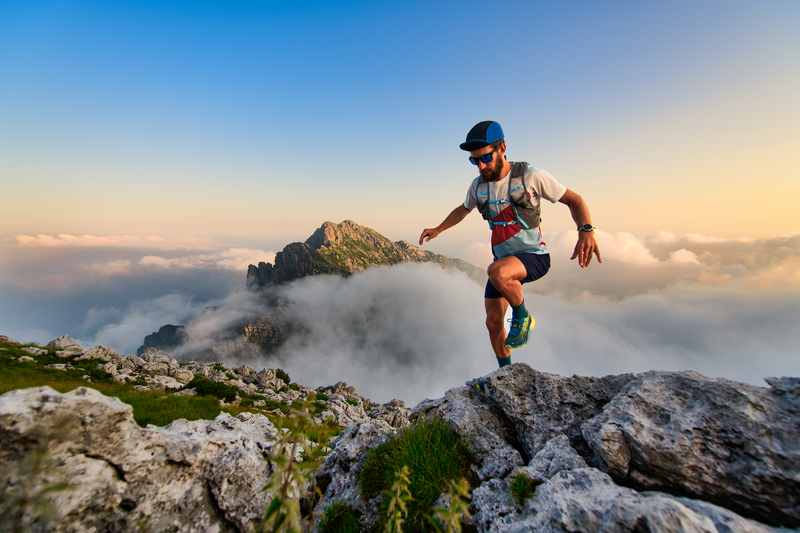
94% of researchers rate our articles as excellent or good
Learn more about the work of our research integrity team to safeguard the quality of each article we publish.
Find out more
ORIGINAL RESEARCH article
Front. Plant Sci. , 10 August 2021
Sec. Plant Breeding
Volume 12 - 2021 | https://doi.org/10.3389/fpls.2021.647599
This article is part of the Research Topic Breeding Innovations in Underutilized Temperate Fruit Trees View all 11 articles
The common fig (Ficus carica L.) has a gynodioecious breeding system, and its sex phenotype is an important trait for breeding because only female plant fruits are edible. During breeding to select for female plants, we analyzed the FcRAN1 genotype, which is strongly associated with the sex phenotype. In 12 F1 populations derived from 13 cross combinations, the FcRAN1 genotype segregation ratio was 1:1, whereas the M119-226 × H238-107 hybridization resulted in an extremely male-biased segregation ratio (178:7 = male:female). This finding suggests that the segregation distortion was caused by some genetic factor(s). A whole-genome resequencing of breeding parents (paternal and maternal lines) identified 9,061 high-impact SNPs in the parents. A genome-wide linkage analysis exploring the gene(s) responsible for the distortion revealed 194 high-impact SNPs specific to Caprifig6085 (i.e., seed parent ancestor) and 215 high-impact SNPs specific to H238-107 (i.e., pollen parent) in 201 annotated genes. A comparison between the annotated genes and the genes required for normal embryo or gametophyte development and function identified several candidate genes possibly responsible for the segregation distortion. This is the first report describing segregation distortion in F. carica.
During cross breeding, the import frequency of alleles into gametes often varies in the progeny population or a specific gene combination causes sterility, resulting in a segregation ratio that is inconsistent with Mendelian inheritance (i.e., segregation distortion). Segregation distortions have been detected in a wide range of taxa (Burt and Trivers, 2006) and are increasingly recognized as a potentially powerful evolutionary force (Taylor and Ingvarsson, 2003). Segregation distortion is thought to have diverse causes, some of which involve gametophytic lethality, embryonic lethality, or cross-incompatibility (Lashermes et al., 2001; Candela et al., 2011) or are related to (selfish) genetic elements that can enhance their own transmission (Dawkins, 1976; Werren et al., 1988). The selfish genetic factors include Cytoplasmic male sterility (Lewis, 1941), B chromosome (Ostergren, 1945), X chromosome drive (Gershenson, 1928; Sturtevant and Dobzhansky, 1936), Y chromosome drive (Hickey and Craig, 1966; Sweeny and Barr, 1978; Wood and Newton, 1991), t haplotype (Dobrovolskaia-Zavadskaia and Kobozieff, 1927), and transposable factors (McClintock, 1950).
