Corrigendum: Role of Hydraulic Signal and ABA in Decrease of Leaf Stomatal and Mesophyll Conductance in Soil Drought-Stressed Tomato
- 1Key Laboratory of Crop Water Use and Regulation, Ministry of Agriculture and Rural Affairs, Farmland Irrigation Research Institute, Chinese Academy of Agricultural Sciences, Xinxiang, China
- 2Graduate School of Chinese Academy of Agricultural Sciences, Beijing, China
- 3School of Applied Meteorology, Nanjing University of Information Science and Technology, Nanjing, China
Drought reduces leaf stomatal conductance (gs) and mesophyll conductance (gm). Both hydraulic signals and chemical signals (mainly abscisic acid, ABA) are involved in regulating gs. However, it remains unclear what role the endogenous ABA plays in gm under decreasing soil moisture. In this study, the responses of gs and gm to ABA were investigated under progressive soil drying conditions and their impacts on net photosynthesis (An) and intrinsic water use efficiency (WUEi) were also analyzed. Experimental tomato plants were cultivated in pots in an environment-controlled greenhouse. Reductions of gs and gm induced a 68–78% decline of An under drought conditions. While soil water potential (Ψsoil) was over −1.01 MPa, gs reduced as leaf water potential (Ψleaf) decreased, but ABA and gm kept unchanged, which indicating gs was more sensitive to drought than gm. During Ψsoil reduction from −1.01 to −1.44 MPa, Ψleaf still kept decreasing, and both gs and gm decreased concurrently following to the sustained increases of ABA content in shoot sap. The gm was positively correlated to gs during a drying process. Compared to gs or gm, WUEi was strongly correlated with gm/gs. WUEi improved within Ψsoil range between −0.83 and −1.15 MPa. In summary, gs showed a higher sensitivity to drought than gm. Under moderate and severe drought at Ψsoil ≤ −1.01 MPa, furthermore from hydraulic signals, ABA was also involved in this co-ordination reductions of gs and gm and thereby regulated An and WUEi.
Introduction
Soil water scarcity is one of the major environmental constraints to the plant physiological processes and yield (Easlon and Richards, 2009; Olsovska et al., 2016). To achieve high plant water-use efficiency under a drier environment in the future, it is essential to improve crop photosynthesis and productivity with a given unit of water (Flexas et al., 2013). For C3 plants, leaf photosynthesis is strongly limited by three factors, i.e., stomatal conductance (gs), mesophyll diffusion conductance to CO2 (gm), and biochemical photosynthetic capacity (Grassi and Magnani, 2005; Cano et al., 2013). gs and gm determine the diffusion of CO2 from ambient air of leaf to sub-stomatal cavities and from the sub-stomatal cavities to chloroplast stroma, respectively (Flexas et al., 2002; Niinemets et al., 2009). Recent studies have shown that both gs and gm were the main limitations for maximum photosynthesis under drought conditions (Tosens et al., 2016; Wang et al., 2018). Therefore, revealing the mechanisms underlying the decreases of gs and gm in response to drought is necessary for enhancing our understanding of plant adaptation to water limitation.
Different regulatory mechanisms such as chemical messengers like abscisic acid (ABA), electrical signals, and hydraulic signals have been identified in the control of stomatal movement (Dodd, 2005; Ache et al., 2010; Tombesi et al., 2015; Huber et al., 2019). Despite the large list of candidates in regulating guard cells, ABA and hydraulic signals have gained most of the attention in regulating stomatal aperture. ABA is a phytohormone that has been involved in different strategies of plants to avoid excessive water loss, and many reports demonstrated its important role in stomatal control (Wilkinson and Davies, 2002; Assmann and Jegla, 2016). The decrease of gs in response to drought has been generally modulated by the accumulation of leaf ABA in a wide number of plant species including soybean, grapevine and tomato (Liu et al., 2005; Tombesi et al., 2015; Yan et al., 2017). However, stomata closed with a wide range of variations of leaf hydraulic signals, such as leaf water potential (Ψleaf), possibly due to differences of experimental plant materials and the intensity of applied drought under investigation. For example, gs decreased with decreasing Ψleaf during leaf dehydration (Kim et al., 2012; Wang et al., 2018). On the contrary, other studies showed that stomata closed with little change in Ψleaf under moderate soil drying, but both parameters decreased under severe drought (Tardieu, 1998; Yan et al., 2017), or gs decreased as Ψleaf increased under mild soil drying but then no significant relationship existed between both variables with continued soil drying (Kudoyarova et al., 2007). It is difficult to explore the response of Ψleaf and gs under a single soil water condition. Progressive soil drying, representing a natural process of soil water loss, could help us explore the dynamic responses of gs to Ψleaf during drying process.
Leaf mesophyll conductance to CO2 (gm) has been recognized to be finite, variable, and rapid acclimation to varying environmental conditions. Although a reduction in gm response to soil drought has been reported in many studies, the mechanisms underlying this reduction have not been elucidated substantially (Flexas et al., 2002; Théroux-Rancourt et al., 2014; Sorrentino et al., 2016). Recent studies on hydraulic signals suggested that the parallel decreases in gs and gm were caused by leaf hydraulic vulnerability as a result of decrease in Ψleaf (Wang et al., 2018). Similarly, gm was strongly correlated with leaf hydraulic conductance (Kleaf), as the ratio of transpiration rate to the water potential driving force across the leaf (Kleaf = transpiration/ΔΨleaf), across species under light-saturated conditions (Xiong et al., 2018). This correlation between gm and leaf hydraulic signals might be due to CO2 partially shared common diffusion pathways with H2O through mesophyll tissues (Ferrio et al., 2012). These studies confirmed that leaf hydraulic signals played an essential role in controlling gm in response to drought. However, the effects of chemical ABA signal on gm are not consistent. Vrabl et al. (2009) did not observe any reduction in gm when applied exogenous ABA in Helianthus annuus plants. In line with this, Flexas et al. (2013) found that gm was highly insensitive to endogenous ABA among ABA-insensitive and ABA-hypersensitive genotypes or to exogenous ABA application in Arabidopsis thaliana. However, several studies yielded contrasting results. For instance, Mizokami et al. (2015) compared the responses of gm to leaf ABA in wild type and ABA-deficient mutant of Nicotiana plumbaginifolia and confirmed that the increase in leaf ABA concentration was crucial for the decrease in gm under drought conditions. Still, gm reduced effectively in response to ABA in a short term in three of the four species in Sorrentino et al. (2016). Recently, Mizokami et al. (2018) examined the responses of gm to high CO2 and ABA application and revealed that gm was able to respond to high ABA levels, which was intrinsically different from the response to the elevated CO2. These contrasting results possibly due to species differences or the experimental approaches utilized to modify ABA, e.g., the exogenous ABA concentration or the applying period. In brief, it has been largely demonstrated that hydraulic signals play an important role in regulating gm, while the role of ABA on gm is still not unequivocal. Therefore, a deep understanding about the mechanisms of gm response to endogenous ABA under progressive soil drying conditions awaits further investigation.
Leaf intrinsic water use efficiency (WUEi), expressed as the ratio of net photosynthetic rate (An) to gs at leaf level, can explain instantaneous responses to environmental factors (Flexas et al., 2016; Qiu et al., 2019). Improving WUEi need increase An and decrease gs simultaneously. Using An/gs to explain the changes of WUEi would be too coarse due to the decrease in gs inevitably affect CO2 uptake and thereby limit An. gm determines the CO2 concentration at the carboxylation site in the chloroplast, increasing gm would increase An without increasing water loss. Therefore, gm might play a role in improving WUEi. Despite all of the negative impacts of drought stress on leaf gas exchange, many studies reported that drought was beneficial to improve WUEi (Liu et al., 2005; Xue et al., 2016). However, the reasons of this improvement of WUEi have not been elucidated clearly. Evidences have suggested that gm/gs played a key role on increasing WUEi in response to water limitation (Flexas et al., 2016; Han et al., 2016). Revealing the exact responses of gm/gs or WUEi to stressed signals especially ABA under progressive soil drought would be of great interest in the selection of varieties with high yield in breeding and strong adaptability under varied environmental conditions.
In this study, relationships between gs, gm, and Ψleaf or ABA were examined in tomato seedlings under progressive soil drying conditions. The objectives of this study were (i) to evaluate the effects of limiting factors of gs and gm on An in tomato plants during progressive soil drying, (ii) to investigate the responses of gs and gm to drought signals (Ψleaf and ABA) under increasing drought stress, and (iii) to reveal the effects of gs/gm on WUEi in tomato seedlings during the progressive soil drying.
