- 1Key Laboratory of Agricultural Soil and Water Engineering of Liaoning Province, College of Water Conservancy, Shenyang Agricultural University, Shenyang, China
- 2The UWA Institute of Agriculture, The University of Western Australia, Perth, WA, Australia
- 3School of Agriculture and Environment, The University of Western Australia, Perth, WA, Australia
The effect of biochar application on photosynthetic traits and yield in peanut (Arachis hypogaea L.) is not well understood. A 2-year field experiment was conducted in Northwest Liaoning, China to evaluate the effect of biochar application [0, 10, 20, and 40 t ha−1 (B0, B10, B20, and B40)] on leaf gas exchange parameters, chlorophyll fluorescence parameters, and yield of peanut. B10 improved photochemical quenching at flowering and pod set and reduced non-photochemical quenching at pod set, relative to B0. B10 and B20 increased actual photochemical efficiency and decreased regulated energy dissipated at pod set, relative to B0. B10 significantly increased net photosynthetic rate, transpiration rate, stomatal conductance, and water use efficiency at flowering and pod set, relative to B0. Compared with B0, B10 significantly improved peanut yield (14.6 and 13.7%) and kernel yield (20.2 and 14.4%). Biochar application increased leaf nitrogen content. B10 and B20 significantly increased plant nitrogen accumulation, as compared to B0. The net photosynthetic rate of peanut leaves had a linear correlation with plant nitrogen accumulation and peanut yield. The application of 10 t ha−1 biochar produced the highest peanut yield by enhancing leaf photosynthetic capacity, and is thus a promising strategy for peanut production in Northwest Liaoning, China.
Introduction
Peanut (Arachis hypogaea L.) is an annual legume crop. Global peanut consumption is increasing at a rate of around 3% per annum. China produces 40% of the world’s peanuts (FAOSTAT, 2018). Liaoning Province, is one of the main areas for peanut production in China and the primary export base of high-quality peanut. The Northwest Liaoning is a competitive producing area for peanut with a typical characteristic of sand and wind in semi-arid regions of Northeast China. However, peanut production in this area is limited by poor soil water and nutrient holding capacities, and water deficiency (Bai et al., 2014). Hence, the incorporation of plastic film mulching and supplemental irrigation have been studied as an extremely effective strategy with potential for decreasing soil evaporation, and enhancing crop growth, yield, and water use efficiency (Li and Gong, 2002; Ali et al., 2018; Xia et al., 2021a). However, the enhanced productivity under plastic mulches has been reported to result in lower soil fertility, which limit the subsequent crop productivity (Li et al., 2007; Steinmetz et al., 2016).
Biochar is produced through pyrolysis of biomass under limited oxygen environment (Lehmann et al., 2011). Generally, biochar with larger specific surface area, pore structure, abundant surface functional groups, and nutrient characteristics (e.g., C, N, P, K, S, Ca, and Mg) could improve soil sustainability (Ippolito et al., 2020; Leng et al., 2020; Ye et al., 2020). Most studies have shown that biochar is an effective agricultural practice for improving water and soil conditions in farmland and increasing crop yield and fertilizer use efficiency due to its unique structure (Clough et al., 2013; Laghari et al., 2015; Haider et al., 2017; Lin et al., 2017). The porous physical structure of biochar induces a sorption capacity to inorganic nitrogen and can potentially allow the slow release of nutrients to improve plant growth (Novak et al., 2012; El-Naggar et al., 2019). Biochar impacts the soil nitrogen, and is expected to enhance leaf nitrogen and photosynthesis (Kammann et al., 2011; Ali et al., 2020). Biochar addition to soil has positive effect on photosynthesis, being an important process that affects crop yield. When biochar application improves nitrogen accumulation, it also helps to increase leaf nitrogen content and therefore increases photosynthesis (Nguyen et al., 2017; Ali et al., 2020). The photosynthetic rate was increased at 40 t ha−1 biochar addition, and this enhancement in the leaf photosynthetic rate was due to the increased nitrogen accumulation (Ali et al., 2020). Biochar amendment increased the effective photochemical quantum yield of PSII and decreased the fluorescence yield for heat dissipation, therefore improved Pn (Abideen et al., 2020).
Most studies on peanut photosynthesis have focused on photosynthetic rate changes at different growth stages (Xu et al., 2015; Sun et al., 2018; Liu et al., 2019). However, limited information is available on the biochar effect on chlorophyll fluorescence and gas exchange parameters of peanut, especially in situ in the field. Aims of our study were to evaluate the effects of biochar application on photosynthesis from the perspective of chlorophyll fluorescence parameters, leaf nitrogen content, and plant nitrogen accumulation. We hypothesized that: (1) biochar application would improve peanut yield via enhancing leaf photosynthetic traits; (2) biochar improves the photosynthetic rate due to increasing the proportion of open photosystem II reaction centers, and nitrogen accumulation at low application rates.
Materials and Methods
Experimental Sites and Materials
The field experiment was carried out at the Scientific Observation Experimental Station in Fuxin (42.11° N, 121.65° E), Liaoning Province, China, during the 2018 and 2019 growing seasons (May to October). This area with typical sand and wind conditions of semi-arid regions in Northeast China, has cold, dry winters and hot summers according to the Köppen-Geiger climate classification (Peel et al., 2007). Average annual rainfall is about 400 mm (60% from June to August), with average annual evaporation greater than 1,800 mm. Droughts are frequent. The daily weather data during the 2018 and 2019 peanut growing seasons are shown in Figure 1. The soil texture was sandy loam with pH 5.96, 1.44 g cm–3 bulk density, 19.5% (w/w) field capacity (FC), 0.62 g kg−1 total nitrogen, 142 mg kg−1 available potassium, and 18.1 mg kg−1 available phosphorus. The biochar was derived from maize straw pyrolyzed at 600°C with pH 8.14, 18.9%, carbon content, 0.58% nitrogen content, 4.76 g kg−1 available potassium, and 0.33 g kg−1 available phosphorus.
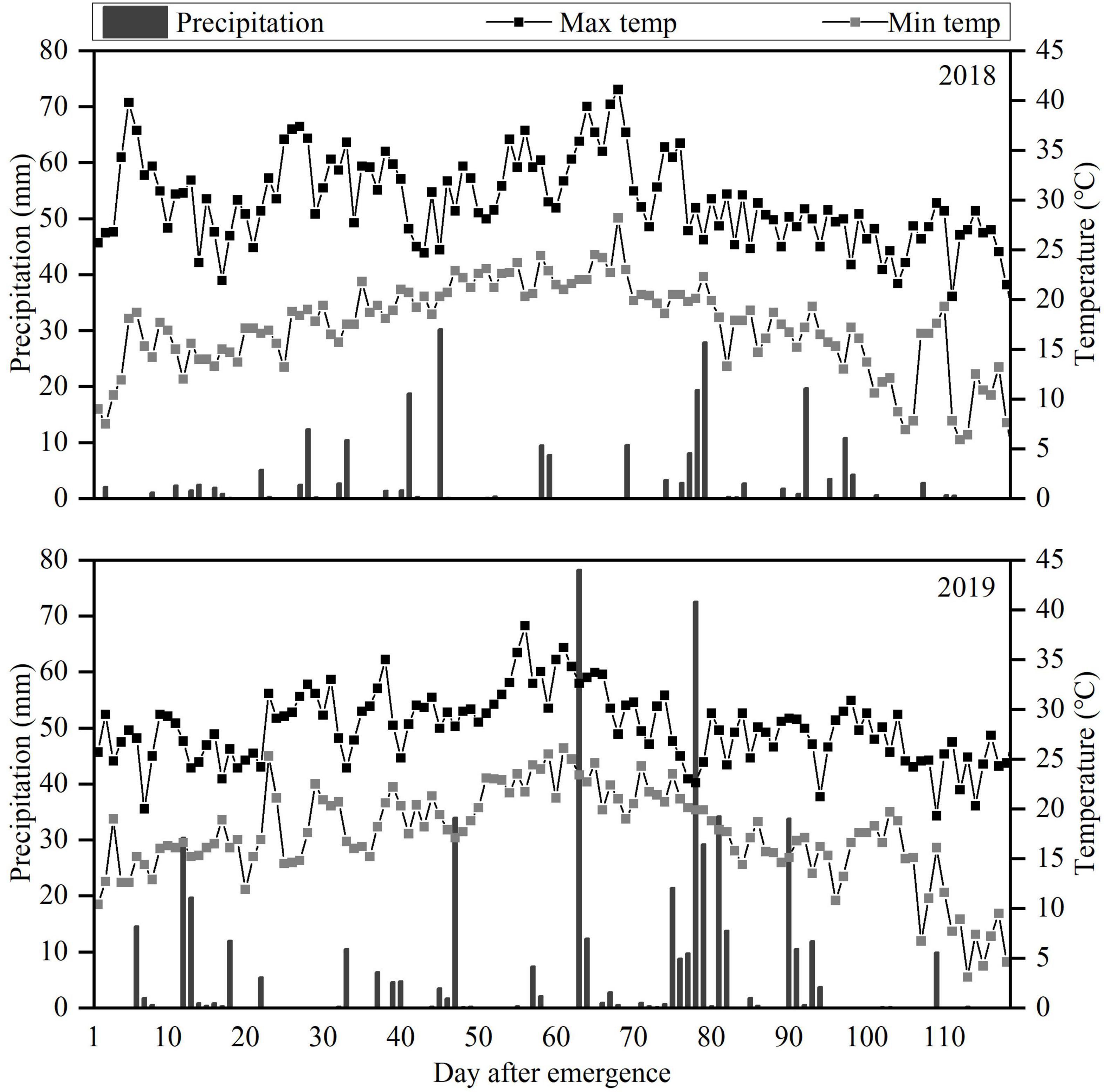
Figure 1. Daily precipitation and maximum (Max) and minimum (Min) temperatures during the 2018 and 2019 peanut growing seasons.
Experimental Design, Establishment and Maintenance
The experiment was a randomized complete block design comprising four biochar application rates (0, 10, 20, and 40 t ha−1; B0, B10, B20, and B40) and three replicates (plots). Supplemental irrigation via a plastic mulched drip system was applied during the flowering and pod setting stages when peanut growth is more sensitive to water deficit than other stages. The field was irrigated up to 90% FC when the soil moisture content dropped to ≤55% FC. The biochar was fully mixed with the upper 0–20 cm soil layer by rotary before sowing in 2018. No additional biochar was applied in the second year. Basal fertilizers were applied at the rate of 50 kg ha−1 N, 170 kg ha−1 P2O5, and 156 kg ha−1 K2O.
Peanut cultivar Baisha 1016 origing in Guangdong Province was sown on 16 May 2018 and 19 May 2019 and harvested on 21 September 2018 and 23 September 2019. A trapezoidal ridge with a width of 0.7 m was formed by plough. Two rows were sown on the ridge of each hill (167,000 hills ha−1). The ridges were covered with white plastic film (0.008 mm thick) immediately after sowing. Each plot was 1 × 7.5 m2. Groundwater was used, with the irrigation amount determined by monitoring the volumetric water meter equipped in each plot. Other field management, including weeds, insects, and diseases control, were in line with local farmer practices.
