- 1State Key Laboratory of Crop Stress Adaptation and Improvement, School of Life Sciences, Henan University, Kaifeng, China
- 2State Key Laboratory of Cotton Biology, School of Life Sciences, Henan University, Kaifeng, China
- 3Jiangsu Key Laboratory of Marine Pharmaceutical Compound Screening, Jiangsu Ocean University, Lianyungang, China
Almost all abiotic stresses induce reactive oxygen species (ROS) overaccumulation, causing oxidative damages to plant cells. Catalase (CAT) plays a vital role in plant oxidative stress tolerance by scavenging stress-induced excess H2O2; thus, the identification of factors regulating catalase function will shed light on the underlying regulatory mechanisms. Here, we identified leucine aminopeptidase 2 (LAP2) as a novel CAT2-interacting protein and showed a mutual promotion effect of the two proteins in plant stress responses. LAP2 has a physical interaction with CAT2 in plant cells. The loss-of-function mutant of LAP2, lap2-3, is hypersensitive to salt or osmotic stress with increased ROS accumulation and malondialdehyde content and decreased catalase activity. The lap2-3 mutant has less CAT2 protein levels as CAT2 protein stability is impaired in the mutant. Scavenging excess ROS by glutathione or overexpressing CAT2 in the lap2-3 mutant recovers its hypersensitive phenotype to salt or osmotic stress. Further study showed that CAT2 promotes LAP2 hydrolysis activity with leucine-4-methylcoumaryl-7-amides as a substrate in vivo and in vitro, and thus, similar to the lap2-3 mutant, the cat2-1 mutant also has lower γ-aminobutyric acid content than the wild type. Together, our study reveals mutual promotion effects of CAT2 and LAP2 in conferring plant salt and osmotic stress tolerance.
Introduction
Soil salinity and water deficit are abiotic stresses that severely affect crop growth, quantity, and quality, causing enormous economic losses and serious food insecurity worldwide (Yang and Guo, 2018; Yu et al., 2020). Due to their sessile lifestyle, plants usually cannot avoid high salinity- or drought-induced injuries by directly changing their location; thus, plants have developed sophisticated adaptive mechanisms to respond to these stresses so as to survive and reproduce themselves (Li et al., 2015). Both salt and drought stresses reduce plant water uptake and disturb plant normal physiological processes, bringing about not only ionic and osmotic stresses but also oxidative damages which are secondary effects resulting from stress-induced overaccumulation of reactive oxygen species (ROS) including hydroxyl radicals, hydrogen peroxide (H2O2), and superoxide anions (Liu and He, 2016; Waszczak et al., 2018; Yang and Guo, 2018). The overaccumulated ROS can damage plant biomacromolecules such as protein, DNA, and lipids, causing protein dysfunction, DNA strand breakage, and lipid peroxidation (Umeno et al., 2017; Yuan et al., 2017). Thus, reducing stress-induced ROS overaccumulation is one of the most important and common protective mechanisms for plants under an adverse environment.
Reactive oxygen species-scavenging enzymes including superoxide dismutase (SOD), catalase (CAT), peroxidase, peroxiredoxin, and non-enzymatic small molecules contribute to maintain ROS homeostasis by removing stress-induced excess ROS in plant cells (Hu et al., 2010; Sanchez-McSweeney et al., 2021). Among these ROS-scavenging enzymes, CAT plays a vital role in plant tolerance to high salinity and drought. Early reports showed that mutation of CAT2 results in increased H2O2 accumulation and decreased tolerance to salt stress in Arabidopsis plants (Bueso et al., 2007). Similarly, a loss-of-function mutant of CAT3 with higher H2O2 accumulation in the leaves is severely sensitive to water deficit, while its overexpression transgenic plants are more tolerant than the wild-type plants when challenged with drought stress (Zou et al., 2015).
To respond to salt or drought stress, plants upregulate the expression of CATs to repress stress-induced ROS accumulation and reduce oxidative damages (Luna et al., 2005; Sharma and Dubey, 2005; Xing et al., 2007; Gondim et al., 2012; Wei et al., 2020). It is reported that a MAP kinase, MEK1, positively regulates the expression of all three Arabidopsis CATs (CAT1, CAT2, and CAT3) (Xing et al., 2007). Thus, compared with the wild-type plants, the mek1 mutant is more sensitive, but MEK1-overexpressing transgenic lines are more tolerant to both salt and drought stresses with lower or higher stress-induced CAT expression, respectively (Xing et al., 2007). In addition to the transcriptional regulation, catalase activity is also regulated by interacting partners in the plant’s response to salt or drought stress. For example, Calcium-dependent Protein Kinases8 (CPK8) interacts with and phosphorylates CAT3, stimulating its catalase activity and thus enhancing drought stress tolerance in Arabidopsis (Zou et al., 2015). Also, the protein NO CATALASE ACTIVITY1 (NCA1), as a chaperone of CAT2, confers Arabidopsis plants increased salt stress tolerance possibly through maintaining the folding of CAT2 protein in a functional state (Li et al., 2015). In addition, a peroxisome-located small heat shock protein, Hsp17.6CII, interacts with and activates catalases, conferring Arabidopsis plants alkaline and salt stress (Li et al., 2017). A recent report documented that Heat Shock Protein90.9 (HSP90.9) interacts with and promotes Catalase1 in Manihot esculenta; thus, MeHSP90.9-silenced plants are more sensitive to drought stress with lower catalase activity and higher H2O2 accumulation (Wei et al., 2020). These reports reveal that catalase-interacting proteins play a vital role in regulating catalase activity and plant abiotic stress tolerance, and thus, the identification of more CAT-interacting proteins would provide more insights into the mechanisms underlying the regulation of oxidative stress tolerance in plant’s response to salt or drought stress.
Peptidases, ubiquitous proteins in all living organisms, play fundamental roles in intracellular protein turnover and have important functions in plant growth and response to exogenous environmental changes (Waditee-Sirisattha et al., 2011). Peptidases have hydrolysis activity of liberating amino acids from the N-terminal end of proteins or peptides (Pautot et al., 2001). Leucine aminopeptidase 2 (LAP2), as a member of the M17 peptidase gene family, affects a variety of plant growth processes and stress sensitivity including high salinity and drought through changes of gene expression, amino acids, and γ-aminobutyric acid (GABA) (Waditee-Sirisattha et al., 2011). Arabidopsis LAP2 and its homologous proteins in tomato (LAP-A and LAP-N) also act as molecular chaperones to alleviate stress-induced damages to target proteins (Scranton et al., 2012). These reports clearly showed an important role of LAP2 in plant stress tolerance; however, the underlying mechanism remains elusive.
In this study, we identified LAP2 as a new CAT2-interacting partner and revealed that, on the one hand, LAP2 increases plant salt and drought stress tolerance by interacting with and stabilizing CAT2 protein to reduce ROS accumulation; on the other hand, CAT2 also stimulates LAP2 hydrolysis activity to increase GABA content. Our study reveals a mutual promotion effect of LAP2 and CAT2 on plant salt and osmotic stress tolerance.
Materials and Methods
Plant Material and Growth Conditions
Arabidopsis thaliana ecotype Columbia was used in this study. The knockout mutant of CAT2 (cat2-1, SALK_076998) was previously reported (Zhang et al., 2020b), and the lap2-3 mutant (SAIL_192_A08) was obtained from the Arabidopsis Biological Resource Center (ABRC). Seeds were surface sterilized for 5 min in 5% commercial bleach, washed three times with sterile water, and plated on half-strength Murashige and Skoog (MS) medium (pH 5.8) (Sigma-Aldrich) containing 1% sucrose and 1% agar. Plants were stratified at 4°C for 3 days in the dark, and then transferred to chambers. The seedlings grown vertically at 22°C and 100 μmol m–2 s–1 illumination under 16 h light/8 h dark conditions for 5 days were transferred to half strength MS medium without or with 125 mM NaCl or 250 mM mannitol (Sigma-Aldrich) for another 5 days, and then the fresh weight was measured.
For germination assays, Arabidopsis seeds were sown on half-strength MS medium with 125 mM NaCl or 250 mM mannitol (Sigma-Aldrich), kept in the dark at 4°C for 3 days, and transferred to chambers for 5 days, and then the percentage of green cotyledons was calculated.
For glutathione (GSH) treatment, GSH was dissolved in ddH2O to make a 100-mM stock solution and frozen at –20°C until used. When the autoclaved agar medium was cooled to below 50°C, the freshly prepared stock solution was directly added to the 1/2 MS medium to a final concentration of 100 μM, and then the medium was poured immediately into petri dishes and used to treat plant seedlings.
For cycloheximide (CHX) treatment, 5-days-old wild-type and lap2-3 mutant seedlings grown on 1/2 MS medium were transferred to liquid 1/2 MS medium containing 50 μM CHX and treated for 0, 2, 4, or 6 h. The treated seedlings were then harvested for immunoblot analysis of CAT2 protein accumulation using anti-CAT2 antibody.
3,3-Diaminobenzidine Staining and Nitroblue Tetrazolium Staining
Five-days-old seedlings were transferred to half-strength MS medium without or with 125 mM NaCl or 250 mM mannitol (Sigma-Aldrich) for 5 days, and then used for 3,3-diaminobenzidine (DAB) or nitroblue tetrazolium (NBT) staining to assay H2O2 or superoxide anion accumulation as described previously (Guan et al., 2013; Zhang et al., 2020b,c). For DAB staining, the seedlings were incubated in freshly prepared DAB staining solution (1 mg/ml DAB and 0.1% Tween 20 in 10 mM Na2HPO4) for 8 h, and then rinsed with 70% ethanol for several times to remove the chlorophyll. The images of the leaves were captured using a digital camera. For superoxide anion staining, the seedlings were vacuum infiltrated with 0.1 mg/ml NBT in 25 mM HEPES buffer (pH 7.6) for 2 h in darkness. Chlorophyll was removed by using 70% ethanol, and then the images of the leaves were captured using a digital camera. For DAB or NBT staining intensity quantification, images were first transformed into 8-bit type images and inverted, and the mean gray values of the image background and seedling leaves were collected using Photoshop CS5 (Adobe). The gray values were used to analyze the relative intensity of DAB staining or NBT staining. Three biological replicates were performed (a group of 10 seedlings was used as one biological sample), and thus, 30 seedlings were employed for the DAB or NBT staining intensity analysis.
