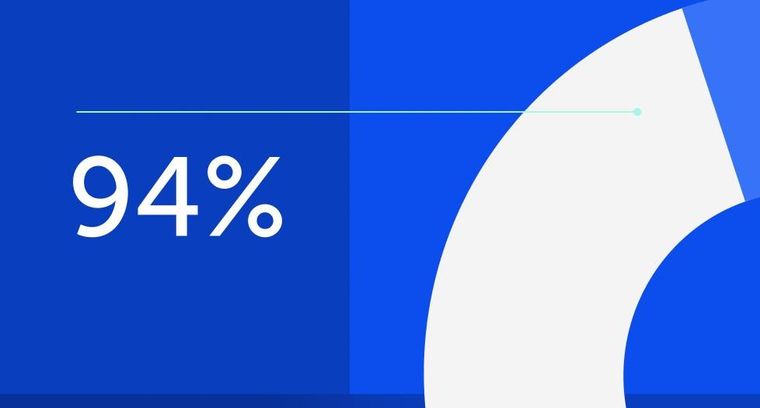
94% of researchers rate our articles as excellent or good
Learn more about the work of our research integrity team to safeguard the quality of each article we publish.
Find out more
REVIEW article
Front. Plant Sci., 17 February 2021
Sec. Plant Physiology
Volume 12 - 2021 | https://doi.org/10.3389/fpls.2021.644823
This article is part of the Research TopicCytokinins as Central Regulators of Plant Growth and DevelopmentView all 14 articles
Plants growing in any particular geographical location are exposed to variable and diverse environmental conditions throughout their lifespan. The multifactorial environmental pressure resulted into evolution of plant adaptation and survival strategies requiring ability to integrate multiple signals that combine to yield specific responses. These adaptive responses enable plants to maintain their growth and development while acquiring tolerance to a variety of environmental conditions. An essential signaling cascade that incorporates a wide range of exogenous as well as endogenous stimuli is multistep phosphorelay (MSP). MSP mediates the signaling of essential plant hormones that balance growth, development, and environmental adaptation. Nevertheless, the mechanisms by which specific signals are recognized by a commonly-occurring pathway are not yet clearly understood. Here we summarize our knowledge on the latest model of multistep phosphorelay signaling in plants and the molecular mechanisms underlying the integration of multiple inputs including both hormonal (cytokinins, ethylene and abscisic acid) and environmental (light and temperature) signals into a common pathway. We provide an overview of abiotic stress responses mediated via MSP signaling that are both hormone-dependent and independent. We highlight the mutual interactions of key players such as sensor kinases of various substrate specificities including their downstream targets. These constitute a tightly interconnected signaling network, enabling timely adaptation by the plant to an ever-changing environment. Finally, we propose possible future directions in stress-oriented research on MSP signaling and highlight its potential importance for targeted crop breeding.
Under natural conditions, plants are need to continuously maintain a balance between growth and defense or adaptive responses that are dictated by the severity, duration, and developmental timing at which any particular stress occurs (Claeys and Inzé, 2013). The essential question fundamental to understanding the mechanisms of hormonal control over any stress response is what is the very first action of the plant in response to (a sudden or long-term) change in environmental conditions? Does the stress response begin by activating the expression of defense/stress-responsive gene(s) that then subsequently impacts the hormonal status, or do plant hormones mediate the expression of stress-responsive transcription factors (TFs), or do hormonal signaling and metabolism act either synergistically with or independently on stress response gene expression?
The answer is not trivial, simply because stress responses employ mutually interconnected molecular cascades and networks, and a detailed description of these networks is outside the scope of this review. Recently available evidence suggests a combination of all the above response mechanisms. For instance, numerous studies have been published that show stress-controlled expression of key members of the MSP pathway, while genome-wide and proteomic studies of cytokinin (one of the key plant hormones and a major regulator of MSP activity, see below) action have revealed that cytokinin modulates levels of stress-related genes/proteins (Brenner et al., 2005, 2012; Bhargava et al., 2013; Černý et al., 2014; Brenner and Schmülling, 2015; Raines et al., 2016). On the other hand, the metabolism of stress-associated plant hormones abscisic acid (ABA) and cytokinins are also rapidly modulated by stress conditions. Stress initiation results in a rapid (under an hour) increase of ABA content but reduced endogenous levels of cytokinins together with attenuated expression of cytokinin receptor genes (Dobrá et al., 2015). Induction of jasmonic acid (JA) production was observed to occur within seconds after wounding (root excision), suggesting that in this case, regulation of endogenous hormonal levels is the primary stress response (Zhou et al., 2019). Down- and up-regulation of signaling components acting downstream of cytokinins and ABA, respectively, suggests a combined effect of hormone metabolism and signal transduction on the regulation of heat-stress responses (Skalák et al., 2016). In the case of ethylene, multiple environmental stresses stimulate its biosynthesis to act antagonistically with ABA in the regulation of shoot and root growth under water-limiting conditions (Sharp and Lenoble, 2002; Skirycz et al., 2011; Dubois et al., 2018). The expression of ETHYLENE RESPONSE FACTOR 1 (ERF1) was gradually induced by drought stress treatment over 12 h (reaching a maximum after 1 h), and this plays a positive role in drought, salt, and heat stress tolerance via stress-specific gene regulation (Cheng et al., 2013).
All these findings (and many others described below) show how important plant hormone signaling (including the MSP) and metabolism are during early stress responses. In the next few sections, we attempt to summarize recent observations on the biological relevance of MSP-mediated stress adaptation strategies in plants (mostly in Arabidopsis thaliana).
Mainly due to historic reasons, much of the information about MSP has been on cytokinin signaling. Cytokinins are recognized by membrane-bound ARABIDOPSIS HISTIDINE KINASES (AHK2, AHK3, and AHK4/CRE1/WOL), located both at the plasma membrane and the endoplasmic reticulum (Caesar et al., 2011; Wulfetange et al., 2011). Recently, the quantitatively minor fraction of plasma membrane-located AHKs was shown to be functional in mediating the extracellular cytokinin signal (Antoniadi et al., 2020; Kubiasová et al., 2020). According to the current model (Figure 1), cytokinins bind to the CHASE domain (Hothorn et al., 2011) and activate intracellular histidine kinase (HK) activity possibly by changing receptor conformation; this then leads to sensor autophosphorylation (Hutchison and Kieber, 2002; Kieber and Schaller, 2018). The presence of phospho-His triggers downstream His-to-Asp-to-His-to-Asp phosphorelay. In the first (intramolecular) step, the phosphoryl group is passed from a conserved His residue of the HK domain to the conserved Asp residue located in the receiver domain of the receptor (Hwang and Sheen, 2001; Müller and Sheen, 2007). The small and mobile cytosolic proteins, ARABIDOPSIS HISTIDINE-CONTAINING PHOSPHOTRANSMITTERs (AHPs) 1-5, which shuttle between the cytosol and the nucleus, mediate the next step (Hwang and Sheen, 2001; Hutchison et al., 2006; Figure 1) and serve as a substrate for the phosphorylation of the terminal phosphate acceptors, the nuclear-located type-B ARABIDOPSIS RESPONSE REGULATORS (B-ARRs). Once the type B-ARRs are phosphorylated, inhibition on their GARP DNA-binding domain is released. This initiates the transcription of cytokinin-regulated genes, including the type-A RESPONSE REGULATORS (A-ARRs), the cytokinin primary-response genes, which also function as negative feedback regulators of the signaling pathway (Kieber and Schaller, 2018). In tandem with type-B ARRs, CYTOKININ RESPONSE FACTORS (CRFs) – closely related members of the Arabidopsis APETALA 2/ETHYLENE RESPONSE FACTOR (AP2/ERF) gene family, mediate a large fraction of the transcriptional response to cytokinins, affecting a set of cytokinin-responsive genes that largely overlaps with targets of type-B ARRs (Rashotte et al., 2006). For more details we would like to point the reader to some excellent comprehensive reviews, e.g., those by Zürcher and Müller (2016) or Kieber and Schaller (2018).
Figure 1. Multistep phosphorelay integrates hormonal and environmental signaling during abiotic stress responses. The output of multistep phosphorelay is affected by crosstalk with a number of individual signaling pathways. The crosstalk takes place either at the level of AHPs phosphorylation by the receptors with varying signal specificities or through interaction of the response regulators (both A-ARRs and B-ARRs) with various transcription factors [e.g., ELONGATED HYPOCOTYL 5 (HY5), CONSTITUTIVE PHOTOMORPHOGENIC 1 (COP1), ABSCISIC ACID RESPONSIVE ELEMENT-BINDING FACTORS (ABFs)], as well as SNF1-RELATED PROTEIN KINASE 2 (SnRK2) and phytochromes. See the main text for a detailed description of each signaling pathway. Color code: MSP signaling is depicted in green, ethylene signaling in blue, light signaling in ochre, abscisic acid in purple. The His/Asp phosphorylation is depicted by circled P in bright yellow, Ser/Thr/Tyr phosphorylation in orange. Created with BioRender.com.
Ethylene is recognized by sensors belonging to subfamily 1, consisting of ETHYLENE RESPONSE 1 (ETR1) and ETHYLENE RESPONSE SENSOR 1 (ERS1) with a functional HK domain, and the HK-like sensor Ser/Thr kinases ETR2, ERS2, and ETHYLENE INSENSITIVE 4 (EIN4), that make up subfamily 2 (Etheridge et al., 2006). Ethylene binds to the hydrophobic pocket of the receptors via a copper cofactor, delivered there by the action of copper transporter, RESPONSE TO ANTAGONIST 1 (RAN1); (Schaller and Bleecker, 1995; Hirayama et al., 1999; Binder et al., 2010). Ethylene binding causes inactivation of both the receptor and the downstream Raf-like Ser/Thr protein kinase CONSTITUTIVE TRIPLE RESPONSE 1 (CTR1), a negative regulator of ethylene signaling (Kieber et al., 1993; Huang et al., 2003). In the absence of ethylene (in air), CTR1 phosphorylates and inhibits ETHYLENE INSENSITIVE 2 (EIN2), an ER membrane-localized Nramp homolog and a positive regulator of ethylene responses (Ju et al., 2012). That allows Skp1/Cullen/F-box (SCF) E3 ubiquitin ligase complexed with the EIN2-TARGETING PROTEIN 1 (ETP1) and ETP2 F-box proteins to target EIN2 for 26S proteasome-mediated degradation (Qiao et al., 2009). In parallel, the TFs and positive regulators of ethylene-induced gene expression EIN3, ETHYLENE-INSENSITIVE3-LIKE 1 (EIL1) and EIL2 are targeted for ubiquitination by an EBF1- and EBF2- (F-box proteins) containing SCF E3 complex (Guo and Ecker, 2003; Potuschak et al., 2003; Gagne et al., 2004; Binder et al., 2007; An et al., 2010). The ethylene-induced inactivation of all ethylene sensors leads to the attenuation of CTR1 kinase activity, dephosphorylation of EIN2 and cleavage of its C-terminus (EIN2-CEND). In the cytoplasm, EIN2-CEND inhibits translation of EBF1 and EBF2 by targeting their mRNAs to the processing bodies and subsequent degradation (Li et al., 2015; Merchante et al., 2015). This then upregulates the levels of their targets EIN3, EIL1 and EIL2. EIN2-CEND is also translocated to the nucleus (Qiao et al., 2012; Wen et al., 2012), where it recognizes EIN2 NUCLEAR ASSOCIATED PROTEIN 1 (ENAP1) and promotes EIN3 binding to specific (ENAP1-recognized) chromatin regions by upregulating histone acetylation (Zhang et al., 2017). Specific histone posttranslational modifications seem to be important for ethylene-regulated gene expression (Binder, 2020; and references therein). EIN2-CEND-stabilized EIN3, EIL1 and EIL2 upregulate the expression of ethylene induced genes, including the TFs ETHYLENE RESPONSE DNA BINDING FACTOR 1 (EDF1), EDF2, EDF3 and EDF4 and ETHYLENE RESPONSE FACTORs (ERF)s. Activated ERFs initiate a transcriptional cascade leading to the activation of many ethylene-responsive genes by binding to specific cis-acting GCC box and DRE elements depending on whether the stress conditions are abiotic or biotic (reviewed in Müller and Munné-Bosch, 2015). For more detailed information about the ethylene signaling pathway, see the recent review by Binder (2020).
The fact that two ethylene sensors belong to HKs indicates possible signaling crosstalk with the MSP pathway. However, ethylene sensors from both subfamilies mediate ethylene signal transduction primarily through the CTR1/EIN2/EIN3 pathway and it took nearly two decades until evidence pointing to intense cytokinin/ethylene signaling crosstalk was revealed (Figure 1). ETR1 (containing a receiver domain) was shown to interact with AHP1, AHP2, AHP3 and AHP5 (Urao et al., 2000; Scharein et al., 2008; Zdarska et al., 2019). ETR1-dependent phosphorylation of type-B ARR2 and activation of the type-A ARR6 promoter was demonstrated in Arabidopsis protoplasts (Hass et al., 2004; Cho and Yoo, 2007). The arr2 mutant is less sensitive to treatment with the ethylene precursor 1-aminocyclopropane-1-carboxylic acid (ACC) probably due to a positive effect of ARR2 on the expression of ERF1, an ethylene-responsive member of the ERF/AP2 transcription factor family (Hass et al., 2004). A recent study showed that ETR1-mediated ethylene signaling controls RAM size via ARR3 independently of EIN2 and in a distinct way from canonical CTR1/EIN2/EIN3 signaling (Street et al., 2015; Zdarska et al., 2019). Importantly, canonical ethylene signaling seems to control the sensitivity of MSP to cytokinins, possibly via ethylene-regulated expression of type-B ARR10 (Zdarska et al., 2019). In rice, the ethylene receptor OsERS2 physically interacts with cytosolic HK MHZ1/OsHK1 (homolog of Arabidopsis AHK5, see below) through its GAF domain in an ethylene-dependent manner and this interaction inhibits MHZ1/OsHK1 kinase activity. OsHK1 phosphorylates the response regulator OsRR21 via the phosphotransfer proteins OsAHP1 and OsAHP2, which are both required for ethylene-mediated root-growth inhibition (Zhao et al., 2020). Considering the strong regulation of ethylene biosynthesis by cytokinins (reviewed in Zdarska et al., 2015) MSP output must be considered as a combined output of both cytokinin and ethylene signals.
Light is an essential environmental factor influencing plant development and adaptive responses. Photochemically active light receptors such as phytochromes, cryptochromes, phototropins, ZEITLUPE (ZTL) and the UV-B RESISTANCE 8 (UVR8) family proteins affect plant behavior in response to both quantity and quality of light. The individual signaling branches mediating responses to a wide range of wavelengths as well as their interplay has been extensively studied (e.g., Quail et al., 1995; Rockwell et al., 2006; Li et al., 2011; Wang Q. et al., 2018). Since light signaling is a very broad topic, we focus here only on the direct interaction of phytochrome-mediated light signaling components with MSP.
