- 1College of Horticulture and Gardening, Yangtze University, Jingzhou, China
- 2National R&D for Se-rich Agricultural Products Processing Technology, Wuhan Polytechnic University, Wuhan, China
Ginkgo biloba has edible, medicinal, and ornamental value. However, the long juvenile phase prevents the development of the G. biloba industry, and there are few reports on the identification and functional analysis of genes regulating the flowering time of G. biloba. EMBRYONIC FLOWER 2 (EMF), an important protein in flower development, functions to promote vegetative growth and repress flowering. In this study, a novel EMF gene (GbEMF2) was cloned and characterized from G. biloba. GbEMF2 contains a 2,193 bp open reading frame (ORF) encoding 730 amino acids. GbEMF2 harbors conserved VEFS-Box domain by the plant EMF protein. The phylogenic analysis showed that GbEMF2 originated from a polycomb-group (Pc-G) protein ancestor and was a member of the EMF2 protein. The quantitative real-time PCR (qRT-PCR) analysis revealed that GbEMF2 was expressed in all detected organs, and it showed a significantly higher level in ovulating strobilus and microstrobilus than in other organs. Compared with emf2 mutant plants, overexpression of GbEMF2 driven by the CaMV 35S promoter in emf2 mutant Arabidopsis plants delayed flowering but earlier than wild-type (WT) plants. This result indicated that GbEMF2 repressed flowering in G. biloba. Moreover, the RNA-seq analysis of GbEMF2 transgenic Arabidopsis plants (GbEMF2-OE/emf2), WT plants, and emf2 mutants screened out 227 differentially expressed genes (DEGs). Among these DEGs, FLC, MAF5, and MAF5-1 genes were related to flower organ development and regulated by GbEMF2. In addition, some genes participating in sugar metabolism, such as Alpha-amylase 1 (AMY1), BAM1, and Sucrose synthase 3 (SUS3) genes, were also controlled by GbEMF2. Overall, our results suggested that GbEMF2 negatively regulates flowering development in G. biloba. This finding provided a foundation and target gene for shortening the Ginkgo juvenile period by genetic engineering technology.
Introduction
Ginkgo biloba L. (G. biloba), which has a long life and is known as “gongsun tree,” is only one of the Ginkgoaceae and appeared in the Mesozoic era with an extremely long juvenile stage (Ye et al., 2019). Ginkgo biloba has a long history of cultivation in China and is widely used in the fields of landscaping, medicine, and food (Crane, 2018). It usually takes 15–20 years for Ginkgo trees to blossom and bear fruit, which is a serious obstacle to breed good Ginkgo varieties (Yan et al., 2017). For many years, breeding scientists have tried to shorten the infancy of G. biloba through asexual propagation methods, such as cutting and grafting. Though in that case, blossoming and bearing fruit takes 6–8 years, it is still a long breeding cycle for trees. Thus, new approaches are needed to further shorten the infancy of G. biloba. Shortening the time from infancy to flowering mechanism in study of Arabidopsis thaliana (Yoshida et al., 2001), Glycine max (Cai et al., 2018), and apple (Charrier et al., 2019) maybe provide a reference for G. biloba.
Flowering, a key developmental trait, has attracted much attention from researchers. With the in-depth study of plant flowering in molecular biology, the theory for a flowering regulation model has been gradually deepened, from the most classic ABC model (Coen et al., 1990) to the classic “Enabling, Promoting, Resetting” model proposed by Boss et al. (2004). Numerous flowering genes are involved in these model systems by regulating different flowering pathways in plants. The development of woody plants is comprised of main stages from vegetative growth to flowering. The first stage is the transition from vegetative growth to reproductive growth, which is synergistically regulated by many genes. Some of them are transcriptional regulators, such as FLOWERING LOCUS C (FLC; Wu et al., 2016), CONSTANS (CO; Mulki and von Korff, 2016), and EMBRYONIC FLOWER 2 (EMF; Liu et al., 2012). Some genes function as signal transduction, for instance, FLOWERING LOCUS T (FT; Navarro et al., 2011), TERMINAL FLOWER (TFL; Wickland and Hanzawa, 2015). The second stage is the transition from inflorescence to flowering. In this stage, the genes associating with flowering mainly include meristem-specific genes and flower organ-specific genes (MADS-box family), which regulate the formation of meristems and flower organs in time and space. For example, LEAFY (LFY) and APETALA1 (AP1) play important roles in flowering initiation in the meristem (Weigel et al., 1992; Eckardt, 2006). The development of flower organs depends on many flower organ-specific genes, such as AGAMOUS, AP1, AP3, and PISTILLATA (PI; Krizek and Fletcher, 2005). Overexpressing and silencing the MAF1 gene led to late flowering and early flowering, respectively. In addition, MAF1 directly repressed AP3 and activated MAF2, which negatively regulated flowering (Huang et al., 2018). FRIGIDA (FRI) delayed flowering by activating the expression of target genes FLC, MAF4, and MAF5 (Kong et al., 2019). FLC, acting as a MADS-box transcription factor and a floral repressor, regulates flowering by directly repressing downstream genes, such as FT and SUPPRESSOR OF OVEREXPRESSION OF CONSTANS1 (SOC1; Deng et al., 2011). This regulating process was enhanced by the interaction of DELLA and FLC (Li et al., 2016a). Further, DELLAs interacted with SQUAMOSA PROMOTER BINDING PROTEIN-LIKE (SPL) to interfere SPL transcriptional activity and affect flowering time (Yu et al., 2012). At present, some flowering-related genes have been identified in G. biloba, such as GbCO (Yan et al., 2017), GbFT (Wang et al., 2019), GbMADS2 (Wang et al., 2015), GbSEP (Cheng et al., 2016), and GbMADS9 (Yang et al., 2016).
The EMF, an important gene inhabiting the flowering of plants, has become a hot topic in recent years. Thus far, scientists have cloned EMF gene family members in A. thaliana (Yoshida et al., 2001), rice (Li et al., 2006), broccoli (Liu et al., 2012), bamboo (Xu et al., 2010), and cotton (Ma et al., 2020). The members of the EMF gene family were EMF1 and EMF2, which encode proteins with great differences in sequence and structure. EMF2, FERTILIZATION INDEPENDENT SEED2 (FIS), and VERNALIZATION2 (VRN2) encode Suppressor of zeste 12 [Su(Z)12] subunit, and EMF2, FIS2, and VRN2 are homologous proteins of Su(Z)12 (Chaudhury et al., 1997; Gendall et al., 2001; Yoshida et al., 2001). Studies in model plants, such as A. thaliana, have shown that EMF regulates the differentiation of plant vegetative growth to the flower meristem, and its expression determines the time of plant vegetative growth (Sung et al., 1992; Yang et al., 1995) and is closely related to the development of inflorescence organs (Chen et al., 1997; Chanvivattana et al., 2004). EMF1 and EMF2 are constitutively expressed in the roots, rosette leaves, stem, mature leaves, and other vegetative organs and flower clusters of A. thaliana (Aubert et al., 2001; Yoshida et al., 2001). However, these findings are based on the research of annual herbs, and which viewpoint can correctly reflect the expression pattern of EMF genes in the woody plant development that remains to be further explored. Therefore, GbEMF2, one member of the EMF gene family, was cloned and characterized from G. biloba, a gymnospermous tree, in this study. GbEMF2 was transferred into Arabidopsis emf2 mutants, which verified that GbEMF2 was also involved in the regulation of flowering. Our findings not only establish a foundation for elucidating the gene regulation network of Ginkgo flowering, but also provide a target gene for using the genetic engineering technology to shorten the infancy of woody plants.
Materials and Methods
Plant Materials and Growth Conditions
Plant materials were collected from 31-year-old G. biloba “Jiafoshou” tree grown in the Ginkgo Science and Technology Garden, Yangtze University (around N30.35, E112.14). The roots, stems, leaves, microstrobilus, ovulate strobilus, and immature fruits of Ginkgo grafts were collected to test the spatial expression profile of GbEMF2. The harvested samples were rapidly frozen in liquid nitrogen and stored at −80°C in reserve.
Arabidopsis wild-type (WT) Landsberg erecta seeds and emf2 mutant seeds (SALK_115527) were purchased from the Arabidopsis Biological Research Center of Ohio State University, Columbus, OH, United States. The mutant seeds of emf2 were all grown in the MS plates with 0, 50, or 100 mg/L kanamycin, cold stratified at 4°C for 72 h, then transferred to an artificial climate incubator for germination and development. The artificial climate incubator was set to 16 h of light, 25°C, 8 h of darkness, at 18°C, 12,000 lex of light, and 70% humidity. About 15-day-old seedlings were transferred into soil and grown under long-day conditions for the observation of bolting and flowering development.
Cloning of Full-Length GbEMF2 cDNA
Total RNA was extracted from G. biloba microstrobilus using a TaKaRa MiniBEST Plant RNA Extraction Kit (Takara Bio Inc., Dalian, China). The first strand of cDNA was synthesized using the PrimeScript™ 1st cDNA Synthesis Kit. Specific primers (GbEMF2-F and GbEMF2-R; Takara Bio Inc., Dalian, China; Supplementary Table S1) were designed based on the EMF2 unigene sequence (CL9451Contig1) from G. biloba transcriptome data. The GbEMF2 gene was amplified using PCR under the following program: 94°C for 3 min; 33 cycles of 94°C for 30 s, 55°C for 30 s, 72°C for 90 s; and a final extension at 72°C for 10 min. The PCR product was tested with 1% agarose gel electrophoresis, and the target fragment was recovered by an Agarose Gel DNA Purification Kit (TaKaRa, Dalian, China). Then, the target fragment was ligated into the pMD19-T vector and transformed into DH5α. The single colony was picked and cultured. Screened positive clones were sent to Shanghai Sangon Biotech (Shanghai, China) for sequencing.
Bioinformatics and Molecular Evolution Analyses
Primers were designed using the Primer 5 software and the online PrimerQuest Tool.1 Higher similarity sequences were obtained by using the BLAST tool2 for homologous alignments. The DNA sequence analysis was completed by using the Vector NT I 11.5 (Invitrogen) and DNAMAN software.3 The protein sequence and oter homologous sequences on GenBank were analyzed using the CLUSTAL X24 and MEGA6 software,5 and a phylogenetic tree was constructed by the Neighbor-Joining method.
