- 1Department of Cell and Molecular Biology, Faculty of Biotechnology and Biomolecular Sciences, Universiti Putra Malaysia, Serdang, Malaysia
- 2Biotechnology and Nanotechnology Research Centre, Malaysian Agricultural Research and Development Institute (MARDI), Kuala Lumpur, Malaysia
- 3Health Sciences Division, Abu Dhabi Women's College, Higher Colleges of Technology, Abu Dhabi, United Arab Emirates
Pluronic F-68 (PF-68) is a non-ionic surfactant used in plant tissue culture as a growth additive. Despite its usage as a plant growth enhancer, the mechanism underlying the growth-promoting effects of PF-68 remains largely unknown. Hence, this study was undertaken to elucidate the growth-promoting mechanism of PF-68 using recalcitrant MR 219 callus as a model. Supplementation of 0.04% PF-68 (optimum concentration) was shown to enhance callus proliferation. The treated callus recorded enhanced sugar content, protein content, and glutamate synthase activity as exemplified in the comparative proteome analysis, showing protein abundance involved in carbohydrate metabolism (alpha amylase), protein biosynthesis (ribosomal proteins), and nitrogen metabolism (glutamate synthase), which are crucial to plant growth and development. Moreover, an increase in nutrients uptake was also noted with potassium topping the list, suggesting a vital role of K in governing plant growth. In contrast, 0.10% PF-68 (high concentration) induced stress response in the callus, revealing an increment in phenylalanine ammonia lyase activity, malondialdehyde content, and peroxidase activity, which were consistent with high abundance of phenylalanine ammonia lyase, peroxidase, and peroxiredoxin proteins detected and concomitant with a reduced level of esterase activity. The data highlighted that incorporation of PF-68 at optimum concentration improved callus proliferation of recalcitrant MR 219 through enhanced carbohydrate metabolism, nitrogen metabolism, and nutrient uptake. However, growth-promoting effects of PF-68 are concentration dependent.
Introduction
Rice is one of the major staple foods serving more than half of the population of the world (Hadiarto and Tran, 2011). In Asian countries, rice provides nearly half of the total dietary carbohydrate and 50–80% of the daily calorie intake (Khush, 2005). Increasing rice production should be prioritized in order to sustain the ever-increasing world population (Sen et al., 2020). Through the advancement of modern biotechnology, genetic manipulation could serve as an alternative solution to meet the increasing demands of rice production (Low et al., 2018). However, genetic manipulation in indica rice cultivars remains a challenge. This is because indica rice cultivars share common recalcitrant properties toward in vitro regeneration responses. For instance, indica rice cultivars suffer from poor callus proliferation, low regeneration efficiency, a long regeneration period, and a low transformation rate (Sah et al., 2014). Therefore, in order to improve the in vitro responses of indica rice cultivar, optimization of the plant growth medium is required.
Additives are the key components in improving the in vitro responses of indica rice cultivars (Abiri et al., 2017; Kok et al., 2018). Some of the commonly used growth additives in plant tissue culture include Pluronic F-68 (PF-68), lignosulfonate, silver nitrate, silicon, coconut, and gibberellic acid (Biswas and Mandal, 2007; He et al., 2013; Yildirim and Turker, 2014; Irshad et al., 2018; Wan Abdullah et al., 2020). PF-68 is a non-ionic, copolymer surfactant that has been employed as an additive in both in vitro animal and plant cultures (Meier et al., 1999; Barbulescu et al., 2011). PF-68 has been widely utilized in animal cell suspension culture to protect and repair damaged cells from constant sparging and agitation (Meier et al., 1999). Further investigation of the application of PF-68 in yeast cells revealed that PF-68 is capable of interacting with the cell surface by increasing the permeability of the cell membrane through the formation of short-lived, transmembrane pores (King et al., 1991; Cho et al., 2007). Through enhanced membrane permeability, the nutrient uptake and cell growth were stimulated in cell culture (Shelat et al., 2013). However, the outcome of this interaction is concentration-dependent. At high concentration, PF-68 is able to disrupt the normal architecture of the lipid bilayer, resulting in a cell lysis (Cho et al., 2007).
Similar to animal cell culture, the application of PF-68 was found to enhance nutrient uptake and plant growth in plant tissue culture. In addition to that, application of PF-68 was demonstrated to enhance the release of anthraquinone into the medium in suspension cultures of Morindacitrifolia, suggesting an increase in membrane or cell wall permeability of Morindacitrifolia (Bassetti et al., 1995). A similar study was demonstrated, whereby secretion of a human granulocyte-macrophage colony-stimulating factor was stimulated when PF-68 was added into the cell suspension culture of transgenic Nicotiana tabacum (Cho et al., 2007). Besides, the application of PF-68 was reported to enhance shoot regeneration in Citrus sinensis (Curtis and Mirkov, 2012), Pyrus communis (Dashti et al., 2012), Ricinus communis (Kulathuran and Narayanasamy, 2015), and Abelmoschus esculentus (Irshad et al., 2018). Notably, PF-68 was demonstrated to improve the growth of roots, callus, and protoplast of Solanum dulcamara (Kumar et al., 1992), shoot regeneration of recalcitrant Brassica napus embryos (Barbulescu et al., 2011), and callus proliferation of recalcitrant indica rice (Kok et al., 2020). This implies that PF-68 could be a good candidate for plant cell growth and regeneration improvement of the recalcitrant cultivars.
An earlier study made by Kok et al. (2020) on PF-68 application has shown successful enhancement of a callus proliferation rate and a number of callus with root-like structure of MR 219 indica rice cultivar. Unfortunately, the underlying growth promoting mechanisms of PF-68 remains largely unknown. Hence, to close this gap and to maximize the usage of PF-68 as a growth additive, understanding its mode of action is crucial. Therefore, the present study aimed to investigate the mode of action of PF-68 in the growth enhancement of MR 219 indica rice cultivar.
Materials and Methods
Plant Materials
MR 219 cultivar was selected as a model of recalcitrant rice cultivar in this study. The seeds of recalcitrant Malaysian cultivar MR 219 used in this study were obtained from the Malaysian Agricultural Research and Development Institute (MARDI), Seberang Prai, Penang, Malaysia.
Pluronic F-68
The analytical grade of PF-68 (10%) used in this research was purchased from Thermo Fisher Scientific, United States.
Seed Sterilization and Growth Conditions
Surface sterilization of the seeds was performed according to previously described protocol by Lim and Lai (2017) with slight modifications. Firstly, mature seeds were de-husked and surface-sterilized using 70% (v/v) ethanol for 1 min, followed by 50% (v/v) Clorox, containing 6% sodium hypochlorite for 30 min. Subsequently, the seeds were washed with distilled water to remove remaining residues and allowed to be air-dried on a sterilized filter paper. The sterilized seeds were then transferred onto previously established a callus induction medium, containing a Gamborg's B5 basal medium (Gamborg et al., 1968), supplemented with 10-g/L maltose, 0.1-g/L L-glutamine, 0.1-g/L L-asparagine, 0.1-g/L L-arginine, 10-mg/L 1-naphthaleneacetic acid, and 1-mg/L 2,4-dichlorophenoxyacetic acid, pH 5.8 for 2-week incubation in the dark at 25 ± 2°C (Low et al., 2019). Subsequently, the calluses induced from the seed were transferred onto a Murashige and Skoog (MS) medium (Murashige and Skoog, 1962), containing 30-g/L sucrose without plant growth regulators (MSO), supplemented with PF-68 (0.04 and 0.10%; w/v) for 2-week incubation in the dark at 25 ± 2°C. Callus cultured onto an MS medium without PF-68 supplementation was used as the experimental control. Morphological changes in the callus were recorded at the end of the incubation period. The fresh weight (FW) of the callus was measured, and its constant dry weight (DW) was recorded after drying at 50°C in an oven for 5 days. The measurement was performed in triplicates with three biological replicates.
Total Soluble Sugar Content
Total soluble sugar was measured using a phenol-sulfuric acid method (Terzi et al., 2014). Approximately, 0.25 g of calli were grounded into powder using liquid nitrogen. Subsequently, the powdered calli were homogenized in 3 ml of 80% (v/v) ethanol and centrifuged at 880 × g for 20 min. The pellet was discarded, and the supernatant was mixed with 1-ml 5% (v/v) phenol and 5-ml concentrated sulfuric acid per 1 ml of supernatant. The absorbance of the samples was measured at 565 nm by using a spectrophotometer (Implen GmbH, Germany). The corresponding concentration was determined, using glucose solution as a standard.
Total Protein Content
The protein content of the callus was determined using the Bradford assay (Kruger, 1994). Approximately, 0.1-g calli were grounded into powder in liquid nitrogen and mixed with 900 μl of 50 mM of ammonium bicarbonate and 100 μl of 50-mM phenylmethylsulfonyl fluoride (PMSF). Subsequently, the mixture was vortexed, sonicated, and centrifuged according to Yang et al. (2019). Acetone precipitation was carried out according to Jiang et al. (2004). The protein pellet was dissolved at a ratio of 9:1 of 50-mM ammonium bicarbonate to 50-mM PMSF. The protein content in the extract was determined at 595 nm (Kruger, 1994). The corresponding concentration was determined, using bovine serum albumin as a standard.
Glutamate Synthase Activity
Glutamate synthase (GOGAT) activity was measured, following a method described by Ertani et al. (2011). The following steps were performed at 4°C. For extraction of GOGAT activity, ~0.2 g of the samples was grounded into powder with the presence of liquid nitrogen. The powdered calli were then homogenized with 2 ml of extraction solution, containing 100-mM Hepes-NaOH at pH 7.5, 5-mM MgCl2, and 1-mM dithiothreitol. The extract was filtered through two layers of muslin cloth. After centrifugation at 20,000 × g at 4°C for 15 min, 100 μl of the supernatant was homogenized with 1 ml of mixture solution, containing 25-mM Hepes-NaOH at pH 7.5, 2-mM L-glutamine, 1-mM α-ketoglutaric acid, 0.1-mM NADH, and 1-mM Na2EDTA. The GOGAT activity was measured spectrophotometrically by monitoring NADH oxidation at 340 nm. The enzyme activity was expressed in μmol−1g−1FW, representing the amount of enzyme, catalyzing the oxidation of 1 μmol of NADH min−1.
Phenylpropanoid Ammonia Lyase Activity
Phenylpropanoid ammonia lyase (PAL) activity was measured, following a protocol as described by Wang et al. (2016) with minor modifications. Approximately, 0.2 g of the sample calli was grounded into powder form in liquid nitrogen. Then, the powdered calli were mixed with 2 ml of the extraction buffer, comprising of 50-mM Tris-HCl buffer at pH 8.5, 5-mM Na2EDTA, 15-mM ß-mercaptoethanol, 1-mM PMSF, and 0.15% (w/v) polyvinylpyrrolidine. The extract was centrifuged at 12,000 × g at 4°C for 20 min. Twenty microliters of the extract were used to measure the proteins content, using the Bradford assay while the remaining extract was used to determine the PAL activity. A total of 500 μl of the extract was mixed with 3 ml of the reaction buffer solution, consisted of 50-mM Tris-HCl buffer (pH 8.5) and 12-mM L-phenyalanine. The mixture was then incubated at 30°C for an hour. The PAL activity was measured at 290 nm. The PAL activity was calculated based on a PAL standard curve made by cinnamic acid where one unit of PAL deaminates L-phenylalanine to trans-cinnamic acid. Specific activity of PAL was calculated by PAL activity at 290 nm (U), divided by PAL protein concentration (μg) and expressed as U/μg of protein.