Phytochromes are light-regulated Ser/Thr kinases, mediating plant response to red (R) and far-red (FR) light. Phytochromes are synthesized as light-insensitive apoproteins and the functional, i.e., light photoswitchable holoproteins (phys) are assembled following binding with the light-sensitive, linear tetrapyrrole chromophore cofactor phytochromobilin, that is imported to the cytosol from plastids (Terry et al., 2002; Wang, 2015). Upon absorbing R-light the phytochromes switch from the inactive (R-light absorbing) Pr form to the active (FR-light absorbing) Pfr form. The only phy activated by FR (thanks to partial overlap between Pr and Pfr absorption spectra) in Arabidopsis is phyA (Casal et al., 2014; Ballaré and Pierik, 2017). Activated phys translocate to the nucleus either alone [as for phytochrome B (phyB)] or complexed to phy-transporting proteins (as in the case of phyA). The nuclear-located phys control light-responsive genes through two main routes. The first is based on phosphorylating PHY-INTERACTING FACTORs (PIFs), thus targeting them for subsequent degradation via the CUL4COP1–SPA E3 ubiquitin ligase (Zhu et al., 2015). PIFs control both skotomorphogenesis (by activating genes expressed during the dark phase) and photomorphogenesis (by inhibiting light-induced genes). The second pathway is controlled by phytochrome-mediated inhibition of transcriptional repressors CONSTITUTIVE PHOTOMORPHOGENIC (COP), DE-ETIOLATED (DET), and FUSCA (FUS; Huang et al., 2014). COP1 interacts with SUPPRESSOR OF PHYA (SPA) proteins, forming an E3 ubiquitin ligase complex that targets light-responsive TFs for 26S proteasome-mediated degradation. Activated phys inhibit the COP1-SPA, allowing accumulation of photomorphogenesis-promoting TFs including ELONGATED HYPOCOTYL 5 (HY5) (Huang et al., 2014; Lu et al., 2015; Sheerin et al., 2015; Figure 1). More details on the complex issue of phytochrome signaling and its interaction with hormonal signaling can be found in excellent reviews by Wang (2015); Xu et al. (2015) and De Wit et al. (2016).
One of the first pieces of evidence for direct crosstalk between MSP and light signaling was presented by Sweere et al. (2001). ARR4, a type-A ARR, interacts with phyB and stabilizes its active Pfr form, preventing dark reversion to the inactive Pr form, thus making plants more sensitive to red light (Sweere et al., 2001). ARR4 action on phyB and ARR4-controlled regulation of photomorphogenesis is dependent on its conserved phosphorylatable Asp 95, and this ARR4-mediated stabilization of phyB is reversed in the presence of cytokinins, providing a direct functional link between MSP and light signaling (Mira-Rodado et al., 2007).
Expression of the constitutively active HK CKI1 was found to be under the control of phyA, probably via PHY-INTERACTING FACTOR 1 (PIF1) and CIRCADIAN CLOCK ASSOCIATED 1 (CCA1). Plants defective in phyA and HEME OXYGENASE1 (HO1), whose product is involved in the production of phytochromobilin, show misregulation of CKI1 expression, associated with attenuation of MSP responsiveness to cytokinins and occurrence of cytokinin-related phenotypes (Dobisova et al., 2017). Light-controlled phyA-dependent transcriptional regulation of CKI1 expression is a conceptually novel signaling mechanism in plants. Here, an environmental regulatory signal (light) controls the activity of (MSP) signaling pathway by transcriptional regulation of the constitutively active HK, thus controlling MSP sensitivity to its primary signal, cytokinin (Dobisova et al., 2017). On the other hand, exogenous cytokinins were reported to negatively modulate phyA expression (Cotton et al., 1990; Brenner et al., 2012). The negative impact of cytokinins on phyA transcription is fine-tuned by up-regulating both PHYTOCHROME A SIGNAL TRANSDUCTION 1 (PAT1), a positive regulator of phyA signaling and SPA1, its negative regulator (Brenner et al., 2005, 2012). Furthermore, cytokinins suppress the function of the E3 ubiquitin-protein ligase COP1, which mediates ubiquitination and subsequent proteasomal degradation of HY5, associated with repression of photomorphogenesis in darkness (Srivastava et al., 2015) and increased accumulation of the bHLH family proteins PIFs and EIN3 (Alabadí and Blázquez, 2008; Zhong et al., 2009; Figure 1). HY5 acts as a downstream intermediate in the CRYPTOCHROME 1 (CRY1; photoreceptor absorbing blue light) signaling pathway (Vandenbussche et al., 2007). Both the cytokinin (via ARR4) and CRY pathways upregulate HY5 protein levels that consequently regulates the transcription of many genes by binding directly to their cis-regulatory elements. Thus, regulation of the stability of HY5 protein has been suggested to be a point of convergence between both signaling pathways (Shin et al., 2007, 2013; Vandenbussche et al., 2007; Gangappa and Botto, 2016).
Apart from the mutual light-MSP interactions, a positive role was shown for light in ethylene signaling, as well as light-dependent stimulation of ETR1 and EIN4 expression. On the other hand, ETR2 and ERS2 gene expression was attenuated during the exposure to light (Grefen et al., 2008). We saw earlier how the ethylene receptor (ETR1) and downstream MSP components (AHP1, AHP2, AHP3, and AHP5) (Hass et al., 2004; Scharein et al., 2008; Street et al., 2015; Zdarska et al., 2019; Zhao et al., 2020) are linked. Thus, the light-controlled expression of ethylene sensor-encoding genes might represent another way of MSP-mediated integration of light with plant hormone signaling pathways.
In Arabidopsis thaliana, several histidine kinases have been identified, namely AHK1, AHK5, and CYTOKININ-INDEPENDENT 1 (CKI1) that do not mediate hormone responses (Figure 1). The sensory histidine kinase AHK1 was previously shown to transduce the external osmolarity signal (Urao et al., 1999; Tran et al., 2007) and its exact role in cytokinin signaling has not been elucidated. CKI1 was identified by Kakimoto (1996) using activation mutagenesis, and was proposed to act as a cytokinin sensor. Later on, however, CKI1 was found to be a constitutively-active HK, activating MSP signaling in a cytokinin-independent fashion (Hwang and Sheen, 2001; Yamada et al., 2001; Hejátko et al., 2009), and controlling many aspects of plant development including root growth, vascular tissue formation in the inflorescence stem and female gametophyte development (Hwang and Sheen, 2001; Pischke et al., 2002; Hejátko et al., 2003, 2009; Deng et al., 2010; Yuan et al., 2016; Liu et al., 2017). AHK5 has been shown to play a key role in integrating multiple signals in guard cells – independent of ABA signaling via H2O2 homeostasis – possibly acting as redox sensor (Desikan et al., 2008). In terms of expression patterns, CKI1 is expressed in female gametophytes, developing seeds, shoot apical meristem, shoot and root vasculature and the lateral root cap, whereas AHK1 transcript levels are most abundant in roots and is transcriptionally regulated by osmotic stress (Urao et al., 1999; Pischke et al., 2002; Hejátko et al., 2003, 2009; Dobisova et al., 2017). The AHK5 transcript was present in roots, flowers and siliques, and at low levels in leaves, where it plays a unique role in stomatal signaling (Desikan et al., 2008; Horak et al., 2011). Several researchers have shown that these non-hormone responsive HKs are necessary for modulating the plant responses to stress, and is described in detail below in Section “Sensing Abiotic Stresses via Multistep Phosphorelay.”
Abscisic acid has been known for decades as an essential plant hormone for plant development and stress responses; for instance, ABA is elevated when plants suffer from decreased water potential, as well as during seed maturation and fruit ripening (Wright, 1977; Rodríguez-Gacio Mdel et al., 2009). The ABA signaling network includes the receptors PYRABACTIN RESISTANCE 1 (PYR1)/PYR1-LIKE (PYLs)/REGULATORY COMPONENTS OF ABA RECEPTORS (RCARs), which, upon ABA binding, form a complex with TYPE 2C PROTEIN PHOSPHATASES (PP2Cs), resulting in the PP2C inactivation (Ma et al., 2009; Park et al., 2009). This, in turn, releases members of SNF1-RELATED PROTEIN KINASEs 2 (SnRK2s) subfamily (see below), which get activated by autophosphorylation (Belin et al., 2006) and further phosphorylate several transcription factors such as ABA INSENSITIVE 4 (ABI4), ABI5, ABA-RESPONSIVE ELEMENT BINDING FACTORS (ABFs) as well as membrane ion channel proteins like SLOW ANION CHANNEL-ASSOCIATED1 (SLAC1) and potassium channel protein 3-KETO-ACYL-COA THIOLASE 1 (KAT-1) as well as MITOGEN-ACTIVATED PROTEIN KINASE1 (MPK1) and MPK2 (reviewed in Fujita et al., 2013; Yu et al., 2016; Dejonghe et al., 2018) (Figure 1). Based on their ability to be activated by ABA, SnRK2s could be divided into three subclasses: Subclass I – not responsive to ABA (SnRK2.1, SnRK2.4, SnRK2.5, SnRK2.9, and SnRK2.10), Subclass II - weakly activated by ABA (SnRK2.7 and SnRK2.8) and Subclass III - strongly activated by ABA (SnRK2.2, SnRK2.3, and SnRK2.6) (Ng et al., 2014). Subclass III kinases are essential components of ABA signaling pathway, as indicated by impaired ABA responses in triple mutants lacking SnRK2.2, SnRK2.3, and SnRK2.6 (Fujii and Zhu, 2009; Fujita et al., 2009; Nakashima et al., 2009).
Another regulatory circuit affecting SnRK activity employs TARGET OF RAPAMYCIN (TOR) kinase (Wang P. et al., 2018; Belda-Palazón et al., 2020). Under unstressed (growth favorable) conditions, TOR signaling complex represses ABA-mediated stress signaling via phosphorylation of PYL receptors. This phosphorylation leads to SnRK2 kinase inactivation, which associates with disruption of PYL and PP2C phosphatase effector function, thus promoting plant growth. In the presence of stress, ABA signaling represses function of TOR complex via phosphorylation of RAPTOR, the regulatory subunit of TOR, leading to stress responses and growth inhibition (Wang P. et al., 2018). Interestingly, the growth promoting role has been attributed to SnRK2s, too. In the absence of ABA, SnRK2s-containing complexes recognize SnRK1, a negative regulator of TOR. That allows TOR activation and growth under non-stress (growth-promoting) conditions (Belda-Palazón et al., 2020). SnRK1, via its α subunit, has been shown to interact and phosphorylate RAPTOR1B, one of two Arabidopsis RAPTOR/KOG1 homologs, which seems to control energy-demanding processes including translation. Surprisingly, SnRK1 might also be involved in regulating photosynthesis via phosphorylaton of chloroplast proteins (Nukarinen et al., 2016). The ability of SnRK1 to act upstream of TOR has been also proposed in stress- (nutrient or energy deficiency) induced autophagy (Soto-Burgos and Bassham, 2017).
The ABA-signaling pathway is an essential regulator of stomatal movement which is either dependent or independent on Ca2+ levels (McAinsh and Hetherington, 1998; Webb et al., 2001; Siegel et al., 2009). Previously was reported that increases in Ca2+ are the components of the guard cell ABA-nuclear as well as ABA-turgor signaling pathway (Webb et al., 2001). However, part of Ca2+ signaling components are found in the guard cells to act as a separate Ca2+-independent ABA signaling pathway (Allan et al., 1994; Blatt and Grabov, 1997; Webb et al., 2001). Ca2+ elevation can accelerate the stomata closure by enhancing plasma membrane SLOW ANION CHANNEL-ASSOCIATED 1 (SLAC1) and cytoskeletal rearrangement (Waidyarathne and Samarasinghe, 2018). Nevertheless, Ca2+ increase seems not to be the only essential component of this process, thus the exact role of Ca2+ in ABA signaling needs to be explained (Waidyarathne and Samarasinghe, 2018).
Similarly to cytokinin and ethylene, ABA responses also include chromatin-mediated control of gene expression by regulation of chromatin-remodeling complexes by, for instance, the SWI/SNF subgroup complexes forming around the chromatin-remodeling ATPase BRAHMA (BRM) (Chinnusamy and Zhu, 2009; Yaish et al., 2011; Han and Wagner, 2014; Han et al., 2015). SnRK2s and PP2Cs directly target conserved phosphorylation site in the C-terminal region of BRM (Peirats-Llobet et al., 2016). Whereas PP2CA dephosphorylates BRM, likely as a repressive mechanism of BRM-dependent modulation of ABA responses, SnRK2s phosphorylate BRM to release BRM-mediated repression of ABI5 expression (Peirats-Llobet et al., 2016).
Since ABA interacts tightly with MSP signaling during the abiotic stress response, the mutual crosstalk might occur at several levels including regulation of phosphorylation of individual signaling components as well as regulation of dynamic chromatin state (Chinnusamy and Zhu, 2009; Yaish et al., 2011; Han and Wagner, 2014). These interactions are described further in the text.
Altogether, the MSP pathway is not just the cytokinin signaling transduction system, but it acts as a signaling hub, integrating both intrinsic (mostly hormonal) regulation with that from environmental signals, including light, osmoregulation, and temperature (Figure 1). The importance of integration of cytokinin, ethylene, ABA, and light signaling pathways is what underlies the ability of plants to display such plasticity in continual adjustment to the changing environment.
Stress, in general, affects the metabolism of cytokinins, ABA, and ethylene, which in turn interact with specific kinases to regulate many stress-related biological processes ranging from regulation of shoot growth under stress to stomata movement. Drought results in the reduction of cytokinin content by modulating cytokinin metabolism and stimulating ABA and ethylene contents in a wide range of terrestrial plants (e.g., Dobra et al., 2010; Guo and Gan, 2011; Nishiyama et al., 2011; Macková et al., 2013; Todaka et al., 2017; Figure 2). Even though there is a correlation between hormone metabolism and MSP activity, a significant set of stress responses take place independently of the hormonal pool (O’Brien and Benková, 2013). In this review, we focus on the role of individual MSP components and molecular links in pathways for abiotic stress responses (Table 1). Detailed descriptions of the stress-related changes in hormonal metabolism are left out as they are beyond the scope of this review.
Figure 2. Integration of the different components of ethylene, cytokinin, ABA and light signaling in multistep phosphorelay during drought and osmotic/salt stress. (A) Drought stress stimulates ABA and ethylene content while reducing the endogenous levels of cytokinins. Both cytokinin-responsive HKs AHK2, AHK3, and AHK4 as well as cytokinin-independent AHK1 and AHK5 contribute to the drought-regulated MSP activity. Strong crosstalk (mostly mutually negative) occurs between MSP and canonical ABA signaling. Ethylene response factors (ERFs) are also induced and play mostly positive role in stress responses. (B) Like drought, osmotic/salt stress also stimulates ABA and ethylene production, thus activating ABA and ethylene signaling components, while downregulating cytokinin levels and the expression of its downstream signaling components. Also, expression of ETR1 is reduced, enabling the continued activity and participation of downstream components of ethylene signaling (EIL1, EIN3, and ERFs) in the activation of different response factors necessary for the osmotic/salt stress response. Phytochromes are also implicated to be involved in drought and osmotic/salt stress responses, particularly phyB which was shown to enhance osmotic and drought tolerance. See the main text for a detailed description of each signaling pathway and their crosstalk in response to stress. Color code: MSP signaling is depicted in green, ethylene signaling in blue, light signaling in ochre, abscisic acid in purple. The His/Asp phosphorylation is depicted by circled P in bright yellow, Ser/Thr/Tyr phosphorylation in orange. Created with BioRender.com.