Fig (Ficus carica L.) is a dioecious or gynodioecious species, and because only female plant fruits are edible, sex is an important target trait during breeding (Stover et al., 2007; Flaishman et al., 2008). The common fig has the following two sexual types: caprifig type (males) with both male and female flowers and fig type (females) with only female flowers. The progeny resulting from a normal fig type (G/G) × caprifig type (G/A) cross should theoretically be 50% fig and 50% caprifig (fig:caprifig = 1:1) (Storey, 1975).
Mori et al. (2017) identified FcRAN1 and determined that it is highly correlated with the fig sex phenotype on the basis of several analyses, including a genome-wide association study. Additionally, because of a single nucleotide polymorphism (SNP), its alleles are heterozygous (G/A) in males (caprifig type) and homozygous (G/G) in females (fig type) (Storey, 1975). Moreover, FcRAN1 is the only gene in the fig genome that carries a polymorphism that perfectly corresponds to the sex phenotype (Mori et al., 2017). In our current fig breeding program, we genotyped the FcRAN1 alleles of some breeding populations to identify sex phenotypes. We observed that individuals with male type alleles are produced at a high rate only in a specific cross combination (M119-226 × H238-107), suggesting that the FcRAN1 locus is affected by segregation distortion. Clarifying the cause of this distortion may lead to improved sex control and selection during fig breeding, but it may also provide insights into sex ratio transitions and sex chromosome evolution in fig.
In the present study, we examined the segregation distortion in fig breeding populations and performed preliminary analyses to explore the responsible genetic factors. More precisely, we investigated the distribution of SNPs specific to each pollen and seed parent generating segregation distortion in a whole-chromosome pseudomolecule and considered the biological and evolutionary background related to the occurrence of the segregation distortion.
Crossing experiments involving 10 cross combinations of seven seed parents (M119-226, V180-13, Masui Dauphine, H180-2, HIG5, Toyomitsuhime, and Burjassotte Greece) and six pollen parents (H238-107, M238-1, VD238-83, VS238-53, NG60, and CH-13) generated 1,232 seedlings. The progeny population sizes ranged from 12 to 370 (Table 1). Three cross combinations involving three seed parents (Violette de Dauphine, Violette de Solies, and Royal Vineyard) and two pollen parents (M106-238 and VC-180) from a previous study (Mori et al., 2017) were also analyzed. In 12 of the 13 cross combinations, the segregation ratio of the male (G/A) and female (G/G) genotypes of FcRAN1 was close to the theoretical segregation ratio of 1:1, ranging from 3:9 to 192:176 (G/A:G/G; χ2-test: p = 0.014–1.00). In contrast, for the cross combination (C100) involving the seed parent M119-226 and the pollen parent H238-107, the segregation ratio was 178:7 (G/A:G/G; χ2-test: p < 2.2e-16) (Table 1 and Figure 1). An examination of pollen conditions using an optical microscope revealed that more than 99% of the pollen grains were clearly stained and had a normal appearance in all parental lines, including H238-107 (Figure 2).
Figure 1. Genotyping results of FcRAN1 SNP by KASP assay. Genotypic results for 10 progenies derived from 10 cross combination using 7 pollen parent lines. The scatter plot with axes x and y represents allelic discrimination of FcRAN1 genotypes. The red, green dots represent the A/A homozygous (female fig type), A/G heterozygous (male caprifig type) respectively. NTC, no template controls, or empty.
Figure 2. Optical microscope images of stained pollen grains (A) H238-107 (distorting line: left) and (B) Kibaru (CH13) (non-distorting line: right). Bar = 40 μm.