Measurement of Malondialdehyde Content
Malondialdehyde (MDA) content was measured according to a previously described method (Zhang et al., 2020a). Briefly, 0.2 g of treated or untreated seedlings were ground in 1 ml of chilled reagent (0.25% thiobarbituric acid in 10% trichloroacetic acid). After incubation at 100°C for 30 min, the extracts were cooled at room temperature and centrifuged at 12,000g for 15 min. The absorbance of the supernatant was measured at 532, 450, and 600 nm. The MDA content was calculated based on the following equation: 6.45 × (OD532 – OD600) – 0.559 × OD450.
Detection of Catalase Activity
Measurement of catalase activity was performed according to our previous report (Zhang et al., 2020b). Briefly, treated or untreated seedlings were ground with liquid nitrogen and then resuspended in cold extraction buffer (50 mM Tris-HCl, pH 7.4 and 10% glycerol). After centrifugation at 12,000g at 4°C for 15 min, the supernatant was used for assaying catalase activity using a Catalase Assay Kit (Beyotime Biotechnology) according to the manufacturer’s instructions.
Generation of Complementation (Com) Lines of the lap2-3 Mutant
The genomic sequence of 1,400 bp upstream of LAP2 translation start codon (ATG) and the full-length coding sequence (CDS) of LAP2 were amplified and cloned into pCAMBIA1300 between the EcoRI/KpnI and SacI/PstI restriction sites, respectively. The resulting plasmid was introduced into the lap2-3 mutant via Agrobacterium tumefaciens-mediated floral transformation. Com T4 transgenic plants were used for phenotypic analysis.
Plasmid Construction and Plant Transformation
The full-length coding sequence of CAT2 was amplified using PCR and cloned into pEGAD, resulting in 35S:GFP-CAT2, and then introduced into the lap2-3 mutant using an A. tumefaciens (GV3101)-mediated transformation and the floral dip method. The T4 transgenic plants were used for phenotypic analysis.
Quantitative Real-Time PCR
Treated or untreated seedlings were collected for total RNA isolation, first-strand cDNA synthesis, and qRT-PCR as we described previously (Liu et al., 2018). The constitutively expressed ACTIN2/8 gene was used as an internal control. All experiments were repeated at least three times. The primer sequences are listed in Suppmentary Table 1.
Yeast Two-Hybrid Assay
To assay the interaction between CAT2 and LAP2 in yeast, the full-length CDS of LAP2 was cloned into pGADT7, and the plasmid pGBKT7–CAT2 was previously reported (Yuan et al., 2017). The yeast transformation and growth were carried out with the Matchmaker system (Clontech). Primer sequences are listed in Suppmentary Table 1.
Luciferase Complementation Imaging Assays
The CDS of CAT2 and LAP2 were in cloned into the JW772 and JW771 vectors containing n-LUC and c-LUC, respectively. The resultant plasmids were transformed into A. tumefaciens (C58C1) and used to infiltrate Nicotiana benthamiana leaves. Three days after infiltration, the leaves were incubated in PBS solution containing 150 μg/ml D-luciferin potassium salt in the dark for 10 min before luminescence assay. The luciferase (LUC) image was captured by NightSHADE LB 985 according to the manufacturer’s instructions.
Co-immunoprecipitation Assays
To assay the interaction between CAT2 and LAP2 in plants, the CDS of CAT2 was amplified using PCR with specific primers containing Flag sequence (GATTACAAGGATGACGACGATAAG), and then inserted into the pBARN vector at SmaI site behind the 35S promoter, resulting in 35S:CAT2-Flag. The CDS of LAP2 was cloned into the pEGAD vector at BamHI site, resulting in 35S:GFP–LAP2. The resultant plasmids were transformed into Agrobacterium GV3101 and used to infiltrate N. benthamiana leaves. Three days after infiltration, total proteins were extracted from the leaves and immunoprecipitated with gel-conjugated mouse anti-GFP mAb (ABclonal, #AE064, dilution at 1:1,000). The precipitants were resuspended, separated by SDS-PAGE gel, and then immunoblotted with anti-Flag.
Bimolecular Fluorescence Complementation Assays
For the bimolecular fluorescence complementation (BiFC) assays, the CDS of Arabidopsis CAT2 and LAP2 were cloned into pSP-YNE and pSP-YCE, respectively, resulting in 35S:CAT2-YFPn and 35S:LAP2-YFPc. The resultant plasmids were introduced into A. tumefaciens (GV3101) and used to infiltrate N. benthamiana leaves. Three days after infiltration, the leaves were used to observe the reconstituted YFP fluorescent signal under a confocal laser scanning microscope (Carl Zeiss LSM710 META laser scanning microscope).
Protein Expression and Purification
The pET28a-CAT2 plasmid and His-tagged CAT2 protein expression and purification were previously reported (Yuan et al., 2017). The CDS of LAP2 was cloned into pGEX4T-1 and transformed into Escherichia coli BL21 (DE3). The GST-fused LAP2 protein was expressed with 0.5 mM isopropyl β-D-thiogalactopyranoside at 22°C for 12 h and then purified using GST agarose (Thermo Scientific) according to the manufacturer’s instructions.
Detection of LAP2 Activity
The LAP2 activity with the synthetic fluorogenic substrate leucine-4-methylcoumaryl-7-amides (Leu-MCA) was performed according to a previously reported method (Waditee-Sirisattha et al., 2011). Briefly, the reaction mixture (100 μM of Leu-MCA, 50 mM Tris-Cl buffer, pH 8.5, 2.0 mM MnSO4) was incubated at 37°C. Once the purified LAP2 or CAT2 protein was added, the fluorescence signal of 7-amino-4-methylcoumarin released by LAP2 hydrolysis activity was measured at an excitation wavelength of 360 nm and an emission wavelength of 460 nm by BioTek Cytation 3 for 45 min. According to a previous report, the fluorescence intensities at 30 min were further analyzed (Waditee-Sirisattha et al., 2011). Three independent biological replicates were performed.
Measurement of GABA Content
Detection of GABA content in the wild-type and mutant seedlings was modified from previously reported methods (Liu et al., 2018; Yue et al., 2018). Briefly, 0.1 g of the wild-type lap2-3 and cat2-1 mutant seedlings were ground with liquid nitrogen to a fine powder and extracted using 1 ml acetonitrile. After centrifugation at 12,000g for 15 min, the supernatant was transferred and dried under nitrogen gas in a new tube. The crude extract resuspended in 100 μl acetonitrile containing 1% 2,4-dinitrofuorobenzene was mixed with 100 μl of 0.1 M Na2B4O7 and then incubated at 60°C for 1 h in the dark. After derivatization, GABA standards and the samples were, respectively, separated and monitored by Agilent 1100 HPLC (C18 reversed-phase column) with the mobile phase (acetonitrile and phosphate buffer) at a flow rate of 1.0 ml/min at 360 nm.
Statistical Analysis
Data are means (±SD) of three biological replicates, and the asterisks indicate statistically significant differences (∗p < 0.05, ∗∗p < 0.01, and ∗∗∗p < 0.001, Student’s t test). Bars with different letters indicate significant differences at p < 0.05 by two-way ANOVA with Tukey’s multiple comparison test.
Results
Leucine Aminopeptidase2 Interacts With CAT2
CAT2 plays a vital role in plant salt and drought stress tolerance by scavenging stress-induced H2O2; thus, identification of factors regulating CAT2 function will shed light on the mechanisms underlying plant responses to high salinity or drought-induced oxidative stress. In our previous report showing that CAT2 functions in plant resistance to the necrotrophic pathogen Botrytis cinerea infection (Yuan et al., 2017), we also identified LAP2 as a CAT2-interacting protein, but we did not show the screening result previously. We therefore cloned the full-length coding sequence of LAP2 and performed a yeast two-hybrid experiment to examine the interaction between CAT2 and LAP2. Our result showed that LAP2 interacts with CAT2 in yeast (Figure 1A). The interaction between CAT2 and LAP2 was confirmed by co-immunoprecipitation (co-IP) experiment. GFP-tagged LAP2 and Flag-tagged CAT2 were transiently co-expressed in N. benthamiana leaves, and CAT2-Flag could be immunoprecipitated using anti-GFP antibody, indicating that CAT2 and LAP2 form a complex in plant cells (Figure 1B). Also, the CAT2–LAP2 interaction was verified using a LUC complementation imaging assay in which nLUC-CAT2 and cLUC-LAP2 were co-expressed in N. benthamiana leaves. Luciferase fluorescence could be detected in N. benthamiana leaves after incubated with the substrate of luciferase–luciferin (Figure 1C). Additionally, we also examined the interaction of the two proteins with BiFC assays by expressing YFPn-tagged CAT2 and YFPc-tagged LAP2. Our confocal microscopy results showed that a strong florescence signal was detected in the cytosol (Figure 1D), suggesting that LAP2 interacts with CAT2 in the cytosol, similar with the previously reported localization of CAT2–NAC1 interaction or CAT2–CPK3 interaction (Li et al., 2015).
