- 1Centro de Biotecnología y Genómica de Plantas, Instituto de Investigación y Tecnología Agraria y Alimentaria, Universidad Politécnica de Madrid, Madrid, Spain
- 2Departamento de Biotecnología-Biología Vegetal, Escuela Técnica Superior de Ingeniería Agronómica, Alimentaria y de Biosistemas, Universidad Politécnica de Madrid, Madrid, Spain
Perennial species in the boreal and temperate regions are subject to extreme annual variations in light and temperature. They precisely adapt to seasonal changes by synchronizing cycles of growth and dormancy with external cues. Annual dormancy–growth transitions and flowering involve factors that integrate environmental and endogenous signals. MADS-box transcription factors have been extensively described in the regulation of Arabidopsis flowering. However, their participation in annual dormancy–growth transitions in trees is minimal. In this study, we investigate the function of MADS12, a Populus tremula × alba SUPPRESSOR OF CONSTANS OVEREXPRESSION 1 (SOC1)-related gene. Our gene expression analysis reveals that MADS12 displays lower mRNA levels during the winter than during early spring and mid-spring. Moreover, MADS12 activation depends on the fulfillment of the chilling requirement. Hybrid poplars overexpressing MADS12 show no differences in growth cessation and bud set, while ecodormant plants display an early bud break, indicating that MADS12 overexpression promotes bud growth reactivation. Comparative expression analysis of available bud break-promoting genes reveals that MADS12 overexpression downregulates the GIBBERELLINS 2 OXIDASE 4 (GA2ox4), a gene involved in gibberellin catabolism. Moreover, the mid-winter to mid-spring RNAseq profiling indicates that MADS12 and GA2ox4 show antagonistic expression during bud dormancy release. Our results support MADS12 participation in the reactivation of shoot meristem growth during ecodormancy and link MADS12 activation and GA2ox4 downregulation within the temporal events that lead to poplar bud break.
Introduction
Woody perennial plants have acquired multiple adaptive mechanisms to coordinate their vegetative and reproductive growth with the seasonal weather changes (Cooke et al., 2012; Brunner et al., 2014). In temperate latitudes, trees switch between growth and dormancy at their shoot apical meristems to survive dehydration and freezing stress during winter months (Yordanov et al., 2014). Deciduous woody plants cease meristem activity to establish a dormant state before winter; this is called endodormancy. The meristem is rendered insensitive to growth-promoting signals until the chilling requirement fulfillment (Rohde et al., 2007). The inability to initiate growth clearly distinguishes between endodormancy and the subsequent stage, called ecodormancy, when tree buds recover growth capacity in late winter without showing changes in morphology, maintaining plants cold protected (Groover and Cronk, 2017; Maurya and Bhalerao, 2017). During ecodormancy, the tree has the capacity to resume growth and bud break. Cell elongation of preformed leaves inside the buds precedes new cell divisions (Rohde et al., 2007). Photoperiod and temperature are the main regulatory signals of Populus dormancy establishment and release (Wareing, 1956; Weiser, 1970; Ding and Nilsson, 2016; Singh et al., 2017). Changes in photoperiod are constant every year in a given location, while the temperature is more variable among the years. This variation in the temperature has been exacerbated by global warming, causing significant ecological and economic detriment. Warmer springs substantially advance leaf unfolding and flowering time in perennials. On the contrary, warmer winters compromise the chilling fulfillment, which results in a delay of bud break (Wang H. et al., 2020). A better understanding of the spring phenology's molecular control in perennials is crucial to avoid global warming damages and help perennials resist future climate.
The photoperiodic pathway drives Arabidopsis flowering and poplar shoot growth (Shim et al., 2017; Singh et al., 2017; Triozzi et al., 2018). The CONSTANS/FLOWERING LOCUS T (CO/FT) module conserves the day length measurement function of Arabidopsis and poplar (Yanovsky and Kay, 2002; Bohlenius, 2006). Shoot growth resumption after winter correlates with activation of FT1 and gibberellin (GA) biosynthetic genes (Rinne et al., 2011; Pin and Nilsson, 2012). More specifically, they encode members of the GA3 and GA20 oxidases. GAs correlate with the activation of glucanase GH17s gene to reopen the plasmodesmata channels. They reinitiate the symplastic growth-promoting cell-to-cell signaling within the SAM (Rinne et al., 2011; Tylewicz et al., 2018). In poplar, GAs work parallel to the FT pathway controlling shoot elongation since high levels of bioactive GA during short-day (SD) conditions were sufficient to sustain shoot elongation growth (Eriksson et al., 2015; Singh et al., 2018). Furthermore, inactivation of GAs in GA 2-OXIDASES (GA2ox) overexpressing (OE) poplars produced extreme tree dwarfism (Zawaski et al., 2011). Additionally, the timing of bud break in poplar requires APETALA2/ethylene-like responsive factor, Early Bud-Break 1 (EBB1), which is a positive regulator of bud break (Yordanov et al., 2014). EBB1 overexpression shows early bud break, and downregulation delayed bud break relative to wild-type (WT) plants (Yordanov et al., 2014). Finally, it is essential to highlight those dynamics in genomic DNA methylation level involved in regulating the dormancy–growth cycle in trees (Santamaría et al., 2009; Conde et al., 2013, 2017a,b). A progressive reduction of genomic DNA methylation in the apex precedes growth in the apical shoot during the bud break. The induction in the apex of a chilling-dependent poplar DEMETER-LIKE 10 (DML10) DNA demethylase causes a global reduction of DNA methylation levels before bud break (Conde et al., 2017a).
Multiple developmental pathways and stress responses in plants include MADS-box transcription factors (TFs), recently reviewed in Castelán-Muñoz et al. (2019). In trees, MADS-box has emerged as a crucial regulator of dormancy–growth transition during vegetative and reproductive development (Rodriguez-A et al., 1994; Hoenicka et al., 2008; Horvath et al., 2010; Leida et al., 2010; Kayal et al., 2011; Klocko et al., 2018; Singh et al., 2018; Falavigna et al., 2019; Wang J. et al., 2020). Genomic study of Prunus persica evergrowing (eve) mutant revealed a deletion of six DORMANCY ASSOCIATED MADS-box (DAM) genes essential for endodormancy maintenance (Rodriguez-A et al., 1994). Consistent with this role of DAM, overexpression of Malus domestica MdDAMb gene showed delayed bud break (Wu et al., 2017). Endodormancy establishment and maintenance in Populus and M. domestica require a gene phylogenetically related to Arabidopsis SHORT VEGETATIVE PHASE (SVP) (Wu et al., 2017; Singh et al., 2018). Opposite to DAM and SVP, heterologous overexpression in poplar of a silver birch FRUITFUL homolog, BpMADS4, prevented bud dormancy (Hoenicka et al., 2008). Seasonal expression studies identified MADS-box homologs Arabidopsis SUPPRESSOR OF OVEREXPRESSION OF CO1 (SOC1) during winter dormancy (Voogd et al., 2015; Kitamura et al., 2016; Wang J. et al., 2020). Arabidopsis SOC1 integrates vernalization, photoperiodic, aging, GAs, and flowering signals and promotes meristematic activity-initiated floral meristems (Moon et al., 2003; Andrés and Coupland, 2012; Immink et al., 2012). SOC1-like homologs display a seasonal expression pattern and form a heterocomplex with SVP and DAM homologs of Arabidopsis, kiwifruit trees, Japanese apricot, and sweet cherry (de Folter et al., 2005; Voogd et al., 2015; Kitamura et al., 2016; Wang J. et al., 2020). Functional analysis of tree SOC1 homologs during growth dormancy transitions is minimal. Nevertheless, Voogd et al. (2015) studied the phenology of kiwifruit AcSOC1i-, AcSOC1e-, and AcSOC1f OE lines, finding that only AcSOC1i presented earlier bud break than control plants. Even though SOC1, SVP, and DAM homologs could coexpress and interact, some members might have unique function during dormancy.
In this study, we investigate the role of the poplar MADS12 gene in the dormancy–growth transition. Our phylogenetic analysis and protein sequence comparison indicate that MADS12 belongs to the SOC1 clade. Our gene expression analysis demonstrates MADS12 induction once chilling is fulfilled, showing a peak of expression before bud break. Moreover, we study the performance of MADS12 OE lines (OE3 and OE5) and WT plants, under phenological assays. Our findings show that hybrid poplar OE MADS12 downregulates GA2ox4 gene and promotes early bud break during ecodormancy.
Materials and Methods
Plant Material and Growth Conditions
The hybrid poplar Populus tremula × alba INRA clone 717 1B4 was used as the experimental model. Poplar plantlets were grown in vitro in Murashige and Skoog (MS) medium 1B (pH 5.7) supplemented with 2% sucrose and with indole acetic and indole butyric acids (0.5 mg/L) containing 0.7% (w/v) plant agar under long-day (LD) 16-h light/8-h dark and 22°C conditions.
