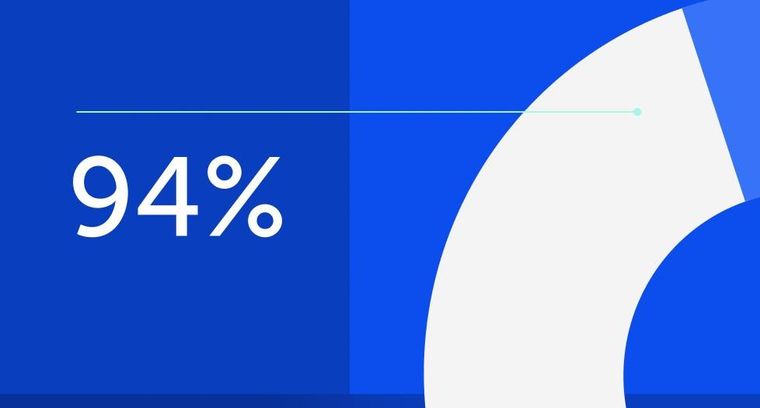
94% of researchers rate our articles as excellent or good
Learn more about the work of our research integrity team to safeguard the quality of each article we publish.
Find out more
ORIGINAL RESEARCH article
Front. Plant Sci., 07 June 2021
Sec. Plant Symbiotic Interactions
Volume 12 - 2021 | https://doi.org/10.3389/fpls.2021.679463
This article is part of the Research TopicMaximizing Nitrogen Fixation in Legumes as a Tool for Sustainable Agriculture IntensificationView all 17 articles
Plants MADS-domain/AGL proteins constitute a large transcription factor (TF) family that controls the development of almost every plant organ. We performed a phylogeny of (ca. 500) MADS-domain proteins from Arabidopsis and four legume species. We identified clades with Arabidopsis MADS-domain proteins known to participate in root development that grouped legume MADS-proteins with similar high expression in roots and nodules. In this work, we analyzed the role of AGL transcription factors in the common bean (Phaseolus vulgaris) – Rhizobium etli N-fixing symbiosis. Sixteen P. vulgaris AGL genes (PvAGL), out of 93 family members, are expressed – at different levels – in roots and nodules. From there, we selected the PvAGL gene denominated PvFUL-like for overexpression or silencing in composite plants, with transgenic roots and nodules, that were used for phenotypic analysis upon inoculation with Rhizobium etli. Because of sequence identity in the DNA sequence used for RNAi-FUL-like construct, roots, and nodules expressing this construct -referred to as RNAi_AGL- showed lower expression of other five PvAGL genes highly expressed in roots/nodules. Contrasting with PvFUL-like overexpressing plants, rhizobia-inoculated plants expressing the RNAi_AGL silencing construct presented affection in the generation and growth of transgenic roots from composite plants, both under non-inoculated or rhizobia-inoculated condition. Furthermore, the rhizobia-inoculated plants showed decreased rhizobial infection concomitant with the lower expression level of early symbiotic genes and increased number of small, ineffective nodules that indicate an alteration in the autoregulation of the nodulation symbiotic process. We propose that the positive effects of PvAGL TF in the rhizobia symbiotic processes result from its potential interplay with NIN, the master symbiotic TF regulator, that showed a CArG-box consensus DNA sequence recognized for DNA binding of AGL TF and presented an increased or decreased expression level in roots from non-inoculated plants transformed with OE_FUL or RNAi_AGL construct, respectively. Our work contributes to defining novel transcriptional regulators for the common bean – rhizobia N-fixing symbiosis, a relevant process for sustainable agriculture.
Transcription factors (TFs) are master control proteins in all living cells, regulating gene expression in response to different stimuli. These exhibit one or more sequence-specific DNA-binding domains that bind to the promoter and/or enhancer regions of multiple target genes to activate or repress transcription. In this way, TF regulates essential biological processes such as development, growth, metabolism, cell cycle progression, and responses to the environment (Czechowski et al., 2004; Libault et al., 2009). An average of 5.7% plant genes code for TF has been distributed among 62 gene families (Libault et al., 2009).
The MADS-domain proteins constitute a eukaryotic family of TFs involved in various relevant functions. Its name derived from the initials of the four founding members of this family MINICHROMOSOME MAINTENANCE 1 (MCM1) from Saccharomyces cerevisiae, which regulates mating type-specific genes, AGAMOUS (AG) from Arabidopsis thaliana (hereafter referred to as Arabidopsis) and DEFICIENS (DEF) form Antirrhinum majus, which act as homeotic factors that control flower development, and SERUM RESPONSE FACTOR (SRF) from Homo sapiens which controls serum inducible and muscle-specific gene expression (Schwarz-Sommer et al., 1990; Yanofsky et al., 1990; Pollock and Treisman, 1991; Mead et al., 2002). MADS TF recognizes a consensus DNA sequence called the CArG-box [CC(A/T)6GG] (Riechmann et al., 1996).
In plants, the MADS-domain TFs family has expanded largely with 109 members in Arabidopsis, compared with just a few in mammals, Drosophila melanogaster, Saccharomyces cerevisiae, and Caenorhabditis elegans (Olson et al., 1995; Parenicova et al., 2003). This family of TFs can be divided into two main lineages, type I and type II, based on their protein structure. The best-studied MADS TFs from Arabidopsis and other plant species belong to the type II lineage. Plants type II MADS proteins have a modular domain structure, referred to as the MIKC structure, that contains the N-terminal located (~ 60 amino acids) conserved DNA-binding domain (M), followed by moderately conserved intervening (I) and keratin-like (K) domains, and a variable C-terminal domain (C) that, together with K, have roles for protein complex formation (Alvarez-Buylla et al., 2000b; Smaczniak et al., 2012). These MADS proteins include the regulators of floral transition and floral organ identity determination that, after over 20 years of intensive study, led to the establishment of a general model for flower organ identity in higher plants, the so-called ABCDE model in which floral whorl-specific combinations of class A, B, C, D, or E genes specify floral organ identity (Alvarez-Buylla et al., 2000b; Murai, 2013).
Although initially, MADS-box genes were relevant for floral organ speciation, more recent studies have revealed that these genes are relevant for the morphogenesis of almost all plant organs. The role of MADS TF in root development has received relatively less attention, but recently it was comprehensively reviewed by Alvarez-Buylla et al. (2019). About 41 Arabidopsis MADS-box genes that show high and intermediate expression levels in different root tissues and zones have been identified (Alvarez-Buylla et al., 2019). Arabidopsis research using genetic, molecular, biochemical approaches has contributed to understanding the role of root-expressed MADS-box genes, from different clades, in primary or lateral root development. The latter include XAL1 (XAANTAL1), XAL2 (XAANTAL2), ANR1 (Arabidopsis NITRATE REGULATED1), and AGL21 (AGAMOUS-Like21) (Gan et al., 2005; Garay-Arroyo et al., 2013; Garcia-Cruz et al., 2016). In addition, orthologs of Arabidopsis root MADS-box genes from different plant species show similar expression patterns and potential functional conservation. These include MADS-box genes from rice (Oryza sativa), sweet potato (Ipomoea batatas), and Chrysanthemum morifolium (Ku et al., 2008; Puig et al., 2013; Sun et al., 2018; Alvarez-Buylla et al., 2019). Studies regarding the expression/function of MADS-box genes in legume species are yet scant. XAL-1 and XAL-2 genes have been identified from soybean (Glycine max) and alfalfa (Medicago sativa). These show a high level of expression in the root in soybean, but expression data from alfalfa genes are not available (Alvarez-Buylla et al., 2019). Also, high expression in roots and root hairs has been documented for AGL21 soybean ortholog (Alvarez-Buylla et al., 2019). Previous work from our group identified orthologs of Arabidopsis root MADS-box genes from common bean (Phaseolus vulgaris) and soybean that are expressed in root tissues (Íñiguez et al., 2015).
Legumes can establish two types of endosymbiotic associations, with arbuscular mycorrhiza fungi and nitrogen-fixing bacteria, collectively called rhizobia (Olah et al., 2005; Oldroyd, 2013). These associations facilitate the plant acquisition of nutrients such as phosphorus and nitrogen (Oldroyd, 2013; Venkateshwaran et al., 2013).
An efficient symbiotic association with rhizobia results in the formation of root nodules where rhizobia are allocated and fix atmospheric nitrogen (N2) informs that the legume host can assimilate in exchange for a carbon source. Symbiotic nitrogen fixation (SNF) is ecologically and economically important for sustainable agriculture because it reduces synthetic fertilizers and the cost of legume cultivation (Venkateshwaran et al., 2013; Castro-Guerrero et al., 2016). The infection of rhizobia to the root tissue is a complex process that involves communication between rhizobia and legumes through molecular signals, such as flavonoids released by the plant root to the rhizosphere that is sensed by compatible rhizobia that, in turn, produce the lipochitooligosaccharides, known as nodulation factors (NF). NF perception by the plant triggers a series of molecular responses essential for rhizobial infection and nodule development (Roy et al., 2020). These responses include the rhizobia-induced root hair deformations or curling required to entrap the rhizobia into an infection chamber and initiate the formation of an infection thread -an invasive invagination of the plant cells- and the infection of the cortical root cells (Suzaki et al., 2015). After rhizobia infection, legumes activate intricate signaling pathways that include finely regulated nodulin genes to develop mature nodules with functionally differentiated bacteroids. Research from the last 20 years has identified a suite of ca. 200 legume genes relevant for establishing an effective symbiosis with rhizobia (Roy et al., 2020). The NODULE INCEPTION (NIN) TF gene is a master regulator for the rhizobia symbiosis. It plays an indispensable role in the rhizobial infection and nodule organogenesis symbiotic processes (Schauser et al., 1999; Marsh et al., 2007; Liu and Bisseling, 2020). Recently, it was shown that NIN also plays a major role in the transition from infection into functional nodules, the development of symbiosomes, and nodule senescence (Liu et al., 2021).