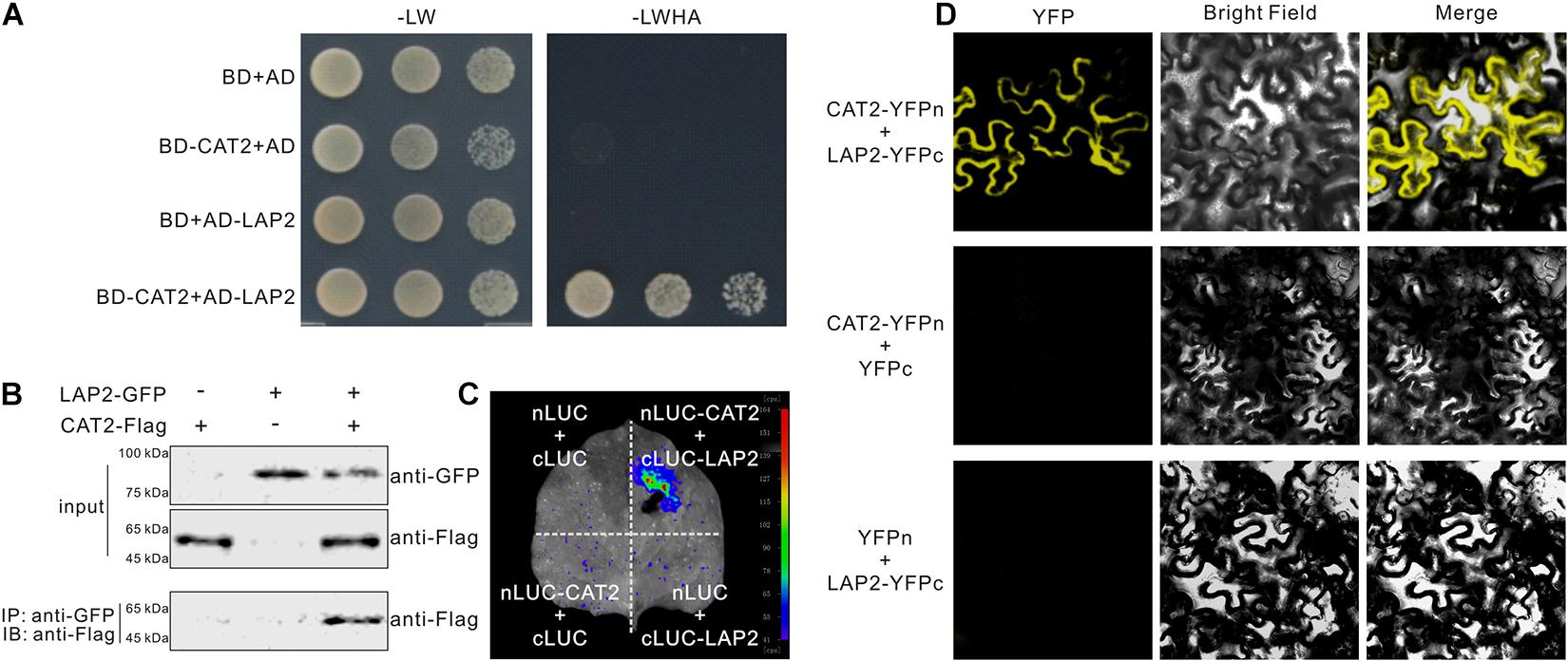
Figure 1. CAT2 interacts with leucine aminopeptidase 2 (LAP2). (A) The interaction between CAT2 and LAP2 was examined by yeast two-hybrid experiment. (B) Co-IP assays showing CAT2–LAP2 interaction. 35S:LAP2-GFP and 35S:CAT2-Flag were co-expressed in Nicotiana benthamiana leaves for 3 days via Agrobacterium-mediated transformation. Total proteins were extracted and immunoprecipitated with anti-GFP antibody, and the precipitants were then immunoblotted with anti-Flag. (C) CAT2 interacts with LAP2 in N. benthamiana leaves by the LCI assay. (D) CAT2 interacts with LAP2 in N. benthamiana leaves as indicated by BiFC assays.
Leucine Aminopeptidase2 Is Required for Plant Stress Tolerance by Affecting ROS Accumulation and Catalase Activity
Previous reports showed that NAC1 and CPK3 interact with CAT2 and positively regulate plant tolerance to salt and drought stress, leading us to further examine the role of LAP2 in the regulation of CAT2 activity in abiotic stresses. A previous report showed that LAP2 mutants, lap2-1 and lap2-2, are sensitive to salt and drought stresses when grown in soil, while the underlying mechanism remains largely unknown (Waditee-Sirisattha et al., 2011). To confirm these results, we identified another LAP2 T-DNA insertion mutant, SAIL_192_A08 (named as lap2-3, Supplementary Figure 1A), and assayed its sensitivity to salt and osmotic stresses by adding high concentrations of NaCl or mannitol in half-strength MS medium. Consistent with previous reports, our results showed that the lap2-3 mutant as well as cat2-1, the knockout mutant of CAT2, was indeed sensitive to high salinity and osmotic stress in terms of green cotyledon rate and changes of fresh weight (Figures 2A–C). We also generated complementation lines (Com#1 and Com#2) expressing the full-length coding sequence of LAP2 driven by its native promoter in the lap2-3 mutant (Supplementary Figure 1B) and tested their stress sensitivity phenotype. Our results showed that the LAP2 complementation lines recovered reduced tolerance to high salinity or osmotic stress (Figures 2A–C), verifying that the hypersensitivity of the lap2-3 mutant to salt or osmotic stress is due to the LAP2 gene knockout.
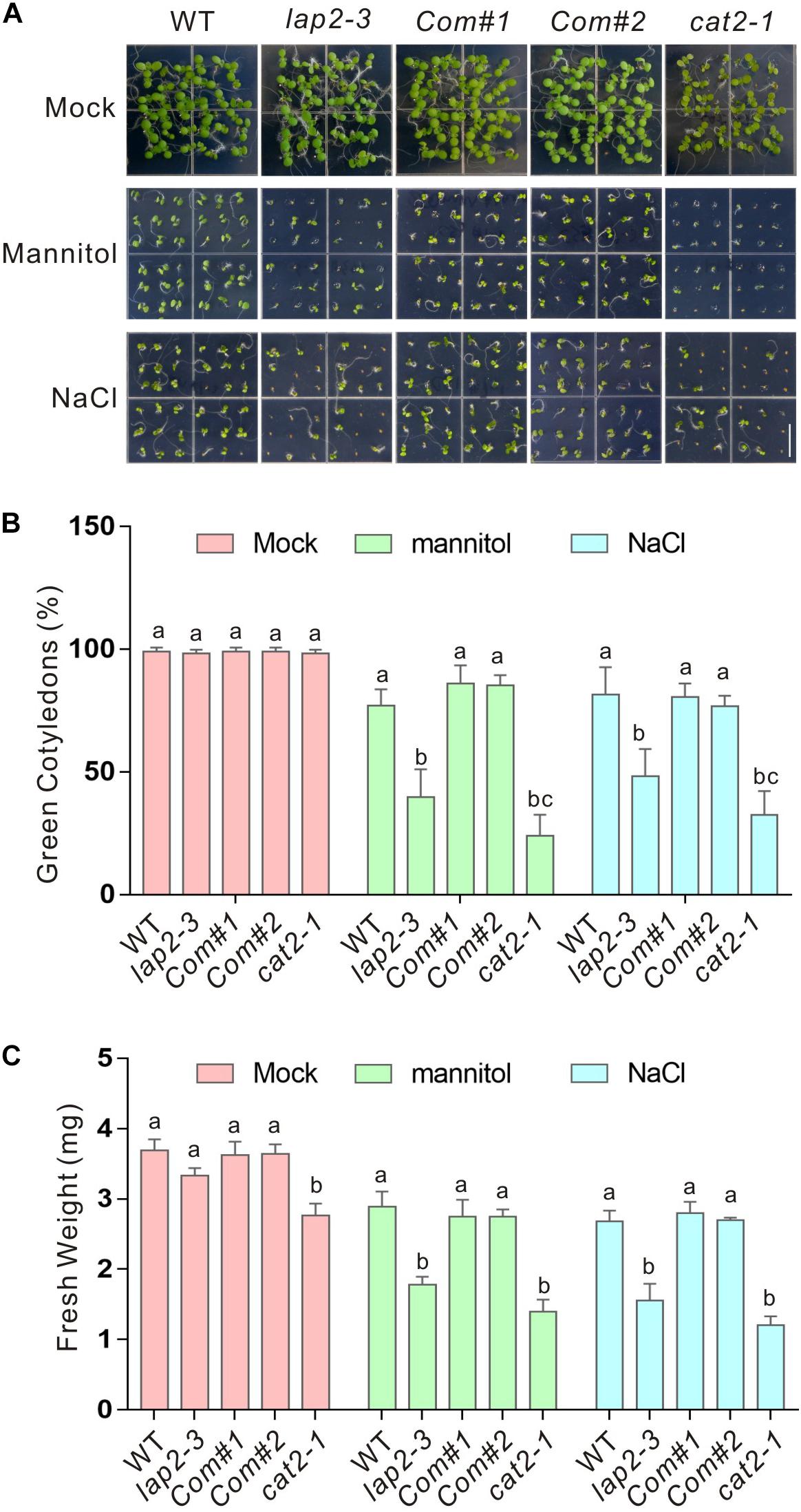
Figure 2. The lap2-3 mutant is sensitive to salt or osmotic stress. (A) Images of 5-days-old wild-type, lap2-3, LAP2 complementation lines and cat2-1 mutant seeds germinated on 1/2 MS medium without or with 250 mM mannitol or 125 mM NaCl. Bars = 0.5 cm. (B) Green cotyledon percentage of the wild-type, lap2-3, LAP2 complementation lines and cat2-1 mutant in panel (A). (C) Five-days-old wild-type, lap2-3, LAP2 complementation lines and cat2-1 mutant seedlings grown on 1/2 MS medium were transferred to 1/2 MS medium without or with 250 mM mannitol or 125 mM NaCl for another 5 days, and the fresh weight was measured. Data are means (±SD) of three biological replicates. Bars with different letters in the same treatment indicate significant differences at p < 0.05, revealed using one-way ANOVA with Tukey’s multiple comparison test.