Malondialdehyde Content
Lipid peroxidation levels from the samples were determined through the malondialdehyde (MDA) assay. MDA is a lipid peroxidation product, which can be quantified in a reaction with thiobarbituric acid (Heath and Packer, 1968). Approximately, 0.2 g of the samples were grounded into powder, using liquid nitrogen and then homogenized with 2 ml of ice-cold phosphate buffered saline (PBS). Subsequently, the mixture was centrifuged at 12,000 × g for 15 min at 4°C. Two hundred microliters of the supernatant obtained were mixed with 800 μl PBS, 25 μl butylhydroxytoluene (8.8 mg/ml), and 500 μl of 50% (w/v) trichloroacetic acid. The mixture was left for 2 h on ice. Then, the mixtures were centrifuged at 12,000 × g for 15 min at 25°C. One milliliter of the sample was then mixed with 75 μl of 100 mM EDTA and 250 μl of 50-mM thiobarbituric acid. The mixture was boiled for 15 min and left to cool to room temperature. The MDA content in the sample was determined at 532 and 600 nm. The corresponding concentration was determined using tetramethoxypropane as a standard.
Peroxidase Activity
Peroxidase activity was determined as described by Agostini et al. (1997) with modifications. Approximately, 2 g of samples were grounded into powder form, using liquid nitrogen and then homogenized with 0.8 ml of an extraction buffer, containing a 10-mM sodium acetate/acetic acid buffer at pH 4.0 and 1-M sodium chloride. After centrifugation at 5,000 × g for 5 min at 4°C, the extract was used to measure protein content, using the Bradford assay while the remaining extract was used to determine the peroxidase activity. A total of 2 μl of the extract was mixed with 1 ml of reaction mixture, containing 630-μM o-dianisidine, 500-μM H2O2, and a 100-mM sodium acetate/acetic acid buffer at pH 5.3. The peroxidase activity was determined immediately by measuring the absorbance at 470 nm. The peroxidase activity was measured based on the amount of an enzyme, forming 1 μmol of product in a minute, produced by o-dianisidine oxidation.
Esterase Activity
Esterase activity was determined through a simple fluorometric method as described by Watanabe and Lam (2008). Fluorescein diacetate (FDA) was used as a fluorescent indicator of cell viability via endogenous esterase activity. Approximate, 0.2-g samples were grounded with liquid nitrogen. Then, the powdered calli were stained with a reaction mixture, containing 2.5 μg/ml of FDA in PBS. The mixture was left to incubate for 10 min at 25°C. Subsequently, the mixture was washed three times, using the reaction mixture, containing 2.5 μg/ml of FDA in PBS. For quantification of endogenous esterase activity, the resulting supernatants were used as samples for direct measurement of esterase activity in vitro by using a fluorescence microplate reader (TECAN infinite 200, Switzerland). The fluorescence value of the suspension was detected with an excitation wavelength at 440 nm and an emission wavelength at 550 nm.
Callus Nutrient Ions Content Analysis via Atomic Absorption Spectrometry (AAS) Analysis
In nutrient ions content study, the callus specimen was dried in an oven at 70°C for 5 days. The specimen was processed as described previously by Karpiuk et al. (2016) with slight modifications. A dried specimen was homogenized into fine powder and kept in a desiccator until subsequent analysis. A mass of 1-g powdered specimen was weighed and transferred into a silica crucible and burnt in a muffle furnace. The muffle furnace temperature was gradually increased from room temperature to 530–550°C for 5 h. The cooled residue was then dissolved in 6 ml of 6-M HCl for an hour. The resulting solutions were then filtered through Whatman filter papers into a volumetric flask. The filtered solution was then transferred to the AAS instrument (Thermo Fisher Scientific, United States) to measure the nutrients content by comparison with the standards. The operation conditions used to operate the AAS instrument were as recommended by the manufacturer.
Proteomic Analysis
In proteomic analysis, plant samples were grounded into fine powder, using liquid nitrogen and homogenized at a ratio of 9:1, consisting of 50 mM of ammonium bicarbonate to 50-mM PMSF. The mixture was then vortexed, sonicated, and centrifuged according to Yang et al. (2019), and solubilized proteins were collected. Desalting was carried out, using an acetone precipitation method (Jiang et al., 2004). The protein pellet was dissolved at a ratio of 9:1 (50-mM ammonium bicarbonate to 50-mM PMSF). The protein content in the extract was determined at 595 nm through the Bradford assay (Kruger, 1994). The protein sample was added with dithiothreitol to a final concentration of 10 mM and then incubated on an orbitor shaker at 25 ± 2°C for 30 min. Then, iodoacetamide was added into the mixture to a final concentration of 10 mM, and the mixture was further incubated in darkness at room temperature for 30 min. Trypsin was added into the protein mixture at a ratio of 1:20 for trypsin to protein (w/w) and incubated overnight at room temperature. The sample was dried in a SpeedVac vacuum concentrator (Thermo Fisher Scientific, United States). Following which, the dried sample was dissolved in 200 μl of molecular biology grade water and dried in the Speedvac. This step was repeated twice. The sample was then stored at −80°C until further analysis.
Nano liquid chromatography tandem-mass spectrometry (nano LC-MS/MS) was performed, using the Dionex 3,000 Ultimate RSLCnano (Thermo Fisher Scientific, United States). An aliquot of a 2-μl-digested-proteins sample was injected into the EASY-Spray Column Acclaim PepMapTM C18 100 (A0, 2 μm particle size, 50 μm id × 15 cm) at 35°C. The sample elution process was performed similarly as described by Yang et al. (2019). The eluent from the LC was directly introduced into the mass spectrometer (Orbitrap Fusion – Thermo Fisher Scientific, United States). The instrument was operated in the data-dependent mode. Full-scan spectra were collected Orbitrap MS (OTMS1) using parameters defined by a previous study (Yang et al., 2019). Only precursors with an assigned monoisotopic m/z and a charge state of 2 to 7 were further analyzed for MS2. All precursors were filtered using a 20-s dynamic exclusion window and an intensity threshold of 5,000. The precursors were fragmented by collision-induced dissociation (CID) and higher-energy collisional dissociation (HCD) at a normalized collision energy of 30 and 28%. The data were analyzed, using the Thermo ScientificTM Proteome DiscovererTM Software 2.1.
Real-Time PCR Analysis
Total RNA was isolated from the powdered calli, incubated in three treatments (control, 0.04%, 0.10% PF-68) using RNeasy Plant Mini Kit (Qiagen, Germany), following the protocol described in Lai and Masatsugu (2013). First-strand cDNA was synthesized from 1 μg of isolated total RNA using QuantiNova Reverse Transcription Kit (Qiagen, Germany). The primers were designed (Supplementary Table 1), using Primer-Blast from the National Center for Biotechnology Information (NCBI) and synthesized by Integrated DNA Technologies (IDT, United States). Real-time PCR was performed, using a aBio-Rad CFX96 system (Bio-Rad, United States) with QuantiNova SYBR Green PCR (Qiagen, Germany), following a protocol described in Lai et al. (2011). The PCR reaction conditions used were as follows: 95°C for 30 s, followed by 40 cycles of 95°C for 5 s and 60°C for 5 s. Three biological replicates and three technical replicates were performed for each sample. The data were analyzed, using the Bio-Rad CFX Manager 3.1 software. The relative expression levels (2−ΔΔCT) were calculated according to Livak's method (Livak and Schmittgen, 2001). The reference genes used in this study were rice cyclophilin (OsCYC) and ubiquitin 5 (OsUBQ5).
Statistical Analysis
All data presented were the average ± standard error mean (SEM) of three biological replicates with three technical replicates. The data were analyzed, using one-way analysis of variance at the significant level of p < 0.05 between each treatment, using the Statistical Package for the Social Sciences version 20 (IBM Corp., Armonk, United States).
Results
Growth-Promoting Response of PF-68 Without the Presence of Plant Hormone
An earlier study on PF-68 in callus proliferation revealed that optimum concentration of PF-68 (0.04%) significantly enhanced callus proliferation of MR 219 (Kok et al., 2020). Meanwhile, high concentration of PF-68 (0.10%) was demonstrated to induce stress response (Kok et al., 2020). In this study, plant hormones were removed from the callus proliferation medium in order to investigate the mechanism governing the growth-promoting response of PF-68 as a plant additive without the interference of plant hormones. Based on the results shown in Figure 1A, the application of 0.04% PF-68 significantly enhanced MR 219 callus proliferation in the MSO medium by 68.1% and 15.02% in FW and DW, respectively.
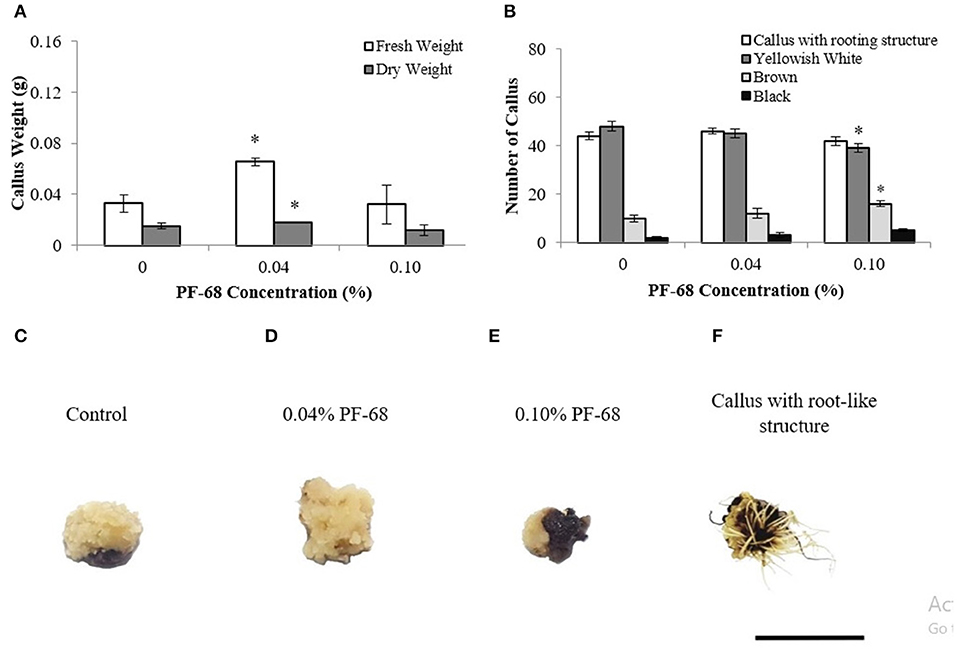
Figure 1. Data obtained from calli proliferated on Murashige and Skoog (MS) medium supplemented with different PF-68 concentrations without plant hormones (MSO) for 3 weeks. (A) Mean fresh and dry weights recorded on 3 weeks old calli; (B) callus morphology on MSO (control), MSO +0.04% and MSO +0.10% PF-68; (C) control callus at week 3; (D) callus on MSO +0.04% PF-68 at week 3; (E) callus on MSO +0.10% PF-68 at week 3; (F) callus with root-like structure at week 3. Data shows the mean of three biological replicates. Asterisks (*) indicate statistical significant difference at p < 0.05 in Dunnet's test. Scale bars represent 0.5 cm. Error bars represent standard error mean.