Materials and Methods
Plant Material and Soil Water Treatments
Seeds of tomato (Solanum lycopersicum L., cv. Helan108) were sown in nursery seedling plate with substrate (sphagnum peat, Pindstrup Mosebrug A/S, Ryomgaard, Denmark). When the second true leaf emerged, tomato seedlings were transplanted into 5.3 L pots (height 30 cm, diameter 15 cm). Each pot was filled with 6.5 kg air-dried sandy loam soil. The gravimetric field water capacity (θFC) and wilting point were 22% (g g−1) and 6.8% (g g−1), respectively. After transplanting, all pots were irrigated to 85% θFC with Hoagland solution [5 mM KNO3, 5 mM Ca (NO3)2 4H2O, 1 mM KH2PO4, and 1 mM MgSO4 7H2O, 1 ml l−1 micronutrients, pH = 6.0]. Seedlings were cultivated in an environment-controlled chamber [day/night air temperature 25/18°C, 50–60% relative humidity, 12 h photoperiod at 600 μmol m−2 s−1 photosynthetic photon flux density (PPFD) supplied by LED lamps from 7:00 to 19:00]. All pots were weighted daily at 8:00 a.m. to calculate daily irrigation amount. During the experiment, same volume of Hoagland solution was applied to all pots to avoid nutrient differences. Soil water content was expressed as relative soil water content (RSWC), i.e., the ratio between the current soil moisture (θC) and θFC.
Water treatments (including well-watered and progressive drought-stressed treatments) were conducted at the 27 day after transplanting (DAT). For the well-watered treatment, RSWC was maintained within the range of 70–82% θFC throughout the experiment. Plants remained well-watered acted as a control group (CK). For the drought-stressed treatment (withholding water), RSWC decreased from 82.90% θFC to 37.27% θFC from 27 to 33 DAT. On each day of the drying period (28–33 DAT), the relevant experimental indexes were measured and collected for the two treatments.
Leaf Gas Exchange and Chlorophyll Fluorescence Measurements
Leaf gas exchange and chlorophyll fluorescence were measured simultaneously using an open gas exchange system Li-Cor 6400 photosynthesis system (Li-Cor Inc., Lincoln, NE, USA) equipped with an integrated leaf fluorometer chamber (Li-Cor 6400-40) from 9:00 to 14:00 h. All measurements were recorded on the same fully expanded leaves (the 6th or 7th leaves from the base of the plant) during 28–33 DAT, using two or six replicate plants for CK and water stressed treatment, respectively. During the measurements, the PPFD was kept at 1500 μmol m−2 s−1, the sample CO2 concentration was maintained at 400 μmol mol−1 with a CO2 cylinder. Relative humidity was kept at 55%. Leaf gas exchange, chlorophyll fluorescence and leaf temperature were recorded when An was stabilized on these conditions (usually 20 min after clamping the leaf). After that, A-Ci response curves were conducted. During the measurements, the PPFD was kept as constant of 1500 μmol m−2 s−1, sample CO2 concentration was adjusted in a series of: 400, 300, 200, 150, 100, 50, 400, 400, 600, 800, 1000, 1200, 1400, 1600 μmol mol−1.
The intrinsic water use efficiency (WUEi, μmol CO2 mol−1 H2O) was calculated as the ratio of net photosynthetic rate divided by stomatal conductance:
where An is net photosynthesis rate (μmol CO2 m−2 s−1), gs is stomatal conductance (mol H2O m−2 s−1).
The actual photochemical efficiency of photosystem II (ΦPSII) was determined by measuring steady-state fluorescence (Fs) and maximum fluorescence () during a light-saturating pulse of ca. 8000 mmol m−2 s−1:
The electron transport rate (Jf) was then calculated as:
where PPFD was maintained at 1500 μmol m−2 s−1 on both the well-watered and water-stressed leaves. α represents the leaf absorptance and β reflects the partitioning of absorbed quanta between photosystems II and I. α and β were assumed to be 0.84 and 0.5 in the study, respectively (Laisk and Loreto, 1996; Flexas et al., 2002).
Estimation of gm by Gas Exchange and Chlorophyll Fluorescence Method
gm was calculated by the variable J method of Harley et al. (1992), as follows:
where Ci represents intercellular CO2 concentration (μmol CO2 mol−1), Rd represents the light mitochondrial respiration (μmol CO2 mol−1), which was calculated as 1/2 of the dark respiration Xiong et al. (2018), Γ* is the chloroplast CO2 compensation point (μmol CO2 mol−1), a leaf temperature-dependent parameter, and estimated as:
where c is the scaling constant (dimensionless), Ha is the energies of activation (KJ mol−1), and R is the molar gas constant (8.314 J K−1 mol−1). At the leaf temperature of 25°C, c and Ha in S. lycopersicum were equal to 12.7 and 23.2 (KJ mol−1), respectively (Hermida-Carrera et al., 2016). Tk is the leaf absolute assay temperature (K), which was recorded by the LI-6400 system and corrected to Kelvin temperature.
Given the potential errors in estimation made by the variable J method, sensitivity analyses were conducted to determine the effect of ±20% error of Rd, Γ*, Jf, and Ci on calculation of gm.
Photosynthetic Limitation Analysis
The relative photosynthesis limitations of An resulting from gs (ls), gm (lm), and biochemical photosynthetic capacity (lb) (ls + lm + lb = 1) was determined using the method of Grassi and Magnani (2005), as follows:
where gsc is the stomatal conductance to CO2 (mol CO2 m−2 s−1), gsc = gs/1.6, gt is the total conductance to CO2 from the leaf surface CO2 to chloroplast (1/gt = 1/gsc + 1/gm). According to the Farquhar model (Farquhar, 1980), ∂A/∂Cc can be calculated as follows:
where Kc and Ko are the Rubisco Michaelis–Menten constants for CO2 and O2, both of them were temperature-dependent and calculated as Equation (5). Specific values of these parameters in Equation (5) were obtained from Sharkey et al. (2007). O is the atmospheric O2 concentration (210 mmol mol−1). Vcmax is the maximum carboxylation capacity (μmol m−2 s−1). Vcmax was calculated from the A/Ci curve fitting method (Long and Bernacchi, 2003).
Soil and Leaf Water Potential Measurement and Shoot Sap Collection
Leaf water potential (Ψleaf) was measured on the same leaves as the measurement of gas exchange. Soil samples at the 10–12 cm under soil surface were collected to measure soil water potential (Ψsoil). Both Ψleaf and Ψsoil were measured by the WP4C Dewpoint Potentiometer (Meter Group Inc., Pullman, WA, USA) with two or six repetitions for CK and water stressed treatment. Meanwhile, the shoot part (including stem and leaf) was put into the Model 3115 pressure chamber (Plant Moisture Equipment, Santa Barbara, CA, USA). Pressure was increased gradually until sap outflowed at the cut surface. After discarding the first 1–2 drops, nearly 2 ml of sap was collected into centrifuge tube frozen in liquid nitrogen and then stored at −80°C for ABA analysis.
ABA Determination
The concentration of ABA was determined as previously described by Li et al. (2020). Briefly, sap ABA concentration was measured with a high-performance liquid chromatography-tandem mass spectrometry (Agilent Technologies Inc., Santa Clara, CA, USA), quantitated as the methods of isotope internal standard.
Statistical Analysis
All statistical analyses were performed using SPSS 16.0 (IBM Corp., Armonk, NY, United States). The significance of differences between mean values was assessed by One-way analysis of variance (ANOVA) according to Dennett's test at P < 0.05 level. Regressions were fitted by linear or non-linear models, and the model with higher regression coefficient (r2) was selected. Regression lines was shown when P < 0.05. All graphics and regressions were performed in Origin-Pro 2017 (Origin Lab, Northampton, MA, USA).
Results
Dynamic of Soil Water Status
Relative soil water content (RSWC) and Ψsoil of the well-watered pots were maintained at an average of 75.13% and −0.43 MPa, indicating no water stress occurred during the experiment. By withholding irrigation from 27 to 33 DAT during the progressive drying process, RSWC in the drought treatment decreased gradually from 82.90 to 37.27% and Ψsoil decreased by 1.04 MPa correspondingly. Interestingly, significant reduction of both RSWC and Ψsoil occurred simultaneously at 29 DAT (Figure 1).
