 250-nt inserts (only size studied) in BMVCP5 was significantly improved over that in the unimproved BMVF13m vector, but partial loss of the full length inserts of ZmPDS or ZmHSP70-1 was apparent even at 5 dpi in inoculated leaves and 5 dpi (PDS) or 10 dpi (HSP70-1) in systemically-infected leaves (Ding et al., 2018). Additionally, using the original in vitro transcription-based vector, C-BMVA/G, it was reported that an 86-nt PDS insert induced extensive light-yellow and white streaks in systemic leaves of maize and rice and significant silencing of the target transcript (Ding et al., 2006), suggesting stability for this small insert in the unimproved vector. These studies indicate that an insert of ~100 nt is the optimal size for the BMVCP5 vector in wheat and likely for other host species.
Interestingly, infection with BVMCP5 containing an even smaller insert, 52-nt TaPDS, triggered no bleaching phenotype (Supplementary Figure 5), but knocked down target mRNA by 68%, a decrease comparable to that induced by larger inserts (compare Figure 3D with Supplementary Figure 5B). Similar findings were reported in a BSMV-VIGS study in barley, where a 128-nt PDS fragment caused a significant reduction in PDS mRNA levels but no bleaching (Bruun-Rasmussen et al., 2007). The cause of disconnect between target mRNA downregulation and visible symptoms for these smaller inserts is not understood. Bruun-Rasmussen et al. (2007) speculated that to trigger a visible bleaching phenotype PDS mRNA levels should be lower than a specific threshold at a certain point in leaf development. Additionally, it is possible that mRNA silencing may be present for a longer period of time, but at a consistently lower level, in more leaf cells from plants infected with BMV:TaPDS52 than with BMVCP5 expressing larger TaPDS inserts. The instability of larger TaPDS inserts would lead to little or no silencing in more leaf cells than during BMV:PDS52 infection and therefore an artificial similarity in measured silencing degrees per whole leaf between BMV:PDS52 and BMV with larger TaPDS inserts. More precise research analyzing different portions of leaf showing patches of visible silencing for presence of insert and level of silencing is necessary to clarify whether both, one or neither interpretation has merit.
Besides insert size, insert sequence may also affect insert stability. In our study, the 180-nt eGFP insert was more stable than the 150-nt TaPDS or TaPHO2 insert (Figure 2). Ding et al. (2006) found a 398-nt insert from an actin gene was more stable than a 240-nt insert from a PDS gene in maize with the BMV vector. In a BSMV-VIGS study in barley roots, Pacak et al. (2010b) reported similar findings: a 251-nt insert from the barley IPS1 gene and a 387-nt insert from the barley PHO2 gene were much more stable than a 250-nt GFP insert. Studies showed that both the viral genome length and structure have been optimized during evolution to enhance the formation of stable viral particles (Perlmutter et al., 2013). For VIGS studies, increases in insert length or insertion of sequence that affects the secondary and tertiary structure of the viral RNA may lead to poor encapsidation, selective encapsidation (insert loss) and native virus spreading. Bruun-Rasmussen et al. (2007) observed that BSMV RNA that had lost insert accumulated to higher levels than BSMV RNA with insert. Additional to encapsidation, the ability of the specific genomic viral RNA with insert and its encoded proteins to accumulate and the ability of the genomic viral RNA with insert to recombine should be considered in future research to fully understand selection exerted by insert sequence or size.
It was interesting that the three TaPHO2 homoeologs were silenced to different degrees by BMVCP5 containing the 114-nt TaPHO2 fragment (Figure 2F), which possessed 100% identity with each homoeolog in the targeted region (Supplementary Figure 1B). This result suggests that the target mRNA secondary structure may affect its interaction with the silencing machinery and therefore its subsequent silencing. Evidence from siRNA-based studies showing target mRNA structure affects siRNA silencing efficiency supports this hypothesis (Shao et al., 2007; Gredell et al., 2008). VIGS is a technology based on the plant siRNA-mediated antiviral defense mechanism, therefore factors affecting siRNA silencing efficiency may also influence VIGS silencing efficiency. Selectively silencing particular homoeologs during VIGS through structure-based differential target gene susceptibility is worthy of further study. The siRNA-Finder (si-Fi) software, si-Fi21, offers effective long RNA interference sequence design (Lück et al., 2019). Future VIGS study may consider using this software for selection of insert sequences.
Many VIGS studies report that inoculation methods affect the success of virus vector delivery and the silencing efficiency. Broderick and Jones (2014) compared four inoculation methods when optimizing the tobacco rattle virus-based VIGS protocol in petunia (Petunia × hybrida), and found inoculation of mechanically wounded shoot apical meristems induced the most effective and consistent silencing. Besides the inoculation method, the plant developmental stage may also affect silencing. A TRV-VIGS study in Antirrhinum showed that inoculation of plants at first- and second-leaf stages were more effective at inducing silencing than at fifth-leaf stage (Tan et al., 2020). Several groups reported successful delivery of BMV, BSMV and a cucumber mosaic virus-based VIGS vector in maize by vascular puncture inoculation of maize seeds (Benavente et al., 2012; Wang et al., 2016; Jarugula et al., 2018). However, direct inoculation of wheat seeds via the vascular puncture method was difficult and the survival rate of the inoculated seeds was low due to the small size of the wheat embryo (Y Wang and RS Nelson, unpublished data). The coleoptile inoculation method developed in this study gave a similar inoculation efficiency and silencing efficiency to that of leaf blade inoculation but allowed inoculation at an earlier seedling developmental stage (3 days post germination). Other inoculation methods, such as seed imbibition or biolistic inoculation (Cheuk and Houde, 2017; Mei et al., 2019), should be investigated for use with the BMVCP5 vector.