To identify the factor(s) responsible for the segregation distortion, a genome-wide linkage analysis (GWLA) was performed using the whole-genome sequence of each seed and pollen parent. However, the seed parent of C100, M119-226, died after seeds were acquired, preventing it from being analyzed. Therefore, the genome sequence of Caprifig6085, which was an ancestral genotype of M119-226 and may have a genomic region associated with segregation distortion, was used for seed parent analysis as an alternative to M119-226 (Table 1 and Figure 3) (Segregation distortion was not observed in the mating with Masui Dauphine and Violette de Solies as parents). The 4,614,159 SNPs generated by Shirasawa et al. (2021, unpublished data) included 9,061 high-impact SNPs in the seed and pollen parents. The GWLA using the SNP panel indicated that 194 high-impact SNPs were specific to Caprifig6085 as the seed parent and 215 high-impact SNPs were specific to H238-107 as the pollen parent. The functional annotation of these SNPs on the basis of a BLAST analysis produced information for 201 SNPs (98 and 103 SNPs for the seed and pollen parents, respectively) (Supplementary Tables 1, 2). The genes with high-impact SNPs included a LEA gene (i.e., encoding a late embryogenesis abundant protein). A sequence analysis revealed that the fig LEA gene (designated as FcLEA1) is a single-copy gene located on chromosome Fc01a, which also includes the FcRAN1 locus (Fc01a: 181,985–187,609). Moreover, the polymorphic site in FcLEA1 corresponds to a non-synonymous substitution that changes a leucine to a proline (Figure 4).
Figure 3. Pedigree chart related to “M119-226.” Seed parent (Masui Dauphine) and paternal grand seed parent (Violette Solies) are marked with “ND” as parents that didn’t cause segregation distortion. “M119-226” and the other genotypes are marked with “D” or “D?” as parents that caused or could cause segregation distortion.
Figure 4. Amino acid sequence alignment of FcLEA genes (LC639910). “Horaishi” is the variety of the reference genome. “Caprifig6085” indicates a variety with segregation distortion, and “Masui Dauphine” indicates a representative varietie of the group without segregation distortion. The leucine residue marked with an asterisk (*) in FcLEA was mutated to proline to make a non-synonymous substitution. Numbers in left side of sequence indicate the position of the beginning amino acid residue in each protein sequence.
The mapping of all GWLA SNPs to whole pseudomolecules indicated that high-impact SNPs were widely distributed throughout the genome in the seed and pollen parents, but there were no high-impact SNPs on chromosomes Fc03 and Fc06 of the seed parents (Figure 5).
Figure 5. Schematic Diagram showing GWLA SNPs that mapped to the whole chromosome pseudomolecules. (A) High impact SNP marker map in pollen parents and (B) high impact SNP marker map in seed parents. Horizontal lines on each chromosome indicate the location of SNP markers. The left side number indicate the distance from a telomere point of Fc01a chromosome pseudomolecule.
It is unclear whether the observed segregation distortion is caused by gametophytic lethality, embryonic lethality, or specific genetic events. Accordingly, in this study, a GWLA was performed assuming that the causative factor is located on the chromosomes of either the seed parent (M119-226) or the pollen parent (H238-107) of the population with distorted segregation. Unfortunately, all M119-226 plants died before their genome sequence data could be obtained, making it impossible to further analyze line M119-226. However, there were four ancestral lines of M119-226, namely Caprifig6085, VC-119, Masui Dauphine, and Violette de Dauphine. Both Masui Dauphine and Violette de Dauphine are not associated with segregation distortion (Table 1). Therefore, during the analysis of the seed parents, it was assumed that the Caprifig6085 and VC-119 genomes include a causative factor of segregation distortion. In this study, Caprifig6085 was used as a substitute for M119-226.
Since it is assumed that there are a wide variety of factors that can cause segregation distortion, the number of candidate genes involved is considered to be large corresponding to be the number of factors. For example, in Arabidopsis thaliana, 510 EMBRYO-DEFECTIVE (EMB) genes (Meinke, 2020) and 129 genes associated with female gametophyte deficiency (Pagnussat et al., 2005) have been identified, all of which may contribute to segregation distortion. During the analysis of the seed parents, 98 genes were detected as specific to M119-226. Additionally, we identified several genes similar to the above-mentioned A. thaliana genes related to female gametophyte deficiency.
The fertilization of the A. thaliana mutant line UNE15, which has a mutation in a gene encoding a LEA protein, is reportedly abnormal because of defective pollen tube guidance (Pagnussat et al., 2005). Accordingly, the female gametophytes of the seed parent M119-226 may be infertile because of a mutation similar to that in UNE15. In addition to the LEA gene, other candidate genes may be responsible for segregation distortion, including genes encoding proteins with unknown functions.
