- 1State Key Laboratory of Desert and Oasis Ecology, Xinjiang Institute of Ecology and Geography, Chinese Academy of Sciences, Urumqi, China
- 2University of Chinese Academy of Sciences, Beijing, China
Halophytes are capable of resisting salinity, and their root system is the part in direct contact with the saline soil environment. The aim of this study was to compare the responses of root morphology and rhizosphere characteristics to salinity between a halophyte, Suaeda salsa (suaeda), and a glycophyte, Beta vulgaris L. (sugar beet). The soil salt content was set to four levels (0.7, 1.2, 1.7, and 2.7%) by NaCl-treated plants. We investigated the soil pH, EC, nutrients and soil, plant ion (Na+, Cl−, K+, and Mg2+) concentration to evaluate the rhizospheric processes, and salt tolerance of suaeda by the root mat method. The highest biomass was in the 1.2% salt level for suaeda and in the 0.7% salt level for sugar beet. The root length and root surface area of suaeda showed similar trends to biomass, but the root diameter decreased by 11.5–17.9% with higher salinity. The Na+, Cl−, and K+ accumulations in the shoot of suaeda displayed higher than that in sugar beet, while the Mg2+ accumulation was lower in suaeda than that in sugar beet. High salinity resulted in increased pH and EC values in the rhizosphere for suaeda, but lower values of these parameters for sugar beet. Under high salinity, the Olsen phosphorus content was 0.50 g·kg−1 and 0.99 g·kg−1 higher in the rhizosphere than in the non-rhizosphere for suaeda and sugar beet. We concluded that the two species [halophyte, Suaeda salsa (suaeda), and a glycophyte, B. vulgaris L. (sugar beet)] showed diverse approaches for nutrient absorption under salinity stress. Suaeda altered its root morphology (smaller root diameter and longer roots) under salt stress to increase the root surface area, while sugar beet activated rhizospheric processes to take up more nutrients.
Introduction
Halophytes are plants that can complete their entire life history on highly saline soils. These plants have a series of adaptive strategies that have arisen during their coordinated evolution with the environment (Flowers, 2004; Shabala et al., 2014; Chaudhary et al., 2018; Qiao et al., 2018). Several studies have shown that halophytes have special mechanisms to resist and alleviate salinity stress (Bennett et al., 2013; Yuan et al., 2016; Dassanayake and Larkin, 2017; Etesami and Beattie, 2018; Ma et al., 2019). Special structures and ion transport systems alleviate the negative effects of salinity and are important adaptations to enhance plant survival under stress (Shabala et al., 2015; Belghith et al., 2018; Nikalje et al., 2018). Osmotic substances are produced inside the cell to reduce water potential and accelerate uptake of water from the outside. Under salinity, the H+-ATPase activities are ameliorated to establish the proton concentration gradient across the membrane, and Na+/H+ antiporter (responsible for counter-transport of Na+ and H+ across membranes) accelerates Na+ compartmentalization into vacuoles (Song et al., 2005; Lv et al., 2012; Wang et al., 2019). Ma et al. (2019) always found that variation in cell size with salinity mostly impacted the growth of euhalophytes and their tissue hydration. To date, despite the large researches conducted on halophytes and focused on the above-ground tissue (Song et al., 2009; Belkheiri and Mulas, 2013; Ma et al., 2019), the comprehension of the plant survival strategy under salinity still remains inadequate, especially in terms of the responses of root morphology and rhizosphere characteristics to high salinity.
Roots are the organ with the most direct exposure to salinity, and their structure and function are one of the main features in the life cycle of halophytes (Shabala et al., 2015; González-Orenga et al., 2020). The root systems of many species exhibit morphological plasticity, which increases the ratio of fine roots and improves the contact area so that they can absorb more nutrients in low-nutrient soils (Zhang et al., 2010; Li et al., 2014; Mai et al., 2018). Like nutrients, various salts show great spatial and temporal variations in their concentrations in saline-alkali soil (Chen et al., 2019). The patterns of salt distribution in the soil strongly affect the spatial distribution of plants, as well as the configuration, length, and size of their root systems. High salinity affects their root morphological traits and restricts the growth of halophytes (Song, 2009; González-Orenga et al., 2020). The roots of halophytes responded directly to sodium (Na+) and chlorine (Cl−) ions are obviously affected by other soil characteristics. This affects plants’ demand for nutrients in certain habitats (Chen et al., 2017). Previous studies have detected increased organic matter content and higher enzyme activity of the soil after planting halophytes (Yi and Zhang, 2011; Yang et al., 2016). Furthermore, in the rhizosphere, changes in the microbial population and acceleration of soil nutrient leaching occur under salt stress (Ilangumaran and Smith, 2017; Radhakrishnan and Baek, 2017). Some studies found that a heterogeneous group of bacteria benefit greatly from the rhizosphere and promote plant growth (Bashan et al., 2000; Jha et al., 2011; Ilangumaran and Smith, 2017; Etesamia and Maheshwarib, 2018; Komaresoflaa et al., 2019). Thus, plants, microorganisms, and soil make up a complex network through which materials are delivered and transferred into the micro-ecological environment of the rhizosphere (Santos et al., 2015; Qin et al., 2017; Langarica-Fuentes et al., 2018). The root exudates of halophytes are another significant factor in salt tolerance and nutrient-use efficiency (Ben Hassine et al., 2009). This suggested that rhizosphere processes also play important roles in the tolerance of halophytes to salinity, where utilizing various physical and chemical mechanisms, such as alteration in root architecture, secretion of organic acids, and production of extracellular enzymes, increase nutrient acquisition efficiency under high salinity (Wang et al., 2020). However, what are the effects of salt stress on rhizosphere characteristics and how the root morphology of halophytes responds to salinization still remain unclear. The clarification of these issues will help us to better understand salt tolerance mechanisms and strategies to absorb nutrients from highly saline soils.
Suaeda salsa (suaeda, in the family Chenopodiaceae) is an annual euhalophyte that grows in saline soils, and the species possesses the capacity in understanding 200–400 mM NaCl and has been ideal species salt tolerance studies (Song, 2009; Liu et al., 2018; Ma et al., 2019). The salt content affects the soil pH in pot experiments (Xian et al., 2019). Thus, we included B. vulgaris L., which is relatively salt tolerant, to maintain experimental consistency. B. vulgaris (also in the family Chenopodiaceae) is an annual glycophyte that has strategy in response to salt stress to a certain extent (Vitali et al., 2021). In this study, therefore, we conducted a pot experiment with two species: the euhalophyte, Suaeda salsa, and the glycophyte, B. vulgaris L. The objectives of the present study were to: (1) evaluate the effect of salt stress on root morphology (root length and surface area) and (2) determine changes in rhizosphere processes in response to salt stress.
Materials and Methods
Field Soil
The experiment was conducted in a greenhouse under a 14-h light/10-h dark photoperiod (25°C days and 20°C nights). The saline soil was collected from an experimental station in Changji, Xinjiang Province, China (44°09'59" N, 87°04'56"E). The soil was sampled in the depth 0–20 cm and then air-dried, passed through a 2-mm sieve. The basic physicochemical properties of the experimental soil were as follows: pH 7.64, EC 1.45 mS, total salt 0.70%, available nitrogen (N, NO3−, and NH4+) 33.68 mg·kg−1, and Olsen phosphorus (Olsen-P) 4.62 mg·kg−1.
Experimental Setup
In this study, the root mat method was used to distinguish rhizosphere soil (0–3 mm) from non-rhizosphere soil (Figure 1A). This system was established in PVC tubes with an inner diameter of 10 cm and height of 15 cm. In the tubes, the upper layer (10 cm) was separated from the lower layer (5 cm) by 30-μm nylon mesh. This allowed us to collect soil samples at defined distances from the roots by slicing the soil parallel to the planar mat of roots developed on the mesh surface (Hinsinger and Gilkes, 1996). Based on the salt content of the original soil, we established four salinity treatments (0.7, 1.2, 1.7, and 2.7%) by adding NaCl dissolved in water.