The interaction between LAP2 and CAT2 and the hypersensitivity of their mutants to salt or osmotic stress suggested an increase of ROS accumulation in the lap2-3 mutant. As expected, significantly higher H2O2 accumulation visualized by DAB staining in the leaves was detected in both lap2-3 and cat2-1 mutants compared with the wild-type plants (Figures 3A,B). H2O2 overaccumulation in plants may also disturb superoxide anion metabolism. Therefore, we examined the accumulation of superoxide anion levels by NBT staining in the wild-type lap2-3 and cat2-1 mutant leaves and found that superoxide anion accumulation in both mutants was also substantially higher than that in the wild type (Figures 3C,D). MDA content is an indicator for the extent of cellular lipid peroxidation caused by excess ROS (Shi et al., 2012; Zhang et al., 2020a). In line with the results of the H2O2 and superoxide anion accumulation, the lap2-3 and cat2-1 mutants indeed had more MDA content than the wild type after treatment with high salinity or mannitol-induced osmotic stress (Figure 3E). Furthermore, we directly determined catalase activity in the wild-type and mutant plants. Our data showed that the catalase activity in the lap2-3 and cat2-1 mutants was significantly lower than that in the wild type either in the presence or absence of high salinity or osmotic stress treatment (Figure 3F), indicating that LAP2 is required for catalase activity in plants under stress conditions. In addition, the LAP2 complementation lines had similar accumulation of H2O2 and superoxide anion, MDA content, and catalase activity to the wild type (Figure 3). Together, these results revealed that LAP2 confers plant salt or osmotic stress tolerance by promoting catalase activity and, thus, reducing ROS accumulation.
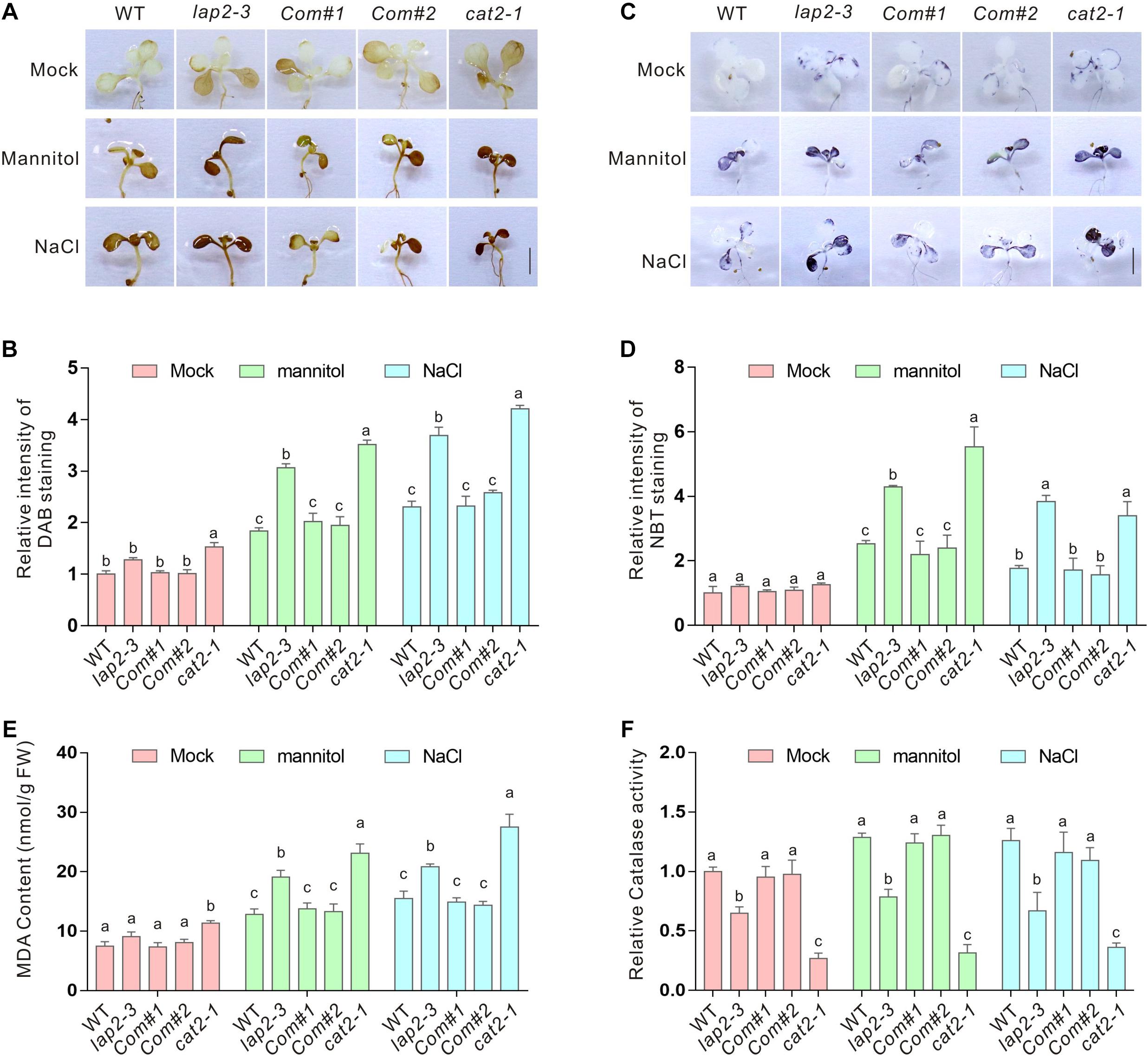
Figure 3. The lap2-3 mutant has higher ROS accumulation and reduced catalase activity. (A) The DAB staining images of the leaves from 5-days-old wild-type, lap2-3, LAP2 complementation lines and cat2-1 mutant seedlings treated with 250 mM mannitol or 125 mM NaCl for 5 days. Bars = 0.5 cm. (B) The relative DAB staining intensity in panel (A). The DAB staining intensity of wild-type leaves without treatment was set to 1. (C) The NBT staining images of the leaves from 5-days-old wild-type, lap2-3, LAP2 complementation lines and cat2-1 mutant seedlings treated with 250 mM mannitol or 125 mM NaCl for 5 days. Bars = 0.5 cm. (D) The relative NBT staining intensity in panel (C). The NBT staining intensity of wild-type leaves without treatment was set to 1. (E) The MDA content of 5-days-old wild-type, lap2-3, LAP2 complementation lines and cat2-1 mutant seedlings treated with 250 mM mannitol or 125 mM NaCl for 5 days. (F) The catalase activities in 5-days-old wild-type, lap2-3, LAP2 complementation lines and cat2-1 mutant seedlings treated with 250 mM mannitol or 125 mM NaCl for 5 days. The catalase activity of the wild type without treatment was set to 1. Data are means (±SD) of three biological replicates. Bars with different letters in the same treatment indicate significant differences at p < 0.05, revealed using one-way ANOVA with Tukey’s multiple comparison test.
Leucine Aminopeptidase2 Increases CAT2 Protein Stability to Promote Plant Catalase Activity
Our above results showed a role of LAP2 in promoting plant catalase activity, raising a possibility that LAP2 may affect CAT2 expression. Thus, we first assayed the transcripts of CAT2 in the wild-type and lap2-3 mutant seedlings treated with high concentrations of salt or mannitol using qRT-PCR. Our results showed that high salinity or osmotic stress-induced CAT2 expression in the lap2-3 mutant was not lower than that in the wild type (Figure 4A), excluding the possibility of LAP2-mediated regulation of CAT2 transcription (Figure 4A). Next, we detected the CAT2 protein levels in the wild-type and lap2-3 mutant plants using anti-CAT2 antibody which was previously reported (Zhang et al., 2020b), and our results showed that CAT2 protein accumulation in the mutant was lower than that in the wild type either treated without or with salt or osmotic stress (Figure 4B), suggesting a role of LAP2 in the regulation of CAT2 protein stability. To do this, we examined the changes of CAT2 protein accumulation in the wild-type and lap2-3 mutant plants treated with the protein synthesis inhibitor CHX and the 26S proteasome inhibitor MG132. Our results showed that CAT2 protein level in the lap2-3 mutant seedlings was dramatically decreased in the presence of CHX compared with that in the wild type, while MG132 significantly represses the degradation of CAT2 protein both in the mutant and wild-type plants (Figure 4C), revealing that LAP2 is necessary for CAT2 protein stability in plants.