Sampling and Measurements
Chlorophyll Fluorescence Parameters
Chlorophyll fluorescence parameters of peanut were measured using the LI-6800 (LI-COR, Lincoln, NE, United States) photosynthesis measurement system with multiphase flash fluorescence (6800–01) at flowering (19 July 2018 and 16 July 2019) and pod set (8 August 2018 and 9 August 2019) on clear and cloudless days. To avoid influence of the changes in CO2 concentration in the air, the CO2 inlet of the instrument was connected to a CO2 cartridge (400 μmol mol−1). The third fully expanded leaf on the main stem were wrapped in aluminum foil. After remaining in complete darkness overnight, we measured minimal fluorescence yield (Fo) using a measuring light (0.005 μmol m–2 s−1). Maximal fluorescence yield (Fm) was measured using a 1 s saturating pulse at 8,000 μmol m–2 s−1 in dark-adapted leaves. The leaves were continuously illuminated for 20 min with an actinic light (1,400 μmol m–2 s−1) to record the steady-state yield of fluorescence (Fs). Maximal light-adapted fluorescence yield (Fm’) was determined by 8,000 μmol m–2 s−1. The actinic light was turned off, and minimal fluorescence yield (Fo’) in light-adapted state was determined after 5 s of far-red illumination. The difference between the measured values of Fm and Fo is the variable fluorescence (Fv). The chlorophyll fluorescence parameters were calculated using the following formulas (Kooten and Snel, 1990; Maxwell and Johnson, 2000; Kramer et al., 2004):
where Fv/Fm is maximal photochemical efficiency of photosystem II (PSII), ΦPSII is actual photochemical efficiency of PSII, ΦNPQ is quantum yield for energy dissipated via Δ pH and xanthophyll-regulated processes, ΦNO is quantum yield of non-regulated energy dissipated in PSII, and qP and NPQ are photochemical and non-photochemical quenching, respectively.
Gas Exchange Parameters, Leaf Nitrogen Content and Plant Nitrogen Accumulation
Gas exchange parameters were measured on the same dates and same leaves as those for chlorophyll fluorescence parameters measurements. Net photosynthesis rate (Pn), transpiration rate (Tr), stomatal conductance (Gs), intercellular CO2 concentration (Ci), and ambient CO2 concentration (Ca) were measured with LI-6800 (LI-COR, Lincoln, NE, United States) photosynthesis measurement system. The stomatal limitation value (Ls) was calculated as 1–Ci/Ca, and WUE was calculated as Pn/Tr (Fang et al., 2018).
After the determination of gas exchange parameters, the third fully expanded leaf on the main stem of 20 plants in each pot was collected. Plant samples were collected at flowering and pod set, and were separated into various parts: roots, stems, leaves, and pods. All the samples were oven-dried at 105°C for 30 min and then at 80°C to constant weight. After weighing, these samples were ground into powder for measuring nitrogen content. The full-automatic KjelFlex K-360 analyzer (BUCHIK, Switzerland) was used to determine nitrogen content. Plant nitrogen accumulation was calculated by multiplying total nitrogen concentration in roots, stems, leaves, and pods with respective dry matter at flowering and pod set stages.
Yield and Yield Components
Peanuts were harvested from 1 m2 in the center of each plot. The pods were air-dried for about 1 week before being measured for peanut yield, kernel yield, 100-pod weight, and 100-kernel weight (Tan et al., 2018). The shelling percentage was calculated as (kernel weight/pod weight) × 100% (Luo et al., 2017).
Statistical Analysis
SPSS 19.0 statistic software (SPSS Inc., Chicago, IL, United States) was used to perform the statistical analysis. Year and biochar application were assumed to be fixed factor and the replicates were assumed to be random factors. Error bars in the figures represent standard errors of the mean. Least significant differences were used to separate treatment means at the 5% probability level. Regression analysis was used to evaluate the relationships between leaf nitrogen content and net photosynthetic rate, net photosynthetic rate and peanut yield. The responses of chlorophyll fluorescence parameters, gas exchange parameters, leaf nitrogen content, yield, and yield components to biochar application were further analyzed with the principal component analysis in R studio version 1.1.442 using the Factoextra package (Kassambara, 2015).
Results
Chlorophyll Fluorescence Parameters
Year, biochar application, and Y × B interaction had no significant effects on Fv/Fm at flowering or pod set (Table 1 and Figures 2A,G). Biochar application had a significant effect on ΦPSII at flowering and pod set, but there were no significant differences for year or Y × B interaction (Table 1). B10 increased ΦPSII at flowering by 7.1 in 2018 and 8.8% in 2019, relative to B0 (Figure 2B). At pod set, B10 increased ΦPSII by 13.0 in 2018 and 14.9% in 2019, and B20 increased ΦPSII by 13.0 in 2018 and 12.8% in 2019, relative to B0 (Figure 2H). Among the four biochar treatments, B10 had the highest ΦPSII values at flowering and pod set each year.
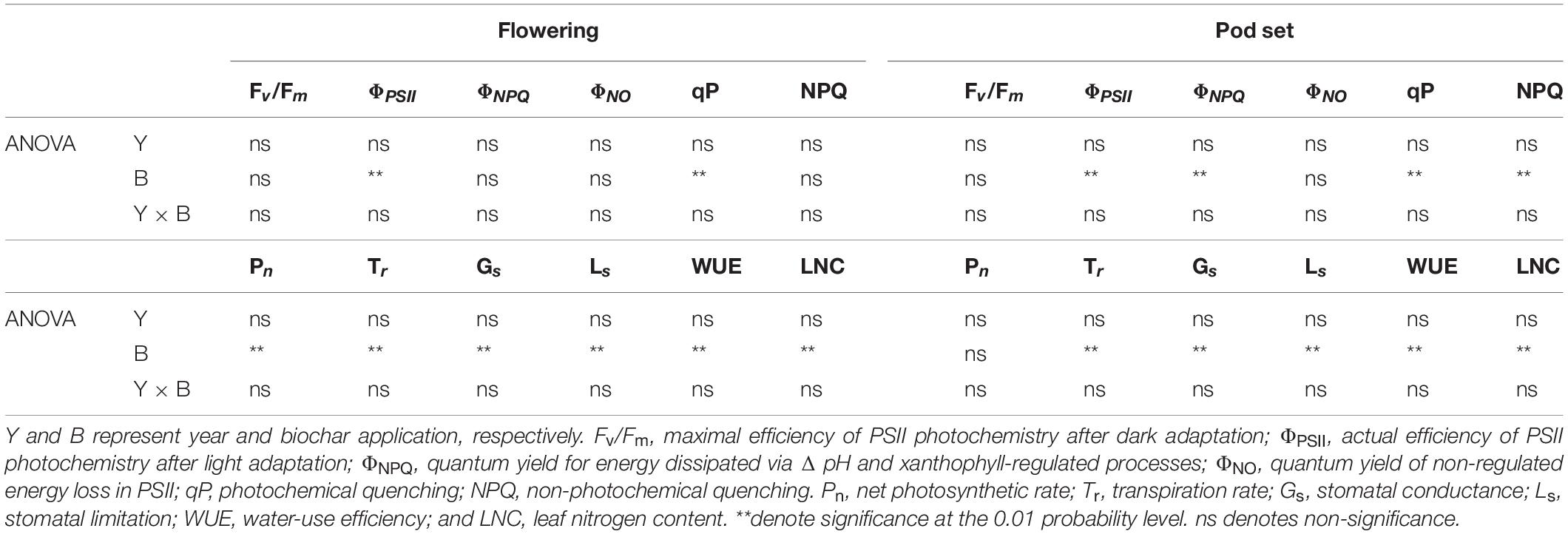
Table 1. Leaf chlorophyll fluorescence parameters and gas exchange parameters at the flowering and pod set in peanut with four rates of biochar in the 2018 and 2019 growing seasons.
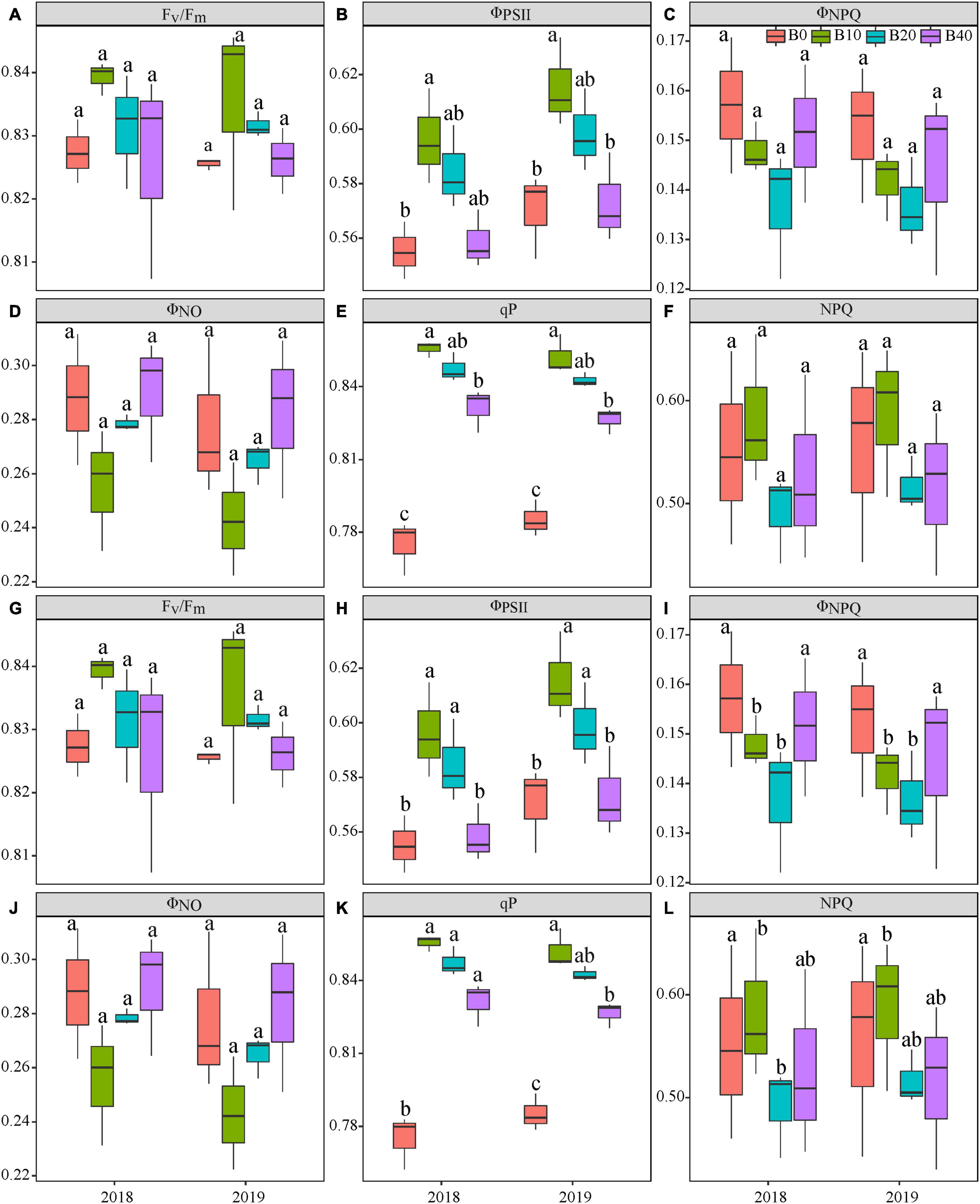
Figure 2. Chlorophyll fluorescence parameters at the flowering (A–F) and pod set (G–L) in peanut with four rates of biochar in the 2018 and 2019 growing seasons. Fv/Fm, maximal efficiency of PSII photochemistry after dark adaptation; ΦPSII, actual efficiency of PSII photochemistry after light adaptation; ΦNPQ, quantum yield for energy dissipated via Δ pH and xanthophyll-regulated processes; ΦNO, quantum yield of non-regulated energy loss in PSII; qP, photochemical quenching; and NPQ, non-photochemical quenching. B0, B10, B20, and B40 represent biochar application rates at 0, 10, 20, and 40 t ha−1, respectively. For each parameter in each year, mean data with different letters denote significant difference among treatments at P < 0.05.