Quantitative Real-Time PCR Analysis
Total RNA was extracted from six organs of G. biloba using a TaKaRa MiniBEST Plant RNA Extraction Kit (TaKaRa, Dalian, China). The total RNA was isolated from each sample by using the PrimeScript™ RT Reagent Kit with the gDNA Eraser (Perfect Real Time; TaKaRa, Dalian, China). First-strand cDNA was generated from 1 μg of total RNA. cDNA was diluted 10 times as a template. The primers, GbEMF2-dF and GbEMF2-dR (Supplementary Table S1), were designed for the quantitative real-time PCR (qRT-PCR) amplification. GbGAPDH (Meng et al., 2018) was used as the internal reference gene, and its primers were GbGAPDH-F and GbGAPDH-R (Supplementary Table S1). qRT-PCR was performed using the BioEasy Master Mix (SYBR Green Mix BIOER, Hangzhou, China) according to the instructions of the manufacturer. The reaction system was 20 μl and contained the following: 10 μl of 2 × SYBR Green Mix, 0.2 μl of each primer (10 μM), 2 μl of diluted cDNA, and 7.6 μl of nuclease-free water. The PCR program was as follows: stage one, 95°C for 30 s; stage two, 40 cycles of 95°C for 10 s and 60°C for 30 s; stage three, 95°C for 15 s, 60°C for 1 min, and 95°C for 15 s. Three biological replicates were prepared per sample, and relative expression levels calculations, which were performed using the 2-△△Ct method (Schmittgen and Livak, 2008).
Vector Construction and Arabidopsis Transformation
The GbEMF2 was amplified using GbEMF2-X and GbEMF2-B (Supplementary Table S1). The restriction enzyme cutting site of Xba I and Bam HI was introduced into GbEMF2-X and GbEMF2-B, respectively. The pBI121-GUS vector was rebuilt and saved by our laboratory. The recombinant GbEMF2-pBI121-GUS vector was obtained by inserting the open reading frames (ORFs) of GbEMF2 into the pBI121-GUS vector digested with Xba I and Bam HI with T4 DNA ligase (TaKaRa, Dalian, China). Recombinant GbEMF2-pBI121-GUS vector was introduced into Agrobacterium tumefaciens strain LBA4404 by the liquid nitrogen freezing–thawing method for A. thaliana transformation of emf2 mutant using the floral dip method (Clough and Bent, 1998).
Screening and Detection of Transgenic A. thaliana
Putative transformants were selected on the MS plates with 100 mg/L kanamycin (primary transformants were defined as T0). T0 seeds were sown on the MS plates with 100 mg/L kanamycin and cold stratified at 4°C for 72 h. Later, the seeds were transferred to an artificial climate incubator for germination and development. The artificial climate incubator was set to 16 h of light, 25°C, 8 h of darkness, 18°C, 12,000 lex of light, and 70% humidity. About 15-day-old seedlings were transferred into soil and grown under long-day condition. T1 and T2 transgenic plants were further confirmed by PCR and β-glucuronidase activity, referring to the method of Wang et al. (2019). T3 seeds from T2 transgenic plants were harvested following the screening by the MS plates with 100 mg/L kanamycin. The flowering time of T3 GbEMF2 transgenic plants, emf2 mutants, and WT A. thaliana plants was recorded (Hanano and Goto, 2011).
RNA-Seq Analysis and qRT-PCR Validation
The WT, emf2 mutants, and GbEMF2-OE/emf2 (T3 generation) transgenic of A. thaliana plants were planted in an artificial climate incubator. The samples (selected above-ground part of the plants) were collected when the GbEMF2-OE/emf2 transgenic was blooming and sent to Biomarker Biotechnology Corporation (Beijing, China) for RNA-seq. Every sample had three biological replicates, and one replicate had 10 plants. Raw data were generated by the Illumina HiSeq 2500 High-Throughput Sequencing (Illumina, San Diego, CA, United States). After removing low-quality clean reads, high-quality clean reads were aligned to the genome data of A. thaliana by HISAT2 (Kim et al., 2015) and assembled by StringTie6 (Pertea et al., 2015). The download for genome data of A. thaliana is available at https://www.arabidopsis.org/download/index-auto.jsp?dir=%2Fdownload_files%2FGenes%2FTAIR10_genome_release%2FTAIR10_chromosome_files. To compare the GbEMF2 T3 group with the emf2 mutant and WT groups, the differentially expressed genes (DEGs) were annotated with Nr7 (NCBI nonredundant protein sequences), Swiss-Prot8 (a manually annotated and reviewed protein sequence database), gene ontology (GO) annotation,9 COG annotation,10 KOG,11 Protein family (Pfam),12 and Kyoto Encyclopedia of Genes and Genomes (KEGG).13 We screened for gene differential expression by DESeq2. Genes with a fold change of ≥2 and FDR < 0.01 were defined as DEGs. qRT-PCR with random selection, some DEGs were conducted for verifying RNA-seq data. AtActin was selected as the internal reference gene of A. thaliana, and its primers were AtActin-F and AtActin-R (Supplementary Table S1). RNA samples were returned by Biomarker Biotechnology Corporation (Beijing, China) for validation of RNA-seq. Three biological replicates were prepared per sample, and relative expression levels calculations, which were performed using the 2-△△Ct method (Schmittgen and Livak, 2008). qRT-PCR was performed using the BioEasy Master Mix (SYBR Green Mix; BIOER, Hangzhou, China) according to the instructions of the manufacturer. The reaction system was 20 μl and contained the following: 10 μl of 2 × SYBR Green Mix, 0.2 μl of each primer (10 μM), 2 μl of diluted cDNA, and 7.6 μl of nuclease-free water. The PCR program was as follows: stage one, 95°C for 30 s; stage two, 40 cycles of 95°C for 10 s and 60°C for 30 s; stage three, 95°C for 15 s, 60°C for 1 min, and 95°C for 15 s. The qRT-PCR primers of selected DEGs are shown in Supplementary Table S1.
Results
Cloning and Characterization of GbEMF2 in G. biloba
The GbEMF2 gene was cloned from G. biloba (GenBank accession no. MH791443) and contained a 2,193 bp ORF, which encodes a 730 amino acid protein (Supplementary Figure S1). Its theoretical molecular weight and pI were 82.6 kDa and 7.33, respectively. The deduced amino acid sequence of GbEMF2 has 55, 54, 55, 52, and 54% similarity with AtrEMF2 (Amborella trichopoda), EgEMF2 (Elaeis guineensis), PdEMF2 (Phoenix dactylifera), PsEMF2 (Papaver somniferum), and CsEMF2 (Camellia sinensis), respectively (Supplementary Table S2). Furthermore, the alignment analysis of these amino acid sequences showed the homology of GbEMF2 with EMF2 proteins of the amborellales (Amborella trichopoda), dicotyledons (P. dactylifera, P, somniferum, and C. sinensis) and monocotyledon (E. guineensis; Figure 1). The conserved domain analysis demonstrated that GbEMF2 contained the VEFS-Box domain belong to a member of Su(Z)12. We constructed a phylogenetic tree for understanding the evolution of GbEMF2 (Supplementary Table S3). As the phylogenetic tree is shown (Figure 2), EMF2 protein, VRN2 protein, and FIS2 protein originated from a common ancestor. GbEMF2 was divided into EMF2 protein and had the closest relationship with AtrEMF2, EgEMF2, PdEMF2, VvEMF2, and RcEMF2.
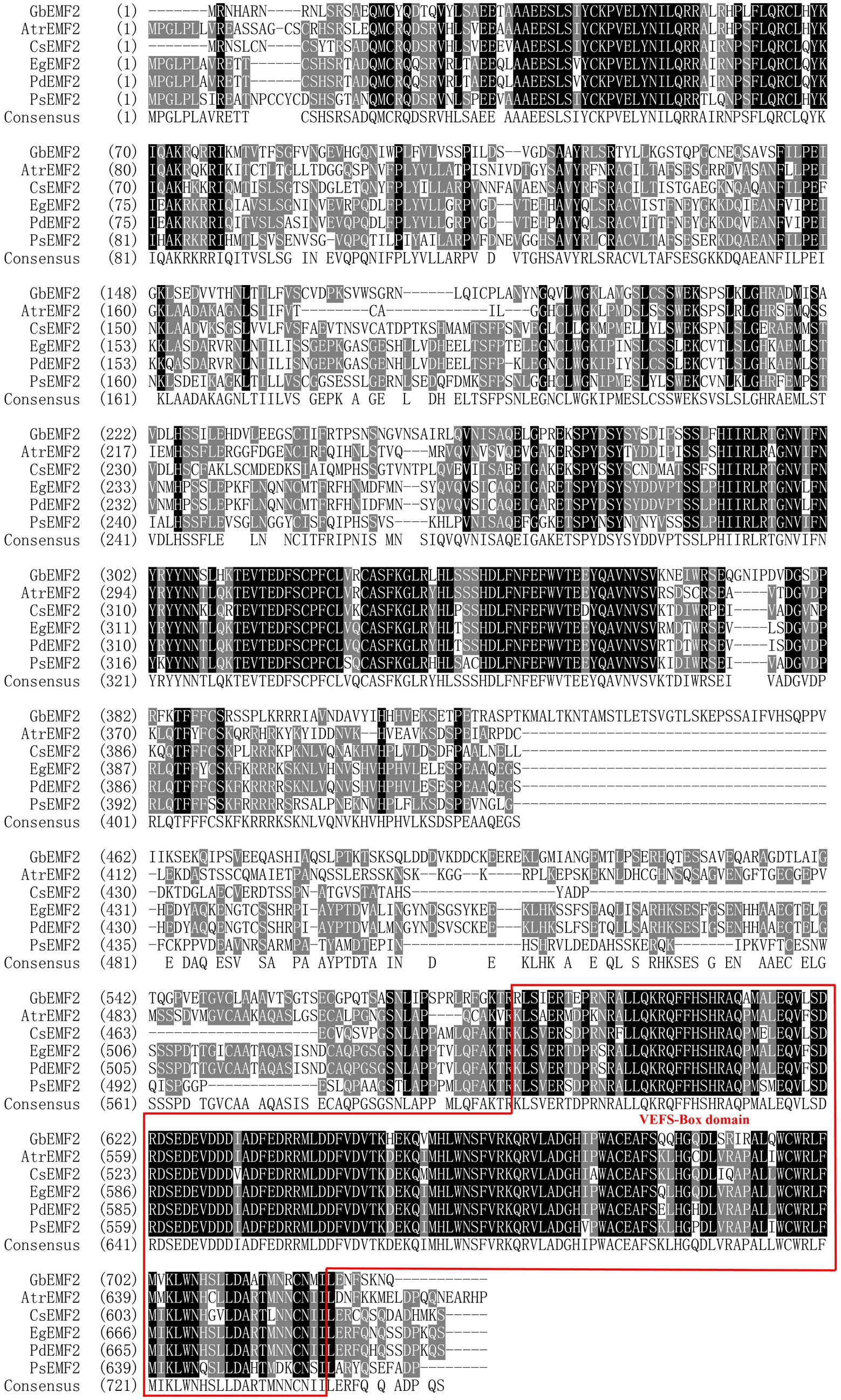
Figure 1. Similarity analysis of GbEMF2 protein and EMF2 proteins in other species. GbEMF2 (Ginkgo biloba), AtrEMF2 (Amborella trichopoda), EgEMF2 (Elaeis guineensis), PdEMF2 (Phoenix dactylifera), PsEMF2 (Papaver somniferum), and CsEMF2 (Camellia sinensis). Shaded in black are identical sequence. Shaded in gray are conservative sequences. The red box is VEFS-Box conserved domain of EMF2 homologous protein family.