Abscisic acid mediates one of the fastest plant adaptations to drought, i.e., stomata closure to control the trade-off between CO2 uptake and water loss by transpiration (Sah et al., 2016; Vishwakarma et al., 2017). However, ABA controls also mid- and long-term plant adaptations to abiotic stresses including control of plant architecture, wherein ABA tightly interacts with cytokinins. The current model predicts that cytokinin-regulated MSP attenuates the expression of ABA-inducible genes involved in the stress response. Stress-induced ABA accumulation in turn downregulates cytokinin biosynthesis via the MYB2 TF, relieving the repression on MSP and activation of ABA- and stress-inducible genes (Li et al., 2016). ABA-mediated inhibition of cytokinin signaling initiates reshaping of the plant body by downregulating growth in the shoot but accelerating root growth. That allows the plant to diminish water loss while increasing water uptake from deeper layers of the soil (Li et al., 2016). In line with that, MSP signaling mutants, including mutants defective in cytokinin sensors AHK2, AHK3, and AHK4 and type-B ARRs ARR1, ARR10, and ARR16 are hypersensitive to ABA and show higher drought resistance (Tran et al., 2007, 2010; Nguyen et al., 2016). Both ABA and drought were shown to downregulate expression of ARR1, ARR10, and ARR12 (Nguyen et al., 2016). ABA also downregulates ARR2 but not AHK3 or AHK4 (Takatsuka and Umeda, 2019), and a possible role for ABA in the control of nucleocytoplasmic partitioning of AHP2 was reported (Marchadier and Hetherington, 2014). Interestingly, the osmosensor AHK1 is not a negative, but rather a positive regulator of the ABA-mediated stress response (Tran et al., 2007), suggesting certain specificity at the level of signals initiating MSP-regulated (drought) stress response (Hai et al., 2020).
The negative interaction between ABA and MSP activity is mediated not only at the level of ABA-controlled downregulation of cytokinin biosynthesis, but also at the level of interaction between components of canonical ABA signaling and MSP. ABA-activated ABI4 binds the promoters and downregulates ARR6, ARR7, and ARR15; single and multiple mutant lines arr4, arr6, arr7, and arr15 were shown to be hypersensitive to ABA (Jeon et al., 2010; Wang et al., 2011; Huang et al., 2017). SnRK2.2, SnRK2.3, and SnRK2.6 phosphorylate several Ser residues of ARR5, the type-A ARR and negative regulator of MSP signaling. This leads to stabilization of the ARR5 protein, thus enhancing ABA response and drought tolerance by suppressing the cytokinin signaling (Huang et al., 2018; Figure 2). On the other hand, the phosphoproteomic study performed by Dautel et al. (2016) proposed an AHK2/AHK3-dependent phosphorylation of Thr6 and Tyr19 of KIN10, one of the two subunits of SnRK1, acting in energy stress (Baena-González and Sheen, 2008). SnRK1 down-regulation has been previously linked to cytokinin and auxin signaling based on global gene regulation by KIN10 (Radchuk et al., 2006; Baena-González et al., 2007), whereas ethylene signaling was negatively regulated by SnRK1 phosphorylation-mediated inactivation of EIN3 (Kim et al., 2017) leading to a growth-defense trade-offs model via cell cycle regulations (Eichmann and Schäfer, 2015; Filipe et al., 2018). The negative relationship between ABA and cytokinin levels/signaling is bi-directional. Upregulation of cytokinin biosynthesis via upregulation of AtIPT8 resulted in ABA insensitivity in seed germination (Wang et al., 2011). Furthermore, when endogenous cytokinins are elevated, ABA was unable to downregulate genes for type-A ARRs ARR4, ARR5, and ARR6 that physically interact with ABI5 and downregulate ABI5 expression (Wang et al., 2011). The lower sensitivity to ABA under high endogenous cytokinin levels is likely mediated by the cytokinin-responsive type-B ARRs ARR1, ARR11, and ARR12 that physically interact with SnRK2s and repress the kinase activity of SnRK2.6 (Huang et al., 2018). At the transcriptional level, the cytokinin-dependent regulation of ABA signaling might be mediated through ARR10, which was found to bind promoters of numerous members of ABA signaling (Zubo et al., 2017). Since canonical ABA signaling stimulates the expression of many transcription factors essential for stress responses and seed development (reviewed in Nakashima and Yamaguchi-Shinozaki, 2013; Kumar et al., 2019), the interaction with MSP components contributes to fine-tuning of the final stress response and regulates developmental processes (Kieber et al., 1993; Clark et al., 1998; Ju et al., 2012).
In the response to salt stress, cytokinins act through ARR1 and ARR12 to repress expression of the Arabidopsis HIGH-AFFINITY K+ TRANSPORTER 1;1 (AtHKT1;1) which is responsible for removing sodium ions from root xylem. Cytokinins were also shown to regulate salt stress−induced expression of the type−A response regulator ARR5 predominantly via ARR1 and ARR12, revealing the action of particular MSP components in the roots to control sodium accumulation in the shoots (Mason et al., 2010).
Interestingly, cold transiently stimulates the expression of ARR5, ARR6, ARR7, and ARR15 similarly to dehydration (Jeon et al., 2010; Kang et al., 2012), most probably to attenuate cytokinin signal transduction and suppress growth. Shi et al. (2012) found that ethylene biosynthesis and signaling negatively regulates the freezing stress response in Arabidopsis by repressing cold-inducible C-REPEAT BINDING FACTORs (CBFs) and the type-A ARR genes ARR5, ARR7, and ARR15. This ethylene-mediated repression was supposed to be mediated by direct binding of EIN3 to the promoters of type-A ARRs, thus potentially representing another mechanistic link between canonical ethylene signaling and MSP during plant desiccation (Figures 2, 3).
Figure 3. Integration of the different components of ethylene, cytokinin, ABA and light signaling pathways in multistep phosphorelay in temperature and light responses. (A) Heat stress: similarly to drought and osmotic stress, heat stress also enhances ABA and ethylene levels while downregulating cytokinin levels and the expression of cytokinin receptors (AHK2, 3, 4). ETR1 and ERF1 play a positive role in heat tolerance; however, downstream components of ABA (PYR/PYL/RCAR, PP2Cs, SnRK2s, and ABFs) are downregulated. (B) Cold stress: low temperature reduces the levels of cytokinin, but activates cytokinin receptors (AHKs), putatively in a cytokinin-independent manner. Several downstream components of the cytokinin signaling pathway are also activated (AHP2,3,5, B-ARR 1, A-ARR 5, 6, 7, 15) by cold temperature and correlated with enhanced cold tolerance in Arabidopsis thaliana (modified from Zdarska et al., 2015). phyB was demonstrated to be a temperature sensor, which interacts with ARR4, the key regulator providing a molecular link between MSP and light (and possibly temperature) signaling. Light response: light can also attenuate the levels of ethylene. ETR1 and EIN4 expression are dependent on light stimulation. ETR1 also genetically interacts with both phyA and phyB that govern germination and the growth response to various light regimes. Cytokinins were also shown to maintain photoprotective mechanisms during high light stress, mediated by AHK2 and AHK3. See the main text for a detailed description of each signaling pathway and their crosstalk in response to stress. Color code: MSP signaling is depicted in green, ethylene signaling in blue, light signaling in ochre, abscisic acid in purple. The His/Asp phosphorylation is depicted by circled P in bright yellow, Ser/Thr/Tyr phosphorylation in orange. Created with BioRender.com.
AHK1 gain-of-function mutations revealed that the osmo-responsive AHK1 was a positive regulator of drought and salt stress responses acting upstream of ABA signaling (Tran et al., 2007) (Figure 2). Disturbed up-regulation in the ahk1 mutant of many stress- or ABA-inducible genes, including ABSCISIC ACID RESPONSIVE ELEMENTS-BINDING PROTEIN 1 (AREB1), NAC DOMAIN CONTAINING PROTEIN (ANAC), and DRE-BINDING PROTEIN 2A (DREB2A) transcription factors and their downstream genes may explain the increased stress tolerance of AHK1 overexpressing plants (Tran et al., 2007). Moreover, AHK1 was shown to control stomatal density as well as the transcription of several stress-responsive genes. ahk1 mutants have a higher transpiration rate and reduced ABA sensitivity, resulting in higher susceptibility to uncontrolled soil drying (Kumar et al., 2013).
Another experiment showed attenuated expression of AHK2 in the first hours after exposure to reduced water potential (Figure 2; Kumar and Verslues, 2015). The examination of ahk2-2 mutant lines showed hypersensitive response to salt stress, while root elongation in ahk3-3 mutants was increased following transfer to low water potential media when compared to wild type (Kumar and Verslues, 2015). These results show the specific role of individual HKs in osmotic stress acclimation and provide evidence for the specificity of individual MSP components to different types of stress.
AHK5 was shown to mediate ABA-independent stomata closure in response to H2O2 produced by the NADPH oxidase AtrbohF (Desikan et al., 2006), which is induced by abiotic stimuli including darkness, nitric oxide (NO), and ethylene (Desikan et al., 2008). AHK5 inhibits root elongation by negatively regulating the ABA and ethylene signaling pathways (Iwama et al., 2007). On the other hand, AHK5 was also demonstrated to be a negative regulator of salinity tolerance, while providing plants with drought tolerance by promoting ABA-independent stomatal closure by maintaining H2O2 homeostasis in guard cells (Desikan et al., 2008; Pham and Desikan, 2012; Figure 2). Moreover, AHK5 is required for full immunity toward the necrotrophic fungus Botrytis cinerea and the virulent bacterium Pseudomonas syringae pv. tomato DC3000. It thus acts as an integrator of both abiotic and biotic stress responses in Arabidopsis (Pham and Desikan, 2012; Pham et al., 2012).
Nitric oxide has been recently characterized as a plant growth regulator involved in the control of many important developmental and adaptive responses, including the abiotic stress response (reviewed e.g., in Sami et al., 2018). Cytokinins induce NO production via MSP signaling, and cytokinin-induced NO contributes substantially toward plant morphological responses to cytokinin (Tun et al., 2008). Importantly, NO was shown to S-nitrosylate the conserved Cys 115 of AHP1, thus impairing its ability to mediate phosphotransfer to ARR1. This is an example of a mechanism by which the redox signal is integrated into cytokinin signaling – highlighting the complex coordination underlying plant growth and development (Feng et al., 2013). NO seems to antagonize not only cytokinin, but also ABA signaling. NO biosynthetic mutant nia1 nia2 noa1-2 is hypersensitive to ABA, leading to highly efficient ABA-mediated control over stomata closure (Lozano-Juste and León, 2010a) and NO production under growth-favorable conditions represses inhibition of seed developmental transitions by ABA (Lozano-Juste and León, 2010b). Increase in NO levels post-translationally modifies members of the ABA receptor PYR/PYL/RCAR family by S-nitrosylation or tyrosine nitration at cysteine residues (Castillo et al., 2015). Tyrosine nitration under conditions in which NO is produced was proposed as a rapid mechanism limiting ABA signaling (Castillo et al., 2015). On the other hand, ABA signaling in guard cells includes H2O2 and NO production, which seems to be necessary for the ABA-induced stomata closure (Castillo et al., 2015). Thus, NO appears as a multifaceted plant growth regulator mediating direct modifications of key components in several hormone signaling pathways.
The ability of the root to grow toward higher water potential (hydrotropism) is an important physiological adaptation trait of plants to drought. The recent findings of Chang et al. (2019) have uncovered the importance of type-A ARRs in controlling root hydrotropism. ARR16 and ARR17 were found to be upregulated at the side of the root tip that “faces” lower water potential, resulting in increased cell division in the meristem zone and consequent bending of the roots toward the opposite side – in the direction of higher water potential.
Furthermore, various types of abiotic stresses including cold, osmotic stress, salt, and drought differentially regulate the expression of several individual MSP components (Argueso et al., 2009). As mentioned above, some of these regulations can be attributed to stress-induced ABA, however, the molecular mechanisms behind most of these regulations remain to be clarified.
Ethylene signaling too affects salt and osmotic stress responses. The receptors ETR1 and EIN4 inhibit, and ETR2 stimulates, seed germination under salt stress (Zhao and Schaller, 2004; Wilson et al., 2014b). It has been demonstrated that seeds of the loss-of-function etr1-6 and etr1-7 mutants have a higher germination rate than wild-type seeds in the dark or following FR illumination (that inhibits seed germination in the WT) indicating a negative role for ETR1 in the control of germination (Wilson et al., 2014a). The authors suggest that ETR1 may genetically interact with phyA and phyB to govern germination and growth under various light conditions (Wilson et al., 2014a; Figure 3). The ETR1 receptor may modulate ABA sensitivity even when ethylene does not bind to this receptor (Wilson et al., 2014a,b). ETR1 and ETR2 indirectly affect the expression of ABA signaling genes, without a requirement for canonical ethylene signaling (Bakshi et al., 2015, 2018). These findings show that ETR1 and ETR2 act independently of ethylene signaling to affect seed germination under salt stress by interacting with ABA signaling (Wilson et al., 2014b). Another crosstalk between ABA and ethylene was associated with TOR protein kinase (Dong et al., 2015). Inhibition of TOR kinase function led to increased expression of ethylene signaling components such ethylene response factors and ethylene responsive element-binding factors while down-regulating type-A response regulators (Dong et al., 2015). In line with that, TOR protein kinase has been shown to act as a central regulator of cell growth by integrating nutrient, energy, and growth factors in photosynthetic organisms (Schepetilnikov and Ryabova, 2018; Jamsheer et al., 2019).
Early osmotic stress response is integrated by ABA signaling through the hyperosmotic activation of RAF-like mitogen-activated protein kinases (MAPKKKs) that further activate downstream ABA-unresponsive and ABA-activated SnRK2s (Katsuta et al., 2020; Lin et al., 2020; Lozano-Juste et al., 2020; Soma et al., 2020). Fast response to osmotic stress is, however, also mediated by ABA-unresponsive subclass I and III SnRK2s independently to ABA (Boudsocq et al., 2007).
Temperature is another fundamental environmental factor, with 10–30°C being optimum for the growth of most higher plants (Źróbek-Sokolnik, 2012). Temperatures below and above this optimum range are referred to as cold or heat stress, respectively (Larkindale et al., 2007; Miura and Furumoto, 2013). Both extremes may have fatal consequences for the plant (for details see, e.g., Xiong et al., 2002; Sung et al., 2003; Kaplan et al., 2004; Ahuja et al., 2010; Johnová et al., 2016).
In a response to adverse ambient temperatures, plants reprogram their transcriptome, metabolome, and proteome as well as their hormonal status to adapt their growth to the unfavorable temperature conditions (Stavang et al., 2009; Mittler et al., 2012). Cytokinin content is generally reduced by cold shock, which seems to be related to the reallocation of energy resources from growth toward defense. Prolonged cold treatment (for more than 3–7 days) is accompanied by plant acclimation (e.g., in winter wheat cultivars), associated with elevation of cytokinin content (Kosová et al., 2012). This finding is in line with the positive effect of exogenous cytokinins on freezing tolerance of Arabidopsis (Jeon et al., 2010; Maruyama et al., 2014). Thus, the role of cytokinins in cold responses and freezing tolerance seems to depend on the severity and phase of the stress response. Additionally, environmental stimuli such as temperature and water stress alter the phosphorylation status of MSP components partly independent of hormonal status suggesting that histidine kinases (and/or thermo-responsive phytochromes, see below) function as abiotic stress sensors, as shown for AHK1 (Tran et al., 2007; Jeon et al., 2010; O’Brien and Benková, 2013).