We found BMVCP5-VIGS efficiency varied in tissues. Compared with leaf or shoot tissue, BMVCP5 exhibited a relatively lower silencing ability in roots, as revealed by parallel analysis of aerial and root tissue from plants silenced for TaPDS, TaPHO2 or ectopic eGFP mRNA expression (Figure 5, Supplementary Figure 7). For the BSMV vector, Pacak et al. (2010b) reported that instability of inserts may be a more severe problem for silencing of genes in roots compared to leaves and advised screening virus constructs for stability. In our studies a difference in insert stability did not correlate with the generally less efficient silencing in root vs. aerial tissue for these inserts (Figure 5, Supplementary Figures 6, 7). It is possible that the lower silencing ability of BMVCP5 in roots may be associated with differences between root and aerial tissue (e.g., their subcellular constitution) that inhibits accumulation in roots.
BMVCP5 Is a Valuable Tool for Functional Genomics Studies in Wheat
We demonstrate that BMVCP5 can be used for rapid gene function characterization in young wheat seedlings. Over 50% reduction of the target mRNA was detected in shoots, leaves and roots within 2–4 weeks after inoculation (Figures 2, 3, 5, Supplementary Figures 3, 7), which is good for various phenotyping experiments. By skipping the insert stability analysis in N. benthamiana, this new BMVCP5 VIGS procedure is easier to use and more time- and cost-efficient. In addition, using a small insert (~100 nt) for silencing in BMVCP5 is particularly useful for gene function characterization in hexaploid wheat because it is relatively easy to find a short fragment with high specificity targeting a single or multiple genes.
Many root genes, especially those responsive to abiotic stresses or associated with nutrient use efficiency, influence the sustainability of plant production under adverse conditions. Characterization of root genes contributing to, and breeding for adapted root system architecture with improved nutrient and water acquisition or use efficiency will address challenges for modern agriculture such as increasing food security and decreasing environmental impact (Comas et al., 2013; Lynch, 2019). Few studies have analyzed VIGS activity in and its effect on roots of monocotyledonous species (Pacak et al., 2010b; Bennypaul et al., 2012; He et al., 2015). In our study, BMVCP5-induced TaPHO2 silencing led to Pi accumulation in wheat leaves (Figure 6B), consistent with previous findings using PHO2 mutants in Arabidopsis (Aung et al., 2006; Bari et al., 2006), or wheat (Ouyang et al., 2016), and BSMV VIGS of HvPHO2 in barley (Pacak et al., 2010b). As in our study (Figure 6B), the latter two studies reported decreased root Pi concentrations when knocking out any TaPHO2 homoeolog in wheat or HvPHO2 in barley. These results indicate that silencing TaPHO2 expression via BMVCP5 functionally mimicked findings with stable mutants of PHO2 in various plant species, underlining the value of BMVCP5 for functional genomic studies in wheat.
Genetic resources and the genetic diversity embodied by those resources are fundamental for sustaining wheat production and critical for enhancing yield potential (Tadesse et al., 2016). Intensive efforts have been made to collect and conserve wheat genetic resources and attain genome-wide genetic variation (Jordan et al., 2015; Tadesse et al., 2016; Liu et al., 2017; Zhou et al., 2017). To take advantage of the wide range of genetic diversity from these abundant genetic resources, gene functional characterization in diverse breeding lines or cultivars is indispensable. Of the few functional genomics tools in wheat, stable transformation and mutant collections are limited to only a few genotypes (Chen et al., 2012; Dhaliwal et al., 2015; Fitzgerald et al., 2015; Guo et al., 2017; Hayta et al., 2019; Wang et al., 2020), while VIGS allows for rapid gene function characterization in many wheat cultivars or breeding lines, as shown by many BSMV VIGS studies (referenced in Dommes et al., 2019). In this study, we demonstrated BMVCP5 had a high infection capacity in wheat (100% infection rate of the 54 wheat cultivars), and half of the screened cultivars exhibited mild or moderate disease symptoms. Moreover, BMVCP5 showed a high success rate of VIGS (100% of plants silenced for TaPDS expression displayed a visual bleaching phenotype in all eight cultivars examined (Figure 7A). These results demonstrate the great potential for application of BMVCP5-mediated VIGS in various wheat genotypes. This could be particular useful in studying cultivar specific traits, such as validation of top candidate genes from genome-wide association studies.
Although all VIGS vectors share some common features such as rapid and capable targeting of single or multiple genes, each VIGS system has unique requirements for its best use. These include optimizing insert length, identifying tissue and cultivar susceptibility, optimizing plant growth conditions, and fulfilling biosafety regulations. For BSMV-based VIGS, insert sizes from 250 to 400 bp were more effective over time than one over 500 bp in silencing MAGNESIUM CHELATASE (TaChlH) expression in wheat (Yuan et al., 2011). CWMV containing a PDS fragment of 500 bp provided better silencing than those of 800, 1,000, or 1,500 bp (Yang et al., 2018). Using inverted-repeat fragments of 60 nucleotides from PDS or CLOROPLASTOS ALTERASDOS1 (CLA1), FoMV efficiently down-regulated target gene expression in wheat (Liu et al., 2016). Shorter sequences make it easier to avoid off-target silencing. BSMV and BMV vectors silence in multiple tissues of wheat (Figures 2–6; Bennypaul et al., 2012), while the CWMV- and FoMV-VIGS have only been studied in leaves (Liu et al., 2016; Yang et al., 2018). BMV and BSMV function in multiple wheat cultivars (Figure 7; Dommes et al., 2019); while CWMV- and FoMV-VIGS have been investigated in one wheat cultivar (Liu et al., 2016; Yang et al., 2018). CWMV-VIGS in wheat functions well at 17°C (Yang et al., 2018), while BSMV showed good silencing at 18–22°C in wheat (Cakir and Tör, 2010; Bennypaul et al., 2012). BMV- and FoMV-based silencing studies in wheat were conducted at 22/19°C (day/night) and 22–24°C, respectively (this study; Liu et al., 2016). Regarding safety and security issues, BMV is poorly seed transmitted, while BSMV could be transmitted through seed (Mise and Pocsai, 2004; Bruun-Rasmussen et al., 2007). Due to the potential impact of these viruses on agriculture, VIGS vectors are strictly controlled by government safety regulations. BMVCP5-VIGS system provides an additional choice for wheat researchers who (1) are limited by local government restrictions from using other vectors, (2) are concerned about seed transmission, or (3) have experience with BMV and prefer to use it in their functional genomics studies. The addition of BMVCP5 to the list of VIGS tools will further accelerate functional genomics studies and productive breeding in this species.