If segregation distortion is due to male gametophytic lethality, the causal allele (gene) is likely located on the X chromosomes of the pollen parents because most of the genotyped alleles in the C100 population were the G/A allele derived from the Y chromosome. However, an examination of the pollen used for mating indicated there were no differences in the shapes and appearance of the pollen grains between the pollen parent lines with and without distorted segregation, implying the distortion was at least independent of the pollen physiological state. It remains possible there is a defect in the process after the pollination event (e.g., pollen tube elongation). The TATA element modulatory factor (TMF) (FCA_r2.3chr09: 8,140,218) may affect the segregation distortion. The A. thaliana TFIID factor AtTAF6 (i.e., a TATA-binding protein) controls pollen tube growth (Lago et al., 2005). Additionally, TMF binds to the HIV-1 TATA element and inhibits the transcriptional activation by the TATA-binding protein (Garcia et al., 1992).
Although the current study did not determine whether FcRAN1 is a true sex-determining gene in F. carica, a male-biased FcRAN1 genotype ratio suggests that Y chromosome drive (Voelker, 1972; Sweeny and Barr, 1978; Wood and Newton, 1991; Presgraves et al., 1997) is important (Figure 6). For a Y chromosome drive system to be functional, the distorter gene and FcRAN1 (i.e., potential sex-determining gene) should be at different loci on the same Y chromosome (Fc01a) of the pollen parent. Of the nine genes detected on chromosome Fc01a (Supplementary Table 2), the RNase H-encoding gene should be investigated for its potential role in segregation distortion. Simoni et al. (2020) introduced a sex-distorter (I-PpoI) and a CRISPR-based gene drive into mosquito (Anopheles gambiae), resulting in stable Y chromosome drive and decreases in the proportion of male individuals in successive generations. Thus, a site-specific DNA double-strand break system involving endonucleases, including I-PpoI, is necessary for a functional Y chromosome drive (Ohle et al., 2016).
Figure 6. Y chromosome drive model for the role of distorter in Segregation Distortion (SD). Segregation distorter, on the fig Y-chromosome, is expressed during male meiosis to cut a target sequence on the X-chromosome. The shredding of the X-chromosome favors the unaffected Y-carrying gametophyte and results in the production of a male-biased progeny.
The FcRAN1 KASP marker analysis indicated that some plants in the C100 population had the G/A genotype. There are two possible explanations for this observation. First, the effect of the distorter may have been incomplete. Second, specific events (e.g., chromosomal recombination) may have occurred in which the distorter gene was not activated. Future studies should further characterize the genetic background of the distortion via crossing experiments involving multiple seed parents, including H238-107, and the female C100 progeny. Moreover, because the candidate genes discussed herein were selected on the basis of bioinformatics data, their functions will need to be validated through genetic transformation or genome editing.
The high-impact SNPs in the pollen parents were within 5 Mbp from the end of Fc01a (Figure 5), suggestive of a relationship between the sex-linked region and the segregation distortion. Because the sex-determining gene (i.e., FcRAN1 or a nearby gene) basically affects the population sex ratio, the distorter gene and FcRAN1 are likely located close together and behave similarly.
The biological or evolutionary significance of the segregation distortion observed in figs is unclear. However, the occurrence of segregation distortion can change the genetic composition of the species population and may maintain or increase the genetic diversity of the population. In fact, segregation distortion reportedly leads to stable large-scale genomic differences between males and females in hybrid ants (Kulmuni et al., 2010).
Because the FcRAN1 genotype is strongly correlated with the sex phenotype, the genotypic distortion could influence the evolution of sexual reproduction and sex chromosomes in various ways (Kozielska et al., 2010). Fig X and Y chromosomes are homomorphic, with no significant differences in their molecular structure (Storey, 1975), indicating that fig sex chromosomes are like autosomal chromosomes and are not well differentiated. Therefore, clarifying the segregation distortion of the FcRAN1 genotype may help elucidate the differentiation of fig sex and autosomal chromosomes.
