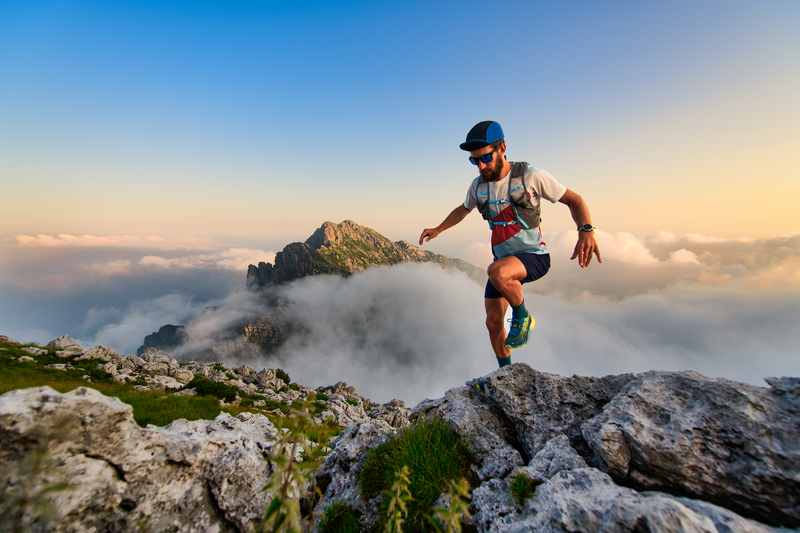
95% of researchers rate our articles as excellent or good
Learn more about the work of our research integrity team to safeguard the quality of each article we publish.
Find out more
ORIGINAL RESEARCH article
Front. Plant Sci. , 20 May 2021
Sec. Plant Abiotic Stress
Volume 12 - 2021 | https://doi.org/10.3389/fpls.2021.656961
This article is part of the Research Topic Microbial Mediation of Crop Abiotic Stress Tolerance View all 10 articles
Soybean (Glycine max L.) future response to elevated [CO2] has been shown to differ when inoculated with B. japonicum strains isolated at ambient or elevated [CO2]. Plants, inoculated with three Bradyrhizobium strains isolated at different [CO2], were grown in chambers at current and elevated [CO2] (400 vs. 700 ppm). Together with nodule and leaf metabolomic profile, characterization of nodule N-fixation and exchange between organs were tested through 15N2-labeling analysis. Soybeans inoculated with SFJ14-36 strain (isolated at elevated [CO2]) showed a strong metabolic imbalance, at nodule and leaf levels when grown at ambient [CO2], probably due to an insufficient supply of N by nodules, as shown by 15N2-labeling. In nodules, due to shortage of photoassimilate, C may be diverted to aspartic acid instead of malate in order to improve the efficiency of the C source sustaining N2-fixation. In leaves, photorespiration and respiration were boosted at ambient [CO2] in plants inoculated with this strain. Additionally, free phytol, antioxidants, and fatty acid content could be indicate induced senescence due to oxidative stress and lack of nitrogen. Therefore, plants inoculated with Bradyrhizobium strain isolated at elevated [CO2] may have lost their capacity to form effective symbiosis at ambient [CO2] and that was translated at whole plant level through metabolic impairment.
Atmospheric carbon dioxide concentration ([CO2]) has increased strongly since preindustrial times (∼280 ppm) to 412.8 ppm registered in November 2020 (1 CO2.earth, 2020), and a further substantial increase is expected during this century. Carbon dioxide is the major greenhouse gas of anthropogenic activity origin and has been demonstrated to participate in climate change whereby global temperature and precipitation patterns will be altered (IPCC, 2013). At the plant level, increasing [CO2] leads to increased photosynthesis while reducing photorespiration and, as a consequence, increasing growth and seed yield (Ainsworth et al., 2002).
Soybean (Glycine max L.) is the fourth most important food crop and the most cultivated legume, with 349 million tons produced in 2018 (FAOSTAT, 2020) being the most traded agricultural commodity, accounting for over 10% of the total value of the global exchange. This legume is a rich source of high-quality proteins and oil and contains a considerable amount of carbohydrates, amino acids, and minerals that contribute to its nutritional value (Medic et al., 2014). Soybean physiological responses to elevated [CO2] (e[CO2]) have been extensively studied in both controlled and in field environments (Ainsworth et al., 2002; Bishop et al., 2015). Increased photosynthesis due to CO2 fertilization leads to increases in leaf carbohydrate content (Ainsworth et al., 2004; Rogers et al., 2006), radiation use efficiency (RUE) (Sanz-Sáez et al., 2017), biomass production, and seed yield (Morgan et al., 2005), while decreasing stomatal conductance (Ainsworth and Rogers, 2007; Soba et al., 2020b) and leaf respiration (Tcherkez et al., 2008).
In some crops, sink limitation and photosynthesis downregulation is sometimes observed in plants grown at e[CO2] as a consequence of sugar overaccumulation in the leaves (Ainsworth and Rogers, 2007; Gutiérrez et al., 2009). However, soybeans, as the rest of legume crops, forms symbiotic relationships with Rhizobiaceae family bacteria, specifically with Bradyrhizobium japonicum, which provide access to atmospheric N2, through biological nitrogen fixation (BNF). According to Kaschuk et al. (2009), Bradyrhizobium bacteria can consume between 4 and 11% of carbohydrates fixed through photosynthesis and, therefore, increase plant sink capacity and stimulate legume growth under e[CO2] avoiding C sink limitation (Ainsworth et al., 2004). On the other hand, BNF and carbohydrate consumption by the nodule is influenced in part by the strain of Bradyrhizobium (Kaschuk et al., 2009; Sanz-Sáez et al., 2015). Besides, the microbial population structure in the rizhosphere has been shown to be altered by e[CO2] (Wang et al., 2017). Therefore, there is great interest in the isolation and selection for Bradyrhizobium strains adapted to future environments, such as e[CO2], which hypothetically could be more N2-fixation efficient ones compared with unselected or native strains. This hypothesis is reinforced by some studies in alfalfa (Bertrand et al., 2007; Sanz-Sáez et al., 2012) and soybean (Bertrand et al., 2007, 2011; Prévost et al., 2010; Sanz-Sáez et al., 2015) which showed that selected strains could improve legume productivity in response to e[CO2] by fixing more N2 and hence consuming more C.
Sugawara and Sadowsky (2013) studied different native B. japonicum strains from soybean nodules of plants grown and isolated at ambient [CO2] (a[CO2]) (390 ppm, strain SFJ4-24) and e[CO2] (550 ppm, strain SFJ14-36), under fully open-field conditions at a FACE site at the University of Illinois at Urbana-Champaign. They observed that the strain SFJ14-36, isolated at e[CO2], significantly overexpressed genes encoding for N2-fixation and nodulation in comparison with the strain isolated at a[CO2] and the control strain (USDA 110). More recently, Sanz-Sáez et al. (2019) tested if the same strain isolated under e[CO2] conditions (SFJ14-36) showed higher BNF and nodule number at e[CO2] compared with plants inoculated with USDA110 as it was suggested by the expression profiles published by Sugawara and Sadowsky (2013); however, no statistical differences were observed between plants inoculated with different strains when grown at e[CO2]. Therefore, the overexpression of genes observed by Sugawara and Sadowsky (2013) were not matched by a higher BNF. In addition, when the plants were grown at a[CO2], those inoculated with the strain isolated at e[CO2] showed lower plant fitness in comparison with USDA110. The authors hypothesized that the strains isolated at e[CO2] may be attracted only by root exudates produced at e[CO2] or that at a[CO2] there is some metabolic restrictions in the nodules that reduce the fitness of the symbiotic relationship.
With the objective of better understanding the C and N metabolism interaction in the plants inoculated with different B. japonicum strains and grown under e[CO2] conditions, that favor yield under climate change conditions, it is needed to study why some rhizobium strains respond better to e[CO2] than others, and how this affects plant fitness.
Together and closely related with these physiological responses, plant metabolome is also perturbed under e[CO2] (Aranjuelo et al., 2015; Tcherkez et al., 2020). The metabolome of the plant is the link between genotype and phenotype, allowing to study changes in gene expression in response to the environment (Saito and Matsuda, 2008). This makes metabolomic profiling an attractive tool for phenotyping, providing a comprehensive perspective of the environmental changes that influence plants (Obata and Fernie, 2012). In opposition to a traditional metabolic analysis, in which the researcher focuses on a specific class of metabolites related with a metabolic route, the simultaneous profiling of metabolites from biosynthetically unrelated pathways has been demonstrated to increase our understanding of the molecular mechanism that underlies plant response to different stresses (Li et al., 2015). While previous studies have explored the Rhizobium-legume specificity and their physiological response to e[CO2] (Das et al., 2017; Rabara et al., 2017), to our knowledge, no previous works have analyzed the metabolic profile of soybean nodules and leaves inoculated with different B. japonicum strains grown under e[CO2] conditions.
The aim of this work was to elucidate the metabolic features involved in Bradyrhizobium-soybean specificity under contrasting CO2 conditions. With this purpose, metabolic profiling analysis were carried out under two contrasting levels of CO2 using the same three B. japonicum strains isolated at different [CO2] (Sugawara and Sadowsky, 2013) and whose physiologic and photosynthetic parameters were studied by Sanz-Sáez et al. (2019).
For this study, the same B. japonicum strains isolated by Sugawara and Sadowsky (2013) were used: SFJ4-24 (serogroup 123) was a strain isolated from nodules of soybean grown at a[CO2] (390 ppm of CO2), meanwhile SFJ14-36 (serogroup 38) was a strain isolated from soybean nodules grown at e[CO2] (550 ppm of CO2). As a control, we used USDA110 strain because it has demonstrated high soybean performance at ambient and elevated [CO2] at SoyFACE facility at the University of Illinois at Urbana-Champaign2 (Sanz-Sáez et al., 2015). Soybean cultivar (Glycine max cv. 93B15; Pioneer Hi-Bred) was used in the same way as being used by Sugawara and Sadowsky (2013) in order to avoid problems of compatibility between the soybean cultivar and the Bradyrhizobium japonicum strains. These strains were provided by Prof. Michael Sadowsky (University of Minnesota; SFJ4-24, and SFJ14-36) and by USDA-ARS Rhizobium Germplasm Resource Collection in Belstville, MD (USDA110). The different B. japonicum cultures were made exactly as explained in Sanz-Sáez et al. (2019).