Most of the calli (Figure 1B) cultured on both control and PF-68 were compact, dry, and yellowish-white (Figures 1C,D). As the concentration of PF-68 increased, decreasing numbers of yellowish-white calli were observed (Figure 1B). The highest number of yellowish-white calli (80%) was recorded in the MSO (control) compared with 75% with 0.04% PF-68 supplementation and 65.00% with 0.10% PF-68 supplementation (Figure 1B). Increasing numbers of brown (Figure 1E) and black (Figure 1F) calli were also noted. The highest number of brown and black calli recorded in 0.10% PF-68 was 26.67 and 8.33%, respectively (Figure 1B). Besides, the application of PF-68 enhanced the numbers of callus with root-like structure (Figure 1F). Supplementation with 0.04% PF-68 recorded the highest number at 76.67% (Figure 1B).
Biochemical Assessments of PF-68 at Optimum and High Concentrations
To further investigate the underlying growth-promoting mechanism of PF-68 in callus proliferation, biochemical assessments were performed on MSO, 0.04% PF-68 and 0.10% PF-68. A significant increment of total sugar content was found in both calli grown on 0.04% PF-68 (0.81 mg/ml) and 0.10% PF-68 (0.75 mg/ml), as compared with the control (0.67 mg/ml) (Figure 2A). Similarly, a significant increase of proteins content was recorded in calli grown on 0.04% PF-68 (0.58 mg/ml) and 0.10% PF-68 (0.49 mg/ml), compared with the control (0.45 mg/ml) (Figure 2B). The increases in proteins content were generally consistent with the increases in GOGAT activity as recorded in calli grown on 0.04% PF-68 with 0.48-μmol/g protein compared with the control 0.38-μmol/g protein (Figure 2C).
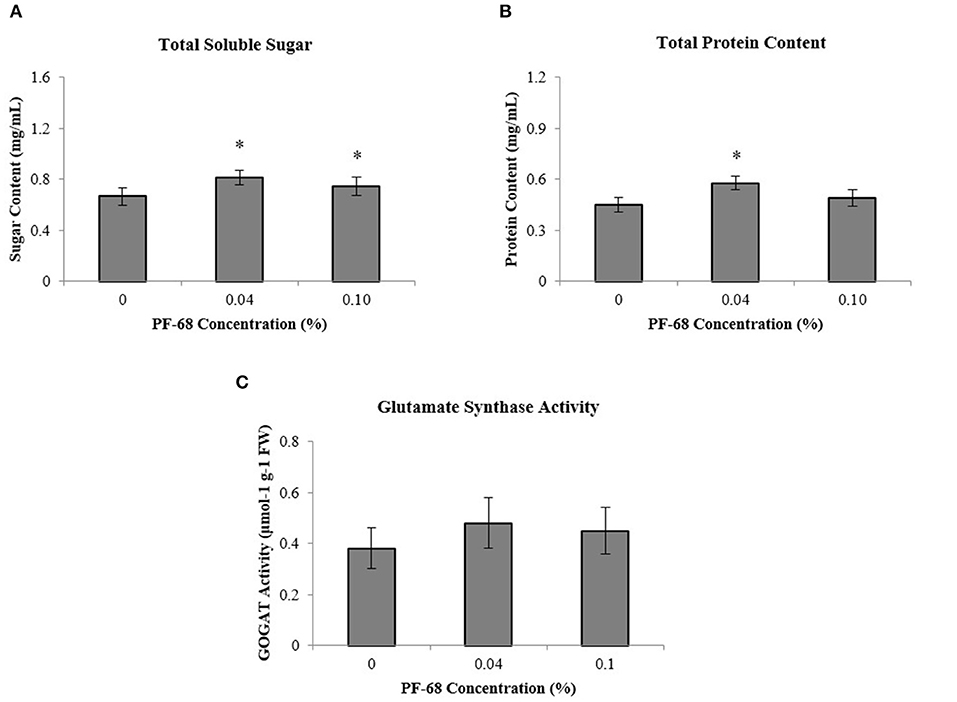
Figure 2. Biochemicals analysis was performed on extracts of calli grown on Murashige and Skoog (MS) medium supplemented with different PF-68 concentrations without plant hormones (MSO). Control (MSO), optimum (MSO +0.04% PF-68), and high (MSO +0.10% PF-68). (A) Total soluble sugar; (B) total protein content; (C) glutamate synthase (GOGAT) activity. Data shows the mean of three biological replicates. Asterisks (*) indicate values were significantly different from those of the control callus at p < 0.05. Error bars represent SD of three biological replicates.
Besides, stress-related biochemical assessments performed in order to investigate the underlying mechanism induced by PF-68 at high concentration have demonstrated that the highest PAL activity was detected in calli grown on 0.10% PF-68 (0.28-U/μg protein) (Figure 3A), which corroborated with its highest MDA content of 0.024-U/μg protein (Figure 3B) and highest peroxidase activity of 0.15-U/μg protein (Figure 3C). In determination of esterase activity, calli grown on 0.10% PF-68 were recorded to have the lowest esterase activity (34,204.50-nmol/ng protein), as compared with 0.04% PF-68 (35,091.67-nmol/ng protein) and control (37,620.17-nmol/ng protein) (Figure 3D).
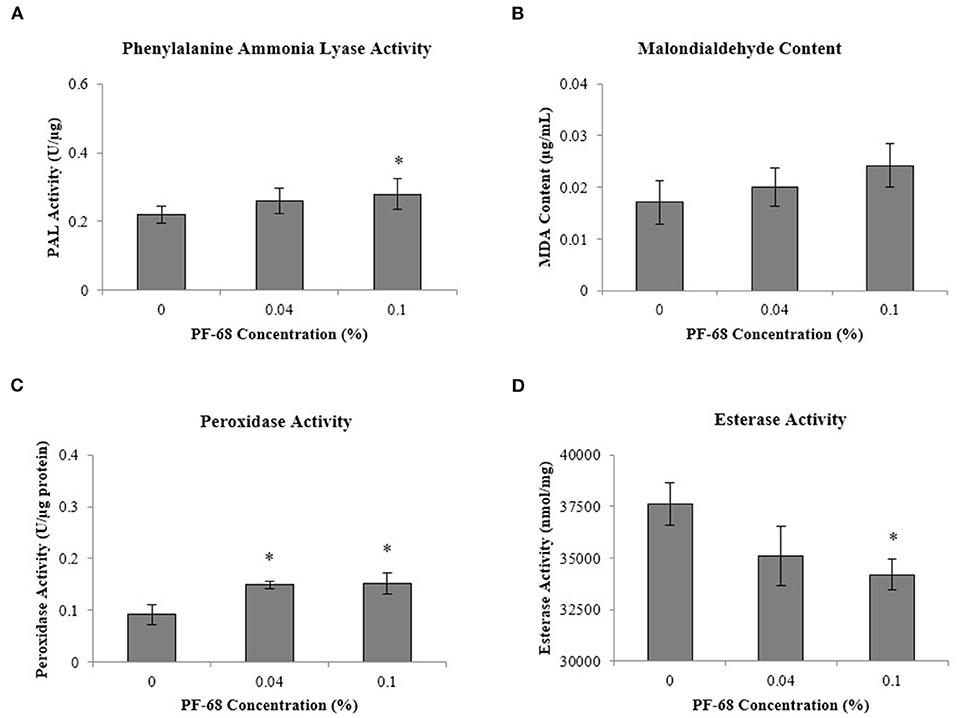
Figure 3. Biochemical analysis performed on extracts of calli grown on Murashige and Skoog (MS) medium supplemented with different PF-68 concentrations without plant hormones (MSO). Control (MSO), optimum (MSO +0.04% PF-68), and high (MSO +0.10% PF-68). (A) Phenylalanine lyase (PAL) activity; (B) malondialdehyde (MDA) content; (C) peroxidase activity; (D) esterase activity. Data shows the mean of three biological replicates. Asterisks (*) indicate values were significantly different from those of the control callus at p < 0.05. Error bars represent SD of three biological replicates.
Nutrient Ions Content Analysis on MR 219 Callus Treated With PF-68
Nutrients availability is crucial to plant growth and development. In order to evaluate the effects of PF-68 on nutrients uptake in MR 219 callus, nutrient ion analysis via atomic absorption spectrometry was performed. Table 1 reveals that calli grown on 0.04% PF-68 contained higher amounts of macronutrients (K, Mg, and Ca) and micronutrients (Fe, Zn, Cu, and Mn), except for Na, which had the same amount as compared with the control. However, calli grown on 0.10% PF-68 contained higher amounts of Ca, Fe, Zn, Cu, and Mn compared with the control. Among these macro- and micronutrients tested, K had the highest increment detected in 0.04% PF-68 (42,600 ppm) when compared with the control (40,600 ppm).

Table 1. The concentration of nutrient ions content in control callus, and calli supplemented with 0.04% PF-68 and 0.10% PF-68 after 4 weeks of incubation.
Comparative Proteomic Analysis on MR 219 Callus Treated With PF-68
Comparative proteomic analysis was carried out between the control, optimum (0.04%), and high concentrations (0.10%) of PF-68 in order to shed light on possible roles of PF-68 in callus growth. Differentially expressed proteins were identified in three different comparing groups; namely, between control and optimum concentration, control and high concentration, and optimum and high concentration. Pearson correlation values in these three groups were of high confidence, indicating the samples used between the different treatment groups were linearly related (Supplementary Figure 1). In addition, principal component analysis revealed good separation between the treatment groups, indicating significant changes in the proteomic abundance between each group (Supplementary Figure 1).
A total of 337 proteins from the control, 353 proteins from the optimum concentration, and 288 proteins from high concentration were successfully identified (Figure 4A). A total of 251 similar proteins were shared between the three treatment groups. In comparison between the control and optimum concentrations, a total of 380 proteins were identified in these two treatments. A total of 315 proteins were identified in both control and high concentrations. When compared between optimum and high concentrations, a total of 320 proteins were identified in both treatments. Based on the analysis, 49 proteins were upregulated, and 62 proteins were downregulated in optimum concentration as compared with the control (Figure 4B; Supplementary Figure 1 and Supplementary Table 2). In comparison between the control and high concentrations, 13 proteins were found to be upregulated, while 19 proteins were downregulated (Figure 4B; Supplementary Figure 1 and Supplementary Table 3). A total of 36 proteins were upregulated, and 48 proteins were downregulated in optimum as compared with a high concentration of PF-68 (Figure 4B; Supplementary Figure 1 and Supplementary Table 4). In addition, 37 proteins were exclusive to the optimum concentration, and 43 proteins were exclusive to the control when compared between the control and optimum concentrations. A total of 18 proteins were exclusive to the high concentration, and 48 proteins were exclusive to the control when compared between the control and high concentrations. When compared between the optimum and high concentrations, 59 proteins were exclusive to the optimum concentration, and 23 proteins were exclusive to the high concentration (Figure 4B). The differentially expressed proteins identified were subjected to Kyoto Encyclopedia of Genes and Genomes (KEGG) pathway analysis, which revealed that PF-68 supplementation affected proteins that are involved in carbohydrate metabolism, amino acid biosynthesis, protein biosynthesis, and secondary metabolites biosynthesis (Figure 4C). Besides, gene expression of selected proteins was observed to be in a similar trend with the proteome profile (Supplementary Figure 2).