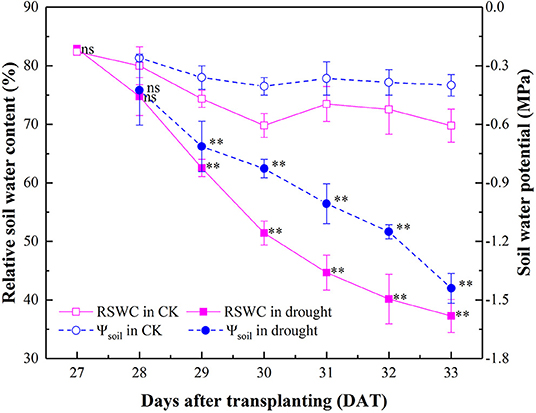
Figure 1. Dynamics of RSWC and Ψsoil in the well-watered (CK) and drought-stressed tomato seedlings during 27–33 DAT. Mean values and SD were presented (n = 6). ns indicated no significant difference and ** indicated significant difference at P < 0.01 level between drought and well-watered treatment.
Effects of Drought on Ψleaf and ABA
In the well-watered treatment, Ψleaf maintained at an average of −0.72 MPa from 27 to 33 DAT. Along with decreasing Ψsoil in the pots, Ψleaf of the drought-stressed tomato seedlings kept almost constant until Ψsoil reached to −0.71 MPa (Figure 2A). However, ABA did not statistically increase within the range of Ψsoil from −0.42 to −0.83 MPa, indicating that compared to Ψleaf, chemical signal, ABA showed a delayed response in face to mild soil drying. As soil further drying, ABA increased exponentially with Ψsoil decreasing from −1.01 to −1.44 MPa (Figure 2B). It should be noteworthy that ABA in the drought-stressed plants increased up to an average of 97.86 ng ml−1 at the end of experiment, resulting in an around 300 times higher than the well-watered treatment.
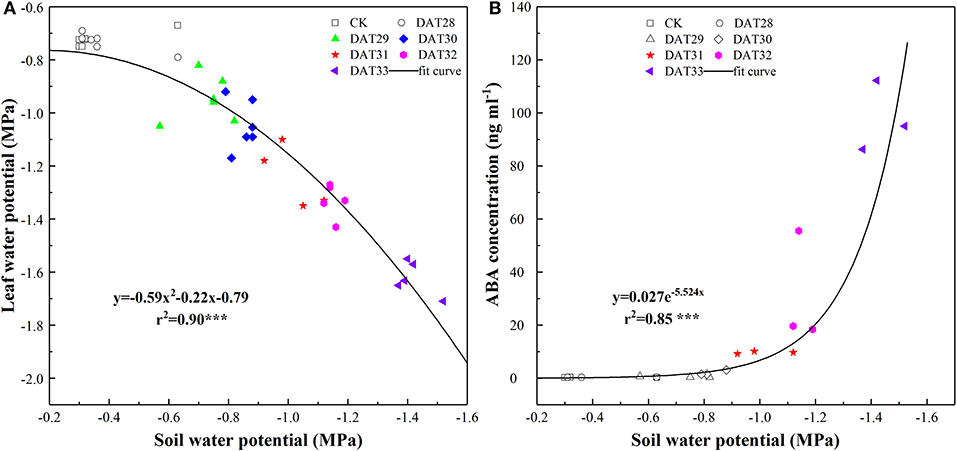
Figure 2. Leaf water potential (n = 6) (A) and shoot sap ABA concentration (n = 3) (B) in response to progressive soil water potential decrease. Colorful labels indicated significant difference at P < 0.001 level between well-watered and drought treatment.
Quantitative Analysis of Photosynthetic Limitation in Response to Soil Drying
The relative contributions of all limiting factors (ls, lm, lb) to photosynthetic capacity can be divided into three stages (Figure 3). Firstly, lb contributed to around an average of 51.46% limitation when Ψsoil was >-0.71 MPa, suggesting that photosynthetic biochemistry was the main factor under no water stressed condition. Secondly, with Ψsoil decreasing from −0.83 to −1.15 MPa, lb declined, whereas both ls and lm increased, but ls was higher than lm, which contributed solely to an almost 50.30% reduction in An, indicating that gs was the main limiting factor to photosynthetic capacity under mild and moderate drought. Thirdly, with Ψsoil decreasing to −1.44 MPa, lm contributed to 41.99% reduction in photosynthesis, followed by ls (36.93%) and lb (21.08%), showing that gm was the most important limiting factor to photosynthetic capacity under the severe drought condition.
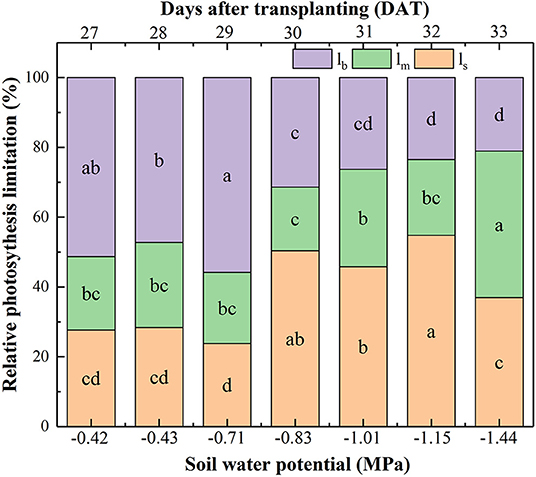
Figure 3. Effect of soil water potential (Ψsoil) on the relative contribution of the photosynthesis capacity limiting factors: limitations of An resulting from gs (ls), gm (lm), and biochemical photosynthetic capacity (lb) after transplanting. Data were means. Different letters indicated statistically significant difference between well-watered (CK) and drought plants at P < 0.05 level.
Ψleaf and ABA in the Regulation of gs, gm, gt, and An
As compared to gs in CK, gs in the water-stressed tomato seedlings increased firstly with Ψleaf decreasing from −0.72 to −0.95 MPa and then decreased with Ψleaf decreasing from −1.05 to −1.63 MPa (Figure 4A). However, gm kept unchanged within the range of Ψleaf from −0.72 to −1.05 MPa and decreased significantly when Ψleaf was <-1.28 MPa (Figure 4C). The output of ANOVA showed that drought had significant effect on the slopes of the regression lines between gs and gm to Ψleaf (Supplementary Figure 1). In addition, under mild and moderate drought, the ratio of gs reduction was higher than gm during 30–32 DAT (Supplementary Figure 2). These results indicated that gs was more sensitive to mild and moderate drought stress than gm. In summary, there was a significant positive relationship between Ψleaf and gs (r = 0.74, P < 0.01) and gm (r = 0.76, P < 0.01) during progressive soil drying (Table 1). We also investigated the relationship between ABA and gs or gm (Figures 4B,D). gs changed with no significant increasing ABA during 28–29 DAT. As soil further dried, gs continued decreasing and gm started to decrease with significant increase in ABA (Figures 4B,D). gm was closely related to gs during drying (r2=0.59, P < 0.01) (Figure 5). Notably, gm and ABA changed concurrently at the threshold of Ψsoil = −1.01 MPa (Figures 2B, 4D). In summary, ABA was negatively related to gm (r = −0.64, P < 0.01) and gs (r = −0.55, P < 0.01) (Table 1). These results indicated that the decline of gs was regulated by Ψleaf in the early stage of drought, whereas under moderate or severe drought, gs and gm were controlled by both Ψleaf and ABA.