For the phenological assays, in vitro-cultivated poplars of WT and four selected independent MADS12 OE lines, OE1, OE3, OE5, and OE7, were transferred to pots containing blond peat, pH 4.5, and grown under LD and 22°C conditions for 3 weeks. The plants were fertilized once every 2 weeks with a solution of 1 g/L of Peters Professional 20-20-20 in LD conditions and 20-10-20 in SD conditions (Comercial Química Massó, Barcelona, Spain). Growth cessation and bud set were induced by exposing plants to SD conditions at 22°C (8-h light/16-h dark) during 8 or 10 weeks. Bud set progression was graded by scoring from stage 3 (fully growing apex) to stage 0 (fully formed apical bud) according to Rohde et al. (2010). Winter conditions were emulated by treating plant under SD 4°C for 4 or 6 weeks to fulfill the chilling requirement. Finally, plants were transferred back to LD 22°C to monitor bud break. The regrowth was scored according to the six developmental stages of bud break (stages 0 to 5) according to Johansson et al. (2014). Phenological assays to evaluate bud break of ecodormant plants were performed twice. In the first round, we assayed MADS12 OE lines OE1, OE5, and OE7 lines and WT. We observed that the highest level of MADS12 expression correlates with the earliest bud break. In the second round, we assayed only the MADS12 OE3 and OE5 lines showing the highest MADS12 expression levels and WT to increase the number of plantlets to make two biological replicates.
Generation of T1 MADS12 Overexpressing Lines
The MADS12 coding region (CDS) was amplified from hybrid poplar using gene-specific primers with attB sites (Supplementary Table 1). For polymerase chain reaction (PCR), Phusion DNA Polymerase (Thermo Fisher Scientific, Massachusetts, USA) was used, and the PCR products were purified and inserted into pDONR207 (Life Technologies, Carlsbad, CA, USA). Insertions in the resulting entry clones were sequence verified. The Gateway cassettes carrying MADS12 CDS were then transferred into the destination binary vectors pGWB15 (Nakagawa et al., 2007). These constructs were transferred into Agrobacterium tumefaciens strain GV3101/pMP90 (Koncz and Schell, 1986). Hybrid poplar was transformed via an Agrobacterium-mediated protocol described previously by Gallardo et al. (1999) with few modifications. Briefly, poplar leaves and stem explants were cultured in minimum inhibitory concentration (MIC) medium, MS medium 1B supplemented with 0.01 mg/L of thidiazuron (TDZ) and 1 mg/L of 2,4-dichlorophenoxyacetic acid in the dark for 48 h. Then explants were placed in an agrobacterium suspension for 15 min and blotted onto sterile filter paper to remove excess bacteria. After 2 days, explants were transferred to MISCT medium composed of MS 1B supplemented with 0.02 mg/L of TDZ, 1 mg/L of 2,4-dichlorophenoxyacetic acid, 250 mg/L of cefotaxime, and 50 mg/mL of kanamycin for 4 weeks in darkness. This medium was used for decontamination and induction of callus growth. After size reached about 0.5 mm2, calli were moved to MIBS medium supplemented with 0.05 mg/mL of alpha-naphthalene acetic acid (NAA), 0.004 mg/L of TDZ, 250 mg/L of cefotaxime, and 50 mg/L of kanamycin to select transformant calli and induce shoot formation. Once shoots grow, they are transferred to MEMS, MS 1B supplemented with 125 mg/L of cefotaxime, kanamycin (50 mg/L), and 0.5 mg/L of indole-3-acetic acid (IAA) to induce root formation. Regenerated plantlets were propagated in MEMS medium and maintained in vitro growth conditions (LD 22°C). MADS12 expression level of individual T1 OE lines was analyzed by reverse transcription-PCR (qRT-PCR).
Plant Material for Gene Expression Studies
To investigate if MADS12 activation is dependent on the fulfillment of the chilling requirements, gene expression analysis was performed on RNA obtained from cuttings collected from five 6-year-old hybrid poplars (P. tremula × alba) growing under natural conditions as reported earlier by Conde et al. (2017a).
Annual expression patterns for poplar MADS12 were initially determined in 2-year-old poplar branches (Populus alba) from adult trees growing under natural conditions in Madrid (Spain) (Conde et al., 2017b).
For comparative gene expression analysis among hybrid poplar MADS12 OE lines, OE3 and OE5, and WT, RNA was obtained from a pool of six ecodormant apical buds grown for 5 days under LD at 22°C, for each biological sample.
Mid-winter to mid-spring transcriptional profiles, used for making heat maps, were collected from hybrid poplar apical bud samples grown in natural conditions in Madrid (Spain). Normalized expression data are available in the gene atlas from Phytozome https://phytozome.jgi.doe.gov/pz/portal.html (Conde et al., 2019).
RNA Extraction, cDNA Synthesis, and qRT-PCR Analysis
RNA extraction was performed adding to frozen bud cetyltrimethylammonium bromide (CTAB) extraction buffer with 2% β-mercaptoethanol at 65°C for 5–8 min, following by three washes with chloroform. After that, 7.5 M LiCl2:50 mM EDTA was added, and samples were precipitated overnight at 4°C. The next day, RNA was collected by centrifuging at 10,000 RPM for 20 min, the supernatant was discarded, and the pellet was resuspended using NR buffer, and RNA was purified according to the manufacturer's instructions of NZY RNA purification kit (NZYTech, Lisboa, Portugal). A total of 500 ng of RNA was retrotranscribed to cDNA using the Maxima First Strand cDNA Synthesis kit from Thermo Scientific (Thermo Fisher Scientific, Massachusetts, USA), and qRT-PCR was performed as described earlier (Ramos-Sánchez et al., 2019). The gene-specific primers used in these studied are listed in Supplementary Table 1.
Phylogenetic Analysis and Sequence Alignment
Arabidopsis protein sequences were obtained from TAIR10 website (www.arabidopsis.org), and Populus trichocarpa protein sequences from (Phytozome v10.3, www.phytozome.net). To construct the phylogenetic tree, protein sequences were aligned with MAFFT E-INS-I algorithm; ProtTest 2.4 was used to select the best evolutionary model (JTT+I+G+F; 5 categories rate; alpha = 1.213); the phylogeny was inferred with RAxML algorithm (perform bootstrap 1000). Tree representation was made using iTol (https://itol.embl.de/) and selecting a bootstrap < 600, and clade coloring was performed according to phylogenetic relationships with Arabidopsis MADS-box genes. Multiple sequence alignment was inferred using ClustalW. MADS-box protein domains were annotated as described in Trainin et al. (2013).
In Silico Analysis of GA2ox4 Promoter
Promoter sequence (2 kb) of GA2ox4 was obtained from ASPENDB (http://aspendb.uga.edu/) and analyzed using the Plant promoter analysis navigator 3.0 (http://plantpan.itps.ncku.edu.tw/). MADS-box TF binding sides were selected and examined to search putative TFs that were identified using phytozome database (https://phytozome.jgi.doe.gov/pz/portal.html).
MADS12 Coexpression Analysis
Genes coexpressed with MADS12 were identified using the Pearson Algorithm on bud mid-winter to mid-spring RNAseq dataset applying correlation coefficient higher than 0.9. We identified 258 genes in apical bud dataset coexpressed with MADS12. Gene expression values for each gene were normalized being the maximum of gene expression 1. From a total of 258 MADS12 coexpressed genes, a total of 242 unique homologs to Arabidopsis genome were identified.
Results
Poplar SOC1 Clade Displays Seven Members
MADS-box TF gene family displays a large number of uncharacterized members in poplar. We investigated the phylogenetic relationship of MADS-box genes in Arabidopsis thaliana and Populus trichocarpa by generating a maximum likelihood phylogenic tree using all annotated poplar and Arabidopsis full-length MADS-box protein sequences. Thirteen major lineages within MIKC-types MADS-box have been resolved and named according to the Arabidopsis gene terminology: FLC/MAF, AP1/FUL, SEP, AGL6, SOC1, AG, AGL12, SVP, AGL15, AGL17, AP3, PI, and TT16. Also, we found two clusters that belong to MIKC-type proteins without any Arabidopsis orthologous (Figure 1A). Even though several SOC1-like genes were associated with dormancy release in trees (Voogd et al., 2015; Kitamura et al., 2016; Wang J. et al., 2020), the poplar SOC1-like members remain uncharacterized during dormancy phenology. We performed protein comparison of identified SOC1-like members of Arabidopsis, poplar, rice, and maize, revealing a high sequence similarity among MADS-box (M) domain and less conserved intervening (I) and keratin-like (K) domains (Supplementary Figure 1). Interestingly, most SOC1-like proteins display conserved C-terminal motif; however, there are members that lack this C-terminal sequence in monocots and dicots (Figure 1B). Together, these analyses indicate that SOC1 members might have originated from a common MIKC-type MADS-box ancestor; nevertheless, they have evolved to a novel C-terminal sequence. Since all Arabidopsis SOC1-like genes promote flowering transition, the SOC1 C-terminal motif is not critical for flowering (Schonrock, 2006; Lee and Lee, 2010; Dorca-Fornell et al., 2011; Pérez-Ruiz et al., 2015).