Expression Patterns of GbEMF2 in Different Organs of G. biloba
To characterize the function of GbEMF2, we performed qRT-PCR experiments in different Ginkgo organs. Our results showed that GbEMF2 was detected in the measured organs (Figure 3). The expression level of the reproductive organs was significantly higher than that of the vegetative organs. In particular, the GbEMF2 gene was mainly expressed in ovulate strobilus and microstrobilus with a significantly higher level than that in immature fruits, stems, leaves, and roots. The expression level of GbEMF2 was rarely observed in the roots.
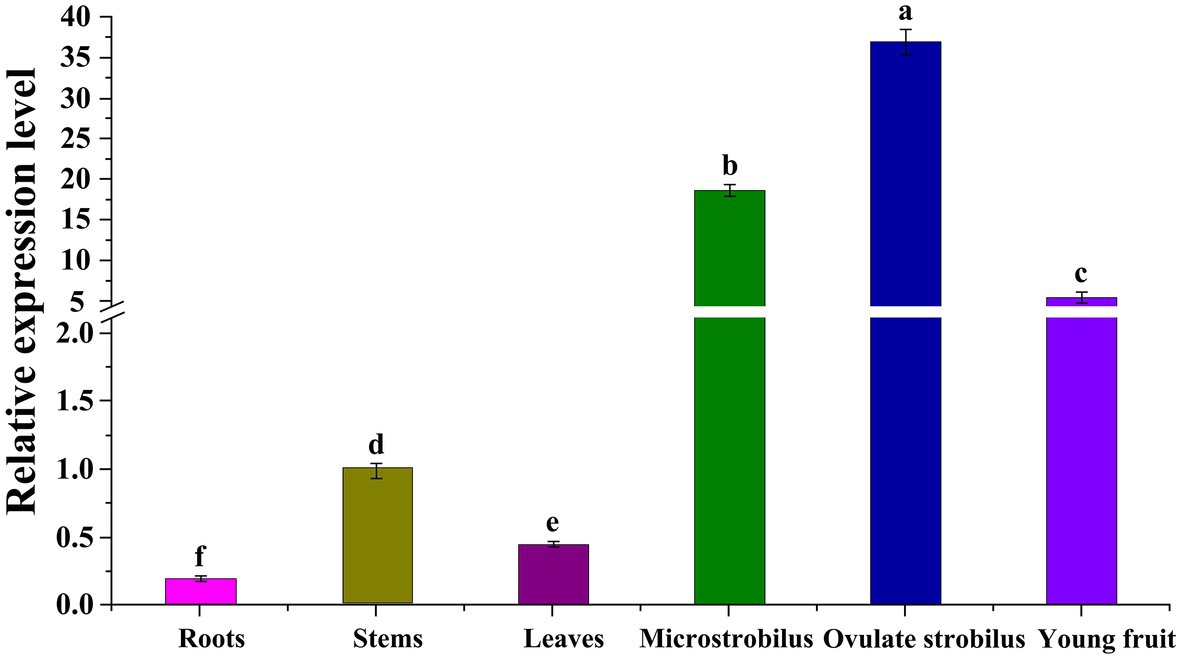
Figure 3. Expression patterns of GbEMF2 in different organs of G. biloba with quantitative real-time PCR (qRT-PCR). Data are means ± SD for n = 3 biological replicates. Letters mean significant difference at p ≤ 0.05 by the Duncan’s multiple range tests.
Ectopic Overexpression of GbEMF2 in Arabidopsis Plants
To further investigate the function of GbEMF2 in flowering, GbEMF2 was transformed into Arabidopsis plants for heterologous expression. After harvesting T0 seeds from primary transformants of A. thaliana, the MS medium containing 100 mg/L kanamycin was used to screen GbEMF2 transgenic plants (Supplementary Figure S2). The T2 generation plants were screened with kanamycin (Supplementary Figure S3A). To further determine whether GbEMF2 had been successfully transformed into A. thaliana, the T2 generation plants were also tested by GUS staining (Supplementary Figure S3B) and the PCR verification with DNA which was served as template (Supplementary Figures S3C,D). After obtaining the verified T3 generation of transgenic A. thaliana (GbEMF2-OE/emf2), WT, and emf2 mutants were planted for phenotypic comparative observation and flowering time. As shown in Figures 4A,B, emf2 mutant flowered significantly earlier than the WT and GbEMF2-OE/emf2. The emf2 mutant bloomed 28 days after sowing, whereas GbEMF2-OE/emf2 flowered approximately 31 days and WT flowered around 32 days after sowing, respectively. Taken together, our data showed that the overexpression of the GbEMF2 gene restored the phenotype of premature flowering in Arabidopsis emf2 mutant plants. It is indicated that the GbEMF2 gene might regulate flowering in G. biloba.
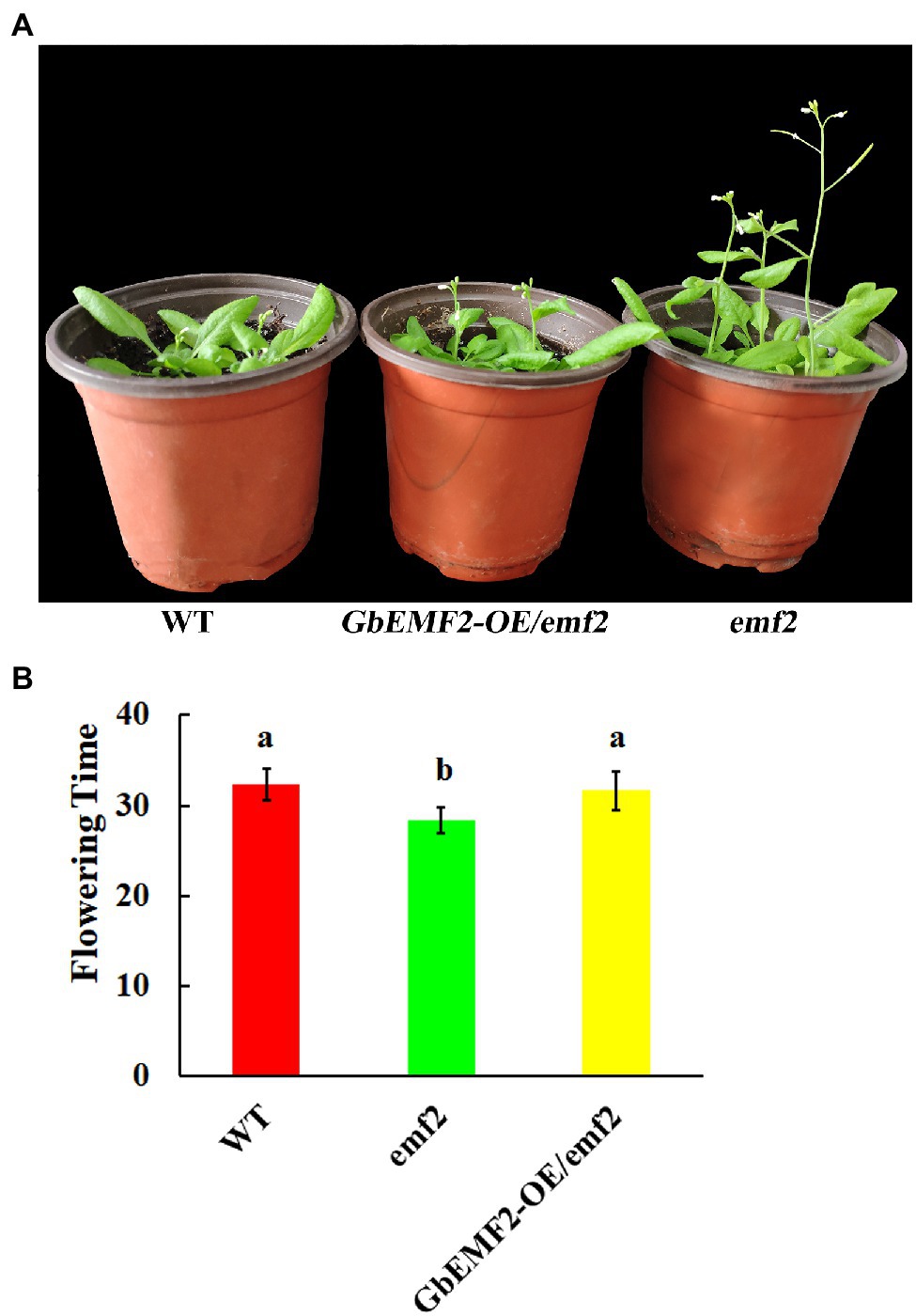
Figure 4. Comparative analysis of the flowering time in GbEMF2-OE/emf2, wild-type (WT), and emf2 mutant of Arabidopsis. GbEMF2-OE/emf2, T3 generation of GbEMF2 transgenic Arabidopsis; WT, wild-type Arabidopsis; emf2, Arabidopsis emf2 mutant plants. (A) Comparison of morphological characteristics of the flowering time. (B) Comparison of the days of flowering time of different plants. Each sample has three biological replicates, and data are mean ± SD for three biological replicates, with 10 plants per replicate. Letters mean significant difference at p ≤ 0.05 by the Duncan’s multiple range tests.
Identification of DEGs Among the WT, emf2 Mutants, and GbEMF2 Transgenic Arabidopsis Plants by RNA-Seq Analysis
To determine the molecular mechanism of early-flowering phenotypes caused by the overexpression of GbEMF2, we studied the global expression pattern of GbEMF2 transgenic Arabidopsis plants (GbEMF2-OE/emf2), emf2 mutants, and WT plants (CK) using RNA-seq assay. A total of nine transcriptomes were generated from the shoot of WT plants (CK group: WT-1, WT-2, and WT-3), emf2 mutant (emf2 group: emf2-1, emf2-2, and emf2-3), and GbEMF2 transgenic Arabidopsis plants (GbEMF2 transgenic group: GbEMF2-OE/emf2-1, GbEMF2-OE/emf2-2, and GbEMF2-OE/emf2-3), each group contained three biological replicates and 10 plants per replicate. Clean reads at 97.69–98.03% of each sample could be matched to the reference Arabidopsis genome (A. thaliana, TAIR10.37). DEGs were first identified through comparisons of the Fragments Per Kilobase of transcript per Million mapped reads (FPKM) values for each gene of the CK group, emf2 group, and GbEMF2 group. A total of 3,600 DEGs were detected in three group pairwise comparisons: 2,480 DEGs in WT_vs_emf2, 2,186 DEGs in WT_vs_GbEMF2-OE/emf2, and 1,142 DEGs in emf2_vs_GbEMF2-OE/emf2 (Supplementary Figure S4). The GO annotation was conducted to determine the function of the three groups in each comparison. Among these DEGs, 3,413 DEGs were annotated into the GO database that could be classified into three GO categories: biological process, cellular component, and molecular function. Within the biological process category, the most highly represented terms were “single-organism process,” “cellular process,” “metabolic process,” and “response to stimulus.” Within the cellular component category were “cell,” “cell part,” “organelle,” and “membrane.” Within the molecular function category, “catalytic activity” and “binding” were the two most abundant terms (Figures 5A–C; Supplementary Tables S4–S6). In addition, we conducted common expression patterns based on the FPKM of 3,600 DEGs in each sample by using the Euclidean distance algorithm combined with the K-means algorithm. These DEGs were classified into 12 clusters (Figure 6A), which had similar expression patterns in the same subclass (Figure 6B).