Biochar application had a significant effect on ΦNPQ at pod set but not at flowering (Table 1 and Figures 2C,I). Year and Y × B interaction had no significant effect on ΦNPQ at flowering or pod set. There were no significant effects of biochar application, year, or Y × B interaction on ΦNO at flowering or pod set (Table 1 and Figures 2D,J). At pod set, ΦNPQ decreased with increasing biochar application rate to B10 and then increased. B10 and B20 decreased ΦNPQ by 30.0 and 26.7% in 2018, and 27.6 and 24.1% in 2019, respectively, as compared to B0.
Biochar application had a significant effect on qP at flowering and pod set, but there were no significant differences for year or Y × B interaction (Table 1). At flowering, B10 enhanced qP by 11.7 and 7.6% in 2018 and 2019, respectively, relative to B0 (Figure 2E). At pod set, B10, B20, and B40 enhanced qP by 8.7, 7.2, and 5.8% in 2018, respectively, as compared to B0, but there were no significant differences between these treatments (Figure 2K). In 2019, B10 enhanced qP by 10.3%, relative to B0. Biochar application had a significant effect on NPQ at pod set but not at flowering. No significant differences were observed for year or Y × B interaction of NPQ at flowering or pod set (Table 1 and Figure 2F). At pod set, B10 and B20 decreased NPQ by up to 31.1% in 2018 and B10 decreased NPQ by 27.4% in 2019, as compared to B0 (Figure 2L).
Gas Exchange Parameters and Leaf Nitrogen Content
Biochar application had a significant effect on Pn at flowering and pod set, but there were no significant effects for year or Y × B interaction (Table 1). B10 increased Pn at flowering by 16.1% in 2018, relative to B0 (Figure 3A). B10 and B20 increased Pn at flowering by up to 16.7% in 2019, as compared to B0. At pod set, B10 increased Pn by 19.6% in 2018 and 25.8% in 2019, relative to B0 (Figure 3G). B10 had the highest Pn at flowering and pod set in both years.
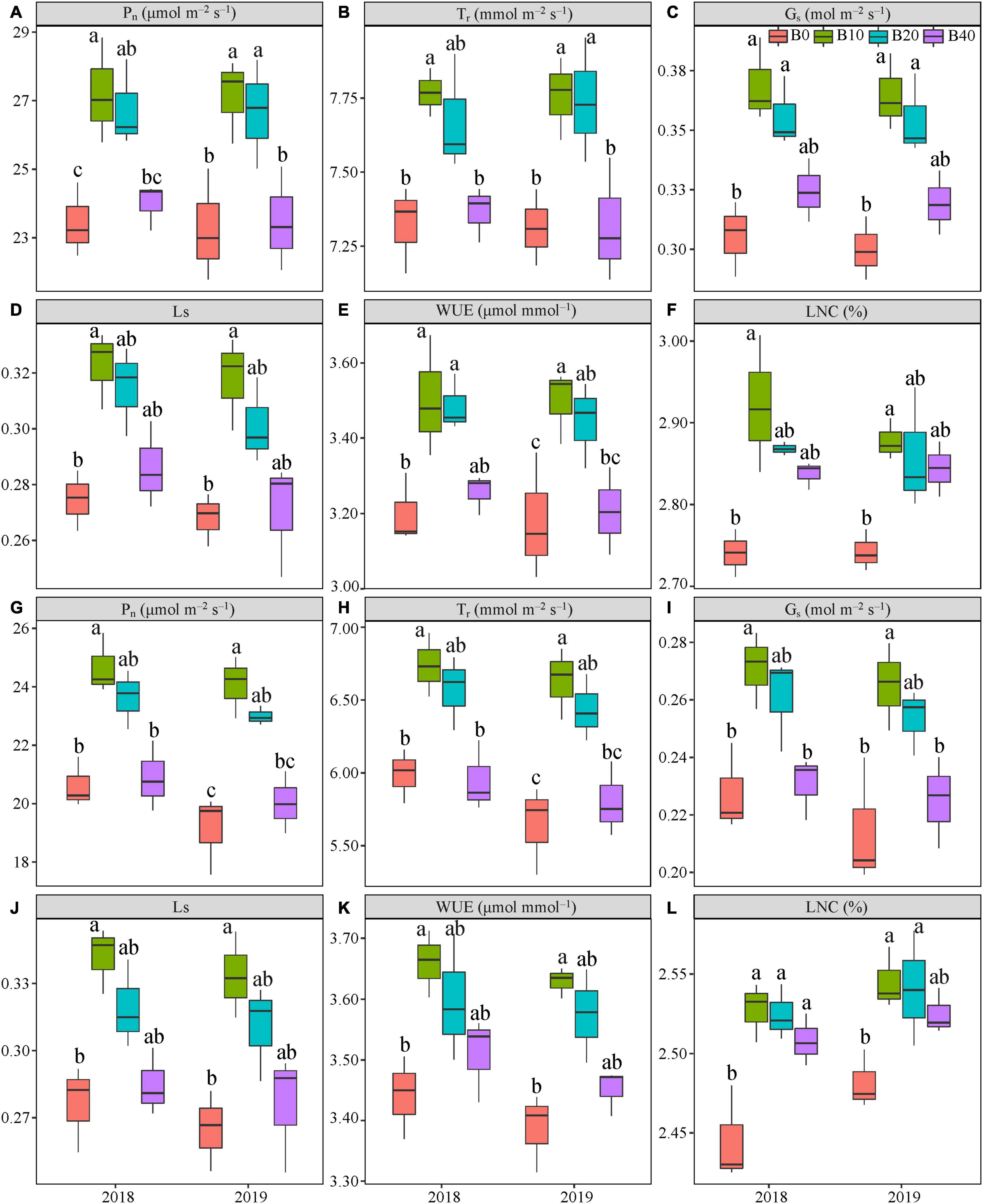
Figure 3. Gas exchange parameters at flowering (A–F) and pod set (G–L) in peanut applied with four biochar rates in 2018 and 2019 growing seasons. Y and B represent year and biochar application, respectively. Pn, net photosynthetic rate; Tr, transpiration rate; Gs, stomatal conductance; Ls, stomatal limitation; WUE, water-use efficiency; and LNC, leaf nitrogen content. B0, B10, B20, and B40 represent biochar application rates of 0, 10, 20, and 40 t ha−1, respectively. For each parameter in each year, mean data with different letters denote significant differences among treatments at P < 0.05.
Application of biochar had significant effect on Tr at flowering and pod set. There were no significant effects of year or Y × B interaction on Tr during these two stages (Table 1). Compared with B0, B10 increased Tr at flowering by 6.1% in 2018. B10 and B20 increased Tr at flowering by up to 6.1% in 2019, relative to B0 (Figure 3B). B10 increased Pn at pod set by 12.5% in 2018 and 17.5% in 2019, relative to B0 (Figure 3H). Among the four biochar treatments, B10 had the highest Tr at flowering and pod set in both years.
The Gs was significantly affected by biochar application at both flowering and pod set stages. No significant differences in year or Y × B interaction of Gs were observed at both stages (Table 1). At flowering, B10 and B20 increased Gs by up to 20.8% in 2018 and 21.6% in 2019, relative to B0 (Figure 3C). B10 increased Gs at pod set by 19.2% in 2018 and 23.6% in 2019, as compared to B0 (Figure 3I). Among the four biochar treatments, B10 had the highest Gs at flowering and pod set in both years.
Application of biochar had a significant effect on Ls at flowering and pod set. There were no significant differences in year or Y × B interaction of Ls during these two stages (Table 1). Compared with B0, B10 increased Ls at flowering by 17.5% in 2018 and 18.6% in 2019, and at pod set by 23.8% in 2018 and 25.8% in 2019 (Figures 3D,J). The highest value of Ls at flowering and pod set in both years were appeared in B10.
Biochar application significantly affected WUE at flowering and pod set, but there were no significant effects for year or Y × B interaction (Table 1). At flowering, B10 and B20 increased WUE by up to 9.4% in 2018, as compared to B0 (Figure 3E). B10 increased WUE by 10.0% in 2019, as compared to B0. At pod set, B10 increased WUE by 6.3% in 2018 and 7.1% in 2019, relative to B0 (Figure 3K). B10 had the highest mean value of WUE at flowering and pod set in both years.
The LNC was significantly affected by biochar application at flowering and pod set. No significant differences in year or Y × B interaction were observed (Table 1). At flowering and pod set, with increasing biochar application rates, LNC increased to B10 and then decreased (Figures 3F,L). Among the four biochar treatments, B10 had the highest LNC at flowering and pod set in both years.
Nitrogen Accumulation and Distribution
The effects of biochar application on root, stem, leaf, and total nitrogen accumulation were significant at flowering, but there were no significant effects for year or Y × B interaction (Table 2). B10 and B20 improved total nitrogen accumulation by 22.5 and 18.6% in 2018, 24.6 and 23.6% in 2019, relative to B0. Compare with B0, B10 and B20 improved root nitrogen accumulation by up to 25.6% in 2018 and 30.8% in 2019. The stem nitrogen accumulation in B10 improved by 21.6% in 2018, relative to B0. B10 and B20 improved stem nitrogen accumulation by up to 28.5% in 2019, as compared to B0. B10 improved leaf nitrogen accumulation by 26.4% in 2018 and 29.0% in 2019.
