- 1College of Life Sciences, Chongqing Normal University, Chongqing, China
- 2Biological Science Research Center, Southwest University, Chongqing, China
- 3College of Resources and Environment Science, Southwest University, Chongqing, China
Plant growth-promoting bacteria (PGPB) are components of the plant rhizosphere that promote plant growth and/or inhibit pathogen activity. To explore the cotton seedlings response to Bacillus circulans GN03 with high efficiency of plant growth promotion and disease resistance, a pot experiment was carried out, in which inoculations levels of GN03 were set at 104 and 108 cfu⋅mL–1. The results showed that GN03 inoculation remarkably enhanced growth promotion as well as disease resistance of cotton seedlings. GN03 inoculation altered the microbiota in and around the plant roots, led to a significant accumulation of growth-related hormones (indole acetic acid, gibberellic acid, and brassinosteroid) and disease resistance-related hormones (salicylic acid and jasmonic acid) in cotton seedlings, as determined with ELISA, up-regulated the expression of phytohormone synthesis-related genes (EDS1, AOC1, BES1, and GA20ox), auxin transporter gene (Aux1), and disease-resistance genes (NPR1 and PR1). Comparative genomic analyses was performed between GN03 and four similar species, with regards to phenotype, biochemical characteristics, and gene function. This study provides valuable information for applying the PGPB alternative, GN03, as a plant growth and disease-resistance promoting fertilizer.
Introduction
In recent years, there has been considerable interest in plant growth-promoting bacteria (PGPB), which are free-living bacteria in the surrounding rhizosphere or in plant. PGPB can improve plant nutrient uptake, enhance plant growth and yield (Lugtenberg and Kamilova, 2009; Hassan et al., 2019), induce resistance against pathogens in plants, and aggressively colonize the root rhizosphere (Lugtenberg and Kamilova, 2009; Emmanuel and Babalola, 2020). The effect of PGPB is primarily explained by their ability to secrete metabolites (Mahmood and Kataoka, 2020) that are able to: (a) stimulate plant growth by inducing the production and release of plant growth regulators or phytohormones such as indole acetic acid (IAA) and gibberellic acid (GA); (b) enhance asymbiotic N2-fixation; (c) solubilize inorganic phosphate and mineralize organic phosphate and/or other nutrients; and (d) resist, tolerate, or compete with detrimental microorganisms (Lugtenberg and Kamilova, 2009; Ramakrishna et al., 2019). PGPB also indirectly promote plant growth by improving the diversity of the rhizosphere microbiome and entering inside the roots to trigger induced systemic resistance (ISR), thereby further antagonizing soil borne pathogens (Araujo et al., 2019; Dahmani et al., 2020).
At present, more than 20 species and genera of PGPB strains, including Agrobacterium, Azospirillum, Azotobacter, Bacillus, Burkholderia, Enterobacter, Klebsiella, and Pseudomonas, have been identified (Aloo et al., 2019). Among them, Bacillus is an important genus that can form long-lived, stress tolerant spores existing inside or outside the plant roots under adverse environmental conditions (Gadhave et al., 2018). It has strong saprophytic ability and competitiveness and is capable of secreting metabolites that stimulate plant growth and prevent pathogen infection (Qiao et al., 2017; Aloo et al., 2019; Hashem et al., 2019). Some studies have reported that three principal plant-associated bacteria, Bacillus cereus, Bacillus subtilis and Bacillus amyloliquefaciens, are colonizers of both the in- and outside of plant roots during the growth stage and can improve nutrient utilization, vegetative growth, flowering and fruit ripening, as well as resistance to diseases, insect pests, and environmental stress (Lugtenberg and Kamilova, 2009; Gadhave et al., 2018). However, these studies did not examine the impact of these bacteria on the rhizosphere and endophytic microflora (Qiao et al., 2017); thus, there remains a disconnect between the theoretical research and the practical application of PGPB (Ramakrishna et al., 2019; Alka et al., 2020). For example, the application of a fertilizer with the incorporation of PGPB reportedly showed inconsistent results between laboratory and field applications (Parnell et al., 2016). Currently, limited information is available on the intrinsic relationship between the bacteria and the soil rhizosphere habitat (soil depth, temperature, pH, and intra-species relationships) (Ahemad and Kibret, 2014), further implying that there is a gap in understanding the mechanistic interaction between PGPB and their host plants. In view of this, it is important to determine the impact of these Bacillus spp. inoculants on the native microbial community, to effectively apply the theory in field. The potential application of Bacillus in the production of a few crops, such as soybean, wheat, and rice (Khatri et al., 2020; Lee et al., 2020; Wang et al., 2020), and its effects on the growth promotion and disease resistance enhancement of upland cotton (Gossypium hirsutum L.) are rarely reported.