Because of the high carbon demand, SNF is an energy-demanding process for the legume host; therefore, the number of infections and the number of nodules per plant is tightly regulated by a systemic feedback regulatory mechanism termed autoregulation of nodulation (AON, Reid et al., 2011; Ferguson et al., 2019). Upon infection, rhizobia induce the root production of CLAVATA3/endosperm surrounding region-related (CLE) peptides, encoded by the RIC1 and RIC2 genes in soybean and common bean (Reid et al., 2011; Ferguson et al., 2014). These CLE peptides are transported from the root to the shoot, where they are detected by the nodule autoregulation receptor kinase (NARK) to activate the generation of a shoot-derived signal (i.e., miR2111 and cytokinin) (Tsikou and Yan, 2018; Ferguson et al., 2019). The shoot signals are transported to the root to inhibit further nodule development through the participation of the negative regulator TML (Too Much Love), a kelch repeat-containing F-box protein (Takahara et al., 2013). By interplay with other regulators, the NIN TF plays a relevant role in the AON process through the transcriptional activation of the CLE peptides (Soyano et al., 2014; Roy et al., 2020).
The information about legume MADS-box genes as regulators of the legume -rhizobia symbiosis is scarce. Three MADS-box genes from alfalfa (Medicago sativa) from the AGL17 subfamily with high expression in nodules were cloned (nmh7, nmhC5, ngl9) (Heard et al., 1997; Zucchero et al., 2001). Expression analysis of the alfalfa NMH7 gene revealed its expression in flowers and nodules and the parenchyma or the root tip and the root elongation zone from seedlings (Páez-Valencia et al., 2008). The soybean GmNMHC5 gene is highly expressed in roots and nodules, and its overexpression promotes lateral root development and nodulation (Liu et al., 2015). Contrastingly, the GmNMH7, MADS-box gene, highly expressed in roots and nodules, exerts negative control to nodulation, probably through regulating the content of gibberellin (Ma et al., 2019).
Common bean (Phaseolus vulgaris) is the most important crop legume for human consumption and the principal source of non-animal protein for human consumption in the developing world (Broughton et al., 2003). Research from our group focused on the common bean – rhizobia symbiosis has contributed to identifying microRNAs/TF target gene nodes that play a relevant role in the regulation of the SNF, such as miR172c/AP2-1 and miR319d/TCP10 (Nova-Franco et al., 2015; Martin-Rodriguez et al., 2018). We have also identified common bean and soybean MADS-box genes expressed in roots, similar to their Arabidopsis orthologs (Íñiguez et al., 2015). This work aimed to analyze the role of MADS-box genes from P. vulgaris, hereafter denominated PvAGL genes, in the common bean – rhizobia symbiosis that, to our knowledge, have not been studied in this legume. We performed a phylogenetic analysis of MADS-box genes for Arabidopsis and four legume species that identified clades, including root-expressed MADS-box genes from Arabidopsis and legumes. Expression analysis revealed that PvAGL genes are highly expressed in both rhizobia-inoculated roots and nodules. The functional analysis of composite Rhizobium etli-inoculated common bean plants with a modulated expression of PvAGL genes revealed the participation of TF from this gene family in rhizobial infection, expression of early symbiotic genes, root architecture, nodulation, nitrogenase activity, and the AON symbiotic process. Our work contributes to the knowledge of the participation of members from the MADS-box/AGL TF family in the legume – rhizobia SNF, a relevant process for sustainable agriculture.
Gene sequences and the RNA-seq gene expression data for the MADS-box TFs genes for each plant species were retrieved from the following databases. For Arabidopsis, The Arabidopsis Information Resource TAIR (https://www.arabidopsis.org); for P. vulgaris the Gene Expression Atlas (GEA), Pv GEA (http://plantgrn.noble.org/PvGEA/) (O'Rourke et al., 2014); for G. max the ePlant BAR page (http://bar.utoronto.ca/eplant_soybean/) (Waese et al., 2017), for Medicago truncatula the MtGEA (https://mtgea.noble.org/v3/) and Lotus japonicus the Lotus Base (https://lotus.au.dk/).
The translated protein sequences from the MADS-box genes were analyzed in Interpro (http://www.ebi.ac.uk/interpro/) to confirm the presence of the characteristic domain IPR002100 from this gene family. Next, the protein sequences were analyzed with blast v2.6.0 and aligned with clustalw v2.1 (Thompson et al., 1994) to confirm that proteins share a stereotypical MIKC structure with four domains characteristic of type II MADS-box genes of the plant (Alvarez-Buylla et al., 2000b; Smaczniak et al., 2012). Finally, the proteins with MIKC structure were re-analyzed by multiple alignments to verify the integrity of the MADS domain (~60 amino acidic region). Some protein sequences from each species were excluded for phylogenetic analysis because these did not have a complete MADS domain sequence. The whole set of 511 MADS protein sequences from Arabidopsis and four legume species used for phylogenetic analysis are listed in the Supplementary Table 1.
The best evolutionary model (JTT+G) was determined with Prottest3 v3.4.2, and a maximum likelihood phylogeny was made with PhyML v3.0 using approximate likelihood-ratio test SH-like as a statistical test for branch support. Finally, the Interactive Tree of Life (iTOL5) (Letunic and Bork, 2019) was used for displaying the phylogenetic tree.
The common bean (P. vulgaris) Mesoamerican cv BAT93 was used in this work (Vlasova et al., 2016). Seeds were surface sterilized in 10% (V/V) commercial sodium hypochlorite for 10 min and finally rinsed three to four times in sterile distilled water. Then seeds were germinated on sterile moistened filter paper at 30°C in darkness for 2 days. Germinated seedlings were planted in pots with wet sterile vermiculite. For SNF conditions, plantlets were inoculated with 1 ml saturated liquid culture of the Rhizobium etli CE3 strain per plant. Plants were grown in growth chambers under controlled environmental conditions (25–28°C, 16 h photoperiod) and were watered every 3 days with N-free B&D nutrient solution (Broughton and Dilworth, 1971). For non-inoculated conditions, a full nutrient B&D solution (5 mM N-content) was used to water the plants. Common bean composite plants with transgenic roots were generated as described below and grown in sterile pots with perlite to prevent harming the transgenic root/nodules when taking these out of the pot at harvest time. The growth conditions of composite plants were similar to those described for wild-type plants.
Total RNA was isolated from frozen tissues collected directly into liquid nitrogen and stored at −80°C. The wet weight used for RNA isolation from each tissue was: 100 mg for nodules detached from roots, 250 mg for roots, and 200 mg for leaves. TrizolTM Reagent (Thermo Fischer Scientific, Inc., Waltham, MA, USA) was used, following the manufacturer's instructions. Total RNA was quantified using the NanoDrop spectrophotometer (Thermo Fischer Scientific, Inc.). For quantification of transcripts levels, total RNA (2 μg) was treated with DNaseI RNase-free (Thermo Fischer Scientific, Inc., Waltham, MA, USA) to remove genomic DNA. First, strand cDNA was synthesized using RevertAid H Minus First Strand cDNA Synthesis Kit (Thermo Fisher Scientific, Inc., Waltham, MA, USA). The resulting cDNAs were then diluted and used to perform qRT-PCR assays using SYBR Green PCR Master Mix (Thermo Fischer Scientific, Inc.), following the manufacturer's instructions. The sequences of oligonucleotide primers used for qRT-PCR of each gene are provided (Supplementary Table 2). Assays were run in 96-well plates using the 7300 Real-Time PCR System and 7300 System Software (Applied Biosystems, Foster City, CA, USA) with settings of 50°C for 2 min, 95°C for 10 min, and 40 cycles of 95°C for 15 s and 57°C for 60 s. Relative expression for each sample was calculated using the “comparative Ct method” and normalized with the geometrical mean of three housekeeping genes (HSP, MDH, and UBQ9) (Vandesompele et al., 2002). Student's t-test was performed to evaluate the significance of the differential expression using the mean values from three biological replicates for each condition, using the GraphPad Prism v8.0 for Windows (GraphPad Software, San Diego, CA, USA).
For overexpression of the Phvul.008G027800.1 P. vulgaris MADS-box gene, hereafter denominated PvFUL-like (Phvul.008G027800) based on its ortholog Arabidospis FUL (FRUITFULL), was PCR-amplified using as template cDNA from common bean roots and the specific primers Up_FUL 5′-CCCTCGAGCTTTTCCACAATTGCC-3′ and Lw_FUL 5′-GCCCGGATCCTAACTAGTAAGTAG-3′. The purified PCR product (767 bp) was cloned into the pTOPO2.1 intermediate vector and confirmed by sequencing. To construct the OE_FUL plasmid, the PvFUL-like DNA region was excised using XhoI/BamHI sites and cloned into the pTDTO plasmid (Aparicio-Fabre et al., 2013). This expression plasmid contains the 35S cauliflower mosaic virus (35SCaMV) promoter and the tdTomato (red fluorescent protein) gene as a visible reporter gene. For silencing PvAGL genes, by RNAi strategy, specific primers flanking a DNA region coding for the MADS-domain from the PvFUL-like gene (Up_RNAi 5′-TCAGCTCAAGCGGATCG-3′ and Lw_RNAi 5′-CACCACGTTCCAAGACATCTT-3′) were used to amplify a 194 bp fragment using as template cDNA from common bean roots. This DNA fragment, that share homology among PvAGL genes highly expressed in roots/nodules, was cloned by the Gateway system into the intermediate vector pENTR and finally in the pTDT-DC-RNAi plasmid (Valdes-Lopez et al., 2008), which also contains the 35S cauliflower mosaic virus (35SCaMV) promoter and the tdT (tandem double Tomato, red fluorescent protein) gene as a visible reporter gene.
The empty vectors pTDTO and pTDT-DC-RNAi, hereafter denominated EV, and the resulting plasmids OE_FUL and RNAi_AGL were introduced by electroporation into Agrobacterium rhizogenes K599, which was then used for plant transformation as described (Estrada-Navarrete et al., 2007) with minor modifications (Aparicio-Fabre et al., 2013). In addition, the presence of red fluorescence from the tdTomato reporter gene was routinely checked in the putative transgenic roots/nodules using a fluorescence stereomicroscope.