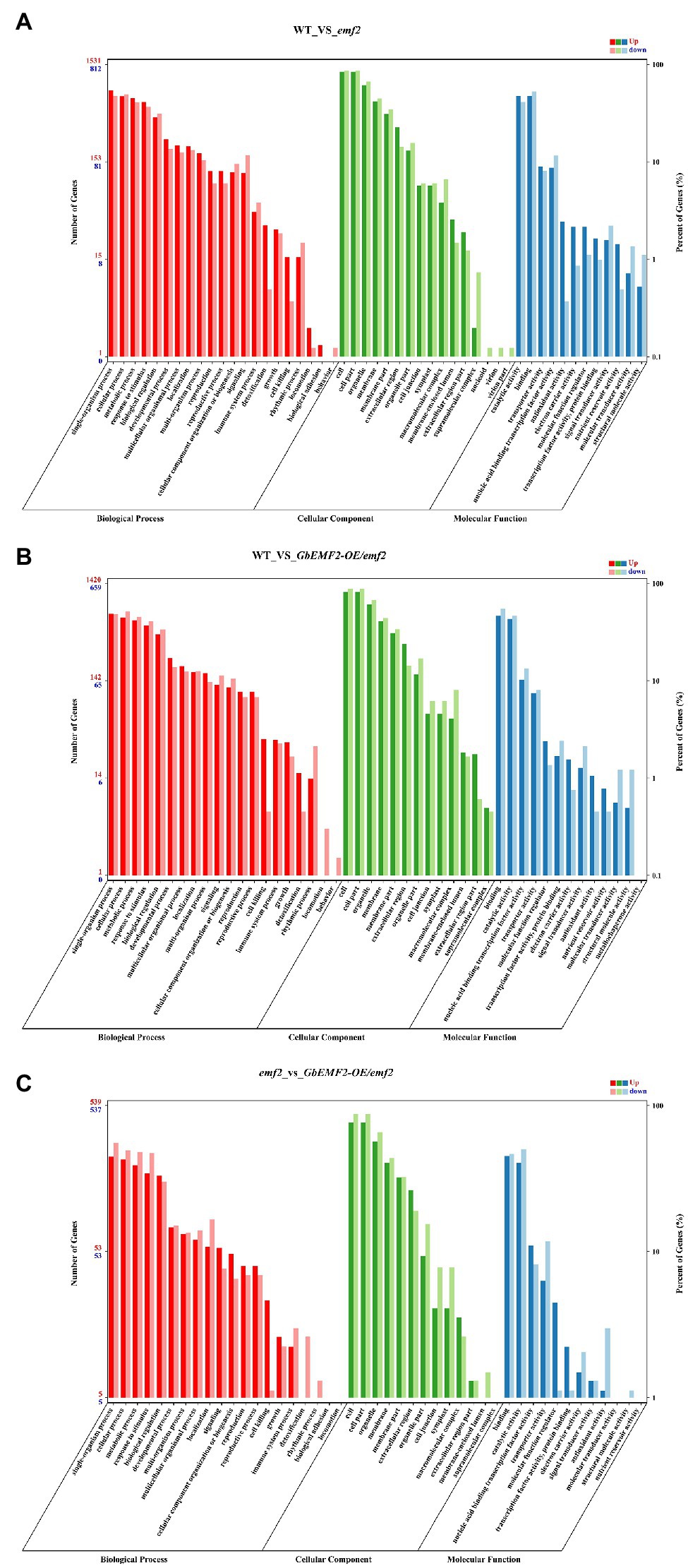
Figure 5. Gene ontology (GO) of differentially expressed genes (DEGs) analysis of WT, emf2 mutant, and GbEMF2-OE/emf2 plant. A total of 3,414 DEGs were annotated by at least one of the three categories: biological process, cellular component, and molecular function. (A) The GO classification of WT_vs_GbEMF2-OE/emf2. (B) The GO classification of WT_vs_emf2. (C) The GO classification of emf2 vs_GbEMF2-OE/emf2. The x-axis indicates the level 2 GO terms, and the double y-axis means the number of genes and percentage. Red, green, and blue represent upregulated of biological process, cellular component, and molecular function, respectively. Dark red, dark green, and dark blue represent downregulated of biological process, cellular component, and molecular function, respectively.
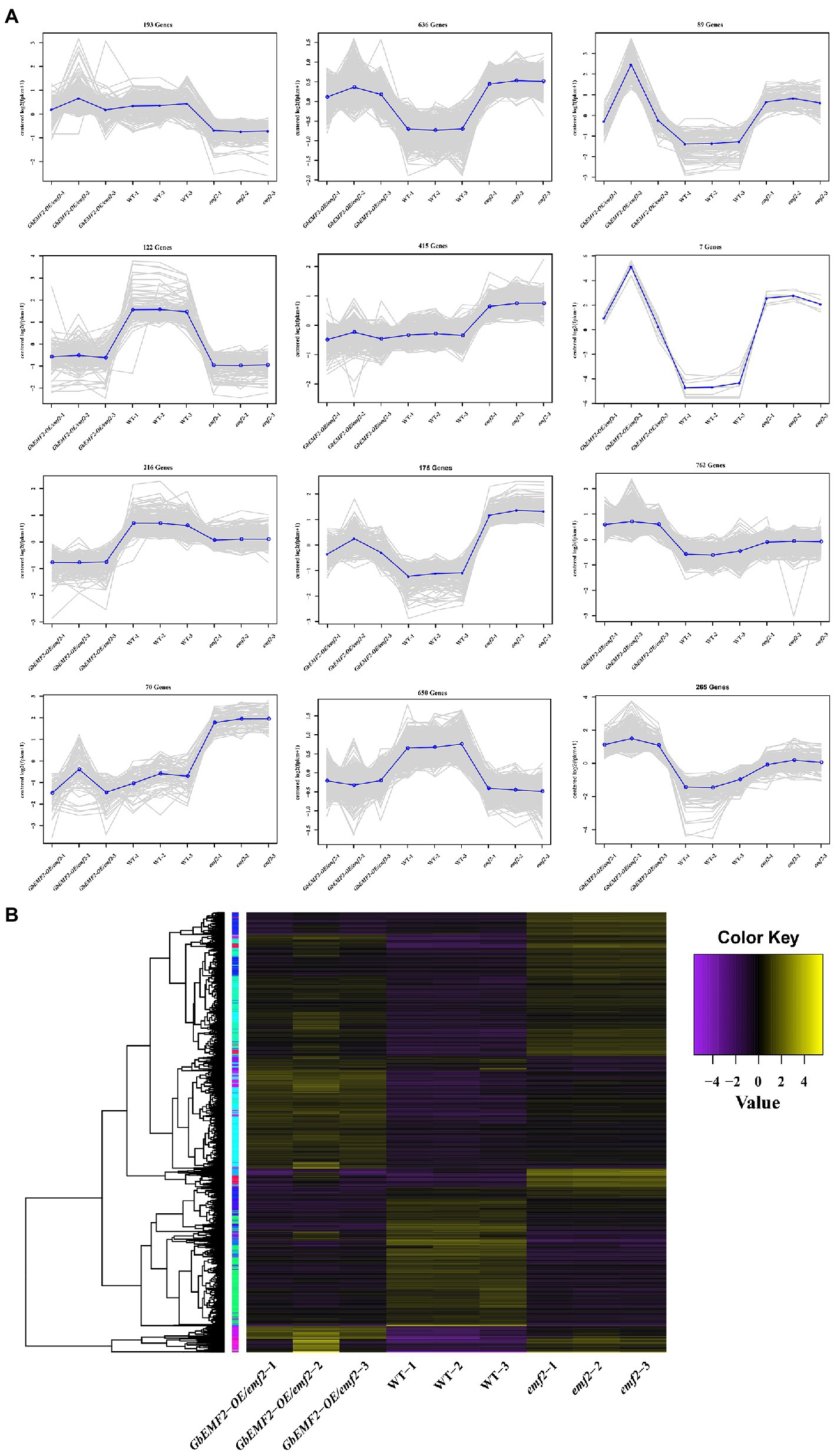
Figure 6. Expression profiles of significant DEGs. (A) Clusters of DEGs were obtained by K-means. DEGs were divided into 12 subclasses. (B) Heat map for cluster analysis of DEGs by hierarchical cluster combined with K-means method. The change in the expression level is represented by the change in color; purple indicates a lower expression level, and yellow indicates a higher expression level.
To further determine how the EMF2 gene affected metabolic pathways in the flowering process, we predicted the KEGG pathways. In WT_vs_emf2, WT_vs_GbEMF2-OE/emf2, and emf2_vs_GbEMF2-OE/emf2 comparisons, 468, 386, and 203 DEGs, respectively, were mapped to 106, 105, and 83 KEGG pathways, respectively (Supplementary Tables S7–S9). The top 20 pathways with significant enrichment were displayed in Figures 7A–C. The DEGs were focused on flowering-related pathways, such as “plant hormone signal transduction (ko04075),” “starch and sucrose metabolism (ko00500),” and “circadian rhythm-plant (ko04712).” Furthermore, the DEGs were focused on other pathways, such as “phenylpropanoid biosynthesis (ko00940)” and “Cyanoamino acid metabolism (ko00460).” Further analysis of the plant hormone signal transduction, four pathways revealed four DEGs, namely, GH3.17, SAUR4, PP2C, and PYL6 (Figure 7D). In addition, we found seven DEGs, including BGLU23, BGLU25, BGLU30, Sucrose synthase 3 (SUS3), AT2G21590, BAM1, and Alpha-amylase 1 (AMY1) in starch and sucrose metabolism (Figure 7E).