Upland cotton is an important cultivated oil and fiber crop worldwide; however, it is prone to the attack by the soil-borne hemibiotrophic fungus Verticillium dahliae associated with Verticillium wilt (Zhang et al., 2013). As microsclerotia, a dormant survival structure formed by V. dahliae, can exist in the soil for a long time, Verticillium wilt is considered the most destructive disease of cotton and is extremely difficult to control (Wang et al., 2016; Zhang et al., 2017a). Breeding of upland cotton varieties with disease resistance is limited by more variation in V. dahliae strains and the lack of V. dahliae resistant germplasm in upland cotton (Zhang et al., 2017a, 2021). Some chemicals, such as benomyl and acibenzolar-S-methyl, seem to work for Verticillium wilt, but they are not environment friendly (Goicoechea, 2009). Therefore, application of PGPB to control this disease is a safer and better alternative to protect upland cotton from Verticillium wilt (Hasan et al., 2020). Accordingly, beneficial PGPB with inhibitory action against V. dahliae are a promising biocontrol agent for the control of cotton Verticillium wilt. To date, some researchers have isolated multiple strains with biocontrol activities against V. dahliae from Bacillus, Enterobacter, Paenibacillus, Pseudomonas, Serratia, and Streptomyces genera (Erdogan and Benlioglu, 2010; Zhang et al., 2018; Cheng et al., 2020; Hasan et al., 2020; Sherzad and Canming, 2020; Tao et al., 2020; Zhang et al., 2020).
Bacillus circulans was first isolated and described in 1890 by Jordon. It is a Gram-positive bacterium (Nakamura and Swezey, 1983) with the ability to secrete polysaccharide degrading enzymes such as β-1,3 and β-1,6 glucanases, β-1,3 and β-1,4-glucanases, α-l,3-glucanases, amylase, chitinase, and xylanase (Tanaka and Watanabe, 1995). Several studies have shown that the B. circulans strain has the ability to promote plant growth and can be developed as a biological fertilizer (Mehta et al., 2010, 2015; Bokhari et al., 2019).
In this study, upland cotton seedlings were applied as the experimental host plant to investigate the plant growth-promoting mechanism and disease-resistance effect of B. circulans GN03 isolated from purple soil. Furthermore, we provided a full overview of the properties attributed by the GN03, and performed its comparative genomic analysis with four other B. circulans strains. The results of this study provide useful information for promoting sustainable agricultural practices for improving the soil environment and crop yield while also providing a basis for more in-depth microbial species interaction research.
Materials and Methods
Bacterial Strain
The GN03 strain was isolated from the root surface of pakchoi cabbage (Brassica chinensis) grown in purple soil in an agricultural field in the Beibei District (30°26′12′′ N, 106°26′25′′ E), Chongqing, China (Shen et al., 2018). Bacterial suspensions were obtained by culturing cells in 50 mL of LB medium (10 g tryptone, 5 g yeast extract, and 10 g NaCl⋅L–1) in 300 mL flasks on a rotary shaker (150 rpm) at 37°C for 12 h. Scanning electron microscopy was used for morphological observations (Golding et al., 2016). The spore staining, Gram staining, and biochemical characteristics were evaluated according to Mehnaz et al. (2010).