Soybean seeds were surface sterilized with sodium hypochlorite (1%) for 10 min and rinsed with sterile water until the smell of bleach disappeared. For the seed inoculation, 200 ml of medium liquid culture containing ≈5 × 109 cell ml–1 of each individual B. japonicum strain was centrifuged for 15 min at 5,100 × g to separate the bacteria from the media. The pellet containing the bacteria was resuspended in 2 ml of sterile deionized water containing 2% polivinylpolypyrrolidone (PVPP) reaching a concentration of ≈1011 cel ml–1. Then 200 seeds were placed in a 500-ml sterile beaker containing 2 ml of concentrated inoculum on a rotatory shaker overnight, and later immediately planted. For the liquid inoculation, 1 L of liquid culture containing ≈5 × 109 cell ml–1 was centrifuged and resuspended as above to a final concentration of 108 cell ml–1.
Five inoculated soybean seeds were planted in 10 L pots containing a mixture of peat moss, perlite, and vermiculite of 1:1:1 v/v/v that was previously sterilized as described in Sanz-Sáez et al. (2015). One week after emergence, plants were thinned to one plant. After plants emerged from the pot, they were inoculated three times coinciding with 2, 9, and 16 days after emergence (DAE) with the liquid inoculum from each B. japonicum strain (USDA110, SFJ4-24, and SFJ14-36). All plants were watered alternatively with Evans N-free solution and distilled water to avoid salt accumulation (Moore, 1974). Soybean plants were grown in two growth chambers (Phytotron Service, SGIker) at the University of the Basque Country (UPV/EHU), from the beginning of the experiment, one maintained at a[CO2] (≈400 ppm CO2), and other maintained at e[CO2] (700 ppm CO2). Both chambers were maintained at 60/70% day/night relative humidity and 25/22°C day/night temperature, and a photosynthetic photon flux density (PPFD) of 1,200 μmol m–2 s–1 from 7:00 to 22:00 h, until developmental stage V5 (Fehr et al., 1971), when the day length was decreased by 2 h to induce flowering. Every 2 weeks, plants and CO2 treatments were rotated among and within chambers in order to reduce potential chamber effects. Harvest was carried out when plants reached full flowering developmental stage (R2). This stage was selected because flowering is the period when N2 fixation is supposed to be in its peak, and nodules have not started to senesce (Rogers et al., 2006). At this moment, six plants per inoculation and CO2 treatment were harvested and separated into organ samples; leaves, stems, roots, and nodules. Each organ sample was oven dried at 65°C for at least 72 h and then weighed. The data is presented as weight of each organ separately (g of dry weight plant–1), and stacked by total dry weight. For metabolic analysis, samples of leaves and nodules from three plants were collected and immediately frozen in liquid nitrogen and stored at −80°C until analysis.
Twenty-four hours before harvest, the underground zone was enriched with 15N2, and after harvest, the labeled 15N incorporation [measured as stable 15N isotope composition (δ15N)] in nodule tissue was measured. This parameter has been recently used as a measure of nodule performance in soybean and other legumes (Soba et al., 2020a) showing that the higher the nodule δ15N the greater the BNF. Three plants (there was one plant per pot) per inoculation and CO2 treatment were labeled, while three plants were used as unlabeled controls and harvested at the same time as the labeled plants. The 15N2 labeling was accomplished by injecting labeled gas into the root zone using handmade labeling pots following the procedure of Sanz-Sáez et al. (2015). Plants were grown in these pots for the duration of the experiment. On the night preceding the labeling experiment, the pots were sealed with plastic lids in order to avoid the escape of the labeled gas. To perform the 15N2 labeling, 10% 15N2-enriched gas was prepared in Supelco-Inert Foil Gas Sampling Bags (Sigma-Aldrich, St Louis, MO, United States) by mixing the 15N2-labeled gas enriched at 99% with ambient air (δ15N2 at 0‰). Two hundred milliliters of 15N2 (10%) mixed gas was injected into the labeling pots using a gas syringe (SGE; Sigma-Aldrich) 2 and 4 h after the lights were turned on, coinciding with the period of greatest N2-fixing activity (Molero et al., 2019). After 24 h, the plants were harvested and separated into nodules, roots, and leaves, then dried at 65°C for at least 72 h. The dried organs were weighed and ground to 1 mm particle size. The samples were analyzed in a Costech 4010 elemental analyzer coupled in continuous flow with a Thermo Fisher Delta V Advantage Isotope Ratio Mass Spectrometer (IRMS Thermo Scientific, Waltham, MA, United States). The 15N/14N ratio in soybean nodule, root, and leaf material was expressed in δ notation (δ15N) following the equation as described in Sanz-Sáez et al. (2019). The amount of 15N fixed during the day of the labeling experiment was calculated as 15N fixed in each organ following the equation, as described in Bei et al. (2013).
Photorespiratory rates were estimated as the rate of ribulose-1,5-bisphosphate (RuBP) oxygenation (vo) derived from the measured rates of CO2 uptake by the leaves according to Sharkey (1988) and von Caemmerer (2000), as described in Noctor et al. (2002):
where A is the measured rate of net CO2 uptake, Rd is non-photorespiratory CO2 release in the light (taken as 50% of the rate measured in the dark in each experiment) and O:C is the ratio of RuBP oxygenation to carboxylation. The ratio O:C was calculated as:
where Srel is the specificity factor of Rubisco (taken as 110; Keys, 1999), and Cc and Oc are the chloroplastic concentrations of CO2 and O2, respectively. Oc was assumed to be that of water in equilibrium with air at 20°C (276 μM) and Cc was derived from Ci by taking a CO2 transfer conductance through the mesophyll (gi) of 0⋅32 mol m–2 s–1 (Gillon and Yakir, 2000) and assuming that the rate of CO2 uptake affects Cc relative to Ci as in Ruuska et al. (2000):
where Cc was converted to a molar concentration by applying a CO2 solubility constant at 20°C of 0.0392 mol L–1 (von Caemmerer, 2000). The gas exchange parameters A, R in the dark, and Cc were measured exactly as described in Sanz-Sáez et al. (2019).
Leaf and nodule samples (20 mg of powder from freeze-dried material) were ground in a mortar in liquid nitrogen, and then in 2 ml of 80% methanol, in which ribitol (100 μmol L–1) was added as an internal standard. After centrifugation at 15,000 rpm for 15 min at 4°C, the supernatant was collected and centrifuged again. Then, the supernatants were spin-dried under vacuum and stored at −80°C until analysis. Relative metabolite content was determined by gas chromatography coupled to time-of-flight mass spectrometry (GC-MS) using a LECO Pegasus III coupled to an Agilent 6890N GC system. Sample derivatization and GC-MS analyses were carried out as described in Aranjuelo et al. (2015). Peak identity was established by comparison of the fragmentation pattern with MS-available databases (NIST). The integration of peaks was performed using the LECO Pegasus software and the automated peak integration was verified manually for each compound in all analyses. The quantification was normalized to dry weight (DW) to avoid any discrepancy due to changes in relative water content.
Statistical analyses were performed with IBM SPSS Statistics for Windows, Version 20.0 (IBM Co., Armonk, NY, United States). Differences among the three Bradyrhizobium strains and the two CO2 levels were evaluated by two-way analyses of variance (ANOVA), with the strain and CO2 as fixed factors. All data were tested for normality (Kolmogorov–Smirnov test) and homogeneity of variances (Levene’s test). For ANOVA analysis, the results were considered to be significant when p < 0.05. In order to reduce the multivariate data complexity and identify patterns between samples, principal component analysis (PCA) was performed for nodules and leaves and the 121 metabolites were taken into account. Heat map and PCA for the two organs were conducted using XLSTAT 2008 (Addinsoft, Paris, France) software. Heat maps were done independently for leaves and nodules with metabolites showing significant differences between B. japonicum strain, [CO2], and/or interactions in each tissue. Clustering was based on the Pearson’s correlation coefficients among the metabolites. In this manuscript, the red color is proportional to a lower concentration; conversely, the intensity of the green color is proportional to higher concentration values.
At the R2 stage, strain, CO2 level, and their interaction were found to have a significant effect on biomass of leaves, stems, and roots (data not shown). In contrast, nodule weight was only significantly affected by CO2 level. Elevated [CO2] significantly increased total biomass in all studied organs for the plants inoculated with B. japonicum strain isolated at e[CO2] (SFJ14-36) but not for the reference strain (USDA110) or in the strain isolated at a[CO2] (SFJ4-24) (Figure 1). However, the final value at e[CO2] of SFJ14-36 strain was similar to the SFJ4-24 strain. Plants inoculated with USDA110, the reference strain, showed the highest values at both CO2 concentrations (Figure 1).
Figure 1. Effect of [CO2] on soybean biomass in plants inoculated with three different B. japonicum strains. Nodule, root, stem, and leaf biomass (g DW plant– 1) of soybean plant grown at a[CO2] (400 ppm) and e[CO2] (700 ppm) and inoculated with three Bradyrhizobium japonicum strains (USDA110, SFJ4-24, and SFJ14-36). Bars correspond to the mean ± SE of n = 6 of the biomass of each tissue. Results of statistics for total biomass (the sum of nodule, root, leaf, and stem) are shown (two-way ANOVA, P < 0.05). Different letters indicate significant differences (Tukey post hoc test P < 0.05).
For nodule 15N isotope labeling (δ15N), greatest value was found in nodules of plants inoculated with USDA110 strain grown at a[CO2] which was significantly higher than plants grown at e[CO2] (Figure 2). Interestingly, nodules of SFJ4-24 and SFJ14-36 did not show significant differences between CO2 levels, and the δ15N was significantly greater in nodules of SFJ14-36 when compared with SFJ4-24 (Figure 2). For a more holistic vision, we calculated the amount of δ15N per organ (mg 15N organ–1) (Figure 3). The results showed a clear significant effect of CO2 for strain SFJ14-36 in the three studied tissues (leaf, root, and nodule) in contrast with USDA110 and SFJ4-24 strains.