Materials and Methods
Plant Materials and Growth Conditions
All 54 wheat cultivars used in this study are hexaploid. Seeds of 48 winter wheat cultivars were obtained from the Small Grains Laboratory at Noble Research Institute, LLC (Ardmore, USA), and seeds of six cultivars (Eltan, Kavkaz, Apogee, WinTex, Chancellor, Golden Chief) were requested from USDA (Supplementary Table 1). Cultivar Overley was used for cloning TaPDS and TaPHO2 fragments and silencing experiments targeting TaPDS and TaPHO2 expression. Homozygous T3 lines of transgenic OsRCg2GFP wheat (background: spring wheat cv. Fielder) were generated from the T2 lines (Xue et al., 2016), and used in silencing experiments targeting eGFP expression. N. benthamiana and wheat plants were grown in the Metro-Mix® 360 medium (Sun Gro Horticulture, USA) in a greenhouse under 16 h light (300–500 μmol m−2 s−1) at 22°C and 8 h dark at 19°C with 60% relative humidity. For silencing studies targeting TaPHO2 expression, wheat plants were grown in a mixture of turface:sand:perlite (2:2:1) under low phosphate treatment (20 μM KH2PO4). For silencing studies targeting eGFP, a hydroponic culture system with a half Hoagland nutrient solution (Hoagland and Arnon, 1950) was used under the same greenhouse conditions.
Construction of BMV VIGS Vectors
PCR primers for cloning of TaPHO2 and TaPDS fragments from wheat (cv. Overley) were designed at the conserved regions of the three homoeologs for each gene. The 52-, 100-, 150-, 200-, and 250-bp fragments of TaPDS (GenBank accession: FJ517553.1) were amplified by RT-PCR using primer pairs of P1-F4 /P1-R2, P1-F4/P1-R1, P1-F3/P1-R1, P1-F2/P1-R1, and P1-F1/P1-R1, respectively. The 114-, 150-, 204-, and 252-bp fragments of TaPHO2 (GenBank accession: AK331438.1) were amplified using primer pairs of P2-F1/P2-R4, P2-F1/P2-R3, P2-F1/P2-R2, and P2-F1/P2-R1, respectively. Similarly, The 107-, 180-, and 220-bp eGFP (GenBank accession: U55761.1) fragments were amplified from the T3 transgenic OsRCg2GFP wheat seedlings using primer pairs P3-F3/P3-R1, P3-F2/P3-R1, and P3-F1/P3-R1, respectively. All forward primers and reverse primers contained, respectively, an AvrII (CCTAGG) or NcoI (CCATGG) restriction enzyme site. The resulting PCR fragments were cloned into the AvrII and NcoI sites of the pC13/F3CP5, encoding RNA3 of the BMVCP5 vector. The pC13/F3CP5 plasmid with pC13/F1+2, encoding RNAs 1 and 2 of BMV, composed the BMVCP5 vector and were used in the VIGS experiments. BMV:GFPuv, a BMVCP5 vector with a 250-bp fragment from a variant of the GFP gene (Ding et al., 2018), was used to screen susceptibility of wheat cultivars for BMV infection. Primers used for construction of BMVCP5 VIGS vectors in this study are listed in Supplementary Table 2.
Total RNA Extraction, RT-PCR, and Real-Time PCR
Plant total RNA was extracted using TRIzol Reagent (Life Technologies, USA) and treated using the TURBO DNA-free™ Kit (Invitrogen, USA) to eliminate genomic DNA contamination before reverse transcription. First-strand cDNA was synthesized from 0.5 μg total RNA by M-MLV reverse transcriptase (ThermoFisher Scientific, USA) using a mixture of oligo (dT18) and random primers. Cloning of BMV-RNA3 insert fragments and analysis of BMV insert stability were performed by PCR using Phusion® High-Fidelity DNA Polymerase (NEB, USA) and Taq DNA Polymerase (NEB, USA), respectively, following the manufacturer's instructions. Primer pair P4-F/P4-R flanking the foreign gene insert site in pC13/F3CP5 was used to determine insert stability in both N. benthamiana and wheat through RT-PCR analysis. Gene expression analyses were evaluated by real-time PCR (qPCR) using gene-specific primers (not amplifying the insert) and the Power SYBR Green PCR Master Mix kit (Applied Biosystems, USA) on an ABI PRISM 7900 HT Sequence Detection System (Applied Biosystems, USA). The wheat translation elongation factor subunit EF1α was used as an internal control for gene expression analyses (Paolacci et al., 2009). All primers used for RT-PCR or qPCR in this study were listed in Supplementary Table 3.
Agroinfiltration of N. benthamiana and Viral Inoculation of Wheat
BMV VIGS vectors were transformed into the Agrobacterium tumefaciens strain C58C1 by using the freeze-thaw transformation method (Chen et al., 1994). Agrobacterium was grown in YEP liquid medium containing rifampicin (10 mg/L) and kanamycin (50 mg/L) at 28°C to OD600 = 1. Agrobacterium cultures containing pC13/F1+2 and pC13/F3CP5 with foreign gene inserts were pelleted, re-suspended in infiltration buffer (10 mM MgCl2, 10 mM MES, 0.2 mM acetosyringone, pH 5.5) at equal amounts to a final OD600 = 2, and infiltrated into the fully expanded leaves of 3-week old N. benthamiana plants. Infiltrated leaves were harvested at 4 days post infiltration, frozen in liquid nitrogen, and stored at −80°C until use. Sap inoculums were prepared by grinding 1 g of infected N. benthamiana leaf in 1 ml of 0.1 M potassium phosphate buffer (pH 7) containing 0.15 g carborundum, and then rub-inoculated onto the abaxial surface of the 1st or the 1st and 2nd leaf blade(s) of 7–9 day-old wheat seedlings. Plants after infiltration or inoculation were covered with a clear plastic dome overnight at room temperature, and then grown under greenhouse conditions as mentioned above.
