- 1National Key Laboratory of Rice Biology, China National Rice Research Institute, Hangzhou, China
- 2Crop Production and Physiology Center (CPPC), College of Plant Science and Technology, Huazhong Agricultural University, Wuhan, China
Photosynthesis is an important biophysical and biochemical reaction that provides food and oxygen to maintain aerobic life on earth. Recently, increasing photosynthesis has been revisited as an approach for reducing rice yield losses caused by high temperatures. We found that moderate high temperature causes less damage to photosynthesis but significantly increases respiration. In this case, the energy production efficiency is enhanced, but most of this energy is allocated to maintenance respiration, resulting in an overall decrease in the energy utilization efficiency. In this perspective, respiration, rather than photosynthesis, may be the primary contributor to yield losses in a high-temperature climate. Indeed, the dry matter weight and yield could be enhanced if the energy was mainly allocated to the growth respiration. Therefore, we proposed that engineering smart rice cultivars with a highly efficient system of energy production, allocation, and utilization could effectively solve the world food crisis under high-temperature conditions.
Introduction
The global mean temperature has increased by about 1°C because of greenhouse gases containing CO2 and CH4 released by human economic activities (IPCC, 2014). By the end of the 21st century, the CO2 concentration will increase to 421–936 ppm, and the temperature is expected to rise by 1.4–5.8°C (IPCC, 2013, 2014). As reported in a previous study, the yields of wheat and rice were increased by CO2 enhancement, but higher temperatures reduced their grain yield (Cai et al., 2016). The increase in CO2 could not compensate for the negative impact on biomass and grain yield caused by higher temperatures; under the combination of elevated CO2 and temperature, there were about 10–12% and 17–35% decrease in the yield of wheat and rice, respectively (Cai et al., 2016). This threatens global food security in the face of a continuously growing world population (Wheeler and von Braun, 2013; Shi et al., 2017; Gong et al., 2020). The greater and more consistent crop production must be achieved against a backdrop of climatic stress that limits yields, and the higher photosynthetic efficiency is therefore required (Shi et al., 2018; Bailey-Serres et al., 2019). Several reports have shown that engineering the D1 subunit of photosystem II and RuBisCo activase to improve the photosynthesis can enhance the thermal resistance in rice and wheat without incurring a yield penalty (Chen et al., 2020; Degen et al., 2020). Therefore, Ahmad et al. (2020) have highlighted the importance of improving photosynthesis in field crops to reduce yield losses caused by high temperature. By contrast, Sinclair et al. (2019) have argued that increasing photosynthesis is unlikely to provide a solution to world food shortages. It, therefore, remains unclear whether photosynthetic engineering can enhance crop yields in a high-temperature climate.
The Function of Respiration and Photosynthesis in Determining Yield Loss Under High-Temperature Conditions
Photosynthesis would be completely inhibited in rice plants by a temperature of approximately 45°C (Crafts-Brandner and Salvucci, 2002; Li et al., 2020). When such stress lasts for more than 24 h, rice plants will die (Liu et al., 2018; Li et al., 2020). However, ambient air temperatures above 40°C typically last for only 1 or 2 h, but not the whole day. Due to intensive transpiration and morphological and phenological factors, the leaf temperatures tend to be 5–10°C lower than the ambient air temperature (Mathur et al., 2014; Fu et al., 2016; Zhang et al., 2016). In order to evaluate the temperature difference between the organ tissues (i.e., leaf and panicle) and air, a study was carried out by authors, and the air temperature was found to be 37–38°C in the field condition, while the panicle and leaf temperatures were about 35.5 and 31.0°C, respectively (Figure 1Aa). In the semi-open greenhouse, the water (1) and leaf (2) temperatures were approximately 37.0 and 31.7°C, respectively, when the air temperature reached 40–41°C (Figure 1Ab). This panicle temperature could induce spikelet sterility (Fu et al., 2016; Zhang et al., 2016), but the corresponding leaf temperature is not sufficient to inhibit photosynthesis unless it is accompanied by another abiotic stress such as high relative humidity, high light, or drought (Mathur et al., 2014; Zhang et al., 2016; Rashid et al., 2020). Also, the leaf temperature of rice plants were significantly lower than the air temperature in a plant growth chamber under different temperature conditions, which might be mainly ascribed to the intensive transpiration (Figure 1B). Accordingly, no obvious difference in net photosynthetic rate (PN) was shown among the temperature treatments of 28, 34, and 38°C in either rice genotypes, but the day respiration was significantly enhanced as the temperature increased (Figure 1C). It has been reported that the yield loss in rice and wheat by high night temperature is mainly ascribed to higher dark respiration, which increases the consumption of photoassimilates and thereby results in the reduction of nonstructural carbohydrates (NSC) in stem tissues (Impa et al., 2021; Xu et al., 2021). Moreover, the enhanced dark respiration restrains source availability under the combined stress of high day-and-night temperatures, leading to a considerably more severe yield penalty due to carbon loss (Xu et al., 2020). Additionally, the high midday temperature stress of 40°C caused less damage to photosynthesis but significantly decreased biomass in seagrass (George et al., 2018). Likewise, the high-temperature-tolerant wheat cultivar in Tascosa exhibited smaller reductions in biomass and lower rates of both net photosynthesis and respiration under high nighttime temperatures compared with other wheat cultivars (Impa et al., 2019). Therefore, photosynthesis might not be the main factor that leads to yield loss of rice in hot climates.