Molecular Identification
After culturing GN03 in LB medium for 48 h (37°C, 150 rpm), the bacterial cells were collected by centrifugation (4,500 × g for 5 min at 4°C). To preliminarily confirm the strain of bacteria isolated, PCR (94°C for 10 min followed by 34 cycles at 94°C for 30 s, 56°C for 30 s, and 72°C for 90 s, with a final extension at 72°C for 10 min) was performed with universal 16S primers 27F (5′-AGAGTTTGATCCTGGCTCAG-3′) and 1492R (5′-TACGGTTACCTTGTTACGACTT-3′). The PCR products were sequenced by BGI (Shenzhen, China), and the resultant sequences were compared with those hosted on the GenBank database using BLAST. Genomic DNA was isolated from the cell pellets with a Bacteria DNA Kit (OMEGA) according to the manufacturer’s instructions, and quantified using TBS-380 fluorometer (BioSystems, CA).
Evaluation of Growth Promotion and Disease Resistance in Cotton Seedlings
Bacteria were resuspended and diluted in deionized water (0 cfu⋅mL–1 for the Mock group, 104 cfu⋅mL–1 for the C1 group and 108 cfu⋅mL–1 for the C2 group). Upland cotton (G. hirsutum ‘Yumian-1’) seeds kindly provided by Dr. Zhengsheng Zhang (Southwest University, China), underwent surface sterilization with 20% (v/v) H2O2 for 2 min, and were then individually sown into plastic pots (12 cm diameter and 16 cm height). The soil was collected at 0–15 cm depth from the campus (30°36′45′′N, 106°17′59′′E, 261 m above sea level) of Chongqing Normal University, China. After being sieved (<1 mm) and air-dried, the soil contained 17.19 ± 0.62 g⋅kg–1 organic matter, 40.76 ± 2.86, 70.19 ± 2.01, and 93.84 ± 10.91 mg⋅kg–1 available nitrogen, phosphorus, and potassium, 0.78 ± 0.05, 1.02 ± 0.12, and 17.21 ± 0.38g⋅kg–1 total nitrogen, phosphorus, and potassium, respectively. Once two cotyledons started to unfold, 5 mL GN03 or an equal volume of deionized water was inoculated in rhizosphere (Supplementary Table 1). After 7 days, the plants were re-inoculated using the same method and concentration as in the initial inoculations. Throughout the experimental period, cotton seedlings were randomly placed in greenhouse conditions, wherein the average day/night period, daytime light intensity, temperature, and humidity were 12.5/11.5 h, 2,000–4,000 lux, 16–30°C and 50–80%, respectively. Twenty-three days after the second inoculation, indicators, including phenotypic data, hormone levels, gene expression, and microbial diversity, were measured. Three independent tests with 30 plants per replicate were performed.
The virulent defoliating V. dahliae strain V991, stored at −80°C, was first isolated from cotton originated in Xinjiang, China, and reactivated on potato dextrose agar (PDA, 200 g⋅L–1 potato, 20 g⋅L–1 dextrose, and 18 g⋅L–1 agar) medium at 25°C. After growing on PDA medium at 25°C for 7 days, mycelia were collected and cultured in potato dextrose broth (PDB, 200 g⋅L–1 potato and 20 g⋅L–1 dextrose) medium for 7 days at 25°C with constant shaking (150 rpm). Once the cotton seedlings presented with two true-leaves, they were inoculated with 5 mL GN03 suspensions (0, 104, and 108 cfu⋅mL–1, respectively) poured into the soil surrounding the roots. After 24 h, the pre-treated seedlings were inoculated with the V991 strain by injecting 10 mL spore suspensions (107 conidia⋅mL–1) into the soil around the roots, and control seedlings were inoculated with an equal volume of sterile distilled water (Zhou et al., 2013). The disease index was calculated by assessing 30 individual plants per treatment and repeated three times with the following formula: disease index = Σ (number of disease leaves × disease grade)/(total number of leaves × 4) × 100. The disease grade was classified as follows: 0 (no symptoms), 1 (>0–25% yellowing or wilting leaves), 2 (25–50% yellowing or wilting leaves), 3 (50–75% yellowing or wilting leaves), and 4 (75–100% yellowing or wilting leaves) (Luo et al., 2020). The assessment was performed every 3 days for 21 days post inoculation (dpi) V991.