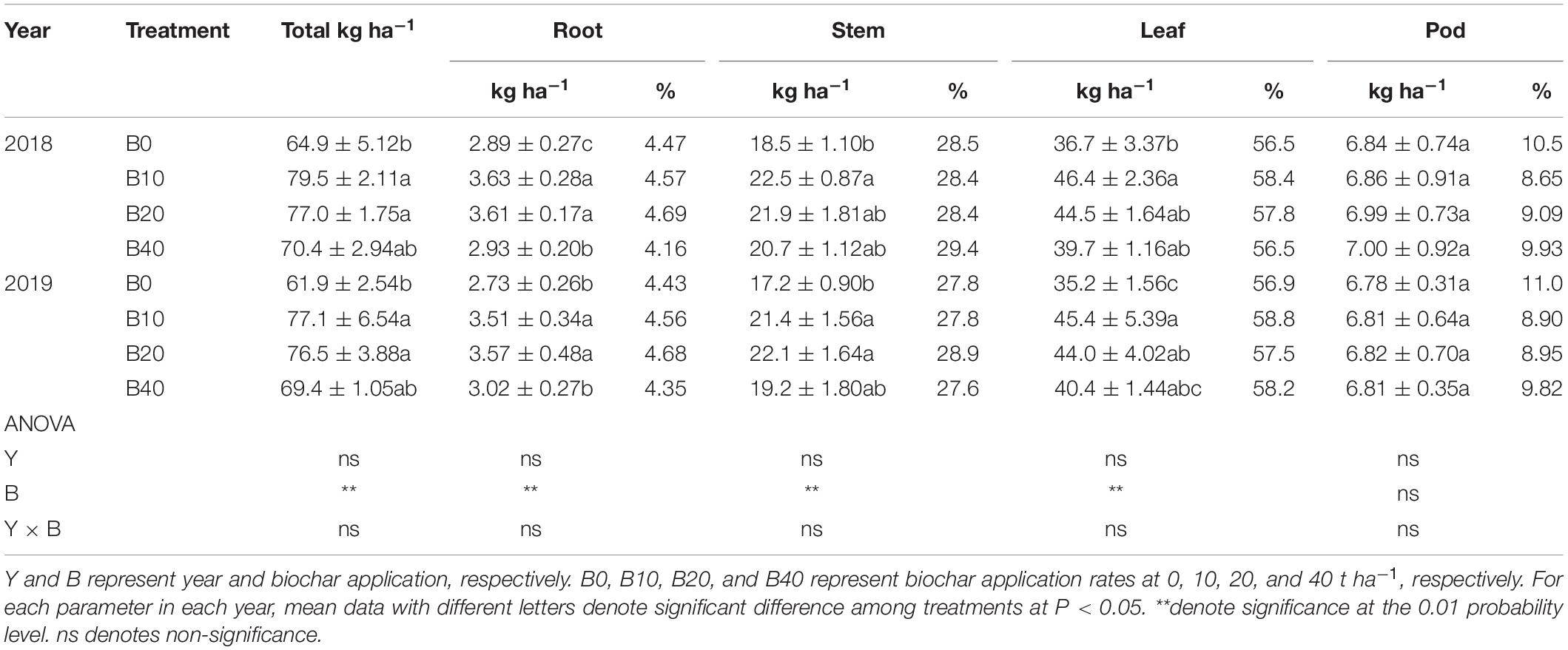
Table 2. Nitrogen accumulation and distribution at the flowering in peanut with four rates of biochar in the 2018 and 2019 growing seasons.
The root, stem, leaf, pod and total nitrogen accumulation were significantly affected by biochar application at pod set. No significant differences in year or Y × B interaction of root, stem, leaf, pod, and total nitrogen accumulation were observed at pod set (Table 3). The total nitrogen accumulation for B10 and B20 were higher than that of B0 by 25.0 and 15.3% in 2018, 23.7 and 20.3% in 2019, respectively, as compared to B0. B10 improved root nitrogen accumulation by 30.4% in 2018, relative to B0. Compared with B0, B10, and B20 improved root nitrogen accumulation by up to 30.0% in 2019. The stem nitrogen accumulation for B10 was 21.0% in 2018 and 17.8% in 2019 higher than that of B0. B10 and B20 improved leaf nitrogen accumulation by up to 24.0% in 2018 and 24.3% in 2019, relative to B0. Compared with B0, B10 improved pod nitrogen accumulation 27.0% in 2018. B10 and B20 improved pod nitrogen accumulation by 26.3% and 20.6% in 2019, as compared to B0.
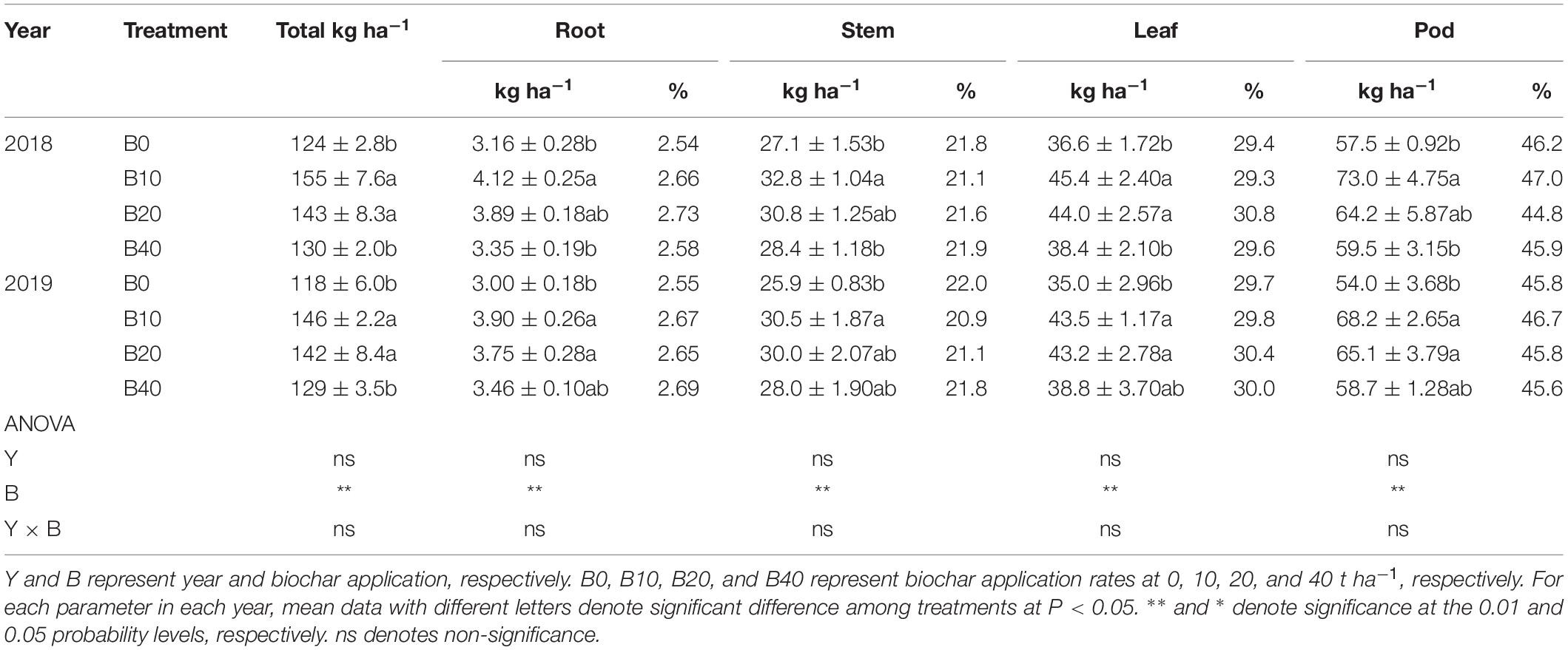
Table 3. Nitrogen accumulation and distribution at the pod set in peanut with four rates of biochar in the 2018 and 2019 growing seasons.
Yield and Yield Components
Peanut yield, kernel yield and shelling percentage were significantly affected by year and biochar application (Table 4). There was a significant Y × B interaction for shelling percentage, but not for peanut yield or kernel yield. B10 and B20 increased peanut yield by 14.6 and 10.7% in 2018, 13.7 and 11.3% in 2019, respectively, relative to B0. B10 increased kernel yield by 20.2% in 2018 and 14.4% in 2019, relative to B0. B10 and B20 had similar shelling percentages to B0, while B40 had 4.4% lower shelling percentage than B0. Among the four biochar treatments, B10 had the highest peanut yield in both years. No significant differences occurred between years, biochar application, or Y × B interaction for 100-pod weight or 100-kernel weight.
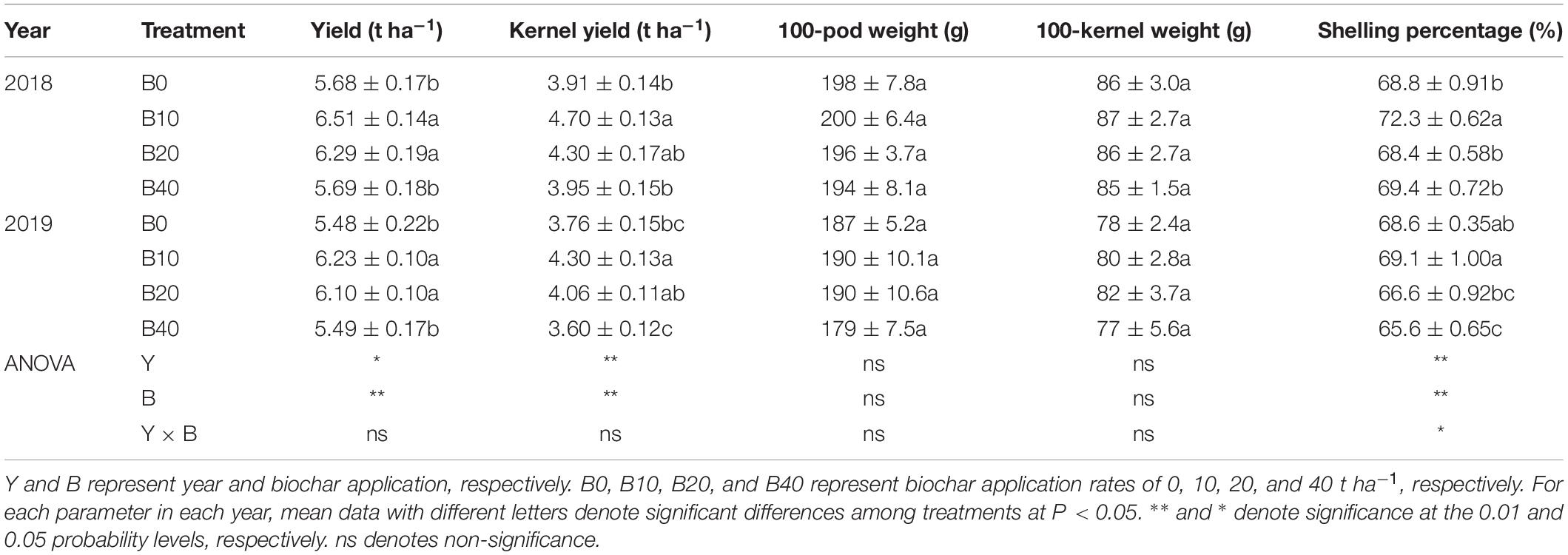
Table 4. Yield and yield components of peanut applied with four rates of biochar in the 2018 and 2019 growing seasons.
Relationship Between Net Photosynthetic Rate, Leaf Nitrogen Content, and Peanut Yield
The regression analysis indicated that Pn had a significant linear correlation with plant nitrogen accumulation at flowering and pod set in 2018 and 2019 (Figures 4A,B). Plant nitrogen accumulation explained 57.1 and 59.5% of the variation in Pn at flowering and pod set in 2018, and 60.2 and 70.3% in 2019, respectively. Positive correlations occurred between Pn at flower and pod set and peanut yield in both years (Figures 4C,D), explaining 74.3 and 86.7% of the variation in peanut yield in 2018, and 71.5 and 85.3% in 2019, respectively.