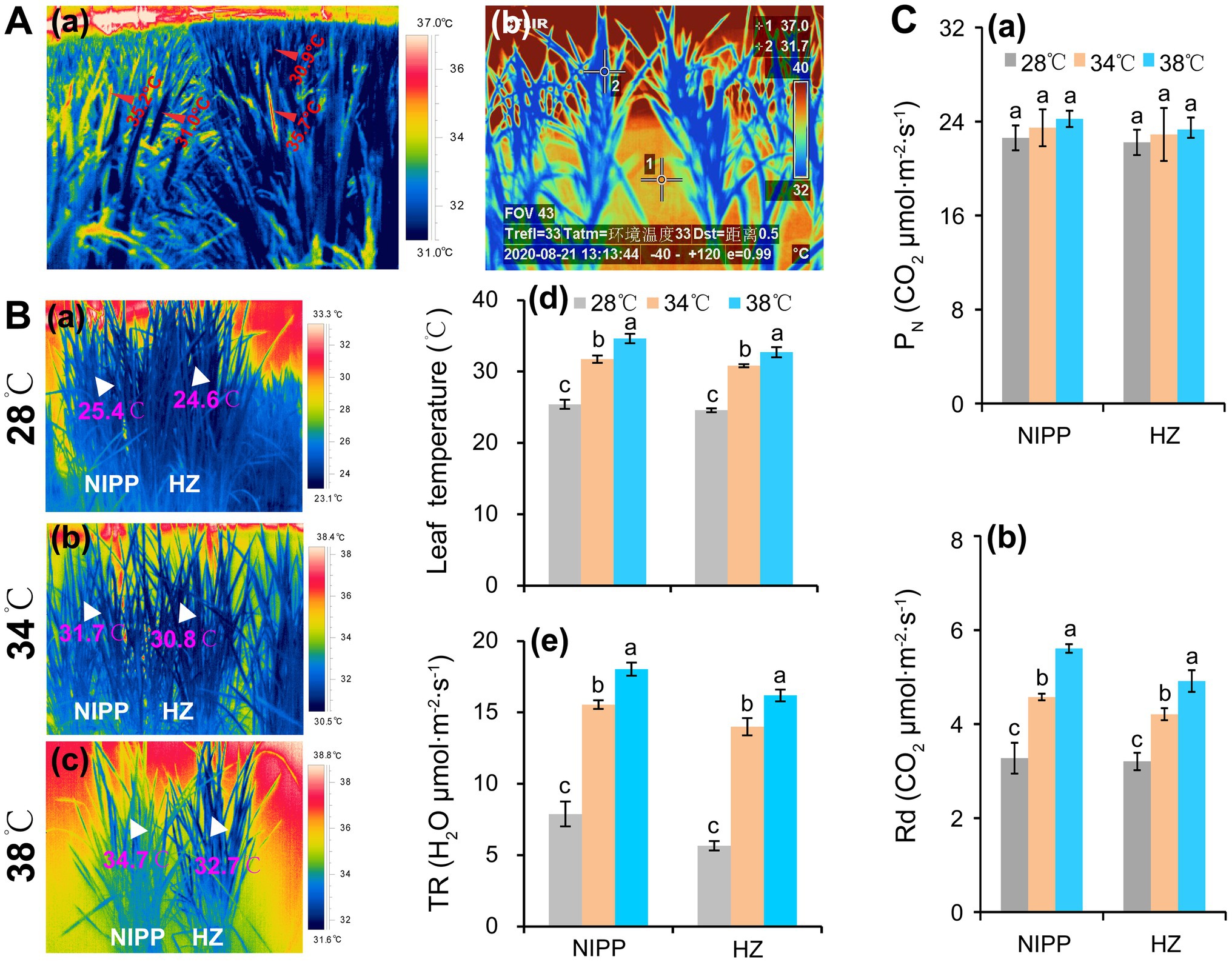
Figure 1. Response of rice plants to high temperatures. (A) Changes in leaf temperature of rice plants under high temperatures: (a) Panicle and leaf temperatures of rice plants grown in the paddy field under a high temperature of 37–38°C at the anthesis stage; (b) Leaf temperatures of rice plants grown in a greenhouse under a high temperature of 41°C at the tillering stage. (Ba–c) The thermal images of rice plants under 28, 34, and 38°C conditions in plant growth chambers; (Bd) Leaf temperature; and (Be) Transpiration rate (TR). (Ca) Net photosynthetic rate (PN); (Cb) Day respiration (Rd).
It has been reported that the average yield of four major field crops, namely, maize (Zea mays), wheat (Triticum aestivum), rice (Oryza sativa), and soybean (Glycine max), is predicted to decline by 7.4, 6.4, 3.2, and 3.1%, respectively, with every 1°C increase in the mean global temperature (Zhao et al., 2017). Interestingly, the greatest yield losses caused by the high-temperature climate were found in the C4 crop maize but not in C3 crops. This finding was inconsistent with the earlier results that C4 plants always showed high-temperature and high-light tolerance than C3 plants (Ishii et al., 1977; Berry and Björkman, 1980; Edwards and Walker, 1983). As reported by Ruiz-Vera et al. (2015), global warming resulted in a decrease in maize yield; however, the CO2 concentrations were enhanced by 200 ppm above the current concentration, and the photosynthetic biochemical parameters and the electron transport rate were adversely inhibited in this process. However, Rotundo et al. (2019) argued that the optimum temperature of photosynthesis in maize was approximately 40°C, rather than the common temperature-limiting functions indicating a decline in carbon assimilation above 30–33°C. This suggests possible overestimations of the negative impacts of global warming on maize yield due to the use of inadequate response functions relating carbon assimilation to temperature (Rotundo et al., 2019). In contrast, the respiration was enhanced irrespective of the enhancement of CO2, temperature, or in combination (Ruiz-Vera et al., 2018). Thus, the effect of global warming on maize yield loss may be due mainly to respiration rather than photosynthesis.
Energy Production Efficiency is Enhanced in Plants Under Moderate High-Temperature Conditions
Respiration is an important biochemical process that produces ATP by oxidizing organic substrates. In annual and perennial crops, about 30–60% of the carbon assimilated during photosynthesis is lost through respiration (Cannell and Thornley, 2000). This percentage may increase with rising global temperatures according to the data presented by our group, that is, respiration is positively correlated with temperature in the physiological temperature ranging from 0 to 38°C (Figure 1Cb). A 10% yield reduction was reported in rice when the minimum nighttime temperature increased by 1°C during the growing season (Peng et al., 2004). Among winter wheat cultivars widely grown in the US Great Plains, every 1°C increase in the nighttime temperature during the seed-fill period decreased the yield by 6% (Hein et al., 2019). All these results were explained by the increased respiration at night (Sadok and Jagadish, 2020; Impa et al., 2021).