Figure 2. Effect of [CO2] on soybean nodule δ15N in plants inoculated with three different B. japonicum strains. Nodule 15N isotope labeling (δ15N, ‰) ± of soybean plants grown at a[CO2] (400 ppm) and e[CO2] (700 ppm) and inoculated with three Bradyrhizobium japonicum strains (USDA110, SFJ4-24, and SFJ14-36). Bars correspond to the mean ± SE of n = 3. Results of statistics are shown (two-way ANOVA, P < 0.05). Different letters indicate significant differences (Tukey post hoc test P < 0.05).
Figure 3. Effect of [CO2] on biomass labeled 15N in three organs of soybean plants inoculated with three different B. japonicum strains. Biomass labeled 15N (mg 15N organ– 1) in (A) nodules, (B) roots, and (C) leaves of soybean grown at a[CO2] (400 ppm) and e[CO2] (700 ppm) and inoculated with three Bradyrhizobium japonicum strains (USDA110, SFJ4-24, and SFJ14-36). Bars correspond to the mean ± SE of n = 3. Results of statistics are shown (two-way ANOVA, P < 0.05). Different letters indicate significant differences (Tukey post hoc test P < 0.05).
The estimated rate of photorespiration (vo) from gas exchange measures showed significant differences between CO2 levels. Whereas both SFJ4-24 and SFJ14-36 showed a significant decrease of photorespiration under e[CO2], USDA 110 did not (Figure 4).
Figure 4. Photorespiratory estimations from gas exchange measures. Estimated rate of RuBP Oxygenation (vo) in soybean leaves grown at a[CO2] (400 ppm) and e[CO2] (700 ppm) and inoculated with three Bradyrhizobium japonicum strains (USDA110, SFJ4-24, and SFJ14-36). Bars correspond to the mean ± SE of n = 6. Results of statistics are shown (two-way ANOVA, P < 0.05). Different letters indicate significant differences (Tukey post hoc test P < 0.05).
Metabolite profiling was performed by gas chromatography coupled with time-of-flight mass spectrometry (GC-MS), and 121 different metabolites were identified by reference to their MS data. These metabolites were classified in eight chemical groups (organic acids, amino acids, sugars, fatty acids, polyols, nucleotides, secondary metabolites, and others), and their relative abundance is shown in Figure S1. The statistical analysis (PCA and heat map hierarchical clustering) of leaf and nodule metabolomic profile indicates that a clear differentiation between organs could be done (Figures 5, 6). While in the nodule metabolome, B. japonicum strain was found to have the main effect, with little impact of CO2 level (except for SFJ14-36) (Figure 5A), in leaf metabolome, CO2 level had the main effect (Figure 5C). Nodule PCA analysis revealed that the two principal components explained 73.5% of total variation between strains and CO2 levels (Figure 5A). The six combinations of strains and CO2 levels were grouped by strains without differences between treatments except for the SFJ14-36, which showed significant differences between CO2 levels. The loading plot revealed that the discrimination of samples by PC1 (48.2% of the total variance) was, in part due to sugars such as maltose and trehalose, whereas amino acids like lysine, methionine, threonine, leucine, glycine, and glutamine contributed to the separation of samples by PC2 (25.3% of the total variance). It is interesting to note that Krebs cycle related metabolites are grouped in the lower-left quadrant of the loading plot (Figure 5B). On the other hand, leaf PCA analysis showed a more unrelated distribution of strains and treatments, but a clear separation between Bradyrhizobium strains grown at ambient and elevated [CO2] could be traced (Figure 5C). The two principal components explained 60.6% of total variation between strains and CO2 levels. No distinctive pattern in metabolites were detected to explain this variation; nevertheless, important metabolites such as D-glucose-6-phosphate, glycine, serine, the polyamines putrescine and spermidine, and shikimic acid were important contributors to the separation of samples by PC2 (Figure 5D).
Figure 5. Graphical representation of metabolomic response to different [CO2] in soybean plants inoculated with three B. japonicum strains. Principal component analysis (PCA) [(A) nodules and (C) leaves] of the different metabolites in soybean plants inoculated with three B. japonicum strains. Scatter plot distribution [(B) nodules and (D) leaves] of the different metabolites in soybean plants inoculated with three B. japonicum strains. Three replicates were examined per strain and CO2 level.
Figure 6. Differentially expressed metabolites involved in [CO2] responses in function of B. japonicum strain. (A) Hierarchically clustered heat maps of metabolites that were found to be significantly different between Bradyrhizobium strains, [CO2], and their interaction in nodules. (B) Hierarchically clustered heat maps of metabolites that were found to be significantly different between Bradyrhizobium strains, [CO2], and their interaction in leaves. Each column represents the average of three replicates. Intensity of red and green indicates increases and decreases relative to the mean respectively, as shown in the color scale.
Of the 121 analyzed metabolites, 64 were significantly affected by CO2, strain or their interaction in nodules and 54 in leaves (Figure 6). In order to provide a better understanding of this variation, metabolite profiling representation (heat map) and hierarchical clustering analysis was undertaken separately for each organ (Figure 6). In nodules, the hierarchical clustering of the six combinations (two levels of CO2 and three Bradyrhizobium strains) formed three clusters, one for each strain with little differences between CO2 treatments, except for the strain SFJ14-36 where differences between CO2 levels were bigger (Figure 6A), as we have seen in the PCA analysis (Figure 5A). On the other hand, the hierarchical clustering of the 64 significant metabolites allowed the grouping of metabolites into two major clusters. Cluster 1 was mostly made of metabolites in higher concentration in plants inoculated with USDA110 strain as compared with other strains and could be subdivided in two subclusters; 1A: sugars (glycolysis pathway: sucrose, glucose, fructose, mannose, etc.) and 1B: N-related compounds such as ureides and urea cycle metabolites (allantoin, uric acid, ornithine, aspartic acid, citrulline, urea, etc.). On the contrary, cluster 2 was formed of metabolites in lower concentration in USDA110 strain and mostly included Krebs cycle metabolites (fumarate, malate, citrate, isocitrate, α-ketoglutarate, etc.) (Figure 6A).
Contrary to nodules, in leaves, the hierarchical clustering of CO2 levels and Bradyrhizobium strain combinations was grouped by CO2 level in two clusters (Figure 6B). Cluster 1 formed by plants grown at e[CO2] and cluster 2 by plants grown at a[CO2]. In general, the metabolites were accumulated in greater quantity at a[CO2]. The hierarchical clustering of the 54 significant metabolites showed also two different clusters. Cluster 1 was formed by two subclusters mainly composed by metabolites related with photorespiration (glycolate, glycine, serine, glycerate) and Krebs cycle (glutamate, citrate, succinate, etc.) and sugar metabolites (fructose, glucose, tagatose, talose, sorbitol, etc.). On the other hand, cluster 2 showed a greater accumulation of metabolites in plants inoculated with SFJ14-36 and lower in SFJ4-24 strain at a[CO2]. This cluster was mainly composed of lipid-related metabolites (free fatty acids and sterols). Figures 7, 8 represent metabolites that were significantly affected by B. japonicum strain, [CO2], or their interaction either on soybean nodule and leaf.
Figure 7. Effects of Bradyrhizobium japonicum strain and [CO2] on soybean nodule metabolism. Bar charts showing the relative abundance of metabolites in ambient [CO2] (white) and elevated [CO2] (black) in soybean plants inoculated with three different Bradyrhizobium japonicum strains. Red, yellow, and blue lightning signs indicate the significant effect of strain, CO2, and their interaction, respectively. Metabolites in bold indicate metabolites that were found to have significant effect of strain, CO2, and/or their interaction; italic metabolites indicate metabolites analyzed that were not found to have significant effect of strain, CO2, and their interaction, and not bold neither italic indicates metabolites not analyzed.
Figure 8. Effects of Bradyrhizobium japonicum strain and [CO2] on soybean leaf metabolism. Bar charts showing the relative abundance of metabolites in ambient [CO2] (white) and elevated [CO2] (black) in soybean plants inoculated with three different Bradyrhizobium japonicum strains. Red, yellow, and blue lightning signs indicate the significant effect of strain, CO2, and their interaction, respectively. Bold metabolites indicate metabolites analyzed that were found to have significant effect of strain, CO2 and/or their interaction; italic metabolites indicate metabolites analyzed that were not found to have significant effect of strain, CO2, and their interaction, and not bold neither italic indicates metabolites not analyzed.
Our study showed that [CO2] effect on soybean biomass was tightly dependent on the B. japonicum strain analyzed. While in SFJ4-24 and USDA110 changes between CO2 levels were not significant, soybeans inoculated with SFJ14-36 strain showed an increase of 322% in their total biomass under e[CO2] (Figure 1). Both, N2 fixation and photosynthetic performance were involved in such different responses. When we analyze δ15N in nodules of SFJ14-36, used as a measure of BNF-specific rate, no significant differences were found. However, significant differences, in nodule, root, and leaf, were found when the amount of total biomass labeled 15N2 in this strain was calculated relating with the total N fixed by BNF and their translocation to other tissues (Figures 2, 3). The greater 15N content under e[CO2] in SFJ14-36 compared with a[CO2] was in contrast with the lack of significant differences between CO2 level for the other two strains in roots and leaves. This difference may be due to a better translocation of fixed N to the aboveground tissues as shown by nodule metabolite data (greater accumulation of aspartic acid (Asp) and allantoin in nodules of plants inoculated with SFJ14-36 at a[CO2]) (Figure 7). Briefly, in the plants inoculated with the strain isolated at e[CO2] (SFJ14-36), even with a similar BNF-specific rate at both CO2 levels, the greater nodule biomass at e[CO2] leads to a greater amount of fixed N and this together with a better translocation to the aerial parts, allows a better biomass growth under e[CO2]. However, nodulation in soybean that were inoculated with SFJ14-36 strain seems to be restricted or delayed when grown at a[CO2] as previously showed by Sanz-Sáez et al. (2019) compromising the whole plant fitness due to a lower N availability. None of these facts seems to occur in the model strain (USDA110) or in the one isolated at a[CO2] (SFJ4-24).