Seven seed parents (M119-226, V180-13, Masui Dauphine, H180-2, HIG5, Toyomitsuhime, and Burjassotte Greece) and six pollen parents (H238-107, M238-1, VD238-83, VS238-53, NG60, and CH13) were used for hybridizations, which were completed between 1989 and 2018 according to Storey (1975). The obtained seeds were sown in a seedbed and the resulting young seedlings were transplanted to 9-cm pots containing dedicated soil. Leaf samples were collected from the seedlings for KASP and CAPS marker analyses as described below.
Of the 1,232 plants resulting from 10 cross combinations, 1,220 (i.e., from the C100, C101, C102, C95, C94, C98, C78, C79, and C74 populations) were included in the FcRAN1 KASP marker analysis, which was performed according to the manufacturer-recommended protocol for the LGC Genomics high-throughput genotyping system. The KASP Master mix and consumable materials were supplied by LGC Genomics (Middlesex, United Kingdom). A PCR primer pair, FcRAN1_f (5′-AGATCCTTAGTTGATGGGGT-3′) and FcRAN1_r (5′-CCTCAAACATGTTTAGACTG-3′), was designed based on the sequences flanking the SNP in the probe sequence: GAAGGTTTAAATTAC[A/G]TGTTGCTAATCCTT. Additionally, the 12 plants in the C205 population were genotyped using the CAPS marker as described by Mori et al. (2017). The SNP genotyping data generated from the KASP marker analysis were visualized using the SNPviewer software (LGC, Biosearch Technologies, Beverly, MA, United States).
The pollen of each paternal line was immersed undisturbed in a 600 μl 1% (w/v) iodine solution for 5 min, after which 50 μl of the mixture was added to a microscope slide, covered with a cover glass, and examined using an optical microscope. Pollen grains stained orange or brown were considered to be viable and were counted.
Total genomic DNA was extracted from the leaves of eight caprifig-type varieties (H238-107, M238-1, VS238-53, M106-238, CH13, VD238-83, NG-60, and VC-180) and eight fig-type varieties (Masui Dauphine, H180-2, HIG5, Toyomitsuhime, Burjassotte Greece, Violette de Dauphine, Violette de Soloes, and Royal Vineyard) using the DNeasy Plant kit (Qiagen, Valencia, CA, United States). Sequencing libraries were constructed using the genomic DNA as previously described (Shirasawa et al., 2016). Sequences were obtained using the HiSeq 2000 system in the paired-end mode, with a read length of 151 bp. The generated data were processed as described by Shirasawa et al. (2020). High-quality reads were selected by trimming adapters with fastx_clipper (parameter: -a AGATCGGAAGAGC) in the FASTX Toolkit (version 0.0.13)1 and deleting low-quality bases with PRINSEQ (version 0.20.4) (Schmieder and Edwards, 2011). Reads were aligned to a common fig reference genome sequence (Shirasawa et al., 2021, unpublished data, the data is available upon request) using Bowtie2 (version 2.2.3) (Langmead and Salzberg, 2012). Sequence variants were detected using the mpileup command in SAMtools (version 0.1.19) (Li et al., 2009). High-confidence heterozygous SNPs were selected using VCFtools (version 0.1.12b) (parameters: –minDP5 –minQ 999 –max-missing 0.75) (Danecek et al., 2011). The SNP effects were categorized using SnpEff (version 3.0) The default parameters of SnpEff were used to analyze variant effects (Cingolani et al., 2012). Genes were predicted and annotated according to previousinformation (Shirasawa et al., 2020).
The GWLAs of 10 seed parents (M119-226, V180-13, Masui Dauphine, H180-2, HIG5, Toyomitsuhime, Burjassotte Greece, Violette de Dauphine, Violette de Soloes, and Royal Vineyard) and eight pollen parents (H238-107, M238-1, VS238-53, CH13, VD238-83, NG-60, M106-238, and VC-180) were performed using the corresponding genotype datasets derived from the whole-genome resequencing. In each panel, only SNPs with a minor allele frequency >5% were used for the linkage analysis. Case-control association analyses treating the non-distorting type as the control and the distorting type as the case for high-, moderate-, and low-impact SNP sets were conducted to calculate allele frequencies using PLINK 1.90 beta (Purcell et al., 2007). The sex phenotype dataset was generated in a recent study (Mori et al., 2017). The SNPs with an F_A (frequency of this allele in cases) of 1.0 or 0.5 and an F_U (frequency of this allele in controls) of 0.0 were selected as candidate SNPs responsible for the difference between distorting and non-distorting lines.