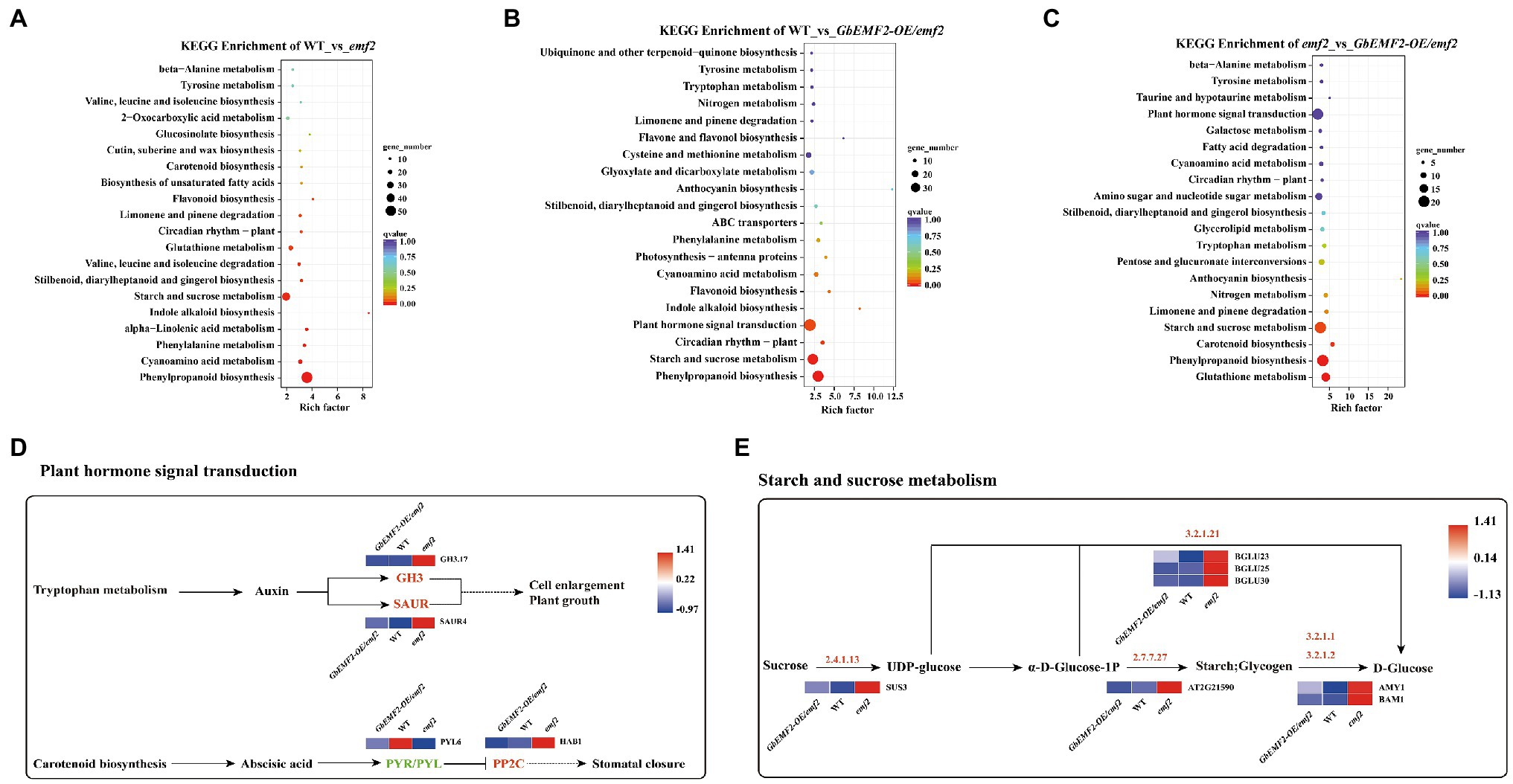
Figure 7. Analysis of DEGs of Kyoto Encyclopedia of Genes and Genomes (KEGG) pathway. (A) Analysis of DEGs of top 20 KEGG pathway in WT_vs_emf2. (B) Analysis of DEGs of top 20 KEGG pathway in WT_vs_GbEMF2-OE/emf2. (C) Analysis of DEGs of top 20 KEGG pathway in emf2_vs_GbEMF2-OE/emf2. (D) The KEGG metabolic pathway of plant hormone signal transduction. Red indicates that the Fragments Per Kilobase of transcript per Million mapped reads (FPKM) value of genes is high, and blue indicates that the FPKM value of genes is low screened from KEGG orthology (KO) database. (E) The KEGG metabolic pathway of starch and sucrose. Red indicates that the FPKM value of genes is highly, and blue indicates that the FPKM value of genes is low screened from KO database.
The transformation of GbEMF2 resulted in a significant change in most flowering-related DEGs, which might lead to later flowering in transgenic Arabidopsis. We screened 40 flowering-related DEGs in Table 1, and these genes were divided into seven categories: flower organ development, ATP-binding cassette (ABC) transporter, auxin pathway, ethylene pathway, abscisic acid pathway, cytochrome P450, and transcription factor. In the emf2 group, the expression of DEGs was significantly upregulated or downregulated to ultimately influencing flowering. The expression of FLP2, M17, LEA2, SRF, FLC, MAF5, and MAF5-1, was not significantly upregulated or downregulated in WT_vs_GbEMF2-OE/emf2. However, the expression of FLP2, M17, LEA2, and MAF5 was significantly upregulated in WT_vs_emf2 and downregulated in emf2_vs_GbEMF2-OE/emf2. FLC was significantly downregulated in WT_vs_emf2 and upregulated in emf2_vs_GbEMF2-OE/emf2. Two ABC transporters were significantly upregulated in WT_vs_GbEMF2-OE/emf2 and WT_vs_emf2, and downregulated in emf2_vs_GbEMF2-OE/emf2. In the auxin pathway, SAUR41 and NRT1 were significantly upregulated in WT_vs_emf2 and downregulated in emf2_vs_GbEMF2-OE/emf2, whereas SAUR41 showed no difference in WT_vs_GbEMF2-OE/emf2. In the abscisic acid pathway, two genes exhibited no difference in WT_vs_GbEMF2-OE/emf2, but were significantly upregulated in WT_vs_emf2 and downregulated in emf2_vs_GbEMF2-OE/emf2. In the CYP450 family, six genes were significantly upregulated and downregulated in WT_vs_emf2 and emf2_vs_GbEMF2-OE/emf2, respectively. However, three genes among six genes were significantly upregulated, and other three genes have no difference in WT_vs_GbEMF2-OE/emf2. In addition, 13 transcription factors had the same expression level in WT_vs_GbEMF2-OE/emf2 but differently upregulated or downregulated in WT_vs_emf2 and emf2_vs_GbEMF2-OE/emf2.
The expression patterns for AtFER3, AtFLC, AtPYL6, and AtFER1 were significantly upregulated in GbEMF2-OE/emf2 compared with the emf2 group and were significantly upregulated in the WT compared to the GbEMF2-OE/emf2 group. In addition, the expression patterns for AtLEA2 and AtERF113 were significantly downregulated in GbEMF2-OE/emf2 compared with the emf2 group. The expression pattern for AtERF113 was significantly downregulated in the WT compared with GbEMF2-OE/emf2 plants. However, relating to the GbEMF2-OE/emf2 plants, the expression of AtLEA2 in WT plants showed no difference. To further validate the RNA-seq results, we randomly selected 16 DEGs for the qRT-PCR-based expression analysis in WT plants, emf2 mutant, and GbEMF2-OE/emf2 plants (Figure 8). The qRT-PCR data were consistent with the RNA-seq data, which confirmed the accuracy of our transcriptomic analysis. These results showed that GbEMF2 played a similar role with AtEMF2.
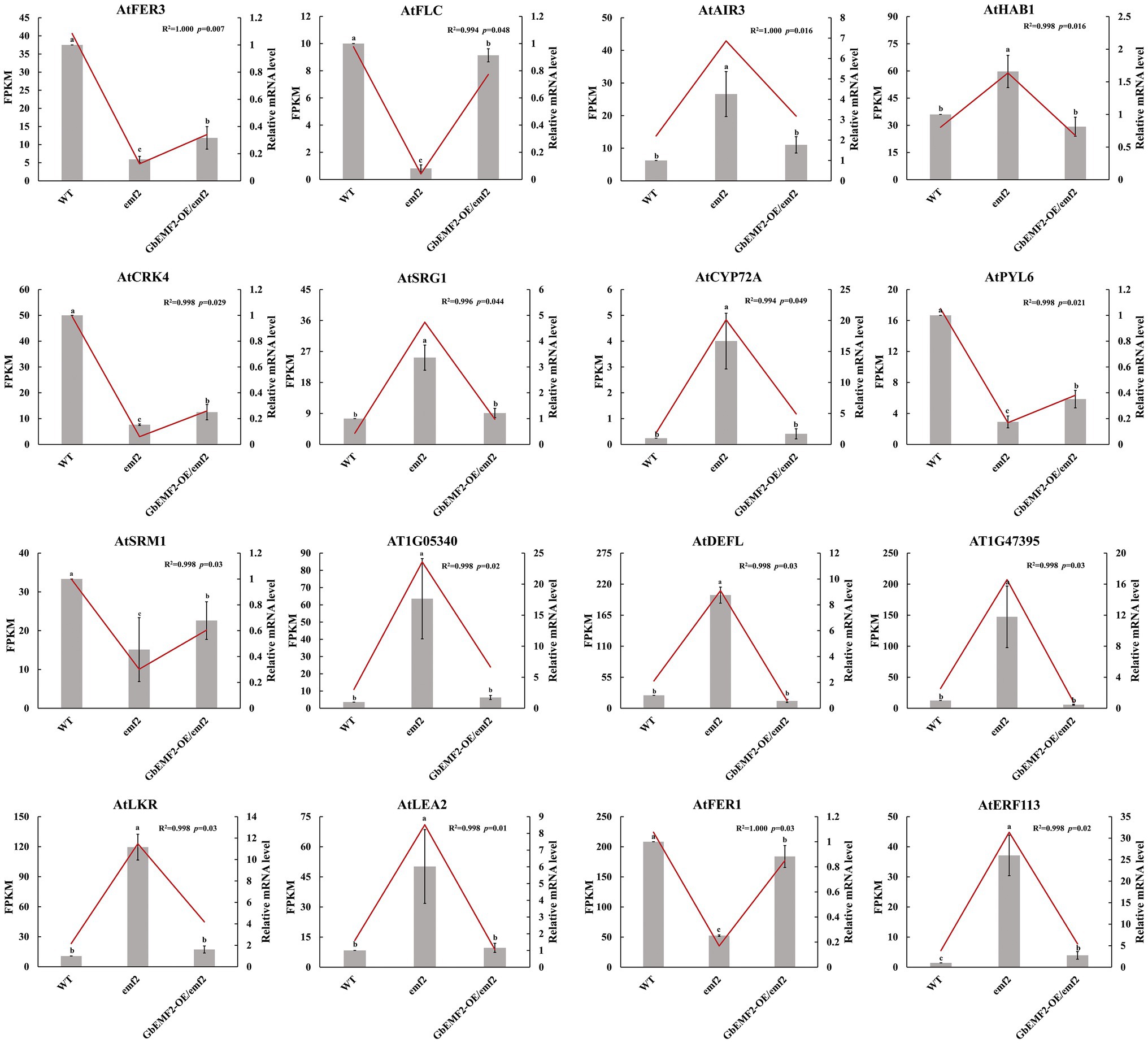
Figure 8. The qRT-PCR validation of the expression patterns of 16 DEGs. Data are mean ± SD of three biological replicates with 10 plants per replicate. Line and block represent RNA-seq and qRT-PCR data. Letters mean significant difference at p ≤ 0.05 by the Duncan’s multiple range tests.