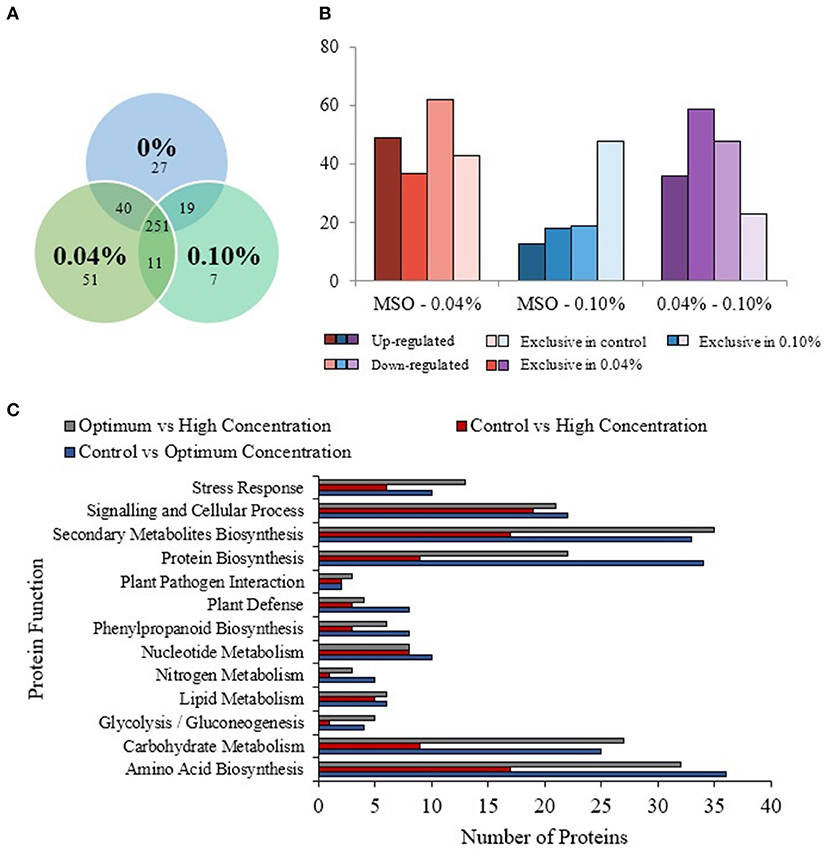
Figure 4. Comparative proteomic analysis of extracts from calli exposed to different PF-68 concentrations. Control (0% PF-68), optimum (0.04% PF-68), and high (0.10% PF-68). (A) Venn diagram of the total proteins obtained from the comparison between three treatments. (B) Total differentially expressed proteins identified in three treatment groups. (C) KEGG-pathway analysis of differentially expressed proteins identified in three treatment groups.
Discussion
The recalcitrant property of indica rice cultivars has been one of the major obstacles in in vitro regeneration of MR 219, particularly poor callus induction and proliferation. Various attempts were made in order to improve in vitro regeneration of MR 219, such as the application of plant additives into the culture medium (Low et al., 2019). A recent study on the application of PF-68 has successfully improved a callus proliferation rate in MR 219 in the presence of plant hormones (Kok et al., 2020). Hence, the present study was undertaken in order to further elucidate the mechanisms governing the growth-promoting response of PF-68 during callus proliferation.
Application of optimum PF-68 concentration alone, without the supplementation of plant hormone, improved callus proliferation of MR 219 rice (Figure 1). These observations were consistent with the previous study made on the application of PF-68 with the presence of plant hormones (Kok et al., 2020). This suggests that PF-68 can act as a growth additive independent of plant growth hormones.
Application of PF-68 was found to be adsorbed onto the cell surfaces, providing protection against physical and chemical stresses in animal cell culture. The interaction between PF-68 and membrane permeability has been studied in yeast, animal cell culture, and plant cell culture (King et al., 1991; Meier et al., 1999; Cho et al., 2007). The incorporation of PF-68 at low concentration has been demonstrated to influence membrane permeability (King et al., 1991). Through enhanced membrane permeability, it was suggested that PF-68 was able to promote nutrient uptake and cell growth in animal cell culture (Shelat et al., 2013).
Soluble sugar molecules such as sucrose, glucose, and fructose play an important role in sugar sensing and plant development. High-sugar content can promote cell growth and carbohydrate storage by producing carbon and energy required for plant growth and development (Eveland and Jackson, 2012). Starch, a branched glucose polymer, also functions as a reserve carbohydrate in plants (Tetlow and Emes, 2014). In the present study, accumulation of soluble sugar was observed in callus treated with optimum PF-68 concentration (Figure 2A). Similar findings were reported in the application of PF-68 during callus growth of S. dulcamarain (Kumar et al., 1992). In response to PF-68, the enhanced accumulation of soluble sugar was reflected with the increases in tissue weights of S. dulcamarain (Kumar et al., 1992). Besides, comparative proteomic analysis revealed that cytosolic invertase 1 protein was found to be exclusive in callus treated with optimum PF-68 (Supplementary Tables 2, 4). In plants, invertase plays a vital role in hydrolyzing sucrose into glucose and fructose, and providing carbon nutrient supply required for cellular biosynthesis and sugar signal transduction (Ruan et al., 2010). For instance, point mutation on cytosolic invertase 1 gene in Arabidopsis was shown to cause sucrose accumulation and reduced plant growth (Qi et al., 2007). Another study reported that mutated cytosolic invertase 1 gene exhibited delayed flowering and partial sterility in rice (Jia et al., 2008). Detection of invertase protein exclusively found in callus treated with optimum PF-68 concentration suggested that production of glucose and fructose from sucrose was enhanced.
Besides, an increase in abundance of alpha-amylase isozyme 3A was detected in callus treated with optimum PF-68 concentration (Table 2). During carbohydrate metabolism, alpha-amylase is produced in abundance, and it is an essential enzyme in plants for catalyzing the hydrolysis of alpha-1,4-glucosidic bonds in starch (Zeeman et al., 2010). In plants, starch degradation is often dependent on carbon availability. For instance, starch degradation was halted when carbon availability was high, while starch degradation was stimulated when low carbon availability was detected in plants (Weise et al., 2006). Supporting this, a 1,4-alpha-glucan-branching enzyme was found to be in low abundance in the callus treated with optimum PF-68 concentration (Supplementary Tables 2, 4). Starch branching enzyme (SBE) like 1,4-alpha-glucan-branching enzyme is one of the major enzymes involved in starch biosynthesis in plants. This SBE can influence the structure of starch in terms of frequency and branch chain length (Tetlow and Emes, 2014). Therefore, the decrease in SBE abundance, as recorded in callus treated with optimum PF68 concentration, suggests that the production of starch has greatly reduced. This further supports that the carbohydrate metabolism was enhanced in order to provide a steady supply of energy and carbon for plant growth and development (Zeeman et al., 2010).
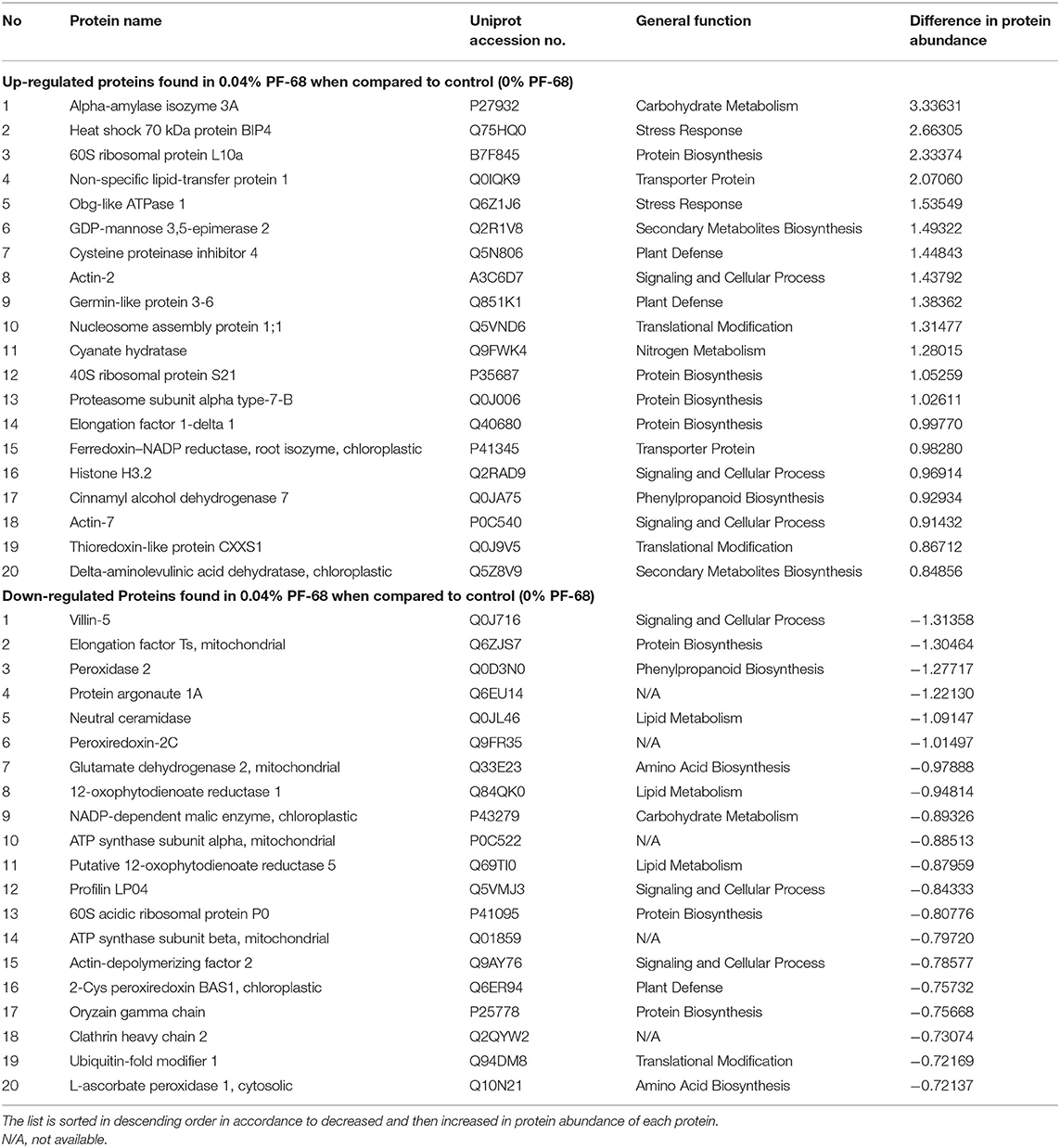
Table 2. Top 20 proteins showing significant abundance difference (together with their accession numbers) between control and optimum PF-68 concentrations.
Aside from soluble sugar accumulation, total protein accumulation plays an important role during plant growth and development as well. Proteins accumulation in plant is often dependent on the availability of nitrogen supply. Nitrogen is one of the essential building blocks of amino acids. In many natural environments, nitrogen is often one of the limiting nutrients, and the decrease in nitrogen availability is often the reason for reduced plant growth. In the present study, enhanced GOGAT activity and increased in abundance of NADH-GOGAT protein were detected in callus treated with optimum PF-68 concentration (Figure 2C and Supplementary Table 2). In plants, GOGAT is one of the key proteins involved in amino acid biosynthesis, specifically nitrogen metabolism. GOGAT isoenzymes catalyze the transfer of the amido nitrogen of glutamine to 2-oxoglutarate, using either pyridine nucleotides (NADH dependent) or ferredoxin (ferredoxin dependent) as a reductant (Konishi et al., 2014). Besides, Chichkova et al. (2001) previously reported that overexpression of the NADH-GOGAT gene in the transgenic tobacco plant was shown to enhance dry weight and total carbon and nitrogen contents. Enhanced GOGAT activity and increased in abundance of NADH-GOGAT protein were observed to be coherent accumulation of total proteins in the callus treated with optimum PF-68 concentration (Figure 2). This indicates that the application of 0.04% PF-68 may play an active role in nitrogen assimilation in callus. The increased nitrogen content will then be used as a source of building blocks for amino acids and subsequently enhances the biosynthesis of proteins, which are crucial to plant growth (Rafiq et al., 2010).