DNA sequences from the region upstream of the initiation codon were retrieved from Phytozome v12.1 (https://phytozome.jgi.doe.gov/pz/portal.html). The length of the intergenic 5′-upstream sequences analyzed was 20 kbp or shorter depending on the position of the next contiguous gene. For PvFUL-like gene 7.3-kb sequence was analyzed using the New PLACE tool (A Database of Plant Cis-acting Regulatory Elements; https://www.dna.affrc.go.jp/PLACE/?action=newplace) (Higo et al., 1999) to identify putative cis-regulatory elements related to nodulation or root development; these are shown in Supplementary Figure 2. Upstream sequences of P. vulgaris early symbiotic genes were analyzed using SnapGene Viewer (GSL Biotech LLC, Chicago, IL, USA; available at snapgene.com) to identify the consensus CArG-box sequence that is recognized by AGL TF (Riechmann et al., 1996).
Common bean composite plants, expressing the empty vectors (EV) or OE_FUL/RNAi_AGL plasmids and growing in 25 cm × 25 cm Petri dishes containing nitrogen-free Fahräeus medium (Catoira et al., 2000), were inoculated with 1 mL of saturated Rhizobium etli CE3 culture (OD600 = 1). Five days after inoculation, tdTomato-positive transgenic roots were collected. The root-susceptible zone for rhizobial infection covered around 2 cm was stained with methylene blue for 1 h to maximize contrast and washed three times with double-distilled water. The quantification of the number of rhizobia-induced root hair deformations was determined from 1 cm root segments from the susceptible zone. Deformation events were observed with a bright-field microscope equipped with an 18 MP Digital Camera with Aptina CMOS Sensor (Cientifica Vela Quin, Iztapalapa, Mexico city, Mexico). A total of 20 independent biological replicates were generated, each one including 10 plants.
For root length, root area, and nodule perimeter evaluations, pictures from root or nodule tissues of composite plants (18 or 28 dpi) were processed with SmartRoot software (Lobet et al., 2011).
Determination of the Nase-enzyme activity was determined in nodulated roots (28 dpi) by acetylene reduction assay (ARA) described by Hardy et al. (1968). Specific activity is expressed as nmol ethylene h−1/plant.
For histological analysis, nodules (28 dpi) were collected from composite plants transformed with EV or OE_FUL/RNAi_AGL plasmids and were treated with the procedure described by Reyero-Saavedra et al. (2017). In addition, representative images from safranine-stained sections (25 μm) were taken with a NIKON camera coupled to a bright-field microscope.
The graphs and statistical analysis were made with the R software 3.0.1 or the GraphPad Prism v8.0. The specific statistical tests performed are indicated in the legend of the corresponding figures.
In plants, the MADS-box is a large gene family of TF. The number of family members varies in different plant species. In this work, we identified MADS-box genes for four legume species. For P. vulgaris (common bean), we identified 93 AGL genes, for G. max (soybean) 183, for M. truncatula (Medicago) 143, and L. japonicus (Lotus) 79. For the phylogenetic analysis of MADS TF from Arabidopsis and four legume species, those MADS protein sequences that did not show a complete MADS domain sequence were excluded. Thus, a 511 MADS-domain protein sequences set (Supplementary Table 1) was used for the maximum likelihood phylogenetic tree shown in Figure 1.
Figure 1. Maximum likelihood phylogenetic tree of the AGL-TFs from Phaseolus vulgaris (blue), Glycine max (black), Medicago truncatula (red), Lotus japonicus (green), and Arabidopsis thaliana (orange). Stars highlight those Arabidopsis AGL genes highly expressed in roots that are analyzed in Figure 2 together with legumes' genes from those clades. SH-like branch support values (not shown) were higher than 0.70 in 80% of the phylogeny branches. The nodes including clades A, B, C, D, or E class MADS-domain proteins from Arabidopsis are indicated Support values for these clades were 0.87, 0.84, 0.85, 0.85, and 0.84, respectively.
The majority of clades from the phylogenetic tree (Figure 1) group MADS from Arabidopsis and the four legumes species analyzed, suggesting that MADS TF from the same clade share their tissue expression and their participation in regulatory networks from a certain vegetative tissue. In Figure 1, we indicated seven Arabidopsis root-expressed MADS-box genes from five different clades involved in regulating root development (Alvarez-Buylla et al., 2019). We assessed if the legume MADS-box genes grouped in these monophyletic clades were also expressed in root and if, additionally, these were expressed in root-nodules elicited by rhizobia. The clade from Figure 2A includes ANR1 (ARABIDOPSIS NITRATE REGULATED 1; (Gan et al., 2005) and six legume MADS-box genes with high expression in root tissues, and five of these also showed expression in nodules. The Arabidopsis XAL1 (Garcia-Cruz et al., 2016) clade (Figure 2B) groups five legume genes that showed enhanced expression in roots, and three of these also showed expression in nodules. The clade depicted in Figure 2C includes XAL2 and AGL19 (Alvarez-Buylla et al., 2000a, 2019; Garay-Arroyo et al., 2013) as well as five legume MADS-box genes that were expressed in root and nodules. Figure 2D shows the clade with AGL17/21 genes (Burgeff et al., 2002; Alvarez-Buylla et al., 2019) grouped with five legume genes that showed expression in roots, and four of these were also expressed in nodules. The clade from Figure 2E includes the AGL16 (Nawy et al., 2005) and five legume genes whose expression in roots or nodules was >50%, compared with the expression level from other organs.
Figure 2. Expression profile by tissue of AGL genes from different plant species grouped in clades including Arabidopsis AGLs highly expressed in roots. (A–E) The left side of each panel shows one selected clade (highlighted in Figure 1); the color code used for genes from each species is the same as in Figure 1. The right side of each panel shows the % expression level in each tissue (flower, purple; stem, ocher; leaf, green; pod, beige; seed, black; root, brown and nodule, red) of the corresponding AGL gene. As indicated in the Material and methods section, expression level values were extracted from the Gene Expression Atlas for each species.
Previous work from our group identified the MADS-box genes from common bean and soybean, an ortholog of root-expressed Arabidopsis MADS-box genes that are expressed in roots and nodules (Íñiguez et al., 2015). Our previous report (Íñiguez et al., 2015) and data presented in Figures 1, 2 prompted us to propose that P. vulgaris AGL genes highly expressed in roots/nodules participate as regulators of the common bean - rhizobia symbiosis. In this work, we updated the analysis of PvAGL genes based on the recent annotation of the P. vulgaris genome sequence (www.phytozome.net/commonbean.php) and the identification of the total number of PvAGL genes from this work (Figure 1). RNA-seq data analysis revealed 16 PvAGL genes, out of 93 gene family members, with expression in root and nodule tissues compared with their expression in the stem, leaf, pod, and seed tissues (Supplementary Figure 1). To assess the reliability of the RNA-seq data, we performed qRT-PCR gene expression analysis from root, nodules, and leaves tissues and confirmed that these PvAGL genes are expressed in root/nodules, albeit at different levels (Supplementary Figure 1).
We pursued the expression analysis of six PvAGL genes that showed the highest expression in root/nodule tissues (Supplementary Figure 1) by assaying their transcript levels from these tissues of R. elti-inoculated plants as well as from root of non-inoculated plants at different developmental stages. According to our phylogenetic analysis, the name we assigned to each PvAGL gene corresponds to its Arabidopsis ortholog (Figure 1). Figure 3 shows expression level values for PvAGL16-like, PvSVP-like (SHORT VEGETATIVE PHASE), PvXAL1-like, PvSOC1-like (SUPPRESSOR OF OVEREXPRESSION OF CONSTANS 1), PvAGL24-like, and PvFUL-like (FRUITFULL). The six genes analyzed showed significantly lower expression in young roots compared with increased levels in roots from later developmental stages, except for PvXAL1-like that showed high expression in 12-h roots (Figure 3). These genes were also expressed in immature (10–15 dpi), mature (22 dpi), and senescent (35 dpi) nodules, albeit at a lower level than in non-inoculated or inoculated roots, except for PvFUL-like (Figure 3).
Figure 3. Expression analysis of P. vulgaris AGL genes highly expressed in root/nodules. Expression levels were determined by qRT-PCR in roots or nodules harvested at the indicated time points, corresponding to days post-inoculation for R. etli-inoculated plants or days after planting for non-inoculated plants. NR: non-inoculated roots, black histograms. IR: inoculated roots, brown histograms. N. nodules, red histograms. Expression level refers to gene expression, based on Ct value, normalized with the expression of the housekeeping genes. The name assigned to each P. vulgaris AGL gene corresponded to its Arabidopsis ortholog. The gene IDs are shown in Supplementary Figure 1. Values from each time point for each gene were statistically analyzed by Student's t-test, in the case of two samples, or one-way analysis of variance (ANOVA), in the case of three samples (p-value < 0.001). Different lower-case letters indicate statistically different groups.
Interestingly, the transcript level of the PvFUL-like gene in rhizobia-inoculated plants was similar in roots compared with immature nodules and was higher in mature nodules compared with roots. In contrast, at nodule senescence, the expression in roots was highly increased (Figure 3). Furthermore, the cis-elements in silico analysis of PvFUL-like gene promoter sequence (Supplementary Figure 2) revealed the presence of the organ-specific elements OSE1ROOTNODULE (Vieweg et al., 2004) and OSE2ROOTNODULE (Fehlberg et al., 2005) with characteristic consensus sequences motifs of the promoters activated in infected cells of root nodules. These were repeated 9 and 18 times, respectively, among the analyzed sequence (Supplementary Figure 2). In addition, one cis-element related to root development, repeated 39 times, was also identified (Supplementary Figure 2).