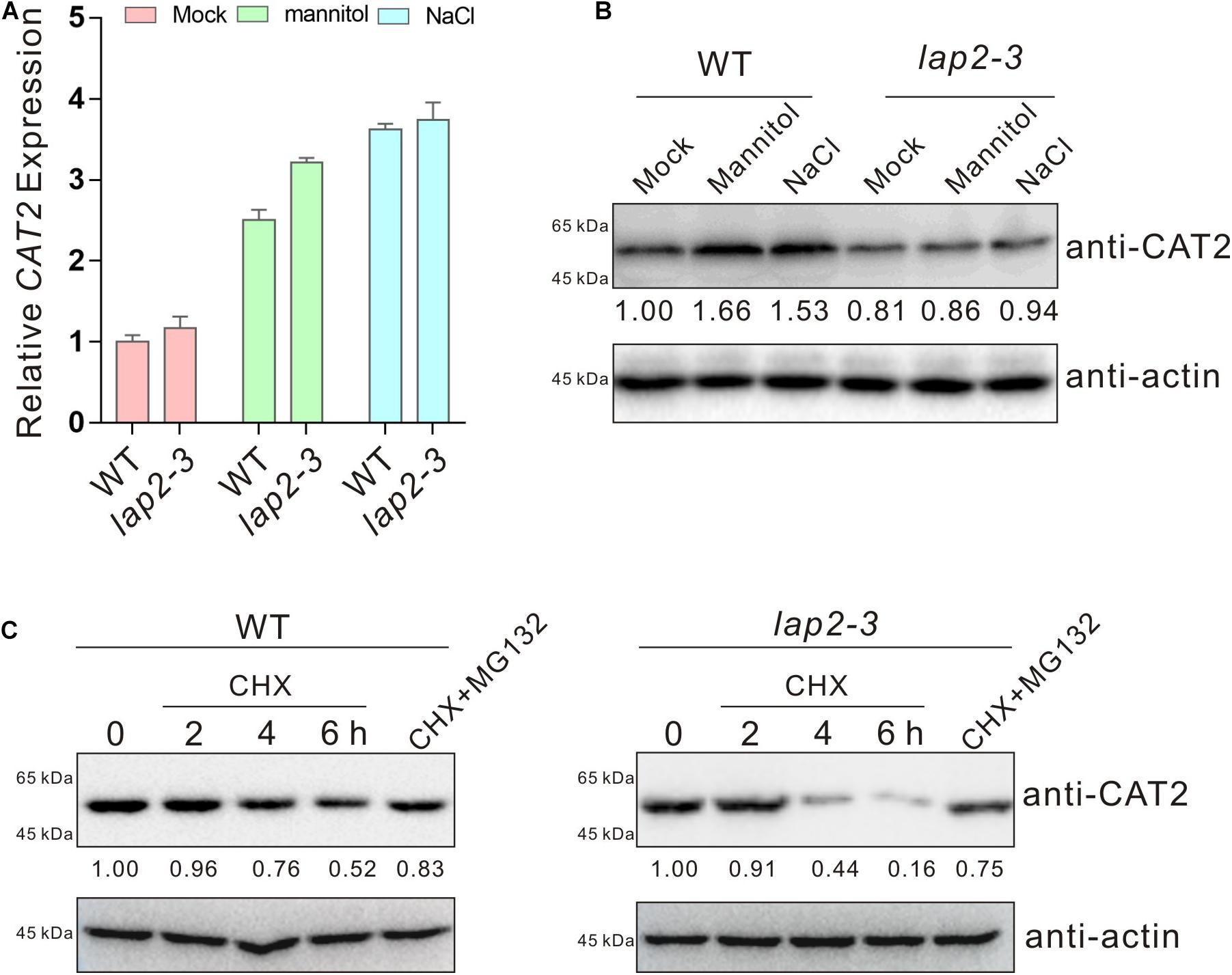
Figure 4. Leucine aminopeptidase2 increases CAT2 protein stability. (A) The expression of CAT2 in 5-days-old wild-type and lap2-3 mutant seedlings treated with 250 mM mannitol or 125 mM NaCl for 5 days assayed by qRT-qPCR. The expression of CAT2 in the untreated wild type was set as 1. Data are means (±SD) of three biological replicates. (B) CAT2 protein accumulation in 5-days-old wild-type and lap2-3 mutant seedlings treated with 250 mM mannitol or 125 mM NaCl for 5 days using anti-CAT2 antibody. CAT2 protein level in the untreated wild type was set as 1. Actin was used as a loading control. (C) Immunoblot analysis of CAT2 protein accumulation in 5-days-old wild-type and lap2-3 mutant seedlings incubated with 50 μM CHX for 2, 4, or 6 h or with 50 μM CHX plus 100 μM MG132 for 6 h using anti-CAT2 antibody. CAT2 protein level in the untreated wild type or lap2-3 mutant was set as 1. Actin was used as a loading control.
If reduced stress tolerance of the lap2-3 mutant was really due to ROS overaccumulation and catalase activity deficiency, scavenging the excess ROS or overexpressing CAT2 to increase CAT2 catalase activity should rescue the phenotype of the mutant. To test this, GSH, a widely used reducing reagent that can lower down cellular ROS accumulation, was employed (Qin et al., 2020; Zhang et al., 2020b). We found that exogenously applied GSH significantly increased the tolerance of the lap2-3 mutant plant to salt or osmotic stress in terms of green cotyledon rate and fresh weight (Figures 5A,B). Furthermore, we generated 35S:GFP–CAT2 lap2-3 transgenic plants by overexpressing CAT2 in the mutant (Supplementary Figure 1C) and tested their stress tolerance. Our results showed that 35S:GFP–CAT2 lap2-3 displayed a more tolerant phenotype under salt or osmotic stress conditions compared with the lap2-3 mutant (Figures 5C,D). Together, these above results indicated that LAP2 functions in salt- or mannitol-induced oxidative stress through increasing CAT2 protein stability.
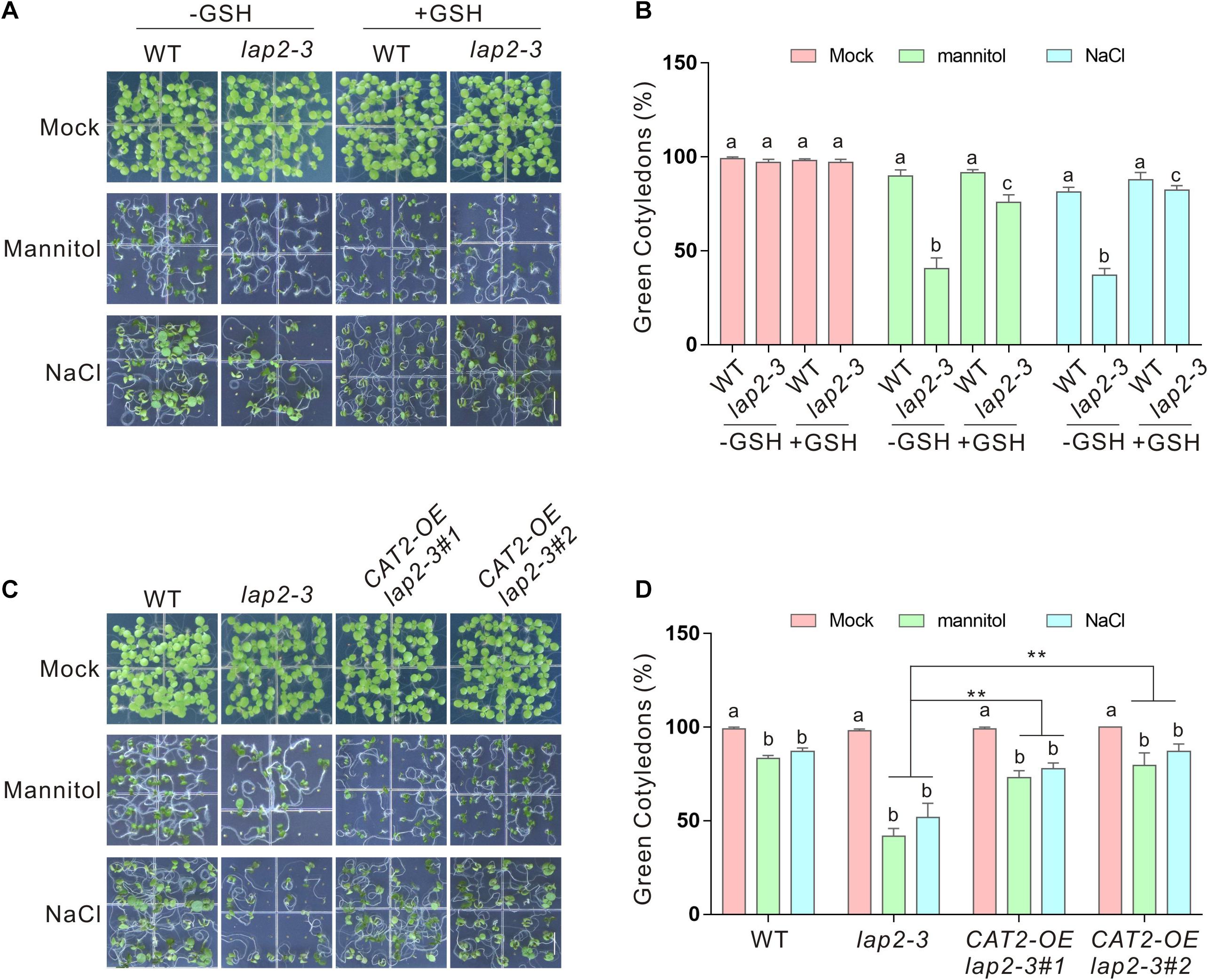
Figure 5. Glutathione (GSH) or overexpressing CAT2 rescues the hypersensitivity of the lap2-3 mutant to salt or osmotic stress. (A) Images of 5-days-old wild-type and lap2-3 mutant seeds germinated on 1/2 MS medium without or with 250 mM mannitol or 125 mM NaCl in the presence or absence of 100 μM GSH. Bars = 0.5 cm. (B) Green cotyledon percentage of the wild type and lap2-3 mutant in panel (A). (C) Images of 5-days-old wild-type, lap2-3, and 35S:GFP-CAT2 lap2-3 (CAT2-OE lap2-3) transgenic plant seeds germinated on 1/2 MS medium without or with 250 mM mannitol or 125 mM NaCl. Bars = 0.5 cm. (D) Green cotyledon percentage of the wild-type, lap2-3, and CAT2-OE lap2-3 plants in panel (C). Data are means (±SD) of three biological replicates. Bars with different letters in the same treatment indicate significant differences at p < 0.05, revealed using one-way ANOVA with Tukey’s multiple comparison test. Asterisks indicate significant differences using Student’s t test (**p < 0.01).