Further comparative proteome analysis revealed an increase in abundance of ribosomal proteins (60S ribosomal protein L10a and 40S ribosomal protein S21) found in callus treated with optimum PF-68 concentration when compared with both control and high PF-68 concentration (Table 3). Ribosomal proteins are well-known for their roles in mediating protein synthesis and maintaining the stability of the ribosomal complex. The ribosome complex, as a whole, ensures the process of initiation of protein synthesis, amino acid assembly, and termination to occur appropriately in the cells (Moin et al., 2016). Regulation of ribosomal proteins is developmental dependent. For instance, high levels of ribosomal proteins were found in plant tissues with active division activities (Ferreyra et al., 2010). Ito et al. (2000) reported that disruption of ribosomal proteins resulted in developmental defects in Arabidopsis, such as an aberrant leaf, retarded root growth, and late flowering. Hence, the increase in the abundance of ribosomal proteins detected in the callus treated with optimum PF-68 concentration may play an important role during cell growth and development. Besides, similar findings were reported on the application of PF-68 on S. dulcamarain, whereby enhanced protein accumulation was observed during callus growth (Kumar et al., 1992).
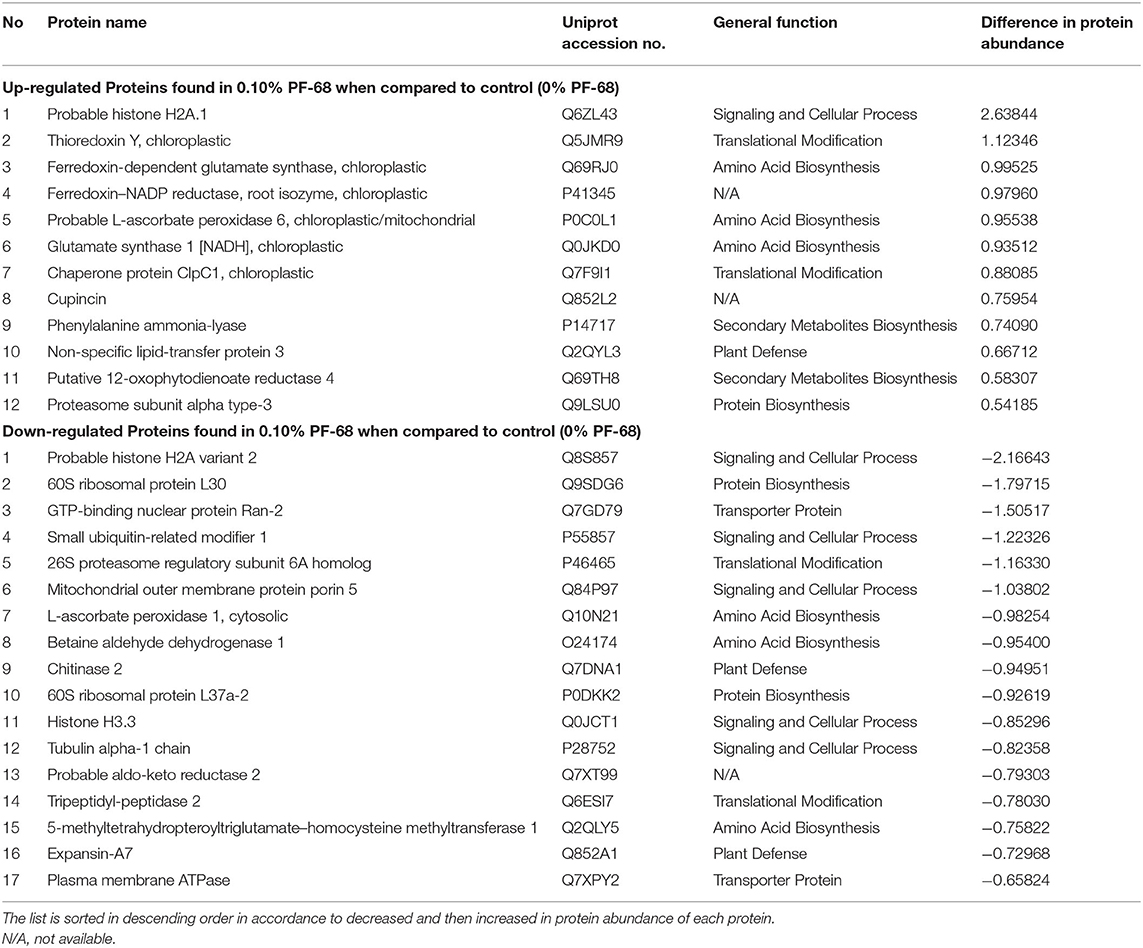
Table 3. Top 20 proteins showing significant abundance difference (together with their accession numbers) when compared between control and high PF-68 concentrations.
Essential nutrients in plant can be classified into macro- or micronutrients. Results obtained in this study revealed that a callus treated with optimum PF-68 concentration had higher amounts of macronutrients (K, Mg, and Ca) and micronutrients (Fe, Zn, Cu, and Mn) compared with the control (Table 1). The increase in nutrient uptake observed may be due to enhanced membrane permeability in response to PF-68. Among these plant nutrients tested, K is an essential nutrient and plays a vital role in plant growth and development, which is supported by the highest amount of K uptake in optimum PF-68 concentration. It is involved in biochemical processes, including protein synthesis and carbohydrate metabolism (Wang et al., 2013). Faust and Schubert (2016) previously reported that protein and sugar contents were significantly reduced in sugar beet due to K deficiency. In addition, accumulation of protein precursors, such as amino acids and amides, was also observed, suggesting that K deficiency can inhibit protein synthesis. Zelelew et al. (2016) also reported that increasing the K level had significant effects on plants, whereby plant height, aerial stem number, and number of leaves per plant were increased in a potato variety they studied. Thus, maintaining a balance of the K level in plants is crucial in plant growth and development. Stunted growth, poor root system, lodging, yield reduction, and yellowing of leaf were also common phenomena that occurred when plants had K deficiency (Wang et al., 2013). Furthermore, K deficiency increased plant susceptibility to various diseases and pest infestation, rendering plants to be more susceptible to damage under various stress conditions (Hasanuzzaman et al., 2018). Based on the results obtained in Table 1, the enhanced nutrients uptake, particularly K, could be one of the effects of PF-68, which contributed to enhanced the callus proliferation rate of MR 219.
Secondary plant metabolites are numerous chemical compounds produced by the plant cells through metabolic pathways derived from the primary metabolic pathways. Phenolic and flavonoid compounds are the largest groups of secondary metabolites in plants, and they play important roles in mediating plant response to biotic and abiotic stresses (Park et al., 2018). In this study, an increase in the abundance of PAL proteins (Table 3 and Supplementary Table 4) and high PAL activity (Figure 3A) were recorded in a callus treated with high PF-68 concentration. PAL catalyzes the first reaction in the biosynthesis of ammonia and trans-cinnamate from phenylalanine. The product is then further transformed into a wide variety of phenylpropanoid natural products, including phenolic, flavonoid, lignin, and phytoalexins (Jun et al., 2018). PAL is crucial in plant growth and development as it is responsible for producing secondary metabolites in response to environmental cues, including UV irradiation, exposure to heavy metals, infection, wounding, low temperatures, and low levels of nitrogen, phosphate, or ions (Zhang and Liu, 2015). In the present study, the increase in PAL activity implied that secondary metabolite biosynthesis was enhanced in a callus treated with a high PF-68 concentration. Notably, the increasing appearance of brown calli observed at a high concentration of PF-68 could be associated with the accumulation of secondary metabolites biosynthesis as well (Figure 1B).
Lignin is a phenolic complex polymer involved in plant growth and development as one of the major components of a secondary wall. It also provides mechanical strength to the cell wall and plays an important role in a defense mechanism against biotic and abiotic stresses (Vanholme et al., 2010). Enhanced cell wall lignification has been widely reported in plants when exposed to environmental stresses. For example, plant adaptation toward salt stress resulted in a significant increase in lignin content in the vascular tissues and thickened cell wall (Chun et al., 2019). In this study, cinnamyl alcohol dehydrogenase (CAD) protein was found to be exclusive in a callus treated with high PF-68 concentration as compared with optimum PF-68 concentration (Supplementary Table 4). This CAD has been widely characterized, and it is known to play a role in lignin biosynthesis via the conversion of phenylpropenyl aldehydes to alcohols (Ma et al., 2018). Suppression of CAD was demonstrated to reduce the lignin content and alter the lignin composition in switchgrass (Fu et al., 2011). Based on these results, the presence of CAD protein exclusive to a callus treated with high PF-68 concentration suggested that lignin biosynthesis was enhanced in response to increasing stress induced by high PF-68 concentration.
Reactive oxygen species (ROS) is one of the by-products of aerobic metabolism. Peroxidation of lipid membrane is one of the damaging effects of ROS. During the membrane lipid peroxidation process, ROS removes electrons from the lipids in the cell membrane, disrupting cell membrane system of the plant. Enhancement in membrane lipid peroxidation caused an increase in membrane permeability of the plant, which eventually caused electrolytes leakage in a plant cell (Campo et al., 2014). The results obtained in this study showed that enhanced MDA content was recorded in 0.10% PF-68 (Figure 3B). MDA is a major product of lipid peroxidation, and it reflects the degree of lipid peroxidation in plant cells in response to stress (Song et al., 2016). Similar significant levels of lipid peroxidation were detected in forest trees (Zhou et al., 2014) and rapeseed (Jin et al., 2010) in response to abiotic stresses. A significant increase in MDA levels indicates severe oxidative damage occurring in the plant cell membrane. However, studies have found that lipid peroxidation is a common phenomenon that occurred in plant cells when subjected to stress. MDA was often used as a marker to determine the physiological status of the plant during plant growth (Talbi et al., 2015; Kong et al., 2016). In this study, a high level of MDA was detected at a high concentration of PF-68, which signified oxidative damage in the cell membrane. This observation is able to prove the previous hypothesis made on high PF-68 concentration, whereby high PF-68 concentration induced detrimental and irreversible changes in the plasma membrane in plant cells (Curtis and Mirkov, 2012; Irshad et al., 2018).
In line with the raised MDA content, an increase in protein abundance involved in the antioxidant defense system, such as peroxidase protein and peroxiredoxin proteins, was also detected in a callus treated with high PF-68 concentration (Table 4 and Supplementary Table 4). Consistently, the biochemical assessment revealed similar high peroxidase activity in a callus treated with high PF-68 concentration (Figure 3C). Low levels of ROS detected are common in plants as ROS is a by-product of plant aerobic metabolism. Moreover, ROS serves as an important signaling molecule to regulate physiological processes (Wang et al., 2016). However, the accumulation of ROS may cause excessive damage toward macromolecules and plant cells, which eventually triggers a hypersensitive response and programmed cell death (PCD) in plant cells (Konieczny et al., 2014). The excessive production of ROS is often facilitated by increasing stress response (Konieczny et al., 2014). In order to regulate ROS intracellular levels in plants, ROS scavenging enzymes, such as catalase, peroxiredoxin, and peroxidase are required (Chou et al., 2012). The increased synthesis of peroxidase and peroxiredoxin proteins detected in the callus treated with high PF-68 concentration showed that the regulation of ROS was enhanced in response to increasing oxidative stress, induced by high PF-68 concentration.