In addition to BNF, gas exchange measures also contributed to explain the different responses of plants inoculated with different B. japonicum strains to e[CO2]. As previously showed by Sanz-Sáez et al. (2019), photosynthesis was reduced when plants grew at a[CO2], but this reduction was significantly greater in SFJ14-36 strain when compared with the other two. One of the most important parameters affecting C fixation through photosynthesis is photorespiration. Zhu et al. (2008) estimated a reduction of gross C3 photosynthesis efficiency by 48% at current [CO2] and temperature conditions, mainly associated with the consumption of fixed C and energy in the glycolate recycling process. As observed in Figure 4, both SFJ4-24 and SFJ14-36 reduced the estimated vo (32.5 and 40.7%, respectively) when grown at e[CO2]; in contrast, values in USDA110 did not change. Therefore, in SFJ4-24 and SFJ14-36, the increase in the photosynthetic rate previously observed under e[CO2] could be in part due to a reduction in the photorespiratory rate, especially in SFJ14-36 as validated below with the metabolic data that shows a decrease in intermediates of the glycolate cycle (glycolic acid, glycine, and serine among others) (Figure 6) in leaves grown under e[CO2].
The decreased nodulation and reduction of the symbiotic fitness of the plants inoculated with SFJ-14-36 and grown at a[CO2] could be due to changes in the quantity and/or quality of phenolic substances excreted by the roots (Sugawara and Sadowsky, 2013; Wang et al., 2017). At e[CO2], roots excrete more and different phenolic compounds that attract rhizobium species (Sugawara and Sadowsky, 2013). As SFJ-14-36 was isolated under e[CO2], an effective nodulation may have been dependent of phenolic substances only emitted under these circumstances whereas at a[CO2], these compounds may have changed (Sugawara and Sadowsky, 2013), reducing the nodule formation and the amount of N that was fixed and fed to the plant. Another reason for the lower nodulation of plants inoculated with SFJ14-36 strain at ambient [CO2] could be the lack of synergy between Bradyrhizobium strain and soybean cultivar. This is not likely because this study used the same soybean cultivar (cv. 93B15; Pioneer Hi-Bred) used in Sugawara and Sadowsky (2013) when the strain was isolated in e[CO2] at the SoyFACE facility in Illinois.
Together with physiologic parameters, plant metabolomic can give us valuable information about the status of the soybean-Bradyrhizobia symbiosis under current and future [CO2]. As shown, [CO2] effect on physiology was dependent on the B. japonicum strain and these differential responses are expected to be reflected in the accumulation of specific metabolites. Therefore, metabolomics are a valuable tool to decode the physiologic differences between plants inoculated with different strains and CO2 levels, helping us understand why the plants symbiosis with the strain that was isolated at e[CO2] does not perform well at a[CO2] or why USDA110 is more effective than the other two strains at a[CO2].
Nitrogen fixation in legume nodules is fueled by C fixed through photosynthesis. The current experiment showed that the amount of sucrose in nodules was affected by CO2 level and by Bradyrhizobium strain. Plants inoculated with USDA110 showed the highest sucrose levels (especially at e[CO2]); on the contrary, SFJ4-24 showed almost no sucrose in both ambient and elevated [CO2] when compared with USDA110 (Figure 7). This strain-specific sucrose content was in opposition with the lack of statistically photosynthetic differences observed by Sanz-Sáez et al. (2019) between USDA110 and SFJ4-24. Such results point to the fact that other reasons may exist behind this contrasting sucrose content between plants inoculated with the two strains. More specifically, obtained data would reveal that a greater use of sucrose in the leaf as observed in metabolites implied in glycolysis (glucose, fructose) in leaves of SFJ4-24 at a[CO2] (Figure 8) which is in accordance of the higher respiration rates observed in leaves of plants inoculated with this strain (Sanz-Sáez et al., 2019) which could limit its export to nodules. On the other hand, nodules of plants inoculated with SFJ14-36 showed greater amount of sucrose under e[CO2] than under a[CO2] probably due to a better photosynthetic rate at e[CO2]. Also, metabolites involved in glycolysis (fructose, glucose, and glucose-6-phosphate) were significant affected by both, Bradyrhizobium strain and CO2 level (Figure 7). These observations suggest a poor supply and/or rapid consumption of C for respiration and C skeletons in nodules of plants inoculated with SFJ4-24 and SFJ14-36 under a[CO2] (Aranjuelo et al., 2014). Surprisingly, when we observed the content of malate, the main dicarboxylic acid formed after glycolysis (Lodwig and Poole, 2003), and other organic acids involved in Krebs cycle, we saw that they were affected by Bradyrhizobium strain but not by CO2 level content. On the other hand, malate content in SFJ4-24 nodules was similar to USDA110 and higher than in SFJ14-36. These two observations could suggest that in nodules of plants inoculated with SFJ4-24, much of the carbon glycolyzed was derived to maintain nodule energy supply through malate production. Meanwhile in USDA110, although malate content is similar to that observed in SFJ4-24, the preceding substrates (sucrose, fructose, and G-6-P) were at much higher concentrations indicating that part of this C was diverted to the production of other compounds, such as phenolic compounds that were in higher concentration than in the other strains (Figure 6), suggesting an active carbon metabolism in soybeans inoculated with USDA110. This depletion in Krebs intermediates could be replenished trough the GABA-shunt allowing the synthesis of succinate that can enter the Krebs cycle (Saiz-Fernández et al., 2020) maintaining high its activity and the levels of malate observed with this strain. This role of the GABA-shunt would be confirmed by the increase of the levels of GABA and polyamines (spermidine and spermine) observed in USDA110 under e[CO2] (Figure 6) as it was proposed by Saiz-Fernández et al. (2020) in corn plants. The low malate content in plants inoculated with SFJ14-36 was also showing this C diversion in plants infected by this strain. A significant portion of carbon entering in the Krebs cycle in bacteroids is diverted, via anaplerotic reactions, into some amino acids: alanine (Ala), through pyruvate (Igamberdiev and Kleczkowski, 2018); aspartic acid (Asp), through oxaloacetate (OAA) (Melzer and O’Leary, 1991); and glutamic acid (Glu), through α-ketoglutarate (α-KG) (Salminen and Streeter, 1987, 1992; Figure 7).
Aspartic acid and Glu were by far the two most abundant amino acids in nodules; whereas by contrast, the content of Ala was low (Figure 7). Aspartic acid is a common amino acid and, in nodulated soybean, has been shown to be a form of N-transport, especially under N-stress conditions (Lima and Sodek, 2003). In our work, the highest content of Asp was found in nodules of plants inoculated with SFJ14-36 at a[CO2], in special when compared with SFJ4-24 strain. However, if a great part of OAA is transaminated to Asp, the Krebs cycle will be shut down because malate and citrate cannot be synthesized (Hayes, 2001), explaining the low content of Krebs cycle metabolites observed in SFJ14-36 strain. Therefore, in nodules, the observed differences in Bradyrhizobium strains between glycolysis metabolites and organic acids involved in Krebs cycle may be due to a diversion of C to Asp production via anaplerotic reaction; and as a result, lesser organic acids were involved in energy production for N2 fixation in plants inoculated with SFJ14-36 strain. In the case of USDA 110, the diversion of C from Krebs cycle to phenolic compounds could be replenished through the GABA-shunt.
Although soybean is a ureides exporter, previous studies have revealed that plants with impaired N2 fixation have shown an enhancement of Asp transport through xylem sap, as a precursor of the products of NH4+ assimilation (Puiatti and Sodek, 1999; Lima and Sodek, 2003). Additionally, when photoassimilates transport to nodules is restricted, as it seems to be the case in plants inoculated with SFJ14-36 strain grown at a[CO2], plants may use C, N, and energy in a more efficient way, through the carboxylation of Phosphoenolpyruvate (PEP) into OAA, required for Asp synthesis, instead entering Krebs cycle through malate (Fischinger and Schulze, 2010). This mechanism has been shown mainly in indeterminate nodules (pea); however, Silvente et al. (2003) showed that in ureides exporter nodules (bean), aspartate aminotransferase (AAT) may be acting as an important switching enzyme in driving the metabolic flow of fixed N through amide or ureides synthesis, helping to explain the significant higher concentration of Asp and Krebs cycle metabolites in nodules of plants inoculated with SFJ14-36 strain at a[CO2] (Figure 7). The use of Asp for N assimilation is connected with the carboxylation of PEP, while any use of C through Krebs cycle for N assimilation is connected with a preceding decarboxylation of pyruvate. This means that, while at first the nodule is fixing CO2, later is losing it. In this sense, nodule CO2 fixation may represent a C-saving mechanism particularly in occasions of limited C availability (Fischinger and Schulze, 2010; Fischinger et al., 2010), such as the ones infected with SFJ14-36 strain. This Asp produced may be exported through xylem or used for glutamic acid formation and consequently the production of glutamine by glutamine synthetase (GS) and ureides (Lima and Sodek, 2003). The high levels of uric acid and allantoin in nodules of plants inoculated with SFJ14-36 strain at a[CO2] could support the idea of Asp as an intermediate metabolite in N assimilation under limited C supply to the nodule. However, more studies are necessary to proof this N assimilation route in determinate nodules. These results are in accordance with the 15N labeled data (Figures 2, 3A) which showed a good SFJ14-36 nodule performance (measured as nodule δ15N) at both CO2 levels, similar to the one observed in the reference strain (USDA110) at e[CO2], however, due to a deficient nodulation in SFJ14-36 at a[CO2] the total amount of N2 fixed by nodules was very low (Figure 3A).