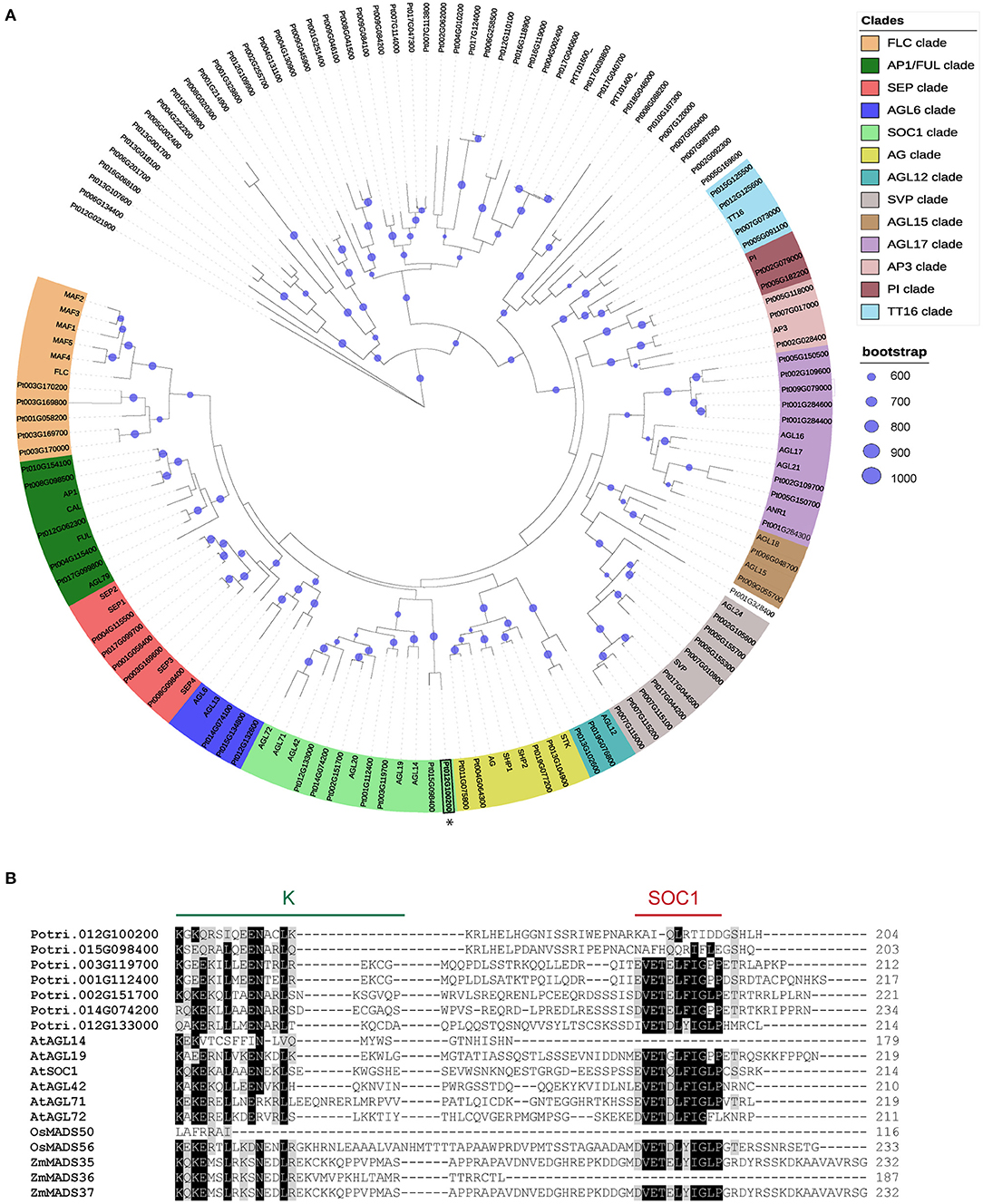
Figure 1. Identification of poplar SOC1-like proteins. (A) Phylogenetic tree analyses of MADS-box proteins from Arabidopsis and poplar. Maximum likelihood tree obtained using the RAxML tool with 1,000 bootstrap replicates. The right panel indicates the color code of the clades and bootstrap values. Clades are named FLOWERING LOCUS C (FLC), APETALA1/FRUITFUL (AP1/FUL), SEPALLATA (SEP), AGAMOUS-LIKE 6 (AGL6), SOC1, AG, AGL12, SVP, AGL15, AGL17, AP3, PISTILLATA (PI), and TRANSPARENT TESTA16 (TT16). (B) MAFF alignment of Populus, Arabidopsis, rice, and maize SOC1-like proteins. The alignment shows part of the K domain (green ink) and the SOC1 motif (red ink). Black boxes indicate identical amino acids. Gray boxes indicate conservative amino acid substitutions.
MADS12 Activation Occurs Once the Chilling Requirement Has Been Fulfilled
To understand the expression pattern of poplar SOC1-like genes during dormancy to growth shift, we studied their temporal expression in the Phytozome public repository, which deposited RNAseq-based gene expression analyses of hybrid poplar apical buds grown under natural conditions (Conde et al., 2019). All these genes display a particular mid-winter to mid-spring pattern of gene expression in shoot apical buds, showing a temporal specialization (Figure 2A). We focused on Potri.012G100200 gene, called MADS12, which shows a low expression level during the winter followed by a transitory expression peak with its maximum at Early Spring f2 (Figure 2A). MADS12 is a spring gene activated during the shoot growth resumption period (Figure 2B). The repression of MADS12 observed during the winter suggested that its activation depends on fulfilling the chilling requirement (Figure 2B). We investigated MADS12 expression in dormant buds of pre-chilling and post-chilling cuttings, after moving them to growth-promoting conditions during 0, 6, and 12 days. Our qRT-PCR analysis confirmed that MADS12 is induced once the chilling requirement has been fulfilled (Figure 2C). Moreover, MADS12 shows its maximal expression at 6 days (83-fold) and decreasing ~50% at 12 days (Figure 2C). However, the expression of CYCLIN C 6 (CYC6) gene, used as marker of cell proliferation (Conde et al., 2017a), peaks at 12 days (Figure 2D), suggesting that MADS12 induction precedes the cell proliferative stage. These results suggest that MADS12 could be involved in the resumption of growth after chilling period.
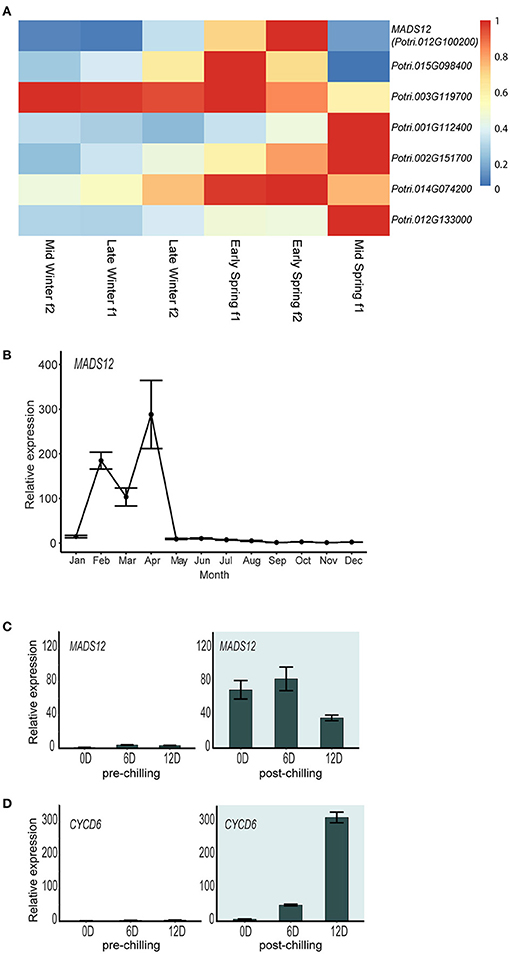
Figure 2. Chilling requirement fulfillment is a prerequisite for MADS12 activation. (A) Heatmap showing normalized expression of hybrid poplar SOC1-like genes from mid-winter to mid-spring in apical buds. Groups are named “early,” “mid,” or “late” when sampling dates were within the first, second, or third month of each season, respectively, following the Northern Meteorological Seasons dates. “f1” or “f2” mean fortnight-1 and fortnight-2 within the month (Conde et al., 2019). (B) MADS12 annual expression pattern measured by qRT-PCR in 2-year-old poplar branches over 1 year. Ubiquitin7 is used as the housekeeping gene. Plotted values and error bars are fold-change means ± s.d. of two biological replicates. Note: MADS12 shows the highest mRNA accumulation during mid-winter–spring period. (C,D) qRT-PCR analysis of MADS12 (C) and CYCD6 (D) genes in dormant shoot apex of pre-chilling and post-chilling cuttings grown under LD and 22°C conditions. Ubiquitin7 is used as the housekeeping gene. Plotted values and error bars are fold-change means ± s.d. of two biological replicates. The x-axis scale is indicated in days. Pre-chilling and post-chilling data are shown in white and green backgrounds, respectively.