The FcLEA gene sequence has been deposited in DDBJ/EMBL/GenBank under accession number LC639910. Genome sequencing reads of caprifig lines have been deposited in the NCBI Sequence Read Archive under accession numbers DRA011350 and DRA012209.
The datasets presented in this study can be found in online repositories. The names of the repository/repositories and accession number(s) can be found in the article/Supplementary Material.
HI and HY designed the study. HI, KS, HY, SY, TH, and HN completed the analyses. HI, KS, HY, SY, MS, TH, KT, and HN interpreted the data. HI, KS, and HY wrote the manuscript. All authors contributed to the article and approved the submitted version.
This work was supported in part by a grant from the Ministry of Education, Culture, Sports, Science and Technology/Japan Society for Promoting Science (KAKENHI grant 19H02952 and 16H04878).
The authors declare that the research was conducted in the absence of any commercial or financial relationships that could be construed as a potential conflict of interest.
All claims expressed in this article are solely those of the authors and do not necessarily represent those of their affiliated organizations, or those of the publisher, the editors and the reviewers. Any product that may be evaluated in this article, or claim that may be made by its manufacturer, is not guaranteed or endorsed by the publisher.
The Supplementary Material for this article can be found online at: https://www.frontiersin.org/articles/10.3389/fpls.2021.647599/full#supplementary-material
Supplementary Table 1 | “Type” refers to the genetic segregation type: 1| 0 means that the target sample is homozygous for the reference sequence and the other samples are homozygous for the other sequence, whereas 0.5| 0 means the target sample is heterozygous for the reference sequence and the other samples are homozygous for the other sequence.
Supplementary Table 2 | “Type” refers to the genetic segregation type: 1| 0 means that the target sample is homozygous for the reference sequence and the other samples are homozygous for the other sequence, whereas 0.5| 0 means the target sample is heterozygous for the reference sequence and the other samples are homozygous for the other sequence.
Burt, A., and Trivers, R. (2006). Genes in Conflict. The Biology of Selfish Genetic Elements. Cambridge, MA: Harvard University Press.
Candela, H., Pérez-Pérez, J. M., and Micol, J. L. (2011). Uncovering the post-embryonic functions of gametophytic- and embryonic-lethal genes. Trends Plant Sci. 16, 336–345. doi: 10.1016/j.tplants.2011.02.007
Cingolani, P., Platts, A., Wang, L. L., Coon, M., Nguyen, T., Wang, L., et al. (2012). A program for annotating and predicting the effects of single nucleotide polymorphisms, SnpEff: SNPs in the genome of Drosophila melanogaster strain w1118; iso-2; iso-3. Fly 6, 80–92. doi: 10.4161/fly.19695
Danecek, P., Auton, A., Abecasis, G., Albers, C. A., Banks, E., DePristo, M. A., et al. (2011). The variant call format and VCFtools. Bioinformatics 27, 2156–2158. doi: 10.1093/bioinformatics/btr330
Dobrovolskaia-Zavadskaia, N., and Kobozieff, N. (1927). Sur la reproduction des souris anoures. C. R. Soc. Biol. 97, 116–118.
Flaishman, M., Rodov, V., and Stover, E. (2008). “The fig: botany, horticulture, and breeding,” in Horticultural Reviews, ed. J. Janick (Hoboken, NJ: John Wiley & Sons), 113–196.