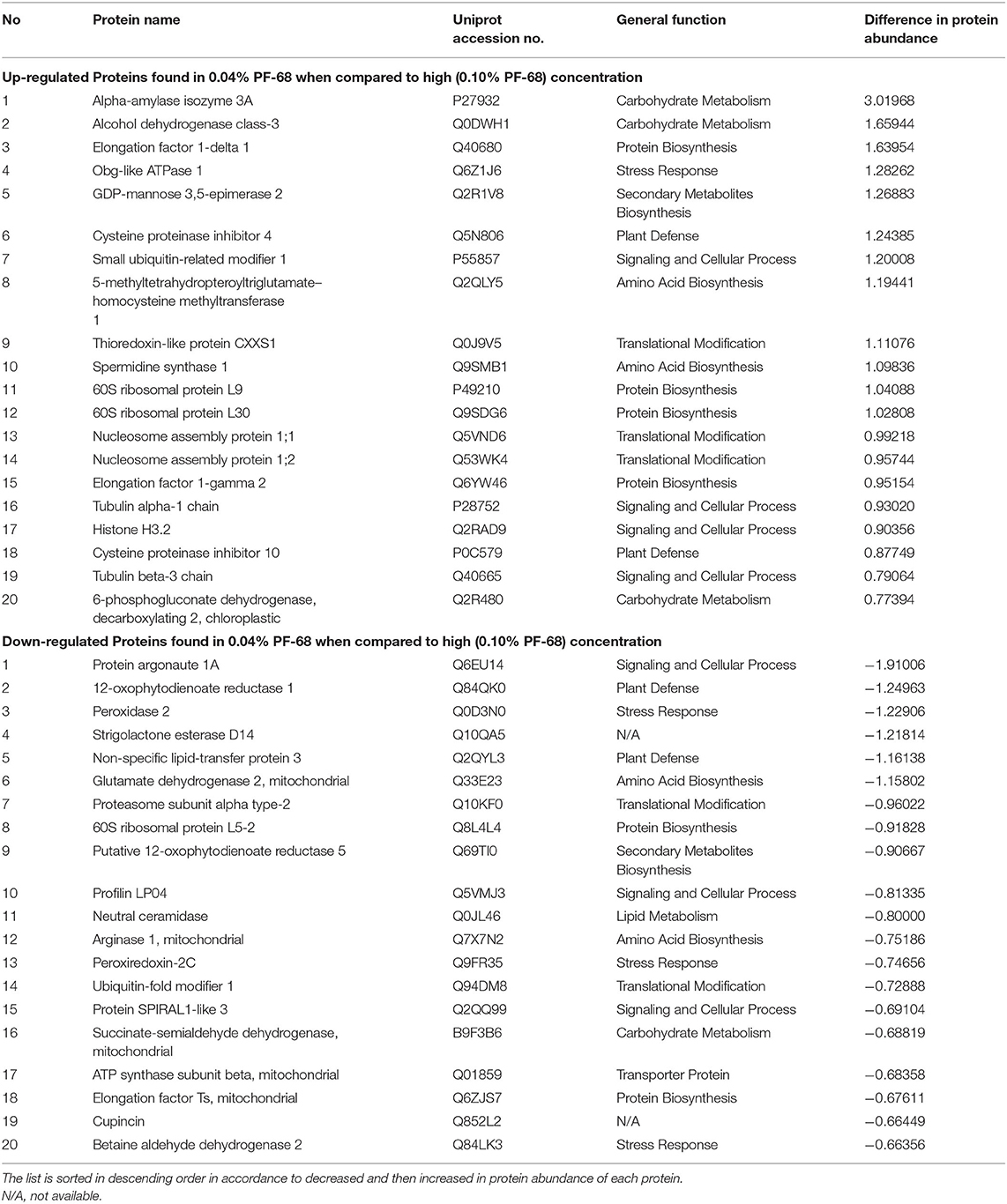
Table 4. Top 20 proteins showing significant abundance difference (together with their accession numbers) when compared between optimum and high PF-68 concentrations.
Aside from protein abundance in plants, esterase could be used to determine cell viability. Several studies have utilized the changes in esterase activity as a marker to study the growth characteristics and cell viability in plants (Amano et al., 2003; Tamás et al., 2005; Víteček et al., 2007). In a way, a decrease in esterase activity in plants corresponds with the decrease in cell viability in plants. Besides, esterase activity can also act as an excellent bioindicator toward environmental stresses (Radić and Pevalek-Kozlina, 2010). In the present study, the decrease in esterase activity in a high concentration of PF-68 could be associated with the decrease in the calli cells viability. This association was supported by the increasing number of black calli recorded at a high concentration of PF-68 (Figure 1B). The decrease in cell viability was most probably due to the accumulation of ROS (Figure 2C) and the incapability of the ROS scavenging system to cope with the increasing stress induced by high concentration of PF-68, which eventually triggered PCD in the callus cells (Figure 3D).
Conclusions
Despite the growth-promoting effects of PF-68 were known, the mechanism governing the growth-promoting effects remains largely fragmented. The present study demonstrates that the growth-promoting effects of PF-68 are independent of a plant hormone. The comparative proteome analysis revealed that optimum PF-68 concentration enhanced callus proliferation of MR 219 cultivar via enhanced sugar accumulation and protein biosynthesis. This was evidenced by the increase in the abundance of carbohydrate metabolism-related proteins, ribosomal proteins, and nitrogen metabolism-related proteins (Figure 5). Moreover, optimum PF-68 concentration enhanced nutrients uptake in the callus, particularly K, suggesting a vital role of K in plant growth and development. However, a callus treated with high PF-68 concentration revealed increasing levels of stress response, such that high levels of PAL activity, MDA content, and peroxidase activity were detected (Figure 5). Consistently, an increased abundance of PAL protein and proteins involved in the antioxidant defense system was detected in a callus treated with high PF-68 concentration as well. In addition, the reduced level of esterase activity detected at a callus treated with high PF-68 concentration suggests that increasing stress response eventually triggered PCD. Taken together, the growth-promoting mechanism of PF-68 is concentration-dependent, and incorporation of PF-68 at different concentrations may either promote plant growth or induce stress.
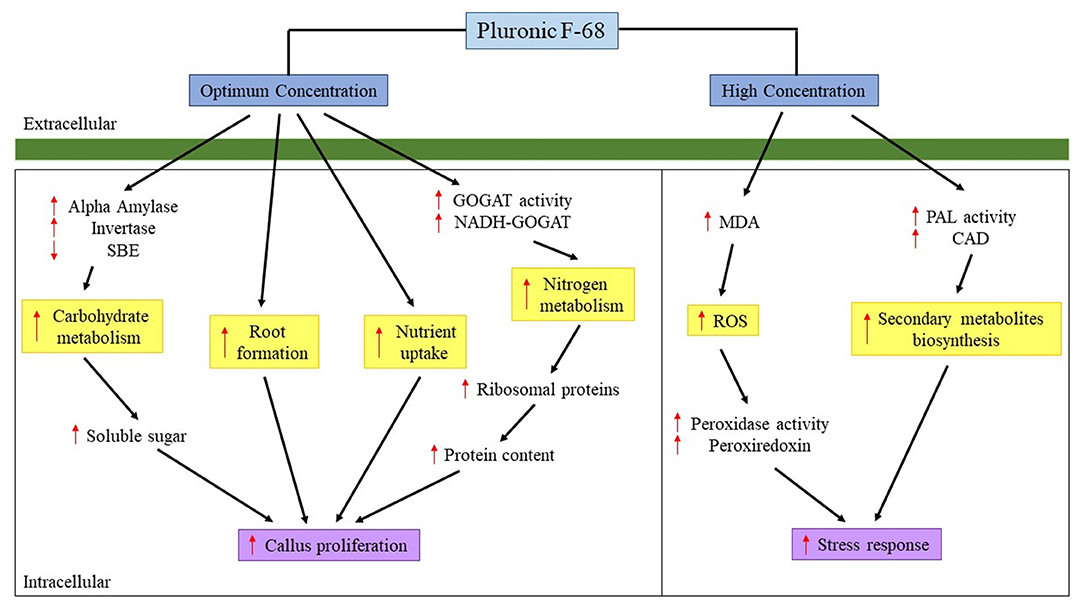
Figure 5. Mechanism proposed on the roles of PF-68 in callus proliferation. CAD, cinnamyl alcohol dehydrogenase; GOGAT, glutamate synthase; MDA, malondialdehyde; NADH-GOGAT, NADH-dependent glutamate synthase; PAL, phenylalanine ammonia lyase; ROS, reactive oxygen species; SBE, starch branching enzyme; ↑, increased; ↓, decreased.
Data Availability Statement
The original contributions presented in the study are included in the article/Supplementary Materials, further inquiries can be directed to the corresponding author/s. Processed proteomic data can be found in the Supplementary Materials.
Author Contributions
K-SL and JO-A conceived and designed the experiments. AK performed research, analyzed data, and wrote the manuscript. NM, RS, C-YW, and DL contributed to analytical tools/reagents/funding acquisition. All the authors have read, contributed, and approved the manuscript.
Conflict of Interest
The authors declare that the research was conducted in the absence of any commercial or financial relationships that could be construed as a potential conflict of interest.
Acknowledgments
The authors would like to acknowledge the Fundamental Research Grant Scheme (FRGS/1/2014/SG05/MOSTI/1), UPM Graduate Research Fellowship, and UPM Putra Grants (GP-IPS/2017/9572000) for the research funds.
Supplementary Material
The Supplementary Material for this article can be found online at: https://www.frontiersin.org/articles/10.3389/fpls.2021.667434/full#supplementary-material
Data Sheet 1. 0.04% PF-68.
Data Sheet 2. 0.10% PF-68.
Data Sheet 3. Control.
Data Sheet 4. Perseus Proteomic Analysis on Pluronic F-68.
Data Sheet 5. Supplementary Tables and Figures.
Data Sheet 6. Proteomic Data Analysis.
Abbreviations
CAD, Cinnamyl alcohol dehydrogenase; DW, Dry weight; FW, Fresh weight; GOGAT, Glutamate synthase; MDA, Malondialdehyde; MS, Murashige and Skoog; MSO, Murashige and Skoog without plant growth regulators; PAL, Phenylalanine ammonia lyase; PF-68, Pluronic F-68; ROS, Reactive oxygen species; SBE, Starch branching enzyme.
References
Abiri, R., Maziah, M., Shaharuddin, N. A., Yusof, Z. N. B., Atabaki, N., Hanafi, M. M., et al. (2017). Enhancing somatic embryogenesis of Malaysian rice cultivar MR219 using adjuvant materials in a high-efficiency protocol. Int. J. Environ. Sci. Technol. 14, 1091–1108. doi: 10.1007/s13762-016-1221-y
Agostini, E., De Forchetti, S. M., and Tigier, H. A. (1997). Production of peroxidases by hairy roots of Brassica napus. Plant Cell Tissue Organ Cult. 47, 177–182. doi: 10.1007/BF02318955
Amano, T., Hirasawa, K. I., O'Donohue, M. J., Pernolle, J. C., and Shioi, Y. (2003). A versatile assay for the accurate, time-resolved determination of cellular viability. Anal. Biochem. 314, 1–7. doi: 10.1016/S0003-2697(02)00653-X
Barbulescu, D. M., Burton, W. A., and Salisbury, P. A. (2011). Pluronic F-68: an answer for shoot regeneration recalcitrance in microspore-derived Brassica napus embryos. In Vitro Cell. Dev. Biol. Plant. 47, 282–288. doi: 10.1007/s11627-011-9353-8
Bassetti, L., Hagendoorn, M., and Tramper, J. (1995). Surfactant-induced non-lethal release of anthraquinones from suspension cultures of Morindacitrifolia. J. Biotechnol. 39, 149–155. doi: 10.1016/0168-1656(95)00004-A
Biswas, A., and Mandal, A. B. (2007). Plant regeneration in different genotypes of indica rice. Indian J. Biotechnol. 6, 532–540.