MADS12 Overexpression Promotes Shoot Growth Reactivation of Ecodormant Plants
To assess the functional role of MADS12 during dormancy–growth cycles, we generated 10 independent OE hybrid poplar lines, characterizing their MADS12 expression levels by qRT-PCR (Supplementary Figure 2). Because of the highest MADS12 expression level, we selected OE3 and OE5 lines to examine the growth and apical bud phenology in growth chambers under photoperiodic and temperature conditions that mimic seasonal transitions autumn–winter–spring (Rohde et al., 2010). Phenology of WT and MADS12 OE3 and OE5 genotypes did not show any differences in growth cessation and bud set, indicating that MADS12 overexpression does not impair these transitions (Supplementary Figures 3A,B).
To evaluate if MADS12 OE plants show changes in endodormancy release, we subjected those plants to 8 weeks of SDs at 22°C followed by 4 or 6 weeks of SD at 4°C. Subsequently, we shifted them to LDs at 22°C for monitoring their bud break (Conde et al., 2017a). Noticeably, all genotypes tested remained dormant under growth-promoting conditions after 4 weeks of SD at 4°C, although they resumed growth after 6 weeks of SD at 4°C (Supplementary Figures 3A,C). We observed no differences in bud break scores among genotypes, suggesting that chilling requirement fulfillment caused an equal reactivation of shoot growth (Figures 3A,B). Once trees entered in endodormancy, WT and OE plants have same chilling requirement, and MADS12 overexpression does not induce endodormancy release.
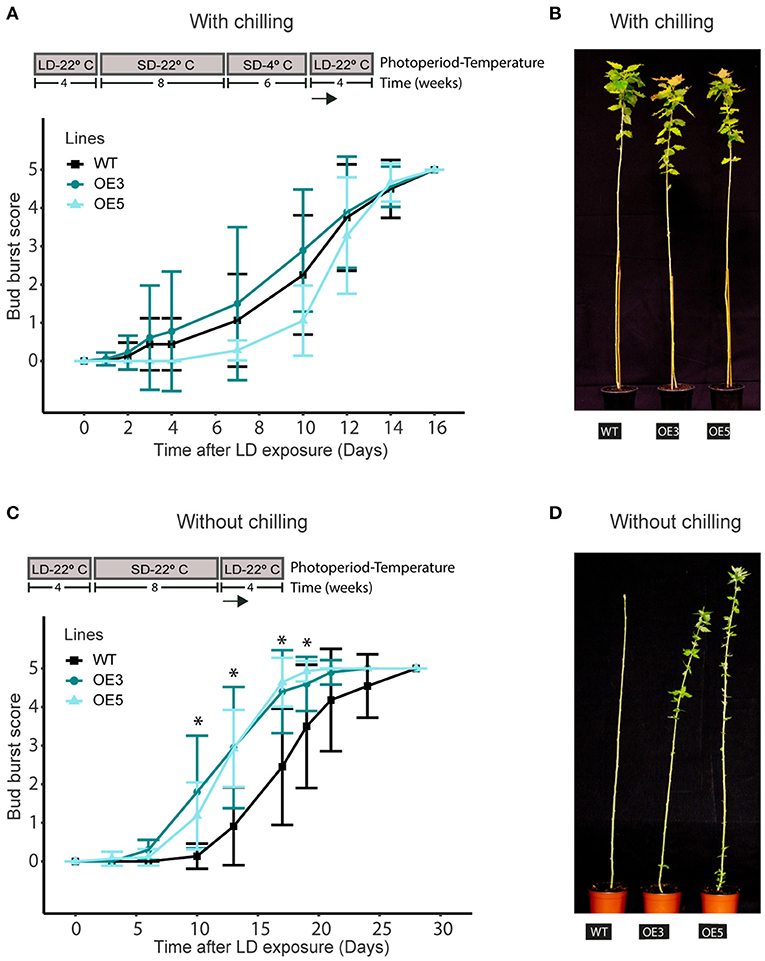
Figure 3. MADS12 overexpression promotes early bud break of ecodormant poplars. Bud break scoring of hybrid poplar MADS12 overexpressing OE3 and OE5 lines and wild type after transferring to LD and 22°C conditions (A,B) with chilling treatment and (C,D) without chilling treatment. (B,D) Representative picture of bud break differences in (B) plants after 15 days of LD exposure and (D) plants after 10 days of LD exposure. Values represent the mean of the bud score measure of n = 10–15 plants. Significant differences between overexpressing (OE) and wild type (WT) were analyzed using Tukey test, *p < 0.05. Top panels indicate photoperiodic and temperature conditions used.
Afterward, we tested whether MADS12 OE could restore growth on plants that had already ceased growing and set buds without any chilling treatment. We arranged a new set of WT, MADS12 OE3, and OE5 plants in LD at 22°C for 4 weeks and placed them under SD at 22°C for 8 or 10 weeks. Then, plants were subjected again to LD at 22°C, and bud break was monitored (Figure 3C and Supplementary Figure 3D). After 10 weeks in SD at 22°C, WT and most MADS12 OE3 and OE5 plants entered endodormancy; therefore, they could not resume growth under favorable environmental conditions without chilling (Supplementary Figure 3D). This observation indicates that MADS12 overexpression does not restrict the plants from reaching the endodormant state and that they could not break endodormancy without chilling. Finally, plants subjected to SD at 22°C conditions for 8 weeks ceased growth and set buds; however, they could not reach the endodormant state since plants resumed total shoot growth under LD at 22°C without chilling (Figure 3C). Significantly, the apical shoot of MADS12 OE3 and OE5 lines restored full growth ~10 days earlier than WT plants (Figures 3C,D). Furthermore, a phenological assay of additional lines confirmed that the lower the MADS12 overexpression level, the lower the differences in bud break observed (Supplementary Figure 4). Collectively, these results indicate that MADS12 overexpression promotes early bud break of ecodormant plants under favorable environmental conditions.
GA2ox4 Is Downregulated in Ecodormant MADS12 Overexpressing Plants
Our phenological assays revealed an accelerated shoot growth resumption of MADS12 OE lines with respect to WT, suggesting an improved growth-stimulating capacity. We hypothesize that MADS12 OE3 and OE5 might differentially express shoot growth-promoting genes with respect to WT. To test this, we collected apical buds of MADS12 OE3, MADS12 OE5, and WT plants treated with 8 weeks of SD at 22°C and exposed during 5 days to LD at 22°C conditions, before the observed phenotypic differences (Figure 3C). In poplar, one of the main factors involved in shoot growth is FT (Bohlenius, 2006; Hsu et al., 2011). qRT-PCR analysis did not show differences in FT1 expression in MADS12 OE3 and MADS12 OE5 lines with respect to WT (Figure 4A). We did not detect FT2 in these bud tissues (data not shown). This result indicates that an FT-independent pathway controls the activation of shoot growth. Bioactive GAs act as an FT parallel pathway to control poplar shoot growth (Eriksson et al., 2015). GA2ox genes cause the inactivation of bioactive GAs (Rieu et al., 2008). Only GA2ox3, GA2ox4, and GA2ox5 are expressed in the apical shoot, and RNAi downregulation of GA2ox4 and GA2ox5 promotes poplar aerial shoot growth (Gou et al., 2011). Our qRT-PCR analysis showed that GA2ox4 and GA2ox5 are downregulated in MADS12 OE3 and OE5 with respect to WT, while only GA2ox4 is found to be significantly repressed (Figure 4A). GA2ox3 shows inconsistent differences in MADS12 OE3 and OE5 with respect to WT (Figure 4A). These results point out that an increase in bioactive GAs could cause the accelerated growth resumption. Accordingly, the mid-winter to mid-spring RNAseq analysis showed an opposite temporal expression pattern, with GA2ox4 and GA2ox5 being greatly downregulated when MADS12 is highly expressed (Figure 4B). This result supports the antagonistic role of MADS12 over GA2ox4 within the temporal events that lead to poplar bud break.