Multiprotein complexes, such as NADH dehydrogenase (Complex I), succinate dehydrogenase (Complex II), cytochrome bc1 (Complex III), cytochrome c oxidase (Complex IV), ATPase (Complex V), and alternative oxidase (AOX), are involved in the respiration process and drive the cellular energy (ATP) production (Millar et al., 2011; Meyer et al., 2019). These proteins are frequently affected by abiotic stresses such as drought (Dahal et al., 2014), high temperature (Li et al., 2020; Rashid et al., 2020), cold stress (Yang et al., 2011), and high light stress (Shameer et al., 2019), thereby influencing the efficiency of ATP production. As reported earlier, respiration is controlled by the demand for ATP utilized in biosynthesis and other energy-demanding processes (Atkin and Tjoelker, 2003). Under high temperatures, rice plants exhibited higher activities of NADH dehydrogenase, cytochrome c oxidase, and ATPase and lower activity of AOX (Figures 2Aa–d). This result indicated that the energy production efficiency was enhanced by moderate high temperature, which might be ascribed primarily to the larger amount of energy required by the plants. Consistent with this interpretation, ATP content and dry matter weight were significantly decreased in plants under moderate high-temperature conditions (Figures 2Ae,f), suggesting that energy produced by respiration under high-temperature condition was mainly allocated to maintenance respiration rather than growth respiration (Amthor et al., 2019).
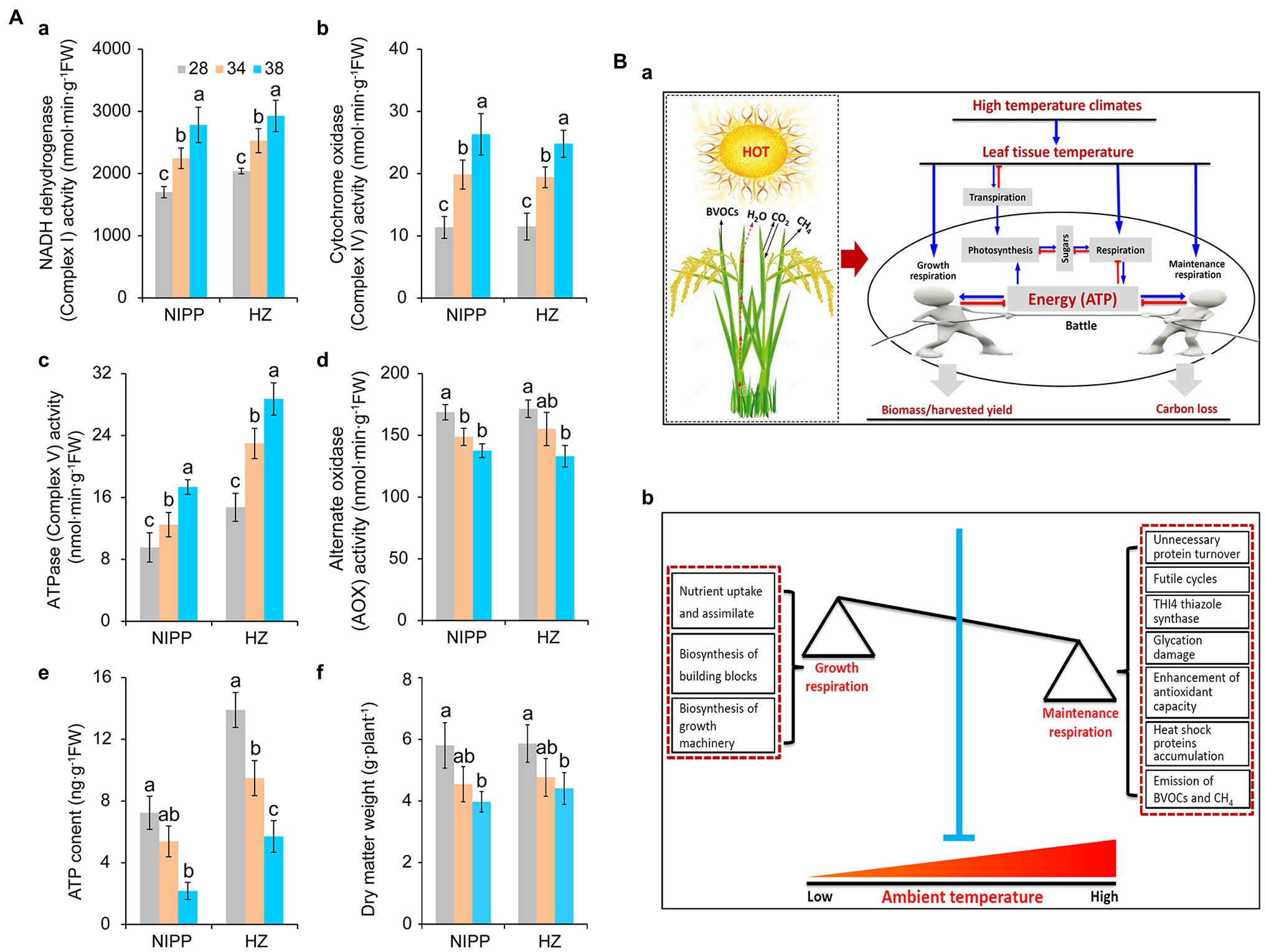
Figure 2. Effect of heat stress on dry weight and energy metabolism of rice plants. (Aa) NADH dehydrogenase; (Ab) Cytochrome c oxidase; (Ac) ATPase; (Ad) Alternative oxidase (AOX); (Ae) ATP content; and (Af) Dry weight. (Ba) The effect of leaf temperature on photosynthesis, respiration, and biomass of rice plants under high-temperature conditions. Leaf temperature and transpiration rate increase with increasing ambient temperature. Due to transpiration, plant leaf temperatures tend to be far below the ambient temperature, particularly under high-temperature conditions. In this case, moderate high temperature caused little damage to photosynthesis but increased respiration, requiring the consumption of more carbohydrates to produce ATP through respiration for the maintenance of biological activity. Plant biomass or harvest yield is determined by growth respiration and maintenance respiration under high-temperature conditions. (Bb) A model of the relationship between growth respiration and maintenance respiration in plants under global warming. The maintenance respiration processes, such as unnecessary protein turnover, futile cycling, THI4 thiazole synthase, glycation, antioxidant capacity, heat shock proteins, and even the emission of BVOCs, are activated under high temperatures. By contrast, the growth respiration processes, such as nutrient uptake and assimilation, biosynthesis of building blocks, and biosynthesis of growth machinery, are inhibited. These effects of high temperature are seen in crops with low energy utilization efficiency. Therefore, crops with high energy utilization efficiency can be engineered by inhibiting maintenance respiration processes and increasing growth respiration activities under high temperatures.