To further investigate the regulatory role of root/nodule-expressed PvAGL TF genes in the common bean-rhizobia N-fixing symbiosis, we aimed to modulate their expression in common bean composite plants – with transgenic root/nodules and untransformed aerial organs – generated through A. rhizogenes-mediated transformation (Estrada-Navarrete et al., 2007).
The PvFUL-like gene was selected for the over-expression construct (OE_FUL) and the silencing construct (RNAi_AGL) driven by the 35SCaMV promotor. These constructs and the empty control vector (EV) contained the tdTomato (red fluorescent protein) reporter gene (Valdes-Lopez et al., 2008; Aparicio-Fabre et al., 2013). As expected, a very high PvFUL-like transcript level was expressed in transgenic roots and nodules from composite plants transformed with OE_FUL (Supplementary Figure 3). The (194 bp) cDNA fragment from the PvFUL-like gene used for the RNAi construct (Supplementary Figure 4) codes for the MDS-box sequence. Multiple sequence alignment analysis of this PvFUL-like gene sequence with a corresponding sequence of PvAGL genes showed similar sequence identity, ranging from ca. 50–70% (Supplementary Figure 4). Gene expression level from the six PvAGL genes highly expressed in root/nodules (Figure 3) was determined from transgenic roots and nodules expressing the EV or the RNAi constructs (Supplementary Figure 5A). Compared with control EV tissues, the roots and nodules expressing the silencing construct have a significantly decreased expression level of PvFUL-like gene and PvXAL1-like, PvSVP-like, PvSOC1-like and PvAGL16-like, and PvAGL24-like. Gene silencing in nodules was high, showing a very low expression level; generally, the silencing effect in roots was lower than in nodules (Supplementary Figure 5A). In agreement with published GEA data (O'Rourke et al., 2014; Íñiguez et al., 2015) and data from Supplementary Figure 1, we confirmed very low, negligible, expression of other 10 PvAGL genes in EV and RNAi_AGL roots/nodules (Supplementary Figure 5B). The simultaneous silencing of several PvAGL genes in transgenic roots/nodules interpreted as a result of the homology among MADS-box cDNA sequence cloned for the RNAi silencing strategy would avoid functional redundancy among these genes. It may result in highly altered phenotypes of RNAi_AGL plants (see below).
Common bean plantlets transformed with A. rhizogenes bearing the RNAi_AGL developed fewer and shorter hairy roots, emerging from the infection site, instead of those transformed with OE_FUL or EV that showed numerous and longer hairy roots (Figure 4A). In addition, the RNAi-AGL composite plants grown under non-inoculated conditions showed reduced root growth (Figure 4D).
Figure 4. Common bean transgenic roots expressing the RNAi_AGL, EV, or OE_FUL constructs. (A) Emerging transgenic roots, 12 days after A. rhizogenes transformation. (B) Fluorescent image showing transgenic expression of red fluorescent protein (tdT) in roots from R. etli-inoculated composite plants, at 18 dpi. (C) Roots from R. etli-inoculated composite plants at 28 dpi. (D) Roots from non-inoculated composite plants at 7 days after planting. Representative images are shown.
To assess if the negative effect of PvAGL silencing in hairy root formation/growth also affects the common bean symbiosis with rhizobia, we first analyzed rhizobial infection to young transgenic roots inoculated with R. etli CE3. No significant differences in root hair development nor root hair density were observed in control (EV) plants than roots with modulation of PvAGL-gene expression (Supplementary Figure 6). Next, we quantified the root hair deformation upon rhizobia infection (Figure 5). Notably, inoculated roots expressing the RNAi_AGL construct showed significantly fewer effective deformed or curled roots hairs (Figures 5A,B) that form an infection chamber, where rhizobia are trapped would allow the formation of the infection thread (Fournier et al., 2015). In addition, PvAGL-silenced roots showed an increased number of non-effective or spatula-like (Reyero-Saavedra et al., 2017) root hair deformations (Figures 5C,D). The inoculated roots overexpressing the PvFUL-like gene showed a similar number of curled root hair than control EV inoculated roots (Figure 5B).
Figure 5. Response to rhizobial infection of transgenic roots expressing the RNAi _AGL, or OE_FUL construct compared with the control vector (EV). (A) Representative images of characteristic rhizobia-induced root hair deformation in common bean transgenic roots. (B) The number of effective rhizobia-induced root hair deformation in transgenic roots expressing each construct. (C) Representative images of spatula-like deformed root hair observed in RNAi_AGL silenced roots. (D) The number of aberrant rhizobia-induced root hair deformations in transgenic roots expressing each construct. Data shown were obtained from 5-days old seedlings and inoculated with R. etli as described in the Material and Methods section. The number of rhizobia-induced root hair deformation events were quantified per cm/root from the susceptible zone from 20 different common bean roots. The horizontal box side represents the first and third quartile in box plots while the outside whiskers the minimum and maximum values. Different lower-case letters indicate a significant difference according to one-way analysis of variance (ANOVA) (p-value < 0.001).
The fact that the silencing of PvAGL genes negatively affected the rhizobia infection process led us to hypothesize that the PvAGL TFs regulate, either directly or indirectly, the expression of key symbiosis-related genes. To test this hypothesis, we evaluated the expression level of PvENOD93 (EARLY NODULIN 93), PvENOD40 (EARLY NODULIN 40), PvNIN, PvNSP2 (NODULATION SIGNALING PATHWAY 2), PvFNSII (FLAVONE SYNTHASE II), and PvFLOT2 (FLOTILLIN 2) genes from RNAi_AGL, OE_FUL and EV roots inoculated with R. etli for 2 days. The data presented in Figure 6 showed that, compared with gene expression level in EV control roots, every gene evaluated showed reduced transcript level in RNAi-AGL roots but an increased transcript level in OE_FUL roots. Thus, there was a correlation between altered root hair deformation and the expression of early symbiotic genes essential for rhizobial infection (Figures 5, 6). Furthermore, to infer if these P. vulgaris early symbiotic genes could be direct targets of PvAGL transcriptional regulation, we searched for the consensus DNA sequence recognized by AGL TFs, the so-called CArG-box, in their promoter region. As a result, we identified a CArG-box in the promoter region of PvNIN and PvNSP2 (Table 1). We then determined the expression level of these two genes in 2-day-old non-inoculated transgenic roots. Our data indicated that the basal expression level of PvNSP2 was similar in the control (EV) and modulated (RNAi_AGL or OE_FUL roots). However, the gene expression level of PvNIN in non-inoculated control EV roots (0.023 ± 0.003) was significantly decreased in RNAi_AGL roots (0.001 + 7 – 0.0001) while it significantly increased in OE-FUL roots (0.052 ± 0.003). This data supported our hypothesis proposing that PvAGL TF might be implicated in PvNIN regulation.
Figure 6. Expression levels of early nodulation genes upon R. etli infection of transgenic roots expressing the RNAi _AGL or OE_FUL construct compared with EV. The common bean early nodulation genes were evaluated at 2 dpi, these were (A) PvENOD93 (Phvul.003G162150), (B) PvENOD40 (Phvul.002G064166), (C) PvNIN (Phvul.009G115800), (D) PvNSP2 (Phvul.009G115800), (E) PvFNSII (Phvul.003G074000), (F) PvFLOT2 (Phvul.009G090700), (G) PvRIC1 (Phvul.005G096900), and (H) PvTML (Phvul.001G09440). Expression levels refer to gene expression, based on Ct value, normalized with the expression of the housekeeping UBC9 gene. Different lower-case letter columns indicate significant difference, according to one-way analysis of variance (ANOVA) (p-value < 0.001). Data showed obtained from three biological replicates, and one technical replicate each from common bean plants.
We assessed if the affection of rhizobia-infection and early symbiotic gene expression in composite plants with modulation in PvAGL-gene expression results in altered root and nodulation phenotype.
An evident decrease in the transgenic roots formed, and the root growth was observed in PvAGL-silenced composite plants (Figures 4B,C). Analysis of root architecture revealed decreased root length, area, and biomass of RNAi_AGL roots compared with control (EV) roots, both at 18 and 28 dpi (Figures 7A–C). In agreement, the foliage/root ratio was increased in RNAi_AGL composite plants (Figure 7D). Contrastingly, plants transformed with OE_FUL showed an adequate formation and root architecture of transgenic roots (Figures 4B,C). In addition, the roots overexpressing PvFUL-like showed increased root length, area, and biomass compared with EV roots at 28 dpi (Figures 7B,C).
Figure 7. Root phenotype of R. etli-inoculated composite plants expressing the RNAi_AGL, or OE_FUL construct compared with EV. (A) Root length, (B) area, (C) dry weight (DW), and (D) foliage DW /root DW ratio were determined at 18 dpi and 28 dpi as indicated. Data shown were obtained from 10 biological replicates per condition. The horizontal box side represents the first and third quartile in box plots while the outside whiskers the minimum and maximum values. Different lower-case letters from each set of values indicate a significant difference according to one-way analysis of variance (ANOVA) (p-value < 0.001).
Regarding the nodule phenotype, we observed that compared with EV plants, RNAi_AGL composite plants formed fewer nodules at 18 dpi, but at a later stage (28 dpi), their nodule number increased (Figure 8A). A high proportion of small nodules were observed in RNAi_AGL transgenic roots at 28 dpi (Figures 8B,C). However, each plant's nodule dry weight per root system was similar among the RNAi_AGL, OE_FUL, and EV plants. Microscopic image of RNAi_AGL nodule sections revealed defective, small nodules with decreased number of infected cells (Figure 9). By contrast, compared with EV plants, OE_FUL forms fewer nodules but with increased size (Figures 8A,B). A high proportion of medium and large size nodules was observed in OE_FUL plants (Figures 8C, 9). The OE_FUL healthy nodules showed a full infected zone (Figure 9).