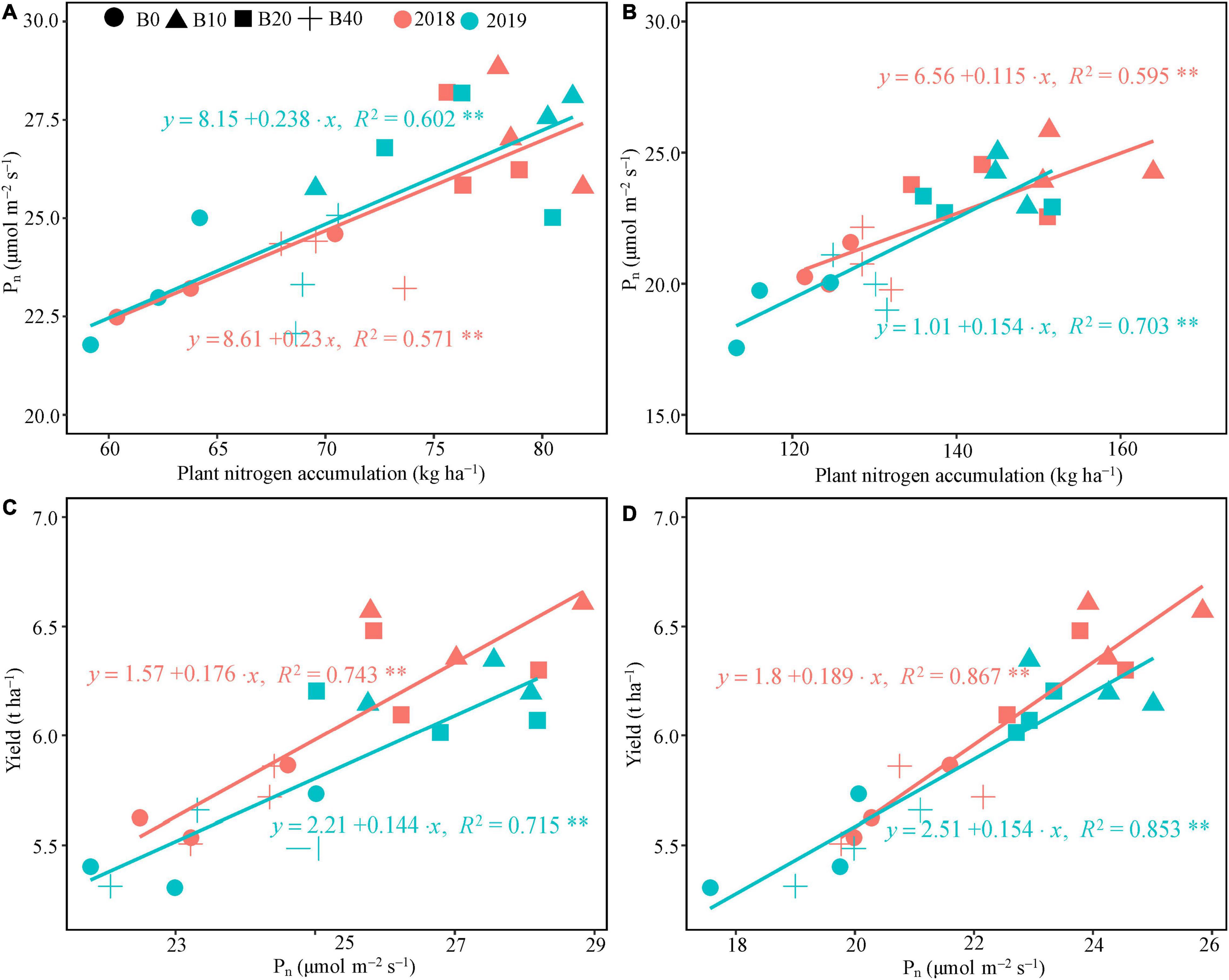
Figure 4. Relationship between plant nitrogen accumulation and photosynthetic rate, and yield and photosynthetic rate at flowering (A,C) and pod set (B,D) in peanut applied with four rates of biochar in the 2018 and 2019 growing seasons. B0, B10, B20, and B40 represent biochar application rates at 0, 10, 20, and 40 t ha−1, respectively. Pn, net photosynthetic rate; **represents significant correlations at the P < 0.01 level.
PCA Analysis for Yield and Photosynthetic Traits of Peanut
The PCA results show that PC1 and PC2 explain 95.9% of the variation in functional traits (Table 5). PC1 explains 83.6% of the variability, and accounted mainly for chlorophyll fluorescence parameters (Fv/Fm, ΦPSII, ΦNPQ, ΦNO, qP, and NPQ), gas exchange parameters (Pn, Tr, Gs, Ls, and WUE), LNC, plant nitrogen accumulation and yield and yield components (kernel yield, 100-pod weight, and 100-kernel weight; Figure 5). PC2 explains 12.3% of the variability and accounts for shelling percentage. The loadings for qP, ΦPSII, gas exchange parameters, LNC, plant nitrogen accumulation, yield, and yield components are in quadrant I and IV, and ΦNPQ, ΦNO, and NPQ are in quadrants II and III, and ΦNPQ, ΦNO, and NPQ represent limitations in photosynthetic capacity. ΦPSII, ΦNPQ, and ΦNO are distributed in different quadrants, indicating compensation effects of photochemical efficiency for dissipation by regulated and non-regulated energy losses. B10 and B20 are located in quadrants I and IV, which have a significant effect on peanut productivity, while B40 and B0 are in quadrant II and III, where absorbed light energy is lost by heat dissipation. The loading arrow of B10 is longer than that of B20. Thus, B10 in quadrant IV is an appropriate biochar application rate for relatively high photosynthetic capacity and peanut productivity.
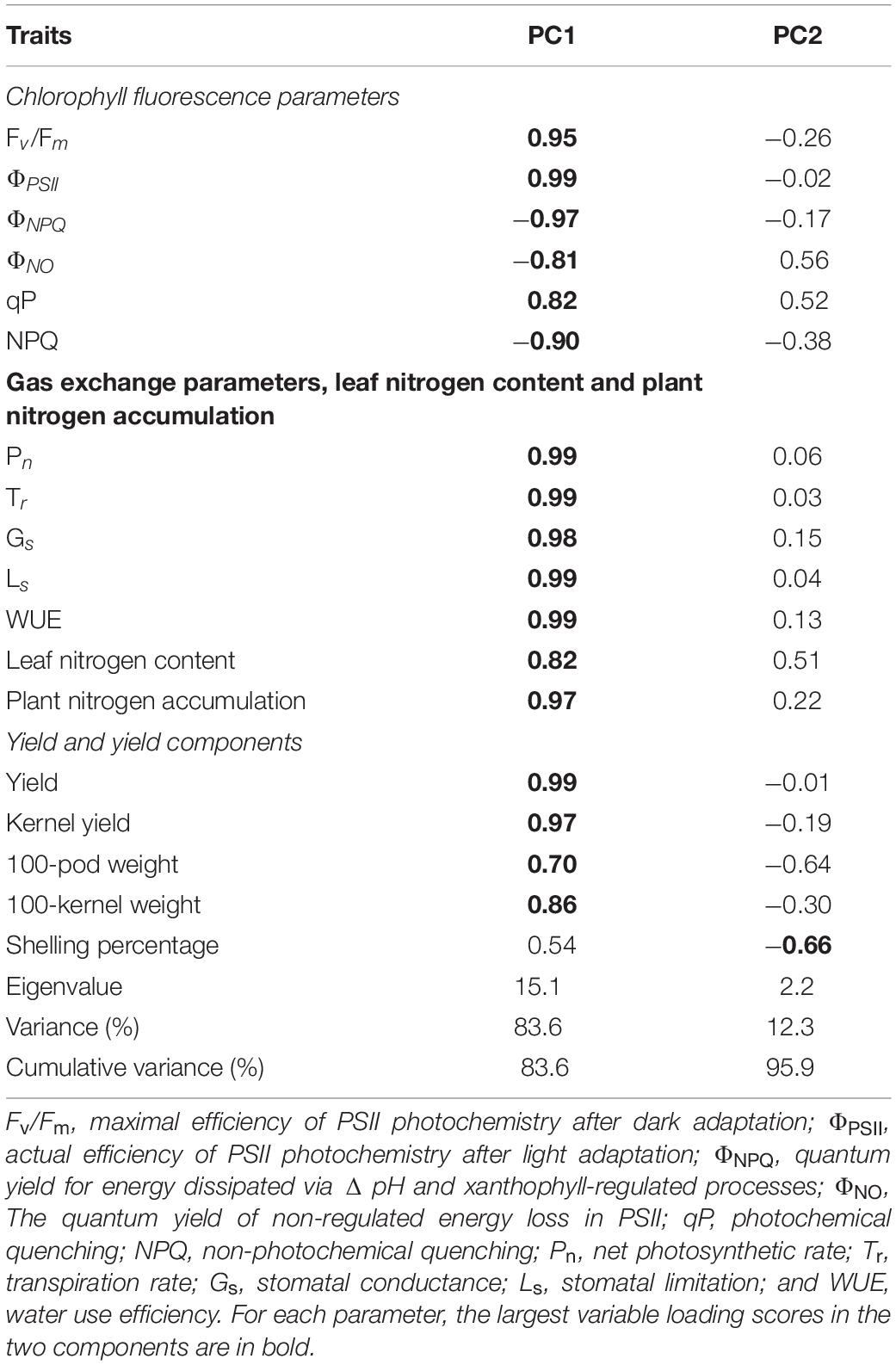
Table 5. Variable loading scores of 18 parameters for four biochar application rate and the proportion of variation of each principal component.
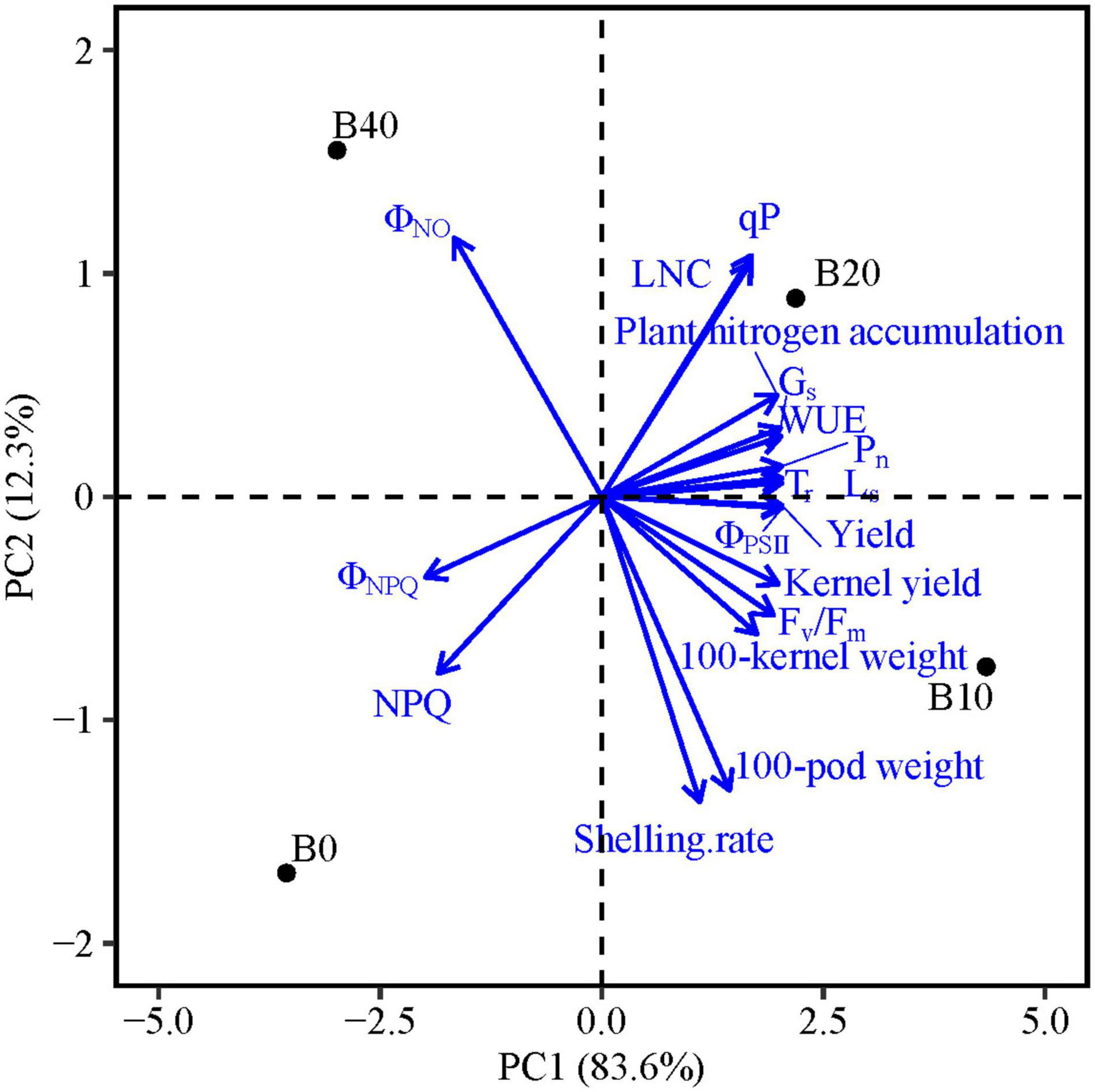
Figure 5. Principal component analyses of chlorophyll fluorescence parameters, gas exchange parameters, leaf nitrogen content, yield, and yield components of peanut in response to four biochar application rates. Means for flowering and pod set are for 2 years. Fv/Fm, maximal efficiency of PSII photochemistry after dark adaptation; ΦPSII, actual efficiency of PSII photochemistry after light adaptation; ΦNPQ, quantum yield for energy dissipated via Δ pH and xanthophyll-regulated processes; ΦNO, quantum yield of non-regulated energy loss in PSII; qP, photochemical quenching; NPQ, non-photochemical quenching; Pn, net photosynthetic rate; Tr, transpiration rate; Gs, stomatal conductance; Ls, stomatal limitation; WUE, water use efficiency; and LNC, leaf nitrogen content; B0, B10, B20, and B40 represent biochar application rates of 0, 10, 20, and 40 t ha−1, respectively.