For rub-inoculation of coleoptile, wheat seeds were pre-soaked with sterile water for 3 h, then incubated on wet germination paper at room temperature in the dark for 3 days. Coleoptiles were inoculated in a petri dish plate in the following manner. N. benthamiana leaf sap inoculum (0.5 ml) was pipetted to the center of the plate. Then a coleoptile (~2.5 mm in length) was lain flat in the leaf sap and a layer of carborundum dusted on it. Holding the crown region with the thumb and pointer fingers from one hand, the coleoptile was rubbed back and forth parallel to its veins and pressing against the plate several times with the pointer finger from the other hand. The coleoptile was then flipped over and the rubbing step repeated. After inoculation, the wheat seedlings were placed onto the wet germination paper in a new plate, covered with the lid of the petri dish plate and cultured at room temperature overnight before growing them in solid media or hydroponic culture system under greenhouse conditions as mentioned above.
All VIGS experiments were repeated two to three times with 9–19 plants inoculated in each experiment. For insert stability and expression analyses, usually five plants were sampled. Detailed sampling information for each experiments are described in the figure legends.
Tissue-Printing Assay of BMV Viral Accumulation in Wheat Roots
An antibody-based tissue-blotting assay (Nelson et al., 1993; Ding et al., 2018) was used to analyze BMVCP5 accumulation in wheat roots. Roots of transgenic OsRCg2GFP wheat plants inoculated with BMV:GFP180 or no virus (mock-inoculated control), were harvested at 21 dpi. Root prints were made on nitrocellulose membranes (Bio-Rad, USA), and BMV accumulation on membranes was visualized by blotting against a BMV CP-specific antibody as previously described (Ding et al., 1999). Images of GFP fluorescence and BMV accumulation in wheat roots were captured with an Olympus SZX 12 microscope. GFP fluorescence was quantified using the ImageJ software (Schneider et al., 2012).
Phosphate Concentration Measurement
Pi concentrations were determined using a colorimetric micromethod with modifications (Itaya and Ui, 1966; Pant et al., 2008). Whole root and single leaf tissues from BMVCP5-infected wheat plants at 24 dpi were harvested and homogenized with liquid nitrogen. Sterile deionized water was added to the tissue powder in a ratio of 10 μl water per mg fresh weight. Supernatants containing extracted soluble Pi were collected by centrifuging at 10,000 g for 10 min, and diluted with deionized sterile water, 10 times for root samples and 50 times for leaf samples. The diluted supernatant (10 μl) was mixed with 1 N HCl (100 μl) and a malachite green solution (100 μl; one volume 4.2% (w/v) (NH4)6Mo7O24·H2O in 5 N HCL and three volumes of 0.2% malachite green dye in water), and incubated at room temperature for 10 min before measuring the absorbance at 630 nm using a TECAN Infinite M200 PRO instrument.
Statistics
Student's t-test or one way ANOVA followed by LSD tests were conducted in Microsoft Excel to determine the significant differences between treatment means.
Data Availability Statement
The original contributions presented in the study are included in the article/Supplementary Material, further inquiries can be directed to the corresponding author/s.
Author Contributions
RSN, MCS, and W-RS conceived the project. YW and RSN designed the experiments. CC performed real-time PCR analysis. BK screened wheat cultivars for sensitivity to BMV infection and was involved in planning the eGFP VIGS experiment. W-RS helped plan and review the TaPHO2 studies and streamlined the high-throughput Pi assay. YK did the statistical analysis. YW did all other experiments, analyzed data, and drafted the manuscript. RSN, YK, W-RS, MKU, MCS, and CC critically revised the manuscript. All authors read and approved the manuscript.
Funding
This work was funded by the Forage 365 Project from Noble Research Institute, LLC.
Conflict of Interest
The authors declare that the research was conducted in the absence of any commercial or financial relationships that could be construed as a potential conflict of interest.
Acknowledgments
We thank Dr. Xuefeng Ma and Josh Anderson (Noble Research Institute, LLC) for providing seeds of winter wheat cultivars, Dr. Gangping Xue (CSIRO, Brisbane, Australia) for providing seeds of the OsRCg2GFP transgenic wheat. We thank Mrs. Lynne Jacobs (Noble Research Institute, LLC) for taking care of the plants in greenhouse, Kim Cooper for technical assistance, Dr. Raul Huertas, Dr. Quina Nogales Diaz and Mrs. Sylvia Warner (Noble Research Institute, LLC) for help with Pi measurement.
Supplementary Material
The Supplementary Material for this article can be found online at: https://www.frontiersin.org/articles/10.3389/fpls.2021.685187/full#supplementary-material
References
Adamski, N. M., Borrill, P., Brinton, J., Harrington, S. A., Marchal, C., Bentley, A. R., et al. (2020). A roadmap for gene functional characterisation in crops with large genomes: lessons from polyploid wheat. elife 9:e55646. doi: 10.7554/eLife.55646
Aung, K., Lin, S. I., Wu, C. C., Huang, Y. T., Su, C. L., and Chiou, T. J. (2006). pho2, a phosphate overaccumulator, is caused by a nonsense mutation in a microRNA399 target gene. Plant Physiol. 141, 1000–1011. doi: 10.1104/pp.106.078063
Avesani, L., Marconi, G., Morandini, F., Albertini, E., Bruschetta, M., Bortesi, L., et al. (2007). Stability of Potato virus X expression vectors is related to insert size: implications for replication models and risk assessment. Transgenic Res. 16, 587–597. doi: 10.1007/s11248-006-9051-1
Babu, P., Baranwal, D. K., Harikrishna Pal, D., Bharti, H., Joshi, P., et al. (2020). Application of genomics tools in wheat breeding to attain durable rust resistance. Front. Plant Sci. 11:567147. doi: 10.3389/fpls.2020.567147
Bari, R., Pant, B. D., Stitt, M., and Scheible, W. R. (2006). PHO2, microRNA399, and PHR1 define a phosphate-signaling pathway in plants. Plant Physiol. 141, 988–999. doi: 10.1104/pp.106.079707
Baulcombe, D. C. (1999). Fast forward genetics based on virus-induced gene silencing. Curr. Opin. Plant Biol. 2, 109–113. doi: 10.1016/S1369-5266(99)80022-3
Bekele, D., Tesfaye, K., and Fikre, A. (2019). Applications of virus induced gene silencing (VIGS) in plant functional genomics studies. J. Plant Biochem. Physiol. 7:1. doi: 10.4172/2329-9029.1000229
Benavente, L. M., Ding, X. S., Redinbaugh, M. G., Nelson, R. S., and Balint-Kurti, P. (2012). Virus-induced gene silencing in diverse maize lines using the Brome mosaic virus-based silencing vector. Maydica 57, 206–214. Available at: https://journals-crea.4science.it/index.php/maydica/article/view/865/746.