Even if enhanced freezing tolerance of ahk2 ahk3 and ahk3 ahk4 double mutants seems to be in accordance with the decreased cytokinin content under cold stress, it may be related, at least partially, to their low growth rate (Jeon et al., 2010). Cytokinin-independent (but nevertheless requiring functional cytokinin-responsive AHKs) upregulation of genes encoding the type-A response regulators ARR5, ARR6, ARR7, and ARR15 was reported as an early cold stress response (Jeon et al., 2010). Interestingly, the enhanced freezing tolerance observed in arr7 and ahk2 ahk3, ahk3 ahk4 mutants seems to act independently of the C-REPEAT-BINDING FACTOR/DRE-BINDING FACTORs (CBF/DREBs)-mediated cold acclimation pathway. Based on the hypersensitive response of ahk2, ahk3, and arr7 mutants, as well as the insensitivity of ARR7 overexpression line to ABA, the authors propose that the MSP-mediated cold response is mediated via negative regulation of ABA signaling by AHK/ARRs, thus following the general scheme of negative cytokinin/ABA interaction as in the drought response described above (Figure 1). Moreover, ARR1 was shown to function as a positive factor for cold signaling, acting downstream of AHK2, AHK3, and AHPs (Jeon and Kim, 2013; Figure 3). Interestingly, the majority of cold-responsive genes that are differentially regulated in ahk2 ahk3 compared to WT, do not seem to be regulated via CBF3 or ARR1, suggesting the existence of yet unidentified (either direct or indirect) regulatory mechanisms, mediating the AHK2/3-dependent cold response (Jeon and Kim, 2013).
The CRF signaling branch of MSP is also involved in the response to cold stress, but also to heat, salt and oxidative stress, as well as elevated hydrogen peroxide content. All these stresses stimulate the activity of the CRF6 promoter. Overexpression of CRF6 diminished oxidative stress impact, improving photosynthesis and stimulating root growth in Arabidopsis and tomato plants (Inzé et al., 2012; Zwack et al., 2013, 2016; Gupta and Rashotte, 2014). Other CRFs were also observed to be regulated by cold, enabling freezing tolerance by either inducing CRF4 or altering lateral root development in response to low temperature through CRF2 and CRF3 (Shi et al., 2014; Zwack and Rashotte, 2015; Jeon et al., 2016).
Light irradiance is an essential environmental factor influencing a wide range of physiological and growth aspects of the plant, and is tightly linked to plant hormonal status. An intimate link between light and cytokinins has been recognized for a long time now (reviewed in Cortleven and Schmülling, 2015; Zdarska et al., 2015). However, light can also act as a stressor, affecting both abiotic and biotic stress responses in plants (Roeber et al., 2020).
Cytokinins were shown to play an important role in photoinhibition induced by high dose irradiation. AHK2 and AHK3 and their downstream targets ARR1 and ARR12 were suggested to mediate cytokinin-dependent protection of the photosynthetic apparatus during high light stress (Cortleven et al., 2014; Cortleven and Schmülling, 2015). Cytokinins (through AHK3) were also found to modulate a novel type of stress called photoperiod stress, identified in plants with reduced endogenous cytokinin content or disturbed cytokinin signaling, and in circadian clock mutants (Nitschke et al., 2017). Photoperiod stress occurs due to abrupt changes in the alternation of light and dark periods, inducing symptoms of stress in Arabidopsis plants, including JA-dependent cell death and downregulation of the key circadian clock regulators CCA1 and LATE ELONGATED HYPOCOTYL [(LHY), (Figure 3; Nitschke et al., 2016, 2017). Recently it was found that the root-derived cytokinin trans-zeatin, acting via AHK2 and AHK3 in cooperation with AHK5, and the downstream type-B ARRs ARR2, ARR10, and ARR12, has a protective role against photoperiod stress, associated with an oxidative burst-like response (Abuelsoud et al., 2020).
Metaphorically, sensor kinases serve as guards at the gate to the cell or nucleus, enabling the perception of various environmental signals. Besides HKs, acting as an integral part of canonical MSP signaling, other sensor kinases integrated into MSP signaling are phytochromes (see above, Figure 1). Besides their major role in the light perception, phytochromes are also involved in osmotic-stress (Balestrasse et al., 2008) and drought responses (Kidokoro et al., 2009; D’Amico-Damião et al., 2015) (Figure 2). For instance, phyB was reported to positively affect drought tolerance in Arabidopsis (González et al., 2012). However, in other studies, phytochrome mutants exhibited improved drought tolerance (Liu et al., 2012), altered responses to high temperature (Njimona et al., 2014), and sensitivity to prolonged UV-B light radiation (Boccalandro et al., 2001; Rusaczonek et al., 2015). phys are necessary also for effective cold acclimation (Crosatti et al., 1999). phyB is stabilized at low temperature by the main cold-inducible TFs CBFs (Jiang et al., 2020). As phyB promotes degradation of PIF1, 4, and 5, which (especially PIF4) repress CBF genes at cold stress (Lee and Thomashow, 2012), phyB enhances freezing tolerance. Positive effect on freezing tolerance was found also in the case of phyA, especially at low R:FR ratio (Wang et al., 2016).
Importantly, phyB and its downstream signaling partner PIF7 were found to act also as thermosensors (Jung et al., 2016; Legris et al., 2016; Chung et al., 2020). PIF7 protein abundance was shown to accumulate in response to warm temperature further stimulating the expression of genes involved in thermomorphogenesis such as the auxin biosynthetic gene YUCCA8 (Chung et al., 2020). Interestingly, secondary structure of PIF7 mRNA forms an RNA hairpin within the 5′UTR region which changes its conformation at higher temperature and stimulating the PIN7 mRNA translation. This unique temperature-controlled mechanism of protein translation was identified also for WRKY22 and heat-shock regulator HSFA2 suggesting that as a conserved mechanism used by plants as a fast response to sudden temperature changes (Chung et al., 2020). Another rapid temperature-regulated mechanism was identified in case of phyB itself. phyB was postulated to be a temperature sensor in plants through its temperature-dependent reversion from the active Pfr state to the inactive Pr state, which affects its association with the promoters of key target genes in a temperature-dependent manner (Jung et al., 2016; Legris et al., 2016). Here, both temperature-dependent transcriptional regulation of PIF4 together and phyB-mediated post-transcriptional regulation of PIF4 ability to activate transcription of target genes was proposed (Jung et al., 2016). The active Pfr form of phyB was shown to be stabilized by ARR4 in planta, which was confirmed by increased hypersensitivity to red light in ARR4 overexpressing transgenic lines (Sweere et al., 2001; Fankhauser, 2002; see above). ARR3 and ARR4 seem to control both length of the period and its phase (Salomé et al., 2006). The loss of ARR4 and its closest homolog ARR3, prolongs the circadian cycle period even in the absence of light, suggesting that ARR3 and ARR4 control the circadian period independently of active phyB through an as yet unknown mechanism. In white light, arr3 and arr4 mutants show a leading phase (i.e., an earlier peak in the expression of genes that oscillate with the circadian rhythm) similar to phyB mutants, demonstrating that circadian light input is modulated by the interaction of phyB with ARR3 and ARR4. Considering the absence of any correlation between sensitivity to cytokinins in higher-order arr mutants and the period phenotype, as well as the distinct (concentration-dependent) effects of exogenous cytokinins, ARR3 and ARR4 seem to control both the length of the period (independent of phyB) and the phase of the period (through phyB) independently of cytokinins (Salomé et al., 2006). Since phyB acts as a light and temperature sensor and type-A ARRs expression responds to temperature fluctuations independently of cytokinin levels (see above), we may say that type-A ARRs could represent an intersection for light and temperature signaling to fine-tune photomorphogenesis under adverse environmental conditions (Figure 3).
Considering that stress responses are also modulated by cryptochromes (D’Amico-Damião et al., 2015), further research should be focused on the interconnection between blue light perception and MSP. Data about blue light – MSP crosstalk in abiotic stress responses is rather rudimentary. One of the potential mechanisms of blue light-cytokinin crosstalk might be mutual regulation of HY5 protein levels, as described above (Gangappa and Botto, 2016).
In our opinion one of the most critical roles of plant hormones is to act as a “universal” transducer between a constantly changing environment and control of the immediate physiological and developmental status of the plant. This transduction is then manifested as the final growth and developmental response. Multistep phosphorelay seems to be an ideal mechanism, allowing for the integration of multiple signaling inputs into a molecular response, characterized by quantitative and readily tunable changes in the transcriptional profile. In the light of several recent findings, implicating cytokinin-regulated MSP signaling (through type-B ARRs) in the control over context-dependent chromatin accessibility (Potter et al., 2018), the well-documented (cytokinin-modulated) role of ethylene in histone acetylation and ABA-dependent regulation of chromatin-remodeling complexes (see above), we can venture to say that epigenetic regulations form part of the repertoire of transcription regulatory tools available to MSP (and interconnected signaling pathways), possibly mediating environmental “memory” used by the plants in their adaptation to short- and medium-term environmental fluctuations. In line with this, Samsonová et al. (2020) showed that cytokinins occupy a prominent position among other hormones as unique ecotype identifiers. A positive correlation between mean ABA content and ABA/cytokinin ratio in the shoots with mean temperature at the site of origin is in line with existing data on the involvement of cytokinin signaling in the cold response (see above). This then means that control over cytokinin metabolism is one of the mechanisms involved in long-term adaptation. Also, the large variability in the ABA/cytokinin ratios observed among the ecotypes in both shoots and roots suggests hormonal plasticity, which might contribute to and/or determine stress responses in particular geographical regions (Samsonová et al., 2020).
The conclusions and implications we summarize above point toward a number of important questions. How is the combination of individual inputs translated into the final MSP signaling output? How is signaling fidelity maintained? In other words, how does the plant recognize and discern the particular origin of the signal, given that a number of sensory kinases recognizing the different type of signals result in phosphorylation of AHPs, and act as a signaling hub within the pathway? Is there any variability in the sensitivity of MSP signaling to individual types of MSP inputs associated with ecotype-specific adaptation to the site of its origin? And finally, what are the mechanisms acting downstream of MSP signaling in the stress response, either independently or in concert with other signaling pathways?
Future studies aimed at potential modification of the MSP signaling output at the level of the regulated genes and characterization of the underlying molecular mechanisms will be necessary to answer some of these questions. Studying the existing pool of natural genetic variability in MSP signaling affecting the responsiveness of the pathway to various inputs might provide important evidence on the potential role of MSP signaling and its downstream targets. They would also go a long way in shedding light on the ability of plants to successfully face environmental stresses which are after all an everyday reality of plant life. In combination with tools for targeted genome editing of plant genomes that have become available recently (Jinek et al., 2012; Anzalone et al., 2019), this type of knowledge will be a powerful enabler for rapid and targeted introduction of valuable alleles into the elite varieties being used in contemporary crop breeding programs.
JS, KN, and JH performed the literature search. KN and JS created the figures. JS, KN, RV, and JH wrote the manuscript. All authors contributed to the article and approved the submitted version.
This work was supported by the European Regional Development Fund project “SINGING PLANT” (No. CZ.02.1.01/0.0/0.0/16_026/0008446), which received a financial contribution from the Ministry of Education, Youth and Sports of the Czechia in the form of special support through the National Programme for Sustainability II program, “Centre for Experimental Plant Biology” (No. CZ.02.1.01/0.0/0.0/16_019/0000738), LTAUSA18161 and the Czech Science Foundation (19-24753S).
The authors declare that the research was conducted in the absence of any commercial or financial relationships that could be construed as a potential conflict of interest.