Evaluation of Hormone Levels, Growth, and Disease Resistance-Related Gene Expression
The contents of IAA, GA, brassinosteroid (BR), salicylic acid (SA), and jasmonic acid (JA) in cotton leaves were measured using the corresponding ELISA Kits (Shanghai Preferred Biotechnology, China), with minimum detection concentration of less than 0.1 nM and accuracy of more than 99%. To determine the expression of genes related to growth and disease resistance, RNA was extracted from cotton roots using a MiniBEST plant RNA Extraction Kit (TaKaRa, Japan) and a RT reagent Kit for Perfect Real Time (TaKaRa) was used to obtain cDNA. Ghhistone3 (the gene of G. hirsutum histone 3) was used as the reference gene in the quantitative reverse transcription-PCR (RT-qPCR). The expression of growth-promoting and disease resistance-related genes in the cotton root was measured in 10 μL PCR reactions containing 5 μL SYBR Green Real-time PCR Master Mix (Bio-Rad, Hercules, CA, United States), 1 μL root cDNA, 2 μL ultrapure water, and 1 μL each of the 10 μM forward and reverse primers (Supplementary Table 2). The following RT-qPCR protocol was used in a CFX96 instrument (Bio-Rad): 94°C for 2 min followed by 39 cycles of 94°C for 5 s and 60°C for 30 s, then 95°C for 5 s, 65°C for 5 s, and 95°C for 5 s.
Assessing the Rhizosphere and Endophytic Microbiota
The cotton roots with rhizosphere soils were placed in 0.02 M phosphate-buffered saline (pH 6.8) and incubated at 180 rpm for 20 min. After taking out the plant roots, the suspension was centrifuged at 12,000 × g for 10 min to collect the sediment containing the rhizosphere soil samples. The sediment was rinsed with 70% ethanol for 2 min and then with sterile water (5 times), whereas the removed cotton roots from the previous step were analyzed for endophytic microbial content. Total microbial genomic DNA was extracted from both the cotton roots and the rhizosphere soil samples using the DNeasy PowerSoil Kit (Qiagen, Hilden, Germany), following the manufacturer’s instructions, and stored at −20°C for further analysis.
PCR amplification of the bacterial 16S rRNA genes V3–V4 region was performed using the forward primer 38F (5′-ACTCCTACGGGAGGCAGCA-3′) and the reverse primer 806R (5′-GGACTACHVGGGTWTCTAAT-3′) (Xu et al., 2016). PCR amplicons were purified with Agencourt AMPure Beads (Beckman Coulter, Brea, CA, United States) and quantified using the PicoGreen dsDNA Assay Kit (Invitrogen, Carlsbad, CA, United States). After the individual quantification step, amplicons were pooled in equal amounts, and paired-end 2 × 300 bp sequencing was performed using the Illumina MiSeq platform with MiSeq Reagent Kit v3 at Shanghai Personal Biotechnology (China). The data were analyzed on the free online Majorbio Cloud Platform1. Operational taxonomic units (OTUs) were clustered at 97% similarity; rarefaction curves (Kemp and Aller, 2004) were prepared using a reasonable amount of sequencing data (Supplementary Figure 1), which produced a flat curve meaning that the amount of sequencing data was large enough to reflect the vast majority of microbial diversity information in the sample. The unclassified bacterial phyla were not applied to analyses. In addition, reads representing chloroplasts were removed prior to further analyses as chloroplasts are abundant in cotton roots.