Bennypaul, H. S., Mutti, J. S., Rustgi, S., Kumar, N., Okubara, P. A., and Gill, K. S. (2012). Virus-induced gene silencing (VIGS) of genes in root, leaf, and meiotic tissues of wheat. Funct. Integr. Genomics 12, 143–156. doi: 10.1007/s10142-011-0245-0
Broderick, S. R., and Jones, M. L. (2014). An optimized protocol to increase virus-induced gene silencing efficiency and minimize viral symptoms in petunia. Plant Mol. Biol. Rep. 32, 219–233. doi: 10.1007/s11105-013-0647-3
Bruun-Rasmussen, M., Madsen, C. T., Jessing, S., and Albrechtsen, M. (2007). Stability of Barley stripe mosaic virus-induced gene silencing in barley. Mol. Plant Microbe Interact. 20, 1323–1331. doi: 10.1094/MPMI-20-11-1323
Cakir, C., and Tör, M. (2010). Factors influencing Barley stripe mosaic virus-mediated gene silencing in wheat. Physiol. Mol. Plant Path. 74, 246–253. doi: 10.1016/j.pmpp.2010.04.001
Chen, H., Nelson, R. S., and Sherwood, J. L. (1994). Enhanced recovery of transformants of Agrobacterium tumefaciens after freeze-thaw transformation and drug selection. BioTechniques 16, 664–668.
Chen, L., Huang, L., Min, D., Phillips, A., Wang, S., Madgwick, P. J., et al. (2012). Development and characterization of a new TILLING population of common bread wheat (Triticum aestivum L.). PLoS ONE 7:e41570. doi: 10.1371/journal.pone.0041570
Chen, L., and Liao, H. (2017). Engineering crop nutrient efficiency for sustainable agriculture. J. Integr. Plant Biol. 59, 710–735. doi: 10.1111/jipb.12559
Cheuk, A., and Houde, M. (2017). A rapid and efficient method for uniform gene expression using the barley stripe mosaic virus. Plant Methods 13:24. doi: 10.1186/s13007-017-0175-5
Comas, L. H., Becker, S. R., Cruz, V. M., Byrne, P. F., and Dierig, D. A. (2013). Root traits contributing to plant productivity under drought. Front. Plant Sci. 4:442. doi: 10.3389/fpls.2013.00442
Csorba, T., Pantaleo, V., and Burgyan, J. (2009). “RNA silencing: an antiviral mechanism,” in Advances in Virus Research, eds G. Loebenstein and J. P. Carr (San Diego, CA: Elsevier Academic Press), 35–71. doi: 10.1016/S0065-3527(09)07502-2
Dhaliwal, A. K., Mohan, A., Sidhu, G., Maqbool, R., and Gill, K. S. (2015). An ethylmethane sulfonate mutant resource in pre-green revolution hexaploid wheat. PLoS ONE 10:e0145227. doi: 10.1371/journal.pone.0145227
Ding, X. S., Flasinski, S., and Nelson, R. S. (1999). Infection of barley by Brome mosaic virus is restricted predominantly to cells in and associated with veins through a temperature-dependent mechanism. Mol. Plant Microbe Interact. 12, 615–623. doi: 10.1094/MPMI.1999.12.7.615
Ding, X. S., Mannas, S. W., Bishop, B. A., Rao, X., Lecoultre, M., Kwon, S., et al. (2018). An improved Brome mosaic virus silencing vector: greater insert stability and more extensive VIGS. Plant Physiol. 176, 496–510. doi: 10.1104/pp.17.00905
Ding, X. S., Schneider, W. L., Chaluvadi, S. R., Mian, M. A., and Nelson, R. S. (2006). Characterization of a Brome mosaic virus strain and its use as a vector for gene silencing in monocotyledonous hosts. Mol. Plant Microbe Interact. 19, 1229–1239. doi: 10.1094/MPMI-19-1229
Dommes, A. B., Gross, T., Herbert, D. B., Kivivirta, K. I., and Becker, A. (2019). Virus-induced gene silencing: empowering genetics in non-model organisms. J. Exp. Bot. 70, 757–770. doi: 10.1093/jxb/ery411
Ellis, J. G., Lagudah, E. S., Spielmeyer, W., and Dodds, P. N. (2014). The past, present and future of breeding rust resistant wheat. Front. Plant Sci. 5:641. doi: 10.3389/fpls.2014.00641
Feldman, M., and Levy, A. (2012). Genome evolution due to allopolyploidization in wheat. Genetics 192, 763–774. doi: 10.1534/genetics.112.146316
Fitzgerald, T. L., Powell, J. J., Stiller, J., Weese, T. L., Abe, T., Zhao, G., et al. (2015). An assessment of heavy ion irradiation mutagenesis for reverse genetics in wheat (Triticum aestivum L.). PLoS ONE 10:e0117369. doi: 10.1371/journal.pone.0117369
Gredell, J. A., Berger, A. K., and Walton, S. P. (2008). Impact of target mRNA structure on siRNA silencing efficiency: a large-scale study. Biotechnol. Bioeng. 100, 744–755. doi: 10.1002/bit.21798
Guo, H., Yan, Z., Li, X., Xie, Y., Xiong, H., Liu, Y., et al. (2017). Development of a high-efficient mutation resource with phenotypic variation in hexaploid winter wheat and identification of novel alleles in the TaAGP.L-B1 gene. Front. Plant Sci. 8:1404. doi: 10.3389/fpls.2017.01404
Hawkesford, M. J. (2017). Genetic variation in traits for nitrogen use efficiency in wheat. J. Exp. Bot. 68, 2627–2632. doi: 10.1093/jxb/erx079
Hayta, S., Smedley, M. A., Demir, S. U., Blundell, R., Hinchliffe, A., Atkinson, N., et al. (2019). An efficient and reproducible Agrobacterium-mediated transformation method for hexaploid wheat (Triticum aestivum L.). Plant Methods 15:121. doi: 10.1186/s13007-019-0503-z
He, X., Zeng, J., Cao, F., Ahmed, I. M., Zhang, G., Vincze, E., et al. (2015). HvEXPB7, a novel beta-expansin gene revealed by the root hair transcriptome of Tibetan wild barley, improves root hair growth under drought stress. J. Exp. Bot. 66, 7405–7419. doi: 10.1093/jxb/erv436
Hoagland, D. R., and Arnon, D. I. (1950). The Water-Culture Method for Growing Plants Without Soil. Berkeley, CA: College of Agriculture, University of California.