Figure 8. Nodulation phenotype of R. etli-inoculated composite plants expressing the RNAi_AGL, or OE_FUL construct compared with EV. Nodule parameters were determined at the indicated dpi. (A) Nodule number from the whole root system per plant was obtained from 10 biological replicates per condition. (B) Nodule perimeter was calculated using the ImageJ program from 50 different nodules obtained from three to five plants per condition. (C) Based on their perimeter values, nodules were classified as small (0.2–0.5 cm; red bar), medium (0.51–0.75 cm; brown bar), or large (>0.76; black bar), the percentage of nodules from each category is shown for each condition. (D) Nitrogenase activity was determined at 28 dpi using the acetylene reduction assay (ARA). Data were obtained from 10 biological replicates per condition. Different lower-case letter columns indicate a significant difference according to one-way analysis of variance (ANOVA) (p-value < 0.001).
Figure 9. Mature transgenic nodules expressing the AGL_RNAi or OE_FUL constructs compared with EV. Nodules were excised from transgenic roots at 28 dpi. Left side: representative images of the most abundant type of nodules from each condition. Right side: Transversal sections of mature nodules from each condition stained with safranine and visualized by microscopy. Representative images are shown.
Nodule function was then evaluated by determining nitrogenase activity through ARA. In agreement with their smaller size and fewer infected cells (Figures 8, 9), RNAi_AGL nodules displayed a significantly lower nitrogenase activity compared with that from EV or OE_FUL nodules (Figure 8D).
Knock-out mutants from different legume species, defective in the shoot nodule autoregulation receptor kinase (NARK, in common bean and soybean) present a characteristic hypernodulation or supernodulation phenotype; but the nodules formed, in higher number, are ineffective, white and small (Reid et al., 2011). This phenotype resembles the one observed in composite common bean plants silenced in PvAGL genes (Figures 8, 9). Thus, we analyzed if RNAi_AGL composite plants are altered in the root components of the AON. As shown in Figure 6G, the expression level of the PvRIC1 gene was very low in R. etli-inoculated roots expressing the RNAi_AGL construct. This correlates with the low expression of PvNIN (Figure 6C), known to be the transcriptional regulator of RIC genes (Soyano et al., 2019; Roy et al., 2020). In addition, the TML gene, encoding an AON negative regulator of further nodule formation, also shows diminished expression in RNAi_AGL transgenic roots (Figure 6H). By contrast, the roots overexpressing PvFUL-like formed a lower number of medium or large nodules compared with EV (control) plants (Figures 8, 9) and showed higher expression of PvNIN and PvTML (Figures 6C,H). Furthermore, we identified CArG-box sequences repeated three times in the PvTML promoter region (Table 1); however, the expression level of PvTML in non-inoculated transgenic roots was similar to EV silenced and overexpressing roots. Taken together, these results indicate a positive effect of PvAGL genes in the AON process of the rhizobia N-fixing symbiosis, possibly exerted through the interaction with PvNIN, relevant for the regulation of this process.
In plants, the MADS-box/AGL genes constitute a large TF family with diverse and essential biological functions to regulate the development of almost every plant organ. Phylogenies of plant MADS-box genes have provided information for studies of evolution and developmental genetic pathways (Alvarez-Buylla et al., 2000a; Liljegren et al., 2000). In this work, we present a phylogeny of MADS-box genes from Arabidopsis and the legumes: common bean, soybean, Medicago, and Lotus. Initial research of Arabidopsis MADS-box genes focused on floral development, and it gave rise to the so-called ABCDE model of floral organ specification (Smaczniak et al., 2012). The phylogenetic tree (Figure 1) indicates the clades of Arabidopsis homeotic genes from A, B, C, D, or E classes that group MADS-box genes from the four legumes analyzed. Data from legume GEAs generally indicated a high expression of such legume genes in flowers. For example, the clade, including the class A AP1 gene from Arabidopsis, involved in specifying sepals and petals (Mandel et al., 1992), also includes genes from the four legume species highly expressed in flowers. However, its expression in specific flower tissues, i.e., sepals and petals, has not been documented. This agrees with previous knowledge indicating that Arabidopsis MADS-box genes that form a monophyletic clade share similar tissue expression and likely share regulatory function (Alvarez-Buylla et al., 2000a).
On the other hand, the phylogenetic analysis suggested that the evolution of the MADS-domain family has involved a simultaneous functional diversification in vegetative and reproductive structures (Alvarez-Buylla et al., 2000a; Liljegren et al., 2000). Examples of such diversification were found in the class B genes represented by the AP3 and PISTILLATA (PI) gene clades (Jack et al., 1992; Goto and Meyerowitz, 1994). The legume genes grouped in the AP3 clade include the soybean genes Glyma04G245500 that, according to GEA data, is expressed in flowers and root; and Glyma.06G027200 (NMH7) gene that is highly expressed in roots and nodules (Ma et al., 2019). According to the GEA data, the PI clade includes the Medicago Medtr1g029670 gene that, in addition to flower, is highly expressed in nodules.
Based on data from Arabidopsis and legumes gene expression atlas, we analyzed the expression pattern of seven genes from five clades that include seven Arabidopsis MADS-box genes known to be important regulators of root development (Alvarez-Buylla et al., 2019; Figure 1). These genes are: ANR1 that is a key determinant for developmental root plasticity and has a regulatory role in nutrient response through controlling lateral root elongation in response to nitrate (Gan et al., 2005); XAL1 that plays a principal role during root development, possibly regulating the expression of genes that are components of the cell cycle (Garcia-Cruz et al., 2016); XAL2, another relevant gene for Arabidopsis root development, that controls auxin transport via PIN (Garay-Arroyo et al., 2013; Alvarez-Buylla et al., 2019); AGL19 that is expressed in the columella, lateral root cap and epidermal cells of the meristematic region of the primary and lateral root tips (Alvarez-Buylla et al., 2000a); AGL17 gene that seems to be a lateral root cap marker in the root tip (Burgeff et al., 2002); AGL21, which is highly expressed in lateral root primordia and it has a punctual expression in the primary root meristem (Alvarez-Buylla et al., 2019) and AGL16 gene that is expressed at relatively high levels in the Arabidopsis root quiescent center (Nawy et al., 2005) and shows an intermediate expression level in phloem, xylem and procambium o the root mature zone (Alvarez-Buylla et al., 2019). Evident variations in the expression pattern from seven plant organs were observed among Arabidopsis and the legume MADS-box genes grouped in each clade (Figure 2). Such variations may be related to the different ages/conditions of the different organs from the consulted database from each plant species. For example, data for AGL genes expression in leaf were from 21-day-old common bean leaves, Gm, 28 days old for Medicago, 42 days old for Lotus, and young leaves for Arabidopsis consulted gene expression atlas. However, all the legume MADS-box genes from analyzed clades showed expression in roots, ranging from 9 to 100% compared with the level of expression in other organs. Notably, all the legume genes, except P. vulgaris (Phvul.002G143866, Figure 2D), were also expressed in legume nodules (7 to 74%), developed from roots inoculated with rhizobia.
Recent research in Lotus and Medicago has demonstrated that legumes co-opted a lateral root developmental program, from Arabidopsis, to control nodule organogenesis (Schiessl et al., 2019; Soyano et al., 2019). The case of study in these reports are the legume orthologs of the Arabidopsis ASYMMETRIC LEAVES 2-LIKE 18/LATERAL ORGAN BOUNDARIES DOMAIN 16a (ASL18/LDB16a) gene. In Arabidopsis, the ASL18/LBD16a TF is involved in establishing the asymmetry required for divisions of the pericycle founder cells that produce lateral root primordia (Goh et al., 2012). In legumes, the nodules are lateral root organs that initiate from the inner root layers in response to rhizobial perception. In contrast, lateral roots emerge from predefined founder cells as an adaptive response to external stimuli. Thus, despite differential induction, lateral roots and nodules share developmental programs through ASL18/LBD16a (Schiessl et al., 2019; Soyano et al., 2019; Shahan and Benfey, 2020). Thus, the master regulator for legume symbioses, the NIN TF, is essential for recruiting the lateral root and nodule organogenesis developmental programs. In Medicago, cytokinin-induction of NIN allows induction of this program during nodulation through activation of ASL18/LBD16a that promotes induction of auxin-responsive gene and auxin biosynthesis (Schiessl et al., 2019). In Lotus, NIN regulates the ASL18/LBD16a and NUCLEAR FACTOR-Y (NF-Y) genes that genetically interact during nodule development (Soyano et al., 2019). On this basis, research presented in this work about root/nodules PvAGL TF genes (namely, PvFUL-like, PvXAL1-like, PvAGL24-like, PvSOC1-like, PvSVP-like, and PvAGL16-like) led us to propose that Arabidopsis AGL TF that regulate root development have been co-opted by legumes to control, both, root and nodule development. However, further experimentation is needed to confirm this hypothesis.
The negative effect of PvAGL gene silencing in root development was evident in non-inoculated and R. etli inoculated plants. These roots showed delayed transgenic roots after A. rhizogenes infection and reduced root growth (Figure 4). Furthermore, the inoculated RNAi_AGL plants showed altered root architecture evidenced by diminished root length, area, and biomass (Figures 4, 7). Thus, orthologs of Arabidopsis AGL genes that regulate root development have a similar function in common beans.
The gene silencing of root/nodule PvAGL genes did not affect the root hair development or density (Supplementary Figure 6). However, it negatively affected rhizobial infection of common bean roots, perhaps because due to ineffective chemical communication between the symbionts, something that was evidenced by a significant reduction in the rhizobia-induced effective deformed root hairs that was concomitant with a significant decrease in the expression level of early symbiotic genes essential for rhizobial infection (Figures 5, 6). The genes analyzed include PvFNSII involved in the synthesis of flavones, the chemical signal of the plant sensed by compatible rhizobia, and genes involved in the infection thread initiation and progression (PvFLOT2, PvENOD40, and PvENOD93) that act downstream of the TF NSP2 and NIN (Roy et al., 2020). Thus, the effect of PvAGL TF in the transcriptional regulation of early symbiotic genes (Figure 6) could be direct or indirect. In addition, several other genes are known to be involved in the rhizobial infection process, and infection thread formation and progression (Roy et al., 2020) could be down-regulated in RNAi_AGL roots and would negatively affect initial symbiotic stages. Future experiments based on transcriptomic approaches would provide evidence about this proposition.