On the other hand, the accumulation of ureides, uric acid and allantoin (Serraj et al., 2001; Ladrera et al., 2007), and Asp (King and Purcell, 2005) has been proposed in soybean nodules to take part in the modulation of the symbiotic activity, acting as an N-feedback mechanism. In our study, allantoin content, similar to Asp, was strongly affected by CO2 level, especially in plants infected with SFJ14-36 strain, suggesting that the greater content of this N-transporting compound under a[CO2] could be related with a decline in shoot N demand (Serraj et al., 1999); however, N-feedback effect by these compounds had not been observed since nodule performance (measured as δ15N) was not reduced at a[CO2] (Figure 2). Additionally, the levels of organic acids implied in Krebs cycle (malate, fumarate, citrate, α-KG) were similar at those observed in plants with this strain grown at e[CO2], suggesting an active nodule N-fixation but a poor N transport to aerial tissues in SFJ14-36 grown at a[CO2]. All this data could imply that poor plant fitness observed in SFJ14-36 at a[CO2] was at the end caused by a N stress. N deficiency was due to a poor nodule implantation, in terms of biomass, to an insufficient C import from leaves (low levels of sucrose), and to a poor N transport of nodule-fixed N that affects N status at whole plant level reducing C fixation through photosynthesis and so total biomass. Nevertheless, these nodules consumed all the sucrose from the aerial part (Figure 7) and they try to fix N in a more efficient way through the carboxylation of PEP to produce Asp; however, more work is needed to confirm the last hypothesis.
Carbon dioxide and O2 are competitive substrates for ribulose-l,5-bisphosphate carboxylase/oxygenase (Rubisco), and their ratio at the site of catalysis affects the rates of ribulose-1,5-bisphosphate (RuBP) carboxylation and oxygenation (Farquhar et al., 1980; Rachmilevitch et al., 2004). For this reason, increasing atmospheric [CO2] is expected to promote photosynthesis (C reduction) over photorespiration (C oxidation) as showed in C3 plants (Lin and Wang, 2002; Woodward, 2002) and seen here with the RuBP oxygenation (vo) estimation (Figure 4A). Our results showed higher concentration of leaf metabolites involved in photorespiratory pathway (glycolate, Gly, Ser, and glycerate) in plants infected with SFJ4-24 and SFJ14-36 grown at a[CO2], although the difference between CO2 levels was greater in SFJ14-36. Additionally, all four metabolites showed a similar trend in the combined response to Bradyrhizobium strain and CO2 level as shown in the heat map where they were grouped in the same cluster (Figure 6B) indicating a clear relationship between them. These metabolic results were in accordance with the estimation of vo, where significant reductions in vo were found between CO2 levels for plants inoculated with SFJ4-24 and SFJ14-36 strains but not for USDA110 (Figure 4A), and previous works with soybeans (Booker et al., 1997; Rogers et al., 2006; Ainsworth and Rogers, 2007).
As in the case of photorespiration, some studies had shown that under a[CO2], respiration and therefore Krebs cycle was up-regulated when compared with plants grown at e[CO2] (Tcherkez et al., 2008; Soba et al., 2019a, b). Sanz-Sáez et al. (2019) showed that leaves of soybean inoculated with SFJ14-36 showed a significant increase of respiration at a[CO2] when compared with e[CO2]; meanwhile, plants inoculated with USDA110 and SFJ14-36 did not show respiratory differences between CO2 levels. Nevertheless, under a[CO2], respiration has been suggested to be inhibited by the high levels of mitochondrial NADH, from photorespiration, and ATP/ADP, from photosynthesis (Padan et al., 2005). On the contrary, as commented before, higher Glu quantities are demanded with increased photorespiration rates and, therefore, an increase in 2-oxoglutarate coming from Krebs cycle is expected. Consequently, a complex respiratory homeostasis between two opposing forces: mitochondrial energy requirements and photorespiratory Glu demand is observed in leaves. Our data showed a general increase of dicarboxylic acids involved in Krebs cycle (fumarate, succinate, citrate, and malate) under a[CO2] that was especially marked in the case of SFJ14-36 strain, which is in accordance with previous respiratory data showed by Sanz-Sáez et al. (2019) and with the enhanced Glu demand by photorespiration in SFJ14-36 under a[CO2]. Interestingly, α-KG was not significantly affected by CO2 treatment in SFJ14-36 and may be due to the fact that is involved in the synthesis of Glu and Gln and, therefore, diverted at a[CO2] to the production of these two amino acids.
In summary, the observed enhancement of photorespiration in plants inoculated with SFJ14-36 strain grown at a[CO2] alters the leaf respiratory homeostasis between the downregulation caused by more NADH and the upregulation by more Glu demand. Our data suggest that at a[CO2], plants inoculated with SFJ14-36 strain showed an upregulation of Krebs cycle to compensate the demand of C skeletons for Glu production. We hypothesize that the imbalance of energy between production (through photosynthesis) and consumption (photorespiration and respiration) observed in SJF14-36 at a[CO2] was compensated by a greater fatty acid synthesis as seen in the significant increase of all free fatty acids analyzed (Figures 6B, 8). This could also indirectly reflect a diversion of photoassimilates to the synthesis of organic acids produced by a lower sucrose export to the nodule especially at a[CO2].
In addition to leaf ontogenic senescence that occurs during the normal aging, prematurely induced senescence can occur when plants are subjected to abiotic/biotic stresses (Troncoso-Ponce et al., 2013). One of the first events that happen is chlorophyll degradation and, as a result, free phytol is produced which can be used as a biomarker of the rapid loss of chlorophyll associated with the degeneration of chloroplast under stress (Lim et al., 2007). In our case, free phytol content in plants inoculated with SFJ14-36 strain at a[CO2] was five times greater compared with e[CO2] and with the other inoculation treatments (Figure 8), suggesting an additional stress in this treatment, probably due to a poor supply of N by the nodules.
The resulting free phytol is highly toxic to proteins and membranes, so a large proportion of phytol is incorporated into α-tocopherol, fatty acid phytyl esters, and triacylglycerol (Peisker et al., 1989; Ischebeck et al., 2006; Figure 8). α-Tocopherol is the most important lipophilic antioxidant in leaves (Munné-Bosch, 2007), protecting membrane lipids against lipid peroxidation. In our work, α-tocopherol content observed in leaves of SFJ14-36 plants at a[CO2] was not significantly higher compared with e[CO2] despite the greater phytol content observed at a[CO2]. Two possible explanations could be: (1) a poor α-tocopherol synthesis from phytol, in opposition to previous works (Lippold et al., 2012; von Dorp et al., 2015; Mach, 2015) or (2) high tocopherol degradation that provides an excess in their synthesis. The last option could occur when the stress is too severe and consequently lipid peroxidation increases (Munné-Bosch, 2005) and is likely to happen in our case. In addition to α-tocopherol, salicylic acid (SA) increased by 6.3-fold at a[CO2] in comparison with e[CO2] in SFJ14-36 plants. Several investigations indicated that SA increases accumulation of phenolics (Kováčik et al., 2008) and enhances the oxidative stress tolerance (Li et al., 2014) through stimulation of enzymatic and non-enzymatic antioxidant mechanism pathways (El-Esawi et al., 2017). In accordance to this, phenolic acids such as caffeic acid, ferulic acid, and coumaric acid were also upregulated at a[CO2] in SFJ14-36 plants (Figure 6B). Phenolic acids have been reported to be antioxidants that are implied in the scavenging of free radicals (Ghasemzadeh and Ghasemzadeh, 2011). These results indicated that, the antioxidant system was activated due to a severe oxidative stress specifically in leaves of soybeans inoculated with SFJ14-36 strain at a[CO2] but not in other treatments.
In plants, increased production of Reactive Oxygen Species and the accumulation of lipid peroxidation products have been associated with oxidation of membrane lipids and membrane catabolism during environmental stresses or senescence (Barclay and McKersie, 1994; Berjak and Pammenter, 2008). The amount of all the free fatty acid analyzed (capric, lauric, palmitic, palmitoleic, oleic, elaidic, arachidic, and linoleic acid) showed a significant increase at a[CO2] when compared with e[CO2] in plants inoculated with SFJ14-36 strain. However, in the other two strains, the values remained unaltered between CO2 levels. During maturation, aging, and senescence, the catabolic enzymatic activities become activated, and free fatty acids have been found to be enhanced considerably (Mishra et al., 2006). Together with free fatty acids, sterols (β-sitosterol and stigmasterol) were enhanced at a[CO2] only in SFJ14-36 plants, remaining unchanged in the other treatments. Sterols have also been found to be enhanced in senescing leaves (Duperon et al., 1984; Bouvier-Navé et al., 2010; Li et al., 2016) where they appear to participate in recycling these fatty acids released from senescing cell membranes to form sterol esters for subsequent transport to other tissues (Holmer et al., 1973; Chen et al., 2007).
All these metabolomic data suggest that in leaves of soybeans inoculated with SFJ14-36 strain grown at a[CO2], chlorophyll degradation and, therefore, lower photosynthetic ability was likely to occur at the same time as thylakoid membrane degradations. This was previously proposed by Li et al. (2016) during leaf senescence, in our case, probably due to enhancement of oxidative stress and N deficiency. However, this happened only when the plants were grown at current CO2 conditions not at e[CO2], showing a soybean-strain specific interaction with atmospheric [CO2]. As N is essential for synthesis of Rubisco and chlorophylls, in soybean plants inoculated with SFJ14-36 strain, insufficient N-fixation at a[CO2] (Figure 3C) decreased leaf N concentration and, as a consequence, photosynthetic rates were decreased in this B. japonicum strains (Sanz-Sáez et al., 2019). In this respect, some studies have shown that a sufficient supply of N through BNF increases photosynthetic rates and delays leaf senescence in soybean (Abu-Shakra et al., 1978; Kaschuk et al., 2010). Therefore, observed induced leaf senescence in SFJ14-36 may be due to an insufficient supply of N from the BNF to the leaves as already observed in soybean (Egli et al., 1978). As stated before, the poor supply of N from nodules to leaves was not likely due to low nodule BNF efficiency (Figure 2) but probably to a deficient nodulation as observed by the significant reduction in nodule biomass in SFJ14-36 grown at a[CO2] when compared with e[CO2].