High Energy Cost of Maintenance Respiration Processes Under Moderate High-Temperature Conditions
Respiration can be separated into two components, namely, growth respiration and maintenance respiration. In growth respiration, reduced carbon compounds are metabolized to provide energy for the addition of new biomass, whereas, in maintenance respiration, this energy is used to maintain the existing mature cells in a viable state (Amthor, 2000). Protein turnover, metabolic activity, ion transport, futile cycling, sucrose transport, and the uptake and utilization of nitrogen are enhanced in plants as temperature increases, and these processes cost large amounts of energy (Amthor et al., 2019). In fact, maintaining energy homeostasis is a challenge for all living organisms under abiotic stress conditions, and an intimate relationship exists between energy availability and stress tolerance in plants (De Block and Van Lijsebettens, 2011; Dröge-Laser and Weiste, 2018). The enhancement of antioxidant capacity and the accumulation of heat shock proteins are such energetically expensive processes that plant growth and development are inhibited, and plants may die if extreme stress lasts until an energy threshold is reached above which the damage can no longer be repaired (Baena-González and Sheen, 2008; Yu et al., 2020). Plants with reduced poly(ADP-ribose) polymerase activity consumed less NAD(H) in stressful environments and improved their energy utilization efficiency by reducing overactive mitochondrial respiration and ROS production, thereby increasing stress tolerance (Vanderauwera et al., 2007; Rissel et al., 2017; Jiang et al., 2020).
An interesting hypothesis is that plant emission of biogenic volatile organic compounds (BVOCs) and methane (CH4) consumes energy produced by respiration (Figure 2Ba). It has been reported that isoprene emissions are increased as the temperature enhanced in velvet bean by regulating the enzyme isoprene synthase activity (Monson et al., 1992). Similarly, the VOCs, such as acetaldehyde and (E)-2-hexenal, were released by leaves exposed to high temperatures (Loreto et al., 2006). Interestingly, these enhancements in the emission of BVOCs could confer thermal tolerance in plants and affect the atmospheric chemistry and physics that increase greenhouse gases (Peñuelas and Llusià, 2003; Peñuelas and Staudt, 2010; Kramshøj et al., 2016; Wang et al., 2019). Temperatures of 20–40°C have a strong and immediate influence on the activity of the enzymes that catalyze the synthesis of many BVOCs (Monson et al., 1992; Loreto et al., 2006; Loreto and Schnitzler, 2010). This process not only consumes energy (Niinemets et al., 1999) but also results in a large carbon loss that up to 10% of that fixed by photosynthesis under stressful conditions (Peñuelas and Llusià, 2003). Similar results were also found in the emission of CH4 in plants (Keppler et al., 2006; Dueck and Van Der Werf, 2008; Fraser et al., 2015; Jansson et al., 2018). Interestingly, whether in paddy fields or plant growth chambers, high-temperature-sensitive cultivars had higher rates of CH4 emission than high-temperature-resistant cultivars (Supplementary Figure S1). This suggests that the energy consumed in the process of CH4 production causes an energy deficit in the high-temperature-sensitive cultivars, thus impairing high-temperature tolerance (Figure 2Ba).
Can the Engineering of Crops with High Energy Utilization Efficiency Reduce Yield Losses Caused by High Temperature?
Low respiration rates are generally correlated with high crop yields (Nunes-Nesi et al., 2005; Hauben et al., 2009), but very low respiration rates may not be sufficient to sustain energy production (De Block and Van Lijsebettens, 2011). Improved energy utilization efficiency is therefore immediately required to increase crop yields in a high-temperature climate. In fact, higher crop respiration does not always reduce biomass accumulation. A recent meta-analysis indicated that a high-temperature climate significantly increased biomass by 12.3% across all terrestrial plants, and this effect did not change with mean annual precipitation, experimental duration, CO2 enrichment, or the addition of nitrogen, drought, or irrigation (Lin et al., 2010). Similarly, grain yield was increased when plants were only subjected to high nighttime temperatures in growth chambers (Glaubitz et al., 2014). Interestingly, indica cultivars had higher respiration rates than japonica cultivars under high nighttime temperatures. Indica cultivars also showed a significant increase in biomass compared with controls, whereas japonica cultivars showed a slight decrease (Peraudeau et al., 2015). This suggested that the cultivars with increased biomass accumulation and higher respiration rates under high nighttime temperatures had greater energy utilization efficiency. More energy was allocated to growth respiration relative to maintenance respiration and even the other futile processes (Figure 2Ba). Therefore, engineering the crops with high energy efficiency in the respiration process is a feasible means of reducing yield losses caused by high temperatures.
Numerous pathways are involved in respiratory metabolism, and they are modulated by multiple genes related to all the facets of crop physiology and growth (Amthor et al., 2019). It is therefore difficult to obtain the low energy use cultivars by using conventional breeding methods. However, it is now possible to pinpoint the specific molecular targets in order to engineer greater respiratory efficiency and minimize CO2 loss. A number of processes have been suggested as targets for the engineering of energy-efficient crops, such as THI4 thiazole synthase activity, protection of proteins from glycation damage, nitrate acquisition, root-to-shoot nitrate assimilation, switching biosynthetic processes from nighttime to daytime, mitochondrial alternative oxidase activity, sucrose synthesis and degradation, and F6P/F16BP cycling (Amthor et al., 2019). These processes are related to energy production and consumption in plants. However, the interactions between these processes and their specific functions in the energy production and consumption of plants have not been fully characterized. Engineering the highly energy-efficient cultivars by improving these processes to increase the yields under a high-temperature climate is a worthwhile, but challenging, task.
Another promising strategy is the engineering of crops with lower BVOCs and CH4 emissions (Figure 2Bb). Such crops not only would have better energy utilization efficiency, reduced carbon loss, and increased yields but would also help to reduce greenhouse gas production as global warming increases. Engineering of isoprene synthesis has been reported in Cyanobacteria (Chaves and Melis, 2018), Escherichia coli (Liu et al., 2019; Lee et al., 2020), and Saccharomyces cerevisiae (Lv et al., 2016) but not in field crops.