Research in different legumes indicates that a delay in the rhizobial infection process results in a defective activation of the nodule organogenesis program (Oldroyd, 2013; Roy et al., 2020). In agreement, a defective nodulation phenotype (Figures 8, 9) was evident in PvAGL-silenced plants affected by a rhizobial infection. The nodule-specific NIN TF is an essential symbiosis regulator expressed in the epidermis and controls rhizobial infection (Schauser et al., 1999; Liu and Bisseling, 2020). NIN is also expressed in the pericycle and is essential for regulating nodule primordia formation in Medicago (Liu et al., 2019). The complex pattern of spatiotemporal regulation exerted by NIN in Medicago requires different upstream cis-regulatory sequences. These include a remote (-18 kb) cis-element with cytokinin-response elements essential for nodule organogenesis (Liu et al., 2019). In addition, recently, it was shown that NIN is expressed in the proximal part of the infection zone in nodules and plays an essential role in the transition from infection to fixation zones for establishing a functional symbiosis (Liu et al., 2021). Our in silico analysis of the PvNIN promoter region revealed a CArG-box cis-element at−9166 bp upstream of the initiation codon (Table 1). It is noteworthy that PvNIN expression was reduced in PvAGL silenced root as opposite to PvFUL-like overexpressing roots both from inoculated and non-inoculated plants, something that supports the hypothesis of its possible direct transcriptional regulation by AGL TF acting as an essential regulator of rhizobial infection and nodule organogenesis in common bean. However, further work is required to identify the P. vulgaris gene targets of PvAGL transcription regulation in common bean roots and nodules. Experimental approaches demonstrate their specific binding to CArG-box present in gene promoter regions.
The observed increased nodule number with altered nodule morphology and function in RNAi_AGL plants (Figures 8, 9) led us to propose that the downregulation of PvAGL genes results in an alteration of the AON symbiotic process. Recently it was shown that gibberellins signaling is a key regulator of the AON process in Lotus (Akamatsu et al., 2020). The endogenous gibberellins from Lotus nodules induce NIN expression via its gibberellin-responsive cis-acting region. NIN directly induces CLE-RS genes (RIC genes in the common bean) to activate the AON process (Soyano et al., 2014; Akamatsu et al., 2020). We showed a significantly low expression of PvRIC1 and PvTML (Figure 6), a negative regulator of AON, in PvAGL-silenced roots/nodules. In addition, we showed that the expression level of PvNIN, with CArG-box cis-elements in its promoter region (Table 1), is up- or down-regulated both in inoculated as well as in non-inoculated roots, with increased or silenced PvAGL expression, respectively. Thus, we propose that PvAGL TF positively regulates PvNIN that, in turn, positively regulates PvRIC1 via gibberellins. The AON is a complex process that includes almost 20 different genes (Roy et al., 2020). Therefore, the down-regulation of the expression of other genes relevant to regulating the AON process in RNAi_AGL roots/nodules could not be excluded. Taken together, our results indicate that the downregulation of PvAGLs in roots/nodules negatively affects the AON resulting in a higher number of small, ineffective nodules formed.
The possible contribution of legume AGL TF as regulators of the rhizobia symbiosis is scarcely documented. In alfalfa, three MADS-box genes expressed in roots and nodules have been reported, though their functional analysis is lacking (Heard et al., 1997; Zucchero et al., 2001; Páez-Valencia et al., 2008). In soybean, one report characterizes the GmNMHC5, MADS-box gene that acts as a positive regulator of root development and nodulation, while the study of GmNMH7 revealed its negative regulation of nodulation (Liu et al., 2015; Ma et al., 2019). Future in-depth studies are needed to find the commonalities and differences among MADS/AGL TF roles from different legumes as regulators of the N-fixing symbiosis.
This is the first report about the participation of PvAGL TF as positive regulators of the common bean – rhizobia symbiosis. The data presented in this study attest to the relevance of PvAGL TF as positive regulators of several processes of the common bean – rhizobia symbioses such as root development, rhizobial infection, nodule organogenesis/function, and the autoregulation of nodulation. Furthermore, we propose that PvAGL control be exerted via interplay with PvNIN, the master symbiosis regulator. Certainly, the knowledge of AGL TF as regulators of N-fixing symbiosis in different legume species would be relevant for a future improvement of this relevant process for ecological and economic reasons.
The original contributions presented in the study are included in the article/Supplementary Material, further inquiries can be directed to the corresponding author.
GH and LA conceived and designed the study. LA, MI-A, MR-S, MR, AL, and S-IF performed the experiments. LA and LL performed in silico analyses. LA, OV-L, LG, and GH adviced experiments and analyzed data. LA and GH wrote the manuscript. All authors contributed to the critical revision of the manuscript, read, and approved the submitted version.
Partial financial support for this work was provided by grants A1-S-22570 to GH and A1-S-9454 to OV-L from CONACyT; IN201320 to OV-L, IN204320 to LG, and IN203819 to GH from DGAPA, UNAM. LA is doctoral student from the Programa de Doctorado en Ciencias Biomédicas - UNAM and she received the Fellowship 582722 form Consejo Nacional de Ciencia y Tecnología and from DGAPA, UNAM (IN203819).
The authors declare that the research was conducted in the absence of any commercial or financial relationships that could be construed as a potential conflict of interest.
We are grateful to Ma. de la Paz Eelizabeth Salas, Víctor Bustos, and Joel Gómez Espíndola for technical assistance and to Damien Formey and Luis P. Íñiguez for advice in data bases and bioinformatic analysis.
The Supplementary Material for this article can be found online at: https://www.frontiersin.org/articles/10.3389/fpls.2021.679463/full#supplementary-material
Akamatsu, A., Nagae, M., Nishimura, Y., Romero Montero, D., Ninomiya, S., Kojima, M., et al. (2020). Endogenous gibberellins affect root nodule symbiosis via transcriptional regulation of NODULE INCEPTION in Lotus japonicus. Plant J. 105, 1507–1520. doi: 10.1111/tpj.15128
Alvarez-Buylla, E. R., Garcia-Ponce, B., Sanchez, M. P., Espinosa-Soto, C., Garcia-Gomez, M. L., Pineyro-Nelson, A., et al. (2019). MADS-box genes underground becoming mainstream: plant root developmental mechanisms. New Phytol. 223, 1143–1158. doi: 10.1111/nph.15793
Alvarez-Buylla, E. R., Liljegren, S. J., Pelaz, S., Gold, S. E., Burgeff, C., Ditta, G. S., et al. (2000a). MADS-box gene evolution beyond flowers: expression in pollen, endosperm, guard cells, roots and trichomes. Plant J. 24, 457–466. doi: 10.1046/j.1365-313x.2000.00891.x
Alvarez-Buylla, E. R., Pelaz, S., Liljegren, S. J., Gold, S. E., Burgeff, C., Ditta, G. S., et al. (2000b). An ancestral MADS-box gene duplication occurred before the divergence of plants and animals. Proc. Natl. Acad. Sci. U. S. A. 97, 5328–5333. doi: 10.1073/pnas.97.10.5328
Aparicio-Fabre, R., Guillen, G., Loredo, M., Arellano, J., Valdes-Lopez, O., Ramirez, M., et al. (2013). Common bean (Phaseolus vulgaris L.) PvTIFY orchestrates global changes in transcript profile response to jasmonate and phosphorus deficiency. BMC Plant Biol. 13:26. doi: 10.1186/1471-2229-13-26
Broughton, W. J., and Dilworth, M. J. (1971). Control of leghaemoglobin synthesis in snake beans. Biochem. J. 125, 1075–1080. doi: 10.1042/bj1251075
Broughton, W. J., Hernández, G., Blair, M., Beebe, S., Gepts, P., and Vanderleyden, J. (2003). Beans (Phaseolus spp.) – model food legumes. Plant Soil 252, 55–128. doi: 10.1023/A:1024146710611
Burgeff, C., Liljegren, S. J., Tapia-Lopez, R., Yanofsky, M. F., and Alvarez-Buylla, E. R. (2002). MADS-box gene expression in lateral primordia, meristems and differentiated tissues of Arabidopsis thaliana roots. Planta 214, 365–372. doi: 10.1007/s004250100637
Castro-Guerrero, N. A., Isidra-Arellano, M. C., Mendoza-Cozatl, D. G., and Valdés-López, O. (2016). Common bean: a legume model on the rise for unraveling responses and adaptations to iron, zinc, and phosphate deficiencies. Front. Plant Sci. 7:600. doi: 10.3389/fpls.2016.00600
Catoira, R., Galera, C., de Billy, F., Penmetsa, R. V., Journet, E. P., Maillet, F., et al. (2000). Four genes of Medicago truncatula controlling components of a nod factor transduction pathway. Plant Cell 12, 1647–1666. doi: 10.1105/tpc.12.9.1647