In this study, with the use of metabolomics and their interaction with physiological measures in soybean nodules and leaves, we revealed alterations in metabolites response to CO2 fertilization in plants inoculated with different Bradyrhizobium strains. This analysis clearly demonstrated that the soybeans inoculated with the strain isolated at e[CO2] (SFJ14-36) when grown at a[CO2] suffers changes in metabolic pathways that affect negatively plant growth and development such as a restricted photoassimilate content (sucrose, glucose, fructose). We hypothesize that under these conditions in the nodule, a more efficient use of C and N happened through a carboxylation of PEP to produce Asp instead of decarboxylation of PEP to produce Krebs dicarboxylic acids. In this way, nodule CO2 fixation may represent a C-saving mechanism and Asp can be used as N-exported compound or to produce ureides. At leaf level, plants inoculated with SFJ14-36 and grown at a[CO2] showed a complete rearrangement of processes such as photorespiration and Krebs cycle. This metabolic change at a[CO2] in plants inoculated with SFJ14-36 that were originally isolated at e[CO2], was probably due to a poor nodulation caused by a change in plant root exudates between elevated and ambient [CO2], affecting legume-bacteria interaction and, as a result, reducing N2-fixation and affecting N metabolism. In parallel, induced senescence likely happened as a result of N deprivation and was shown by the enhanced levels of free phytol, free fatty acids, and other compound related with chlorophyll and membrane degradation that may be caused by an oxidative stress due to the poor N status. The metabolism in leaves of the plants inoculated with the SFJ14-36 strain and grown at a[CO2] seems to swift from C assimilation to catabolism of chlorophyll and macromolecules such as fatty acids caused by N deficiency. However, more research is needed with other strains isolated at e[CO2] and more soybean cultivars in order to confirm this observations.
The original contributions presented in the study are included in the article/Supplementary Material, further inquiries can be directed to the corresponding author/s.
DS: formal analysis and writing original draft. IA: conceptualization, resource managing, review and editing. UP-L: experimentation, data curation, review, and editing. AM-P: experimentation, data curation, review, and editing. AM-R: resource managing, experimentation, review, and editing. ML: conceptualization, resource managing, experimentation, supervision, review, and editing. AS-S: conceptualization, experimentation, data curation, formal analysis, project administration, supervision, and writing original draft. All authors contributed to the article and approved the submitted version.
This work was financially supported by the following grants: GRUPO Gobierno Vasco IT1022-16 and projects 32-2016-00043, 37-2017-00047, and 000049-IDA2019-38 from the Economic Development and Infrastructures Department of the Basque Country, Spain.
The authors declare that the research was conducted in the absence of any commercial or financial relationships that could be construed as a potential conflict of interest.
DS was a recipient of a Ph.D. grant supported by the Public University of Navarra. AS-S was partially supported by a postdoctoral fellowship granted by the Education, and Linguistic Policy Department of the Basque Country, Spain and the Alabama Agricultural Experiment Station and the Hatch Program of the National Institute of Food and Agriculture, United States Department of Agriculture. Technical support by Azucena González, Phytotron Service, SGIker (UPV/EHU) is gratefully acknowledged.
The Supplementary Material for this article can be found online at: https://www.frontiersin.org/articles/10.3389/fpls.2021.656961/full#supplementary-material
Supplementary Figure 1 | Classification of metabolites detected by GC-TOF/MS-based analysis in leaves and nodules of plants inoculated with USDA110, SFJ4-24, and SFJ14-36 B. japonicum strains under ambient and elevated [CO2].
Abu-Shakra, S. S., Phillips, D. A., and Huffaker, R. A. (1978). Nitrogen Fixation and Delayed Leaf Senescence in Soybeans. Science 199, 973–975. doi: 10.1126/science.199.4332.973
Ainsworth, E. A., and Rogers, A. (2007). The response of photosynthesis and stomatal conductance to rising [CO2]: mechanisms and environmental interactions. Plant. Cell Environ. 30, 258–270. doi: 10.1111/j.1365-3040.2007.01641.x
Ainsworth, E. A., Davey, P. A., Bernacchi, C. J., Dermody, O. C., Heaton, E. A., Moore, D. J., et al. (2002). A meta-analysis of elevated [CO2] effects on soybean (Glycine max) physiology, growth and yield. Glob. Chang. Biol. 8, 695–709. doi: 10.1046/j.1365-2486.2002.00498.x
Ainsworth, E. A., Rogers, A., Nelson, R., and Long, S. P. (2004). Testing the “source–sink” hypothesis of down-regulation of photosynthesis in elevated [CO2] in the field with single gene substitutions in Glycine max. Agric. For. Meteorol. 122, 85–94. doi: 10.1016/J.AGRFORMET.2003.09.002
Aranjuelo, I., Arrese-Igor, C., and Molero, G. (2014). Nodule performance within a changing environmental context. J. Plant Physiol. 171, 1076–1090. doi: 10.1016/J.JPLPH.2014.04.002
Aranjuelo, I., Tcherkez, G., Jauregui, I., Gilard, F., Ancín, M., Millán, A. F.-S., et al. (2015). Alteration by thioredoxin f over-expression of primary carbon metabolism and its response to elevated CO2 in tobacco (Nicotiana tabacum L.). Environ. Exp. Bot. 118, 40–48. doi: 10.1016/J.ENVEXPBOT.2015.05.008
Barclay, K. D., and McKersie, B. D. (1994). Peroxidation reactions in plant membranes: Effects of free fatty acids. Lipids 29, 877–882. doi: 10.1007/BF02536256
Bei, Q., Liu, G., Tang, H., Cadisch, G., Rasche, F., and Xie, Z. (2013). Heterotrophic and phototrophic 15N2 fixation and distribution of fixed 15N in a flooded rice–soil system. Soil Biol. Biochem. 59, 25–31. doi: 10.1016/j.soilbio.2013.01.008
Berjak, P., and Pammenter, N. W. (2008). From Avicennia to Zizania: Seed Recalcitrance in Perspective. Ann. Bot. 101, 213–228. doi: 10.1093/aob/mcm168
Bertrand, A., Prévost, D., Bigras, F. J., Lalande, R., Tremblay, G. F., Castonguay, Y., et al. (2007). Alfalfa response to elevated atmospheric CO2 varies with the symbiotic rhizobial strain. Plant Soil 301, 173–187. doi: 10.1007/s11104-007-9436-9
Bertrand, A., Prévost, D., Juge, C., and Chalifour, F. (2011). Impact of elevated CO2 on carbohydrate and ureide concentrations in soybean inoculated with different strains of Bradyrhizobium japonicum. Botany 89, 481–490. doi: 10.1139/b11-034
Bishop, K. A., Betzelberger, A. M. Y. M., Long, S. P., and Ainsworth, E. A. (2015). Is there potential to adapt soybean (Glycine max Merr.) to future [CO2]? An analysis of the yield response of 18 genotypes in free-air CO2 enrichment. Plant. Cell Environ. 38, 1765–1774. doi: 10.1111/pce.12443
Booker, F. L., Reid, C. D., Brunschön-Harti, S., Fiscus, E. L., and Miller, J. E. (1997). Photosynthesis and photorespiration in soybean [Glycine max (L.) Merr.] chronically exposed to elevated carbon dioxide and ozone. J. Exp. Bot. 48, 1843–1852. doi: 10.1093/jxb/48.10.1843
Bouvier-Navé, P., Berna, A., Noiriel, A., Compagnon, V., Carlsson, A., Banas, A., et al. (2010). Involvement of the Phospholipid Sterol Acyltransferase1 in Plant Sterol Homeostasis and Leaf Senescence. Plant Physiol. 152, 107–119. doi: 10.1104/pp.109.145672
Chen, L.-L., Wang, G.-Z., and Zhang, H.-Y. (2007). Sterol biosynthesis and prokaryotes-to-eukaryotes evolution. Biochem. Biophys. Res. Commun. 363, 885–888. doi: 10.1016/J.BBRC.2007.09.093
CO2.earth (2020). CO2 Earth: A Pro Oxygen Website. URL: https://www.co2.earth
Das, A., Rushton, P. J., and Rohila, J. S. (2017). Metabolomic Profiling of Soybeans (Glycine max L.) Reveals the Importance of Sugar and Nitrogen Metabolism under Drought and Heat Stress. Plants 6:21. doi: 10.3390/plants6020021
Dorp, K., Vom Hölzl, G., Plohmann, C., Eisenhut, M., Abraham, M., Weber, A. P. M., et al. (2015). Remobilization of phytol from chlorophyll degradation is essential for Tocopherol synthesis and growth of arabidopsis. Plant Cell 27, 2846–2859. doi: 10.1105/tpc.15.00395
Duperon, R., Thiersault, M., and Duperon, P. (1984). High level of glycosylated sterols in species of solanum and sterol changes during the development of the tomato. Phytochemistry 23, 743–746. doi: 10.1016/S0031-9422(00)85016-5
Egli, D. B., Leggett, J. E., and Duncan, W. G. (1978). Influence of N Stress on Leaf Senescence and N Redistribution in Soybeans. Agron. J. 70, 43–47. doi: 10.2134/agronj1978.00021962007000010011x
El-Esawi, M. A., Elansary, H. O., El-Shanhorey, N. A., Abdel-Hamid, A. M. E., Ali, H. M., and Elshikh, M. S. (2017). Salicylic Acid-Regulated Antioxidant Mechanisms and Gene Expression Enhance Rosemary Performance under Saline Conditions. Front. Physiol. 8:716. doi: 10.3389/fphys.2017.00716
FAOSTAT (2020). ProdStat. Core Production Data Base, Electronic resource. URL: http://faostat.fao.org/.