Campo, S., Baldrich, P., Messeguer, J., Lalanne, E., Coca, M., and Segundo, B. S. (2014). Overexpression of a calcium-dependent protein kinase confers salt and drought tolerance in rice by preventing membrane lipid peroxidation. Plant Physiol. 165, 688–704. doi: 10.1104/pp.113.230268
Chichkova, S., Arellano, J., Vance, C. P., and Hernández, G. (2001). Transgenic tobacco plants that overexpress alfalfa NADH-glutamate synthase have higher carbon and nitrogen content. J. Exp. Bot. 52, 2079–2087. doi: 10.1093/jexbot/52.364.2079
Cho, J.-M., Kwon, J.-Y., Lim, J.-A., and Kim, D.-I. (2007). Increased hGM-CSF production and secretion with Pluronic F-68 in transgenicNicotiana tabacum suspension cell cultures. Biotechnol. Bioprocess Eng. 12, 594–600. doi: 10.1007/BF02931074
Chou, T.-S., Chao, Y.-Y., and Kao, C. H. (2012). Involvement of hydrogen peroxide in heat shock- and cadmium-induced expression of ascorbate peroxidase and glutathione reductase in leaves of rice seedlings. J. Plant Physiol. 169, 478–486. doi: 10.1016/j.jplph.2011.11.012
Chun, H. J., Baek, D., Cho, H. M., Lee, S. H., Jin, B. J., Yun, D. J., et al. (2019). Lignin biosynthesis genes play critical roles in the adaptation of Arabidopsis plants to high-salt stress. Plant Signal. Behav. 14:1625697. doi: 10.1080/15592324.2019.1625697
Curtis, I. S., and Mirkov, T. E. (2012). Influence of surfactants on growth and regeneration from mature internodal stem segments of sweet orange (Citrus sinensis) cv. Hamlin. Plant Cell Tissue Organ Cult. 108, 345–352. doi: 10.1007/s11240-011-0037-1
Dashti, S., Habashi, A. A., Azghandi, A. V., Abdollahi, H., and Chamani, M. (2012). Effects of pluronic F-68 on regeneration and rooting of two pear cultivars (Pyrus communiscvs Dar Gazi and Bartlett). Int. Res. J. Appl. Basic Sci. 3, 190–196.
Eckermann, C., Eichel, J., and Schröder, J. (2000). Plant methionine synthase: new insights into properties and expression. Biol. Chem. 381, 695–703. doi: 10.1515/BC.2000.090
Ertani, A., Francioso, O., Tugnoli, V., Righi, V., and Nardi, S. (2011). Effect of commercial lignosulfonate-humate on Zea mays L. metabolism. J. Agric. Food Chem. 59, 11940–11948. doi: 10.1021/jf202473e
Eveland, A. L., and Jackson, D. P. (2012). Sugars, signaling, and plant development. J. Exp. Bot. 63, 3367–3377. doi: 10.1093/jxb/err379
Faust, F., and Schubert, S. (2016). Protein synthesis is the most sensitive process when potassium is substituted by sodium in the nutrition of sugar beet (Beta vulgaris). Plant Physiol. Biochem. 107, 237–247. doi: 10.1016/j.plaphy.2016.06.009
Ferreyra, M. L. F., Pezza, A., Biarc, J., Burlingame, A. L., and Casati, P. (2010). Plant L10 ribosomal proteins have different roles during development and translation under ultraviolet-B stress. Plant Physiol. 153, 1878–1894. doi: 10.1104/pp.110.157057
Fu, C., Xiao, X., Xi, Y., Ge, Y., Chen, F., Bouton, J., et al. (2011). Downregulation of cinnamyl alcohol dehydrogenase (CAD) leads to improved saccharification efficiency in switchgrass. Bioenergy Res. 4, 153–164. doi: 10.1007/s12155-010-9109-z
Gamborg, O. L., Miller, R. A., and Ojima, K. (1968). Nutrient requirements of suspension cultures of soybean root cells. Exp. Cell Res. 50, 151–158. doi: 10.1016/0014-4827(68)90403-5
Hadiarto, T., and Tran, L. S. P. (2011). Progress studies of drought-responsive genes in rice. Plant Cell Rep. 30, 297–310. doi: 10.1007/s00299-010-0956-z
Hasanuzzaman, M., Bhuyan, M., Nahar, K., Hossain, M., Mahmud, J., Hossen, M., et al. (2018). Potassium: a vital regulator of plant responses and tolerance to abiotic stresses. Agron. J. 8:31. doi: 10.3390/agronomy8030031
He, C., Wang, L., Liu, J., Liu, X., Li, X., Ma, J., et al. (2013). Evidence for ‘silicon' within the cell walls of suspension-cultured rice cells. New Phytol. 2013, 700–709. doi: 10.1111/nph.12401
Heath, R. L., and Packer, L. (1968). Photoperoxidation in isolated chloroplasts. I. Kinetics and stoichiometry of fatty acid peroxidation. Arch. Biochem. Biophys. 125, 189–198. doi: 10.1016/0003-9861(68)90654-1
Irshad, M., Rizwan, H. M., Debnath, B., Anwar, M., Li, M., Liu, S., et al. (2018). Ascorbic acid controls lethal browning and Pluronic F-68 promotes high-frequency multiple shoot regeneration from cotyldonary node explant of Okra (Abelmoschus esculentus L.). HortScience 53, 183–190. doi: 10.21273/HORTSCI12315-17
Ito, K., Yamaguchi, M., Noma, T., Yamaji, T., Itoh, H., and Oda, M. (2000). Whey protein hydrolysates enhance water absorption in the perfused small intestine of anesthetized rats. Biosci. Biotechnol. Biochem. 80, 1587–1593. doi: 10.1080/09168451.2016.1166931
Jia, L. Q., Zhang, B., Mao, C., Li, J., Wu, Y., Wu, P., et al. (2008). OsCYT-INV1 for alkaline/neutral invertase is involved in root cell development and reproductivity in rice (Oryza sativa L.). Planta 228, 51–59. doi: 10.1007/s00425-008-0718-0
Jiang, L., He, L., and Fountoulakis, M. (2004). Comparison of protein precipitation methods for sample preparation prior to proteomic analysis. J. Chromatogr. A 1023, 317–320. doi: 10.1016/j.chroma.2003.10.029
Jin, Z. L., Zhang, F., Ahmed, Z. I., Rasheed, M., Naeem, M. S., Ye, Q. F., et al. (2010). Differential morphological and physiological responses of two oilseed Brassica species to a new herbicide ZJ0273 used in rapeseed fields. Pestic. Biochem. Physiol. 98, 1–8. doi: 10.1016/j.pestbp.2010.04.002
Jun, S. Y., Sattler, S. A., Cortez, G. S., Vermerris, W., Sattler, S. E., and Kang, C. H. (2018). Biochemical and structural analysis of substrate specificity of a phenylalanine ammonia-lyase. Plant Physiol. 176, 1452–1468. doi: 10.1104/pp.17.01608
Karpiuk, U. V., Al Azzam, K. M., Mahmoud Abudayeh, Z. H., Kislichenko, V., Naddaf, A., Cholak, I., et al. (2016). Qualitative and quantitative content determination of macro-minor elements in Bryonia alba l. roots using flame atomic absorption spectroscopy technique. Adv. Pharm. Bull. 6, 285–291. doi: 10.15171/apb.2016.040
Khush, G. S. (2005). What it will take to feed 5.0 billion rice consumers in 2030. Plant Mol. Biol. 59, 1–6. doi: 10.1007/s11103-005-2159-5
King, A. T., Davey, M. R., Mellor, I. R., Mulligan, B. J., and Lowe, K. C. (1991). Surfactant effects on yeast cells. Enzyme Microb. Technol. 13, 148–153. doi: 10.1016/0141-0229(91)90171-6
Kok, A. D. X., Low, L. Y., Sekeli, R., Wee, C. Y., Yusof, Z. N. B., and Lai, K. S. (2018). “Iron biofortification of rice: progress and prospects,” in Rice Crop - Current Developments, eds F. Shah, Z. H. Khan and A. Iqbal (Norderstedt: IntechOpen), 25–44.
Kok, A. D. X., Wan Abdullah, W. M. A. N., Tan, N.-P., Ong-Abdullah, J., Sekeli, R., Wee, C.-Y., et al. (2020). Growth promoting effects of Pluronic F-68 on callus proliferation of recalcitrant rice cultivar. 3Biotech 10:116. doi: 10.1007/s13205-020-2118-5
Kong, W., Liu, F., Zhang, C., Zhang, J., and Feng, H. (2016). Non-destructive determination of malondialdehyde (MDA) distribution in oilseed rape leaves by laboratory scale NIR hyperspectral imaging. Sci. Rep. 6, 1–8. doi: 10.1038/srep35393
Konieczny, R., Banaś, A. K., Surówka, E., Michalec, Z., Miszalski, Z., and Libik-Konieczny, M. (2014). Pattern of antioxidant enzyme activities and hydrogen peroxide content during developmental stages of rhizogenesis from hypocotyl explants of Mesembryanthemum crystallinum L. Plant Cell Rep. 33, 165–177. doi: 10.1007/s00299-013-1520-4
Konishi, N., Ishiyama, K., Matsuoka, K., Maru, I., Hayakawa, T., Yamaya, T., et al. (2014). NADH-dependent glutamate synthase plays a crucial role in assimilating ammonium in the Arabidopsis root. Physiol. Plant 152, 138–151. doi: 10.1111/ppl.12177
Kruger, N. J. (1994). The Bradford method for protein quantitation. Methods Mol. Biol. 32, 9–15. doi: 10.1385/0-89603-268-X:9
Kulathuran, G. K., and Narayanasamy, J. (2015). Evaluation of Pluronic F68 and PGR's for high frequency somatic embryogenesis and plant regeneration in castor (Ricinus communis L.) through solid culture. Int. J. Curr. Biotechnol. 3, 1–10.
Kumar, V., Laouar, L., Davey, M. R., Mulligan, B. J., and Lowe, K. C. (1992). Pluronic F-68 stimulates growth of Solanum dulcamarain culture. J. Exp. Bot. 43, 487–493. doi: 10.1093/jxb/43.4.487
Kumari, S., Roy, S., Singh, P., Singla-Pareek, S. L., and Pareek, A. (2013). Cyclophilin: Proteins in search of function. Plant Signal Behav. 8:e22734. doi: 10.4161/psb.22734
Lai, K. S., Abdullah, P., Yusoff, K., and Mahmood, M. (2011). An efficient protocol for particle bombardment-mediated transformation of Centella asiatica callus. Acta Physiol. Plant 33, 2547–2552. doi: 10.1007/s11738-011-0786-9
Lai, K. S., and Masatsugu, T. (2013). Isolation and characterization of an Arabidopsis thaliana self-incompatibility mutant induced by heavy-ion beam irradiation. Acta Biol. Crac. Ser. Bot. 55, 146–152. doi: 10.2478/abcsb-2013-0024
Lim, Y. Y., and Lai, K. S. (2017). Generation of transgenic rice expressing cyclotide precursor Oldenlandia affinis kalata B1 protein. J. Anim. Plant Sci. 27, 667–671.