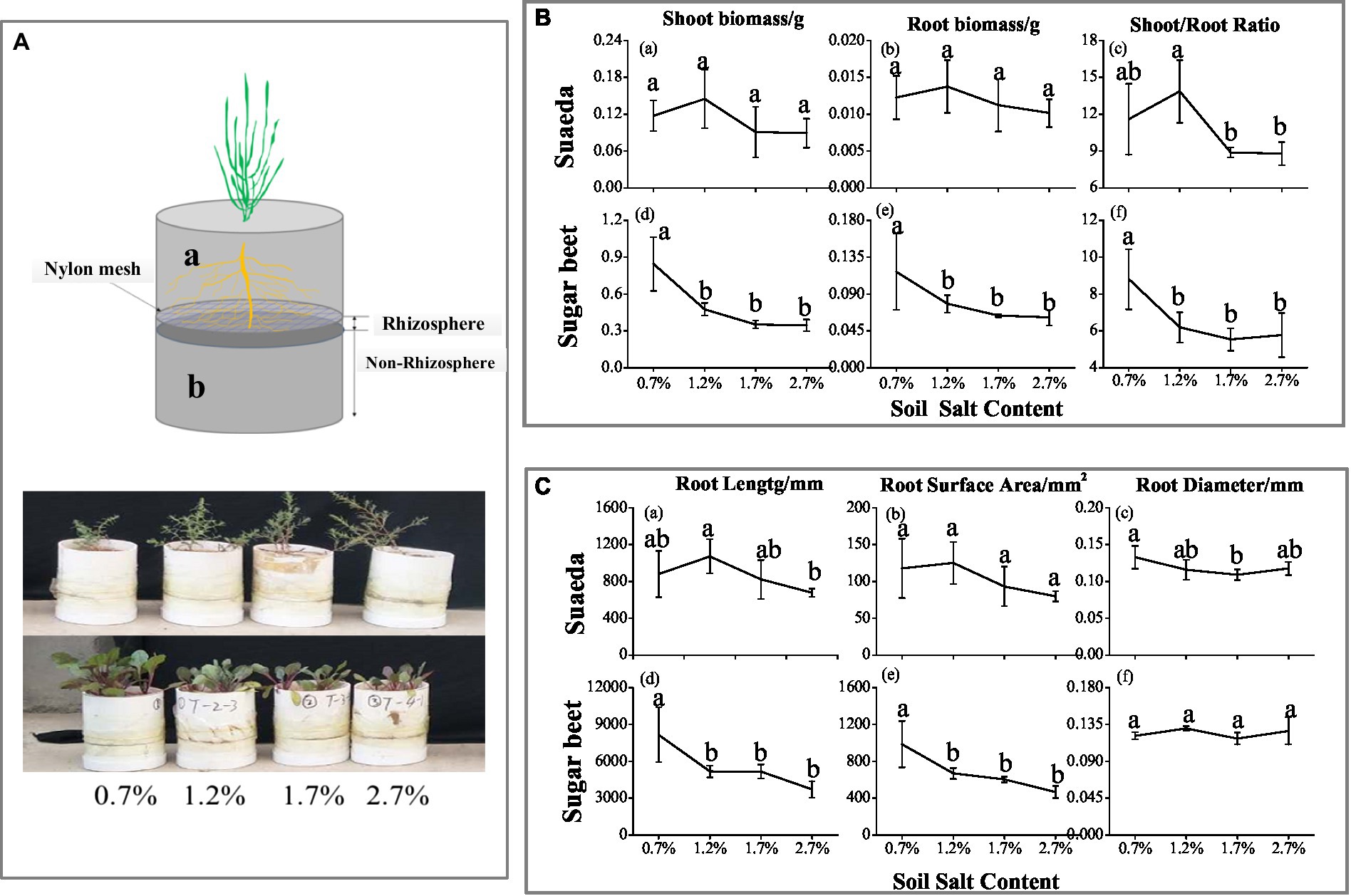
Figure 1. (A) Schematic diagram of the root system. Growing tube was divided into upper 10-cm height (A-a) and lower 5-cm depth (A-b), separated by 30-μm nylon mesh. After harvesting, the lower root system was divided into eight parts (0–1 mm, 1–2 mm, 2–3 mm, 3–5 mm, 5–10 mm, 10–20 mm, 20–30 mm, and 30–50 mm). (B) Shoot biomass (a, d), root biomass (b, e), and top root ratio (c, f) of suaeda and sugar beet under different soil salinity treatments. (C) Root length (a, d), root surface area (b, e), and root diameter (c, f) of suaeda and sugar beet under different soil salinity treatments.
Each pot was filled with 1.5 kg air-dried soil. To ensure adequate nutrient supply for plant growth, the soil was also fertilized with basal nutrients as follows (mg per pot): KH2PO4, 630 mg, and urea, 711 mg. Then, 20 suaeda seeds or 6 sugar beet seeds were sown into each pot and grown to 4-cm height before thinning. The NaCl was dissolved in water and added to the pot every 3 days for a total five times. The weighing method was used to supply water to field capacity (20%, w/w).
After 45 days of growth, the shoots and roots of suaeda and sugar beet were separated and collected for biomass measurements. The roots were washed in tap water. The root and shoot samples were killed by heating at 105°C (30 min) and then dried at 65°C for 48 h to calculate the biomass.
Sampling Analysis
The soil in the “b” portion of the tube was divided into eight parts from top to bottom, and each part was dried and passed through a 2-mm sieve (Figure 1A–b). Soil pH and EC were measured in 1: 5 mixtures (10 g soil and 50 ml water), and soil available nitrogen (N) was extracted with 0.01 mol·L−1 CaCl2 (5 g soil and 50 ml solution), and soil available phosphorus (Olsen-P) was extracted with 0.5 mol·L−1 NaHCO3 (2.5 g soil and 50 ml solution; Bao, 2000). Diluted extracts were analyzed for Na+, K+, and Mg2+ (Flame Photometer, 735 ICP-OES, Agilent Technologies, United States) and Cl− (AgNO3 titration method; Bao, 2000).
Roots were washed with deionized water and then scanned with an EPSON root scanner at 400 dots per inch resolution (Epson Expression 1600 pro, Model EU-35, Epson, Tokyo, Japan). The total root length was calculated using root analysis software DT-scan version 1.0 (Delta-T Devices, Burwell, United Kingdom). After scanning, the roots were killed by heating to 105°C (30 min) and then dried at 65°C for 48 h to calculate the biomass.
Statistical Analysis
Analysis of variance (ANOVA, one-way) was conducted to detect differences in root morphological parameters among treatments using SPSS statistical software (SPSS version 19.0, IBM SPSS Inc., Chicago, IL, United States) and R software (version 4.0.3-win). Furthermore, the differences in soil ions content between the rhizosphere and the non-rhizosphere, and the shoot ions content between suaeda and sugar beet under different salinity were investigated. Significant differences among means were separated by the (LSD) test at the p < 0.05 probability level, and it was reflected by different letters in the figures by Duncan’s test. Using smooth fitting, we investigated the spatial variations of the pH and nutrients to explore the potential effects of salinity on halophyte rhizosphere processes. To clarify these effects, we compared parameters and responses between 0.7 and 2.7% salt levels.
Results
Plant Biomass and Root Morphology
The root biomass and shoot biomass of sugar beet decreased as the salt content in soil increased (Figure 1B–d,e), while the highest biomass of suaeda was in the 1.2% salt level (Figure 1B–a,b). And the shoot/root ratio in suaeda showed higher in the 1.2% salt level (Figure 1B–c,f), while it displayed decreasing trend in sugar beet. The shoot/root ratio was significantly higher in suaeda than that in sugar beet (p < 0.05). The variations in root morphological characteristics of suaeda and sugar beet are shown in Figure 1C. The root length and surface area always preformed higher in the 1.2% salt level, and a decreasing trend in sugar beet (Figure 1C–a,b,d,e). However, the root diameter of suaeda significantly decreased by 11.5–17.9% with salinity in soil, while it displayed non-significant (Figure 1C–c,f).