Czechowski, T., Bari, R. P., Stitt, M., Scheible, W. R., and Udvardi, M. K. (2004). Real-time RT-PCR profiling of over 1400 Arabidopsis transcription factors: unprecedented sensitivity reveals novel root- and shoot-specific genes. Plant J. 38, 366–379. doi: 10.1111/j.1365-313X.2004.02051.x
Estrada-Navarrete, G., Alvarado-Affantranger, X., Olivares, J. E., Guillen, G., Diaz-Camino, C., Campos, F., et al. (2007). Fast, efficient and reproducible genetic transformation of Phaseolus spp. by Agrobacterium rhizogenes. Nat. Protoc. 2, 1819–1824. doi: 10.1038/nprot.2007.259
Fehlberg, V., Vieweg, M. F., Dohmann, E. M., Hohnjec, N., Puhler, A., Perlick, A. M., et al. (2005). The promoter of the leghaemoglobin gene VfLb29: functional analysis and identification of modules necessary for its activation in the infected cells of root nodules and in the arbuscule-containing cells of mycorrhizal roots. J. Exp. Bot. 56, 799–806. doi: 10.1093/jxb/eri074
Ferguson, B. J., Li, D., Hastwell, A. H., Reid, D. E., Li, Y., Jackson, S. A., et al. (2014). The soybean (Glycine max) nodulation-suppressive CLE peptide, GmRIC1, functions interspecifically in common white bean (Phaseolus vulgaris), but not in a supernodulating line mutated in the receptor PvNARK. Plant Biotechnol. J. 12, 1085–1097. doi: 10.1111/pbi.12216
Ferguson, B. J., Mens, C., Hastwell, A. H., Zhang, M., Su, H., Jones, C. H., et al. (2019). Legume nodulation: the host controls the party. Plant Cell Environ. 42, 41–51. doi: 10.1111/pce.13348
Fournier, J., Teillet, A., Chabaud, M., Ivanov, S., Genre, A., Limpens, E., et al. (2015). Remodeling of the infection chamber before infection thread formation reveals a two-step mechanism for rhizobial entry into the host legume root hair. Plant Physiol. 167, 1233–1242. doi: 10.1104/pp.114.253302
Gan, Y., Filleur, S., Rahman, A., Gotensparre, S., and Forde, B. G. (2005). Nutritional regulation of ANR1 and other root-expressed MADS-box genes in Arabidopsis thaliana. Planta 222, 730–742. doi: 10.1007/s00425-005-0020-3
Garay-Arroyo, A., Ortiz-Moreno, E., de la Paz Sanchez, M., Murphy, A. S., Garcia-Ponce, B., Marsch-Martinez, N., et al. (2013). The MADS transcription factor XAL2/AGL14 modulates auxin transport during Arabidopsis root development by regulating PIN expression. EMBO J. 32, 2884–2895. doi: 10.1038/emboj.2013.216
Garcia-Cruz, K. V., Garcia-Ponce, B., Garay-Arroyo, A., Sanchez, M. P., Ugartechea-Chirino, Y., Desvoyes, B., et al. (2016). The MADS-box XAANTAL1 increases proliferation at the Arabidopsis root stem-cell niche and participates in transition to differentiation by regulating cell-cycle components. Ann. Bot. 118, 787–796. doi: 10.1093/aob/mcw126
Goh, T., Joi, S., Mimura, T., and Fukaki, H. (2012). The establishment of asymmetry in Arabidopsis lateral root founder cells is regulated by LBD16/ASL18 and related LBD/ASL proteins. Development 139, 883–893. doi: 10.1242/dev.071928
Goto, K., and Meyerowitz, E. M. (1994). Function and regulation of the Arabidopsis floral homeotic gene PISTILLATA. Genes Dev. 8, 1548–1560. doi: 10.1101/gad.8.13.1548
Hardy, R. W., Holsten, R. D., Jackson, E. K., and Burns, R. C. (1968). The acetylene-ethylene assay for n(2) fixation: laboratory and field evaluation. Plant Physiol. 43, 1185–1207. doi: 10.1104/pp.43.8.1185
Heard, J., Caspi, M., and Dunn, K. (1997). Evolutionary diversity of symbiotically induced nodule MADS box genes: characterization of nmhC5, a member of a novel subfamily. Mol. Plant Microbe Interact. 10, 665–676. doi: 10.1094/MPMI.1997.10.5.665
Higo, K., Ugawa, Y., Iwamoto, M., and Korenaga, T. (1999). Plant cis-acting regulatory DNA elements (PLACE) database: 1999. Nucleic Acids Res. 27, 297–300. doi: 10.1093/nar/27.1.297
Íñiguez, L. P., Nova-Franco, B., and Hernandez, G. (2015). Novel players in the AP2-miR172 regulatory network for common bean nodulation. Plant Signal Behav. 10:e1062957. doi: 10.1080/15592324.2015.1062957
Jack, T., Brockman, L. L., and Meyerowitz, E. M. (1992). The homeotic gene APETALA3 of Arabidopsis thaliana encodes a MADS box and is expressed in petals and stamens. Cell 68, 683–697. doi: 10.1016/0092-8674(92)90144-2
Ku, A. T., Huang, Y. S., Wang, Y. S., Ma, D., and Yeh, K. W. (2008). IbMADS1 (Ipomoea batatas MADS-box 1 gene) is involved in tuberous root initiation in sweet potato (Ipomoea batatas). Ann. Bot. 102, 57–67. doi: 10.1093/aob/mcn067
Letunic, I., and Bork, P. (2019). Interactive Tree Of Life (iTOL) v4: recent updates and new developments. Nucleic Acids Res. 47, W256–W259. doi: 10.1093/nar/gkz239
Libault, M., Joshi, T., Benedito, V. A., Xu, D., Udvardi, M. K., and Stacey, G. (2009). Legume transcription factor genes: what makes legumes so special? Plant Physiol. 151, 991–1001. doi: 10.1104/pp.109.144105
Liljegren, S. J., Ditta, G. S., Eshed, Y., Savidge, B., Bowman, J. L., and Yanofsky, M. F. (2000). SHATTERPROOF MADS-box genes control seed dispersal in Arabidopsis. Nature 404, 766–770. doi: 10.1038/35008089
Liu, J., and Bisseling, T. (2020). Evolution of NIN and NIN-like genes in relation to nodule symbiosis. Genes 11:777. doi: 10.3390/genes11070777
Liu, J., Rasing, M., Zeng, T., Klein, J., Kulikova, O., and Bisseling, T. (2021). NIN is essential for development of symbiosomes, suppression of defence and premature senescence in Medicago truncatula nodules. New Phytol. 230, 290–303. doi: 10.1111/nph.17215
Liu, J., Rutten, L., Limpens, E., van der Molen, T., van Velzen, R., Chen, R., et al. (2019). A remote cis-regulatory region is required for NIN expression in the pericycle to initiate nodule primordium formation in medicago truncatula. Plant Cell 31, 68–83. doi: 10.1105/tpc.18.00478
Liu, W., Han, X., Zhan, G., Zhao, Z., Feng, Y., and Wu, C. (2015). A novel sucrose-regulatory MADS-box transcription factor GmNMHC5 promotes root development and nodulation in soybean (Glycine max [L.] Merr.). Int. J. Mol. Sci. 16, 20657–20673. doi: 10.3390/ijms160920657
Lobet, G., Pages, L., and Draye, X. (2011). A novel image-analysis toolbox enabling quantitative analysis of root system architecture. Plant Physiol. 157, 29–39. doi: 10.1104/pp.111.179895
Ma, W.-y., Liu, W., Hou, W.-s., Sun, S., Jiang, B.-j., Han, T.-f., et al. (2019). GmNMH7, a MADS-box transcription factor, inhibits root development and nodulation of soybean (Glycine max [L.] Merr.). J. Integr. Agric. 18, 553–562. doi: 10.1016/S2095-3119(18)61992-6
Mandel, M. A., Gustafson-Brown, C., Savidge, B., and Yanofsky, M. F. (1992). Molecular characterization of the Arabidopsis floral homeotic gene APETALA1. Nature 360, 273–277. doi: 10.1038/360273a0
Marsh, J. F., Rakocevic, A., Mitra, R. M., Brocard, L., Sun, J., Eschstruth, A., et al. (2007). Medicago truncatula NIN is essential for rhizobial-independent nodule organogenesis induced by autoactive calcium/calmodulin-dependent protein kinase. Plant Physiol. 144, 324–335. doi: 10.1104/pp.106.093021
Martin-Rodriguez, J. A., Leija, A., Formey, D., and Hernandez, G. (2018). The MicroRNA319d/TCP10 node regulates the common bean - rhizobia nitrogen-fixing symbiosis. Front. Plant Sci. 9:1175. doi: 10.3389/fpls.2018.01175
Mead, J., Bruning, A. R., Gill, M. K., Steiner, A. M., Acton, T. B., and Vershon, A. K. (2002). Interactions of the Mcm1 MADS box protein with cofactors that regulate mating in yeast. Mol. Cell Biol. 22, 4607–4621. doi: 10.1128/MCB.22.13.4607-4621.2002
Murai, K. (2013). Homeotic genes and the ABCDE model for floral organ formation in wheat. Plants 2, 379–395. doi: 10.3390/plants2030379
Nawy, T., Lee, J. Y., Colinas, J., Wang, J. Y., Thongrod, S. C., Malamy, J. E., et al. (2005). Transcriptional profile of the Arabidopsis root quiescent center. Plant Cell 17, 1908–1925. doi: 10.1105/tpc.105.031724
Nova-Franco, B., Iniguez, L. P., Valdes-Lopez, O., Alvarado-Affantranger, X., Leija, A., Fuentes, S. I., et al. (2015). The micro-RNA72c-APETALA2-1 node as a key regulator of the common bean-Rhizobium etli nitrogen fixation symbiosis. Plant Physiol. 168, 273–291. doi: 10.1104/pp.114.255547
Olah, B., Briere, C., Becard, G., Denarie, J., and Gough, C. (2005). Nod factors and a diffusible factor from arbuscular mycorrhizal fungi stimulate lateral root formation in Medicago truncatula via the DMI1/DMI2 signalling pathway. Plant J. 44, 195–207. doi: 10.1111/j.1365-313X.2005.02522.x
Oldroyd, G. E. (2013). Speak, friend, and enter: signalling systems that promote beneficial symbiotic associations in plants. Nat. Rev. Microbiol. 11, 252–263. doi: 10.1038/nrmicro2990
Olson, E. N., Perry, M., and Schulz, R. A. (1995). Regulation of muscle differentiation by the MEF2 family of MADS box transcription factors. Dev. Biol. 172, 2–14. doi: 10.1006/dbio.1995.0002