CAT2 Facilitates LAP2 Hydrolysis Activity
The above results indicate that LAP2, as a molecular chaperone, promotes CAT2 protein stability so as to confer plant-enhanced salt or osmotic stress. Considering their physical interaction, we wondered whether CAT2 affects LAP2 activity in turn. Therefore, His-tagged CAT2 and GST-tagged LAP2 proteins were expressed and purified from E. coli BL21 (DE3), and Leu-MCA was used as a synthetic substrate of LAP2 according to a previous report where Leu-MCA was the optimal substrate among various synthetic aminoacyl-MCAs (Waditee-Sirisattha et al., 2011). Our enzyme kinetics experiment showed that no significant changes of fluorescence signal were detected in the reaction mixture containing Leu-MCA when in the absence of LAP2 or in the presence of CAT2 alone, while fluorescence signal was strongly induced over time when LAP2 was added (Figures 6A,B). Interestingly, fluorescence signal was much higher in the presence of both CAT2 and LAP2, revealing that CAT2 stimulates LAP2 hydrolysis activity. Additionally, when more CAT2 protein was added, fluorescence signal elicited by LAP2 activity with Leu-MCA as the substrate was further increased (Figures 6A,B), indicating that CAT2 promotes LAP2 activity in a dosage-dependent manner in vitro.
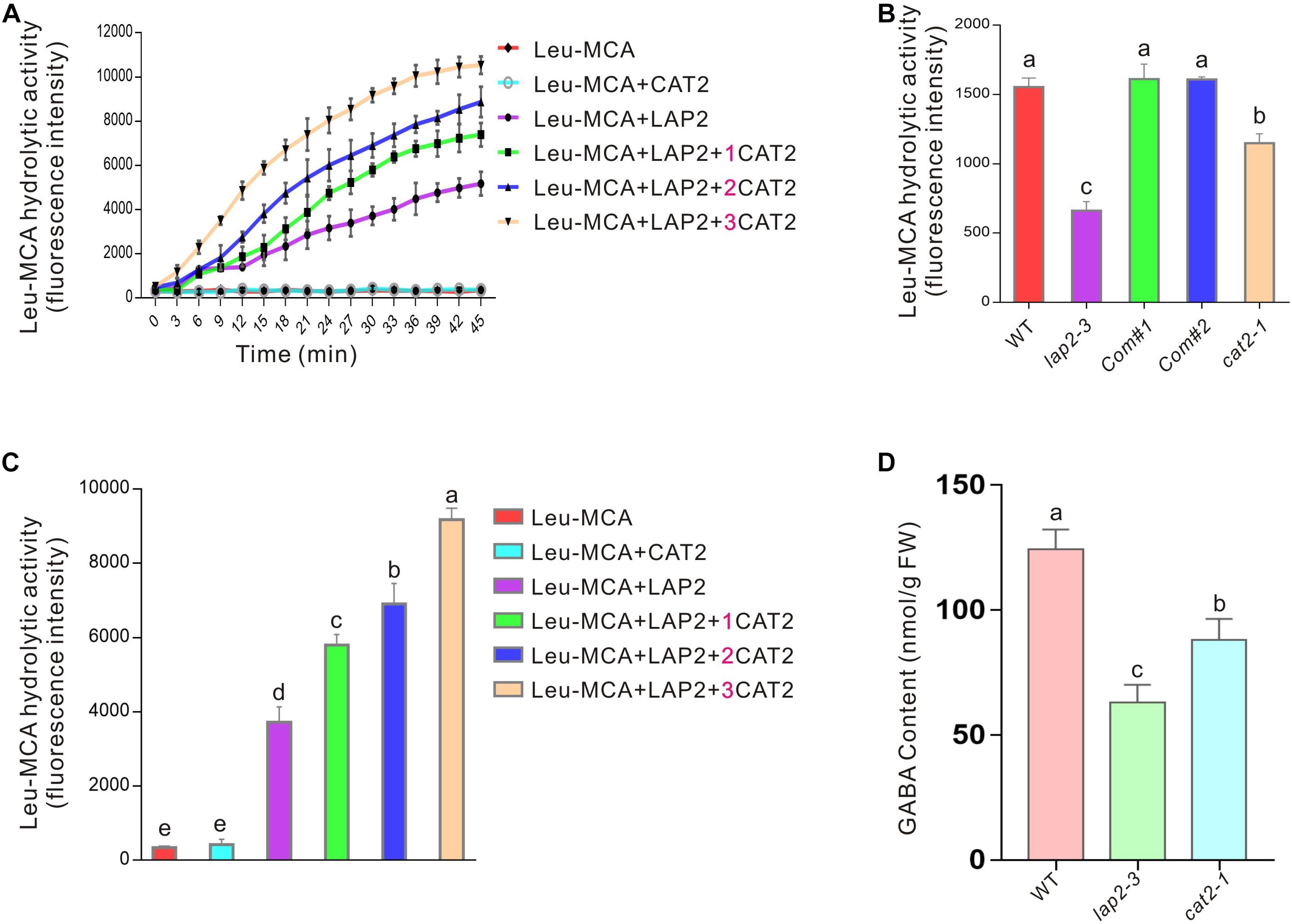
Figure 6. CAT2 promotes LAP2 activity. (A) The kinetics experiment of LAP2 hydrolysis activity with Leu-MCA as the substrate in the presence or absence of CAT2 for 45 min; 1 μg of purified LAP2 protein and different amounts of purified CAT2 protein (0, 1, 2, or 3 μg) were added in the reaction mixture. (B) The fluorescence intensities of the above experiment (A) at 30 min were analyzed. (C) The leucine peptidase activity of 10-days-old wild-type, lap2-3, LAP2 complementation lines and cat2-1 mutant seedlings with Leu-MCA as the substrate. (D) GABA content of 10-days-old wild-type, lap2-3, LAP2 complementation lines and cat2-1 mutant seedlings. Data are means (±SD) of three biological replicates. Bars with different letters indicate significant differences at p < 0.05, revealed using one-way ANOVA with Tukey’s multiple comparison test.
The effect of CAT2 on LAP2 activity was further verified by monitoring the leucine aminopeptidase activities of the wild-type lap2-3 and cat2-1 mutant plants. Our results showed that both lap2-3 and cat2-1 harbored a lower leucine aminopeptidase activity toward the substrate Leu-MCA compared with the wild type (Figure 6C), supporting the stimulative effect of CAT2 on LAP2 activity in vivo. It is also reported that LAP2 regulates amino acid turnover and the metabolism of a vital signaling molecule, GABA, in Arabidopsis (Waditee-Sirisattha et al., 2011). Thus, we further examined the GABA content in the wild-type lap2-3 and cat2-1 mutant plants and found that, similar to the lap2-3 mutant, the cat2-1 mutant also had lower GABA content than the wild type (Figure 6D).
In summary, our study revealed that LAP2 promotes CAT2 protein stability, while CAT2 stimulates LAP2 hydrolysis activity in turn, together contributing to plant tolerance to salt or osmotic stress-induced oxidative damages.
Discussion
Catalases are ancient and conservative antioxidant enzymes across various species, catalyzing the dismutation of H2O2 to oxygen and water (Hu et al., 2010; Su et al., 2018). In contrast with other peroxidases with H2O2 as substrate, catalases do not need any reducing co-factors including ascorbate and GSH for their catalytic activity, but this may also make catalases themselves more vulnerable to excess ROS (Hu et al., 2010; Mhamdi et al., 2012; Li et al., 2015). For example, it is reported that pathogen infection-induced ROS overaccumulation results in dysfunction of catalase through direct oxidative modification (Kneeshaw et al., 2017). In this study, we identified LAP2 as a new interacting protein of CAT2 and also found that LAP2 increases CAT2 protein stability through their interaction, raising a possibility that LAP2 may act as a molecular chaperone to protect CAT2 from oxidative damages or maintain its structure in a functional status. Similar to this, NCA1 increases CAT2 activity possibly through interacting with CAT2 and facilitating its tetramer assembly (Li et al., 2015). Another CAT2-interacting protein, LSD1, is also required for CAT2 activity as its loss-of-function mutant has impaired catalase activity and excess ROS (Li et al., 2013), whereas the underlying mechanism remains unclear. We have noticed that many catalase-interacting proteins have been identified including NCA1, LSD1, CPK8, MeHSP90.9, and Hsp17.6II in previous reports as well as LAP2 in this study, and they play roles in the plant’s response and tolerance to salt, osmotic, and/or drought stresses (Figure 7). These reports unravel the mechanisms underlying the regulation of ROS homeostasis and catalase activity in plant salt or osmotic stress responses.