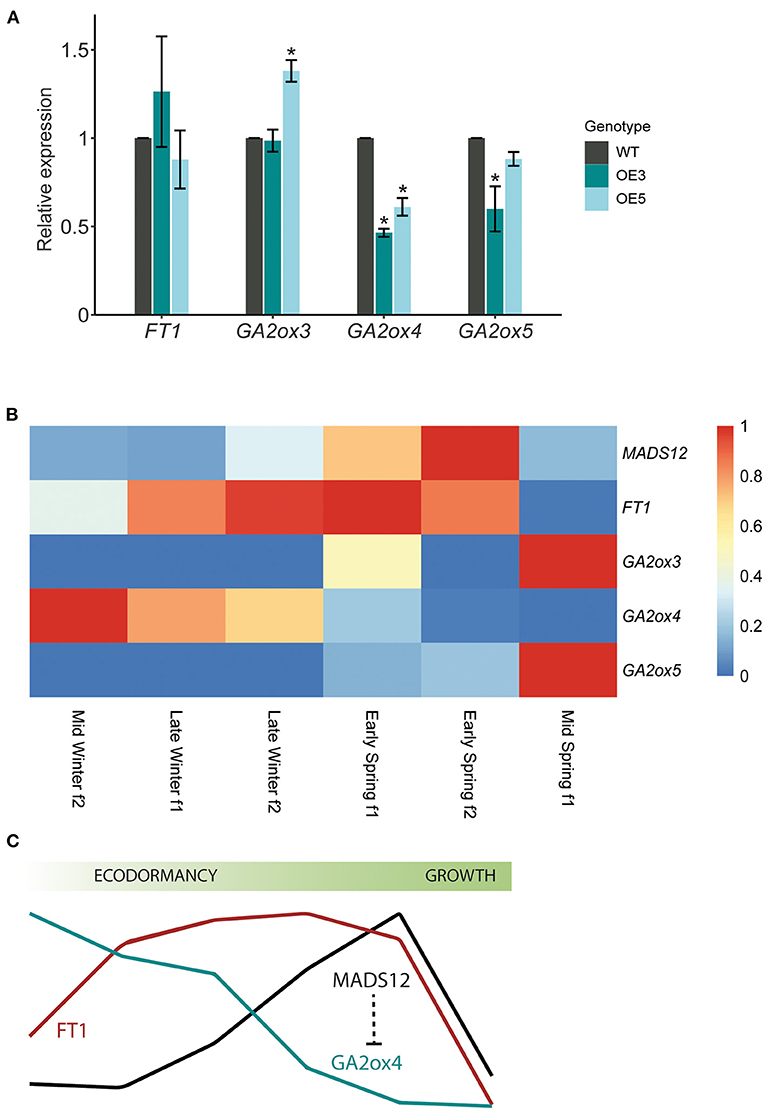
Figure 4. GA2ox4 is downregulated in MADS12 overexpressing lines during bud dormancy release. (A) qRT-PCR analysis of FT1, GA2ox3, GA2ox4, and GA2ox5 genes in ecodormant MADS12 overexpressing OE3 and OE5 and wild-type (WT) apices collected after 5 days in long day (LD) and 22°C treatment. Ubiquitin7 is used as the housekeeping gene. Plotted values and error bars are fold-change means ± s.d. of two biological replicates. Asterisks (*) represent statistical differences assessed by one-way ANOVA followed by Tukey post-hoc test (p < 0.05). (B) Heatmap showing RNAseq expression data of MADS12, FT1, GA2ox3, GA2x4, and GA2ox5 genes in apical buds from mid-winter f2 to mid-spring f1. (C) Schematic representation of MADS12 and GA2ox4 antagonist expression pattern during bud dormancy release.
Discussion
MADS-box homologs to Arabidopsis SOC1 have been identified in trees; however, their function remains very little understood. Arabidopsis SOC1 is expressed in the shoot apical meristem and plays a key role during floral transition, integrating vernalization, photoperiod, GAs, and the aging pathways (Amasino, 2010; Lee and Lee, 2010). Furthermore, Arabidopsis SOC1-related genes, AGL14, AGL19, AGL42, AGL71, and AGL72, are also implicated in the control of the floral transition (Schonrock, 2006; Dorca-Fornell et al., 2011; Pérez-Ruiz et al., 2015). Our phylogenetic tree of poplar and Arabidopsis MADS-box proteins identifies a SOC1 clade including seven poplar SOC1-like family members (Figure 1A). These seven poplar SOC1-like genes show a specific expression pattern during mid-winter to mid-spring, suggesting their role in dormancy release (Figure 2A). Among them, MADS12 and Potri.015G098400 do not conserve a C-terminal SOC1 motif, suggesting that MADS12 C-terminal domain undergoes a divergent evolution (Figure 1B). The functional importance of C-terminal SOC1 motif is still unclear. Structure-function analysis of Arabidopsis with intragenic mutations in the SOC1 gene shows that deletion of C-terminal and part of the K domain renders weak suppression of early flowering of FRIGIDA mutants (Lee et al., 2000, 2008). This observation and the fact that AGL14, also lacking the C-terminal SOC1 motif, is required for Arabidopsis flowering (Pérez-Ruiz et al., 2015) indicate that the C-terminal SOC1 motif is not essential for SOC1 and its homologs function in flowering.
Functional analyses of three SOC1 homolog genes in the kiwifruit tree, which exhibited a C-terminal SOC1 motif, resulted in only AcSOC1i overexpression that promoted early bud break under ecodormant conditions (Voogd et al., 2015). MADS12 OE lines also display an accelerated bud break of ecodormant poplars. Moreover, the seasonal expression pattern of AcSOC1i resembles one of the MADS12 showing a seasonal induction at the end of the winter (Figures 2A,B; Voogd et al., 2015). These two studies point out that AcSOC1i and MADS12 play identical functions during dormancy phenology, despite C-terminal SOC1 motif differences. Similar to our hybrid poplar MADS12 OE lines, overexpression of Arabidopsis SOC1 in poplar did not show phenotypical differences during vegetative shoot growth, but phenological assays were not performed (Bruegmann and Fladung, 2019). Additional functional studies of poplar SOC1-like genes are needed.
This work shows that chilling requirement fulfillment is necessary for MADS12 activation, as it does for FT1, EEB1, and DML10, already described as bud break promoter genes in poplar (Hsu et al., 2011; Yordanov et al., 2014; Conde et al., 2017a). Our results show that MADS12 participates in ecodormancy release, concurring with a single-nucleotide polymorphism's (SNP's) presence associated with bud flush (Evans et al., 2014). We find that ecodormant MADS12 OE lines resume growth under LDs and warm temperatures 10 days earlier than WT, consistent with its possible function as a growth inductor during ecodormancy. Our phenological assays indicate that bud break of MADS12 OE poplars has no differences to WT after chilling fulfillment. Possibly, MADS12 OE phenotype would have been masked by the induction of endogenous MADS12 after chilling. To test this hypothesis, bud break analysis of MADS12 knockout lines should be performed. Another possibility is that the levels of a transcriptional coregulator limit the activity of MADS12 OE plants in vivo. MADS-box transcriptional regulation operates forming heterocomplexes (de Folter et al., 2005). We found that AGAMOUS-LIKE MADS-BOX PROTEIN AGL16 (AGL16-like) TF coexpressed with MADS12 (Supplementary Figures 5, 6A, and Supplementary Table 3). Moreover, AGL16-like expression is not induced in MADS12 OE lines (Supplementary Figure 6B). Thus, we proposed that chilling dependent bud break in MADS12 OE lines could be conditioned by a limiting factor such as AGL16-like. Future protein–protein interaction and coexpression assays in transgenic poplar will sort this out.
During the ecodormancy stage before bud break, reactivation of growth correlates with upregulation of GA biosynthetic genes and simultaneous downregulation of GA catabolic genes, particularly GA2ox4 (Karlberg et al., 2010). Likewise, bud break correlates with downregulation of GA2 oxidases in Vitis vinifera and sweet cherry (Zheng et al., 2018; Vimont et al., 2019). Exogenous addition of GAs to winter buds promoted poplar bud break (Rinne et al., 2011). A functional study demonstrate that the oveexpression of a Gibberellin Oxidase gene caused delayed bud break pointing to GA signaling involvement during bud break (Singh et al., 2018). Recent work showed that MADS12 activates PIN5b in Populus deltoides × euramericana stem tissue during full-growth conditions (Zheng et al., 2020). Unexpectedly, our qRT-PCR analysis showed that PIN5b expression is significantly repressed in both MADS12 OE3 and OE5 than WT, suggesting an impaired polar auxin efflux in MADS12 OE ecodormant buds (Supplementary Figure 7). The differences in seasonal stages or Populus ecotypes might explain this contrasting activity of MADS12. Arabidopsis PIN5 localizes in endoplasmic reticulum (ER) membrane and mediates polar auxin efflux from the cytosol to ER (Mravec et al., 2009). This intracellular auxin transport plays a role in regulating auxin homeostasis by compartmentalizing cellular auxin pools (Barbez and Kleine-Vehn, 2013). Thus, repression of PIN5b in ecodormant buds could increase cytoplasmatic auxin pool. That could contribute to the faster shoot growth resumption capacity observed in MADS12 OE plants, along with GAs. Phenological assays of PIN5b RNAi lines are necessary to investigate its role during winter-to-spring transition.