Engineering “Smart Crops” to Reduce Yield Losses Caused by Moderate High Temperature
Recently, Yu and Li (2021) proposed their views of breeding the so-called “smart crops,” which were defined as novel crop cultivars or even nonexisting cultivars, beyond the improved existing crop varieties. In their views, smart crops with high yield, superior quality, and high stress resistance can adapt to the climate changes rapidly by sensing the environmental signaling, nutrient, and energy status. In this case, smart crops must have a highly efficient system of energy production, utilization, and allocation to balance the growth and stress response. As analyzed earlier, the energy production efficiency is improved under higher temperature conditions, but most of which is allocated to maintenance respiration, leading to lower energy utilization efficiency (Figure 2). Therefore, energy allocation might be the most important component of smart crops, and the energy sensors of SNF1-related kinases (SnRKs) and target of rapamycin (TOR) might play a key role in this process (Dobrenel et al., 2016; Rosenberger and Chen, 2018; Crepin and Rolland, 2019). Although very few studies were conducted to reveal the relationship among SnRKs, TOR, and growth and maintenance respiration in plants under high-temperature conditions, smart crops with intelligent energy allocation are worthy of breeding to reduce the yield loss.
Future Perspectives
The moderate high temperature causes less damage to photosynthesis but significantly increases crop respiration, and this is the main determinant of crop yield losses. The energy production efficiency is enhanced in crops under moderate high temperature, but most of this energy is allocated to maintenance respiration, decreasing energy utilization efficiency and reducing yields. In addition to protein turnover, metabolic activities, ion transport, futile cycling, sucrose transport, nitrogen uptake and utilization, antioxidant capacity, and the accumulation of heat shock proteins, plant emissions of BVOCs and CH4 may also consume the energy produced by respiration and release carbon fixed by photosynthesis. Engineering crops with low respiration and high energy utilization efficiency by improving these biochemical processes is both promising and challenging. The engineering of smart rice cultivars with intelligent energy allocation is seemingly an effective strategy for ensuring food security under high-temperature conditions.
The utilization of energy produced by respiration is important for crop growth and development under high temperatures; nonetheless, it has attracted relatively less attention, and there are still many outstanding questions. The interactions among the maintenance respiration processes and their functions in energy production and consumption require further characterization. The specific mechanisms by which crop plants consume energy to release BVOCs and CH4 must be investigated. Engineering crops with low BVOCs and CH4 emissions holds promise for reducing yield losses in a high-temperature climate.
Data Availability Statement
The original contributions presented in the study are included in the article/Supplementary Material, further inquiries can be directed to the corresponding authors.
Author Contributions
GL and TC collected the data and drafted the manuscript. BF revised the manuscript. GF, LT, and SP conceived the idea and revised the manuscript. All authors contributed to the article and approved the submitted version.
Funding
This work was funded by the National Food Science and Technology Project (grant/award number 2017YFD0300100), Zhejiang Provincial Natural Science Foundation, China (grant/award numbers LY19C130006 and LY20C130011), the Open Project Program of State Key Laboratory of Rice Biology (20190403), the National Rice Industry Technology System (CARS-01), and the Central Public Interest Research Institute Special Fund in China (grant number 2017RG004-1).
Conflict of Interest
The authors declare that the research was conducted in the absence of any commercial or financial relationships that could be construed as a potential conflict of interest.
Acknowledgments
We thank Hubo Li and Jiaying Ma of China National Rice Research Institute for their useful discussion of the earlier version of this manuscript. Ning Jiang, Liping Chen, and Weimeng Fu are acknowledged for determining the emission rate of CH4 for this manuscript.
Supplementary Material
The Supplementary Material for this article can be found online at: https://www.frontiersin.org/articles/10.3389/fpls.2021.678653/full#supplementary-material
References
Ahmad, N., Zaidi, S. S., and Mansoor, S. (2020). Alternative routes to improving photosynthesis in field crops. Trends Plant Sci. 25, 958–960. doi: 10.1016/j.tplants.2020.07.003
Amthor, J. S. (2000). The McCree–de Wit–Penning de Vries–Thornley respiration paradigms: 30 years later. Ann. Bot. 86, 1–20. doi: 10.1006/anbo.2000.1175
Amthor, J. S., Bar-Even, A., Hanson, A. D., Millar, A. H., Stitt, M., Sweetlove, L. J., et al. (2019). Engineering strategies to boost crop productivity by cutting respiratory carbon loss. Plant Cell 31, 297–314. doi: 10.1105/tpc.18.00743
Atkin, O. K., and Tjoelker, M. G. (2003). Thermal acclimation and the dynamic response of plant respiration to temperature. Trends Plant Sci. 8, 343–351. doi: 10.1016/S1360-1385(03)00136-5
Baena-González, E., and Sheen, J. (2008). Convergent energy and stress signaling. Trends Plant Sci. 13, 474–482. doi: 10.1016/j.tplants.2008.06.006
Bailey-Serres, J., Parker, J. E., Ainsworth, E. A., Oldroyd, G. E. D., and Schroeder, J. I. (2019). Genetic strategies for improving crop yields. Nature 575, 109–118. doi: 10.1038/s41586-019-1679-0