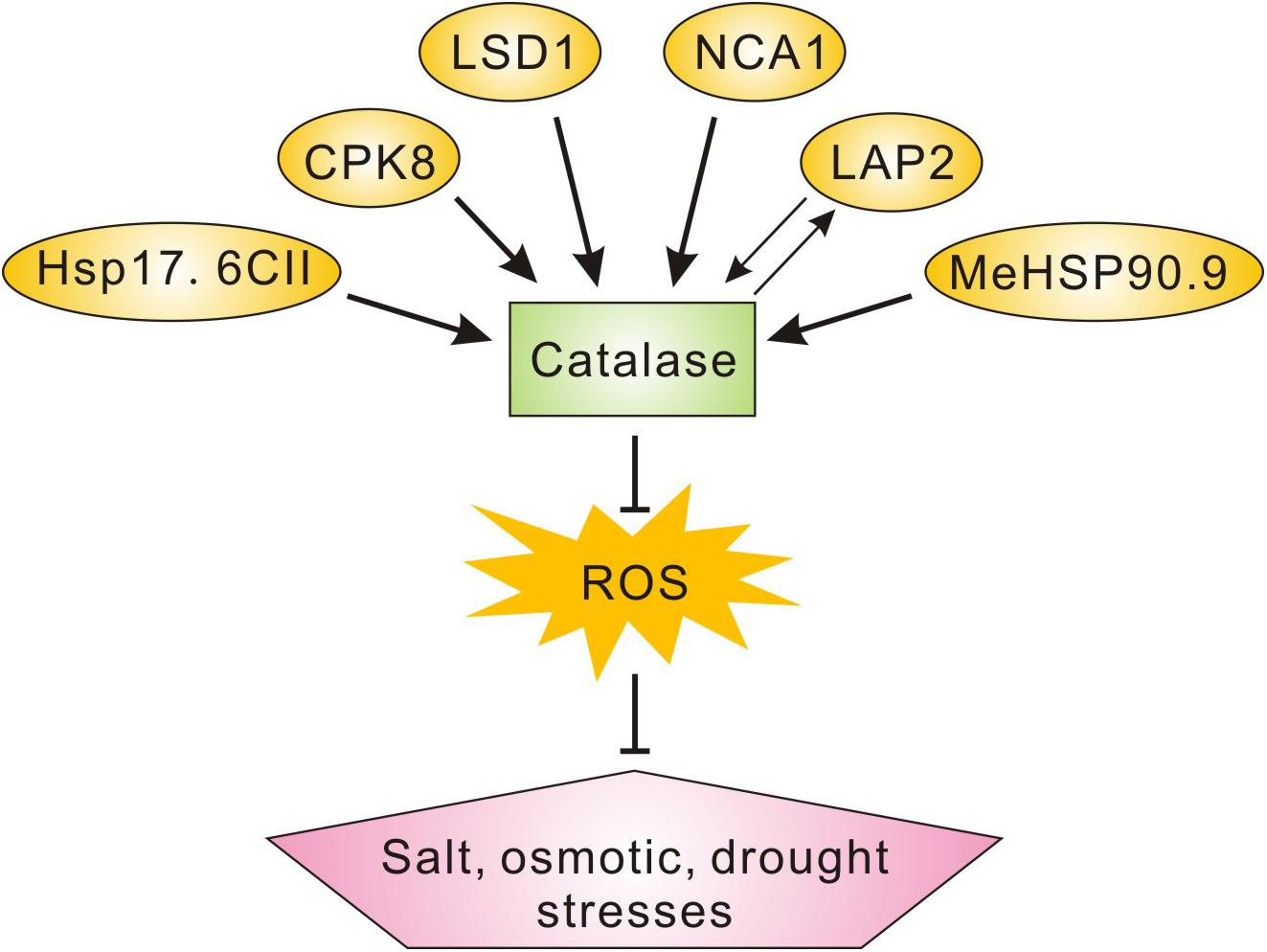
Figure 7. A scheme showing the catalase-interacting proteins involved in plant salt, osmotic, or drought stress tolerance. Catalase-interacting proteins including NCA1, LSD1, CPK8, MeHSP90.9, and Hsp17.6II in previous reports as well as LAP2 in this study play roles in plant salt, osmotic, and/or drought stresses tolerance through the regulation of catalase activity and ROS homeostasis.
Our study also showed that CAT2 could stimulate LAP2 hydrolysis activity, affecting LAP2-mediated regulation of the GABA metabolism. However, we still do not know how CAT2 affects LAP2 activity. Previous reports showed that the peptidase activity of human dipeptidyl peptidase 8 and 9 was severely inhibited following H2O2 treatment, and moreover, reducing agents completely reversed this oxidation-mediated enzyme activity abrogation (Park et al., 2008); in addition, human dipeptidyl peptidase III could be inactivated by oxidized glutathione (GSSH), and the oxidation of the cysteine 176 participated in this enzyme inhibition (Karacic et al., 2012). These reports revealed that peptidases may be ROS-sensitive enzymes and their hydrolysis activity requires a reducing environment. In line with this, we previously also reported a similar stimulative effect of CAT2 on acyl-CoA oxidase (ACX) 2 and ACX3 activity in the plant’s response to necrotrophic pathogen infection or during postgerminative seedling growth (Liu et al., 2017; Yuan et al., 2017). We speculated that, on the one hand, CAT2 creates a reducing environment for these enzymes by scavenging H2O2 and, thus, protects them from oxidative damages; on the other hand, CAT2 may affect their protein structure through interaction so as to promote their activity. These results suggest a novel role of CAT2 in the modulation of protein activity as a regulator, in addition to its H2O2-decomposing function.
We also noticed that LAP2 mutant leaves exhibit an early-senescence phenotype, which is similar with the cat2-1 mutant, further supporting a tight correlation between CAT2 and LAP2 in plant-regulating growth and stress responses. Besides, we previously reported that jasmonic acid (JA) represses CAT2 expression to increase H2O2 accumulation, accelerating leaf senescence (Zhang et al., 2020b). Thus, we speculated that JA-mediated repression of CAT2 expression may also disturb LAP2 activity, resulting in changes of metabolite flux in the leaves and, consequently, the occurrence of senescence. In addition to high salinity and osmotic stresses as we reported in this study, LAP2 may also function in plant responses to other environmental stresses such as alkaline pH, high-intensity light, and low temperature through interacting with CAT2.
In summary, the results presented in this study demonstrate that LAP2 interacts with CAT2 and increases its protein stability, thus functioning in plant responses to ROS accumulation caused by high salinity or osmotic stress; also, CAT2 promotes LAP2 hydrolysis activity in turn, thus affecting GABA metabolism and plant tolerance to stresses.
Data Availability Statement
The raw data supporting the conclusions of this article will be made available by the authors, without undue reservation.
Author Contributions
W-CL, YZ, and L-FW designed the research. YZ, L-FW, and T-TL performed the research. YZ and L-FW analyzed the data. W-CL wrote the manuscript with contribution and approval from all authors. All authors contributed to the article and approved the submitted version.
Funding
This work was supported by National Natural Science Foundation of China (#32000150) and the Program for Innovative Research Team (in Science and Technology) in University of Henan Province (21IRTSTHN019).
Conflict of Interest
The authors declare that the research was conducted in the absence of any commercial or financial relationships that could be construed as a potential conflict of interest.
Supplementary Material
The Supplementary Material for this article can be found online at: https://www.frontiersin.org/articles/10.3389/fpls.2021.672672/full#supplementary-material
Supplementary Figure 1 | Identification of lap2-3 mutant and complementation lines. (A) Genomic characterization of T-DNA insertion lines of lap2-3 (SAIL_192_A08) with the wild type as control. Primers used for PCR were listed in Supplementary Table 1. (B) The expression of LAP2 in the wild-type, lap2-3 and complementation lines were analyzed by RT-PCR. Actin was used as an internal control. Primers used for RT-PCR were listed in Supplementary Table 1. (C) The expression of CAT2 in the wild-type, lap2-3 and 35S:GFP-CAT2 lap2-3 (CAT2-OE lap2-3) lines were assayed by qRT-PCR. The expression of CAT2 in the wild type was set as 1. Data are means (±SD) of three biological replicates.