Abuelsoud, W., Cortleven, A., and Schmülling, T. (2020). Photoperiod stress induces an oxidative burst-like response and is associated with increased apoplastic peroxidase and decreased catalase activities. J. Plant Physiol. 253, 153252. doi: 10.1016/j.jplph.2020.153252
Ahuja, I., de Vos, R. C. H., Bones, A. M., and Hall, R. D. (2010). Plant molecular stress responses face climate change. Trends Plant Sci. 15, 664–674. doi: 10.1016/j.tplants.2010.08.002
Alabadí, D., and Blázquez, M. A. (2008). Integration of light and hormone signals. Plant Signal. Behav. 3, 448–449. doi: 10.4161/psb.3.7.5558
Allan, A. C., Fricker, M. D., Ward, J. L., Beale, M. H., and Trewavas, A. J. (1994). Two transduction pathways mediate rapid effects of abscisic acid in commelina guard cells. Plant Cell 6, 1319–1328. doi: 10.2307/3869829
An, F., Zhao, Q., Ji, Y., Li, W., Jiang, Z., Yu, X., et al. (2010). Ethylene-induced stabilization of ETHYLENE INSENSITIVE3 and EIN3-LIKE1 is mediated by proteasomal degradation of EIN3 binding F-box 1 and 2 that requires EIN2 in arabidopsis. Plant Cell 22, 2384–2401. doi: 10.1105/tpc.110.076588
Antoniadi, I., Novák, O., Gelová, Z., Johnson, A., Plíhal, O., Simerský, R., et al. (2020). Cell-surface receptors enable perception of extracellular cytokinins. Nat. Commun. 11:4284. doi: 10.1038/s41467-020-17700-9
Anzalone, A. V., Randolph, P. B., Davis, J. R., Sousa, A. A., Koblan, L. W., Levy, J. M., et al. (2019). Search-and-replace genome editing without double-strand breaks or donor DNA. Nature 576, 149–157. doi: 10.1038/s41586-019-1711-4
Argueso, C. T., Ferreira, F. J., and Kieber, J. J. (2009). Environmental perception avenues: the interaction of cytokinin and environmental response pathways. Plant Cell Environ. 32, 1147–1160. doi: 10.1111/j.1365-3040.2009.01940.x
Baena-González, E., Rolland, F., Thevelein, J. M., and Sheen, J. (2007). A central integrator of transcription networks in plant stress and energy signalling. Nature 448, 938–942. doi: 10.1038/nature06069
Baena-González, E., and Sheen, J. (2008). Convergent energy and stress signaling. Trends Plant Sci. 13, 474–482. doi: 10.1016/j.tplants.2008.06.006
Bakshi, A., Piy, S., Fernandez, J. C., Chervin, C., Hewezi, T., and Binder, B. M. (2018). Pethylene receptors signal via a noncanonical pathway to regulate abscisic acid responses. Plant Physiol. 176, 910–929. doi: 10.1104/pp.17.01321
Bakshi, A., Wilson, R. L., Lacey, R. F., Kim, H., Wuppalapati, S. K., and Binder, B. M. (2015). Identification of regions in the receiver domain of the ETHYLENE RESPONSE1 ethylene receptor of arabidopsis important for functional divergence. Plant Physiol. 169, 219–232. doi: 10.1104/pp.15.00626
Balestrasse, K. B., Zilli, C. G., and Tomaro, M. L. (2008). Signal transduction pathways and haem oxygenase induction in soybean leaves subjected to salt stress. Redox Rep. 13, 255–262. doi: 10.1179/135100008X308966
Ballaré, C. L., and Pierik, R. (2017). The shade-avoidance syndrome: multiple signals and ecological consequences. Plant Cell Environ. 40, 2530–2543. doi: 10.1111/pce.12914
Belda-Palazón, B., Adamo, M., Valerio, C., Ferreira, L. J., Confraria, A., Reis-Barata, D., et al. (2020). A dual function of SnRK2 kinases in the regulation of SnRK1 and plant growth. Nat. Plants 6, 1345–1353. doi: 10.1038/s41477-020-00778-w
Belin, C., De Franco, P. O., Bourbousse, C., Chaignepain, S., Schmitter, J. M., Vavasseur, A., et al. (2006). Identification of features regulating OST1 kinase activity and OST1 function in guard cells. Plant Physiol. 141, 1316–1327. doi: 10.1104/pp.106.079327
Bhargava, A., Clabaugh, I., To, J. P., Maxwell, B. B., Chiang, Y. H., Schaller, G. E., et al. (2013). Identification of cytokinin-responsive genes using microarray meta-analysis and RNA-seq in Arabidopsis. Plant Physiol. 162, 272–294. doi: 10.1104/pp.113.217026
Binder, B. M. (2020). Ethylene signaling in plants. J. Biol. Chem. 295, 7710–7725. doi: 10.1074/jbc.REV120.010854
Binder, B. M., Rodríguez, F. I., and Bleecker, A. B. (2010). The copper transporter RAN1 is essential for biogenesis of ethylene receptors in Arabidopsis. J. Biol. Chem. 285, 37263–37270. doi: 10.1074/jbc.M110.170027
Binder, B. M., Walker, J. M., Gagne, J. M., Emborg, T. J., Hemmann, G., Bleecker, A. B., et al. (2007). The Arabidopsis EIN3 binding F-box proteins EBF1 and EBF2 have distinct but overlapping roles in ethylene signaling. Plant Cell 19, 509–523. doi: 10.1105/tpc.106.048140
Blatt, M. R., and Grabov, A. (1997). Signal redundancy, gates and integration in the control of ion channels for stomatal movement. J. Exp. Bot. 48, 529–537. doi: 10.1093/jxb/48.special_issue.529
Boccalandro, H. E., Mazza, C. A., Mazzella, M. A., Casal, J. J., and Ballaré, C. L. (2001). Ultraviolet B radiation enhances a phytochrome-B-mediated photomorphogenic response in Arabidopsis. Plant Physiol. 126, 780–788. doi: 10.1104/pp.126.2.780
Boudsocq, M., Droillard, M. J., Barbier-Brygoo, H., and Laurière, C. (2007). Different phosphorylation mechanisms are involved in the activation of sucrose non-fermenting 1 related protein kinases 2 by osmotic stresses and abscisic acid. Plant Mol. Biol. 63, 491–503. doi: 10.1007/s11103-006-9103-1
Brenner, W. G., Ramireddy, E., Heyl, A., and Schmülling, T. (2012). Gene regulation by cytokinin in Arabidopsis. Front. Plant Sci. 3:8. doi: 10.3389/fpls.2012.00008
Brenner, W. G., Romanov, G. A., Köllmer, I., Bürkle, L., and Schmülling, T. (2005). Immediate-early and delayed cytokinin response genes of Arabidopsis thaliana identified by genome-wide expression profiling reveal novel cytokinin-sensitive processes and suggest cytokinin action through transcriptional cascades. Plant J. 44, 314–333. doi: 10.1111/j.1365-313X.2005.02530.x
Brenner, W. G., and Schmülling, T. (2015). Summarizing and exploring data of a decade of cytokinin-related transcriptomics. Front. Plant Sci. 6:29. doi: 10.3389/fpls.2015.00029
Caesar, K., Thamm, A. M. K., Witthöft, J., Elgass, K., Huppenberger, P., Grefen, C., et al. (2011). Evidence for the localization of the Arabidopsis cytokinin receptors AHK3 and AHK4 in the endoplasmic reticulum. J. Exp. Bot. 62, 5571–5580. doi: 10.1093/jxb/err238
Casal, J. J., Candia, A. N., and Sellaro, R. (2014). Light perception and signalling by phytochrome A. J. Exp. Bot. 65, 2835–2845. doi: 10.1093/jxb/ert379
Castillo, M. C., Lozano-Juste, J., González-Guzmán, M., Rodriguez, L., Rodriguez, P. L., and León, J. (2015). Inactivation of PYR/PYL/RCAR ABA receptors by tyrosine nitration may enable rapid inhibition of ABA signaling by nitric oxide in plants. Sci. Signal. 8:ra89. doi: 10.1126/scisignal.aaa7981
Černý, M., Jedelský, P. L., Novák, J., Schlosser, A., and Brzobohatý, B. (2014). Cytokinin modulates proteomic, transcriptomic and growth responses to temperature shocks in Arabidopsis. Plant Cell Environ. 37, 1641–1655. doi: 10.1111/pce.12270
Chang, J., Li, X., Fu, W., Wang, J., Yong, Y., Shi, H., et al. (2019). Asymmetric distribution of cytokinins determines root hydrotropism in Arabidopsis thaliana. Cell Res. 29, 984–993. doi: 10.1038/s41422-019-0239-3
Cheng, M.-C., Liao, P.-M., Kuo, W.-W., and Lin, T.-P. (2013). The Arabidopsis ETHYLENE RESPONSE FACTOR1 regulates abiotic stress-responsive gene expression by binding to different cis-acting elements in response to different stress signals. Plant Physiol. 162, 1566–1582. doi: 10.1104/pp.113.221911
Chinnusamy, V., and Zhu, J. K. (2009). Epigenetic regulation of stress responses in plants. Curr. Opin. Plant Biol. 12, 133–139. doi: 10.1016/j.pbi.2008.12.006
Cho, Y. H., and Yoo, S. D. (2007). ETHYLENE RESPONSE 1 histidine kinase activity of arabidopsis promotes plant growth. Plant Physiol. 143, 612–616. doi: 10.1104/pp.106.091504
Chung, B. Y. W., Balcerowicz, M., Di Antonio, M., Jaeger, K. E., Geng, F., Franaszek, K., et al. (2020). An RNA thermoswitch regulates daytime growth in Arabidopsis. Nat. Plants 6, 522–532. doi: 10.1038/s41477-020-0633-3
Claeys, H., and Inzé, D. (2013). The agony of choice: how plants balance growth and survival under water-limiting conditions. Plant Physiol. 162, 1768–1779. doi: 10.1104/pp.113.220921
Clark, K. L., Larsen, P. B., Wang, X., and Chang, C. (1998). Association of the Arabidopsis CTR1 Raf-like kinase with the ETR1 and ERS ethylene receptors. Proc. Natl. Acad. Sci. U.S.A. 95, 5401–5406. doi: 10.1073/pnas.95.9.5401
Cortleven, A., Nitschke, S., Klaumünzer, M., AbdElgawad, H., Asard, H., Grimm, B., et al. (2014). A novel protective function for cytokinin in the light stress response is mediated by the ARABIDOPSIS HISTIDINE KINASE2 and ARABIDOPSIS HISTIDINE KINASE3 receptors. Plant Physiol. 164, 1470–1483. doi: 10.1104/pp.113.224667
Cortleven, A., and Schmülling, T. (2015). Regulation of chloroplast development and function by cytokinin. J. Exp. Bot. 66, 4999–5013. doi: 10.1093/jxb/erv132
Cotton, J. L. S., Ross, C. W., Byrne, D. H., and Colbert, J. T. (1990). Down-regulation of phytochrome mRNA abundance by red light and benzyladenine in etiolated cucumber cotyledons. Plant Mol. Biol. 14, 707–714. doi: 10.1007/BF00016503
Crosatti, C., De Laureto, P. P., Bassi, R., and Cattivelli, L. (1999). The interaction between cold and light controls the expression of the cold-regulated barley gene cor14b and the accumulation of the corresponding protein. Plant Physiol. 119, 671–680. doi: 10.1104/pp.119.2.671
D’Amico-Damião, V., Cruz, F. J. R., Gavassi, M. A., Santos, D. M. M., Melo, H. C., and Carvalho, R. F. (2015). Photomorphogenic modulation of water stress in tomato (Solanum lycopersicum L.): the role of phytochromes A, B1, and B2. J. Hortic. Sci. Biotechnol. 90, 25–30. doi: 10.1080/14620316.2015.11513149
Dautel, R., Wu, X. N., Heunemann, M., Schulze, W. X., and Harter, K. (2016). The sensor histidine kinases AHK2 and AHK3 proceed into multiple serine/threonine/tyrosine phosphorylation pathways in Arabidopsis thaliana. Mol. Plant 9, 182–186. doi: 10.1016/j.molp.2015.10.002
De Wit, M., Galvão, V. C., and Fankhauser, C. (2016). Light-mediated hormonal regulation of plant growth and development. Annu. Rev. Plant Biol. 67, 513–537. doi: 10.1146/annurev-arplant-043015-112252
Dejonghe, W., Okamoto, M., and Cutler, S. R. (2018). Small molecule probes of ABA biosynthesis and signaling. Plant Cell Physiol. 59, 1490–1499. doi: 10.1093/pcp/pcy126
Deng, Y., Dong, H., Mu, J., Ren, B., Zheng, B., Ji, Z., et al. (2010). Arabidopsis histidine kinase CKI1 acts upstream of histidine phosphotransfer proteins to regulate female gametophyte development and vegetative growth. Plant Cell 22, 1232–1248. doi: 10.1105/tpc.108.065128
Desikan, R., Horák, J., Chaban, C., Mira-Rodado, V., Witthöft, J., Elgass, K., et al. (2008). The histidine kinase AHK5 integrates endogenous and environmental signals in Arabidopsis guard cells. PLoS One 3:e2491. doi: 10.1371/journal.pone.0002491
Desikan, R., Last, K., Harrett-Williams, R., Tagliavia, C., Harter, K., Hooley, R., et al. (2006). Ethylene-induced stomatal closure in Arabidopsis occurs via AtrbohF-mediated hydrogen peroxide synthesis. Plant J. 47, 907–916. doi: 10.1111/j.1365-313X.2006.02842.x
Dobisova, T., Hrdinova, V., Cuesta, C., Michlickova, S., Urbankova, I., Hejatkova, R., et al. (2017). Light controls cytokinin signaling via transcriptional regulation of constitutively active sensor histidine kinase CKI1. Plant Physiol. 174, 387–404. doi: 10.1104/pp.16.01964
Dobrá, J., Černý, M., Štorchová, H., Dobrev, P., Skalák, J., Jedelský, P. L., et al. (2015). The impact of heat stress targeting on the hormonal and transcriptomic response in Arabidopsis. Plant Sci. 231, 52–61. doi: 10.1016/j.plantsci.2014.11.005
Dobra, J., Motyka, V., Dobrev, P., Malbeck, J., Prasil, I. T., Haisel, D., et al. (2010). Comparison of hormonal responses to heat, drought and combined stress in tobacco plants with elevated proline content. J. Plant Physiol. 167, 1360–1370. doi: 10.1016/j.jplph.2010.05.013
Dong, P., Xiong, F., Que, Y., Wang, K., Yu, L., Li, Z., et al. (2015). Expression profiling and functional analysis reveals that TOR is a key player in regulating photosynthesis and phytohormone signaling pathways in Arabidopsis. Front. Plant Sci. 6:677. doi: 10.3389/fpls.2015.00677
Dubois, M., Van den Broeck, L., and Inzé, D. (2018). The pivotal role of ethylene in plant growth. Trends Plant Sci. 23, 311–323. doi: 10.1016/j.tplants.2018.01.003
Eichmann, R., and Schäfer, P. (2015). Growth versus immunity-a redirection of the cell cycle? Curr. Opin. Plant Biol. 26, 106–112. doi: 10.1016/j.pbi.2015.06.006