An increasing amount of evidence points out that dormancy and flowering transition sharing conserved regulatory elements (Maurya and Bhalerao, 2017; Triozzi et al., 2018; Falavigna et al., 2019). In Arabidopsis shoot apical meristem, SOC1 is the major hub in regulatory networks underlying flowering (Immink et al., 2012). SOC1 antagonizes SVP repressive action over GA20 oxidases 2 (GA20ox2), promoting GA biosynthesis and flowering induction at the shoot apex (Andrés et al., 2014). It is unknown if this model could be conserved during dormancy to growth transition. An inspection of public mid-winter to mid-spring RNAseq data indicates that SVL shows an opposite expression pattern with SOC1-like genes encoded by Potri.001G112400 and Potri.012G13300, and GA20 oxidases 3, 5, and 6, but not with MADS12 (Supplementary Figure 8). This observation suggests a possible interplay between SOC1-like genes and SVL to modulate GA biosynthesis in poplar during dormancy to growth transition. However, MADS12 might operate in an SVL-independent manner.
Our results revealed that ecodormant MADS12 OE poplars show accelerated bud break and GA2ox4 repression. Moreover, the RNAseq mid-winter to mid-spring profiling demonstrates that MADS12 and GA2ox4 show opposite expression patterns during dormancy release. In silico analysis of GA2ox4 promoter identified 10 potential MADS-box-binding elements, supporting the idea that GA2ox4 could be transcriptionally repressed by MADS12 (Supplementary Table 2). Future transactivation assays should test this hypothesis. It has been shown that RNAi downregulation of GA2ox4 increased shoot growth in poplar; however, it is unknown whether GA2ox4 RNAi lines could reactivate earlier shoot growth of ecodormant plants (Gou et al., 2011; Singh et al., 2018). Phenological assays of GA2ox4 RNAi lines are critical to sort the importance of downregulation of GA catabolism during winter-to-spring transition. We propose that the seasonal function of MADS12 is to promote growth reactivation during ecodormancy by downregulating GA2ox4 (Figure 4C). Activation of GA signaling promotes reactive oxygen species (ROS) signaling and bud break in Japanese apricot (Zhuang et al., 2013). A transitory activation of oxidative stress has been proposed to play a pivotal role in dormancy in several plant models (Beauvieux et al., 2018). Whether downregulation of GA2ox4 by MADS12 promotes GAs and ROS signaling should be explored in poplar.
Data Availability Statement
The original contributions presented in the study are included in the article/Supplementary Materials, further inquiries can be directed to the Corresponding authors.
Author Contributions
DG-S, JR-S, DA, DC, PT, and MP performed the experiments. All authors participated in the design of the experiments and in the discussions described here. DG-S, MP, and IA wrote the manuscript.
Funding
This study was supported by grants AGL2014-53352-R and PGC2018-093922-B-I00, awarded to IA and MP; and SEV-2016-0672 (2017-2021) to the CBGP Severo Ochoa Programme for Centres of Excellence in R&D from the Agencia Estatal de Investigación of Spain. The work of MP was supported by the Ramón y Cajal MINECO program (RYC-2012-10194), of whom DG-S has an FPI fellowship (PRE2019-089312) from Ministerio de Ciencia e Innovacion de España.
Conflict of Interest
The authors declare that the research was conducted in the absence of any commercial or financial relationships that could be construed as a potential conflict of interest.
Supplementary Material
The Supplementary Material for this article can be found online at: https://www.frontiersin.org/articles/10.3389/fpls.2021.670497/full#supplementary-material
References
Amasino, R. (2010). Seasonal and developmental timing of flowering. Plant J. 61, 1001–1013. doi: 10.1111/j.1365-313X.2010.04148.x
Andrés, F., and Coupland, G. (2012). The genetic basis of flowering responses to seasonal cues. Nat. Rev. Genet. 13, 627–639. doi: 10.1038/nrg3291
Andrés, F., Porri, A., Torti, S., Mateos, J., Romera-Branchat, M., García-Martínez, J. L., et al. (2014). SHORT VEGETATIVE PHASE reduces gibberellin biosynthesis at the Arabidopsis shoot apex to regulate the floral transition. Proc. Natl. Acad. Sci. U.S.A. 111, E2760–E2769. doi: 10.1073/pnas.1409567111
Barbez, E., and Kleine-Vehn, J. (2013). Divide et impera—cellular auxin compartmentalization. Curr. Opin. Plant Biol. 16, 78–84. doi: 10.1016/j.pbi.2012.10.005
Beauvieux, R., Wenden, B., and Dirlewanger, E. (2018). Bud dormancy in perennial fruit tree species: a pivotal role for oxidative cues. Front. Plant Sci. 9:657. doi: 10.3389/fpls.2018.00657
Bohlenius, H. (2006). CO/FT regulatory module controls timing of flowering and seasonal growth cessation in trees. Science 312, 1040–1043. doi: 10.1126/science.1126038
Bruegmann, T., and Fladung, M. (2019). Overexpression of both flowering time genes AtSOC1 and SaFUL revealed huge influence onto plant habitus in poplar. Tree Genet. Genom. 15:20. doi: 10.1007/s11295-019-1326-9
Brunner, A. M., Evans, L. M., Hsu, C.-Y., and Sheng, X. (2014). Vernalization and the chilling requirement to exit bud dormancy: Shared or separate regulation? Front. Plant Sci. 5:732. doi: 10.3389/fpls.2014.00732
Castelán-Muñoz, N., Herrera, J., Cajero-Sánchez, W., Arrizubieta, M., Trejo, C., García-Ponce, B., et al. (2019). MADS-box genes are key components of genetic regulatory networks involved in abiotic stress and plastic developmental responses in plants. Front. Plant Sci. 10:853. doi: 10.3389/fpls.2019.00853
Conde, D., González-Melendi, P., and Allona, I. (2013). Poplar stems show opposite epigenetic patterns during winter dormancy and vegetative growth. Trees 27, 311–320. doi: 10.1007/s00468-012-0800-x
Conde, D., Le Gac, A.-L., Perales, M., Dervinis, C., Kirst, M., Maury, S., et al. (2017a). Chilling-responsive DEMETER-LIKE DNA demethylase mediates in poplar bud break: Role of active DNA demethylase in trees' bud break. Plant Cell Environment 40, 2236–2249. doi: 10.1111/pce.13019
Conde, D., Moreno-Cortés, A., Dervinis, C., Ramos-Sánchez, J. M., Kirst, M., Perales, M., et al. (2017b). Overexpression of DEMETER, a DNA demethylase, promotes early apical bud maturation in poplar. Plant Cell Environ. 40, 2806–2819. doi: 10.1111/pce.13056
Conde, D., Perales, M., Sreedasyam, A., Tuskan, G. A., Lloret, A., Badenes, M. L., et al. (2019). Engineering tree seasonal cycles of growth through chromatin modification. Front. Plant Sci. 10:412. doi: 10.3389/fpls.2019.00412
Cooke, J. E. K., Eriksson, M. E., and Junttila, O. (2012). The dynamic nature of bud dormancy in trees: Environmental control and molecular mechanisms: bud dormancy in trees. Plant Cell Environ. 35, 1707–1728. doi: 10.1111/j.1365-3040.2012.02552.x
de Folter, S., Immink, R. G. H., Kieffer, M., Parenicová, L., Henz, S. R., Weigel, D., et al. (2005). Comprehensive interaction map of the arabidopsis mads box transcription factors. Plant Cell 17, 1424–1433. doi: 10.1105/tpc.105.031831
Ding, J., and Nilsson, O. (2016). Molecular regulation of phenology in trees—because the seasons they are a-changin.' Curr. Opin. Plant Biol. 29, 73–79. doi: 10.1016/j.pbi.2015.11.007