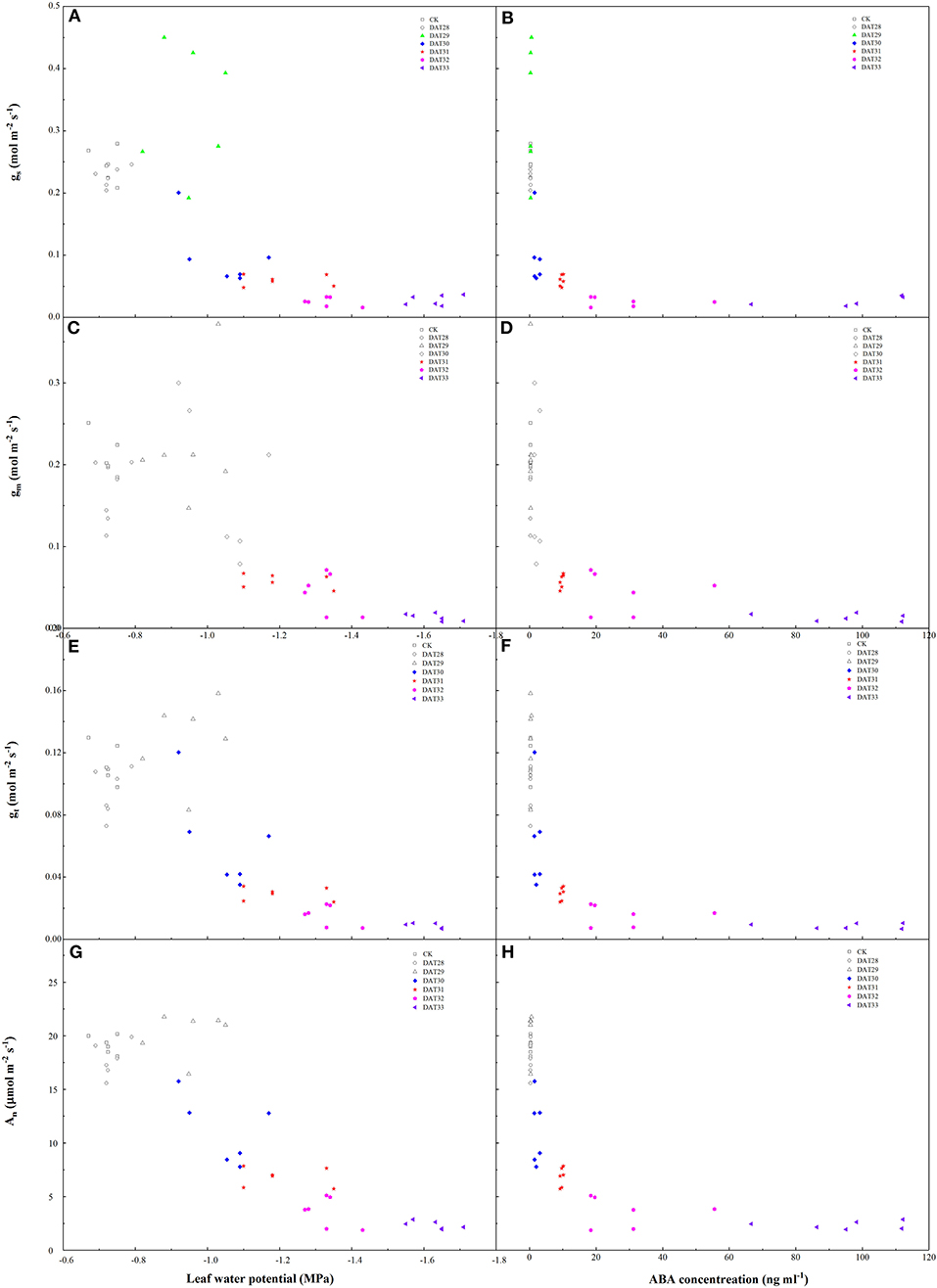
Figure 4. Effects of leaf water potential, ABA concentration on stomatal conductance (gs) (A,B), mesophyll conductance (gm) (C,D), total conductance (gt) (E,F), and net photosynthesis (An) (G,H). Colorful labels indicated significant difference between the well-watered (CK) and drought treatments at P < 0.01 level.
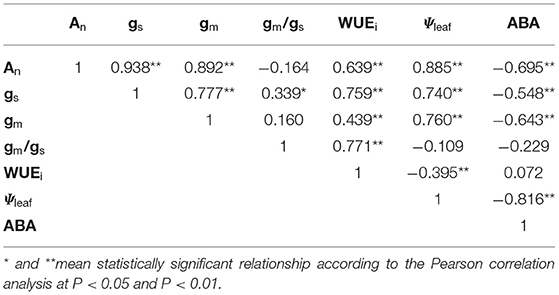
Table 1. Correlation matrix between studied parameters including intrinsic water use efficiency (WUEi), net photosynthesis (An), mesophyll conductance (gm), stomatal conductance (gs) and the ratio (gm/gs), abscisic acid (ABA), and leaf water potential (Ψleaf).
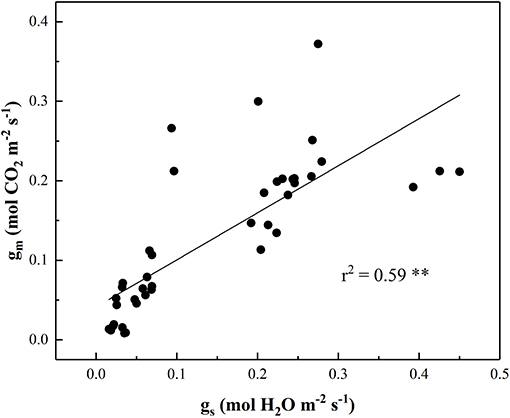
Figure 5. The relationship between the stomatal conductance to H2O (gs, mol H2O m−2 s−1) and mesophyll conductance to CO2 (gm, mol CO2 m−2 s−1) in the leaves under progressive drought. Data were fitted by a linear regression with r2 = 0.59 at P < 0.01 level.
Drought significantly affected An and gt during 30–33 DAT. When Ψleaf decreased to −1.05 MPa or ABA increased to 2.04 ng ml−1, An and gt declined by 40.18 and 45.13%, respectively (Figures 4E–H). As soil further dried, i.e., Ψleaf decreasing from −1.28 to −1.63 MPa, An and gt reduced by 62.84–88.94% and 74.33–92.92% in the drought-stressed plants as compared with the well-watered plants, respectively (Figures 4E,G).
gm/gs and WUEi in Response to Ψleaf and ABA Under Progressive Soil Drying
The dynamics of gm/gs in response to Ψleaf and ABA during progressive soil drying were presented in Figure 6. Higher gm/gs was observed as Ψleaf decreased from −1.05 to −1.33 MPa or as ABA increased from 2.04 to 31.23 ng ml−1 (Figures 6A,B), indicating that gs declined more than gm under mild or moderate drought. However, no significant difference of gm/gs between CK and the intense water stress with Ψleaf = −1.63 MPa was found. WUEi in response to these signals changed in the same way as gm/gs (Figures 6C,D), it increased firstly and then decreased. In addition, WUEi was positively related to gm/gs with a logarithmic relationship (r2 = 0.62, P < 0.001) during the progressive soil drying (Figure 6E), indicating that WUEi was strongly correlated to gm/gs.
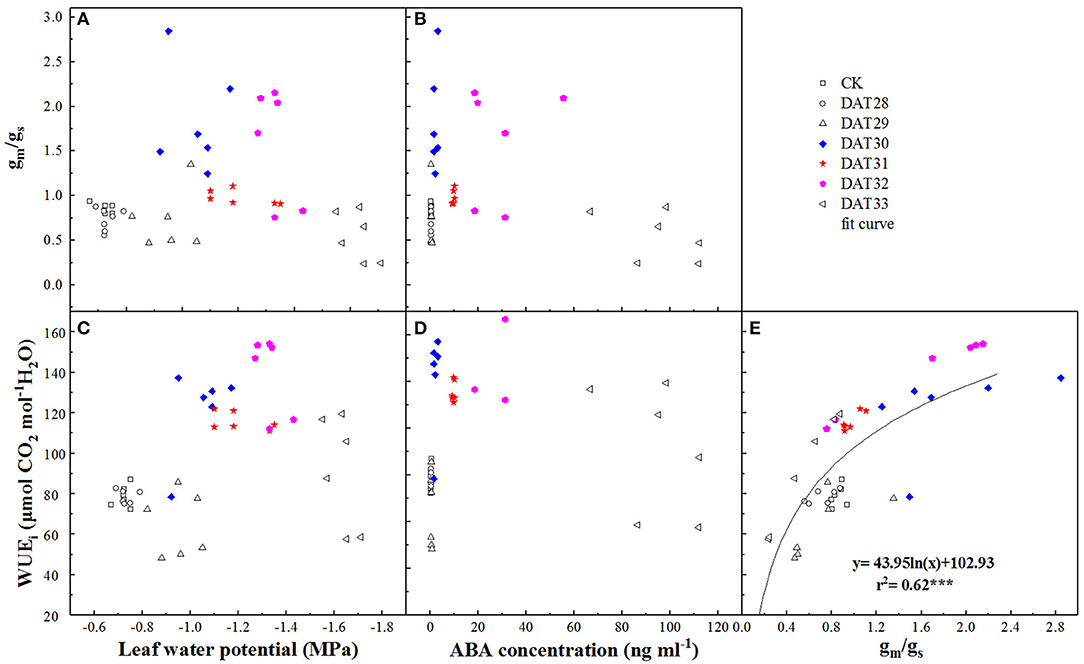
Figure 6. Correlation between ratio of mesophyll conductance to stomatal conductance (gm/gs) or intrinsic water use efficiency (WUEi) and leaf water potential (A,C) or shoot sap ABA (B,D) under progressive drought. The relationship between gm/gs and WUEi was presented with a non-linear regression at P < 0.001 level (E). Colorful labels indicated significant difference between the well-watered (CK) and drought treatments at P < 0.01 level.