Etheridge, N., Hall, B. P., and Schaller, G. E. (2006). Progress report: ethylene signaling and responses. Planta 223, 387–391. doi: 10.1007/s00425-005-0163-2
Fankhauser, C. (2002). Light perception in plants: cytokinins and red light join forces to keep phytochrome B active. Trends Plant Sci. 7, 143–145. doi: 10.1016/S1360-1385(02)02228-8
Feng, J., Wang, C., Chen, Q., Chen, H., Ren, B., Li, X., et al. (2013). S-nitrosylation of phosphotransfer proteins represses cytokinin signaling. Nat. Commun. 4:1529. doi: 10.1038/ncomms2541
Filipe, O., De Vleesschauwer, D., Haeck, A., Demeestere, K., and Höfte, M. (2018). The energy sensor OsSnRK1a confers broad-spectrum disease resistance in rice. Sci. Rep. 8:3864. doi: 10.1038/s41598-018-22101-6
Fujii, H., and Zhu, J.-K. (2009). Arabidopsis mutant deficient in 3 abscisic acid-activated protein kinases reveals critical roles in growth, reproduction, and stress. Proc. Natl. Acad. Sci. U.S.A. 106, 8380–8385. doi: 10.1073/pnas.0903144106
Fujita, Y., Nakashima, K., Yoshida, T., Katagiri, T., Kidokoro, S., Kanamori, N., et al. (2009). Three SnRK2 protein kinases are the main positive regulators of abscisic acid signaling in response to water stress in Arabidopsis. Plant Cell Physiol. 50, 2123–2132. doi: 10.1093/pcp/pcp147
Fujita, Y., Yoshida, T., and Yamaguchi-Shinozaki, K. (2013). Pivotal role of the AREB/ABF-SnRK2 pathway in ABRE-mediated transcription in response to osmotic stress in plants. Physiol. Plant 147, 15–27. doi: 10.1111/j.1399-3054.2012.01635.x
Gagne, J. M., Smalle, J., Gingerich, D. J., Walker, J. M., Yoo, S. D., Yanagisawa, S., et al. (2004). Arabidopsis EIN3-binding F-box 1 and 2 form ubiquitin-protein ligases that repress ethylene action and promote growth by directing EIN3 degradation. Proc. Natl. Acad. Sci. U.S.A. 101, 6803–6808. doi: 10.1073/pnas.0401698101
Gangappa, S. N., and Botto, J. F. (2016). The multifaceted roles of HY5 in plant growth and development. Mol. Plant 9, 1353–1365. doi: 10.1016/j.molp.2016.07.002
González, C. V., Ibarra, S. E., Piccoli, P. N., Botto, J. F., and Boccalandro, H. E. (2012). Phytochrome B increases drought tolerance by enhancing ABA sensitivity in Arabidopsis thaliana. Plant Cell Environ. 35, 1958–1968. doi: 10.1111/j.1365-3040.2012.02529.x
Grefen, C., Städele, K., Růžiěka, K., Obrdlik, P., Harter, K., and Horák, J. (2008). Subcellular localization and in vivo interactions of the Arabidopsis thaliana ethylene receptor family members. Mol. Plant 1, 308–320. doi: 10.1093/mp/ssm015
Guo, H., and Ecker, J. R. (2003). Plant responses to ethylene gas are mediated by SCF(EBF1/EBF2)-dependent proteolysis of EIN3 transcription factor. Cell 115, 667–677. doi: 10.1016/s0092-8674(03)00969-3
Guo, Y., and Gan, S. (2011). AtMYB2 regulates whole plant senescence by inhibiting cytokinin-mediated branching at late stages of development in Arabidopsis. Plant Physiol. 156, 1612–1619. doi: 10.1104/pp.111.177022
Gupta, S., and Rashotte, A. M. (2014). Expression patterns and regulation of SlCRF3 and SlCRF5 in response to cytokinin and abiotic stresses in tomato (Solanum lycopersicum). J. Plant Physiol. 171, 349–358. doi: 10.1016/j.jplph.2013.09.003
Hai, N. N., Chuong, N. N., Tu, N. H. C., Kisiala, A., Hoang, X. L. T., and Thao, N. P. (2020). Role and regulation of cytokinins in plant response to drought stress. Plants 9:422. doi: 10.3390/plants9040422
Han, S. K., and Wagner, D. (2014). Role of chromatin in water stress responses in plants. J. Exp. Bot. 65, 2785–2799. doi: 10.1093/jxb/ert403
Han, S. K., Wu, M. F., Cui, S., and Wagner, D. (2015). Roles and activities of chromatin remodeling ATPases in plants. Plant J. 83, 62–77. doi: 10.1111/tpj.12877
Hass, C., Lohrmann, J., Albrecht, V., Sweere, U., Hummel, F., Yoo, S. D., et al. (2004). The response regulator 2 mediates ethylene signalling and hormone signal integration in Arabidopsis. Embo J. 23, 3290–3302. doi: 10.1038/sj.emboj.7600337
Hejátko, J., Pernisová, M., Eneva, T., Palme, K., and Brzobohatý, B. (2003). The putative sensor histidine kinase CKI1 is involved in female gametophyte development in Arabidopsis. Mol. Genet. Genomics 269, 443–453. doi: 10.1007/s00438-003-0858-7
Hejátko, J., Ryu, H., Kim, G. T., Dobešová, R., Choi, S., Choi, S. M., et al. (2009). The Histidine kinases cytokinin-independent1 and arabidopsis histidine kinase2 and 3 regulate vascular tissue development in arabidopsis shoots. Plant Cell 21, 2008–2021. doi: 10.1105/tpc.109.066696
Hirayama, T., Kieber, J. J., Hirayama, N., Kogan, M., Guzman, P., Nourizadeh, S., et al. (1999). RESPONSIVE-TO-ANTAGONIST1, a Menkes/Wilson disease-related copper transporter, is required for ethylene signaling in Arabidopsis. Cell 97, 383–393. doi: 10.1016/S0092-8674(00)80747-3
Horak, J., Janda, L., Pekarova, B., and Hejatko, J. (2011). Molecular mechanisms of signalling specificity via phosphorelay pathways in Arabidopsis. Curr. Protein Pept. Sci. 12, 126–136. doi: 10.2174/1389211213488452037
Hothorn, M., Dabi, T., and Chory, J. (2011). Structural basis for cytokinin recognition by Arabidopsis thaliana histidine kinase 4. Nat. Chem. Biol. 7, 766–768. doi: 10.1038/nchembio.667
Huang, X., Hou, L., Meng, J., You, H., Li, Z., Gong, Z., et al. (2018). The antagonistic action of abscisic acid and cytokinin signaling mediates drought stress response in Arabidopsis. Mol. Plant 11, 970–982. doi: 10.1016/j.molp.2018.05.001
Huang, X., Ouyang, X., and Deng, X. W. (2014). Beyond repression of photomorphogenesis: role switching of COP/DET/FUS in light signaling. Curr. Opin. Plant Biol. 21, 96–103. doi: 10.1016/j.pbi.2014.07.003
Huang, X., Zhang, X., Gong, Z., Yang, S., and Shi, Y. (2017). ABI4 represses the expression of type-A ARRs to inhibit seed germination in Arabidopsis. Plant J. 89, 354–365. doi: 10.1111/tpj.13389
Huang, Y., Li, H., Hutchison, C. E., Laskey, J., and Kieber, J. J. (2003). Biochemical and functional analysis of CTR1, a protein kinase that negatively regulates ethylene signaling in Arabidopsis. Plant J. 33, 221–233. doi: 10.1046/j.1365-313X.2003.01620.x
Hutchison, C. E., and Kieber, J. J. (2002). Cytokinin signaling in Arabidopsis. Plant Cell 14, S47–S59. doi: 10.1105/tpc.010444
Hutchison, C. E., Li, J., Argueso, C., Gonzalez, M., Lee, E., Lewis, M. W., et al. (2006). The Arabidopsis histidine phosphotransfer proteins are redundant positive regulators of cytokinin signaling. Plant Cell 18, 3073–3087. doi: 10.1105/tpc.106.045674
Hwang, I., and Sheen, J. (2001). Two-component circuitry in Arabidopsis cytokinin signal transduction. Nature 413, 383–389. doi: 10.1038/35096500
Inzé, A., Vanderauwera, S., Hoeberichts, F. A., Vandorpe, M., van Gaever, T., and van Breusegem, F. (2012). A subcellular localization compendium of hydrogen peroxide-induced proteins. Plant Cell Environ. 35, 308–320. doi: 10.1111/j.1365-3040.2011.02323.x
Iwama, A., Yamashino, T., Tanaka, Y., Sakakibara, H., Kakimoto, T., Sato, S., et al. (2007). AHK5 histidine kinase regulates root elongation through an ETR1-dependent abscisic acid and ethylene signaling pathway in Arabidopsis thaliana. Plant Cell Physiol. 48, 375–380. doi: 10.1093/pcp/pcl065
Jamsheer, M. K., Jindal, S., and Laxmi, A. (2019). Evolution of TOR–SnRK dynamics in green plants and its integration with phytohormone signaling networks. J. Exp. Bot. 70, 2239–2259. doi: 10.1093/jxb/erz107
Jeon, J., Cho, C., Lee, M. R., Van Binh, N., and Kim, J. (2016). CYTOKININ RESPONSE FACTOR2 (CRF2) and CRF3 regulate lateral root development in response to cold stress in Arabidopsis. Plant Cell 28, 1828–1843. doi: 10.1105/tpc.15.00909
Jeon, J., and Kim, J. (2013). Arabidopsis response regulator1 and arabidopsis histidine phosphotransfer protein2 (AHP2), AHP3, and AHP5 function in cold signaling. Plant Physiol. 161, 408–424. doi: 10.1104/pp.112.207621
Jeon, J., Kim, N. Y., Kim, S., Kang, N. Y., Novák, O., Ku, S. J., et al. (2010). A subset of cytokinin two-component signaling system plays a role in cold temperature stress response in Arabidopsis. J. Biol. Chem. 285, 23371–23386. doi: 10.1074/jbc.M109.096644
Jiang, B., Shi, Y., Peng, Y., Jia, Y., Yan, Y., Dong, X., et al. (2020). Cold-Induced CBF–PIF3 interaction enhances freezing tolerance by stabilizing the phyB thermosensor in Arabidopsis. Mol. Plant 13, 894–906. doi: 10.1016/j.molp.2020.04.006
Jinek, M., Chylinski, K., Fonfara, I., Hauer, M., Doudna, J. A., and Charpentier, E. (2012). A programmable dual-RNA-guided DNA endonuclease in adaptive bacterial immunity. Science 337, 816–821. doi: 10.1126/science.1225829
Johnová, P., Skalák, J., Saiz-Fernández, I., and Brzobohatý, B. (2016). Plant responses to ambient temperature fluctuations and water-limiting conditions: a proteome-wide perspective. Biochim. Biophys. Acta Proteins Proteomics 1864, 916–931. doi: 10.1016/j.bbapap.2016.02.007
Ju, C., Yoon, G. M., Shemansky, J. M., Lin, D. Y., Ying, Z. I., Chang, J., et al. (2012). CTR1 phosphorylates the central regulator EIN2 to control ethylene hormone signaling from the ER membrane to the nucleus in Arabidopsis. Proc. Natl. Acad. Sci. U.S.A. 109, 19486–19491. doi: 10.1073/pnas.1214848109
Jung, J. H., Domijan, M., Klose, C., Biswas, S., Ezer, D., Gao, M., et al. (2016). Phytochromes function as thermosensors in Arabidopsis. Science 354, 886–889. doi: 10.1126/science.aaf6005
Kakimoto, T. (1996). CKI1, a histidine kinase homolog implicated in cytokinin signal transduction. Science 274, 982–985. doi: 10.1126/science.274.5289.982
Kang, N. Y., Cho, C., Kim, N. Y., and Kim, J. (2012). Cytokinin receptor-dependent and receptor-independent pathways in the dehydration response of Arabidopsis thaliana. J. Plant Physiol. 169, 1382–1391. doi: 10.1016/j.jplph.2012.05.007
Kaplan, F., Kopka, J., Haskell, D. W., Zhao, W., Schiller, K. C., Gatzke, N., et al. (2004). Exploring the temperature-stress metabolome. Plant Physiol. 136, 4159–4168. doi: 10.1104/pp.104.052142.1
Katsuta, S., Masuda, G., Bak, H., Shinozawa, A., Kamiyama, Y., Umezawa, T., et al. (2020). Arabidopsis Raf-like kinases act as positive regulators of subclass III SnRK2 in osmostress signaling. Plant J. 103, 634–644. doi: 10.1111/tpj.14756
Kidokoro, S., Maruyama, K., Nakashima, K., Imura, Y., Narusaka, Y., Shinwari, Z. K., et al. (2009). The phytochrome-interacting factor PIF7 negatively regulates dreb1 expression under circadian control in Arabidopsis. Plant Physiol. 151, 2046–2057. doi: 10.1104/pp.109.147033
Kieber, J. J., Rothenberg, M., Roman, G., Feldmann, K. A., and Ecker, J. R. (1993). CTR1, a negative regulator of the ethylene response pathway in arabidopsis, encodes a member of the Raf family of protein kinases. Cell 72, 427–441. doi: 10.1016/0092-8674(93)90119-B
Kieber, J. J., and Schaller, G. E. (2018). Cytokinin signaling in plant development. Development 145:dev149344. doi: 10.1242/dev.149344
Kim, G. D., Cho, Y. H., and Yoo, S. D. (2017). Regulatory functions of cellular energy sensor SNF1-Related Kinase1 for leaf senescence delay through ETHYLENE- INSENSITIVE3 repression. Sci. Rep. 7:3193. doi: 10.1038/s41598-017-03506-1
Kosová, K., Prášil, I. T., Vítámvás, P., Dobrev, P., Motyka, V., Floková, K., et al. (2012). Complex phytohormone responses during the cold acclimation of two wheat cultivars differing in cold tolerance, winter Samanta and spring Sandra. J. Plant Physiol. 169, 567–576. doi: 10.1016/j.jplph.2011.12.013
Kubiasová, K., Montesinos, J. C., Šamajová, O., Nisler, J., Mik, V., Semerádová, H., et al. (2020). Cytokinin fluoroprobe reveals multiple sites of cytokinin perception at plasma membrane and endoplasmic reticulum. Nat. Commun. 11:4285. doi: 10.1038/s41467-020-17949-0
Kumar, M., Kesawat, M. S., Ali, A., Lee, S. C., Gill, S. S., and Kim, H. U. (2019). Integration of abscisic acid signaling with other signaling pathways in plant stress responses and development. Plants 8:592. doi: 10.3390/plants8120592
Kumar, M. N., Jane, W. N., and Verslues, P. E. (2013). Role of the putative osmosensor Arabidopsis histidine kinase1 in dehydration avoidance and low-water-potential response. Plant Physiol. 161, 942–953.
Kumar, M. N., and Verslues, P. E. (2015). Stress physiology functions of the Arabidopsis histidine kinase cytokinin receptors. Physiol. Plant 154, 369–380. doi: 10.1111/ppl.12290
Larkindale, J., Mishkind, M., and Vierling, E. (2007). “Plant responses to high temperature,” in Plant Abiotic Stress, eds A. Muhammed, R. Aksel, and R. C. von Borstel (Boston, MA: Springer).