Discussion
Effect of Biochar on Gas Exchange Parameters of Peanut
Peanut is a C3 crop with high potential for photosynthetic capacity. Therefore, exploring the photosynthetic capacity of peanut is an effective method for improving its productivity (Zelitch, 1982). Some studies have shown that biochar might improve the photosynthetic capacity of crop leaves (Rehman et al., 2016, 2019; Abbas et al., 2017). Biochar application improved leaf photosynthetic rate, which was due to the amelioration of soil physicochemical properties that ultimately increased nitrogen accumulation, and consequently enhanced photosynthetic rate (Liu et al., 2018; Huang et al., 2019; He et al., 2020). In our study, B10 enhanced Pn, Tr, Gs, Ls, and WUE at critical periods of peanut growth. In contrast, 40 t ha−1 biochar decreased these parameters, relative to 10 t ha−1 biochar (Figure 3), indicating that more biochar is not always beneficial for leaf photosynthesis. Differences in Pn among the four biochar rates could be due to the positive correlation between nitrogen accumulation and Pn at flowering and pod set in both years, as biochar application increased plant nitrogen accumulation. Plant nitrogen accumulation was higher in B10 and B20, but decreased in B40 at flowering and pod set (Tables 2, 3). The highest rate of biochar (40 t ha−1) may limit plant nitrogen accumulation which decreased leaf photosynthesis, which was likely attributed to nitrogen immobilization caused by the high C/N ratio (Asai et al., 2009). Photosynthetic rate had a positive relationship with LNC (Evans, 1989). In this study, B10 improved LNC at flowering (vegetative growth) by up to 6.6%. The significant increase in LNC at pod set was modest and may be due to reduction at pod set (reproductive growth), with more nitrogen transformed to pod (Tables 2, 3). Furthermore, the increase in dry matter production may have decreased leaf nitrogen due to the dilution effect (Guo et al., 2021). This enhancement of leaf photosynthetic rate could be explained by increased Gs and Tr after biochar application (Figure 3). The improving Gs and Tr may be associated with the increased soil water holding capacity, which might be resulting from the porous physical structure of biochar (Laghari et al., 2015; He et al., 2020). Additionally, some evidences suggested that biochar benefited root morphological development, including increased root volume, surface area and root density, to acquire more nutrients and water for enhancing photosynthesis (Bruun et al., 2014; Xiang et al., 2017). In fact, our study observed that 10 t ha−1 biochar promoted root morphology of peanut (Xia et al., 2021b). Hence, 10 t ha−1 biochar improved the nitrogen accumulation and photosynthetic rate, and consequently peanut yield.
Effect of Biochar on Chlorophyll Fluorescence Parameters of Peanut
Chlorophyll fluorescence is an important photosynthetic parameter that reflects the absorption and utilization of light energy in PSII. Fv/Fm represents the conversion efficiency of primary light energy in the PSII reaction center. Decreases in Fv/Fm are often observed when plants are exposed to abiotic and biotic stresses in the light (Baker, 2008). In our study, Fv/Fm did not significantly differ between treatments at flowering and pod set in either year (Figures 2A,G), which is consistent with Marks et al. (2016). ΦPSII is an indicator of the electron transport rate in leaves, and higher ΦPSII indicates a higher capacity of leaves to convert photon energy into chemical energy (Li et al., 2010). ΦNPQ is an important indicator of photo-protection energy dissipation, and higher ΦNPQ value shows a higher capacity to eliminate redundancy light energy by regulatory heat dissipation mechanism. ΦNO is the combined pathway of radiative and non-radiative deexcitation reactions, and higher ΦNO indicates that the absorbed light energy cannot be consumed completely through photochemical energy conversion and protective regulation mechanisms (Kramer et al., 2004; Klughammer and Schreiber, 2008; Chen et al., 2017). In this study, no significant difference in ΦNO occurred between treatments at flowering or pod set in either year (Figures 2D,J). In terms of energy distribution, B10 promoted photosynthetic activity in peanut leaves, significantly increasing ΦPSII, and decreasing ΦNPQ and ΦNO at flowering and pod set in both years (Figure 2). B0 and B40 decreased ΦPSII and increased ΦNPQ, indicating that an increase in regulated heat dissipation could protect the photosynthetic apparatus. qP represents the proportion of open PSII reaction centers (Hazrati et al., 2016). NPQ mainly comprises regulated and non-regulated energy dissipation and indicates that the light energy absorbed by PSII antenna pigments cannot be used for photochemical electron transfer, which dissipates as heat (Long et al., 2013; Perkins et al., 2018). Tang et al. (2020) reported that biochar pyrolyzed at 600°C increased qP and decreased NPQ, relative to the no-biochar treatment. Our results showed that B10 and B20 improved qP at flowering and pod set, and reduced NPQ at pod set in both years (Figure 2). It shown that 10 and 20 t ha−1 biochar enhanced the proportion of open PSII reaction centers and photosynthetic electron transfer rates in peanut leaves and reduced heat dissipation, which enable full use of the light energy absorbed in leaves for photosynthesis, and increased peanut yield. Our results are in agreement with those of Ali et al. (2020), who reported that appropriate rate of biochar increased qP and decreased NPQ at maturity stage. Biochar application improved nitrogen uptake from the soil (Sadaf et al., 2017), and a higher nitrogen concentration increased ΦPSII, qP and decreased NPQ (Lin et al., 2013). Additionally, it’s probably because biochar application enhanced leaf chlorophyll content (Feng et al., 2021), which ensured the synthesis of various enzymes and electron transporter in photosynthetic carbon assimilation, and consequently ameliorate photosynthetic function in leaves (Hou et al., 2021). Thus, the light energy absorbed by leaf was more used in photochemical processes, which led to the increase of qP and decrease of NPQ. In Summary, these results confirmed the potential of biochar for improving chlorophyll fluorescence traits. The internal mechanisms for biochar improving chlorophyll fluorescence traits merit further investigation.
Effect of Biochar on Peanut Yield
Significant differences of pod yield were observed at least 20–40 t ha−1 biochar application in pot experiment (Xu et al., 2015). In our study, 10 t ha−1 biochar produced the maximum peanut yield (and kernel yield and shelling percentage; Table 4), as reported by Ye et al. (2019). Yamato et al. (2006) reported that 10 t ha−1 biochar application combined with fertilizer in infertile soil increased peanut yield by 50%. Similarly, the biochar application rate of 10 t ha−1 significantly increased peanut pod yield by 23% compared to the inorganic fertilizer only treatment (Agegnehu et al., 2015). In another study, rice husk and cottonseed husk biochar applications at 50 t ha−1 increased peanut yields by 16.8 and 14.4%, respectively, relative to the no-biochar amendment treatment (Tan et al., 2018). In this study, B40 decreased peanut yield, relative to B10 (Table 4). Some studies have reported that high rates of biochar can cause nitrogen immobilization and decrease nitrogen accumulation due to the high C/N ratio, reducing yield (Lehmann et al., 2002; Asai et al., 2009; Li et al., 2018; Yan et al., 2019). Despite the variation between studies, legumes generally respond better to biochar than other crops. For example, biochar application increased the yields of legumes, wheat, maize, and rice by about 30, 11, 8, and 7%, respectively, Liu et al. (2013). Biochar has strong potential to improve crop productivity, especially in drought and poor soils (Batool et al., 2015; Haider et al., 2017; Hussain et al., 2017). The large interannual variability in rainfall is the main climatic factor during pod formation period, causing fluctuations in peanut yield (Craufurd et al., 2006). High soil moisture content is conducive to pod filling in peanut. In our study, August 2019 had more rainfall than August 2018 (Figure 1), and the peanut yields differed accordingly.
The pod setting stage is critical for peanut yield formation. In our study, B10 significantly improved the photosynthetic capacity of peanut at pod set (Figure 4), ensuring reproductive growth during the critical growth period and increasing peanut yield. The regression coefficient between Pn and peanut yield was higher at pod set than at flowering in both years (Figure 4), indicating that photosynthetic capacity at pod set had a positive effect on yield. Overall, the increased yield at 10 t ha−1 biochar might be due to an enhanced photosynthetic capacity of functional leaves (Figure 5).
Conclusion
Biochar application had a significant positive effect on photosynthetic capacity and yield in peanut. Maximum photochemical efficiency, actual photochemical efficiency, photochemical quenching, gas exchange parameters, leaf nitrogen content, plant nitrogen accumulation, yield, and yield components of peanut with increasing biochar application rate to 10 t ha−1 (B10). B10 significantly enhanced ΦPSII and qP in functional leaves of peanut due to the transfer of more absorbed energy to photochemical reactions, ensuring a higher photosynthetic capacity at flowering and pod set and higher peanut yield than the other biochar rates. These results are in agreement with our hypothesis. Therefore, 10 t ha−1 biochar is recommended for increasing peanut yield in Northwest Liaoning, China. The results from this study enhances our understanding of the effects of biochar application on peanut photosynthesis and yield.
Data Availability Statement
The original contributions presented in the study are included in the article/supplementary material, further inquiries can be directed to the corresponding author/s.