Garcia, J. A., Ignatius Ou, S.-H., Wu, F., Lusis, A. J., Sparkes, R. S., and Gaynor, R. B. (1992). Cloning and chromosomal mapping of a human immunodeficiency virus 1 “TATA” element modulatory factor. Proc. Natl. Acad. Sci. U.S.A. 89, 9372–9376. doi: 10.1073/pnas.89.20.9372
Gershenson, S. (1928). A new sex-ratio abnormality in DROSOPHILA OBSCURA. Genetics 13, 488–507. doi: 10.1093/genetics/13.6.488
Hickey, W. A., and Craig, G. B. Jr. (1966). Genetic distortion of sex ratio in a mosquito, Aedes aegypti. Genetics 53, 1177–1196. doi: 10.1093/genetics/53.6.1177
Kozielska, M., Weissing, F. J., Beukeboom, L. W., and Pen, I. (2010). Segregation distortion and the evolution of sex-determining mechanisms. Heredity 104, 100–112. doi: 10.1038/hdy.2009.104
Kulmuni, J., Seifert, B., and Pamilo, P. (2010). Segregation distortion causes large-scale differences between male and female genomes in hybrid ants. Proc. Natl. Acad. Sci. U.S.A. 107, 7371–7376. doi: 10.1073/pnas.0912409107
Lago, C., Clerici, E., Dreni, L., Horlow, C., Caporali, E., Colombo, L., et al. (2005). The Arabidopsis TFIID factor AtTAF6 controls pollen tube growth. Dev. Biol. 285, 91–100. doi: 10.1016/j.ydbio.2005.06.006
Langmead, B., and Salzberg, S. L. (2012). Fast gapped-read alignment with Bowtie 2. Nat. Methods 9, 357–359. doi: 10.1038/nmeth.1923
Lashermes, P., Combes, M. C., Prakash, N. S., Trouslot, P., Lorieux, M., and Charrier, A. (2001). Genetic linkage map of Coffea canephora: effect of segregation distortion and analysis of recombination rate in male and female meioses. Genome 44, 589–596. doi: 10.1139/gen-44-4-589
Lewis, D. (1941). Male sterility in natural populations of hermaphrodite plants the equilibrium between females and hermaphrodites to be expected with different types of inheritance. New Phytol. 40, 56–63. doi: 10.1111/j.1469-8137.1941.tb07028.x
Li, H., Handsaker, B., Wysoker, A., Fennell, T., Ruan, J., Homer, N., et al. (2009). The sequence alignment/map format and SAMtools. Bioinformatics 25, 2078–2079. doi: 10.1093/bioinformatics/btp352
McClintock, B. (1950). The origin and behavior of mutable loci in maize. Proc. Natl. Acad. Sci. U.S.A. 36, 344–355. doi: 10.1073/pnas.36.6.344
Meinke, D. W. (2020). Genome-wide identification of EMBRYO-DEFECTIVE (EMB) genes required for growth and development in Arabidopsis. New Phytol. 226, 306–325. doi: 10.1111/nph.16071
Mori, K., Shirasawa, K., Nogata, H., Hirata, C., Tashiro, K., Habu, T., et al. (2017). Identification of RAN1 orthologue associated with sex determination through whole genome sequencing analysis in fig (Ficus carica L.). Sci. Rep. 7:41124.
Ohle, C., Tesorero, R., Schermann, G., Dobrev, N., Sinning, I., and Fischer, T. (2016). Transient RNA-DNA hybrids are required for efficient double-strand break repair. Cell 167, 1001–1013.e7.