Sensitivity Analyses of Parameters in the Estimation gm
10% variation of Rd and Jf did not affect gm significantly, whereas Γ* has a significantly effect on gm in well-watered plants (Table 2). As compared to gm in the well-watered plants, gm in the drought treatment was unaffected by the 20% underestimation of Jf, showing that gm in the drought treatment was less sensitive to Jf than in the well-watered plants. Variation of Ci resulted in an overestimation of gm in well-watered plants, whereas gm in drought treatment was unaffected by overestimation of Ci. These results indicated that overestimation of Ci had a slighter effect on calculation of gm than underestimation in the current study.
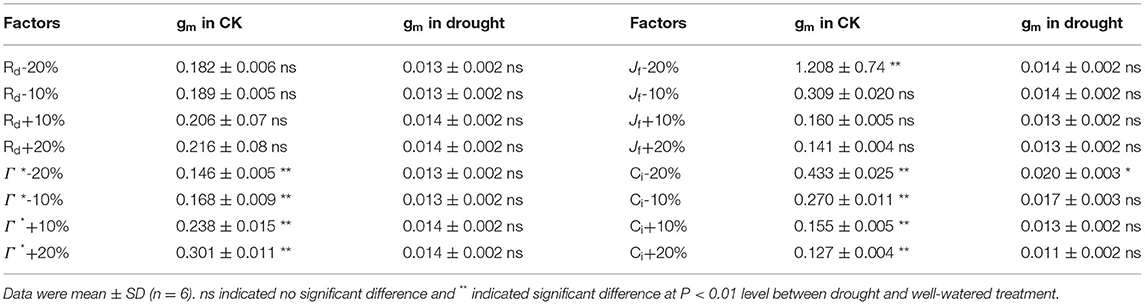
Table 2. Sensitivity analyses of the effects of ±20% error of light mitochondrial respiration (Rd), chloroplast CO2 compensation point (Γ*), electron transport rate (Jf), and intercellular CO2 concentration (Ci) on calculation of gm in well-watered and severe drought tomato at Ψsoil = −1.44 MPa as compared with the original value of gm.
Discussion
Effects of gs and gm on An Under Soil Drought
Efficient CO2 fixation is important for plant acclimation to environmental factors. In the present study, the total diffusion conductance of CO2 (gt) and An declined synchronously under drought (Figures 4E–H). The total diffusion conductance of CO2 mainly includes gs and gm (Grassi and Magnani, 2005). Many authors have reported that CO2 diffusion from sub-stomatal cavities to chloroplasts is a significant factor determining photosynthetic capacity in C3 plants such as tomato (Han et al., 2016; Du et al., 2019; Xu et al., 2019). Our analysis showed that ls and lm increased as soil drying proceeded and contributed to an almost 68–78% reduction in An when Ψsoil was <-0.83 MPa (Figure 3). Our results, as well as those of previous studies (Niinemets et al., 2009; Wang et al., 2018), confirmed the significance of gs and gm on assimilation rate under various drought conditions. It should be acknowledged that, many authors have highlighted the effects of leaf anatomical traits on gm, such as cell thickness, cell packing and area of chloroplasts exposed to the intercellular air spaces (Sc/S) across many species including tomato (Tomas et al., 2013; Muir et al., 2014). This effect was a result of plants acclimation to the long-term stressed environmental factors lasting for weeks. However, rapid response of gm to stress could occur within minutes response to elevating CO2 (Mizokami et al., 2018) or hours response to application of ABA (Sorrentino et al., 2016). Perhaps this meant that different mechanisms of gm determination existed under short and long term drought conditions. Therefore, to minimize the effects of leaf anatomy on gm, we focused on the responses of gm to drought stress and the involvement of ABA in a short water stress cycle.
Response of gs to Ψleaf and ABA Under Soil Drought
We found that gs generally decreased as Ψleaf decreased (Figures 2A, 4A), suggesting that Ψleaf might induce stomatal closure at the early stage of drought. The mechanisms of this hydraulic regulation remain unclear, but the reduction in Ψleaf has been tightly associated with decreasing leaf hydraulic signals (leaf turgor or Kleaf) in understanding the closure of stomata (Ripullone et al., 2007; Wang et al., 2018). On the one hand, evidences have suggested that decline of leaf turgor could explain the decrease in gs within no change of ABA (Rodriguez-Dominguez et al., 2016; Huber et al., 2019), possibly due to the decrease of elastic modulus and the activity of anion channel in guard cell during leaf dehydration (Ache et al., 2010; Saito and Terashima, 2010). On the other hand, progressive drop of plant water potential might decrease xylem pressure and increase the likelihood of embolism and hydraulic failure (Martorell et al., 2014; Tombesi et al., 2015). Responding to the future unpredictable soil water availability, stomata closed to prevent water loss and avoid xylem cavitation. Here, the increase of shoot sap ABA concentration was statistically insignificant, which implied that stomatal closure was not initiated by ABA with Ψsoil not approaching to −1.01 MPa (Figures 2B, 4B). Indeed, the delayed increase in leaf ABA in the present study was consistent with the recent findings that leaf ABA did not increase until after stomata closed, which was different from the actions of leaf turgor subjected to drought stress (Huber et al., 2019). However, as soil drought proceeded, gs continued decreasing with significant changes in both ABA and Ψleaf, suggesting that Ψleaf was not solely controlling gs, but chemical ABA was also involved in the reduction of gs. A similar variation between ABA and gs was also reported by Tombesi et al. (2015), who indicated that ABA played a crucial role in maintaining stomatal closure under long and severe drought. However, it should be noteworthy that our data need to be further interpreted, as shoot sap ABA was collected in the pressurized stem and leaf tissues instead of in localized guard cells.
Response of gm to Ψleaf and ABA Under Soil Drought
The variable J method (Harley et al., 1992), as the most commonly and easily accessible approach, was used to determine gm during the dry-down stage. To obtain precise calculation of gm, the highest possible accuracy of gas exchange and chlorophyll fluorescence were required during the process of measurement. As reported previously, the decrease in gm under drought was likely to associate with an overestimation of Ci due to stomatal closure (Pons et al., 2009). However, the sensitivity analyses showed that an overestimation of Ci did not induce gm decline in drought-stressed plants (Table 2). Thus, overestimation of Ci was unlikely to have a significant effect on gm in this study, might due to the influence of other environmental variations was ruled out under controlled environment. Therefore, it is reasonable to conclude that the reduction in gm during drought was mostly attributed to the decline of gm per se rather than the overestimation of Ci.
Compared to the response of gs, gm in the drought-stressed seedlings remained almost constant with Ψleaf not decrease to −1.28 MPa (Figures 4A,C), indicating that gm was less sensitive to the decrease in Ψleaf than gs at the beginning of soil drought. This result was in agreement with an earlier study conducted by Théroux-Rancourt et al. (2014), who found that gm only responded to more negative Ψleaf or more severe soil drought, e.g., Ψsoil < −1.01 MPa in the present study. Hydraulic compartmentalization of the mesophyll cell from the transpiration stream may account for this delayed response of gm to Ψleaf (Zwieniecki et al., 2007; Théroux-Rancourt et al., 2014). This delayed response of gm under the mild soil drought might be beneficial for mesophyll cells to be buffered against little variation in leaf water status and allow plants to maintain a greater An (Figure 4G).
However, as soil drought proceeded, gm declined as Ψleaf continued decreasing (Figures 4C,D). Based on literature surveys, the causes of this decrease in gm may be influenced by three main factors: mesophyll structure, membrane permeability, and biochemical enzymes activity (Flexas et al., 2008; Evans et al., 2009; Sorrentino et al., 2016). Mesophyll structural properties may not be involved in this rapid reduction of gm under the short-term drought. Instead, it is well-established that the Kleaf-induced reduction in gm was associated with the decrease in mesophyll density or membrane permeability under drought conditions (Aasamaa et al., 2005; Xiong et al., 2018). Water moves through leaf mesophyll tissues via apoplastic, symplastic and vapor phase pathways, which shared a part of pathways of CO2 diffusion (Xiong and Nadal, 2020). The decline in hydraulic conductance under drought usually leads to reductions in water supply to the leaves, therefore affecting mesophyll cells water relations and functions. Although the effect of Kleaf on gm was not investigated in this study, we observed a strong and positive relationship between Ψleaf and gm (r2 = 0.77, P < 0.01) (Figure 4C), because Kleaf was strongly influenced by Ψleaf under drought stress (Wang et al., 2018). Therefore, the decline in Ψleaf might contribute to this decrease in gm, as CO2 diffusion and liquid water shared partly common pathways within leaves (Xiong et al., 2018).