Soil pH and Electrical Conductivity
We determined the pH and EC values in the rhizosphere of sugar beet and suaeda under 0.7 and 2.7% salt levels (Figure 2). For both species, the soil pH was lower in the 2.7% salt level than in the 0.7% salt level. In the 2.7% salt level, for suaeda, the pH and EC value were 0.12 and 0.22 higher in rhizosphere soil than in non-rhizosphere soil; for sugar beet, the pH and EC value were 0.05 and 0.22 lower in rhizosphere soil than in non-rhizosphere soil.
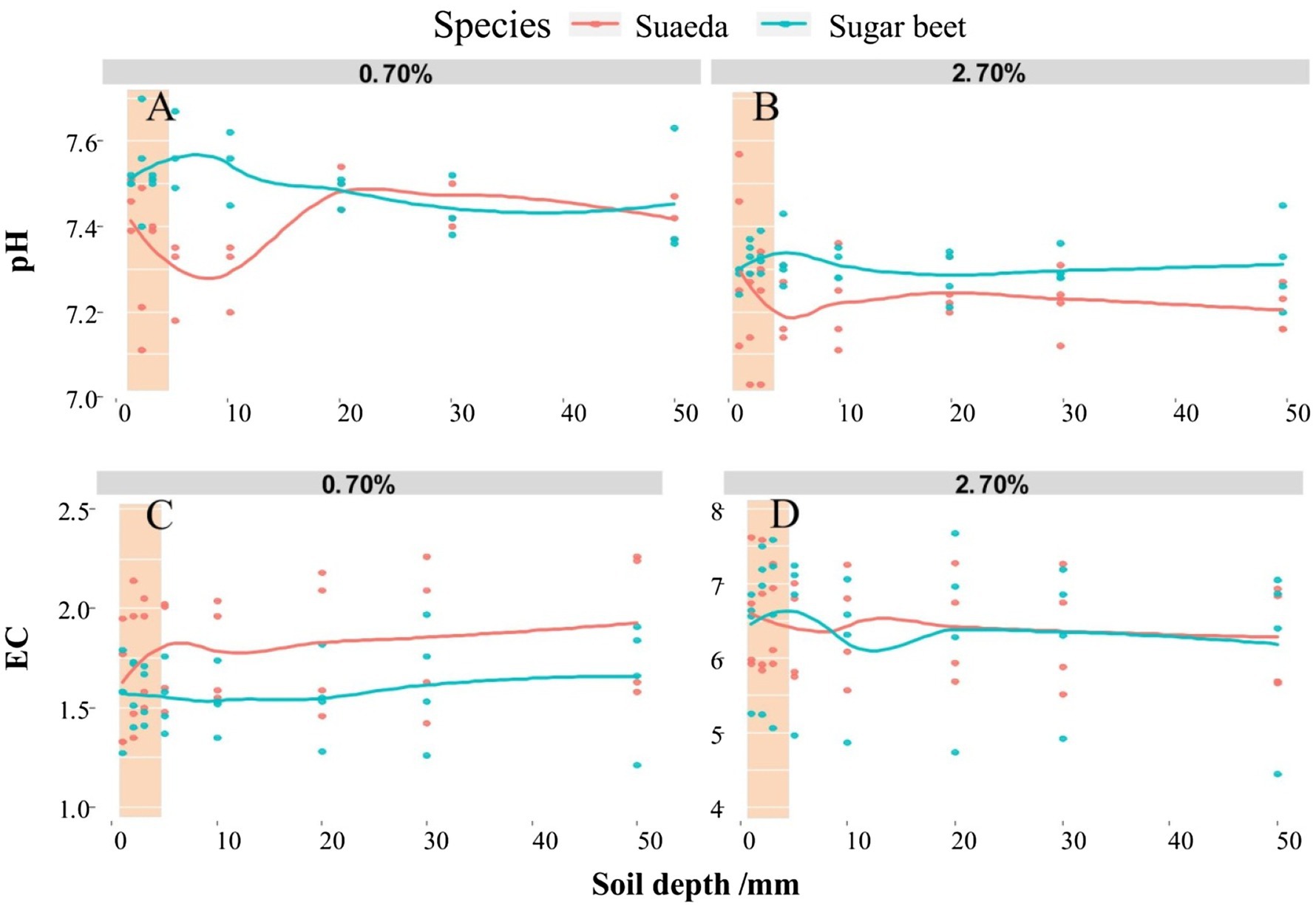
Figure 2. Spatial variations in pH (A,B) and electrical conductivity (EC; C,D) in different soil layers of the root systems of suaeda and sugar beet. Columnar shadow represents pH and EC in the rhizosphere.
Spatial Variations in Available N and Olsen-P Contents
The spatial variations in available N and Olsen-P contents in the root-soil system of suaeda and sugar beet are shown in Figure 3. The available N content was generally higher in the 0.7% salt level than in the salt level for suaeda, but not significantly different between the two treatments for sugar beet (Figures 3A,B). In the 0.7% salt level, the available N content in the rhizosphere was lower for suaeda and higher for sugar beet. The Olsen-P content decreased by 0.50 g·kg−1 and 0.99 g·kg−1 from the rhizosphere to the non-rhizosphere in suaeda and sugar beet under 2.7% salt level. The Olsen-P concentration in the rhizosphere was higher than in the non-rhizosphere for sugar beet under 0.7% salt level (Figures 3C,D).
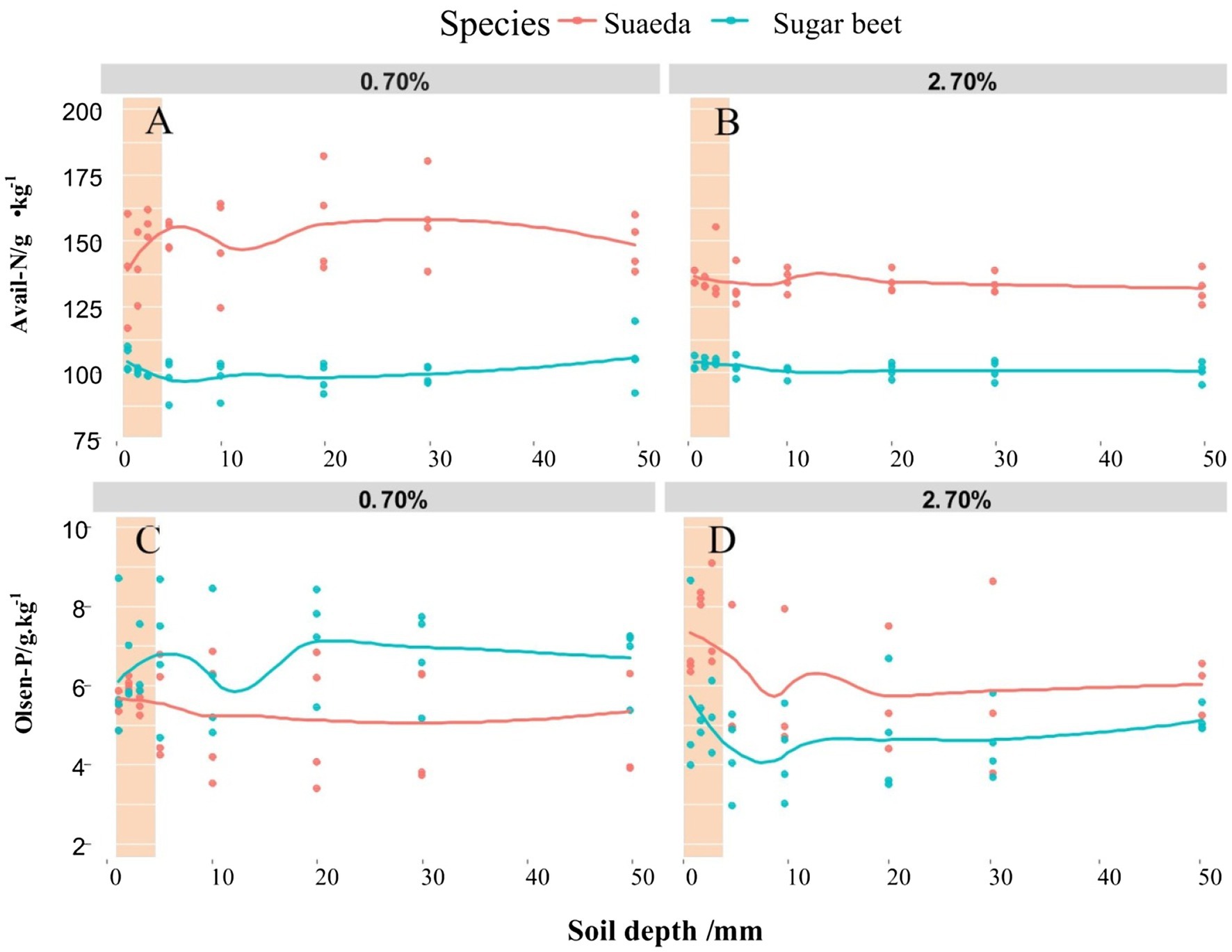
Figure 3. Spatial variations in available N (A,B) and Olsen-P (C,D) contents in different soil layers of the root system of suaeda and sugar beet. Columnar shadow represents available N and P contents in the rhizosphere.