References
Bueso, E., Alejandro, S., Carbonell, P., Perez-Amador, M. A., Fayos, J., Belles, J. M., et al. (2007). The lithium tolerance of the Arabidopsis cat2 mutant reveals a cross-talk between oxidative stress and ethylene. Plant J. 52, 1052–1065. doi: 10.1111/j.1365-313x.2007.03305.x
Gondim, F. A., Gomes-Filho, E., Costa, J. H., Mendes Alencar, N. L., and Prisco, J. T. (2012). Catalase plays a key role in salt stress acclimation induced by hydrogen peroxide pretreatment in maize. Plant Physiol. Biochem. 56, 62–71. doi: 10.1016/j.plaphy.2012.04.012
Guan, Q. M., Lu, X. Y., Zeng, H. T., Zhang, Y. Y., and Zhu, J. H. (2013). Heat stress induction of miR398 triggers a regulatory loop that is critical for thermotolerance in Arabidopsis. Plant J. 74, 840–851. doi: 10.1111/tpj.12169
Hu, Y. Q., Liu, S., Yuan, H. M., Li, J., Yan, D. W., Zhang, J. F., et al. (2010). Functional comparison of catalase genes in the elimination of photorespiratory H2O2 using promoter- and 3’-untranslated region exchange experiments in the Arabidopsis cat2 photorespiratory mutant. Plant Cell Environ. 33, 1656–1670. doi: 10.1111/j.1365-3040.2010.02171.x
Karacic, Z., Spoljaric, J., Rozman, M., and Abramic, M. (2012). Molecular determinants of human dipeptidyl peptidase III sensitivity to thiol modifying reagents. Biol. Chem. 393, 1523–1532. doi: 10.1515/hsz-2012-0181
Kneeshaw, S., Keyani, R., Delorme-Hinoux, V., Imrie, L., Loake, G. J., Le Bihan, T., et al. (2017). Nucleoredoxin guards against oxidative stress by protecting antioxidant enzymes. Proc. Natl. Acad. Sci. U.S.A. 114, 8414–8419. doi: 10.1073/pnas.1703344114
Li, G., Li, J., Hao, R., and Guo, Y. (2017). Activation of catalase activity by a peroxisome-localized small heat shock protein Hsp17.6CII. J. Genet. Genomics 44, 395–404. doi: 10.1016/j.jgg.2017.03.009
Li, J., Liu, J., Wang, G., Cha, J. Y., Li, G., Chen, S., et al. (2015). A chaperone function of NO CATALASE ACTIVITY1 is required to maintain catalase activity and for multiple stress responses in Arabidopsis. Plant Cell 27, 908–925. doi: 10.1105/tpc.114.135095
Li, Y., Chen, L., Mu, J., and Zuo, J. (2013). LESION SIMULATING DISEASE1 interacts with catalases to regulate hypersensitive cell death in Arabidopsis. Plant Physiol. 163, 1059–1070. doi: 10.1104/pp.113.225805
Liu, W. C., Han, T. T., Yuan, H. M., Yu, Z. D., Zhang, L. Y., Zhang, B. L., et al. (2017). CATALASE2 functions for seedling postgerminative growth by scavenging H2 O2 and stimulating ACX2/3 activity in Arabidopsis. Plant Cell Environ. 40, 2720–2728. doi: 10.1111/pce.13031
Liu, W. C., Zheng, S. Q., Yu, Z. D., Gao, X., Shen, R., and Lu, Y. T. (2018). WD40-REPEAT 5a represses root meristem growth by suppressing auxin synthesis through changes of nitric oxide accumulation in Arabidopsis. Plant J. 93, 883–893. doi: 10.1111/tpj.13816
Liu, Y., and He, C. (2016). Regulation of plant reactive oxygen species (ROS) in stress responses: learning from AtRBOHD. Plant Cell Rep. 35, 995–1007. doi: 10.1007/s00299-016-1950-x
Luna, C. M., Pastori, G. M., Driscoll, S., Groten, K., Bernard, S., and Foyer, C. H. (2005). Drought controls on H2O2 accumulation, catalase (CAT) activity and CAT gene expression in wheat. J. Exp. Bot. 56, 417–423. doi: 10.1093/jxb/eri039
Mhamdi, A., Noctor, G., and Baker, A. (2012). Plant catalases: peroxisomal redox guardians. Arch. Biochem. Biophys. 525, 181–194. doi: 10.1016/j.abb.2012.04.015
Park, J., Knott, H. M., Nadvi, N. A., Collyer, C. A., and Gorrell, M. D. (2008). Reversible inactivation of human dipeptidyl peptidases 8 and 9 by oxidation. Open Enzyme Inhib. J. 1, 52–61. doi: 10.2174/1874940200801010052
Pautot, V., Holzer, F. M., Chaufaux, J., and Walling, L. L. (2001). The induction of tomato leucine aminopeptidase genes (LapA) after Pseudomonas syringae pv. tomato infection is primarily a wound response triggered by coronatine. Mol. Plant Microb. Interact. 14, 214–224. doi: 10.1094/mpmi.2001.14.2.214
Qin, X., Duan, Z., Zheng, Y., Liu, W. C., Guo, S., Botella, J. R., et al. (2020). ABC1K10a, an atypical kinase, functions in plant salt stress tolerance. BMC Plant Biol. 20:270. doi: 10.1186/s12870-020-02467-4
Sanchez-McSweeney, A., Gonzalez-Gordo, S., Aranda-Sicilia, M. N., Rodriguez-Rosales, M. P., Venema, K., Palma, J. M., et al. (2021). Loss of function of the chloroplast membrane K(+)/H(+) antiporters AtKEA1 and AtKEA2 alters the ROS and NO metabolism but promotes drought stress resilience. Plant Physiol. Biochem. 160, 106–119. doi: 10.1016/j.plaphy.2021.01.010
Scranton, M. A., Yee, A., Park, S. Y., and Walling, L. L. (2012). Plant leucine aminopeptidases moonlight as molecular chaperones to alleviate stress-induced damage. J. Biol. Chem. 287, 18408–18417. doi: 10.1074/jbc.m111.309500
Sharma, P., and Dubey, R. S. (2005). Drought induces oxidative stress and enhances the activities of antioxidant enzymes in growing rice seedlings. Plant Growth Regul. 46, 209–221. doi: 10.1007/s10725-005-0002-2
Shi, H. T., Li, R. J., Cai, W., Liu, W., Wang, C. L., and Lu, Y. T. (2012). Increasing nitric oxide content in Arabidopsis thaliana by expressing rat neuronal nitric oxide synthase resulted in enhanced stress tolerance. Plant Cell Physiol. 53, 344–357. doi: 10.1093/pcp/pcr181
Su, T., Wang, P., Li, H., Zhao, Y., Lu, Y., Dai, P., et al. (2018). The Arabidopsis catalase triple mutant reveals important roles of catalases and peroxisome-derived signaling in plant development. J. Integr. Plant Biol. 60, 591–607. doi: 10.1111/jipb.12649
Umeno, A., Biju, V., and Yoshida, Y. (2017). In vivo ROS production and use of oxidative stress-derived biomarkers to detect the onset of diseases such as Alzheimer’s disease, Parkinson’s disease, and diabetes. Free Radic. Res. 51, 413–427. doi: 10.1080/10715762.2017.1315114
Waditee-Sirisattha, R., Shibato, J., Rakwal, R., Sirisattha, S., Hattori, A., Nakano, T., et al. (2011). The Arabidopsis aminopeptidase LAP2 regulates plant growth, leaf longevity and stress response. New Phytol. 191, 958–969. doi: 10.1111/j.1469-8137.2011.03758.x
Waszczak, C., Carmody, M., and Kangasjarvi, J. (2018). Reactive oxygen species in plant signaling. Annu. Rev. Plant Biol. 69, 209–236.
Wei, Y., Liu, W., Hu, W., Yan, Y., and Shi, H. (2020). The chaperone MeHSP90 recruits MeWRKY20 and MeCatalase1 to regulate drought stress resistance in cassava. New Phytol. 226, 476–491. doi: 10.1111/nph.16346
Xing, Y., Jia, W., and Zhang, J. (2007). AtMEK1 mediates stress-induced gene expression of CAT1 catalase by triggering H2O2 production in Arabidopsis. J. Exp. Bot. 58, 2969–2981. doi: 10.1093/jxb/erm144
Yang, Y., and Guo, Y. (2018). Elucidating the molecular mechanisms mediating plant salt-stress responses. New Phytol. 217, 523–539. doi: 10.1111/nph.14920
Yu, F., Cao, X., Liu, G., Wang, Q., Xia, R., Zhang, X., et al. (2020). ESCRT-I component VPS23A Is targeted by E3 ubiquitin ligase XBAT35 for proteasome-mediated degradation in modulating ABA signaling. Mol. Plant 13, 1556–1569. doi: 10.1016/j.molp.2020.09.008
Yuan, H. M., Liu, W. C., and Lu, Y. T. (2017). CATALASE2 coordinates SA-mediated repression of both auxin accumulation and JA biosynthesis in plant defenses. Cell Host Microb. 21, 143–155. doi: 10.1016/j.chom.2017.01.007
Yue, J., Du, C., Ji, J., Xie, T., Chen, W., Chang, E., et al. (2018). Inhibition of alpha-ketoglutarate dehydrogenase activity affects adventitious root growth in poplar via changes in GABA shunt. Planta 248, 963–979. doi: 10.1007/s00425-018-2929-3
Zhang, Q., Cai, W., Ji, T. T., Ye, L., Lu, Y. T., and Yuan, T. T. (2020a). WRKY13 enhances cadmium tolerance by promoting D-CYSTEINE DESULFHYDRASE and hydrogen sulfide production. Plant Physiol. 183, 345–357. doi: 10.1104/pp.19.01504
Zhang, Y., Ji, T. T., Li, T. T., Tian, Y. Y., Wang, L. F., and Liu, W. C. (2020b). Jasmonic acid promotes leaf senescence through MYC2-mediated repression of CATALASE2 expression in Arabidopsis. Plant Sci. 299:110604. doi: 10.1016/j.plantsci.2020.110604
Zhang, Y., Tian, Y. Y., Wang, L. F., Li, Y. H., Li, T. T., and Liu, W. C. (2020c). WDR5a functions in cadmium-inhibited root meristem growth by regulating nitric oxide accumulation in Arabidopsis. Planta 252:78.
Zou, J. J., Li, X. D., Ratnasekera, D., Wang, C., Liu, W. X., Song, L. F., et al. (2015). Arabidopsis CALCIUM-DEPENDENT PROTEIN KINASE8 and CATALASE3 function in abscisic acid-mediated signaling and H2O2 homeostasis in stomatal guard cells under drought stress. Plant Cell 27, 1445–1460. doi: 10.1105/tpc.15.00144
Keywords: H2O2, CAT2, LAP2, oxidative stress, interaction
Citation: Zhang Y, Wang L-F, Li T-T and Liu W-C (2021) Mutual Promotion of LAP2 and CAT2 Synergistically Regulates Plant Salt and Osmotic Stress Tolerance. Front. Plant Sci. 12:672672. doi: 10.3389/fpls.2021.672672
Received: 26 February 2021; Accepted: 19 April 2021;
Published: 09 June 2021.
Edited by:
Honghong Wu, Huazhong Agricultural University, ChinaReviewed by:
Jiangman He, University of California, Riverside, United StatesMaría C. Romero-Puertas, Departamento de Bioquímica, Biología Celular y Molecular de Plantas, Estación Experimental del Zaidín (EEZ), Spain
Copyright © 2021 Zhang, Wang, Li and Liu. This is an open-access article distributed under the terms of the Creative Commons Attribution License (CC BY). The use, distribution or reproduction in other forums is permitted, provided the original author(s) and the copyright owner(s) are credited and that the original publication in this journal is cited, in accordance with accepted academic practice. No use, distribution or reproduction is permitted which does not comply with these terms.
*Correspondence: Wen-Cheng Liu, liuwencheng@henu.edu.cn
†These authors have contributed equally to this work