Berry, J. A., and Björkman, O. (1980). Photosynthetic response and adaptation to temperature in higher plants. Annu. Rev. Plant Physiol. 31, 491–543. doi: 10.1146/annurev.pp.31.060180.002423
Cai, C., Yin, X., He, S., Jiang, W., Si, C., Struik, P. C., et al. (2016). Responses of wheat and rice to factorial combinations of ambient and elevated CO2 and temperature in FACE experiments. Glob. Chang. Biol. 22, 856–874. doi: 10.1111/gcb.13065
Cannell, M. G. R., and Thornley, J. H. M. (2000). Modelling the components of plant respiration: some guiding principles. Ann. Bot. 85, 45–54. doi: 10.1006/anbo.1999.0996
Chaves, J. E., and Melis, A. (2018). Engineering isoprene synthesis in cyanobacteria. FEBS Lett. 592, 2059–2069. doi: 10.1002/1873-3468.13052
Chen, J. H., Chen, S. T., He, N. Y., Wang, Q. L., Zhao, Y., Gao, W., et al. (2020). Nuclear-encoded synthesis of the D1 subunit of photosystem II increases photosynthetic efficiency and crop yield. Nat. Plants 6, 570–580. doi: 10.1038/s41477-020-0629-z
Crafts-Brandner, S. J., and Salvucci, M. E. (2002). Sensitivity of photosynthesis in a C4 plant, maize, to heat stress. Plant Physiol. 129, 1773–1780. doi: 10.1104/pp.002170
Crepin, N., and Rolland, F. (2019). SnRK1 activation, signaling, and networking for energy homeostasis. Curr. Opin. Plant Biol. 51, 29–36. doi: 10.1016/j.pbi.2019.03.006
Dahal, K., Wang, J., Martyn, G. D., Rahimy, F., and Vanlerberghe, G. C. (2014). Mitochondrial alternative oxidase maintains respiration and preserves photosynthetic capacity during moderate drought in Nicotiana tabacum. Plant Physiol. 166, 1560–1574. doi: 10.1104/pp.114.247866
De Block, M., and Van Lijsebettens, M. (2011). Energy efficiency and energy homeostasis as genetic and epigenetic components of plant performance and crop productivity. Curr. Opin. Plant Biol. 14, 275–282. doi: 10.1016/j.pbi.2011.02.007
Degen, G. E., Worrall, D., and Carmo-Silva, E. (2020). An isoleucine residue acts as a thermal and regulatory switch in wheat Rubisco activase. Plant J. 103, 742–751. doi: 10.1111/tpj.14766
Dobrenel, T., Caldana, C., Hanson, J., Robaglia, C., Vincentz, M., Veit, B., et al. (2016). TOR signaling and nutrient sensing. Annu. Rev. Plant Biol. 67, 261–285. doi: 10.1146/annurev-arplant-043014-114648
Dröge-Laser, W., and Weiste, C. (2018). The C/S1 bZIP network: a regulatory hub orchestrating plant energy homeostasis. Trends Plant Sci. 23, 422–433. doi: 10.1016/j.tplants.2018.02.003
Dueck, T. A., and Van Der Werf, A. (2008). Are plants precursors for methane? New Phytol. 178, 693–695. doi: 10.1111/j.1469-8137.2008.02468.x
Edwards, G., and Walker, D. (1983). C3, C4: Mechanisms, and Cellular and Environmental Regulation, of Photosynthesis. Oxford, UK: Blackwell Scientific, 542.
Fraser, W. T., Blei, E., Fry, S. C., Newman, M. F., Reay, D. S., Smith, K. A., et al. (2015). Emission of methane, carbon monoxide, carbon dioxide and short-chain hydrocarbons from vegetation foliage under ultraviolet irradiation. Plant Cell Environ. 38, 980–989. doi: 10.1111/pce.12489
Fu, G., Feng, B., Zhang, C., Yang, Y., Yang, X., Chen, T., et al. (2016). Heat stress is more damaging to superior spikelets than inferiors of rice (Oryza sativa L.) due to their different organ temperatures. Front. Plant Sci. 7:1637. doi: 10.3389/fpls.2016.01637
George, R., Gullström, M., Mangora, M. M., Mtolera, M. S. P., and Björk, M. (2018). High midday temperature stress has stronger effects on biomass than on photosynthesis: a mesocosm experiment on four tropical seagrass species. Ecol. Evol. 8, 4508–4517. doi: 10.1002/ece3.3952
Glaubitz, U., Li, X., Koehl, K. I., van Dongen, J. T., Hincha, D. K., and Zuther, E. (2014). Differential physiological responses of different rice (Oryza sativa) cultivars to elevated night temperature during vegetative growth. Funct. Plant Biol. 41, 437–448. doi: 10.1071/FP13132
Gong, Z., Xiong, L., Shi, H., Yang, S., Herrera-Estrella, L. R., Xu, G., et al. (2020). Plant abiotic stress response and nutrient use efficiency. Sci. China Life Sci. 63, 635–674. doi: 10.1007/s11427-020-1683-x
Hauben, M., Haesendonckx, B., Standaert, E., Van Der Kelen, K., Azmi, A., Akpo, H., et al. (2009). Energy use efficiency is characterized by an epigenetic component that can be directed through artificial selection to increase yield. Proc. Natl. Acad. Sci. U. S. A. 106, 20109–20114. doi: 10.1073/pnas.0908755106
Hein, N. T., Wagner, D., Bheemanahalli, R., Šebela, D., Bustamante, C., Chiluwal, A., et al. (2019). Integrating field-based heat tents and cyber-physical system technology to phenotype high night-time temperature impact on winter wheat. Plant Methods 15:41. doi: 10.1186/s13007-019-0424-x
Impa, S. M., Raju, B., Hein, N. T., Sandhu, J., Prasad, P. V. V., Walia, H., et al. (2021). High night temperature effects on wheat and rice: current status and way forward. Plant Cell Environ. 1–17. doi: 10.1111/pce.14028 [Epub ahead of print].
Impa, S. M., Sunoj, V. S. J., Krassovskaya, I., Bheemanahalli, R., Obata, T., and Jagadish, S. V. K. (2019). Carbon balance and source-sink metabolic changes in winter wheat exposed to high night-time temperature. Plant Cell Environ. 42, 1233–1246. doi: 10.1111/pce.13488
IPCC (2013). Climate Change 2013: The Physical Science Basis: Working GroupIContribution to the Fifth Assessment Report of the Intergovernmental Panel on Climate change. Cambridge University Press.
IPCC (2014). Climate change 2014: Synthesis report. Contribution of Working Groups I, II and III to the Fifth Assessment Report of the Intergovernmental Panel on Climate Change. eds. Core Writing Team, R. K. Pachauri, and L. A. Meyer (Geneva, Switzerland: IPCC).