Most notably, rapid reduction of gm occurred following with increase of ABA when Ψsoil was below −1.01 MPa in the current study. Fast fluctuations in gm have also been recorded in response to ABA application (Sorrentino et al., 2016; Mizokami et al., 2018). The concurrent responses between gm and ABA with Ψsoil decreasing from −1.01 to −1.44 MPa was not a mere coincidence. This might suggest that Ψleaf was not the only factor influencing gs and gm under drought, other signals (ABA) could be involved in this reduction. Though mechanisms for the effect of ABA on gm remain unclear, the results from both Sorrentino et al. (2016) and the current studies indicated that the reduction in gm was most likely regulated by biochemical components due to the rapid reduction of gm to ABA (Flexas et al., 2008; Kaldenhoff et al., 2008; Xiong et al., 2018). Evidences have indicated two candidates are likely to play this biochemical role: carbonic anhydrase and aquaporins. CO2 molecules passing from sub-stomatal cavities to chloroplasts diffuse through the gas phase among intercellular air spaces and the liquid phase from the cell wall to stroma. Carbonic anhydrase (CA) plays a key role on the conversion of gaseous CO2 to aqueous carbonic acid (H2CO3) (Flexas et al., 2008). Higher ABA accumulation was likely to change the extracellular pH and decrease the activity of H+-ATP-ase, an important ion transporter in plant cell plasma membrane, thus affect the CA activity (Hayat et al., 2001; Sukhov et al., 2017). Aquaporins (AQPs) are pore-forming integral membrane proteins that transport of water, CO2 and other small neutral molecules across the plasma membrane (Flexas et al., 2006; Kaldenhoff, 2012). A higher abundance of AQPs increased the cellular CO2 uptake rates several folds. Expressions of plant AQPs could be influenced by drought stress and ABA (Kapilan et al., 2018). Additionally, an indirect role of ABA on decreasing Kleaf might also be involved in regulating gm, due to the ability of ABA on inactivation bundle sheath aquaporins such as the plasma membrane intrinsic proteins (PIPs) (Shatil-Cohen et al., 2011; Pantin et al., 2013). Based on these, we considered that the reduction in gm was not attributed solely to hydraulic regulation, ABA seemed to maintain the decrease in gm under moderate or severe soil drought, e.g., Ψsoil < −1.01 MPa in the present study. The regulation of gm is complex, and regulated by many factors, including hydraulic or chemical signaling and mesophyll structure. It is still unclear the mechanism of gm response to ABA under stress, further analysis of the expressions of carbonic anhydrase and cooporin protein in membrane may elucidate the biochemical mechanisms underlying this response. Notably, gs and gm decreased as ABA significantly increased (Figures 4B,D). Pooling all the data, a strong and positive relationship between both variables was observed in Table 1. In addition, 59% of the variation in gm can be explained by gs (Figure 5). Coupled changes between gs and gm was also found in response to drought (Perez-Martin et al., 2009; Han et al., 2016; Olsovska et al., 2016) or ABA application (Mizokami et al., 2018). Therefore, it seems that drought regulated gm in order to match the variation of gs, thereby optimization balance between CO2 uptake and water loss. However, the role of gs on regulating gm response to ABA is still debated by many scientists (Sorrentino et al., 2016; Mizokami et al., 2018), further detail investigations are needed to address this issue.
Variability of WUEi Under Drought Depends on gm/gs
In this study, gm/gs and WUEi increased concurrently with Ψsoil in the range of −0.83 to −1.15 MPa with a strong correlation (Figure 6E). Our results showed that WUEi was closely related to gm/gs compared to the correlation between WUEi and gm or gs (Table 1). This result was consistent with Han et al. (2016) who also found WUEi and gm/gs were closely correlated compared to the correlation between WUEi and gs or gm. These suggested that variations in WUEi were much more sensitive to changes of gm/gs. Stomata controls the water loss and mesophyll determines the photosynthesis, thus it would be better that using gm/gs instead of An/gs explained the variations of WUEi. Interestingly, this improvement of WUEi were coupled with increase in ABA. This might due to gs reduced more in response to ABA than gm under moderate drought. Though the mechanisms of ABA improving WUEi remain largely unknown, it is likely to be one of the most promising strategies to improve WUEi by means of decoding of the ABA signaling pathway or manipulating the expression of ABA-related genes on stomatal conductance or CA activity (Flexas et al., 2016; Cardoso et al., 2020). Nonetheless, such improvement of WUEi controlled by ABA could only be beneficial for maintaining water status under short-term drought during Ψsoil reduction from −0.83 to −1.15 MPa, not for long and serious drought (Figure 6D). This was beacuse the increase in WUEi at leaf scale may not always mean an improvement of WUE at the whole plant scale under serious soil drought, as the closure of stomata restricts CO2 uptake and hence diminish plant productivity (Xue et al., 2016).
Conclusion
The limitation of gs and gm increased along with progressive soil drying and diffusive conductance to CO2 from ambient air to chloroplasts was the crucial constraints to photosynthesis under drought conditions. The decrease in Ψleaf triggered stomata closure at the onset of drought. As soil drying proceeded, gs and gm declined synchronously. Both hydraulic and ABA signals were involved in this consistent decrease under moderate and severe drought. WUEi improved as gm/gs increased under mild and moderated drought due to a larger reduction of gs to ABA than gm. Manipulation of ABA levels might be a promising approach to improve plant water use efficiency for breeding project. For future research, examining the influence of stomatal closure on gm response to ABA will give further detailed insight on working of gm to ABA.
Data Availability Statement
The original contributions presented in the study are included in the article/Supplementary Material, further inquiries can be directed to the corresponding authors.
Author Contributions
AD and YG planned and designed the experiments. SL and JL performed the experiments and analyzed the data. SL wrote the draft manuscript. AD, YG, HL, and RQ revised the manuscript. All authors read and approved the final manuscript.
Funding
This work was supported by the National Natural Science Foundation of China (51790534, 51879267, 51779259), and the Agricultural Science and Technology Innovation Program (ASTIP), Chinese Academy of Agricultural Sciences.
Conflict of Interest
The authors declare that the research was conducted in the absence of any commercial or financial relationships that could be construed as a potential conflict of interest.
Supplementary Material
The Supplementary Material for this article can be found online at: https://www.frontiersin.org/articles/10.3389/fpls.2021.653186/full#supplementary-material
Supplementary Figure 1. Relationships between stomatal conductance (gs) or mesophyll conductance (gm) under drought during 30-33 DAT. Closed circles indicated gs, open circles indicated gm. Slope with P value indicates significant difference between the slopes of the regression lines for gs and gm to Ψleaf.
Supplementary Figure 2. The decreasing ratio of stomatal conductance (gs) or mesophyll conductance (gm) under drought compared CK during 30 to 33 DAT.