Author Contributions
GX, TW, DC, and TC designed the experiment. SW, YW, and QY conducted the experiments, collected and analyzed the data, and prepared the manuscript. JZ, YC, and KS revised the manuscript. All authors contributed to the article and approved the submitted version.
Funding
This research was funded by the Natural Science Foundation of Liaoning Province (Project No. 20180550819) and Scientific Research Fund of Liaoning Provincial Education Department (Project No. LSNJC202003).
Conflict of Interest
The authors declare that the research was conducted in the absence of any commercial or financial relationships that could be construed as a potential conflict of interest.
References
Abbas, T., Rizwan, M., Ali, S., Adrees, M., Zia-ur-Rehman, M., Qayyum, M. F., et al. (2017). Effect of biochar on alleviation of cadmium toxicity in wheat (Triticum aestivum L.) grown on Cd-contaminated saline soil. Environ. Sci. Pollut. Res. 25, 25668–25680. doi: 10.1007/s11356-017-8987-4
Abideen, Z., Koyro, H. W., Huchzermeyer, B., Gul, B., and Khan, M. A. (2020). Impact of a biochar or a biochar-compost mixture on water relation, nutrient uptake and photosynthesis of Phragmites karka. Pedosphere 30, 466–477. doi: 10.1016/S1002-0160(17)60362-X
Agegnehu, G., Bass, A. M., Nelson, P. N., Muirhead, B., Wright, G., and Bird, M. I. (2015). Biochar and biochar-compost as soil amendments: effects on peanut yield, soil properties and greenhouse gas emissions in tropical North Queensland, Australia. Agric. Ecosys. Environ. 213, 72–85. doi: 10.1016/j.agee.2015.07.027
Ali, I., Ullah, S., He, L., Zhao, Q., Iqbal, A., Wei, S. Q., et al. (2020). Combined application of biochar and nitrogen fertilizer improves rice yield, microbial activity and N-metabolism in a pot experiment. Peer J. 8:e10311. doi: 10.7717/peerj.10311
Ali, S., Xu, Y., Jia, Q., Ma, X., Ahmad, I., Adnan, M., et al. (2018). Interactive effects of plastic film mulching with supplemental irrigation on winter wheat photosynthesis, chlorophyll fluorescence and yield under simulated precipitation conditions. Agri. Water Manag. 207, 1–14. doi: 10.1016/j.agwat.2018.05.013
Asai, H., Samson, B. K., Stephan, H. M., Songyikhangsuthor, K., Homma, K., Kiyono, Y., et al. (2009). Biochar amendment techniques for upland rice production in Northern Laos 1. Soil physical properties, leaf SPAD and grain yield. Field Crops Res. 111, 81–84. doi: 10.1016/j.fcr.2008.10.008
Bai, W., Sun, Z., Zheng, J., Hou, Z., Liu, Y., Feng, L., et al. (2014). Effect of different planting patterns on maize growth and yield in western Liaoning province. Acta Agron. Sinica. 40, 181–189. doi: 10.3724/SP.J.1006.2014.00181
Baker, N. R. (2008). Chlorophyll fluorescence: a probe of photosynthesis in vivo. Ann. Rev. Plant Biol. 59, 89–113. doi: 10.1146/annurev.arplant.59.032607.092759
Batool, A., Taj, S., Rashid, A., Khalid, A., Qadeer, S., Saleem, A. R., et al. (2015). Potential of soil amendments (biochar and gypsum) in increasing water use efficiency of Abelmoschus esculentus L. Moench. Front. Plant Sci. 6:733. doi: 10.3389/fpls.2015.00733
Bruun, E. W., Petersen, C. T., Hansen, E., Holm, J. K., and Hauggaard-Nielsen, H. (2014). Biochar amendment to coarse sandy subsoil improves root growth and increases water retention. Soil Use Manage. 30, 109–118. doi: 10.1111/sum.12102
Chen, Y. S., Wang, Z. J., Shen, Z. J., Ou, Z. L., Xu, D. C., Yuan, Z. X., et al. (2017). Effects of oxytetracycline on growth and chlorophyll fluorescence in rape (Brassica campestris L.). Pol. J. Environ. Stud. 26, 995–1001. doi: 10.15244/pjoes/67575
Clough, T. J., Condron, L. M., Kammann, C., and Muller, C. (2013). A review of biochar and soil nitrogen dynamics. Agronomy 3, 275–293. doi: 10.3390/agronomy3020275
Craufurd, P. Q., Prasad, P. V. V., Waliyar, F., and Taheri, A. (2006). Drought, pod yield, pre-harvest Aspergillus infection and aflatoxin contamination on peanut in Niger. Field Crops Res. 98, 20–29. doi: 10.1016/j.fcr.2005.12.001
El-Naggar, A., Lee, S. S., Rinklebe, J., Farooq, M., Song, H., Sarmah, A. K., et al. (2019). Biochar application to low fertility soils: a review of current status, and future prospects. Geoderma 337, 536–554. doi: 10.1016/j.geoderma.2018.09.034
Evans, J. R. (1989). Photosynthesis and nitrogen relationships in leaves of C3 plants. Oecologia 78, 9–19. doi: 10.1007/bf00377192
Fang, X., Li, Y., Nie, J., Wang, C., Huang, K., Zhang, Y. K., et al. (2018). Effects of nitrogen fertilizer and planting density on the leaf photosynthetic characteristics, agronomic traits and grain yield in common buckwheat (Fagopyrum esculentum M.). Field Crops Res. 219, 160–168. doi: 10.1016/j.fcr.2018.02.001
FAOSTAT (2018). Food Agriculture and Organization of the United Nations (FAO). Available online at: http://www.fao.org/faostat/en/#data/QC (accessed 8 Aug, 2020).
Feng, W. Y., Yang, F., Cen, R., Liu, J., Qu, Z. Y., Miao, Q. F., et al. (2021). Effects of straw biochar application on soil temperature, available nitrogen and growth of corn. J. Environ. Manage. 277:111331. doi: 10.1016/j.jenvman.2020.111331
Guo, L. L., Bornø, M. L., Niu, W. Q., and Liu, F. L. (2021). Biochar amendment improves shoot biomass of tomato seedlings and sustains water relations and leaf gas exchange rates under different irrigation and nitrogen regimes. Agri. Water Manag. 245:106580. doi: 10.1016/j.agwat.2020.106580
Haider, G., Steffens, D., Moser, G., Müller, C., and Kammann, C. I. (2017). Biochar reduced nitrate leaching and improved soil moisture content without yield improvements in a four-year field study. Agric. Ecosyst. Environ. 237, 80–94. doi: 10.1016/j.agee.2016.12.019
Hazrati, S., Tahmasebi, S. Z., Modarres, S. S. A. M., Mokhtassi, B. A., and Nicola, S. (2016). Effects of water stress and light intensity on chlorophyll fluorescence parameters and pigments of Aloe vera L. Plant Physiol. Biochem. 106, 141–148. doi: 10.1016/j.plaphy.2016.04.046
He, Y. H., Yao, Y. X., Ji, Y. H., Deng, J., Zhou, G. Y., Liu, R. Q., et al. (2020). Biochar amendment boosts photosynthesis and biomass in C3 but not C4 plants: a global synthesis. GCB Bioenergy 12, 605–617. doi: 10.1111/gcbb.12720
Hou, W. H., Zhang, Y. X., Wang, H. J., Zhang, Q. X., Hou, M. L., Cong, B. M., et al. (2021). Effects of nitrogen application level on leaf photosynthetic characteristics and chlorophyll fluorescence characteristics of Leymus chinensis. Acta Agrestia Sinica 29, 532–536. doi: 10.11733/j.issn.1007-0435.2021.03.014
Huang, X. F., Li, S. Q., Li, S. Y., Ye, G. Y., Lu, L. J., Zhang, L., et al. (2019). The effects of biochar and dredged sediments on soil structure and fertility promote the growth, photosynthetic and rhizosphere microbial diversity of Phragmites communis (Cav.) Trin. ex Steud. Sci. Total Environ. 697:134073. doi: 10.1016/j.scitotenv.2019.134073
Hussain, M., Farooq, M., Nawaz, A., Al-Sadi, A. M., Solaiman, Z. M., Alghamdi, S. S., et al. (2017). Biochar for crop production: potential benefits and risks. J. Soil Sediment. 17, 685–716. doi: 10.1007/s11368-016-1360-2
Ippolito, J. A., Cui, L. Q., Kammann, C., Mönnig, N. W., Estavillo, J. M., Mendizabal, T. F., et al. (2020). Feedstock choice, pyrolysis temperature and type influence biochar characteristics: a comprehensive meta-data analysis review. Biochar 2, 421–438. doi: 10.1007/s42773-020-00067-x
Kammann, C. I., Linsel, S., Gößling, J. W., and Koyro, H. W. (2011). Influence of biochar on drought tolerance of Chenopodium quinoa Willd and on soil-plant relations. Plant Soil 345, 195–210. doi: 10.1007/s11104-011-0771-5
Kassambara, A. (2015). Factoextra: Visualization of the Outputs of a Multivariate Analysis. R Package Version 1: Statistical Tools for Highthroughput Data Analysis. Available online at: http://www.sthda.com
Klughammer, C., and Schreiber, U. (2008). Complementary PS II quantum yields calculated from simple fluorescence parameters measured by PAM fluorometry and the saturation pulse method. PAM Appl. Notes 1, 27–35.