Hodge, B. A., Salgado, J. D., Paul, P. A., and Stewart, L. R. (2019). Characterization of an Ohio isolate of Brome mosaic virus and its impact on the development and yield of soft red winter wheat. Plant Dis. 103, 1101–1111. doi: 10.1094/PDIS-07-18-1282-RE
Itaya, K., and Ui, M. (1966). A new micromethod for the colorimetric determination of inorganic phosphate. Clin. Chim. Acta 14, 361–366. doi: 10.1016/0009-8981(66)90114-8
Jarugula, S., Willie, K., and Stewart, L. R. (2018). Barley stripe mosaic virus (BSMV) as a virus-induced gene silencing vector in maize seedlings. Virus Genes 54, 616–620. doi: 10.1007/s11262-018-1569-9
Jia, M., Guan, J., Zhai, Z., Geng, S., Zhang, X., Mao, L., et al. (2018). Wheat functional genomics in the era of next generation sequencing: an update. Crop J. 6, 7–14. doi: 10.1016/j.cj.2017.09.003
Jiao, Z., Tian, Y., Cao, Y., Wang, J., Zhan, B., Zhao, Z., et al. (2021). A novel pathogenicity determinant hijacks maize catalase 1 to enhance viral multiplication and infection. New Phytol. 230, 1126–1141. doi: 10.1111/nph.17206
Jordan, K. W., Wang, S., Lun, Y., Gardiner, L. J., MacLachlan, R., Hucl, P., et al. (2015). A haplotype map of allohexaploid wheat reveals distinct patterns of selection on homoeologous genomes. Genome Biol. 16:48. doi: 10.1186/s13059-015-0606-4
Kant, R., and Dasgupta, I. (2019). Gene silencing approaches through virus-based vectors: speeding up functional genomics in monocots. Plant Mol. Biol. 100, 3–18. doi: 10.1007/s11103-019-00854-6
Lesk, C., Rowhani, P., and Ramankutty, N. (2016). Influence of extreme weather disasters on global crop production. Nature 529, 84–87. doi: 10.1038/nature16467
Li, G. Z., Li, H. X., Xu, M. J., Wang, P. F., Xiao, X. H., and Kang, G. Z. (2020). Functional characterization and regulatory mechanism of wheat CPK34 kinase in response to drought stress. BMC Genomics 21:577. doi: 10.1186/s12864-020-06985-1
Liang, Z., Chen, K., Li, T., Zhang, Y., Wang, Y., Zhao, Q., et al. (2017). Efficient DNA-free genome editing of bread wheat using CRISPR/Cas9 ribonucleoprotein complexes. Nat. Commun. 8:14261. doi: 10.1038/ncomms14261
Liang, Z., Chen, K., Zhang, Y., Shan, Q., Wang, Y., Li, J., et al. (2018). Genome editing of bread wheat using biolistic delivery of CRISPR/Cas9 in vitro transcripts or ribonucleoproteins. Nat. Protoc. 13, 413–430. doi: 10.1038/nprot.2017.145
Liu, N., Xie, K., Jia, Q., Zhao, J., Chen, T., Li, H., et al. (2016). Foxtail mosaic virus-induced gene silencing in monocot plants. Plant Physiol. 171, 1801–1807. doi: 10.1104/pp.16.00010
Liu, Y., Lin, Y., Gao, S., Li, Z., Ma, J., Deng, M., et al. (2017). A genome-wide association study of 23 agronomic traits in Chinese wheat landraces. Plant J. 91, 861–873. doi: 10.1111/tpj.13614
López-Arredondo, D. L., Leyva-González, M. A., González-Morales, S. I., López-Bucio, J., and Herrera-Estrella, L. (2014). Phosphate nutrition: improving low-phosphate tolerance in crops. Annu. Rev. Plant Biol. 65, 95–123. doi: 10.1146/annurev-arplant-050213-035949
Lück, S., Kreszies, T., Strickert, M., Schweizer, P., Kuhlmann, M., and Douchkov, D. (2019). siRNA-Finder (si-Fi) software for RNAi-target design and off-target prediction. Front. Plant Sci. 10:1023. doi: 10.3389/fpls.2019.01023
Lynch, J. P. (2019). Root phenotypes for improved nutrient capture: an underexploited opportunity for global agriculture. New Phytol. 223, 548–564. doi: 10.1111/nph.15738
Mei, Y., Beernink, B. M., Ellison, E. E., Konečná, E., Neelakandan, A. K., Voytas, D. F., et al. (2019). Protein expression and gene editing in monocots using foxtail mosaic virus vectors. Plant Direct. 3, 1–16. doi: 10.1002/pld3.181
Mei, Y., Zhang, C., Kernodle, B. M., Hill, J. H., and Whitham, S. A. (2016). A Foxtail mosaic virus vector for virus-induced gene silencing in maize. Plant Physiol. 171, 760–772. doi: 10.1104/pp.16.00172
Mise, K., and Pocsai, E. (2004). “Brome mosaic,” in Viruses and Virus Diseases of Poaceae (Gramineae), eds H. Lapierre and P. A. Signoret (Paris, France: INRA), 735–739.