The Na+, Cl−, K+, and Mg2+ Contents
For suaeda, the Na+ and K+ concentrations in the rhizosphere were comparatively higher than that in the non-rhizosphere under salinity, while for sugar beet the performance was not obvious (Figures 4A,C). The differegher than that in sugar beet, and it showed an increasing trend with salt level the two species (Figures 5A,B). The K+ content in suaeda and sugar beet displayed a decreasing trend, and it preformed higher in suaeda than that in sugar beet under 2.7% salt level (Figure 5C). The Mg2+ content in suaeda was significantly lower than that in sugar beet under 0.7 and 1.2% salt levels, and it always expressed highest in sugar beet under 0.7% salt level, but not obvious in suaeda (Figure 5D).
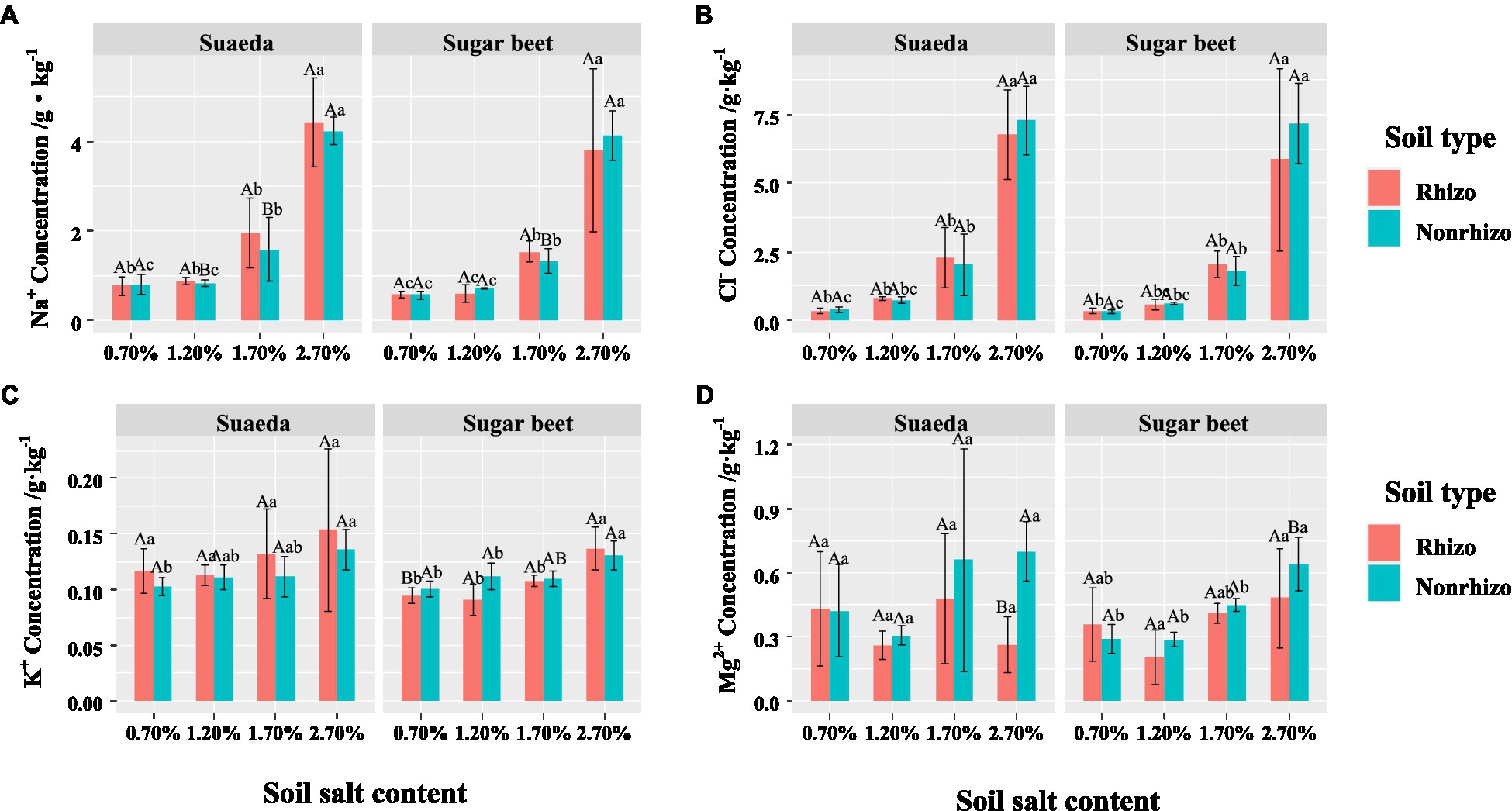
Figure 4. Na+ (A), Cl− (B), K+ (C), and Mg2+ (D) in the rhizosphere and non-rhizosphere of suaeda and sugar beet. Capital letters indicate the significant difference between species. Lower case letters indicate significant differences of four salt levels. Rhizo and Nonrhizo indicate the rhizosphere (0–3 mm) and non-rhizosphere (3–50 mm), respectively.