References
Aasamaa, K., Niinemets, U., and Sober, A. (2005). Leaf hydraulic conductance in relation to anatomical and functional traits during Populus tremula leaf ontogeny. Tree Physiol. 25, 1409–1418. doi: 10.1093/treephys/25.11.1409
Ache, P., Bauer, H., Kollist, H., Al-Rasheid, K. A., Lautner, S., Hartung, W., et al. (2010). Stomatal action directly feeds back on leaf turgor: new insights into the regulation of the plant water status from non-invasive pressure probe measurements. Plant J. 62, 1072–1082. doi: 10.1111/j.1365-313X.2010.04213.x
Assmann, S. M., and Jegla, T. (2016). Guard cell sensory systems: recent insights on stomatal responses to light, abscisic acid, and CO2. Curr. Opin. Plant Biol. 33, 157–167. doi: 10.1016/j.pbi.2016.07.003
Cano, F. J., SÁnchez-GÓmez, D., RodrÍguez-Calcerrada, J., Warren, C. R., Gil, L., and Aranda, I. (2013). Effects of drought on mesophyll conductance and photosynthetic limitations at different tree canopy layers. Plant Cell Environ. 36, 1961–1980. doi: 10.1111/pce.12103
Cardoso, A. A., Gori, A., Da-Silva, C. J., and Brunetti, C. (2020). Abscisic acid biosynthesis and signaling in plants: key targets to improve water use efficiency and drought tolerance. Appl. Sci. 10:6322. doi: 10.3390/app10186322
Dodd, I. C. (2005). Root-to-shoot signalling: assessing the roles of “up” in the up and down world of long-distance signalling in planta. Plant Soil 274, 251–270. doi: 10.1007/s11104-004-0966-0
Du, Q., Liu, T., Jiao, X., Song, X., Zhang, J., and Li, J. (2019). Leaf anatomical adaptations have central roles in photosynthetic acclimation to humidity. J. Exp. Bot. 70, 4949–4962. doi: 10.1093/jxb/erz238
Easlon, H. M., and Richards, J. H. (2009). Drought response in self-compatible species of tomato (Solanaceae). Am. J. Bot. 96, 605–611. doi: 10.3732/ajb.0800189
Evans, J. R., Kaldenhoff, R., Genty, B., and Terashima, I. (2009). Resistances along the CO2 diffusion pathway inside leaves. J. Exp. Bot. 60, 2235–2248. doi: 10.1093/jxb/erp117
Farquhar, G. C. S. V. B. J. A. (1980). A biochemical model of photosynthetic carbon di oxide assimilation in leaves of C3 carbon pathway species. Planta 149, 79–80. doi: 10.1007/BF00386231
Ferrio, J. P., Pou, A., Florez-Sarasa, I., Gessler, A., Kodama, N., Flexas, J., et al. (2012). The peclet effect on leaf water enrichment correlates with leaf hydraulic conductance and mesophyll conductance for CO(2). Plant Cell Environ. 35, 611–625. doi: 10.1111/j.1365-3040.2011.02440.x
Flexas, J., Bota, J., Escalona, J. M., Sampol, B., and Medrano, H. (2002). Effects of drought on photosynthesis in grapevines under field conditions: an evaluation of stomatal and mesophyll limitations. Funct. Plant Biol. 29, 461–471. doi: 10.1071/PP01119
Flexas, J., Diaz-Espejo, A., Conesa, M. A., Coopman, R. E., Douthe, C., Gago, J., et al. (2016). Mesophyll conductance to CO2 and Rubisco as targets for improving intrinsic water use efficiency in C3 plants. Plant Cell Environ. 39, 965–982. doi: 10.1111/pce.12622
Flexas, J., Niinemets, U., Galle, A., Barbour, M. M., Centritto, M., Diaz-Espejo, A., et al. (2013). Diffusional conductances to CO2 as a target for increasing photosynthesis and photosynthetic water-use efficiency. Photosyn. Res. 17, 45–59. doi: 10.1007/s11120-013-9844-z
Flexas, J., Ribas-Carbo, M., Diaz-Espejo, A., Galmes, J., and Medrano, H. (2008). Mesophyll conductance to CO2: current knowledge and future prospects. Plant Cell Environ. 31, 602–621. doi: 10.1111/j.1365-3040.2007.01757.x
Flexas, J., Ribas-Carbo, M., Hanson, D. T., Bota, J., Otto, B., Cifre, J., et al. (2006). Tobacco aquaporin NtAQP1 is involved in mesophyll conductance to CO2 in vivo. Plant J. 48, 427–439. doi: 10.1111/j.1365-313X.2006.02879.x
Grassi, G., and Magnani, F. (2005). Stomatal, mesophyll conductance, and biochemical limitations to photosynthesis as affected by drought and leaf ontogeny in ash and oak trees. Plant Cell Environ. 28, 834–849. doi: 10.1111/j.1365-3040.2005.01333.x
Han, J.-M., Meng, H.-F., Wang, S.-Y., Jiang, C.-D., Liu, F., Zhang, W.-F., et al. (2016). Variability of mesophyll conductance and its relationship with water use efficiency in cotton leaves under drought pretreatment. J. Plant Physiol. 194, 61–71. doi: 10.1016/j.jplph.2016.03.014
Harley, P. C., Loreto, F., Marco, G. D., and Sharkey, T. D. (1992). Theoretical considerations when estimating the mesophyll conductance to CO2 flux by analysis of the response of photosynthesis to CO2. Plant Physiol. 98, 1429–1436. doi: 10.1104/pp.98.4.1429
Hayat, S., Ahmad, A., Mobin, M., Fariduddin, Q., and Azam, Z. M. (2001). Carbonic anhydrase, photosynthesis, and seed yield in mustard plants treated with phytohormones. Photosynthetica 39, 111–114. doi: 10.1023/A:1012456205819
Hermida-Carrera, C., Kapralov, M. V., and Galmés, J. (2016). Rubisco catalytic properties and temperature response in crops. Plant Physiol. 171, 2549–2561. doi: 10.1104/pp.16.01846
Huber, A. E., Melcher, P. J., Piñeros, M. A., Setter, T. L., and Bauerle, T. L. (2019). Signal coordination before, during, and after stomatal closure in response to drought stress. New Phytol. 224, 675–688. doi: 10.1111/nph.16082
Kaldenhoff, R. (2012). Mechanisms underlying CO2 diffusion in leaves. Curr. Opin. Plant Biol. 15, 276–281. doi: 10.1016/j.pbi.2012.01.011
Kaldenhoff, R., Ribas-Carbo, M., Sans, J. F., Lovisolo, C., Heckwolf, M., and Uehlein, N. (2008). Aquaporins and plant water balance. Plant Cell Environ. 31, 658–666. doi: 10.1111/j.1365-3040.2008.01792.x
Kapilan, R., Vaziri, M., and Zwiazek, J. J. (2018). Regulation of aquaporins in plants under stress. Biol. Res. 51:4. doi: 10.1186/s40659-018-0152-0
Kim, J., Malladi, A., and Van Iersel, M. W. (2012). Physiological and molecular responses to drought in Petunia: the importance of stress severity. J. Exp. Bot. 63, 6335–6345. doi: 10.1093/jxb/ers285
Kudoyarova, G. R., Vysotskaya, L. B., Cherkozyanova, A., and Dodd, I. C. (2007). Effect of partial rootzone drying on the concentration of zeatin-type cytokinins in tomato (Solanum lycopersicum L.) xylem sap and leaves. J. Exp. Bot. 58, 161–168. doi: 10.1093/jxb/erl116
Laisk, A., and Loreto, F. (1996). Determining photosynthetic parameters from leaf CO2 exchange and chlorophyll fluorescence (ribulose-1,5-bisphosphate carboxylase/oxygenase specificity factor, dark respiration in the light, excitation distribution between photosystems, alternative electron transport rate, and mesophyll diffusion resistance. Plant Physiol. 110, 903–912. doi: 10.1104/pp.110.3.903
Li, S., Hamani, A. K. M., Si, Z., Liang, Y., Gao, Y., and Duan, A. (2020). Leaf gas exchange of tomato depends on abscisic acid and jasmonic acid in response to neighboring plants under different soil nitrogen regimes. Plants (Basel) 9:1674. doi: 10.3390/plants9121674
Liu, F., Andersen, M. N., Jacobsen, S.-E., and Jensen, C. R. (2005). Stomatal control and water use efficiency of soybean (Glycine max L. Merr.) during progressive soil drying. Environ. Exp. Bot. 54, 33–40. doi: 10.1016/j.envexpbot.2004.05.002
Long, S. P., and Bernacchi, C. J. (2003). Gas exchange measurements, what can they tell us about the underlying limitations to photosynthesis? Procedures and sources of error. J. Exp. Bot. 54, 2393–2401. doi: 10.1093/jxb/erg262
Martorell, S., Diaz-Espejo, A., Medrano, H., Ball, M. C., and Choat, B. (2014). Rapid hydraulic recovery in Eucalyptus pauciflora after drought: linkages between stem hydraulics and leaf gas exchange. Plant Cell Environ. 37, 617–626. doi: 10.1111/pce.12182
Mizokami, Y., Noguchi, K., Kojima, M., Sakakibara, H., and Terashima, I. (2018). Effects of instantaneous and growth CO2 levels and abscisic acid on stomatal and mesophyll conductances. Plant Cell Environ. 42, 1257–1269. doi: 10.1111/pce.13484
Mizokami, Y., Noguchi, K. O., Kojima, M., Sakakibara, H., and Terashima, I. (2015). Mesophyll conductance decreases in the wild type but not in an ABA-deficient mutant (aba1) ofNicotiana plumbaginifoliaunder drought conditions. Plant Cell Environ. 38, 388–398. doi: 10.1111/pce.12394