Dorca-Fornell, C., Gregis, V., Grandi, V., Coupland, G., Colombo, L., and Kater, M. M. (2011). The Arabidopsis SOC1-like genes AGL42, AGL71 and AGL72 promote flowering in the shoot apical and axillary meristems: SOC1-like genes control floral transition. Plant J. 67, 1006–1017. doi: 10.1111/j.1365-313X.2011.04653.x
Eriksson, M. E., Hoffman, D., Kaduk, M., Mauriat, M., and Moritz, T. (2015). Transgenic hybrid aspen trees with increased gibberellin (GA) concentrations suggest that GA acts in parallel with FLOWERING LOCUS T2 to control shoot elongation. New Phytol. 205, 1288–1295. doi: 10.1111/nph.13144
Evans, L. M., Slavov, G. T., Rodgers-Melnick, E., Martin, J., Ranjan, P., Muchero, W., et al. (2014). Population genomics of Populus trichocarpa identifies signatures of selection and adaptive trait associations. Nat. Genet. 46, 1089–1096. doi: 10.1038/ng.3075
Falavigna, V., da, S., Guitton, B., Costes, E., and Andrés, F. (2019). I want to (Bud) break free: the potential role of DAM and SVP-like genes in regulating dormancy cycle in temperate fruit trees. Front. Plant Sci. 9:1990. doi: 10.3389/fpls.2018.01990
Gallardo, F., Fu, J., Cantón, F. R., García-Gutiérrez, A., Cánovas, F. M., and Kirby, E. G. (1999). Expression of a conifer glutamine synthetase gene in transgenic poplar. Planta 210, 19–26. doi: 10.1007/s004250050649
Gou, J., Ma, C., Kadmiel, M., Gai, Y., Strauss, S., Jiang, X., et al. (2011). Tissue-specific expression of Populus C19 GA 2-oxidases differentially regulate above- and below-ground biomass growth through control of bioactive GA concentrations. New Phytol. 192, 626–639. doi: 10.1111/j.1469-8137.2011.03837.x
Groover, A., and Cronk, Q., (Eds.). (2017). Comparative and Evolutionary Genomics of Angiosperm Trees (Vol. 21). New York, NY: Springer. doi: 10.1007/978-3-319-49329-9
Hoenicka, H., Nowitzki, O., Hanelt, D., and Fladung, M. (2008). Heterologous overexpression of the birch FRUITFULL-like MADS-box gene BpMADS4 prevents normal senescence and winter dormancy in Populus tremula L. Planta 227, 1001–1011. doi: 10.1007/s00425-007-0674-0
Horvath, D. P., Sung, S., Kim, D., Chao, W., and Anderson, J. (2010). Characterization, expression and function of DORMANCY ASSOCIATED MADS-BOX genes from leafy spurge. Plant Mol. Biol. 73, 169–179. doi: 10.1007/s11103-009-9596-5
Hsu, C.-Y., Adams, J. P., Kim, H., No, K., Ma, C., Strauss, S. H., et al. (2011). FLOWERING LOCUS T duplication coordinates reproductive and vegetative growth in perennial poplar. Proc. Natl. Acad. Sci. U.S.A. 108, 10756–10761. doi: 10.1073/pnas.1104713108
Immink, R. G. H., Posé, D., Ferrario, S., Ott, F., Kaufmann, K., Valentim, F. L., et al. (2012). Characterization of SOC1's central role in flowering by the identification of its upstream and downstream regulators. Plant Physiol. 160, 433–449. doi: 10.1104/pp.112.202614
Johansson, M., Takata, N., Ibáñez, C., and Eriksson, M. E. (2014). “Monitoring seasonal bud set, bud burst, and cold hardiness in populus,” in Plant Circadian Networks, Vol. 1158, ed E. D. Staiger (New York, NY: Springer), 313–324. doi: 10.1007/978-1-4939-0700-7_21
Karlberg, A., Englund, M., Petterle, A., Molnar, G., Sjödin, A., Bako, L., et al. (2010). Analysis of global changes in gene expression during activity-dormancy cycle in hybrid aspen apex. Plant Biotechnol. 27, 1–16. doi: 10.5511/plantbiotechnology.27.1
Kayal, W. E., Allen, C. C., JU, C. J. T., Adams, E. R. I., King-Jones, S., Zaharia, L. I., et al. (2011). Molecular events of apical bud formation in white spruce, Picea glauca. Plant Cell Environ. 34, 480–500. doi: 10.1111/j.1365-3040.2010.02257.x
Kitamura, Y., Takeuchi, T., Yamane, H., and Tao, R. (2016). Simultaneous down-regulation of DORMANCY-ASSOCIATED MADS-box6 and SOC1 during dormancy release in Japanese apricot (Prunus mume) flower buds. J. Horticult. Sci. Biotechnol. 91, 476–482. doi: 10.1080/14620316.2016.1173524
Klocko, A. L., Lu, H., Magnuson, A., Brunner, A. M., Ma, C., and Strauss, S. H. (2018). Phenotypic expression and stability in a large-scale field study of genetically engineered poplars containing sexual containment transgenes. Front. Bioeng. Biotechnol. 6:100. doi: 10.3389/fbioe.2018.00100
Koncz, C., and Schell, J. (1986). The promoter of TL-DNA gene 5 controls the tissue-specific expression of chimaeric genes carried by a novel type of agrobacterium binary vector. Mol. Gen. Genet. MGG 204, 383–396. doi: 10.1007/BF00331014
Lee, H., Suh, S.-S., Park, E., Cho, E., Ahn, J. H., Kim, S.-G., et al. (2000). The AGAMOUS-LIKE 20 MADS domain protein integrates floral inductive pathways in Arabidopsis. Genes Dev. 14, 2366–2376. doi: 10.1101/gad.813600
Lee, J., and Lee, I. (2010). Regulation and function of SOC1, a flowering pathway integrator. J. Exp. Bot. 61, 2247–2254. doi: 10.1093/jxb/erq098
Lee, J., Oh, M., Park, H., and Lee, I. (2008). SOC1 translocated to the nucleus by interaction with AGL24 directly regulates LEAFY. Plant J. 55, 832–843. doi: 10.1111/j.1365-313X.2008.03552.x
Leida, C., Terol, J., Marti, G., Agusti, M., Llacer, G., Badenes, M. L., et al. (2010). Identification of genes associated with bud dormancy release in Prunus persica by suppression subtractive hybridization. Tree Physiol. 30, 655–666. doi: 10.1093/treephys/tpq008
Maurya, J. P., and Bhalerao, R. P. (2017). Photoperiod- and temperature-mediated control of growth cessation and dormancy in trees: a molecular perspective. Ann. Bot. 120, 351–360. doi: 10.1093/aob/mcx061
Moon, J., Suh, S.-S., Lee, H., Choi, K.-R., Hong, C. B., Paek, N.-C., et al. (2003). The SOC1 MADS-box gene integrates vernalization and gibberellin signals for flowering in Arabidopsis: SOC1 integrates vernalization and GA signals for flowering. Plant J. 35, 613–623. doi: 10.1046/j.1365-313X.2003.01833.x
Mravec, J., Skupa, P., Bailly, A., Hoyerová, K., Kreček, P., Bielach, A., et al. (2009). Subcellular homeostasis of phytohormone auxin is mediated by the ER-localized PIN5 transporter. Nature 459, 1136–1140. doi: 10.1038/nature08066
Nakagawa, T., Suzuki, T., Murata, S., Nakamura, S., Hino, T., Maeo, K., et al. (2007). Improved gateway binary vectors: high-performance vectors for creation of fusion constructs in transgenic analysis of plants. Biosci. Biotechnol. Biochem. 71, 2095–2100. doi: 10.1271/bbb.70216
Pérez-Ruiz, R. V., García-Ponce, B., Marsch-Martínez, N., Ugartechea-Chirino, Y., Villajuana-Bonequi, M., de Folter, S., et al. (2015). XAANTAL2 (AGL14) is an important component of the complex gene regulatory network that underlies arabidopsis shoot apical meristem transitions. Mol. Plant 8, 796–813. doi: 10.1016/j.molp.2015.01.017
Pin, P. A., and Nilsson, O. (2012). The multifaceted roles of FLOWERING LOCUS T in plant development: FT, a multifunctional protein. Plant Cell Environ. 35, 1742–1755. doi: 10.1111/j.1365-3040.2012.02558.x
Ramos-Sánchez, J. M., Triozzi, P. M., Alique, D., Geng, F., Gao, M., Jaeger, K. E., et al. (2019). LHY2 Integrates night-length information to determine timing of poplar photoperiodic growth. Curr. Biol. 29, 2402–2406.e4. doi: 10.1016/j.cub.2019.06.003
Rieu, I., Eriksson, S., Powers, S. J., Gong, F., Griffiths, J., Woolley, L., et al. (2008). Genetic analysis reveals that C 19 -GA 2-oxidation is a major gibberellin inactivation pathway in Arabidopsis. Plant Cell 20, 2420–2436. doi: 10.1105/tpc.108.058818