Whole Genome Sequencing and Comparative Genome Analysis
The genome was sequenced by Genedenovo (Guangzhou, China) using a combination of the PacBio RS II system (Menlo Park, CA, United States) and Illumina sequencing platforms (San Diego, CA, United States). The GN03 gene model identified by Glimmer V3 was got by the Ab initio prediction method (Delcher et al., 2007). Then, all gene models were blasted against the non-redundant (NR)2, SwissProt3, KEGG4, and COG5 databases for functional annotation using the BLASTp module. In addition, tRNAs were identified using tRNAscan-SE (v1.236) and rRNAs were determined using RNAmmer (v1.27).
Comparative genomic analysis was carried out by comparing the genome sequence of the GN03 strain with that of four other B. circulans stains (Supplementary Table 3). Among them, PK3-109 and PK3-138 strains were isolated from plant root endophytes that grow in the Thar Desert, India (Bokhari et al., 2019); RIT379 strain was isolated from the internal stem tissue of Costus igneus, which grows in Puerto Rico (Polter et al., 2015); and a model strain NCTC26108. PK3-109 strain has been shown to produce IAA and exopolysaccharide, and to increase the total fresh weight of Arabidopsis thaliana under conditions of salt stress (Bokhari et al., 2019).
Statistical Analysis
Biochemical and physiological measurements were evaluated using an analysis of variance (ANOVA) followed by Dunnett’s post hoc test using the Statistical Package for the Social Sciences, v22.0 (SPSS, Chicago, IL, United States). Means among treatments were considered significantly different when the probability (p-value) was less than 0.05. All data in the tables and figures are presented as the mean ± SE (standard error). Values were compared using a one-way ANOVA least significant difference (LSD) test, or a non-parametric Kruskal–Wallis test when the data were not normally distributed. The Bray–Curtis dissimilarity metric and analysis of similarities (ANOSIM) with 999 permutations were performed when comparing groups.
Data Deposition
16S rDNA amplicon raw sequencing data were deposited in the NCBI Short Read Archive (SRA) BioProject PRJNA631145 under the accession numbers SRR11735611-SRR11735637. The B. circulans GN03 genome was deposited under accession numbers CP053315 and CP053316 (the latter is for the plasmid).
Results
Morphological, Biochemical and Molecular Characteristics of GN03
Bacterial characterization of the GN03 strain revealed that it formed white round micro-bulge colonies with a small diameter and neat edges. The cells were rod-shaped and approximately 2 μm long. After fission, the daughter cells separated to form single cells (Figure 1A). GN03 presented as Gram-positive bacterium with oval-shaped mid-spores and capsular hypertrophy (Supplementary Figure 2). Biochemical characterization of GN03 revealed that it produced catalase and nitrate reductase. However, it did not produce phenylalanine deaminase or cytochrome oxidase c, neither degraded tryptophan to produce hydrazine, or use citrate as its sole carbon source. Sugar fermentation tests of GN03 resulted in the production of acid, but not gas. Fermentation of glucose by GN03 resulted in the production of acidic compounds as well as acetyl-methyl-methanol. Furthermore, GN03 was able to hydrolyze starch and liquefy gelatin (Supplementary Table 4). The characteristics of the GN03 strain conformed to the general characteristics of B. circulans (Buchanan et al., 1994). Sequencing of 16S rDNA and analysis via NCBI BLAST also revealed GN03 to be a strain of B. circulans with the genome characteristics of GN03, similar to the average B. circulans genomes recorded by the NCBI. Hence, the complete taxonomic descriptor is: Firmicutes, Bacilli, Bacillales, Bacillaceae, Bacillus, B. circulans GN03.