Lee, C.-M., and Thomashow, M. F. (2012). Photoperiodic regulation of the C-repeat binding factor (CBF) cold acclimation pathway and freezing tolerance in Arabidopsis thaliana. Proc. Natl. Acad. Sci. U.S.A. 109, 15054–15059. doi: 10.1073/pnas.1211295109
Legris, M., Klose, C., Burgie, E. S., Rojas, C. C., Neme, M., Hiltbrunner, A., et al. (2016). Phytochrome B integrates light and temperature signals in Arabidopsis. Science 354, 897–900. doi: 10.1126/science.aaf5656
Li, J., Li, G., Wang, H., and Wang Deng, X. (2011). Phytochrome signaling mechanisms. Arab. B. 9, e0148. doi: 10.1199/tab.0148
Li, W., Herrera-Estrella, L., and Tran, L.-S. P. (2016). The Yin–Yang of cytokinin homeostasis and drought acclimation/adaptation. Trends Plant Sci. 21, 548–550. doi: 10.1016/j.tplants.2016.05.006
Li, W., Ma, M., Feng, Y., Li, H., Wang, Y., Ma, Y., et al. (2015). EIN2-directed translational regulation of ethylene signaling in Arabidopsis. Cell 163, 670–683. doi: 10.1016/j.cell.2015.09.037
Lin, Z., Li, Y., Zhang, Z., Liu, X., Hsu, C. C., Du, Y., et al. (2020). A RAF-SnRK2 kinase cascade mediates early osmotic stress signaling in higher plants. Nat. Commun. 11:613. doi: 10.1038/s41467-020-14477-9
Liu, J., Zhang, F., Zhou, J., Chen, F., Wang, B., and Xie, X. (2012). Phytochrome B control of total leaf area and stomatal density affects drought tolerance in rice. Plant Mol. Biol. 78, 289–300. doi: 10.1007/s11103-011-9860-3
Liu, Z., Yuan, L., Song, X., Yu, X., and Sundaresan, V. (2017). AHP2, AHP3, and AHP5 act downstream of CKI1 in Arabidopsis female gametophyte development. J. Exp. Bot. 68, 3365–3373. doi: 10.1093/jxb/erx181
Lozano-Juste, J., Alrefaei, A. F., and Rodriguez, P. L. (2020). Plant osmotic stress signaling: MAPKKKs Meet SnRK2s. Trends Plant Sci. 25, 1179–1182. doi: 10.1016/j.tplants.2020.09.003
Lozano-Juste, J., and León, J. (2010a). Enhanced abscisic acid-mediated responses in nia1nia2noa1-2 triple mutant impaired in NIA/NR- and AtNOA1-dependent nitric oxide biosynthesis in Arabidopsis. Plant Physiol. 152, 891–903. doi: 10.1104/pp.109.148023
Lozano-Juste, J., and León, J. (2010b). Nitric oxide modulates sensitivity to ABA. Plant Signal. Behav. 5, 314–316. doi: 10.4161/psb.5.3.11235
Lu, X. D., Zhou, C. M., Xu, P. B., Luo, Q., Lian, H. L., and Yang, H. Q. (2015). Red-light-dependent interaction of phyB with SPA1 promotes COP1-SPA1 dissociation and photomorphogenic development in Arabidopsis. Mol. Plant 8, 467–478. doi: 10.1016/j.molp.2014.11.025
Ma, Y., Szostkiewicz, I., Korte, A., Moes, D., Yang, Y., Christmann, A., et al. (2009). Regulators of PP2C phosphatase activity function as abscisic acid sensors. Science 324, 1064–1068. doi: 10.1126/science.1172408
Macková, H., Hronková, M., Dobrá, J., Turečková, V., Novák, O., Lubovská, Z., et al. (2013). Enhanced drought and heat stress tolerance of tobacco plants with ectopically enhanced cytokinin oxidase/dehydrogenase gene expression. J. Exp. Bot. 64, 2805–2815. doi: 10.1093/jxb/ert131
Marchadier, E., and Hetherington, A. M. (2014). Involvement of two-component signalling systems in the regulation of stomatal aperture by light in Arabidopsis thaliana. New Phytol. 203, 462–468. doi: 10.1111/nph.12813
Maruyama, K., Urano, K., Yoshiwara, K., Morishita, Y., Sakurai, N., Suzuki, H., et al. (2014). Integrated analysis of the effects of cold and dehydration on rice metabolites, phytohormones, and gene transcripts. Plant Physiol. 164, 1759–1771. doi: 10.1104/pp.113.231720
Mason, M. G., Jha, D., Salt, D. E., Tester, M., Hill, K., Kieber, J. J., et al. (2010). Type-B response regulators ARR1 and ARR12 regulate expression of AtHKT1;1 and accumulation of sodium in Arabidopsis shoots. Plant J. 64, 753–763. doi: 10.1111/j.1365-313X.2010.04366.x
McAinsh, M. R., and Hetherington, A. M. (1998). Encoding specificity in Ca2+ signalling systems. Trends Plant Sci. 3, 32–36. doi: 10.1016/S1360-1385(97)01150-3
Merchante, C., Brumos, J., Yun, J., Hu, Q., Spencer, K. R., Enríquez, P., et al. (2015). Gene-specific translation regulation mediated by the hormone-signaling molecule EIN2. Cell 163, 684–697. doi: 10.1016/j.cell.2015.09.036
Mira-Rodado, V., Sweere, U., Grefen, C., Kunkel, T., Fejes, E., Nagy, F., et al. (2007). Functional cross-talk between two-component and phytochrome B signal transduction in Arabidopsis. J. Exp. Bot. 58, 2595–2607. doi: 10.1093/jxb/erm087
Mittler, R., Finka, A., and Goloubinoff, P. (2012). How do plants feel the heat? Trends Biochem. Sci. 37, 118–125. doi: 10.1016/j.tibs.2011.11.007
Miura, K., and Furumoto, T. (2013). Cold signaling and cold response in plants. Int. J. Mol. Sci. 14, 5312–5337. doi: 10.3390/ijms14035312
Müller, B., and Sheen, J. (2007). Arabidopsis cytokinin signaling pathway. Sci. STKE 2007:cm5. doi: 10.1126/stke.4072007cm5
Müller, M., and Munné-Bosch, S. (2015). Ethylene response factors: a key regulatory hub in hormone and stress signaling. Plant Physiol. 169, 32–41. doi: 10.1104/pp.15.00677
Nakashima, K., Fujita, Y., Kanamori, N., Katagiri, T., Umezawa, T., Kidokoro, S., et al. (2009). Three arabidopsis SnRK2 protein kinases, SRK2D/SnRK2.2, SRK2E/SnRK2.6/OST1 and SRK2I/SnRK2.3, involved in ABA signaling are essential for the control of seed development and dormancy. Plant Cell Physiol. 50, 1345–1363. doi: 10.1093/pcp/pcp083
Nakashima, K., and Yamaguchi-Shinozaki, K. (2013). ABA signaling in stress-response and seed development. Plant Cell Rep. 32, 959–970. doi: 10.1007/s00299-013-1418-1
Ng, L. M., Melcher, K., Teh, B. T., and Xu, H. E. (2014). Abscisic acid perception and signaling: structural mechanisms and applications. Acta Pharmacol. Sin. 35, 567–584. doi: 10.1038/aps.2014.5
Nguyen, K. H., Ha, C., Van, Nishiyama, R., Watanabe, Y., Leyva-González, M. A., et al. (2016). Arabidopsis type B cytokinin response regulators ARR1, ARR10, and ARR12 negatively regulate plant responses to drought. Proc. Natl. Acad. Sci. U.S.A. 113, 3090–3095. doi: 10.1073/pnas.1600399113
Nishiyama, R., Watanabe, Y., Fujita, Y., Le, D. T., Kojima, M., Werner, T., et al. (2011). Analysis of cytokinin mutants and regulation of cytokinin metabolic genes reveals important regulatory roles of cytokinins in drought, salt and abscisic acid responses, and abscisic acid biosynthesis. Plant Cell 23, 2169–2183. doi: 10.1105/tpc.111.087395
Nitschke, S., Cortleven, A., Iven, T., Feussner, I., Havaux, M., Riefler, M., et al. (2016). Circadian stress regimes affect the circadian clock and cause jasmonic acid-dependent cell death in cytokinin-deficient Arabidopsis plants. Plant Cell 28, 1616–1639. doi: 10.1105/tpc.16.00016
Nitschke, S., Cortleven, A., and Schmülling, T. (2017). Novel stress in plants by altering the photoperiod. Trends Plant Sci. 22, 913–916. doi: 10.1016/j.tplants.2017.09.005
Njimona, I., Yang, R., and Lamparter, T. (2014). Temperature effects on bacterial phytochrome. PLoS One 9:e109794. doi: 10.1371/journal.pone.0109794
Nukarinen, E., Ngele, T., Pedrotti, L., Wurzinger, B., Mair, A., Landgraf, R., et al. (2016). Quantitative phosphoproteomics reveals the role of the AMPK plant ortholog SnRK1 as a metabolic master regulator under energy deprivation. Sci. Rep. 6:31697. doi: 10.1038/srep31697
O’Brien, J. A., and Benková, E. (2013). Cytokinin cross-talking during biotic and abiotic stress responses. Front. Plant Sci. 4:451. doi: 10.3389/fpls.2013.00451
Park, S.-Y., Fung, P., Nishimura, N., Jensen, D. R., Fujii, H., Zhao, Y., et al. (2009). Abscisic acid inhibits type 2C protein phosphatases via the PYR/PYL family of START proteins. Science 324, 1068–1071. doi: 10.1126/science.1173041
Peirats-Llobet, M., Han, S. K., Gonzalez-Guzman, M., Jeong, C. W., Rodriguez, L., Belda-Palazon, B., et al. (2016). A direct link between abscisic acid sensing and the chromatin-remodeling ATPase BRAHMA via Core ABA signaling pathway components. Mol. Plant 9, 136–147. doi: 10.1016/j.molp.2015.10.003
Pham, J., and Desikan, R. (2012). Modulation of ROS production and hormone levels by AHK5 during abiotic and biotic stress signaling. Plant Signal. Behav. 7, 893–897. doi: 10.4161/psb.20692
Pham, J., Liu, J., Bennett, M. H., Mansfield, J. W., and Desikan, R. (2012). Arabidopsis histidine kinase 5 regulates salt sensitivity and resistance against bacterial and fungal infection. New Phytol. 194, 168–180. doi: 10.1111/j.1469-8137.2011.04033.x
Pischke, M. S., Jones, L. G., Otsuga, D., Fernandez, D. E., Drews, G. N., and Sussman, M. R. (2002). An Arabidopsis histidine kinase is essential for megagametogenesis. Proc. Natl. Acad. Sci. U.S.A. 99, 15800–15805. doi: 10.1073/pnas.232580499
Potter, K. C., Wang, J., Schaller, G. E., and Kieber, J. J. (2018). Cytokinin modulates context-dependent chromatin accessibility through the type-B response regulators. Nat. Plants 4, 1102–1111. doi: 10.1038/s41477-018-0290-y
Potuschak, T., Lechner, E., Parmentier, Y., Yanagisawa, S., Grava, S., Koncz, C., et al. (2003). EIN3-dependent regulation of plant ethylene hormone signaling by two Arabidopsis F box proteins: EBF1 and EBF2. Cell 115, 679–689. doi: 10.1016/S0092-8674(03)00968-1
Qiao, H., Chang, K. N., Yazaki, J., and Ecker, J. R. (2009). Interplay between ethylene, ETP1/ETP2 F-box proteins, and degradation of EIN2 triggers ethylene responses in Arabidopsis. Genes Dev. 23, 512–521. doi: 10.1101/gad.1765709
Qiao, H., Shen, Z., Huang, S. S. C., Schmitz, R. J., Urich, M. A., Briggs, S. P., et al. (2012). Processing and subcellular trafficking of ER-tethered EIN2 control response to ethylene gas. Science 338, 390–393. doi: 10.1126/science.1225974
Quail, P. H., Boylan, M. T., Parks, B. M., Short, T. W., Xu, Y., and Wagner, D. (1995). Phytochromes: photosensory perception and signal transduction. Science 268, 675–680. doi: 10.1126/science.7732376
Radchuk, R., Radchuk, V., Weschke, W., Borisjuk, L., and Weber, H. (2006). Repressing the expression of the SUCROSE NONFERMENTING-1-RELATED PROTEIN KINASE gene in pea embryo causes pleiotropic defects of maturation similar to an abscisic acid-insensitive phenotype. Plant Physiol. 140, 263–278. doi: 10.1104/pp.105.071167
Raines, T., Blakley, I. C., Tsai, Y. C., Worthen, J. M., Franco-Zorrilla, J. M., Solano, R., et al. (2016). Characterization of the cytokinin-responsive transcriptome in rice. BMC Plant Biol. 16:260. doi: 10.1186/s12870-016-0932-z
Rashotte, A. M., Mason, M. G., Hutchison, C. E., Ferreira, F. J., Schaller, G. E., and Kieber, J. J. (2006). A subset of Arabidopsis AP2 transcription factors mediates cytokinin responses in concert with a two-component pathway. Proc. Natl. Acad. Sci. U.S.A. 103, 11081–11085. doi: 10.1073/pnas.0602038103
Rockwell, N. C., Su, Y. S., and Lagarias, J. C. (2006). Phytochrome structure and signaling mechanisms. Annu. Rev. Plant Biol. 57, 837–858. doi: 10.1146/annurev.arplant.56.032604.144208
Rodríguez-Gacio Mdel, C., Matilla-Vázquez, M. A., and Matilla, A. J. (2009). Seed dormancy and ABA signaling: the breakthrough goes on. Plant Signal. Behav. 4, 1035–1049. doi: 10.4161/psb.4.11.9902
Roeber, V. M., Bajaj, I., Rohde, M., Schmülling, T., and Cortleven, A. (2020). Light acts as a stressor and influences abiotic and biotic stress responses in plants. Plant. Cell Environ. 1–20. doi: 10.1111/pce.13948
Rusaczonek, A., Czarnocka, W., Kacprzak, S., Witoń, D., Ślesak, I., Szechyńska-Hebda, M., et al. (2015). Role of phytochromes A and B in the regulation of cell death and acclimatory responses to UV stress in Arabidopsis thaliana. J. Exp. Bot. 6679–6695. doi: 10.1093/jxb/erv375
Sah, S. K., Reddy, K. R., and Li, J. (2016). Abscisic acid and abiotic stress tolerance in crop plants. Front. Plant Sci. 7:571. doi: 10.3389/fpls.2016.00571
Salomé, P. A., To, J. P. C., Kieber, J. J., and McClung, C. R. (2006). Arabidopsis response regulators ARR3 and ARR4 play cytokinin-independent roles in the control of circadian period. Plant Cell 18, 55–69. doi: 10.1105/tpc.105.037994
Sami, F., Faizan, M., Faraz, A., Siddiqui, H., Yusuf, M., and Hayat, S. (2018). Nitric oxide-mediated integrative alterations in plant metabolism to confer abiotic stress tolerance, NO crosstalk with phytohormones and NO-mediated post translational modifications in modulating diverse plant stress. Nitric Oxide Biol. Chem. 73, 22–38. doi: 10.1016/j.niox.2017.12.005
Samsonová, Z., Kiran, N. S., Novák, O., Spyroglou, I., Skalák, J., Hejátko, J., et al. (2020). Steady-state levels of cytokinins and their derivatives may serve as a unique classifier of arabidopsis ecotypes. Plants 9:116. doi: 10.3390/plants9010116
Schaller, G. E., and Bleecker, A. B. (1995). Ethylene-binding sites generated in yeast expressing the Arabidopsis ETR1 gene. Science 270, 1809–1811. doi: 10.1126/science.270.5243.1809
Scharein, B., Voet-van-Vormizeele, J., Harter, K., and Groth, G. (2008). Ethylene signaling: identification of a putative ETR1-AHP1 phosphorelay complex by fluorescence spectroscopy. Anal. Biochem. 377, 72–76. doi: 10.1016/j.ab.2008.03.015
Schepetilnikov, M., and Ryabova, L. A. (2018). Recent discoveries on the role of tor (Target of rapamycin) signaling in translation in plants. Plant Physiol. 176, 1095–1105. doi: 10.1104/pp.17.01243
Sharp, R. E., and Lenoble, M. E. (2002). ABA, ethylene and the control of shoot and root growth under water stress. J. Exp. Bot. 53, 33–37. doi: 10.1093/jxb/53.366.33
Sheerin, D. J., Menon, C., zur Oven-Krockhaus, S., Enderle, B., Zhu, L., Johnen, P., et al. (2015). Light-activated Phytochrome A and B interact with members of the SPA family to promote photomorphogenesis in Arabidopsis by reorganizing the COP1/SPA complex. Plant Cell 27, 189–201. doi: 10.1105/tpc.114.134775
Shi, X., Gupta, S., and Rashotte, A. M. (2014). Characterization of two tomato AP2/ERF genes, SlCRF1 and SlCRF2 in hormone and stress responses. Plant Cell Rep. 33, 35–45. doi: 10.1007/s00299-013-1510-6
Shi, Y., Tian, S., Hou, L., Huang, X., Zhang, X., Guo, H., et al. (2012). Ethylene signaling negatively regulates freezing tolerance by repressing expression of and Type-A genes in Arabidopsis. Plant Cell 24, 2578–2595. doi: 10.1105/tpc.112.098640