Kooten, O. V., and Snel, J. F. H. (1990). The use of chlorophyll fluorescence nomenclature in plant stress physiology. Photosynth. Res. 25, 147–150. doi: 10.1007/BF00033156
Kramer, D. M., Johnson, G., Kiirats, O., and Edwards, G. E. (2004). New fluorescence parameters for the determination of QA redox state and excitation energy fluxes. Photosynth. Res. 79, 209–218. doi: 10.1023/B:PRES.0000015391.99477.0d
Laghari, M., Mirjat, M. S., Hu, Z., Fazal, S., Xiao, B., Hu, M., et al. (2015). Effects of biochar application rate on sandy desert soil properties and sorghum growth. Catena 135, 313–320. doi: 10.1016/j.catena.2015.08.013
Lehmann, J., da Silva, J. P. Jr., Steiner, C., Nehls, T., Zech, W., and Glaser, B. (2002). Nutrient availability and leaching in an archaeological Anthrosol and a Ferralsol of the Central Amazon basin: fertilizer, manure and charcoal amendments. Plant Soil 249, 343–357. doi: 10.1023/A:1022833116184
Lehmann, J., Rillig, M. C., Thies, J., Masiello, C. A., Hockaday, W. C., and Crowley, D. (2011). Biochar effects on soil biota-a review. Soil Biol. Biochem. 43, 1812–1836. doi: 10.1016/j.soilbio.2011.04.022
Leng, L. J., Xu, S. Y., Liu, R. F., Yu, T., Zhou, X. M., Leng, S. Q., et al. (2020). Nitrogen containing functional groups of biochar: an overview. Bioresour. Technol. 298:122286. doi: 10.1016/j.biortech.2019.122286
Li, G., Wan, S., Zhou, J., Yang, Z., and Qin, P. (2010). Leaf chlorophyll fluorescence, hyperspectral reflectance, pigments content, malondialdehyde and proline accumulation responses of castor bean (Ricinus communis L.) seedlings to salt stress levels. Ind. Crops Prod. 31, 13–19. doi: 10.1016/j.indcrop.2009.07.015
Li, S. L., Zhou, P., and Shang, G. (2018). Positive effects of apple branch biochar on wheat yield only appear at a low application rate, regardless of nitrogen and water conditions. J. Soil Sediment. 18, 3235–3243. doi: 10.1007/s11368-018-1994-3
Li, X. Y., and Gong, J. D. (2002). Effects of different ridge: furrow ratios and supplemental irrigation on crop production in ridge and furrow rainfall harvesting system with mulches. Agri Water Manag. 54, 243–254. doi: 10.1016/S0378-3774(01)00172-X
Li, Y. S., Wu, L. H., Zhao, L. M., Lu, X. H., Fan, Q. L., and Zhang, F. S. (2007). Influence of continuous plastic film mulching on yield, water use efficiency and soil properties of rice fields under nonflooding condition. Soil Tillage Res. 93, 370–378. doi: 10.1016/j.still.2006.05.010
Lin, Y. C., Hu, Y. G., Ren, C. Z., Guo, L. C., Wang, C. L., Jiang, Y., et al. (2013). Effects of nitrogen application on chlorophyll fluorescence parameters and leaf gas exchange in naked oat. J. Integr. Agric. 12, 2164–2171. doi: 10.1016/S2095-3119(13)60346-9
Lin, Z., Liu, Q., Liu, G., Cowie, A. L., Bei, Q., Liu, B. I. U., et al. (2017). Effects of different biochars on Pinus elliottii growth, N use efficiency, soil N2O and CH4 emissions and C storage in a subtropical area of China. Pedosphere 27, 248–261. doi: 10.1016/S1002-0160(17)60314-X
Liu, Q., Zhang, Y. H., Liu, B. J., Amonette, J. E., Lin, Z. B., Liu, G., et al. (2018). How does biochar influence soil N cycle? A meta-analysis. Plant Soil 426, 211–225. doi: 10.1007/s11104-018-3619-4
Liu, X. Y., Zhang, A. F., Ji, C. Y., Joseph, S., Bian, R. J., Li, L. Q., et al. (2013). Biochar’s effect on crop productivity and the dependence on experimental conditions—a meta-analysis of literature data. Plant Soil 373, 583–594. doi: 10.1007/s11104-013-1806-X
Liu, Z. X., Gao, F., Yang, J. Q., Zhen, X. Y., Li, Y., Zhao, J. H., et al. (2019). Photosynthetic characteristics and uptake and translocation of nitrogen in peanut in a wheat-peanut rotation system under different fertilizer management regimes. Front. Plant Sci. 10:86. doi: 10.3389/fpls.2019.000086
Long, J. R., Ma, G. H., Wan, Y. Z., Song, C. F., Sun, J., and Qin, R. J. (2013). Effects of nitrogen fertilizer level on chlorophyll fluorescence characteristics in flag leaf of super hybrid rice at late growth stage. Rice Sci. 20, 220–228. doi: 10.1016/S1672-6308(13)60138-9
Luo, H. Y., Xu, Z. J., Li, Z. D., Li, X. P., Lv, J. W., Ren, X. P., et al. (2017). Development of SSR markers and identification of major quantitative trait loci controlling shelling percentage in cultivated peanut (Arachis hypogaea L.). Theor. Appl. Genet. 130, 1635–1648. doi: 10.1007/s00122-017-2915-3
Marks, E. A. N., Mattana, S., Alcañiz, J. M., Pérez-Herrero, E., and Domene, X. (2016). Gasifier biochar effects on nutrient availability, organic matter mineralization, and soil fauna activity in a multi-year Mediterranean trial. Agric. Ecosyst. Environ. 215, 30–39. doi: 10.1016/j.agee.2015.09.004
Maxwell, K., and Johnson, G. N. (2000). Chlorophyll fluorescence-a practical guide. J. Exp. Bot. 51, 659–668. doi: 10.1093/jexbot/51.345.659
Nguyen, T. T. N., Wallace, H. M., Xu, C. Y., Xu, Z. H., Farrar, M. B., Joseph, S., et al. (2017). Short-term effects of organo-mineral biochar and organic fertilisers on nitrogen cycling, plant photosynthesis, and nitrogen use efficiency. J. Soil. Sediment. 17, 2763–2774. doi: 10.1007/s11368-017-1839-5
Novak, J. M., Busscher, W. J., Watts, D. W., Amonette, J. E., Ippolito, J. A., Lima, I. M., et al. (2012). Biochars impact on soil-moisture storage in an ultisol and two aridisols. Soil Sci. 177, 310–320. doi: 10.1097/SS.0b013e31824e5593
Peel, M. C., Finlayson, B. L., and McMahon, T. A. (2007). Updated world map of the Köppen Geiger climate classification. Hydrol. Earth Syst. Sci. Discuss. 11, 1633–1644. doi: 10.5194/hess-11-1633-2007
Perkins, R., Williamson, C., Lavaud, J., Mouget, J. L., and Campbell, D. A. (2018). Time-dependent upregulation of electron transport with concomitant induction of regulated excitation dissipation in Haslea diatoms. Photosynth. Res. 137, 377–388. doi: 10.1007/s11120-018-0508-X
Rehman, M., Liu, L. J., Bashir, S., Saleema, M. H., Chen, C., Peng, D. X., et al. (2019). Influence of rice straw biochar on growth, antioxidant capacity and copper uptake in ramie (Boehmeria nivea L.) grown as forage in aged copper contaminated soil. Plant Physiol. Biochem. 138, 121–129. doi: 10.1016/j.plaphy.2019.02.021
Rehman, M. Z., Rizwan, M., Ali, S., Fatima, N., Yousaf, B., Naeem, A., et al. (2016). Contrasting effects of biochar, compost and farm manure on alleviation of nickel toxicity in maize (Zea mays L.) in relation to plant growth, photosynthesis and metal uptake. Ecotoxicol. Environ. Saf. 133, 218–225. doi: 10.1016/j.ecoenv.2016.07.023
Sadaf, J., Shah, G. A., Shahzad, K., Ali, N., Shahid, M., Ali, S., et al. (2017). Improvements in wheat productivity and soil quality can accomplish by co-application of biochars and chemical fertilizers. Sci. Total Environ. 607–608, 715–724. doi: 10.1016/j.scitotenv.2017.06.178
Steinmetz, Z., Wollmann, C., Schaefer, M., Buchmann, C., David, J., Tröger, J., et al. (2016). Plastic mulching in agriculture. Trading short-term agronomic benefits for long-term soil degradation? Sci. Total Environ. 550, 690–705. doi: 10.1016/j.scitotenv.2016.01.153
Sun, T., Li, G., Ning, T. Y., Zhang, Z. M., Mi, Q. H., and Lal, R. (2018). Suitability of mulching with biodegradable film to moderate soil temperature and moisture and to increase photosynthesis and yield in peanut. Agri Water Manag. 208, 214–223. doi: 10.1016/j.agwat.2018.06.027
Tan, G. C., Wang, H. Y., Xu, N., Liu, H. B., and Zhai, L. M. (2018). Biochar amendment with fertilizers increases peanut N uptake, alleviates soil N2O emissions without affecting NH3 volatilization in field experiments. Environ. Sci. Pollut. Res. 25, 8817–8826. doi: 10.1007/s11356-017-1116-6
Tang, J. W., Zhang, S. D., Zhang, X. T., Chen, J. H., He, X. Y., and Zhang, Q. Z. (2020). Effects of pyrolysis temperature on soil-plant-microbe responses to Solidago canadensis L.-derived biochar in coastal saline-alkali soil. Sci. Total Environ. 731:138938. doi: 10.1016/j.scitotenv.2020.138938
Xia, G. M., Wang, Y. J., Hu, J. Q., Wang, S. J., Zhang, Y., Wu, Q., et al. (2021a). Effects of supplemental irrigation on water and nitrogen use, yield, and kernel quality of peanut under nitrogen-supplied conditions. Agri Water Manag. 243:106518. doi: 10.1016/j.agwat.2020.106518
Xia, G. M., Wang, Y. J., Wang, S. J., Yang, Q. F., and Chi, D. C. (2021b). Effects of irrigation methods and biochar on peanut root, phosphorus utilization and yield. T. Chin. Soc. Agric. Mach.
Xiang, Y. Z., Deng, Q., Duan, H. L., and Guo, Y. (2017). Effects of biochar application on root traits: a meta-analysis. GCB Bioenergy 9, 1563–1572. doi: 10.1111/gcbb.12449
Xu, C. Y., Hosseini, B. S., Hao, Y., Rachaputi, R. C. N., Wang, H., Xu, Z. H., et al. (2015). Effect of biochar amendment on yield and photosynthesis of peanut on two types of soils. Environ. Sci. Pollut. Res. 22, 6112–6125. doi: 10.1007/s11356-014-3820-9
Yamato, M., Okimori, Y., Wibowo, I. F., Anshori, S., and Ogawa, M. (2006). Effects of the application of charred bark of Acacia mangium on the yield of maize, cowpea and peanut, and soil chemical properties in South Sumatra, Indonesia. Soil Sci. Plant Nutr. 52, 489–495. doi: 10.1111/j.1747-0765.2006.00065.x
Yan, Q. Y., Dong, F., Li, J. H., Duan, Z. Q., Yang, F., Li, X., et al. (2019). Effects of maize straw-derived biochar application on soil temperature, water conditions and growth of winter wheat. Eur. J. Soil Sci. 70, 1280–1289. doi: 10.1111/ejss.12863
Ye, L. L., Camps, A. M., Shen, Q. H., Lehmann, J., Singh, B., and Sabir, M. (2019). Biochar effects on crop yields with and without fertilizer: a meta-analysis of field studies using separate controls. Soil Use Manage. 36, 2–18. doi: 10.1111/sum.12546
Ye, Z. X., Liu, L. Y., Tan, Z. X., Zhang, L. M., and Huang, Q. Y. (2020). Effects of pyrolysis conditions on migration and distribution of biochar nitrogen in the soil-plant-atmosphere system. Sci. Total Environ. 723:138006. doi: 10.1016/j.scitotenv.2020.138006
Keywords: biochar, chlorophyll fluorescence, plant nitrogen accumulation, photosynthetic traits, peanut yield
Citation: Wang S, Zheng J, Wang Y, Yang Q, Chen T, Chen Y, Chi D, Xia G, Siddique KHM and Wang T (2021) Photosynthesis, Chlorophyll Fluorescence, and Yield of Peanut in Response to Biochar Application. Front. Plant Sci. 12:650432. doi: 10.3389/fpls.2021.650432
Received: 07 January 2021; Accepted: 03 May 2021;
Published: 31 May 2021.
Edited by:
Maxwell Ware, Colorado State University, United StatesReviewed by:
Gretchen Kroh, Texas A&M University, United StatesSam Wilson, Queen Mary University of London, United Kingdom
Copyright © 2021 Wang, Zheng, Wang, Yang, Chen, Chen, Chi, Xia, Siddique and Wang. This is an open-access article distributed under the terms of the Creative Commons Attribution License (CC BY). The use, distribution or reproduction in other forums is permitted, provided the original author(s) and the copyright owner(s) are credited and that the original publication in this journal is cited, in accordance with accepted academic practice. No use, distribution or reproduction is permitted which does not comply with these terms.
*Correspondence: Guimin Xia, eGlhZ20xMjI5QDEyNi5jb20=; Tieliang Wang, dGllbGlhbmd3YW5nQHN5YXUuZWR1LmNu