Livak, K. J., and Schmittgen, T. D. (2001). Analysis of relative gene expression data using real-time quantitative PCR and the 2−ΔΔCT method. Methods 25, 402–408. doi: 10.1006/meth.2001.1262
Low, L. Y., Ong-Abdullah, J., Wee, C. Y., Sekeli, R., Tan, C. K., Loh, J. Y., et al. (2019). Effects of lignosulfonates on callus proliferation and shoot induction of recalcitrant indica rice. Sains Malays. 48, 7–13. doi: 10.17576/jsm-2019-4801-02
Low, L. Y., Yang, S. K., Kok, A. D. X., Ong-Abdullah, J., Tan, N. P., and Lai, K. S. (2018). “Transgenic plants: gene constructs, vector and transformation method,” in New Visions in Plant Science, ed Y. O. Çiftçi (Norderstedt: IntechOpen), 41–61.
Ma, D. M., Xu, C., Alejos-Gonzalez, F., Wang, H., Yang, J., Judd, R., et al. (2018). Overexpression of Artemisia annua cinnamyl alcohol dehydrogenase increases lignin and coumarin and reduces artemisinin and other sesquiterpenes. Front. Plant Sci. 9:828. doi: 10.3389/fpls.2018.00828
Mata-Pérez, C., and Spoel, S. H. (2014). Thioredoxin-mediated redox signalling in plant immunity. Plant Sci. 279, 27–33. doi: 10.1016/j.plantsci.2018.05.001
Meier, S. J., Hatton, T. A., and Wang, D. I. C. (1999). Cell death from bursting bubbles: role of cell attachment to rising bubbles in sparged reactors. Biotechnol. Bioeng. 62, 468–478.
Moin, M., Bakshi, A., Saha, A., Dutta, M., Madhav, S. M., and Kirti, P. B. (2016). Rice ribosomal protein large subunit genes and their spatio-temporal and stress regulation. Front. Plant Sci. 7:1284. doi: 10.3389/fpls.2016.01284
Murashige, T., and Skoog, F. (1962). A revised medium for rapid growth and bio assays with tobacco tissue cultures. Physiol. Plant 15, 473–497. doi: 10.1111/j.1399-3054.1962.tb08052.x
Park, S. H., Lee, C. W., Cho, S. M., Lee, H., Park, H., Lee, J., et al. (2018). Crystal structure and enzymatic properties of chalcone isomerase from the Antarctic vascular plant Deschampsia antarctica Desv. PLoS ONE 13:e0192415. doi: 10.1371/journal.pone.0192415
Qi, X., Wu, Z., Li, J., Mo, X., Wu, S., Chu, J., et al. (2007). AtCYT-INV1, a neutral invertase, is involved in osmotic stress-induced inhibition on lateral root growth in Arabidopsis. Plant Mol. Biol. 64, 575–587. doi: 10.1007/s11103-007-9177-4
Radić, S., and Pevalek-Kozlina, B. (2010). Differential esterase activity in leaves and roots of Centaurea ragusina L. as a consequence of salinity. Period. Biol. 112, 253–258.
Rafiq, M. A., Ali, A., Malik, M. A., and Hussain, M. (2010). Effect of fertilizer levels and plant densities on yield and protein contents of autumn planted maize. P. K. Agric. Sci. 6, 201–208.
Ruan, Y. L., Jin, Y., Yang, Y. J., Li, G. J., and Boyer, J. S. (2010). Sugar input, metabolism, and signaling mediated by invertase: roles in development, yield potential, and response to drought and heat. Mol. Plant 3, 942–955. doi: 10.1093/mp/ssq044
Sah, S. K., Kaur, A., Kaur, G., and Cheema, G. S. (2014). Genetic transformation of rice: Problems, progress and prospects. Rice Res. 3, 1–10. doi: 10.4172/2375-4338.1000132
Sen, S., Chakraborty, R., and Kalita, P. (2020). Rice-not just a staple food: a comprehensive review on its phytochemicals and therapeutic potential. Trends Food Sci. Technol. 97, 265–285. doi: 10.1016/j.tifs.2020.01.022
Sharma, B., Joshi, D., Yadav, P. K., Gupta, A. K., and Bhatt, T. K. (2016). Role of ubiquitin-mediated degradation systemin plant biology. Front. Plant Sci. 7:806. doi: 10.3389/fpls.2016.00806
Shelat, P. B., Plant, L. D., Wang, J. C., Lee, E., and Marks, J. D. (2013). The membrane-active tri-block copolymer Pluronic F-68 profoundly rescues rat hippocampal neurons from oxygen-glucose deprivation-induced death through early inhibition of apoptosis. J. Neurosci. 33, 12287–12299. doi: 10.1523/JNEUROSCI.5731-12.2013
Song, X., Wang, Y., and Lv, X. (2016). Responses of plant biomass, photosynthesis and lipid peroxidation to warming and precipitation change in two dominant species (Stipa grandis and Leymus chinensis) from North China Grasslands. Ecol. Evol. 6, 1871–1882. doi: 10.1002/ece3.1982
Talbi, S., Romero-Puertas, M. C., Hernández, A., Terrón, L., Ferchichi, A., and Sandalio, L. M. (2015). Drought tolerance in a Saharian plant Oudneyaafricana: role of antioxidant defences. Environ. Exp. Bot. 111, 114–126. doi: 10.1016/j.envexpbot.2014.11.004
Tamás, L., Huttová, J., Mistrík, I., Šimonovičová, M., and Široká, B. (2005). Aluminium induced esterase activity and isozyme pattern in barley root tip. Plant Soil Environ. 51, 220–225. doi: 10.17221/3577-PSE
Terzi, R., Kadioglu, A., Kalaycioglu, E., and Saglam, A. (2014). Hydrogen peroxide pretreatment induces osmotic stress tolerance by influencing osmolyte and abscisic acid levels in maize leaves. J. Plant Interact. 9, 559–565. doi: 10.1080/17429145.2013.871077
Tetlow, I. J., and Emes, M. J. (2014). A review of starch-branching enzymes and their role in amylopectin biosynthesis. IUBMB Life 66, 546–558. doi: 10.1002/iub.1297
Valentini, G., Chiarelli, L., Fortin, R., Speranza, M. L., Galizzi, A., and Mattevi, A. (2000). The allosteric regulation of pyruvate kinase. J. Biol. Chem. 275, 18145–18152. doi: 10.1074/jbc.M001870200
Vanholme, R., Demedts, B., Morreel, K., Ralph, J., and Boerjan, W. (2010). Lignin biosynthesis and structure. Plant Physiol. 153, 895–905. doi: 10.1104/pp.110.155119
Víteček, J., Wünschová, A., Petrek, J., Adam, V., Kizek, R., and Havel, L. (2007). Cell death induced by sodium nitroprusside and hydrogen peroxide in tobacco BY-2 cell suspension. Biol. Plant 51, 472–479. doi: 10.1007/s10535-007-0099-4
Wan Abdullah, W. M. A. N., Low, L. Y., Mumaiyizah, S. B., Chai, Q. Y., Loh, J. Y., Ong-Abdullah, J., et al. (2020). Effect of lignosulphonates on Vanilla planifolia shoot multiplication, regeneration and metabolism. Acta Physiol. Plant 42, 1–8. doi: 10.1007/s11738-020-03099-9
Wang, C.-H., Yu, J., Cai, Y.-X., Zhu, P.-P., Liu, C.-Y., Zhao, A.-C., et al. (2016). Characterization and functional analysis of 4-coumarate:CoA ligase genes in mulberry. PLoS ONE 11:e0155814. doi: 10.1371/journal.pone.0155814
Wang, M., Zheng, Q., Shen, Q., and Guo, S. (2013). The critical role of potassium in plant stress response. Int. J. Mol. Sci. 14, 7370–7390. doi: 10.3390/ijms14047370
Watanabe, N., and Lam, E. (2008). BAX inhibitor-1 modulates endoplasmic reticulum stress-mediated programmed cell death in Arabidopsis. J. Biol. Chem. 283, 3200–3210. doi: 10.1074/jbc.M706659200
Weise, S. E., Schrader, S. M., Kleinbeck, K. R., and Sharkey, T. D. (2006). Carbon balance and circadian regulation of hydrolytic and phosphorolytic breakdown of transitory starch. Plant Physiol. 141, 879–886. doi: 10.1104/pp.106.081174
Yang, S. K., Yusoff, K., Ajat, M., Thomas, W., Abushelaibi, A., Akseer, R., et al. (2019). Disruption of KPC-producing Klebsiella pneumoniae membrane via induction of oxidative stress by cinnamon bark (Cinnamomum verum J. Presl) essential oil. PLoS ONE. 14:e0214326. doi: 10.1371/journal.pone.0214326
Yildirim, A. B., and Turker, A. U. (2014). Effects of regeneration enhancers on micropropagation of Fragaria vesca L. and phenolic content comparison of field-grown and in vitro-grown plant materials by liquid chromatography-electrospray tandem mass spectrometry (LC-ESI-MS/MS). Sci Hortic. 169, 169–178. doi: 10.1016/j.scienta.2014.01.038
Zeeman, S. C., Kossmann, J., and Smith, A. M. (2010). Starch: its metabolism, evolution, and biotechnological modification in plants. Annu. Rev. Plant Biol. 61, 209–234. doi: 10.1146/annurev-arplant-042809-112301
Zelelew, D. Z., Lal, S., Kidane, T. T., and Ghebreslassie, B. M. (2016). Effect of potassium levels on growth and productivity of potato varieties. Am. J. Plant Sci. 7, 1629–1638. doi: 10.4236/ajps.2016.712154
Zhang, H., Qu, X., Bao, C., Khurana, P., Wang, Q., Xie, Y., et al. (2010). Arabidopsis VILLIN5, an actin filament bundling and severing protein, is necessary for normal pollen tube growth. Plant Cell. 22, 2749–2767. doi: 10.1105/tpc.110.076257
Zhang, X., and Liu, C. J. (2015). Multifaceted regulations of gateway enzyme phenylalanine ammonia-lyase in the biosynthesis of phenylpropanoids. Mol. Plant 8, 17–27. doi: 10.1016/j.molp.2014.11.001
Keywords: growth additive, growth promoting effects, mode of action, recalcitrant indica cv. MR 219, stress response
Citation: Kok AD-X, Mohd Yusoff NF, Sekeli R, Wee C-Y, Lamasudin DU, Ong-Abdullah J and Lai K-S (2021) Pluronic F-68 Improves Callus Proliferation of Recalcitrant Rice Cultivar via Enhanced Carbon and Nitrogen Metabolism and Nutrients Uptake. Front. Plant Sci. 12:667434. doi: 10.3389/fpls.2021.667434
Received: 03 March 2021; Accepted: 27 April 2021;
Published: 02 June 2021.
Edited by:
Luis Herrera-Estrella, Texas Tech University, United StatesReviewed by:
Yaroslav B. Blume, Institute of Food Biotechnology and Genomics, UkraineAhmad A. Omar, Zagazig University, Egypt
Copyright © 2021 Kok, Mohd Yusoff, Sekeli, Wee, Lamasudin, Ong-Abdullah and Lai. This is an open-access article distributed under the terms of the Creative Commons Attribution License (CC BY). The use, distribution or reproduction in other forums is permitted, provided the original author(s) and the copyright owner(s) are credited and that the original publication in this journal is cited, in accordance with accepted academic practice. No use, distribution or reproduction is permitted which does not comply with these terms.
*Correspondence: Janna Ong-Abdullah, amFubmEmI3gwMDA0MDt1cG0uZWR1Lm15; Kok-Song Lai, bGtva3NvbmcmI3gwMDA0MDtoY3QuYWMuYWU=