Muir, C. D., Hangarter, R. P., Moyle, L. C., and Davis, P. A. (2014). Morphological and anatomical determinants of mesophyll conductance in wild relatives of tomato (Solanum sect. Lycopersicon, sect. Lycopersicoides; Solanaceae). Plant Cell Environ. 37, 1415–1426. doi: 10.1111/pce.12245
Niinemets, U., Diaz-Espejo, A., Flexas, J., Galmes, J., and Warren, C. R. (2009). Importance of mesophyll diffusion conductance in estimation of plant photosynthesis in the field. J. Exp. Bot. 60, 2271–2282. doi: 10.1093/jxb/erp063
Olsovska, K., Kovar, M., Brestic, M., Zivcak, M., Slamka, P., and Shao, H. B. (2016). Genotypically identifying wheat mesophyll conductance regulation under progressive drought stress. Front. Plant Sci. 7:1111. doi: 10.3389/fpls.2016.01111
Pantin, F., Monnet, F., Jannaud, D., Costa, J. M., Renaud, J., Muller, B., et al. (2013). The dual effect of abscisic acid on stomata. New Phytol. 197, 65–72. doi: 10.1111/nph.12013
Perez-Martin, A., Flexas, J., Ribas-Carbo, M., Bota, J., Tomas, M., Infante, J. M., et al. (2009). Interactive effects of soil water deficit and air vapour pressure deficit on mesophyll conductance to CO2 in Vitis vinifera and Olea europaea. J. Exp. Bot. 60, 2391–2405. doi: 10.1093/jxb/erp145
Pons, T. L., Flexas, J., Von Caemmerer, S., Evans, J. R., Genty, B., Ribas-Carbo, M., et al. (2009). Estimating mesophyll conductance to CO2: methodology, potential errors, and recommendations. J. Exp. Bot. 60, 2217–2234. doi: 10.1093/jxb/erp081
Qiu, R., Liu, C., Li, F., Wang, Z., Yang, Z., and Cui, N. (2019). An investigation on possible effect of leaching fractions physiological responses of hot pepper plants to irrigation water salinity. BMC Plant Biol. 19:297. doi: 10.1186/s12870-019-1910-z
Ripullone, F., Guerrieri, M. R., Nole', A., Magnani, F., and Borghetti, M. (2007). Stomatal conductance and leaf water potential responses to hydraulic conductance variation in Pinus pinaster seedlings. Trees 21, 371–378. doi: 10.1007/s00468-007-0130-6
Rodriguez-Dominguez, C. M., Buckley, T. N., Egea, G., De Cires, A., Hernandez-Santana, V., Martorell, S., et al. (2016). Most stomatal closure in woody species under moderate drought can be explained by stomatal responses to leaf turgor. Plant Cell Environ. 39, 2014–2026. doi: 10.1111/pce.12774
Saito, T., and Terashima, I. (2010). Reversible decreases in the bulk elastic modulus of mature leaves of deciduous Quercus species subjected to two drought treatments. Plant Cell Environ. 27, 863–875. doi: 10.1111/j.1365-3040.2004.01192.x
Sharkey, T. D., Bernacchi, C. J., Farquhar, G. D., and Singsaas, E. L. (2007). Fitting photosynthetic carbon dioxide response curves for C3leaves. Plant Cell Environ. 30, 1035–1040. doi: 10.1111/j.1365-3040.2007.01710.x
Shatil-Cohen, A., Attia, Z., and Moshelion, M. (2011). Bundle-sheath cell regulation of xylem-mesophyll water transport via aquaporins under drought stress: a target of xylem-borne ABA? Plant J. 67, 72–80. doi: 10.1111/j.1365-313X.2011.04576.x
Sorrentino, G., Haworth, M., Wahbi, S., Mahmood, T., Zuomin, S., and Centritto, M. (2016). Abscisic acid induces rapid reductions in mesophyll conductance to carbon dioxide. PLoS ONE 11:e0148554. doi: 10.1371/journal.pone.0148554
Sukhov, V. S., Gaspirovich, V. V., Gromova, E. N., Ladeynova, M. M., Sinitsyna, Y. V., Berezina, E. V., et al. (2017). Decrease of mesophyll conductance to CO2 is a possible mechanism of abscisic acid influence on photosynthesis in seedlings of pea and wheat. Biochem. (Moscow) Suppl. Ser. A 11, 237–247. doi: 10.1134/S1990747817030096
Tardieu, F. (1998). Variability among species of stomatal control under fluctuating soil water status and evaporative demand: modelling isohydric and anisohydric behaviours. J. Exp. Bot. 49, 419–432. doi: 10.1093/jxb/49.Special_Issue.419
Théroux-Rancourt, G., Éthier, G., and Pepin, S. (2014). Threshold response of mesophyll CO2conductance to leaf hydraulics in highly transpiring hybrid poplar clones exposed to soil drying. J. Exp. Bot. 65, 741–753. doi: 10.1093/jxb/ert436
Tomas, M., Flexas, J., Copolovici, L., Galmes, J., Hallik, L., Medrano, H., et al. (2013). Importance of leaf anatomy in determining mesophyll diffusion conductance to CO2 across species: quantitative limitations and scaling up by models. J. Exp. Bot. 64, 2269–2281. doi: 10.1093/jxb/ert086
Tombesi, S., Nardini, A., Frioni, T., Soccolini, M., Zadra, C., Farinelli, D., et al. (2015). Stomatal closure is induced by hydraulic signals and maintained by ABA in drought-stressed grapevine. Sci. Rep. 5:12449. doi: 10.1038/srep12449
Tosens, T., Nishida, K., Gago, J., Coopman, R. E., Cabrera, H. M., Carriqui, M., et al. (2016). The photosynthetic capacity in 35 ferns and fern allies: mesophyll CO2 diffusion as a key trait. New Phytol. 209, 1576–1590. doi: 10.1111/nph.13719
Vrabl, D., Vaskova, M., Hronkova, M., Flexas, J., and Santrucek, J. (2009). Mesophyll conductance to CO(2) transport estimated by two independent methods: effect of variable CO(2) concentration and abscisic acid. J. Exp. Bot. 60, 2315–2323. doi: 10.1093/jxb/erp115
Wang, X., Du, T., Huang, J., Peng, S., and Xiong, D. (2018). Leaf hydraulic vulnerability triggers the decline in stomatal and mesophyll conductance during drought in rice. J. Exp. Bot. 69, 4033–4045. doi: 10.1093/jxb/ery188
Wilkinson, S., and Davies, W. J. (2002). ABA-based chemical signalling: the co-ordination of responses to stress in plants. Plant Cell Environ. 25, 195–210. doi: 10.1046/j.0016-8025.2001.00824.x
Xiong, D., Douthe, C., and Flexas, J. (2018). Differential coordination of stomatal conductance, mesophyll conductance, and leaf hydraulic conductance in response to changing light across species. Plant Cell Environ. 41, 436–450. doi: 10.1111/pce.13111
Xiong, D., and Nadal, M. (2020). Linking water relations and hydraulics with photosynthesis. Plant J. 101, 800–815. doi: 10.1111/tpj.14595
Xu, Y., Feng, Z., Shang, B., Dai, L., Uddling, J., and Tarvainen, L. (2019). Mesophyll conductance limitation of photosynthesis in poplar under elevated ozone. Sci. Total Environ. 657, 136–145. doi: 10.1016/j.scitotenv.2018.11.466
Xue, W., Nay-Htoon, B., Lindner, S., Dubbert, M., Otieno, D., Ko, J., et al. (2016). Soil water availability and capacity of nitrogen accumulation influence variations of intrinsic water use efficiency in rice. J. Plant Physiol. 193, 26–36. doi: 10.1016/j.jplph.2016.02.003
Yan, F., Li, X., and Liu, F. (2017). ABA signaling and stomatal control in tomato plants exposure to progressive soil drying under ambient and elevated atmospheric CO2 concentration. Environ. Exp. Bot. 139, 99–104. doi: 10.1016/j.envexpbot.2017.04.008
Keywords: drought, leaf water potential, abscisic acid, stomatal conductance, mesophyll conductance, intrinsic water use efficiency
Citation: Li S, Liu J, Liu H, Qiu R, Gao Y and Duan A (2021) Role of Hydraulic Signal and ABA in Decrease of Leaf Stomatal and Mesophyll Conductance in Soil Drought-Stressed Tomato. Front. Plant Sci. 12:653186. doi: 10.3389/fpls.2021.653186
Received: 14 January 2021; Accepted: 30 March 2021;
Published: 29 April 2021.
Edited by:
Iker Aranjuelo, Superior Council of Scientific Investigations, SpainReviewed by:
Ismael Aranda, Instituto Nacional de Investigación y Tecnología Agroalimentaria (INIA), SpainAntonio Diaz Espejo, Institute of Natural Resources and Agrobiology of Seville (CSIC), Spain
Copyright © 2021 Li, Liu, Liu, Qiu, Gao and Duan. This is an open-access article distributed under the terms of the Creative Commons Attribution License (CC BY). The use, distribution or reproduction in other forums is permitted, provided the original author(s) and the copyright owner(s) are credited and that the original publication in this journal is cited, in accordance with accepted academic practice. No use, distribution or reproduction is permitted which does not comply with these terms.
*Correspondence: Yang Gao, Z2FveWFuZ0BjYWFzLmNu; Aiwang Duan, ZHVhbmFpd2FuZ0BjYWFzLmNu