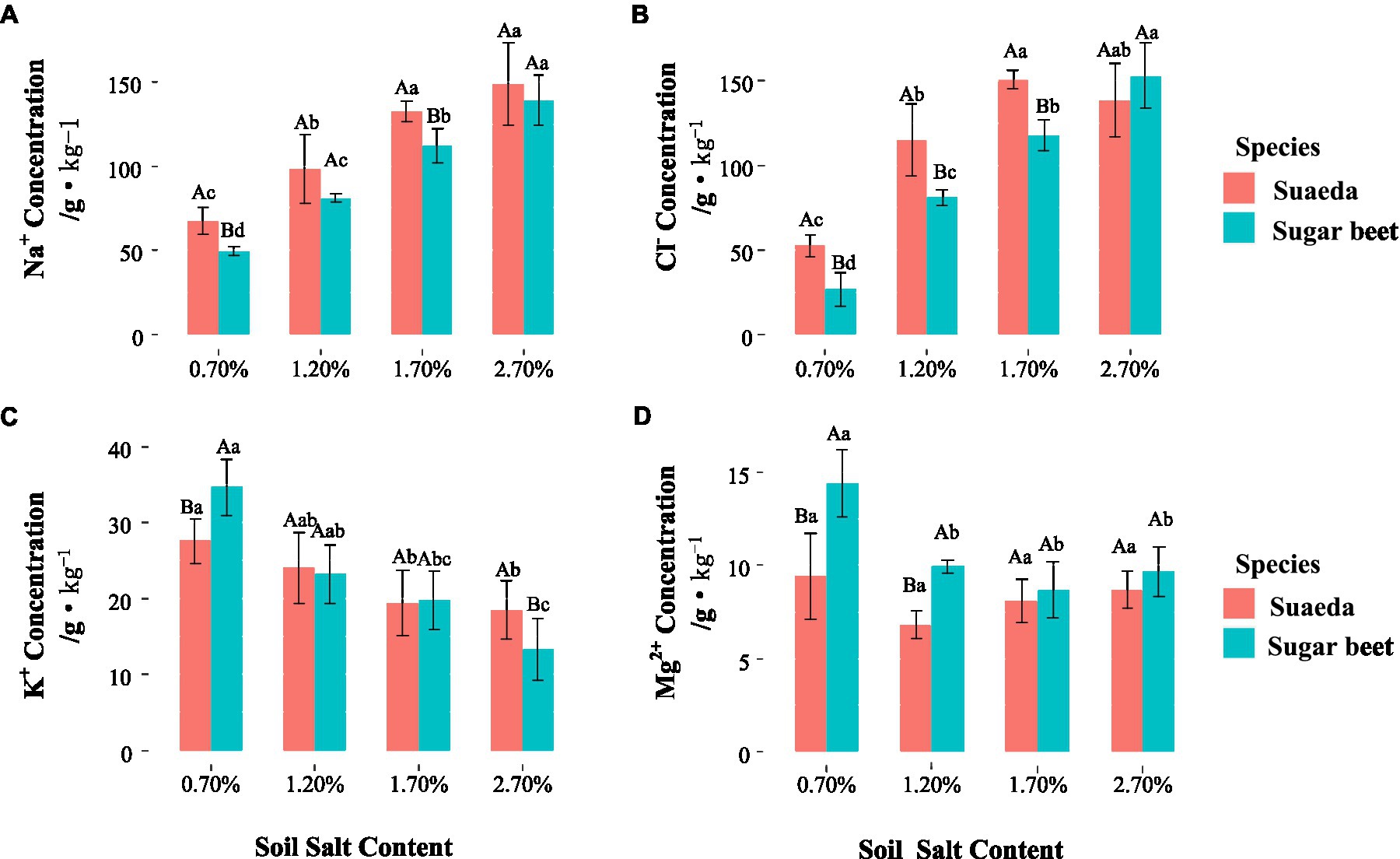
Figure 5. Na+ (A), Cl− (B), K+ (C), and Mg2+ (D) in the shoot of suaeda and sugar beet. Capital letters indicate the significant difference between species. Lower case letters indicate significant differences of four salt levels.
Discussion
Species-Dependent Growth Responses to Salt Stress
In the present study, we detected differences in the effects of salt stress on growth and root morphology between suaeda and beet. Different responses were detected between the two species and among the salt treatments (Figure 1). The results showed that the biomass of suaeda was highest in the 1.2% salt level, while that of sugar beet gradually decreased with increasing NaCl concentrations in soil. Our results are similar to those of previsssous studies in which suaeda biomass was higher in soils containing 100–200 mM NaCl than in soils containing lower concentrations of NaCl (Nobtitoshi and Iloru, 1991; Belkheiri and Mulas, 2013; Zouhaier et al., 2015; Feng et al., 2017; Ma et al., 2019). This would be explained by ion compartmentalization that vacuoles accumulate a large amount of sodiusssm ions so as to decrease water potentials and weaken salt stress (Song, 2009). Guo et al. (2020) explored the brown seeds of Suaeda salsa from NaCl-treated plants and discovered a higher germination rate under high NaCl conditions, even at 400 mM NaCl. This suggested that Na+ can be an essential factor for development of Suaeda salsa in life history. Leng et al. (2019) always found sodium (Na+) is the critical factor leading to the positive halotropism of the halophyte Limonium bicolor. The Na+ and Cl− concentratiosssns in the shoot of suaeda were displayed higher than that in sugar beet (Figure 4), which always suggests that suaeda has more adaptive capacity to salt stress. Moreover, under high salinity, the K+ and Na+ might exist competition and induce K+ deficiency in th4 rhizosphere, and depolarization of the plasma membrane also stimulates the K+ outward rectifying channels to mediate the efflux of K+ and the influx of Na+, which could be causing the decrement of K+ concentration in the shoot of suaeda (Behdad et al., 2021). The higher Mg2+ content in sugar beet would be benefit for activating chlorophyll and enzyme to strengthen salt stress (Guo et al., 2020). In present study, the salinity levels for maximum growth and the upper limits for survival were somewhat inconsistent with other studies, which might be influenced by the soil moisture content, culture method, and plant species (Liu et al., 2018; Xu et al., 2020). The shoot/root ratio of suaeda was significantly higher than that of sugar beet, indicating that there might be an underlying mechanism for the smaller roots of halophytes to satisfy the needs of above-ground growth. For suaeda, the shoot/root ratio was significantly lower under high salinity than under lower salinity (p < 0.05), suggesting that this halophyte might reinforce certain root traits to promote salt resistance of below-ground parts. An adjustment in biomass allocation as an adaptive strategy to salt stress would ensure the growth of halophytes (Yuan et al., 2010; González-Orenga et al., 2020).
Changes in Root Morphology Under Salt Stress
Roots are subject to direct damage by saline-alkali soil. Thus, changes in root morphology (root length and root diameter) and physiological manifestations are the most direct adaptive strategies for the effective absorption and utilization of soil nutrients under adverse conditions (Bates and Lynch, 1996; Mao et al., 2016; Alharby et al., 2018; Yin et al., 2021). The responses of root morphology to salt stress differed between suaeda and sugar beet (Figure 1). For suaeda, the higher root biomass and longer root length in the 1.2% salt level suggested that root length is an important factor in the adaptation to salt stress. Thus, a certain amount of salt in soil promotes root formation in halophytes, although excessive salt concentrations can have an inhibitory effect, as reported in other studies (Xiong et al., 2020; Yin et al., 2021). Xu et al. (2021) cloned the Limonium bicolor homolog of Arabidopsis thaliana and determined 100 mM NaCl-enhanced germination and root lengths. Glycophytes have also been reported to increase their root mass to increase nutrient uptake from infertile soils (Li et al., 2014; González-Orenga et al., 2020). The increase in specific root length may reflect a greater ability to absorb salt. Changes in root diameter in response to salt levels differed between the two species in this study, the diameter decreased with increasing salinity and suaeda, but this did not occur in sugar beet. This implied that suaeda increases the specific surface area of roots to increase nutrient and salt uptake under high salinity, as reported in other studies (Yang et al., 2016; Regine et al., 2018).