Shin, D. H., Choi, M., Kim, K., Bang, G., Cho, M., Choi, S.-B., et al. (2013). HY5 regulates anthocyanin biosynthesis by inducing the transcriptional activation of the MYB75/PAP1 transcription factor in Arabidopsis. FEBS Lett. 587, 1543–1547. doi: 10.1016/j.febslet.2013.03.037
Shin, J., Park, E., and Choi, G. (2007). PIF3 regulates anthocyanin biosynthesis in an HY5-dependent manner with both factors directly binding anthocyanin biosynthetic gene promoters in Arabidopsis. Plant J. 49, 981–994. doi: 10.1111/j.1365-313X.2006.03021.x
Siegel, R. S., Xue, S., Murata, Y., Yang, Y., Nishimura, N., Wang, A., et al. (2009). Calcium elevation-dependent and attenuated resting calcium-dependent abscisic acid induction of stomatal closure and abscisic acid-induced enhancement of calcium sensitivities of S-type anion and inward-rectifying K+ channels in Arabidopsis guard cells. Plant J. 59, 207–220. doi: 10.1111/j.1365-313X.2009.03872.x
Skalák, J., Černý, M., Jedelský, P., Dobrá, J., Ge, E., Novák, J., et al. (2016). Stimulation of ipt overexpression as a tool for elucidation of the role of cytokinins in high temperature responses of Arabidopsis thaliana. J. Exp. Bot. 67, 2861–2873. doi: 10.1093/jxb/erw129
Skirycz, A., Claeys, H., De Bodt, S., Oikawa, A., Shinoda, S., Andriankaja, M., et al. (2011). Pause-and-stop: the effects of osmotic stress on cell proliferation during early leaf development in Arabidopsis and a role for ethylene signaling in cell cycle arrest. Plant Cell 23, 1876–1888. doi: 10.1105/tpc.111.084160
Soma, F., Takahashi, F., Suzuki, T., Shinozaki, K., and Yamaguchi-Shinozaki, K. (2020). Plant Raf-like kinases regulate the mRNA population upstream of ABA-unresponsive SnRK2 kinases under drought stress. Nat. Commun. 11:1373. doi: 10.1038/s41467-020-15239-3
Soto-Burgos, J., and Bassham, D. C. (2017). SnRK1 activates autophagy via the TOR signaling pathway in Arabidopsis thaliana. PLoS One 12:e0182591. doi: 10.1371/journal.pone.0182591
Srivastava, A. K., Senapati, D., Srivastava, A. K., Chakraborty, M., Gangappa, S. N., and Chattopadhyay, S. (2015). SHW1 interacts with HY5 and COP1, and promotes COP1-mediated degradation of HY5 during Arabidopsis seedling development. Plant Physiol. 169, 2922–2934. doi: 10.1104/pp.15.01184
Stavang, J. A., Gallego-Bartolomé, J., Gómez, M. D., Yoshida, S., Asami, T., Olsen, J. E., et al. (2009). Hormonal regulation of temperature-induced growth in Arabidopsis. Plant J. 60, 589–601. doi: 10.1111/j.1365-313X.2009.03983.x
Street, I. H., Aman, S., Zubo, Y., Ramzan, A., Wang, X., Shakeel, S. N., et al. (2015). Ethylene inhibits cell proliferation of the Arabidopsis root meristem. Plant Physiol. 169, 338–350. doi: 10.1104/pp.15.00415
Sung, D. Y., Kaplan, F., Lee, K. J., and Guy, C. L. (2003). Acquired tolerance to temperature extremes. Trends Plant Sci. 8, 179–187. doi: 10.1016/S1360-1385(03)00047-5
Sweere, U., Eichenberg, K., Lohrmann, J., Mira-Rodado, V., Bäurle, I., Kudla, J., et al. (2001). Interaction of the response regulator ARR4 with phytochrome B in modulating red light signaling. Science 294, 1108–1111. doi: 10.1126/science.1065022
Takatsuka, H., and Umeda, M. (2019). ABA inhibits root cell elongation through repressing the cytokinin signaling. Plant Signal. Behav. 14:e1578632. doi: 10.1080/15592324.2019.1578632
Terry, M. J., Linley, P. J., and Kohchi, T. (2002). Making light of it: the role of plant haem oxygenases in phytochrome chromophore synthesis. Biochem. Soc. Trans. 30, 604–609. doi: 10.1042/bst0300604
Todaka, D., Zhao, Y., Yoshida, T., Kudo, M., Kidokoro, S., Mizoi, J., et al. (2017). Temporal and spatial changes in gene expression, metabolite accumulation and phytohormone content in rice seedlings grown under drought stress conditions. Plant J. 90, 61–78. doi: 10.1111/tpj.13468
Tran, L. S. P., Shinozaki, K., and Yamaguchi-Shinozaki, K. (2010). Role of cytokinin responsive two-component system in ABA and osmotic stress signalings. Plant Signal. Behav. 5, 148–150. doi: 10.4161/psb.5.2.10411
Tran, L.-S. P., Urao, T., Qin, F., Maruyama, K., Kakimoto, T., Shinozaki, K., et al. (2007). Functional analysis of AHK1/ATHK1 and cytokinin receptor histidine kinases in response to abscisic acid, drought, and salt stress in Arabidopsis. Proc. Natl. Acad. Sci. U.S.A. 104, 20623–20628. doi: 10.1073/pnas.0706547105
Tun, N. N., Livaja, M., Kieber, J. J., and Scherer, G. F. E. (2008). Zeatin-induced nitric oxide (NO) biosynthesis in Arabidopsis thaliana mutants of NO biosynthesis and of two-component signaling genes. New Phytol. 178, 515–531. doi: 10.1111/j.1469-8137.2008.02383.x
Urao, T., Miyata, S., Yamaguchi-Shinozaki, K., and Shinozaki, K. (2000). Possible his to Asp phosphorelay signaling in an Arabidopsis two- component system. FEBS Lett. 478, 227–232. doi: 10.1016/S0014-5793(00)01860-3
Urao, T., Yakubov, B., Satoh, R., Yamaguchi-Shinozaki, K., Seki, M., Hirayama, T., et al. (1999). A transmembrane hybrid-type histidine kinase in Arabidopsis functions as an osmosensor. Plant Cell 11, 1743–1754. doi: 10.1105/tpc.11.9.1743
Vandenbussche, F., Habricot, Y., Condiff, A. S., Maldiney, R., Van Der Straeten, D., and Ahmad, M. (2007). HY5 is a point of convergence between cryptochrome and cytokinin signalling pathways in Arabidopsis thaliana. Plant J. 49, 428–441. doi: 10.1111/j.1365-313X.2006.02973.x
Vishwakarma, K., Upadhyay, N., Kumar, N., Yadav, G., Singh, J., Mishra, R. K., et al. (2017). Abscisic acid signaling and abiotic stress tolerance in plants: a review on current knowledge and future prospects. Front. Plant Sci. 8:161. doi: 10.3389/fpls.2017.00161
Waidyarathne, P., and Samarasinghe, S. (2018). Boolean calcium signalling model predicts calcium role in acceleration and stability of abscisic acid-mediated stomatal closure. Sci. Rep. 8:17635. doi: 10.1038/s41598-018-35872-9
Wang, F., Guo, Z., Li, H., Wang, M., Onac, E., Zhou, J., et al. (2016). Phytochrome A and B function antagonistically to regulate cold tolerance via abscisic acid-dependent jasmonate signaling. Plant Physiol. 170, 459–471. doi: 10.1104/pp.15.01171
Wang, H. (2015). Phytochrome signaling: time to tighten up the loose ends. Mol. Plant 8, 540–551. doi: 10.1016/j.molp.2014.11.021
Wang, P., Zhao, Y., Li, Z., Hsu, C. C., Liu, X., Fu, L., et al. (2018). Reciprocal regulation of the TOR Kinase and ABA receptor balances plant growth and stress response. Mol. Cell 69, 100.e10–112.e10. doi: 10.1016/j.molcel.2017.12.002
Wang, Q., Liu, Q., Wang, X., Zuo, Z., Oka, Y., and Lin, C. (2018). New insights into the mechanisms of phytochrome–cryptochrome coaction. New Phytol. 217, 547–551. doi: 10.1111/nph.14886
Wang, Y., Li, L., Ye, T., Zhao, S., Liu, Z., Feng, Y. Q., et al. (2011). Cytokinin antagonizes ABA suppression to seed germination of Arabidopsis by downregulating ABI5 expression. Plant J. 68, 249–261. doi: 10.1111/j.1365-313X.2011.04683.x
Webb, A. A. R., Larman, M. G., Montgomery, L. T., Taylor, J. E., and Hetherington, A. M. (2001). The role of calcium in ABA-induced gene expression and stomatal movements. Plant J. 26, 351–362. doi: 10.1046/j.1365-313X.2001.01032.x
Wen, X., Zhang, C., Ji, Y., Zhao, Q., He, W., An, F., et al. (2012). Activation of ethylene signaling is mediated by nuclear translocation of the cleaved EIN2 carboxyl terminus. Cell Res. 22, 1613–1616. doi: 10.1038/cr.2012.145
Wilson, R. L., Bakshi, A., and Binder, B. M. (2014a). Loss of the ETR1 ethylene receptor reduces the inhibitory effect of far-red light and darkness on seed germination of Arabidopsis thaliana. Front. Plant Sci. 5:433. doi: 10.3389/fpls.2014.00433
Wilson, R. L., Kim, H., Bakshi, A., and Binder, B. M. (2014b). The ethylene receptors ethylene response1 and ethylene response2 have contrasting roles in seed germination of arabidopsis during salt stress. Plant Physiol. 165, 1353–1366. doi: 10.1104/pp.114.241695
Wright, S. T. C. (1977). The relationship between leaf water potential ψleaf and the levels of abscisic acid and ethylene in excised wheat leaves. Planta 134, 183–189. doi: 10.1007/BF00384969
Wulfetange, K., Lomin, S. N., Romanov, G. A., Stolz, A., Heyl, A., and Schmülling, T. (2011). The cytokinin receptors of arabidopsis are located mainly to the endoplasmic reticulum. Plant Physiol. 156, 1808–1818. doi: 10.1104/pp.111.180539
Xiong, L., Schumaker, K. S., and Zhu, J.-K. (2002). Cell signaling during cold, drought, and salt stress. Plant Cell 14, S165–S183. doi: 10.1105/tpc.000596
Xu, X., Paik, I., Zhu, L., and Huq, E. (2015). Illuminating progress in phytochrome-mediated light signaling pathways. Trends Plant Sci. 20, 641–650. doi: 10.1016/j.tplants.2015.06.010
Yaish, M. W., Colasanti, J., and Rothstein, S. J. (2011). The role of epigenetic processes in controlling flowering time in plants exposed to stress. J. Exp. Bot. 62, 3727–3735. doi: 10.1093/jxb/err177
Yamada, H., Suzuki, T., Terada, K., Takei, K., Ishikawa, K., Miwa, K., et al. (2001). The arabidopsis AHK4 histidine kinase is a cytokinin-binding receptor that transduces cytokinin signals across the membrane. Plant Cell Physiol. 42, 1017–1023. doi: 10.1093/pcp/pce127
Yu, F., Wu, Y., and Xie, Q. (2016). Ubiquitin-proteasome system in ABA signaling: from perception to action. Mol. Plant 9, 21–33. doi: 10.1016/j.molp.2015.09.015
Yuan, L., Liu, Z., Song, X., Johnson, C., Yu, X., and Sundaresan, V. (2016). The CKI1 histidine kinase specifies the female gametic precursor of the endosperm. Dev. Cell 37, 34–46. doi: 10.1016/j.devcel.2016.03.009
Zdarska, M., Cuyacot, A. R., Tarr, P. T., Yamoune, A., Szmitkowska, A., Hrdinová, V., et al. (2019). ETR1 integrates response to ethylene and cytokinins into a single multistep phosphorelay pathway to control root growth. Mol. Plant 12, 1338–1352. doi: 10.1016/j.molp.2019.05.012
Zdarska, M., Dobisová, T., Gelová, Z., Pernisová, M., Dabravolski, S., and Hejátko, J. (2015). Illuminating light, cytokinin, and ethylene signalling crosstalk in plant development. J. Exp. Bot. 66, 4913–4931. doi: 10.1093/jxb/erv261
Zhang, F., Wang, L., Qi, B., Zhao, B., Ko, E. E., Riggan, N. D., et al. (2017). EIN2 mediates direct regulation of histone acetylation in the ethylene response. Proc. Natl. Acad. Sci. U.S.A. 114, 10274–10279. doi: 10.1073/pnas.1707937114
Zhao, H., Duan, K.-X., Ma, B., Yin, C.-C., Hu, Y., Tao, J.-J., et al. (2020). Histidine kinase MHZ1/OsHK1 interacts with ethylene receptors to regulate root growth in rice. Nat. Commun. 11:518. doi: 10.1038/s41467-020-14313-0
Zhao, X. C., and Schaller, G. E. (2004). Effect of salt and osmotic stress upon expression of the ethylene receptor ETR1 in Arabidopsis thaliana. FEBS Lett. 562, 189–192. doi: 10.1016/S0014-5793(04)00238-8
Zhong, S., Zhao, M., Shi, T., Shi, H., An, F., Zhao, Q., et al. (2009). EIN3/EIL1 cooperate with PIF1 to prevent photo-oxidation and to promote greening of Arabidopsis seedlings. Proc. Natl. Acad. Sci. U.S.A. 106, 21431–21436. doi: 10.1073/pnas.0907670106
Zhou, W., Lozano-Torres, J. L., Blilou, I., Zhang, X., Zhai, Q., Smant, G., et al. (2019). A jasmonate signaling network activates root stem cells and promotes regeneration. Cell 177, 942.e14–956.e14. doi: 10.1016/j.cell.2019.03.006
Zhu, L., Bu, Q., Xu, X., Paik, I., Huang, X., Hoecker, U., et al. (2015). CUL4 forms an E3 ligase with COP1 and SPA to promote light-induced degradation of PIF1. Nat. Commun. 6:7245. doi: 10.1038/ncomms8245
Źróbek-Sokolnik, A. (2012). Temperature stress and responses of plants, eds P. Ahmad and M. N. V. Prasad (Berlin: Springer).
Zubo, Y. O., Blakley, I. C., Yamburenko, M. V., Worthen, J. M., Street, I. H., Franco-Zorrilla, J. M., et al. (2017). Cytokinin induces genome-wide binding of the type-B response regulator ARR10 to regulate growth and development in Arabidopsis. Proc. Natl. Acad. Sci. U.S.A. 114, E5995–E6004. doi: 10.1073/pnas.1620749114
Zürcher, E., and Müller, B. (2016). Cytokinin synthesis, signaling, and function-advances and new insights. Int. Rev. Cell Mol. Biol. 324, 1–38. doi: 10.1016/bs.ircmb.2016.01.001
Zwack, P. J., De Clercq, I., Howton, T. C., Hallmark, H. T., Hurny, A., Keshishian, E. A., et al. (2016). Cytokinin response factor 6 represses cytokinin-associated genes during oxidative stress. Plant Physiol. 172, 1249–1258. doi: 10.1104/pp.16.00415
Zwack, P. J., and Rashotte, A. M. (2015). Interactions between cytokinin signalling and abiotic stress responses. J. Exp. Bot. 66, 4863–4871. doi: 10.1093/jxb/erv172
Keywords: multistep phosphorelay (MSP), cytokinin, ethylene, abscisic acid, light signaling, temperature, abiotic stress, Arabidopsis
Citation: Skalak J, Nicolas KL, Vankova R and Hejatko J (2021) Signal Integration in Plant Abiotic Stress Responses via Multistep Phosphorelay Signaling. Front. Plant Sci. 12:644823. doi: 10.3389/fpls.2021.644823
Received: 21 December 2020; Accepted: 26 January 2021;
Published: 17 February 2021.
Edited by:
Alejandro Ferrando, Universitat Politècnica de València, SpainReviewed by:
Borja Belda-Palazon, Instituto Gulbenkian de Ciência (IGC), PortugalCopyright © 2021 Skalak, Nicolas, Vankova and Hejatko. This is an open-access article distributed under the terms of the Creative Commons Attribution License (CC BY). The use, distribution or reproduction in other forums is permitted, provided the original author(s) and the copyright owner(s) are credited and that the original publication in this journal is cited, in accordance with accepted academic practice. No use, distribution or reproduction is permitted which does not comply with these terms.
*Correspondence: Jan Hejatko, aGVqYXRrb0BzY2kubXVuaS5jeg==
†These authors have contributed equally to this work
Disclaimer: All claims expressed in this article are solely those of the authors and do not necessarily represent those of their affiliated organizations, or those of the publisher, the editors and the reviewers. Any product that may be evaluated in this article or claim that may be made by its manufacturer is not guaranteed or endorsed by the publisher.
Research integrity at Frontiers
Learn more about the work of our research integrity team to safeguard the quality of each article we publish.