Discussion
The EMF gene plays an important role in maintaining vegetative development and repressing flower development. Together, EMF2 protein shares the VEFS-Box domain with homologous FIS2 and VRN (Yoshida et al., 2001). In this study, we characterized a G. biloba EMBRYONIC FLOWER gene named GbEMF2 that contains a VEFS-Box domain (Figure 1) and belongs to one member of the EMF2 family based on phylogenetic analysis.
GbEMF2 Was Predominately Expressed in Reproductive Organs
The expression patterns of AtEMF2, BoEMF2, and DlEMF2 and the expression pattern in G. biloba orthologue GbEMF2 had similarities and differences. The AtEMF2 gene was expressed in developing embryos, vegetative and reproductive shoot meristems, and lateral organ primordia in A. thaliana (Yoshida et al., 2001). A similar expression pattern can be found in broccoli, in which the expression of flower buds was the highest, followed by leaves, stems and siliques, and roots (Liu et al., 2012). The OsEMF2 gene expression level was the highest in the shoot apical meristem and inflorescence meristem, followed by leaves, roots, immature seeds, and calli in rice (Conrad et al., 2014). Xu et al. (2010) also found that the DlEMF2 gene also was expressed in all bamboo organs. However, the expression level in the shoot organ was higher than that in the inflorescences. In this study, we found that GbEMF2 was expressed in the roots, stems, leaves, microstrobilus, ovulate strobilus, and young fruits of G. biloba. Meanwhile, the expression level in microstrobilus, ovulate strobilus, and young fruits was higher than in roots, stems, and leaves. GbEMF2 was predominantly expressed in reproductive organs, indicating that GbEMF2 may be involved in flowering regulation in G. biloba.
Ectopic Expression of GbEMF2 Led to Delayed Flowering in Transgenic Arabidopsis
To further clarify the biological function of GbEMF2, we expressed it in Arabidopsis driven by the 35S promoter. The flowering time of GbEMF2-OE/emf2 was later than that of the emf2 mutant; however, it was earlier than that of the WT. GbEMF2 restored the phenotype of the emf2 mutant. This result was consistent with the study in broccoli (Liu et al., 2012). The emf2 mutants showed an early flowering phenotype that skips rosette growth and directly produces small inflorescences (Yoshida et al., 2001). The expression of BoEMF2.1 in the Arabidopsis emf2 mutant partially rescued the mutant phenotype by delaying flowering time and increasing the number of rosette leaves. The BoEMF2.1 reduced the expression of flower organ identity genes and changed the flowering time gene in emf2 mutants (Liu et al., 2012). Therefore, GbEMF2-OE/emf2 experiments verified that GbEMF2 had the function of repressing plant flowering.
GbEMF2 Regulating Flowering-Related DEGs Could Result in Later Flowering in GbEMF2 Transgenic Arabidopsis
The MADS-box gene family has been widely characterized in many woody plants, including Gossypium hirsutum (Nardeli et al., 2018), Populus trichocarpa (Leseberg et al., 2006), and G. biloba (Yang et al., 2016). MADS genes function in floral development and regulate flowering time. For example, the FLC gene represses the floral integrator genes SOC1 and FT to control flowering (Helliwell et al., 2006). In addition, MAF represses flowering by directly activating TEM1 (Huang et al., 2019). In the present study, FLC, MAF5, and MAF5-1 were downregulated and upregulated in WT_vs_emf2 and emf2_vs_GbEMF2-OE/emf2. ANR1 was upregulated and downregulated in WT_vs_emf2 and emf2_vs_GbEMF2-OE/emf2. These results indicated that these genes might be regulated by GbEMF2, resulting in affecting flower development. We proposed a hypothetical network model for the GbEMF2 gene by regulating FLC, MAF5, ANR1, and MAF5-1 to control flowering (Figure 9).
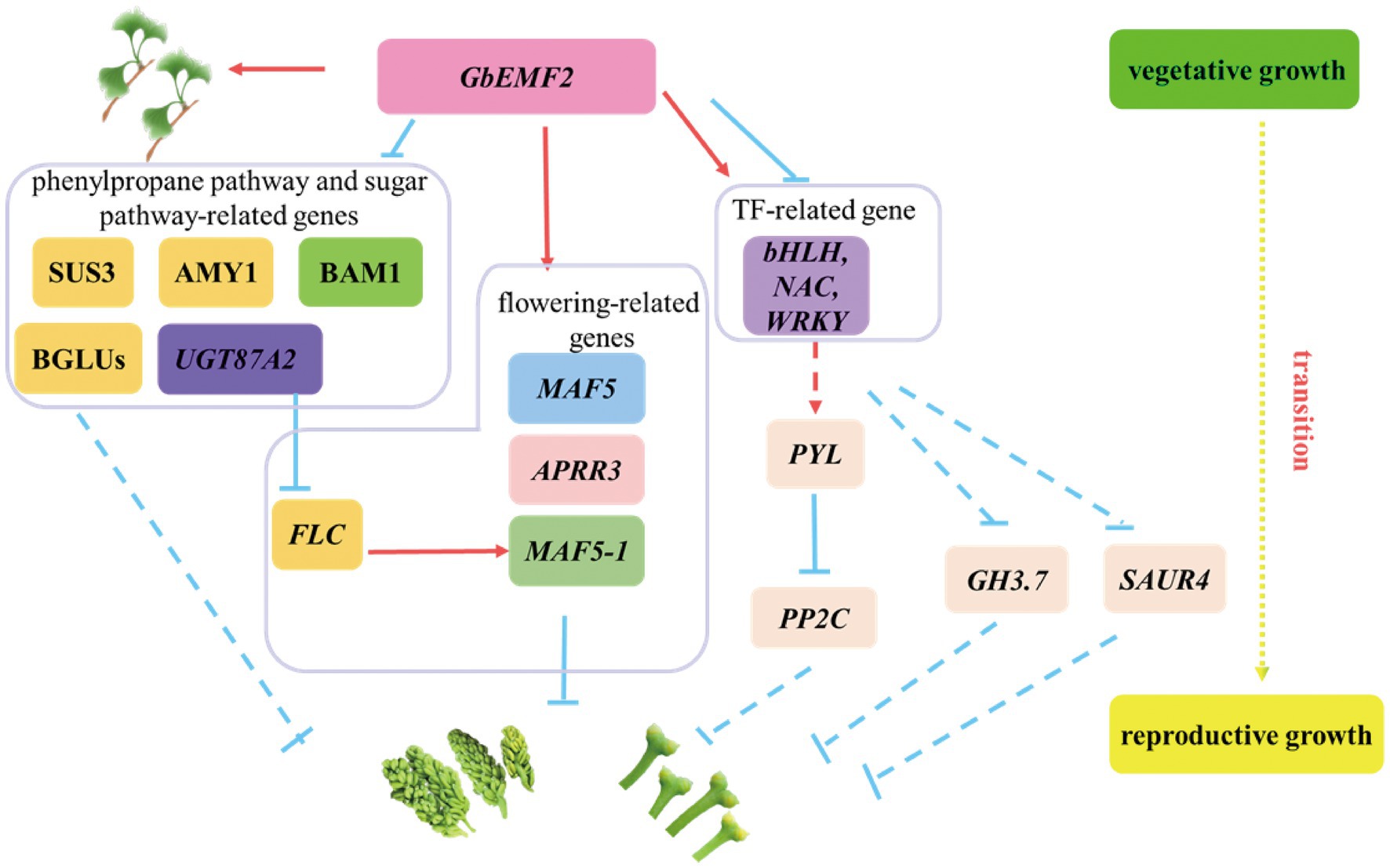
Figure 9. A hypothetical model for the GbEMF2 regulated other genes controlling flowering in G. biloba. Red arrow represents promoting. The inhibition is represented by the blue inverted T line. The solid lines represent by verified in the literature, and the dashed lines represent speculations.
GbEMF2 Regulating Phenylpropane Pathway and Sugar Pathway-Related DEGs Could Result in Later Flowering in GbEMF2 Transgenic Arabidopsis
The phenylpropane biosynthesis pathway and sugar pathway were very important secondary metabolic pathways in plants. For example, downregulation of genes in phenylpropane pathway and sugar pathway may ultimately stunt isonuclear alloplasmic male sterility in wheat (Liu et al., 2020). Cinnamoyl-CoA reductase 2 (CCR2), β-glucosidase genes, and peroxidase genes were involved in phenylpropane biosynthesis pathway (Cheng et al., 2012; Li et al., 2018). Moreover, pollination changes the expression of β-glucosidase gene 15 (BGLU15) and peroxidase gene 47 (PER47) to improve the quality of the pears (Li et al., 2018). In our study, we found GbEMF2 changed the expression of CCR2, BGLU23, BGLU25, BGLU30, PER47, and PER70 to regulate flower. Furthermore, BGLU23, BGLU25, and BGLU30 were annotated sugar pathway. Sugar functions as a source of energy and florigenic signal in plants. The flower induction was mediated by sugar and hormone pathways in apples (Xing et al., 2015). The transcript levels of the SUS3 gene were the lowest in Arabidopsis flower and the highest in siliques (Fallahi et al., 2008). In our study, we found that the transcript levels of the AtSUS3 gene were the lowest in the WT plant, and highest in the emf2 mutant; these levels were intermediate in GbEMF2-OE/emf2 plants. Therefore, we speculated that GbEMF2 controlled flowering by regulating the expression of the SUS3 gene. BAM1 and BAM2 genes are involved in cell division and the differentiation of flowering development (Hord et al., 2006). Additionally, CIK genes interact with BAM1/2 genes and RPK2 genes to regulate flowering development in Arabidopsis (Cui et al., 2018). The AMY1 gene is not only involved in starch metabolism but also suppresses the expression of CO and FT. Meanwhile, the expression of the AMY1 gene was induced by GA and ABA (Jie et al., 2009). The BAM1 and AMY1 genes were significantly expressed in emf2_vs_GbEMF2-OE/emf2. Therefore, the two genes may be regulated by the GbEMF2 gene. Given the joint analysis of our data and flowering-related genes, we speculated that GbEMF2 may control the flowering process by regulating the expression of SUS3, BAM1, and AMY1 (Figure 9).