Variations in Rhizosphere Properties
We detected significant differences in rhizosphere processes between the halophyte and the non-halophyte in this study. The EC in the rhizosphere was higher for suaeda, indicating that the roots of suaeda had a stronger ability to absorb salt. This is also explained that the Na+ and K+ in the rhizosphere of suaeda displayed relatively higher than that in the non-rhizosphere (Figure 4). However, the pH in the rhizosphere was higher for suaeda than in the non-rhizosphere, different from the situation in glycophytes reported in previous studies (Lyu et al., 2016; Ramzani et al., 2017). Generally, H+ secretion would lead to a decrease in the pH of the rhizosphere, thereby altering the physicochemical and biological processes in the rhizosphere under adverse conditions (Gahoonia et al., 2000). The variations in pH might be because CO32− and HCO3− accumulated in the root zone of suaeda, as reported elsewhere (Yi et al., 2008). A critical aspect of halophytes is that they can adapt to saline conditions to ensure their survival (Xiong et al., 2020). Analyses of nutrient contents in the rhizosphere suggested that the ability to assimilate and activate soil Olsen-P in the root zone was enhanced under salt stress in sugar beet, whereas the ability to uptake available N was increased in suaeda (Figure 3). This suggests that sugar beet relies on root activities to increase the absorption of phosphate for plant growth under adverse conditions, as was found in previous studies (Gahoonia et al., 2000; Radhakrishnan and Baek, 2017; Chaudhary et al., 2018). For suaeda, the available P content was higher in the rhizosphere than in the non-rhizosphere, which indicated that H+ secretion activated soil nutrients to facilitate microbial activity and absorption by the roots (Zou et al., 2018). In our study, the soil pH decreased with increasing salt content (Figure 3), mainly because salinity stress results in the discharge of H+ (Yi et al., 2008). Higher salt concentrations promote the absorption of positive ions by halophytes so that the rhizosphere soil becomes enriched with anions and secreted H+ (Ben Hassine et al., 2009). Both salinity and pH affect bacterial communities, extracellular enzyme, and thus nutrient availability in saline soil (Lauber et al., 2009; Zhao et al., 2018). In addition, the roots of halophytes can secrete metabolites into the rhizosphere to stimulate microorganisms and regulate nutrient availability, ultimately enhancing plant growth and salt tolerance (Badri and Vivanco, 2009; Sashidhar and Podile, 2010; Jha et al., 2011; Yuan et al., 2017).
Conclusion
In this study, moderate salinity positively affected the biomass of suaeda, but negatively affected that of sugar beet. A certain salt always promoted root elongation of suaeda, but high salinity decreased the root diameter. For suaeda, high salinity increased the pH, EC, and Na+ content to higher levels in the rhizosphere than in the non-rhizosphere. For sugar beet, high salinity resulted in lower pH and higher available N and Olsen-P contents in the rhizosphere. This suggested that the two species use different strategies to absorb nutrients under high salinity: that is, suaeda acquires more nutrients by increasing root formation, whereas sugar beet adjusts rhizosphere processes. This study provides useful information for better figuring out the salt tolerance mechanism and strategy in nutrient uptake of halophyte under high salinity based on root morphological and rhizospheric traits. The further researches would be to detect the root system architecture, the influence of root secretion on the rhizosphere properties, and interactions among root-microorganism-soil under salinity in the future.
Data Availability Statement
The original contributions presented in the study are included in the article/supplementary material, further inquiries can be directed to the corresponding authors.
Author Contributions
SW: data curation, investigation, methodology, software, and writing – original draft. ZZ: conceptualization, formal analysis, investigation, methodology, supervision, and validation. SG: data curation, investigation, methodology, and resources – equal. BP: data curation, formal analysis, investigation, methodology, and software. KZ: conceptualization, investigation, methodology, and resources. MH: data curation, investigation, methodology, and supervision. WM: conceptualization, funding acquisition, methodology, project administration, validation – lead, visualization, and writing – review and editing. CT: funding acquisition, methodology, project administration, validation, visualization, and writing – review and editing.
Funding
This study was supported by the National Natural Science Foundation of China (Grant No. U1803233), Xinjiang Water Resources and Hydropower Planning and Design Administration Bureau (Grant No. SQ20200528151870), and the West Light Talent Program of the Chinese Academy of Sciences (Grant No. 2019-YDYLTD-001).
Conflict of Interest
The authors declare that the research was conducted in the absence of any commercial or financial relationships that could be construed as a potential conflict of interest.
Acknowledgments
We thank Jennifer Smith, PhD, from Liwen Bianji, Edanz Group China (www.liwenbianji.cn/ac), for editing the English text of a draft of this manuscript.
Abbreviations
N, nitrogen; P, phosphorus; Olsen-P, available phosphorus; pH, pondus hydrogenii; EC, electrical conductivity.
References
Alharby, H. F., Colmer, T. D., and Barrett-Lennard, E. G. (2018). Salinization of the soil solution decreases the further accumulation of salt in the root zone of the halophyte Atriplex nummularia Lindl. Growing above shallow saline groundwater. Plant Cell Environ. 41, 99–110. doi: 10.1111/pce.12958
Badri, D. V., and Vivanco, J. M. (2009). Regulation and function of root exudates. Plant Cell Environ. 32, 666–681. doi: 10.1111/j.1365-3040.2009.01926.x
Bao, S. D. (2000). Soil and agricultural chemistry analysis. Beijing: China Agriculture Press, 81–200.
Bashan, Y., Moreno, M., and Troyo, E. (2000). Growth promotion of the seawater-irrigated oilseed halophyte Salicornia bigelovii inoculated with mangrove rhizosphere bacteria and halotolerant Azospirillum spp. Biol. Fertil. Soils 32, 265–272. doi: 10.1007/s003740000246
Bates, T. R., and Lynch, J. P. (1996). Stimulation of root hair elongation in Arabidopsis thaliana by low phosphorus availability. Plant Cell Environ. 19, 529–538. doi: 10.1111/j.1365-3040.1996.tb00386.x
Behdad, A., Mohsenzadeh, S., and Azizi, M. (2021). Growth, leaf gas exchange and physiological parameters of two Glycyrrhiza glabra L. populations subjected to salt stress condition. Rhizosphere 17:100319. doi: 10.1016/j.rhisph.2021.100319
Belghith, I., Senkler, J., Hildebrandt, T., Abdelly, C., Braun, H. P., and Debez, A. (2018). Comparative analysis of salt-induced changes in the root proteome of two accessions of the halophyte Cakile maritima. Plant Physiol. Biochem. 130, 20–29. doi: 10.1016/j.plaphy.2018.06.029
Belkheiri, O., and Mulas, M. (2013). The effects of salt stress on growth, water relations and ion accumulation in two halophyte Atriplex species. Environ. Exp. Bot. 86, 17–28. doi: 10.1016/j.envexpbot.2011.07.001
Ben Hassine, A., Ghanem, M. E., Bouzid, S., and Lutts, S. (2009). Abscisic acid has contrasting effects on salt excretion and polyamine concentrations of an inland and a coastal population of the Mediterranean xero-halophyte species Atriplex halimus. Ann. Bot. 104, 925–936. doi: 10.1093/aob/mcp174