Farquhar, G. D., von Caemmerer, S., and Berry, J. A. (1980). A biochemical model of photosynthetic CO2 assimilation in leaves of C3 species. Planta 149, 78–90. doi: 10.1007/BF00386231
Fehr, W. R., Caviness, C. E., Burmood, D. T., and Pennington, J. S. (1971). Stage of Development Descriptions for Soybeans, Glycine Max (L.) Merrill1. Crop Sci. 11, 929–931. doi: 10.2135/cropsci1971.0011183X001100060051x
Fischinger, S. A., Hristozkova, M., Zaman-Allah, M., and Schulze, J. (2010). Elevated CO2 concentration around alfalfa nodules increases N2 fixation. J. Exp. Bot. 61, 121–130. doi: 10.1093/jxb/erp287
Fischinger, S., and Schulze, J. (2010). The importance of nodule CO2 fixation for efficiency of symbiotic nitrogen fixation in pea at vegetative growth and during pod formation. J. Exp. Bot. 61, 2281–2291. doi: 10.1093/jxb/erq055
Ghasemzadeh, A., and Ghasemzadeh, N. (2011). Flavonoids and phenolic acids: Role and biochemical activity in plants and human. J. Med. Plants Res. 5, 6697–6703. doi: 10.5897/JMPR11.1404
Gillon, J. S., and Yakir, D. (2000). Internal conductance to CO2 diffusion and C18OO discrimination in C3 leaves. Plant Physiol. 123, 201–214. doi: 10.1104/pp.123.1.201
Gutiérrez, D., Gutiérrez, E., Pérez, P., Morcuende, R., Verdejo, A. L., and Martinez-Carrasco, R. (2009). Acclimation to future atmospheric CO2 levels increases photochemical efficiency and mitigates photochemistry inhibition by warm temperatures in wheat under field chambers. Physiol. Plant. 137, 86–100. doi: 10.1111/j.1399-3054.2009.01256.x
Hayes, J. M. (2001). Fractionation of Carbon and Hydrogen Isotopes in Biosynthetic Processes. Rev. Mineral. Geochem. 43, 225–277. doi: 10.2138/gsrmg.43.1.225
Holmer, G., Ory, R. L., and Høy, C.-E. (1973). Changes in lipid composition of germinating barley embryo. Lipids 8, 277–283. doi: 10.1007/BF02531905
Igamberdiev, A. U., and Kleczkowski, L. A. (2018). The Glycerate and Phosphorylated Pathways of Serine Synthesis in Plants: The Branches of Plant Glycolysis Linking Carbon and Nitrogen Metabolism. Front. Plant Sci. 9:318. doi: 10.3389/fpls.2018.00318
IPCC (2013). “Summary for policymakers,” in Climate Change 2013: The Physical ScienceBasis. Contribution of Working Group I to the Fifth Assessment Report of the Intergovern-mental Panel on Climate Change, eds T. F. Stocker, D. Qin, G.-K. Plattner, M. Tignor, S. K. Allen, J. Boschung, et al. (Cambridge, UK: Cambridge University Press), 3–29.
Ischebeck, T., Zbierzak, A. M., Kanwischer, M., and Dörmann, P. (2006). A salvage pathway for phytol metabolism in Arabidopsis. J. Biol. Chem. 281, 2470–2477. doi: 10.1074/jbc.M509222200
Kaschuk, G., Hungria, M., Leffelaar, P. A., Giller, K. E., and Kuyper, T. W. (2010). Differences in photosynthetic behaviour and leaf senescence of soybean (Glycine max [L.] Merrill) dependent on N2 fixation or nitrate supply. Plant Biol. 12, 60–69. doi: 10.1111/j.1438-8677.2009.00211.x
Kaschuk, G., Kuyper, T. W., Leffelaar, P. A., Hungria, M., and Giller, K. E. (2009). Are the rates of photosynthesis stimulated by the carbon sink strength of rhizobial and arbuscular mycorrhizal symbioses? Soil Biol. Biochem. 41, 1233–1244. doi: 10.1016/j.soilbio.2009.03.005
Keys, A. J. (1999). “Biochemistry of photorespiration and the consequences for plant performance,” in Plant carbohydrate biochemistry, eds J. A. Bryant, M. M. Burrell, and N. J. Kruger (Oxford, UK: BIOS ScientiÆc), 147–161.
King, C. A., and Purcell, L. C. (2005). Inhibition of N2 Fixation in Soybean Is Associated with Elevated Ureides and Amino Acids. Plant Physiol. 137, 1389–1396. doi: 10.1104/pp.104.056317
Kováčik, J., Grúz, J., Bačkor, M., Strnad, M., and Repčák, M. (2008). Salicylic acid-induced changes to growth and phenolic metabolism in Matricaria chamomilla plants. Plant Cell Rep. 28:135. doi: 10.1007/s00299-008-0627-5
Ladrera, R., Marino, D., Larrainzar, E., González, E. M., and Arrese-Igor, C. (2007). Reduced carbon availability to bacteroids and elevated ureides in nodules, but not in shoots, are involved in the nitrogen fixation response to early drought in soybean. Plant Physiol. 145, 539–546. doi: 10.1104/pp.107.102491
Li, L., Hur, M., Lee, J.-Y., Zhou, W., Song, Z., Ransom, N., et al. (2015). A systems biology approach toward understanding seed composition in soybean. BMC Genomics 16:S9. doi: 10.1186/1471-2164-16-S3-S9
Li, L., Zhao, J., Zhao, Y., Lu, X., Zhou, Z., Zhao, C., et al. (2016). Comprehensive investigation of tobacco leaves during natural early senescence via multi-platform metabolomics analyses. Sci. Rep. 6, 2–11. doi: 10.1038/srep37976
Li, T., Hu, Y., Du, X., Tang, H., Shen, C., and Wu, J. (2014). Salicylic Acid Alleviates the Adverse Effects of Salt Stress in Torreya grandis cv. Merrillii Seedlings by Activating Photosynthesis and Enhancing Antioxidant Systems. PLoS One 9:e109492. doi: 10.1371/journal.pone.0109492
Lim, P. O., Kim, H. J., and Gil Nam, H. (2007). Leaf Senescence. Annu. Rev. Plant Biol. 58, 115–136. doi: 10.1146/annurev.arplant.57.032905.105316
Lima, J. D., and Sodek, L. (2003). N-stress alters aspartate and asparagine levels of xylem sap in soybean. Plant Sci. 165, 649–656. doi: 10.1016/S0168-9452(03)00251-6
Lin, J.-S., and Wang, G.-X. (2002). Doubled CO2 could improve the drought tolerance better in sensitive cultivars than in tolerant cultivars in spring wheat. Plant Sci. 163, 627–637. doi: 10.1016/S0168-9452(02)00173-5
Lippold, F., vom Dorp, K., Abraham, M., Hölzl, G., Wewer, V., Yilmaz, J. L., et al. (2012). Fatty acid phytyl ester synthesis in chloroplasts of Arabidopsis. Plant Cell 24, 2001–2014. doi: 10.1105/tpc.112.095588
Lodwig, E., and Poole, P. (2003). Metabolism of Rhizobium bacteroids. CRC. Crit. Rev. Plant Sci. 22, 37–78. doi: 10.1080/713610850
Mach, J. (2015). Phytol from Degradation of Chlorophyll Feeds Biosynthesis of Tocopherols. Plant Cell 27:2676. doi: 10.1105/tpc.15.00860
Medic, J., Atkinson, C., and Hurburgh, C. R. Jr. (2014). Current Knowledge in Soybean Composition. J. Am. Oil Chem. Soc. 91, 363–384. doi: 10.1007/s11746-013-2407-9
Melzer, E., and O’Leary, M. H. (1991). Aspartic-acid synthesis in C3 plants. Planta 185, 368–371. doi: 10.1007/BF00201058
Mishra, S., Tyagi, A., Singh, I. V., and Sangwan, R. S. (2006). Changes in lipid profile during growth and senescence of Catharanthus roseus leaf. Brazilian J. Plant Physiol. 18, 447–454. doi: 10.1590/S1677-04202006000400002
Molero, G., Tcherkez, G., Roca, R., Mauve, C., Cabrera-Bosquet, L., Araus, J. L., et al. (2019). Do metabolic changes underpin physiological responses to water limitation in alfalfa (Medicago sativa) plants during a regrowth period? Agric. Water Manag. 212, 1–11. doi: 10.1016/j.agwat.2018.08.021
Moore, T. C. (1974). Symbiotic Nitrogen Fixation in Legume Nodules. In: Research Experiences in Plant Physiology. Berlin: Springer, doi: 10.1007/978-3-642-96168-7-26
Morgan, P., Bollero, G., Nelson, R., Dohleman, F., and Long, S. (2005). Smaller than predicted increase in aboveground net primary production and yield of field-grown soybean under fully open-air [CO2] elevation. Glob. Chang. Biol. 11, 1856–1865. doi: 10.1111/j.1365-2486.2005.001017.x
Munné-Bosch, S. (2005). The role of α-tocopherol in plant stress tolerance. J. Plant Physiol. 162, 743–748. doi: 10.1016/J.JPLPH.2005.04.022
Munné-Bosch, S. (2007). α−Tocopherol: A Multifaceted Molecule in Plants. Vitam. Horm. 76, 375–392. doi: 10.1016/S0083-6729(07)76014-4
Noctor, G., Veljovic-Jovanovic, S., Driscoll, S., Novitskaya, L., and Foyer, C. (2002). Drought and Oxidative Load in the Leaves of C3 Plants: a Predominant Role for Photorespiration? 89, 841–850. doi: 10.1093/aob/mcf096
Obata, T., and Fernie, A. R. (2012). The use of metabolomics to dissect plant responses to abiotic stresses. Cell. Mol. Life Sci. 69, 3225–3243. doi: 10.1007/s00018-012-1091-5
Padan, E., Bibi, E., Ito, M., and Krulwich, T. A. (2005). Alkaline pH homeostasis in bacteria: New insights. Biochim. Biophys. Acta Biomembr. 1717, 67–88. doi: 10.1016/J.BBAMEM.2005.09.010
Peisker, C., Düggelin, T., Rentsch, D., and Matile, P. (1989). Phytol and the Breakdown of Chlorophyll in Senescent Leaves. J. Plant Physiol. 135, 428–432. doi: 10.1016/S0176-1617(89)80099-9
Prévost, D., Bertrand, A., Juge, C., and Chalifour, F. P. (2010). Elevated CO2 induces differences in nodulation of soybean depending on bradyrhizobial strain and method of inoculation. Plant Soil 331, 115–127. doi: 10.1007/s11104-009-0238-0
Puiatti, M., and Sodek, L. (1999). Waterlogging affects nitrogen transport in the xylem of soybean. Plant Physiol. Biochem. 37, 767–773. doi: 10.1016/S0981-9428(00)86690-5