Montenegro, J. D., Golicz, A. A., Bayer, P. E., Hurgobin, B., Lee, H., Chan, C. K., et al. (2017). The pangenome of hexaploid bread wheat. Plant J. 90, 1007–1013. doi: 10.1111/tpj.13515
Nelson, R. S., Li, G. X., Hodgson, R. A. J., Beachy, R. N., and Shintaku, M. H. (1993). Impeded phloem-dependent accumulation of the masked strain of Tobacco mosaic virus. Mol. Plant Microbe Interact. 6, 45–54. doi: 10.1094/MPMI-6-045
Noueiry, A. O., and Ahlquist, P. (2003). Brome mosaic virus RNA replication: revealing the role of the host in RNA virus replication. Annu. Rev. Phytopathol. 41, 77–98. doi: 10.1146/annurev.phyto.41.052002.095717
Ouyang, X., Hong, X., Zhao, X., Zhang, W., He, X., Ma, W., et al. (2016). Knock out of the PHOSPHATE 2 gene TaPHO2-A1 improves phosphorus uptake and grain yield under low phosphorus conditions in common wheat. Sci. Rep. 6:29850. doi: 10.1038/srep29850
Pacak, A., Geisler, K., Jørgensen, B., Barciszewska-Pacak, M., Nilsson, L., Nielsen, T. H., et al. (2010b). Investigations of Barley stripe mosaic virus as a gene silencing vector in barley roots and in Brachypodium distachyon and oat. Plant Methods 6:26. doi: 10.1186/1746-4811-6-26
Pacak, A., Strozycki, P. M., Barciszewska-Pacak, M., Alejska, M., Lacomme, C., Jarmołowski, A., et al. (2010a). The Brome mosaic virus-based recombination vector triggers a limited gene silencing response depending on the orientation of the inserted sequence. Arch. Virol. 155, 169–179. doi: 10.1007/s00705-009-0556-9
Pant, B. D., Buhtz, A., Kehr, J., and Scheible, W. R. (2008). MicroRNA399 is a long-distance signal for the regulation of plant phosphate homeostasis. Plant J. 53, 731–738. doi: 10.1111/j.1365-313X.2007.03363.x
Paolacci, A. R., Tanzarella, O. A., Porceddu, E., and Ciaffi, M. (2009). Identification and validation of reference genes for quantitative RT-PCR normalization in wheat. BMC Mol. Biol. 10:11. doi: 10.1186/1471-2199-10-11
Perlmutter, J. D., Qiao, C., and Hagan, M. F. (2013). Viral genome structures are optimal for capsid assembly. eLife 2:e00632. doi: 10.7554/eLife.00632
Ramanna, H., Ding, X. S., and Nelson, R. S. (2013). Rationale for developing new virus vectors to analyze gene function in grasses through virus-induced gene silencing. Methods Mol. Biol. 975, 15–32. doi: 10.1007/978-1-62703-278-0_2
Ramírez-González, R. H., Borrill, P., Lang, D., Harrington, S. A., Brinton, J., Venturini, L., et al. (2018). The transcriptional landscape of polyploid wheat. Science 361:eaar6089. doi: 10.1126/science.aar6089
Ruiz-Sola, M. Á., and Rodríguez-Concepción, M. (2012). Carotenoid biosynthesis in Arabidopsis: a colorful pathway. Arab B. 10:e0158. doi: 10.1199/tab.0158
Safar, J., Simkova, H., Kubalakova, M., Cihalikova, J., Suchankova, P., Bartos, J., et al. (2010). Development of chromosome-specific BAC resources for genomics of bread wheat. Cytogenet. Genome Res. 129, 211–223. doi: 10.1159/000313072
Schneider, C. A., Rasband, W. S., and Eliceiri, K. W. (2012). NIH Image to ImageJ: 25 years of image analysis. Nat. Methods. 9, 671–675. doi: 10.1038/nmeth.2089
Scofield, S. R., and Nelson, R. S. (2009). Resources for virus-induced gene silencing in the grasses. Plant Physiol. 149, 152–157. doi: 10.1104/pp.108.128702
Senthil-Kumar, M., and Mysore, K. S. (2011). Virus-induced gene silencing can persist for more than 2 years and also be transmitted to progeny seedlings in Nicotiana benthamiana and tomato. Plant Biotechnol. J. 9, 797–806. doi: 10.1111/j.1467-7652.2011.00589.x
Shao, Y., Chan, C. Y., Maliyekkel, A., Lawrence, C. E., Roninson, I. B., and Ding, Y. (2007). Effect of target secondary structure on RNAi efficiency. RNA 13, 1631–1640. doi: 10.1261/rna.546207
Singh, D. K., Lee, H. K., Dweikat, I., and Mysore, K. S. (2018). An efficient and improved method for virus-induced gene silencing in sorghum. BMC Plant Biol. 18:123. doi: 10.1186/s12870-018-1344-z
Sun, L., Zhang, H., Li, D., Huang, L., Hong, Y., Ding, X. S., et al. (2013). Functions of rice NAC transcriptional factors, ONAC122 and ONAC131, in defense responses against Magnaporthe grisea. Plant Mol. Biol. 81, 41–56. doi: 10.1007/s11103-012-9981-3
Tadesse, W., Amri, A., Ogbonnaya, F. C., Sanchez-Garcia, M., Sohail, Q., and Baum, B. (2016). “Wheat,” in Genetic and Genomic Resources for Grain Cereals Improvement, eds M. Singh and H. D. Upadhyaya (San Diego, CA: Academic Press), 81–124. doi: 10.1016/B978-0-12-802000-5.00002-2
Tan, Y., Bukys, A., Molnár, A., and Hudson, A. (2020). Rapid, high efficiency virus-mediated mutant complementation and gene silencing in Antirrhinum. Plant Methods 16:145. doi: 10.1186/s13007-020-00683-5
Uauy, C. (2017). Wheat genomics comes of age. Curr. Opin. Plant Biol. 36, 142–148. doi: 10.1016/j.pbi.2017.01.007