Bennett, T. H., Flowers, T. J., and Bromham, L. (2013). Repeated evolution of salt-tolerance in grasses. Biol. Lett. 9:20130029. doi: 10.1098/rsbl.2013.0029
Chaudhary, D. R., Kim, J., and Kang, H. (2018). Influences of different halophyte vegetation on soil microbial community at temperate salt marsh. Microb. Ecol. 75, 729–738. doi: 10.1007/s00248-017-1083-y
Chen, S., Wang, Z. C., Guo, X. P., Rasool, G., Zhang, J., Xie, Y., et al. (2019). Effects of vertically heterogeneous soil salinity on tomato photosynthesis and related physiological parameters. Sci. Hortic. 249, 120–130. doi: 10.1016/j.scienta.2019.01.049
Chen, Y. T., Wang, Y., and Yeh, K. C. (2017). Role of root exudates in metal acquisition and tolerance. Curr. Opin. Plant Biol. 39, 66–72. doi: 10.1016/j.pbi.2017.06.004
Dassanayake, M., and Larkin, J. C. (2017). Making plants break a sweat, the structure, function, and evolution of plant salt glands. Front. Plant Sci. 8:406. doi: 10.3389/fpls.2017.00406
Etesami, H., and Beattie, G. A. (2018). Mining halophytes for plant growth-promoting halotolerant bacteria to enhance the salinity tolerance of non-halophytic crops. Front. Microbiol. 9:148. doi: 10.3389/fmicb.2018.00148
Etesamia, H., and Maheshwarib, D. K. (2018). Use of plant growth promoting rhizobacteria (PGPRs) with multiple plant growth promoting traits in stress agriculture_Action mechanisms and future prospects. Ecotox. Environ. Safe. 156, 225–246. doi: 10.1016/j.ecoenv.2018.03.013
Feng, X. H., An, P., Guo, K., Li, X. G., Liu, X. J., and Zhang, X. M. (2017). Growth, root compensation and ion distribution in Lycium chinense under heterogeneous salinity stress. Sci. Hortic. 226, 24–32. doi: 10.1016/j.scienta.2017.08.011
Flowers, T. J. (2004). Improving crop salt tolerance. J. Exp. Bot. 55, 307–319. doi: 10.1093/jxb/erh003
Gahoonia, T. S., Asmar, F., and Giese, H. (2000). Root-released organic acid and phosphorus uptake of two barley cultivars in laboratory and field experiments. Eur. J. Agron. 12, 281–289. doi: 10.1016/S1161-0301(00)00052-6
González-Orenga, S., Llinares, J. V., Al Hassan, M., Fita, A., Collado, F., Lisón, P., et al. (2020). Physiological and morphological characterisation of Limonium species in their natural habitats, insights into their abiotic stress responses. Plant Soil 449, 267–284. doi: 10.1007/s11104-020-04486-4
Guo, J. R., Liu, L., Du, M. Y., Tian, H., and Wang, B. S. (2020). Cation and Zn accumulation in brown seeds of the euhalophyte Suaeda salsa improves germination under saline conditions. Front. Plant Sci. 11:602427. doi: 10.3389/fpls.2020.602427
Hinsinger, P., and Gilkes, R. J. (1996). Mobilization of phosphate from phosphate rock and alumina-sorbed phosphate by the roots of ryegrass and clover as related to rhizosphere pH. Eur. J. Soil Sci. 47, 533–544. doi: 10.1111/j.1365-2389.1996.tb01853.x
Ilangumaran, G., and Smith, D. L. (2017). Plant growth promoting rhizobacteria in amelioration of salinity stress: A systems biology perspective. Front. Plant Sci. 8:1768. doi: 10.3389/fpls.2017.01768
Jha, B., Gontia, I., and Hartmann, A. (2011). The roots of the halophyte Salicornia brachiata are a source of new halotolerant diazotrophic bacteria with plant growth-promoting potential. Plant Soil 356, 265–277. doi: 10.1007/s11104-011-0877-9
Komaresoflaa, B. R., Alikhania, H. A., Etesamia, H., and Khoshkholgh-Sima, N. A. (2019). Improved growth and salinity tolerance of the halophyte Salicornia sp. by co-inoculation with endophytic and rhizosphere bacteria. Appl. Soil Ecol. 138, 160–170. doi: 10.1016/j.apsoil.2019.02.022
Langarica-Fuentes, A., Manrubia, M., Giles, M. E., Mitchell, S., and Daniell, T. J. (2018). Effect of model root exudate on denitrifier community dynamics and activity at different water-filled pore space levels in a fertilised soil. Soil Biol. Biochem. 120, 70–79. doi: 10.1016/j.soilbio.2018.01.034
Lauber, C. L., Hamady, M., Knight, R., and Fierer, N. (2009). Pyrosequencing-based assessment of soil pH as a predictor of soil bacterial community structure at the continental scale. Appl. Environ. Microb. 75, 5111–5120. doi: 10.1128/AEM.00335-09
Leng, B. Y., Geng, F., Dong, X. X., Yuan, F., and Wang, B. S. (2019). Sodium is the critical factor leading to the positive halotropism of the halophyte Limonium bicolor. Plant Biosyst 153, 544–551. doi: 10.1080/11263504.2018.1508085
Li, H. B., Ma, Q. H., Li, H. G., Zhang, F. S., Rengel, Z., and Shen, J. B. (2014). Root morphological responses to localized nutrient supply differ among crop species with contrasting root traits. Plant Soil 376, 151–163. doi: 10.1007/s11104-013-1965-9
Liu, Q. Q., Liu, R. R., Ma, Y. C., and Song, J. (2018). Physiological and molecular evidence for Na+ and cl− exclusion in the roots of two Suaeda salsa populations. Aquat. Bot. 146, 1–7. doi: 10.1016/j.aquabot.2018.01.001
Lv, S., Jiang, P., Chen, X., Fan, P., Wang, X., and Li, Y. (2012). Multiple compartmentalization of sodium conferred salt tolerance in Salicornia europaea. Plant Physiol. Biochem. 51, 47–52. doi: 10.1016/j.plaphy.2011.10.015
Lyu, Y., Tang, H. L., Li, H. G., Zhang, F. S., Rengel, Z., Whalley, R. W., et al. (2016). Major crop species show differential balance between root morphological and physiological responses to variable phosphorus supply. Front. Plant Sci. 7:1939. doi: 10.3389/fpls.2016.01939
Ma, F. L., Barrett-Lennard, E. G., and Tian, C. Y. (2019). Changes in cell size and tissue hydration (‘succulence’) cause curvilinear growth responses to salinity and watering treatments in euhalophytes. Environ. Exp. Bot. 159, 87–94. doi: 10.1016/j.envexpbot.2018.12.003
Mai, W. X., Xue, X. R., Feng, G., Yang, R., and Tian, C. Y. (2018). Can optimization of phosphorus input lead to high productivity and high phosphorus use efficiency of cotton through maximization of root/mycorrhizal efficiency in phosphorus acquisition? Field Crop Res. 216, 100–108. doi: 10.1016/j.fcr.2017.11.017
Mao, P., Zhang, Y., Cao, B., Guo, L., Shao, H., Cao, Z., et al. (2016). Effects of salt stress on eco-physiological characteristics in Robinia pseudoacacia based on salt-soil rhizosphere. Sci. Total Environ. 568, 118–123. doi: 10.1016/j.scitotenv.2016.06.012
Nikalje, G. C., Variyar, P. S., Joshi, M. V., Nikam, T. D., and Suprasanna, P. (2018). Temporal and spatial changes in ion homeostasis, antioxidant defense and accumulation of flavonoids and glycolipid in a halophyte Sesuvium portulacastrum (L.). PLoS One 13:e0193394. doi: 10.1371/journal.pone.0193394
Nobtitoshi, M., and Iloru, M. (1991). Characterization of Na+ exclusion mechanisms of salt-tolerant reed plants in comparison with salt-sensitive rice plants. Physiol. Plant. 83, 170–176. doi: 10.1111/j.1399-3054.1991.tb01298.x
Qiao, L. T., Mohsin, T., Wang, L., and Tian, C. Y. (2018). Subcellular distribution and chemical forms of lithium in Li-accumulator Apocynum venetum. Plant Physiol. Biochem. 132, 341–344. doi: 10.1016/j.plaphy.2018.09.022
Qin, Y., Pan, X. Y., Kubicek, C., Druzhinina, I., Chenthamara, K., Labbe, J., et al. (2017). Diverse plant-associated pleosporalean fungi from saline areas: ecological tolerance and nitrogen-status dependent effects on plant growth. Front. Microbiol. 8:158. doi: 10.3389/fmicb.2017.00158