Pagnussat, G. C., Yu, H. J., Ngo, Q. A., Rajani, S., Mayalagu, S., Johnson, C. S., et al. (2005). Genetic and molecular identification of genes required for female gametophyte development and function in Arabidopsis. Development 132, 603–614. doi: 10.1242/dev.01595
Presgraves, D. C., Severance, E., and Wilkinson, G. S. (1997). Sex chromosome meiotic drive in stalk-eyed flies. Genetics 147, 1169–1180. doi: 10.1093/genetics/147.3.1169
Purcell, S., Neale, B., Todd-Brown, K., Thomas, L., Ferreira, M. A., Bender, D., et al. (2007). PLINK: a tool set for whole-genome association and population-based linkage analyses. Am. J. Hum. Genet. 81, 559–575. doi: 10.1086/519795
Schmieder, R., and Edwards, R. (2011). Fast identification and removal of sequence contamination from genomic and metagenomic datasets. PLoS One 6:e17288. doi: 10.1371/journal.pone.0017288
Shirasawa, K., Hirakawa, H., and Isobe, S. (2016). Analytical workflow of double-digest restriction site-associated DNA sequencing based on empirical and in silico optimization in tomato. DNA Res. 23, 145–153. doi: 10.1093/dnares/dsw004
Shirasawa, K., Yakushiji, H., Nishimura, R., Morita, T., Jikumaru, S., Ikegami, H., et al. (2020). The Ficus erecta genome aids Ceratocystis canker resistance breeding in common fig (F. carica). Plant J. 102, 1313–1322. doi: 10.1111/tpj.14703
Simoni, A., Hammond, A. M., Beaghton, A. K., Galizi, R., Taxiarchi, C., Kyrou, K., et al. (2020). A male-biased sex-distorter gene drive for the human malaria vector Anopheles gambiae. Nat. Biotechnol. 38, 1054–1060. doi: 10.1038/s41587-020-0508-1
Storey, W. B. (1975). “Figs,” in Advances in Fruit Breeding, eds J. Janick and J. N. Moore (West Lafayette, IN: Purdue University Press), 568–589.
Stover, E., Aradhya, M., Ferguson, L., and Crisosto, C. H. (2007). The fig: overview of an ancient fruit. HortScience 42:1083. doi: 10.21273/hortsci.42.5.1083
Sturtevant, A. H., and Dobzhansky, T. (1936). Geographical distribution and cytology of “Sex Ratio” in Drosophila pseudoobscura and related species. Genetics 21, 473–490. doi: 10.1093/genetics/21.4.473
Sweeny, T. L., and Barr, A. R. (1978). Sex ratio distortion caused by meiotic drive in a mosquito, Culex pipiens L. Genetics 88, 427–446. doi: 10.1093/genetics/88.3.427
Taylor, D. R., and Ingvarsson, P. K. (2003). Common features of segregation distortion in plants and animals. Genetica 117, 27–35.
Voelker, R. A. (1972). Preliminary characterization of “sex ratio” and rediscovery and reinterpretation of “male sex ratio” in Drosophila affinis. Genetics 71, 597–606. doi: 10.1093/genetics/71.4.597
Werren, J. H., Nur, U., and Wu, C.-I. (1988). Selfish genetic elements. Trends Ecol. Evol. 3, 297–302.
Keywords: segregation distortion, male-biased, genome-wide linkage analysis, embryo, gametophyte
Citation: Ikegami H, Shirasawa K, Yakushiji H, Yabe S, Sato M, Hayashi T, Tashiro K and Nogata H (2021) Analysis of the Segregation Distortion of FcRAN1 Genotypes Based on Whole-Genome Resequencing of Fig (Ficus carica L.) Breeding Parents. Front. Plant Sci. 12:647599. doi: 10.3389/fpls.2021.647599
Received: 30 December 2020; Accepted: 08 June 2021;
Published: 10 August 2021.
Edited by:
Giuseppe Ferrara, University of Bari Aldo Moro, ItalyReviewed by:
Amir Sherman, University of North Carolina at Chapel Hill, United StatesCopyright © 2021 Ikegami, Shirasawa, Yakushiji, Yabe, Sato, Hayashi, Tashiro and Nogata. This is an open-access article distributed under the terms of the Creative Commons Attribution License (CC BY). The use, distribution or reproduction in other forums is permitted, provided the original author(s) and the copyright owner(s) are credited and that the original publication in this journal is cited, in accordance with accepted academic practice. No use, distribution or reproduction is permitted which does not comply with these terms.
*Correspondence: Hidetoshi Ikegami, aWtlZ2FtaUBmYXJjLnByZWYuZnVrdW9rYS5qcA==
Disclaimer: All claims expressed in this article are solely those of the authors and do not necessarily represent those of their affiliated organizations, or those of the publisher, the editors and the reviewers. Any product that may be evaluated in this article or claim that may be made by its manufacturer is not guaranteed or endorsed by the publisher.
Research integrity at Frontiers
Learn more about the work of our research integrity team to safeguard the quality of each article we publish.