O'Rourke, J. A., Iniguez, L. P., Fu, F., Bucciarelli, B., Miller, S. S., Jackson, S. A., et al. (2014). An RNA-Seq based gene expression atlas of the common bean. BMC Genomics 15:866. doi: 10.1186/1471-2164-15-866
Páez-Valencia, J., Sánchez-Gómez, C., Valencia-Mayoral, P., Contreras-Ramos, A., Hernández-Lucas, I., Orozco-Segovia, A., et al. (2008). Localization of the MADS domain transcriptional factor NMH7 during seed, seedling and nodule development of Medicago sativa. Plant Sci. 175, 596–603. doi: 10.1016/j.plantsci.2008.06.008
Parenicova, L., de Folter, S., Kieffer, M., Horner, D. S., Favalli, C., Busscher, J., et al. (2003). Molecular and phylogenetic analyses of the complete MADS-box transcription factor family in Arabidopsis: new openings to the MADS world. Plant Cell 15, 1538–1551. doi: 10.1105/tpc.011544
Pollock, R., and Treisman, R. (1991). Human SRF-related proteins: DNA-binding properties and potential regulatory targets. Genes Dev. 5, 2327–2341. doi: 10.1101/gad.5.12a.2327
Puig, J., Meynard, D., Khong, G. N., Pauluzzi, G., Guiderdoni, E., and Gantet, P. (2013). Analysis of the expression of the AGL17-like clade of MADS-box transcription factors in rice. Gene Expr. Patterns 13, 160–170. doi: 10.1016/j.gep.2013.02.004
Reid, D. E., Ferguson, B. J., Hayashi, S., Lin, Y. H., and Gresshoff, P. M. (2011). Molecular mechanisms controlling legume autoregulation of nodulation. Ann. Bot. 108, 789–795. doi: 10.1093/aob/mcr205
Reyero-Saavedra, M. D. R., Qiao, Z., Sanchez-Correa, M. D. S., Diaz-Pineda, M. E., Reyes, J. L., Covarrubias, A. A., et al. (2017). Gene silencing of Argonaute5 negatively affects the establishment of the legume-rhizobia symbiosis. Genes 8:352. doi: 10.3390/genes8120352
Riechmann, J. L., Wang, M., and Meyerowitz, E. M. (1996). DNA-binding properties of Arabidopsis MADS domain homeotic proteins APETALA1, APETALA3, PISTILLATA and AGAMOUS. Nucleic Acids Res. 24, 3134–3141. doi: 10.1093/nar/24.16.3134
Roy, S., Liu, W., Nandety, R. S., Crook, A., Mysore, K. S., Pislariu, C. I., et al. (2020). Celebrating 20 Years of genetic discoveries in legume nodulation and symbiotic nitrogen fixation. Plant Cell 32, 15–41. doi: 10.1105/tpc.19.00279
Schauser, L., Roussis, A., Stiller, J., and Stougaard, J. (1999). A plant regulator controlling development of symbiotic root nodules. Nature 402, 191–195. doi: 10.1038/46058
Schiessl, K., Lilley, J. L. S., Lee, T., Tamvakis, I., Kohlen, W., Bailey, P. C., et al. (2019). NODULE INCEPTION recruits the lateral root developmental program for symbiotic nodule organogenesis in Medicago truncatula. Curr. Biol. 29, 3657–3668. doi: 10.1016/j.cub.2019.09.005
Schwarz-Sommer, Z., Huijser, P., Nacken, W., Saedler, H., and Sommer, H. (1990). Genetic control of flower development by homeotic genes in Antirrhinum majus. Science 250, 931–936. doi: 10.1126/science.250.4983.931
Shahan, R., and Benfey, P. N. (2020). A co-opted regulator of lateral root development controls nodule organogenesis in lotus. Dev. Cell 52, 6–7. doi: 10.1016/j.devcel.2019.12.009
Smaczniak, C., Immink, R. G., Angenent, G. C., and Kaufmann, K. (2012). Developmental and evolutionary diversity of plant MADS-domain factors: insights from recent studies. Development 139, 3081–3098. doi: 10.1242/dev.074674
Soyano, T., Hirakawa, H., Sato, S., Hayashi, M., and Kawaguchi, M. (2014). Nodule Inception creates a long-distance negative feedback loop involved in homeostatic regulation of nodule organ production. Proc. Natl. Acad. Sci. U. S. A. 111, 14607–14612. doi: 10.1073/pnas.1412716111
Soyano, T., Shimoda, Y., Kawaguchi, M., and Hayashi, M. (2019). A shared gene drives lateral root development and root nodule symbiosis pathways in Lotus. Science 366, 1021–1023. doi: 10.1126/science.aax2153
Sun, C. H., Yu, J. Q., Duan, X., Wang, J. H., Zhang, Q. Y., Gu, K. D., et al. (2018). The MADS transcription factor CmANR1 positively modulates root system development by directly regulating CmPIN2 in chrysanthemum. Hortic. Res. 5:52. doi: 10.1038/s41438-018-0061-y
Suzaki, T., Yoro, E., and Kawaguchi, M. (2015). Leguminous plants: inventors of root nodules to accommodate symbiotic bacteria. Int. Rev. Cell Mol. Biol. 316, 111–158. doi: 10.1016/bs.ircmb.2015.01.004
Takahara, M., Magori, S., Soyano, T., Okamoto, S., Yoshida, C., Yano, K., et al. (2013). Too much love, a novel Kelch repeat-containing F-box protein, functions in the long-distance regulation of the legume-Rhizobium symbiosis. Plant Cell Physiol. 54, 433–447. doi: 10.1093/pcp/pct022
Thompson, J. D., Higgins, D. G., and Gibson, T. J. (1994). CLUSTAL W: improving the sensitivity of progressive multiple sequence alignment through sequence weighting, position-specific gap penalties and weight matrix choice. Nucleic Acids Res. 22, 4673–4680. doi: 10.1093/nar/22.22.4673
Tsikou, D., and Yan, Z. (2018). Systemic control of legume susceptibility to rhizobial infection by a mobile microRNA. Science 362, 233–236. doi: 10.1126/science.aat6907
Valdes-Lopez, O., Arenas-Huertero, C., Ramirez, M., Girard, L., Sanchez, F., Vance, C. P., et al. (2008). Essential role of MYB transcription factor: PvPHR1 and microRNA: PvmiR399 in phosphorus-deficiency signalling in common bean roots. Plant Cell Environ. 31, 1834–1843. doi: 10.1111/j.1365-3040.2008.01883.x
Vandesompele, J., De Preter, K., Pattyn, F., Poppe, B., Van Roy, N., De Paepe, A., et al. (2002). Accurate normalization of real-time quantitative RT-PCR data by geometric averaging of multiple internal control genes. Genome Biol. 3:RESEARCH0034. doi: 10.1186/gb-2002-3-7-research0034
Venkateshwaran, M., Volkening, J. D., Sussman, M. R., and Ane, J. M. (2013). Symbiosis and the social network of higher plants. Curr. Opin. Plant Biol. 16, 118–127. doi: 10.1016/j.pbi.2012.11.007
Vieweg, M. F., Frühling, M., Quandt, H. J., Heim, U., Bäumlein, H., Pühler, A., et al. (2004). The promoter of the Vicia faba L. leghemoglobin gene VfLb29 is specifically activated in the infected cells of root nodules and in the arbuscule-containing cells of mycorrhizal roots from different legume and nonlegume plants. Mol. Plant Microbe Interact. 17, 62–69. doi: 10.1094/MPMI.2004.17.1.62
Vlasova, A., Capella-Gutierrez, S., Rendon-Anaya, M., Hernandez-Onate, M., Minoche, A. E., Erb, I., et al. (2016). Genome and transcriptome analysis of the Mesoamerican common bean and the role of gene duplications in establishing tissue and temporal specialization of genes. Genome Biol. 17:32. doi: 10.1186/s13059-016-0883-6
Waese, J., Fan, J., Pasha, A., Yu, H., Fucile, G., Shi, R., et al. (2017). ePlant: visualizing and exploring multiple levels of data for hypothesis generation in plant biology. Plant Cell 29, 1806–1821. doi: 10.1105/tpc.17.00073
Yanofsky, M. F., Ma, H., Bowman, J. L., Drews, G. N., Feldmann, K. A., and Meyerowitz, E. M. (1990). The protein encoded by the Arabidopsis homeotic gene agamous resembles transcription factors. Nature 346, 35–39. doi: 10.1038/346035a0
Keywords: transcription factors, MADS, AGL, common bean, rhizobia, symbiotic nitrogen fixation, nodule
Citation: Ayra L, Reyero-Saavedra MdR, Isidra-Arellano MC, Lozano L, Ramírez M, Leija A, Fuentes S-I, Girard L, Valdés-López O and Hernández G (2021) Control of the Rhizobia Nitrogen-Fixing Symbiosis by Common Bean MADS-Domain/AGL Transcription Factors. Front. Plant Sci. 12:679463. doi: 10.3389/fpls.2021.679463
Received: 11 March 2021; Accepted: 10 May 2021;
Published: 07 June 2021.
Edited by:
Juan Imperial, Consejo Superior de Investigaciones Científicas (CSIC), SpainReviewed by:
Elena A. Dolgikh, All-Russian Research Institute of Agricultural Microbiology of the Russian Academy of Agricultural Sciences, RussiaCopyright © 2021 Ayra, Reyero-Saavedra, Isidra-Arellano, Lozano, Ramírez, Leija, Fuentes, Girard, Valdés-López and Hernández. This is an open-access article distributed under the terms of the Creative Commons Attribution License (CC BY). The use, distribution or reproduction in other forums is permitted, provided the original author(s) and the copyright owner(s) are credited and that the original publication in this journal is cited, in accordance with accepted academic practice. No use, distribution or reproduction is permitted which does not comply with these terms.
*Correspondence: Georgina Hernández, Z2luYUBjY2cudW5hbS5teA==
Disclaimer: All claims expressed in this article are solely those of the authors and do not necessarily represent those of their affiliated organizations, or those of the publisher, the editors and the reviewers. Any product that may be evaluated in this article or claim that may be made by its manufacturer is not guaranteed or endorsed by the publisher.
Research integrity at Frontiers
Learn more about the work of our research integrity team to safeguard the quality of each article we publish.