Radhakrishnan, R., and Baek, K. H. (2017). Physiological and biochemical perspectives of non-salt tolerant plants during bacterial interaction against soil salinity. Plant Physiol. Biochem. 116, 116–126. doi: 10.1016/j.plaphy.2017.05.009
Ramzani, P. M. A., Lin, S., Anjum, S., Khan, W. D., Ronggui, H., Iqbal, M., et al. (2017). Improved quinoa growth, physiological response, and seed nutritional quality in three soils having different stresses by the application of acidified biochar and compost. Plant Physiol. Biochem. 116, 127–138. doi: 10.1016/j.plaphy.2017.05.003
Regine, R., Thomas, D., Dietrich, H., and Christoph, L. (2018). Effects of inundation, nutrient availability and plant species diversity on fine root mass and morphology across a saltmarsh flooding gradient. Front. Plant Sci. 19:98. doi: 10.3389/fpls.2018.00098
Santos, E. S., Abreu, M. M., Peres, S., Magalhães, M. C. F., Leitão, S., Pereira, A. S., et al. (2015). Potential of Tamarix africana and other halophyte species for phytostabilisation of contaminated salt marsh soils. J. Soils Sediments 17, 1459–1473. doi: 10.1007/s11368-015-1333-x
Sashidhar, B., and Podile, A. R. (2010). Mineral phosphate solubilization by rhizosphere bacteria and scope for manipulation of the direct oxidation pathway involving glucose dehydrogenase. J. Appl. Microbiol. 109, 1–12. doi: 10.1111/j.1365-2672.2009.04654.x
Shabala, S., Bose, J., and Hedrich, R. (2014). Salt bladders: do they matter? Trends Plant Sci. 19, 687–691. doi: 10.1016/j.tplants.2014.09.001
Shabala, S., Wu, H. H., and Bose, J. (2015). Salt stress sensing and early signalling events in plant roots: current knowledge and hypothesis. Plant Sci. 241, 109–119. doi: 10.1016/j.plantsci.2015.10.003
Song, J. (2009). Root morphology is related to the phenotypic variation in waterlogging tolerance of two populations of Suaeda salsa under salinity. Plant Soil 324, 231–240. doi: 10.1007/s11104-009-9949-5
Song, J., Feng, G., Tian, C. Y., and Zhang, F. S. (2005). Strategies for adaptation of Suaeda physophora, Haloxylon ammodendron and Haloxylon persicum to a saline environment during seed-germination stage. Ann. Bot. 96, 399–405. doi: 10.1093/aob/mci196
Vitali, V., Sutka, M., Ojeda, L., Aroca, R., and Amodeo, G. (2021). Root hydraulics adjustment is governed by a dominant cell-to-cell pathway in Beta vulgaris seedlings exposed to salt stress. Plant Sci. 306:110873. doi: 10.1016/j.plantsci.2021.110873
Wang, J., Qiu, N. W., Wang, P., Zhang, W. R., Yang, X. Y., Chen, M., et al. (2019). Na+ compartmentation strategy of Chinese cabbage in response to salt stress. Plant Physiol. Biochem. 140, 151–157. doi: 10.1016/j.plaphy.2019.05.001
Wang, Z. Y., Yue, L., Dhankher, O. P., and Xing, B. S. (2020). Nano-enabled improvements of growth and nutritional quality in food plants driven by rhizosphere processes. Environ. Int. 142:105831. doi: 10.1016/j.envint.2020.105831
Xian, X. X., Kong, F. L., and Zhu, M. K. (2019). Effects of water and salt gradients on soil nutrient indices and enzyme activities in coastal wetlands. Bull. Soil Water Conser. 39, 65–71. doi: 10.13961/j.cnki.stbctb.2019.01.011
Xiong, Y. W., Li, X. W., Wang, T. T., Gong, Y., Zhang, C. M., Xing, K., et al. (2020). Root exudates-driven rhizosphere recruitment of the plant growth-promoting rhizobacterium Bacillus flexus KLBMP 4941 and its growth-promoting effect on the coastal halophyte Limonium sinense under salt stress. Ecotox. Environ. Safe. 194:110374. doi: 10.1016/j.ecoenv.2020.110374
Xu, Y. Y., Jiao, X. M., Wang, X., Zhang, H. N., Wang, B. S., and Yuan, F. (2021). Importin-β from the recretohalophyte Limonium bicolor enhances salt tolerance in Arabidopsis thaliana by reducing root hair development and abscisic acid sensitivity. Front. Plant Sci. 11:582459. doi: 10.3389/fpls.2020.582459
Xu, Z., Zhou, J., Ren, T., Du, H., Liu, H., Li, Y., et al. (2020). Salt stress decreases seedling growth and development but increases quercetin and kaempferol content in Apocynum venetum. Plant Biol. 22, 813–821. doi: 10.1111/plb.13128
Yang, H., Hu, J. X., Long, X. H., Liu, Z. P., and Rengel, Z. (2016). Salinity altered root distribution and increased diversity of bacterial communities in the rhizosphere soil of Jerusalem artichoke. Sci. Rep. 6:20687. doi: 10.1038/srep20687
Yi, L. P., Ma, J., and Li, Y. (2008). Distribution of soil nutrient concentration in the rhizosphere system of desert halophytes under two soil conditions. J. Des. Res. 28, 443–448.
Yi, L. P., and Zhang, H. (2011). Characteristics of soil enzymatic activitys and relationship with the main nutrient in the rhizosphere of four littoral halophytes. Ecol. Environ. Sci. 20, 270–275. doi: 10.16258/j.cnki.1674-5906.2011.02.020
Yin, J. F., Zhou, X. B., Yin, B. F., Li, Y. G., and Zhang, Y. M. (2021). Species-dependent responses of root growth of herbaceous plants to snow cover changes in a temperate desert, Northwest China. Plant Soil 459, 249–260. doi: 10.1007/s11104-020-04756-1
Yuan, F., Leng, B. Y., and Wang, B. S. (2016). Progress in studying salt secretion from the salt glands in recretohalophytes: how do plants secrete salt? Front. Plant Sci. 7:977. doi: 10.3389/fpls.2016.00977
Yuan, J. F., Feng, G., Ma, H. Y., and Tian, C. Y. (2010). Effect of nitrate on root development and nitrogen uptake of Suaeda physophora under NaCl salinity. Pedosphere 20, 536–544. doi: 10.1016/S1002-0160(10)60043-4
Yuan, Y. S., Zhao, W. Q., Xiao, J., Zhang, Z. L., Qiao, M. F., Liu, Q., et al. (2017). Exudate components exert different influences on microbially mediated C losses in simulated rhizosphere soils of a spruce plantation. Plant Soil 419, 127–140. doi: 10.1007/s11104-017-3334-6
Zhang, F. S., Shen, J. B., Zhang, J. L., Li, L., and Chen, X. P. (2010). Rhizosphere processes and management for improving nutrient use efficiency and crop productivity. Adv. Agron. 107, 1–32. doi: 10.1016/S0065-2113(10)07001-X
Zhao, S., Liu, J. J., Banerjee, S., Zhou, N., Zhao, Z. Y., Zhang, K., et al. (2018). Soil pH is equally important as salinity in shaping bacterial communities in saline soils under halophytic vegetation. Sci. Rep. 8:4550. doi: 10.1038/s41598-018-22788-7
Zou, X. H., Wei, D., Wu, P. F., Zhang, Y., Hu, Y. N., Chen, S., et al. (2018). Strategies of organic acid production and exudation in response to low-phosphorus stress in Chinese fir genotypes differing in phosphorus-use efficiencies. Trees 32, 897–912. doi: 10.1007/s00468-018-1683-2
Keywords: Suaeda salsa, Beta vulgaris, root morphology, rhizosphere, salinity, nutrition
Citation: Wang S, Zhao Z, Ge S, Peng B, Zhang K, Hu M, Mai W and Tian C (2021) Root Morphology and Rhizosphere Characteristics Are Related to Salt Tolerance of Suaeda salsa and Beta vulgaris L. Front. Plant Sci. 12:677767. doi: 10.3389/fpls.2021.677767
Edited by:
Honghong Wu, Huazhong Agricultural University, ChinaReviewed by:
Lan Zhu, Huazhong Agricultural University, ChinaZiming Wu, Jiangxi Agricultural University, China
Copyright © 2021 Wang, Zhao, Ge, Peng, Zhang, Hu, Mai and Tian. This is an open-access article distributed under the terms of the Creative Commons Attribution License (CC BY). The use, distribution or reproduction in other forums is permitted, provided the original author(s) and the copyright owner(s) are credited and that the original publication in this journal is cited, in accordance with accepted academic practice. No use, distribution or reproduction is permitted which does not comply with these terms.
*Correspondence: Changyan Tian, dGlhbmNoeUBtcy54amIuYWMuY24=; Wenxuan Mai, bWFpd3hAbXMueGpiLmFjLmNu