Ishii, R., Ohsugi, R., and Murata, Y. (1977). The effect of temperature on the rates of photosynthesis, respiration and the activity of RuDP carboxylase in barley, rice and maize leaves. Jpn. J. Crop Sci. 46, 516–523. doi: 10.1626/jcs.46.516
Jansson, C., Vogel, J., Hazen, S., Brutnell, T., and Mockler, T. (2018). Climate-smart crops with enhanced photosynthesis. J. Exp. Bot. 69, 3801–3809. doi: 10.1093/jxb/ery213
Jiang, N., Yu, P., Fu, W., Li, G., Feng, B., Chen, T., et al. (2020). Acid invertase confers heat tolerance in rice plants by maintaining energy homoeostasis of spikelets. Plant Cell Environ. 43, 1273–1287. doi: 10.1111/pce.13733
Keppler, F., Hamilton, J. T., Bras, M., and Rockmann, T. (2006). Methane emissions from terrestrial plants under aerobic conditions. Nature 439, 187–191. doi: 10.1038/nature04420
Kramshøj, M., Vedel-Petersen, I., Schollert, M., Rinnan, Å., Nymand, J., Ro-Poulsen, H., et al. (2016). Large increases in Arctic biogenic volatile emissions are a direct effect of warming. Nat. Geosci. 9, 349–352. doi: 10.1038/ngeo2692
Lee, H. W., Park, J. H., Kim, W. K., Lee, J. G., Lee, J. S., Ahn, J. O., et al. (2020). Engineered Escherichia coli strains as platforms for biological production of isoprene. FEBS Open Bio 10, 780–788. doi: 10.1002/2211-5463.12829
Li, G., Zhang, C., Zhang, G., Fu, W., Feng, B., Chen, T., et al. (2020). Abscisic acid negatively modulates heat tolerance in rolled leaf rice by increasing leaf temperature and regulating energy homeostasis. Rice 13:18. doi: 10.1186/s12284-020-00379-3
Lin, D., Xia, J., and Wan, S. (2010). Climate warming and biomass accumulation of terrestrial plants: a meta-analysis. New Phytol. 188, 187–198. doi: 10.1111/j.1469-8137.2010.03347.x
Liu, C. L., Bi, H. R., Bai, Z., Fan, L. H., and Tan, T. W. (2019). Engineering and manipulation of a mevalonate pathway in Escherichia coli for isoprene production. Appl. Microbiol. Biotechnol. 103, 239–250. doi: 10.1007/s00253-018-9472-9
Liu, J., Sun, X., Xu, F., Zhang, Y., Zhang, Q., Miao, R., et al. (2018). Suppression of OsMDHAR4 enhances heat tolerance by mediating H2O2-induced stomatal closure in rice plants. Rice 11:38. doi: 10.1186/s12284-018-0230-5
Loreto, F., Barta, C., Brilli, F., and Nogues, I. (2006). On the induction of volatile organic compound emissions by plants as consequence of wounding or fluctuations of light and temperature. Plant Cell Environ. 29, 1820–1828. doi: 10.1111/j.1365-3040.2006.01561.x
Loreto, F., and Schnitzler, J. P. (2010). Abiotic stresses and induced BVOCs. Trends Plant Sci. 15, 154–166. doi: 10.1016/j.tplants.2009.12.006
Lv, X., Wang, F., Zhou, P., Ye, L., Xie, W., Xu, H., et al. (2016). Dual regulation of cytoplasmic and mitochondrial acetyl-CoA utilization for improved isoprene production in Saccharomyces cerevisiae. Nat. Commun. 7:12851. doi: 10.1038/ncomms12851
Mathur, S., Agrawal, D., and Jajoo, A. (2014). Photosynthesis: response to high temperature stress. J. Photochem. Photobiol. B 137, 116–126. doi: 10.1016/j.jphotobiol.2014.01.010
Meyer, E. H., Welchen, E., and Carrie, C. (2019). Assembly of the complexes of the oxidative phosphorylation system in land plant mitochondria. Annu. Rev. Plant Physiol. 70, 23–50. doi: 10.1146/annurev-arplant-050718-100412
Millar, A. H., Whelan, J., Soole, K. L., and Day, D. A. (2011). Organization and regulation of mitochondrial respiration in plants. Annu. Rev. Plant Physiol. 62, 79–104. doi: 10.1146/annurev-arplant-042110-103857
Monson, R. K., Jaeger, C. H., Adams, W. W., Driggers, E. M., Silver, G. M., and Fall, R. (1992). Relationships among isoprene emission rate, photosynthesis, and isoprene synthase activity as influenced by temperature. Plant Physiol. 98, 1175–1180. doi: 10.1104/pp.98.3.1175
Niinemets, Ü., Tenhunen, J. D., Harley, P. C., and Steinbrecher, R. (1999). A model of isoprene emission based on energetic requirements for isoprene synthesis and leaf photosynthetic properties for Liquidambar and Quercus. Plant Cell Environ. 22, 1319–1335. doi: 10.1046/j.1365-3040.1999.00505.x
Nunes-Nesi, A., Carrari, F., Lytovchenko, A., Smith, A. M., Loureiro, M. E., Ratcliffe, R. G., et al. (2005). Enhanced photosynthetic performance and growth as a consequence of decreasing mitochondrial malate dehydrogenase activity in transgenic tomato plants. Plant Physiol. 137, 611–622. doi: 10.1104/pp.104.055566
Peng, S., Huang, J., Sheehy, J. E., Laza, R. C., Visperas, R. M., Zhong, X., et al. (2004). Rice yields decline with higher night temperature from global warming. Proc. Natl. Acad. Sci. U. S. A. 101, 9971–9975. doi: 10.1073/pnas.0403720101
Peñuelas, J., and Llusià, J. (2003). BVOCs: plant defense against climate warming? Trends Plant Sci. 8, 105–109. doi: 10.1016/S1360-1385(03)00008-6
Peñuelas, J., and Staudt, M. (2010). BVOCs and global change. Trends Plant Sci. 15, 133–144. doi: 10.1016/j.tplants.2009.12.005
Peraudeau, S., Roques, S., Quiñones, C. O., Fabre, D., Van Rie, J., Ouwerkerk, P. B. F., et al. (2015). Increase in night temperature in rice enhances respiration rate without significant impact on biomass accumulation. Field Crop Res. 171, 67–78. doi: 10.1016/j.fcr.2014.11.004
Rashid, F. A. A., Crisp, P. A., Zhang, Y., Berkowitz, O., Pogson, B. J., Day, D. A., et al. (2020). Molecular and physiological responses during thermal acclimation of leaf photosynthesis and respiration in rice. Plant Cell Environ. 43, 594–610. doi: 10.1111/pce.13706