van der Linde, K., Kastner, C., Kumlehn, J., Kahmann, R., and Doehlemann, G. (2011). Systemic virus induced gene silencing allows functional characterization of maize genes during biotrophic interaction with Ustilago maydis. New Phytol. 189, 471–483. doi: 10.1111/j.1469-8137.2010.03474.x
Vance, V., and Vaucheret, H. (2001). RNA silencing in plants—defense and counterdefense. Science 292, 2277–2280. doi: 10.1126/science.1061334
Voinnet, O. (2001). RNA silencing as a plant immune system against viruses. Trends Genet. 17, 449–459. doi: 10.1016/S0168-9525(01)02367-8
Wang, K., Gong, Q., and Ye, X. (2020). Recent developments and applications of genetic transformation and genome editing technologies in wheat. Theor. Appl. Genet. 133, 1603–1622. doi: 10.1007/s00122-019-03464-4
Wang, R., Yang, X., Wang, N., Liu, X., Nelson, R. S., Li, W., et al. (2016). An efficient virus-induced gene silencing vector for maize functional genomics research. Plant J. 86, 102–115. doi: 10.1111/tpj.13142
Wanjugi, H., Coleman-Derr, D., Huo, N., Kianian, S. F., Luo, M. C., Wu, J., et al. (2009). Rapid development of PCR-based genome-specific repetitive DNA junction markers in wheat. Genome 52, 576–587. doi: 10.1139/G09-033
Xue, G. P., Rae, A. L., White, R. G., Drenth, J., Richardson, T., and McIntyre, C. L. (2016). A strong root-specific expression system for stable transgene expression in bread wheat. Plant Cell Rep. 35, 469–481. doi: 10.1007/s00299-015-1897-3
Yang, J., Zhang, T. Y., Liao, Q. S., He, L., Li, J., Zhang, H. M., et al. (2018). Chinese wheat mosaic virus-induced gene silencing in monocots and dicots at low temperature. Front. Plant Sci. 9:1627. doi: 10.3389/fpls.2018.01627
Yu, H., Zhang, Q., Sun, P., and Song, C. (2018). Impact of droughts on winter wheat yield in different growth stages during 2001–2016 in eastern China. Int. J. Disaster Risk Sci. 9, 376–391. doi: 10.1007/s13753-018-0187-4
Yuan, C., Li, C., Yan, L., Jackson, A. O., Liu, Z., Han, C., et al. (2011). A high throughput barley stripe mosaic virus vector for virus induced gene silencing in monocots and dicots. PLoS ONE 6:e26468. doi: 10.1371/journal.pone.0026468
Zhao, Y., Zhang, C., Liu, W., Gao, W., Liu, C., Song, G., et al. (2016). An alternative strategy for targeted gene replacement in plants using a dual-sgRNA/ Cas9 design. Sci. Rep. 6:23890. doi: 10.1038/srep23890
Zhou, X., Zhu, X., Shao, W., Song, J., Jiang, W., He, Y., et al. (2020). Genome-wide mining of wheat DUF966 gene family provides new insights into salt stress responses. Front. Plant Sci. 11:569838. doi: 10.3389/fpls.2020.569838
Zhou, Y., Tang, H., Cheng, M. P., Dankwa, K. O., Chen, Z. X., Li, Z. Y., et al. (2017). Genome-wide association study for pre-harvest sprouting resistance in a large germplasm collection of Chinese wheat landraces. Front. Plant Sci. 8:401. doi: 10.3389/fpls.2017.00401
Zhu, M., Chen, Y., Ding, X. S., Webb, S. L., Zhou, T., Nelson, R. S., et al. (2014). Maize Elongin C interacts with the viral genome-linked protein, VPg, of Sugarcane mosaic virus and facilitates virus infection. New Phytol. 203, 1291–1304. doi: 10.1111/nph.12890
Keywords: Brome mosaic virus, virus-induced gene silencing, BMV-VIGS, insert stability, Wheat (Triticum aestivum), PHYTOENE DESATURASE, PHOSPHATE2, functional genomics
Citation: Wang Y, Chai C, Khatabi B, Scheible WR, Udvardi MK, Saha MC, Kang Y and Nelson RS (2021) An Efficient Brome mosaic virus-Based Gene Silencing Protocol for Hexaploid Wheat (Triticum aestivum L.). Front. Plant Sci. 12:685187. doi: 10.3389/fpls.2021.685187
Received: 24 March 2021; Accepted: 07 May 2021;
Published: 18 June 2021.
Edited by:
Guo-qing Song, Michigan State University, United StatesReviewed by:
Zhiyou Du, Zhejiang Sci-Tech University, ChinaYuanyue Shen, Beijing University of Agriculture, China
Tao Zhou, China Agricultural University, China
Copyright © 2021 Wang, Chai, Khatabi, Scheible, Udvardi, Saha, Kang and Nelson. This is an open-access article distributed under the terms of the Creative Commons Attribution License (CC BY). The use, distribution or reproduction in other forums is permitted, provided the original author(s) and the copyright owner(s) are credited and that the original publication in this journal is cited, in accordance with accepted academic practice. No use, distribution or reproduction is permitted which does not comply with these terms.
*Correspondence: Yun Kang, eXVua2FuZ0Bva3N0YXRlLmVkdQ==; Richard S. Nelson, cm5lbHNvQG9rc3RhdGUuZWR1
†Present address: Behnam Khatabi, Department of Agriculture, Food and Resource Sciences, University of Maryland Eastern Shore, Princess Anne, MD, United States