GbEMF2 Regulating TF-Related DEGs Could Result in Later Flowering in GbEMF2 Transgenic Arabidopsis
The TFs, including MYB, bHLH, NAC, and WRKY, play essential roles in the reproductive development of plants. The MYB genes are potentially involved in flower development in Rafflesia cantleyi (Amini et al., 2019). Some genes of bHLH families act as flower developmental regulators that control flowering time (Ito et al., 2012). In our study, AtbHLH47, AtbHLH101, and AtbHLH167 were upregulated in WT_vs_emf2 and downregulated in emf2_vs_GbEMF2-OE/emf2; no significant difference was found in WT_vs_GbEMF2-OE/emf2 (Table 1), which showed that GbEMF2 might regulate bHLH genes to control flower. Moreover, losing NAC transcription factors, including ANAC050, ANAC052, and ANAC075, led to early flowering phenotype (Fujiwara and Mitsuda, 2016). In this study, the expression of NAC003 changed significantly in three groups. The expression of NAC047 exhibited no significant difference in WT_vs_GbEMF2-OE/emf2, but showed a significant difference between emf2 and GbEMF2-OE/emf2, which implied that GbEMF2 regulated flower development by influencing NAC. In addition, WRKY regulates flowering time in different ways. For instance, an opposite changing trend was exhibited between WRKY12 and WRKY13. The WRKY12 positively regulates flowering time under short-day, whereas WRKY13 serves as a negative regulator (Li et al., 2016b). Thus, we speculated that GbEMF2 might regulate flowering by MYB, bHLH, NAC, and WRKY. Overall, based on these results, a hypothetical network model was proposed for the GbEMF2 gene to regulate TFs further influencing flowering (Figure 9).
Conclusion
In summary, the present study cloned and characterized GbEMF2 from G. biloba. The GbEMF2 protein contains a conserved VEFS-Box domain, which was homologous with VRN2 and FIS2 proteins. The expression level of GbEMF2 in reproductive organs was significantly higher than that in vegetative organs. Overexpressing the GbEMF2 in transgenic Arabidopsis plants, the flowering time of ectopic was later than that of emf2 mutant plants but was earlier than that of the WT plant. In addition, GbEMF2 overexpression in transgenic plants changed the expression levels of flowering-related DEGs, sugar-related DEGs, and TF-related DEGs. Based on our results, we speculated that GbEMF2 may regulate the flowering of G. biloba by regulating MADS genes, BAM1, AMY1, or interacting with NAC, BHLH, and WRKY transcription factors. Our study provides a foundation for understanding GbEMF2, which is involved in the flowering of G. biloba.
Data Availability Statement
The raw sequence data reported in this paper have been deposited in the Genome Sequence Archive (Wang et al., 2017) in National Genomics Data Center (CNCB-NGDC Members and Partners, 2021), China National Center for Bioinformation/Beijing Institute of Genomics, Chinese Academy of Sciences, under accession number CRA003802 that are publicly accessible at https://bigd.big.ac.cn.
Author Contributions
FX designed the whole experiment and drafted the manuscript. XZ performed the part experiment and wrote the manuscript. JYa contributed to cDNA cloning and qRT-PCR analysis. LW and XZ performed the transgenic experiment. XL, WZ, and YL guided the experiment. JYe, SC, and GW revised the manuscript. All authors contributed to the article and approved the submitted version.
Funding
This study was supported by the National Natural Science Foundation of China (No. 31670608).
Conflict of Interest
The authors declare that the research was conducted in the absence of any commercial or financial relationships that could be construed as a potential conflict of interest.
Supplementary Material
The Supplementary Material for this article can be found online at: https://www.frontiersin.org/articles/10.3389/fpls.2021.681166/full#supplementary-material
Footnotes
1. ^https://sg.idtdna.com/PrimerQuest/Home/Index
2. ^https://blast.ncbi.nlm.nih.gov/
3. ^https://www.lynnon.com/dnaman.html
4. ^http://downloads.fyxm.net/Clustal-X-58923.html
5. ^https://www.megasoftware.net/
6. ^https://ccb.jhu.edu/software/stringtie/index.shtml
7. ^ftp://ftp.ncbi.nih.gov/blast/db/
9. ^http://www.geneontology.org/
10. ^http://www.ncbi.nlm.nih.gov/COG/
References
Amini, S., Rosli, K., Abu-Bakar, M. F., Alias, H., Mat-Isa, M. N., Juhari, M. A., et al. (2019). Transcriptome landscape of Rafflesia cantleyi floral buds reveals insights into the roles of transcription factors and phytohormones in flower development. PLoS One 14:e0226338. doi: 10.1371/journal.pone.0226338
Aubert, D., Chen, L., Moon, Y. H., Martin, D., Castle, L. A., Yang, C. H., et al. (2001). EMF1, a novel protein involved in the control of shoot architecture and flowering in Arabidopsis. Plant Cell 13, 1865–1875. doi: 10.1105/TPC.010094
Boss, P. K., Bastow, R. M., Mylne, J. S., and Dean, C. (2004). Multiple pathways in the decision to flower: enabling, promoting, and resetting. Plant Cell 16, S18–S31. doi: 10.1105/tpc.015958
Cai, Y., Chen, L., Liu, X., Guo, C., Sun, S., Wu, C., et al. (2018). CRISPR/Cas9-mediated targeted mutagenesis of GmFT2a delays flowering time in soya bean. Plant Biotechnol. J. 16, 176–185. doi: 10.1111/pbi.12758
Chanvivattana, Y., Bishopp, A., Schubert, D., Stock, C., Moon, Y. H., Sung, Z. R., et al. (2004). Interaction of polycomb-group proteins controlling flowering in Arabidopsis. Development 131, 5263–5276. doi: 10.1242/dev.01400
Charrier, A., Vergne, E., Dousset, N., Richer, A., Petiteau, A., and Chevreau, E. (2019). Efficient targeted mutagenesis in apple and first time edition of pear using the CRISPR-Cas9 system. Front. Plant Sci. 10:40. doi: 10.3389/fpls.2019.00040
Chaudhury, A. M., Ming, L., Miller, C., Craig, S., Dennis, E. S., and Peacock, W. J. (1997). Fertilization-independent seed development in Arabidopsis thaliana. Proc. Natl. Acad. Sci. U. S. A. 94, 4223–4228. doi: 10.1073/pnas.94.8.4223
Chen, L., Cheng, J. C., Castle, L., and Sung, Z. R. (1997). EMF genes regulate Arabidopsis inflorescence development. Plant Cell 9, 2011–2024. doi: 10.1105/tpc.9.11.2011
Cheng, S., Cheng, J., Xu, F., Ye, J., and Wang, X. (2016). Molecular cloning and expression analysis of a putative E class MADS-box gene, GbSEP, from Ginkgo biloba. J. Anim. Plant Sci. 26, 253–260.
Cheng, H., Li, L. L., Cheng, S. Y., Cao, F. L., Xu, F., Wang, Y., et al. (2012). Characterization of a cinnamoyl-CoA reductase gene in Ginkgo biloba: effects on lignification and environmental stresses. Afr. J. Biotechnol. 11, 6780–6794. doi: 10.5897/ajb11.3704
Clough, S. J., and Bent, A. F. (1998). Floral dip: a simplified method for agrobacterium-mediated transformation of Arabidopsis thaliana. Plant J. 16, 735–743. doi: 10.1046/j.1365-313x.1998.00343.x
CNCB-NGDC Members and Partners (2021). Database Resources of the National Genomics Data Center, China National Center for Bioinformation in 2021. Nucleic Acids Res. 49, D18–D28. doi: 10.1093/nar/gkaa1022
Coen, E. S., Romero, J. M., Doyle, S., Elliott, R., Murphy, G., and Carpenter, R. (1990). Floricaula: a homeotic gene required for flower development in antirrhinum majus. Cell 63, 1311–1322. doi: 10.1016/0092-8674(90)90426-f
Conrad, L. J., Khanday, I., Johnson, C., Guiderdoni, E., An, G., Vijayraghavan, U., et al. (2014). The polycomb group gene EMF2B is essential for maintenance of floral meristem determinacy in rice. Plant J. 80, 883–894. doi: 10.1111/tpj.12688
Crane, P. R. (2018). An evolutionary and cultural biography of ginkgo. Plants People Planet 1, 32–37. doi: 10.1002/ppp3.7
Cui, Y., Hu, C., Zhu, Y., Cheng, K., Li, X., Wei, Z., et al. (2018). CIK receptor kinases determine cell fate specification during early anther development in Arabidopsis. Plant Cell 30, 2383–2401. doi: 10.1105/tpc.17.00586
Deng, W., Ying, H., Helliwell, C. A., Taylor, J. M., Peacock, W. J., and Dennis, E. S. (2011). FLOWERING LOCUS C (FLC) regulates development pathways throughout the life cycle of Arabidopsis. Proc. Natl. Acad. Sci. U. S. A. 108, 6680–6685. doi: 10.1073/pnas.1103175108
Eckardt, N. (2006). Functional divergence of AP3 genes in the MADS world of flower development. Plant Cell 18, 1779–1781. doi: 10.1105/tpc.106.045849
Fallahi, H., Scofield, G. N., Badger, M. R., Chow, W. S., Furbank, R. T., and Ruan, Y. L. (2008). Localization of sucrose synthase in developing seed and siliques of Arabidopsis thaliana reveals diverse roles for SUS during development. J. Exp. Bot. 59, 3283–3295. doi: 10.1093/jxb/ern180
Fujiwara, S., and Mitsuda, N. (2016). ANAC075, a putative regulator of vascular-related nac-domain7, is a repressor of flowering. Plant Biotechnol. 33, 255–265. doi: 10.5511/plantbiotechnology.16.0215b
Gendall, A. R., Levy, Y. Y., Wilson, A., and Dean, C. (2001). The VERNALIZATION 2 gene mediates the epigenetic regulation of vernalization in Arabidopsis. Cell 107, 525–535. doi: 10.1016/S0092-8674(01)00573-6
Hanano, S., and Goto, K. (2011). Arabidopsis TERMINAL FLOWER1 is involved in the regulation of flowering time and inflorescence development through transcriptional repression. Plant Cell 23, 3172–3184. doi: 10.1105/tpc.111.088641