Rabara, R. C., Tripathi, P., and Rushton, P. J. (2017). Comparative Metabolome Profile between Tobacco and Soybean Grown under Water-Stressed Conditions. Biomed. Res. Int. 2017:3065251. doi: 10.1155/2017/3065251
Rachmilevitch, S., Cousins, A. B., and Bloom, A. J. (2004). Nitrate assimilation in plant shoots depends on photorespiration. Proc. Natl. Acad. Sci. U. S. A. 101, 11506–11510. doi: 10.1073/pnas.0404388101
Rogers, A., Gibon, Y., Stitt, M., Morgan, P., Bernacchi, C., Ort, D., et al. (2006). Increased C availability at elevated carbon dioxide concentration improves N assimilation in a legume. Plant Cell Environ. 29, 1651–1658. doi: 10.1111/j.1365-3040.2006.01549.x
Ruuska, S. A., Badger, M. R., Andrews, T. J., and von Caemmerer, S. (2000). Photosynthetic electron sinks in transgenic tobacco with reduced amounts of Rubisco: little evidence for significant Mehler reaction. J. Exp. Bot. 51, 357–368. doi: 10.1093/jexbot/51.suppl_1.357
Saito, K., and Matsuda, F. (2008). Metabolomics for Functional Genomics, Systems Biology, and Biotechnology. Annu. Rev. Plant Biol. 61, 463–489. doi: 10.1146/annurev.arplant.043008.092035
Saiz-Fernández, I., Lacuesta, M., Pérez-López, U., Sampedro, M. C., Barrio, R. J., and De Diego, N. (2020). Interplay between 1-aminocyclopropane-1-carboxylic acid, γ-aminobutyrate and D-glucose in the regulation of high nitrate-induced root growth inhibition in maize. Plant Sci. 293:110418. doi: 10.1016/j.plantsci.2020.110418
Salminen, S. O., and Streeter, J. G. (1987). Involvement of glutamate in the respiratory metabolism of Bradyrhizobium japonicum bacteroids. J. Bacteriol. 169, 495–499. doi: 10.1128/jb.169.2.495-499.1987
Salminen, S. O., and Streeter, J. G. (1992). Labeling of Carbon Pools in Bradyrhizobium japonicum and Rhizobium leguminosarum bv viciae Bacteroids following Incubation of Intact Nodules with 14CO2. Plant Physiol. 100, 597–604. doi: 10.1104/pp.100.2.597
Sanz-Sáez, Á, Erice, G., Aguirreolea, J., Irigoyen, J. J., and Sánchez-Díaz, M. (2012). Alfalfa yield under elevated CO2 and temperature depends on the Sinorhizobium strain and growth season. Environ. Exp. Bot. 77, 267–273. doi: 10.1016/J.ENVEXPBOT.2011.11.017
Sanz-Sáez, Á, Heath, K. D., Burke, P. V., and Ainsworth, E. A. (2015). Inoculation with an enhanced N2-fixing Bradyrhizobium japonicum strain (USDA110) does not alter soybean (Glycine max Merr.) response to elevated [CO2]. Plant. Cell Environ. 38, 2589–2602. doi: 10.1111/pce.12577
Sanz-Sáez, Á, Koester, R. P., Rosenthal, D. M., Montes, C. M., Ort, D. R., and Ainsworth, E. A. (2017). Leaf and canopy scale drivers of genotypic variation in soybean response to elevated carbon dioxide concentration. Glob. Chang. Biol. 23, 3908–3920. doi: 10.1111/gcb.13678
Sanz-Sáez, A., Pérez-López, U., del-Canto, A., Ortiz-Barredo, A., Mena-Petite, A., Aranjuelo, I., et al. (2019). Changes in environmental CO2 concentration can modify Rhizobium-soybean specificity and condition plant fitness and productivity. Environ. Exp. Bot. 162, 133–143. doi: 10.1016/J.ENVEXPBOT.2019.01.013
Serraj, R., Vadez, V., and Sinclair, T. (2001). Feedback regulation of symbiotic N2 fixation under drought stress. Agronomie 21, 621–626. doi: 10.1051/agro:2001153
Serraj, R., Vadez, V., Denison, R. F., and Sinclair, T. R. (1999). Involvement of Ureides in Nitrogen Fixation Inhibition in Soybean. Plant Physiol. 119, 289–296. doi: 10.1104/pp.119.1.289
Sharkey, T. D. (1988). Estimating the rate of photorespiration in leaves. Physiol. Plant. 73, 147–152. doi: 10.1111/j.1399-3054.1988.tb09205.x
Silvente, S., Camas, A., and Lara, M. (2003). Molecular cloning of the cDNA encoding aspartate aminotransferase from bean root nodules and determination of its role in nodule nitrogen metabolism. J. Exp. Bot. 54, 1545–1551. doi: 10.1093/jxb/erg161
Soba, D., Ben Mariem, S., Fuertes-Mendizábal, T., Méndez-Espinoza, A. M., Gilard, F., González-Murua, C., et al. (2019a). Metabolic Effects of Elevated CO2 on Wheat Grain Development and Composition. J. Agric. Food Chem. 67, 8441–8451. doi: 10.1021/acs.jafc.9b01594
Soba, D., Müller, M., Aranjuelo, I., and Munné-Bosch, S. (2020a). Vitamin E in legume nodules: Occurrence and antioxidant function. Phytochemistry 172:112261. doi: 10.1016/j.phytochem.2020.112261
Soba, D., Shu, T., Runion, G. B., Prior, S. A., Fritschi, F. B., Aranjuelo, I., et al. (2020b). Effects of elevated [CO2] on photosynthesis and seed yield parameters in two soybean genotypes with contrasting water use efficiency. Environ. Exp. Bot. 178:104154. doi: 10.1016/j.envexpbot.2020.104154
Soba, D., Zhou, B., Arrese-Igor, C., Munné-Bosch, S., and Aranjuelo, I. (2019b). Physiological, hormonal and metabolic responses of two alfalfa cultivars with contrasting responses to drought. Int. J. Mol. Sci. 20:5099. doi: 10.3390/ijms20205099
Sugawara, M., and Sadowsky, M. (2013). Influence of Elevated Atmospheric Carbon Dioxide on Transcriptional Responses of Bradyrhizobium japonicum in the Soybean Rhizoplane. Microbes Environ. 28, 217–227. doi: 10.1264/jsme2.ME12190
Tcherkez, G., Ben Mariem, S., Larraya, L., García-Mina, J. M., Zamarreño, A. M., Paradela, A., et al. (2020). Elevated CO2 has concurrent effects on leaf and grain metabolism but minimal effects on yield in wheat. J. Exp. Bot. 71, 5990–6003. doi: 10.1093/jxb/eraa330
Tcherkez, G., Bligny, R., Gout, E., Mahé, A., Hodges, M., and Cornic, G. (2008). Respiratory metabolism of illuminated leaves depends on CO2 and O2 conditions. Proc. Natl. Acad. Sci. U. S. A. 105, 797–802. doi: 10.1073/pnas.0708947105
Troncoso-Ponce, M. A., Cao, X., Yang, Z., and Ohlrogge, J. B. (2013). Lipid turnover during senescence. Plant Sci. 20, 13–19. doi: 10.1016/j.plantsci.2013.01.004
von Caemmerer, S. (2000). Biochemical models of leaf photosynthesis. Collingwood. Australia: CSIRO Publishing.
von Caemmerer, S., Evans, J., Hudson, G., and Andrews, T. (1994). The kinetics of ribulose-1,5-bisphosphate carboxylase/oxygenase in vivo inferred from measurements of photosynthesis in leaves of transgenic tobacco. Planta 195, 88–97. doi: 10.1007/BF00206296
Wang, P., Marsh, E. L., Ainsworth, E. A., Leakey, A. D. B., Sheflin, A. M., and Schachtman, D. P. (2017). Shifts in microbial communities in soil, rhizosphere and roots of two major crop systems under elevated CO2 and O3. Sci. Rep. 7:15019. doi: 10.1038/s41598-017-14936-2
Woodward, F. I. (2002). Potential impacts of global elevated CO2 concentrations on plants. Curr. Opin. Plant Biol. 5, 207–211. doi: 10.1016/S1369-5266(02)00253-4
Keywords: Bradyrhizobium strains, C and N metabolism, elevated [CO2], metabolomics, N-fixation, nodule, soybean
Citation: Soba D, Aranjuelo I, Gakière B, Gilard F, Pérez-López U, Mena-Petite A, Muñoz-Rueda A, Lacuesta M and Sanz-Saez A (2021) Soybean Inoculated With One Bradyrhizobium Strain Isolated at Elevated [CO2] Show an Impaired C and N Metabolism When Grown at Ambient [CO2]. Front. Plant Sci. 12:656961. doi: 10.3389/fpls.2021.656961
Received: 21 January 2021; Accepted: 31 March 2021;
Published: 20 May 2021.
Edited by:
Luisa M. Sandalio, Departamento de Bioquímica, Biología Celular y Molecular de Plantas, Estación Experimental del Zaidín (EEZ), SpainReviewed by:
Juan Sanjuan, Estación Experimental de Zaidín, Consejo Superior de Investigaciones Cient ficas, Spanish National Research Council, SpainCopyright © 2021 Soba, Aranjuelo, Gakière, Gilard, Pérez-López, Mena-Petite, Muñoz-Rueda, Lacuesta and Sanz-Saez. This is an open-access article distributed under the terms of the Creative Commons Attribution License (CC BY). The use, distribution or reproduction in other forums is permitted, provided the original author(s) and the copyright owner(s) are credited and that the original publication in this journal is cited, in accordance with accepted academic practice. No use, distribution or reproduction is permitted which does not comply with these terms.
*Correspondence: Maite Lacuesta, bWFpdGUubGFjdWVzdGFAZWh1LmVz; Alvaro Sanz-Saez, YXpzMDIyM0BhdWJ1cm4uZWR1
†These authors have contributed equally to this work
Disclaimer: All claims expressed in this article are solely those of the authors and do not necessarily represent those of their affiliated organizations, or those of the publisher, the editors and the reviewers. Any product that may be evaluated in this article or claim that may be made by its manufacturer is not guaranteed or endorsed by the publisher.
Research integrity at Frontiers
Learn more about the work of our research integrity team to safeguard the quality of each article we publish.