Rissel, D., Heym, P. P., Thor, K., Brandt, W., Wessjohann, L. A., and Peiter, E. (2017). No silver bullet-canonical poly(ADP-ribose) polymerases (PARPs) are no universal factors of abiotic and biotic stress resistance of Arabidopsis thaliana. Front. Plant Sci. 8:59. doi: 10.3389/fpls.2017.00059
Rosenberger, C. L., and Chen, J. (2018). To grow or not to grow: TOR and SnRK2 coordinate growth and stress response in Arabidopsis. Mol. Cell 69, 3–4. doi: 10.1016/j.molcel.2017.12.013
Rotundo, J. L., Tang, T., and Messina, C. D. (2019). Response of maize photosynthesis to high temperature: implications for modeling the impact of global warming. Plant Physiol. Biochem. 141, 202–205. doi: 10.1016/j.plaphy.2019.05.035
Ruiz-Vera, U. M., Siebers, M. H., Drag, D. W., Ort, D. R., and Bernacchi, C. J. (2015). Canopy warming caused photosynthetic acclimation and reduced seed yield in maize grown at ambient and elevated [CO2]. Glob. Chang. Biol. 21, 4237–4249. doi: 10.1111/gcb.13013
Ruiz-Vera, U. M., Siebers, M. H., Jaiswal, D., Ort, D. R., and Bernacchi, C. J. (2018). Canopy warming accelerates development in soybean and maize, offsetting the delay in soybean reproductive development by elevated CO2 concentrations. Plant Cell Environ. 41, 2806–2820. doi: 10.1111/pce.13410
Sadok, W., and Jagadish, S. V. K. (2020). The hidden costs of nighttime warming on yields. Trends Plant Sci. 25, 644–651. doi: 10.1016/j.tplants.2020.02.003
Shameer, S., Ratcliffe, R. G., and Sweetlove, L. J. (2019). Leaf energy balance requires mitochondrial respiration and export of chloroplast NADPH in the light. Plant Physiol. 180, 1947–1961. doi: 10.1104/pp.19.00624
Shi, W., Li, X., Schmidt, R. C., Struik, P. C., Yin, X., and Jagadish, S. V. K. (2018). Pollen germination and in vivo fertilization in response to high-temperature during flowering in hybrid and inbred rice. Plant Cell Environ. 41, 1287–1297. doi: 10.1111/pce.13146
Shi, W., Yin, X., Struik, P. C., Solis, C., Xie, F., Schmidt, R. C., et al. (2017). High day‐ and night-time temperatures affect grain growth dynamics in contrasting rice genotypes. J. Exp. Bot. 68, 5233–5245. doi: 10.1093/jxb/erx344
Sinclair, T. R., Rufty, T. W., and Lewis, R. S. (2019). Increasing photosynthesis: unlikely solution for world food problem. Trends Plant Sci. 24, 1032–1039. doi: 10.1016/j.tplants.2019.07.008
Vanderauwera, S., De Block, M., Van de Steene, N., van de Cotte, B., Metzlaff, M., and Van Breusegem, F. (2007). Silencing of poly(ADP-ribose) polymerase in plants alters abiotic stress signal transduction. Proc. Natl. Acad. Sci. U. S. A. 104, 15150–15155. doi: 10.1073/pnas.0706668104
Wang, B., Shugart, H. H., and Lerdau, M. T. (2019). Complexities between plants and the atmosphere. Nat. Geosci. 12, 693–694. doi: 10.1038/s41561-019-0413-8
Wheeler, T., and von Braun, J. (2013). Climate change impacts on global food security. Science 341, 508–513. doi: 10.1126/science.1239402
Xu, J., Henry, A., and Sreenivasulu, N. (2020). Rice yield formation under high day and night temperatures-A prerequisite to ensure future food security. Plant Cell Environ. 43, 1595–1608. doi: 10.1111/pce.13748
Xu, J., Misra, G., Sreenivasulu, N., and Henry, A. (2021). What happens at night? Physiological mechanisms related to maintaining grain yield under high night temperature in rice. Plant Cell Environ. 1–17. doi: 10.1111/pce.14046
Yang, X. Y., Chen, Z. W., Xu, T., Qu, Z., Pan, X. D., Qin, X. H., et al. (2011). Arabidopsis kinesin KP1 specifically interacts with VDAC3, a mitochondrial protein, and regulates respiration during seed germination at low temperature. Plant Cell 23, 1093–1106. doi: 10.1105/tpc.110.082420
Yu, P., Jiang, N., Fu, W., Zheng, G., Li, G., Feng, B., et al. (2020). ATP hydrolysis determines cold tolerance by regulating available energy for glutathione synthesis in rice seedling plants. Rice 13:23. doi: 10.1186/s12284-020-00383-7
Yu, H., and Li, J. (2021). Short and long term challenges in crop breeding. Natl. Sci. Rev. 8:nwab002. doi: 10.1093/nsr/nwab002
Zhang, C. X., Fu, G. F., Yang, X. Q., Yang, Y. J., Zhao, X., Chen, T. T., et al. (2016). Heat stress effects are stronger on spikelets than on flag leaves in rice due to differences in dissipation capacity. J. Agron. Crop Sci. 202, 394–408. doi: 10.1111/jac.12138
Keywords: energy utilization efficiency, photosynthesis, respiration, yield loss, smart crops breeding, high temperature
Citation: Li G, Chen T, Feng B, Peng S, Tao L and Fu G (2021) Respiration, Rather Than Photosynthesis, Determines Rice Yield Loss Under Moderate High-Temperature Conditions. Front. Plant Sci. 12:678653. doi: 10.3389/fpls.2021.678653
Edited by:
Raul Antonio Sperotto, Universidade do Vale do Taquari – Univates, BrazilReviewed by:
Fabricio Eulalio Leite Carvalho, Corporación Colombiana de Investigación Agropecuaria (Agrosavia) ‐ CI La Suiza, ColombiaCarolina Elisa Sanhueza, University of Concepcion, Chile
Copyright © 2021 Li, Chen, Feng, Peng, Tao and Fu. This is an open-access article distributed under the terms of the Creative Commons Attribution License (CC BY). The use, distribution or reproduction in other forums is permitted, provided the original author(s) and the copyright owner(s) are credited and that the original publication in this journal is cited, in accordance with accepted academic practice. No use, distribution or reproduction is permitted which does not comply with these terms.
*Correspondence: Longxing Tao, dGFvbG9uZ3hpbmdAY2Fhcy5jbg==; Guanfu Fu, ZnVnZjE5ODFAc2luYS5jb20=
†These authors have contributed equally to this work and share first authorship