Helliwell, C. A., Wood, C. C., Robertson, M., Peacock, W. J., and Dennis, E. S. (2006). The Arabidopsis FLC protein interacts directly in vivo with SOC1 and FT chromatin and is part of a high-molecular-weight protein complex. Plant J. 46, 183–192. doi: 10.1111/j.1365-313X.2006.02686.x
Hord, C. L., Chen, C., Deyoung, B. J., Clark, S. E., and Ma, H. (2006). The BAM1/BAM2 receptor-like kinases are important regulators of Arabidopsis early anther development. Plant Cell 18, 1667–1680. doi: 10.1105/tpc.105.036871
Huang, F., Liu, T., and Hou, X. (2018). Isolation and functional characterization of a floral repressor, BcMAF1, from Pak-choi (Brassica rapa ssp.). Front. Plant Sci. 9:290. doi: 10.3389/fpls.2018.00290
Huang, F., Liu, T., Tang, J., Duan, W., and Hou, X. (2019). BcMAF2 activates BcTEM1 and represses flowering in Pak-choi (Brassica rapa ssp. chinensis). Plant Mol. Biol. 100, 19–32. doi: 10.1007/s11103-019-00867-1
Ito, S., Song, Y. H., Josephson-Day, A. R., Miller, R. J., Breton, G., Olmstead, R. G., et al. (2012). FLOWERING BHLH transcriptional activators control expression of the photoperiodic flowering regulator CONSTANS in Arabidopsis. Proc. Natl. Acad. Sci. U. S. A. 109, 3582–3587. doi: 10.1073/pnas.1118876109
Jie, W., Dashi, Y., XinHong, G., and Xuanming, L. (2009). Arabidopsis AMY1 expressions and early flowering mutant phenotype. BMB Rep. 42, 101–105. doi: 10.5483/BMBRep.2009.42.2.101
Kim, D., Langmead, B., and Salzberg, S. L. (2015). HISAT: a fast spliced aligner with low memory requirements. Nat. Methods 12, 357–360. doi: 10.1038/nmeth.3317
Kong, X., Luo, L., Zhao, J., Chen, Q., Chang, G., Huang, J., et al. (2019). Expression of FRIGIDA in root inhibits flowering in Arabidopsis thaliana. J. Exp. Bot. 70, 5101–5114. doi: 10.1093/jxb/erz287
Krizek, B. A., and Fletcher, J. C. (2005). Molecular mechanisms of flower development: an armchair guide. Nat. Rev. Genet. 6, 688–698. doi: 10.1038/nrg1675
Leseberg, C. H., Li, A., Kang, H., Duvall, M., and Mao, L. (2006). Genome-wide analysis of the MADS-box gene family in Populus trichocarpa. Gene 378, 84–94. doi: 10.1016/j.gene.2006.05.022
Li, M., An, F., Li, W., Ma, M., Feng, Y., Zhang, X., et al. (2016a). DELLA proteins interact with FLC to repress flowering transition. J. Integr. Plant Biol. 58, 642–655. doi: 10.1111/jipb.12451
Li, S., Su, X., Jin, Q., Li, G., Sun, Y., Abdullah, M., et al. (2018). iTRAQ-based identification of proteins related to lignin synthesis in the pear pollinated with pollen from different varieties. Molecules 23:548. doi: 10.3390/molecules23030548
Li, W., Wang, H., and Yu, D. (2016b). Arabidopsis WRKY transcription factors WRKY12 and WRKY13 oppositely regulate flowering under short-day conditions. Mol. Plant 9, 1492–1503. doi: 10.1016/j.molp.2016.08.003
Li, K., Yang, J., Liu, J., Du, X., Wei, C., Su, W., et al. (2006). Cloning, characterization and tissue-specific expression of a cDNA encoding a novel EMBRYONIC FLOWER 2 gene (OsEMF2) in Oryza sativa. DNA Seq. 17, 74–78. doi: 10.1080/10425170500151961
Liu, M. S., Chen, L. F., Lin, C. H., Lai, Y. M., Huang, J. Y., and Sung, Z. R. (2012). Molecular and functional characterization of broccoli EMBRYONIC FLOWER 2 genes. Plant Cell Physiol. 53, 1217–1231. doi: 10.1093/pcp/pcs063
Liu, Z., Li, S., Li, W., Liu, Q., Zhang, L., and Song, X. (2020). Comparative transcriptome analysis indicates that a core transcriptional network mediates isonuclear alloplasmic male sterility in wheat (Triticum aestivum L.). BMC Plant Biol. 20:10. doi: 10.1186/s12870-019-2196-x
Ma, Q., Qu, Z., Wang, X., Qiao, K., Mangi, N., and Fan, S. (2020). EMBRYONIC FLOWER2B, coming from a stable QTL, represses the floral transition in cotton. Int. J. Biol. Macromol. 163, 1087–1096. doi: 10.1016/j.ijbiomac.2020.07.116
Meng, X., Xu, F., Song, Q., Ye, J., Liao, Y., and Zhang, W. (2018). Isolation, characterization and functional analysis of a novel 3-hydroxy-3-methylglutaryl-coenzyme A synthase gene (GbHMGS2) from Ginkgo biloba. Acta Physiol. Plant. 40:72. doi: 10.1007/s11738-018-2650-7
Mulki, M. A., and von Korff, M. (2016). CONSTANS controls floral repression by up-regulating VERNALIZATION2 (VRN-H2) in barley. Plant Physiol. 170, 325–337. doi: 10.1104/pp.15.01350
Nardeli, S. M., Artico, S., Aoyagi, G. M., de Moura, S. M., da Franca Silva, T., Grossi-de-Sa, M. F., et al. (2018). Genome-wide analysis of the MADS-box gene family in polyploid cotton (Gossypium hirsutum) and in its diploid parental species (Gossypium arboreum and Gossypium raimondii). Plant Physiol. Biochem. 127, 169–184. doi: 10.1016/j.plaphy.2018.03.019
Navarro, C., Abelenda, J. A., Cruz-Oro, E., Cuellar, C. A., Tamaki, S., Silva, J., et al. (2011). Control of flowering and storage organ formation in potato by FLOWERING LOCUS T. Nature 478, 119–122. doi: 10.1038/nature10431
Pertea, M., Pertea, G. M., Antonescu, C. M., Chang, T.-C., Mendell, J. T., and Salzberg, S. L. (2015). StringTie enables improved reconstruction of a transcriptome from RNA-seq reads. Nat. Biotechnol. 33, 290–295. doi: 10.1038/nbt.3122
Schmittgen, T. D., and Livak, K. J. (2008). Analyzing real-time PCR data by the comparative C(T) method. Nat. Protoc. 3, 1101–1108. doi: 10.1038/nprot.2008.73
Sung, Z., Belachew, A., Shunong, B., and Bertrand-Garcia, R. (1992). EMF, an Arabidopsis gene required for vegetative shoot development. Science 258, 1645–1647. doi: 10.1126/science.258.5088.1645
Wang, X., Cheng, J., Xu, F., Li, X., Zhang, W., Liao, Y., et al. (2015). Molecular cloning and expression analysis of a MADS-Box gene (GbMADS2) from Ginkgo biloba. Not. Bot. Horti. Agrobo. 43, 19–24. doi: 10.15835/nbha4319760
Wang, Y., Song, F., Zhu, J., Zhang, S., Yang, Y., Chen, T., et al. (2017). GSA: genome sequence archive. Genomics Proteomics Bioinformatics 15, 14–18. doi: 10.1016/j.gpb.2017.01.001
Wang, L., Yan, J., Zhou, X., Cheng, S., Chen, Z., Song, Q., et al. (2019). GbFT, a FLOWERING LOCUS T homolog from Ginkgo biloba, promotes flowering in transgenic Arabidopsis. Sci. Hortic. 247, 205–215. doi: 10.1016/j.scienta.2018.12.020
Weigel, D., Alvarez, J., Smyth, D., Yanofsky, M., and Meyerowitz, E. (1992). LEAFY controls floral meristem identity in Arabidopsis. Cell 69, 843–859. doi: 10.1016/0092-8674(92)90295-N
Wickland, D. P., and Hanzawa, Y. (2015). The FLOWERING LOCUS T/TERMINAL FLOWER 1 gene family: functional evolution and molecular mechanisms. Mol. Plant 8, 983–997. doi: 10.1016/j.molp.2015.01.007
Wu, Z., Ietswaart, R., Liu, F., Yang, H., Howard, M., and Dean, C. (2016). Quantitative regulation of FLC via coordinated transcriptional initiation and elongation. Proc. Natl. Acad. Sci. U. S. A. 113, 218–223. doi: 10.1073/pnas.1518369112
Xing, L. B., Zhang, D., Li, Y. M., Shen, Y. W., Zhao, C. P., Ma, J. J., et al. (2015). Transcription profiles reveal sugar and hormone signaling pathways mediating flower induction in apple (Malus domestica Borkh.). Plant Cell Physiol. 56, 2052–2068. doi: 10.1093/pcp/pcv124
Xu, H., Chen, L. J., Qu, L. J., Gu, H. Y., and Li, D. Z. (2010). Functional conservation of the plant EMBRYONIC FLOWER2 gene between bamboo and Arabidopsis. Biotechnol. Lett. 32, 1961–1968. doi: 10.1007/s10529-010-0362-1
Yan, J., Mao, D., Liu, X., Wang, L., Xu, F., Wang, G., et al. (2017). Isolation and functional characterization of a circadian-regulated CONSTANS homolog (GbCO) from Ginkgo biloba. Plant Cell Rep. 36, 1387–1399. doi: 10.1007/s00299-017-2162-8
Yang, C., Chen, L., and Sung, Z. (1995). Genetic regulation of shoot development in Arabidopsis: role of the EMF genes. Dev. Biol. 169, 421–425. doi: 10.1006/dbio.1995.1158
Yang, F., Xu, F., Wang, X., Liao, Y., Chen, Q., and Meng, X. (2016). Characterization and functional analysis of a MADS-box transcription factor gene (GbMADS9) from Ginkgo biloba. Sci. Hortic. 212, 104–114. doi: 10.1016/j.scienta.2016.09.042
Ye, J., Cheng, S., Zhou, X., Chen, Z., Kim, S. U., Tan, J., et al. (2019). A global survey of full-length transcriptome of Ginkgo biloba reveals transcript variants involved in flavonoid biosynthesis. Ind. Crop. Prod. 139:111547. doi: 10.1016/j.indcrop.2019.111547
Yoshida, N., Yanai, Y., Chen, L., Kato, Y., Hiratsuka, J., Miwa, T., et al. (2001). EMBRYONIC FLOWER2, a novel polycomb group protein homolog, mediates shoot development and flowering in Arabidopsis. Plant Cell 13, 2471–2481. doi: 10.1105/tpc.010227
Keywords: Ginkgo biloba, GbEMF2, flowering, emf2 mutant, RNA-seq
Citation: Zhou X, Wang L, Yan J, Ye J, Cheng S, Xu F, Wang G, Zhang W, Liao Y and Liu X (2021) Functional Characterization of the EMBRYONIC FLOWER 2 Gene Involved in Flowering in Ginkgo biloba. Front. Plant Sci. 12:681166. doi: 10.3389/fpls.2021.681166
Edited by:
Songling Bai, Zhejiang University, ChinaReviewed by:
Dong Meng, Cornell University, United StatesQing Yang, Beijing Forestry University, China
Copyright © 2021 Zhou, Wang, Yan, Ye, Cheng, Xu, Wang, Zhang, Liao and Liu. This is an open-access article distributed under the terms of the Creative Commons Attribution License (CC BY). The use, distribution or reproduction in other forums is permitted, provided the original author(s) and the copyright owner(s) are credited and that the original publication in this journal is cited, in accordance with accepted academic practice. No use, distribution or reproduction is permitted which does not comply with these terms.
*Correspondence: Feng Xu, eHVmZW5nQHlhbmd0emV1LmVkdS5jbg==