Rinne, P. L. H., Welling, A., Vahala, J., Ripel, L., Ruonala, R., Kangasjärvi, J., et al. (2011). Chilling of dormant buds hyperinduces FLOWERING LOCUS T and recruits GA-inducible 1,3-β-glucanases to reopen signal conduits and release dormancy in Populus. Plant Cell 23, 130–146. doi: 10.1105/tpc.110.081307
Rodriguez-A, J., Sherman, W. B., Scorza, R., Wisniewski, M., and Okie, W. R. (1994). “Evergreen” peach, its inheritance and dormant behavior. J. Am. Soc. Horticult. Sci. 119, 789–792. doi: 10.21273/JASHS.119.4.789
Rohde, A., Ruttink, T., Hostyn, V., Sterck, L., Van Driessche, K., and Boerjan, W. (2007). Gene expression during the induction, maintenance, and release of dormancy in apical buds of poplar. J. Exp. Bot. 58, 4047–4060. doi: 10.1093/jxb/erm261
Rohde, A., Storme, V., Jorge, V., Gaudet, M., Vitacolonna, N., Fabbrini, F., et al. (2010). Bud set in poplar – genetic dissection of a complex trait in natural and hybrid populations. New Phytol. 16, 106–121. doi: 10.1111/j.1469-8137.2010.03469.x
Santamaría, M., Hasbún, R., Valera, M., Meijón, M., Valledor, L., Rodríguez, J. L., et al. (2009). Acetylated H4 histone and genomic DNA methylation patterns during bud set and bud burst in Castanea sativa. J. Plant Physiol. 166, 1360–1369. doi: 10.1016/j.jplph.2009.02.014
Schonrock, N. (2006). Polycomb-group proteins repressthe floral activator AGL19 in the FLC-independent vernalization pathway. Genes Dev. 20, 1667–1678. doi: 10.1101/gad.377206
Shim, J. S., Kubota, A., and Imaizumi, T. (2017). Circadian clock and photoperiodic flowering in Arabidopsis: CONSTANS is a hub for signal integration. Plant Physiol. 173, 5–15. doi: 10.1104/pp.16.01327
Singh, R. K., Maurya, J. P., Azeez, A., Miskolczi, P., Tylewicz, S., Stojkovič, K., et al. (2018). A genetic network mediating the control of bud break in hybrid aspen. Nat. Commun. 9:4173. doi: 10.1038/s41467-018-06696-y
Singh, R. K., Svystun, T., AlDahmash, B., Jönsson, A. M., and Bhalerao, R. P. (2017). Photoperiod- and temperature-mediated control of phenology in trees—A molecular perspective. New Phytol. 213, 511–524. doi: 10.1111/nph.14346
Trainin, T., Bar-Ya'akov, I., and Holland, D. (2013). ParSOC1, a MADS-box gene closely related to Arabidopsis AGL20/SOC1, is expressed in apricot leaves in a diurnal manner and is linked with chilling requirements for dormancy break. Tree Genet. Genom. 9, 753–766. doi: 10.1007/s11295-012-0590-8
Triozzi, P. M., Ramos-Sánchez, J. M., Hernández-Verdeja, T., Moreno-Cortés, A., Allona, I., and Perales, M. (2018). Photoperiodic regulation of shoot apical growth in poplar. Front. Plant Sci. 9:1030. doi: 10.3389/fpls.2018.01030
Tylewicz, S., Petterle, A., Marttila, S., Miskolczi, P., Azeez, A., Singh, R. K., et al. (2018). Photoperiodic control of seasonal growth is mediated by ABA acting on cell-cell communication. Science 360, 212–215. doi: 10.1126/science.aan8576
Vimont, N., Schwarzenberg, A., Domijan, M., Beauvieux, R., Arkoun, M., Jamois, F., et al. (2019). Hormonal balance finely tunes dormancy status in sweet cherry flower buds. Tree Physiol. 4, 544–561. doi: 10.1093/treephys/tpaa122
Voogd, C., Wang, T., and Varkonyi-Gasic, E. (2015). Functional and expression analyses of kiwifruit SOC1-like genes suggest that they may not have a role in the transition to flowering but may affect the duration of dormancy. J. Exp. Bot. 66, 4699–4710. doi: 10.1093/jxb/erv234
Wang, H., Wu, C., Ciais, P., Peñuelas, J., Dai, J., Fu, Y., et al. (2020). Overestimation of the effect of climatic warming on spring phenology due to misrepresentation of chilling. Nat. Commun. 11:4945. doi: 10.1038/s41467-020-18743-8
Wang, J., Gao, Z., Li, H., Jiu, S., Qu, Y., Wang, L., et al. (2020). Dormancy-associated MADS-Box (DAM) genes influence chilling requirement of sweet cherries and co-regulate flower development with SOC1 gene. Int. J. Mol. Sci. 21:921. doi: 10.3390/ijms21030921
Wareing, P. F. (1956). Photoperiodism in woody plants. Annu. Rev. Plant Physiol. 7, 191–214. doi: 10.1146/annurev.pp.07.060156.001203
Weiser, C. J. (1970). Cold resistance and injury in woody plants: knowledge of hardy plant adaptations to freezing stress may help us to reduce winter damage. Science 169, 1269–1278. doi: 10.1126/science.169.3952.1269
Wu, R., Tomes, S., Karunairetnam, S., Tustin, S. D., Hellens, R. P., Allan, A. C., et al. (2017). SVP-like MADS box genes control dormancy and budbreak in apple. Front. Plant Sci. 8:477. doi: 10.3389/fpls.2017.00477
Yanovsky, M. J., and Kay, S. A. (2002). Molecular basis of seasonal time measurement in Arabidopsis. Nature 419, 308–312. doi: 10.1038/nature00996
Yordanov, Y. S., Ma, C., Strauss, S. H., and Busov, V. B. (2014). EARLY BUD-BREAK 1 (EBB1) is a regulator of release from seasonal dormancy in poplar trees. Proc. Natl. Acad. Sci. U.S.A. 111, 10001–10006. doi: 10.1073/pnas.1405621111
Zawaski, C., Kadmiel, M., Pickens, J., Ma, C., Strauss, S., and Busov, V. (2011). Repression of gibberellin biosynthesis or signaling produces striking alterations in poplar growth, morphology, and flowering. Planta 234, 1285–1298. doi: 10.1007/s00425-011-1485-x
Zheng, C., Kwame Acheampong, A., Shi, Z., Halaly, T., Kamiya, Y., Ophir, R., et al. (2018). Distinct gibberellin functions during and after grapevine bud dormancy release. J. Exp. Bot. 69, 1635–1648. doi: 10.1093/jxb/ery022
Zheng, S., He, J., Lin, Z., Zhu, Y., Sun, J., and Li, L. (2020). Two MADS-box genes regulate vascular cambium activity and secondary growth by modulating auxin homeostasis in Populus. Plant Commun. 100134. doi: 10.1016/j.xplc.2020.100134 (in press).
Keywords: poplar, MADS-box family transcription factors, dormancy, gibberellins, growth reactivation, SOC1, ecodormancy, bud break
Citation: Gómez-Soto D, Ramos-Sánchez JM, Alique D, Conde D, Triozzi PM, Perales M and Allona I (2021) Overexpression of a SOC1-Related Gene Promotes Bud Break in Ecodormant Poplars. Front. Plant Sci. 12:670497. doi: 10.3389/fpls.2021.670497
Received: 21 February 2021; Accepted: 06 April 2021;
Published: 25 May 2021.
Edited by:
Amy Brunner, Virginia Tech, United StatesReviewed by:
Yan Bao, Shanghai Jiao Tong University, ChinaFelipe Dos Santos Maraschin, Federal University of Rio Grande do Sul, Brazil
Copyright © 2021 Gómez-Soto, Ramos-Sánchez, Alique, Conde, Triozzi, Perales and Allona. This is an open-access article distributed under the terms of the Creative Commons Attribution License (CC BY). The use, distribution or reproduction in other forums is permitted, provided the original author(s) and the copyright owner(s) are credited and that the original publication in this journal is cited, in accordance with accepted academic practice. No use, distribution or reproduction is permitted which does not comply with these terms.
*Correspondence: Mariano Perales, bWFyaWFuby5wZXJhbGVzJiN4MDAwNDA7dXBtLmVz; Isabel Allona, aXNhYmVsLmFsbG9uYSYjeDAwMDQwO3VwbS5lcw==
†ORCID: Daniela Gómez-Soto orcid.org/0000-0003-0318-3079
Mariano Perales orcid.org/0000-0002-7351-8439
Isabel Allona orcid.org/0000-0002-7012-2850
‡Present address: Daniel Conde, School of Forest, Fisheries and Geomatics Sciences, University of Florida, Gainesville, FL, United States
Paolo M. Triozzi, School of Forest, Fisheries and Geomatics Sciences, University of Florida, Gainesville, FL, United States