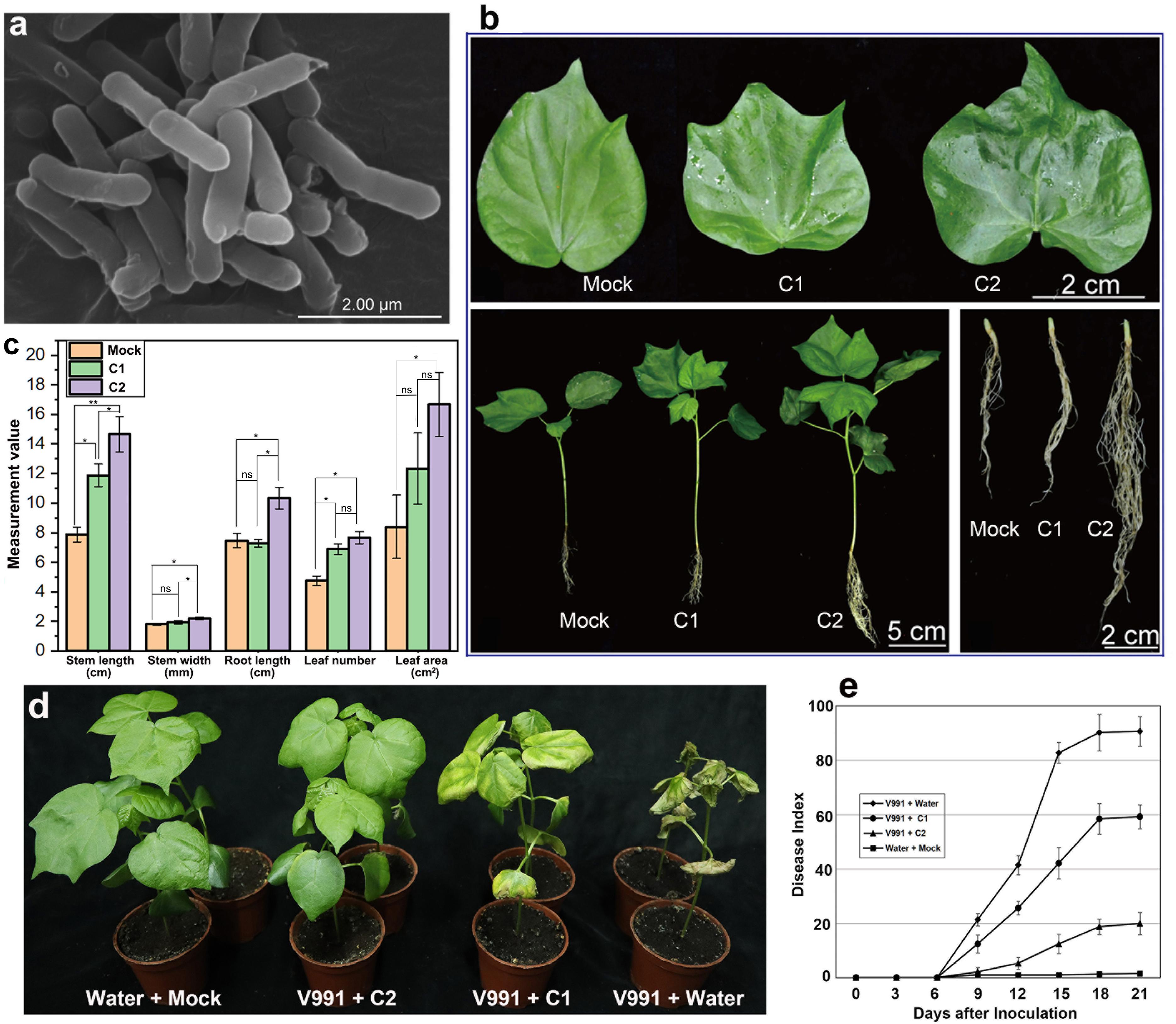
Figure 1. Morphological characteristics and phenotypic changes in cotton seedling 30 days after inoculation of GN03. (a) Scanning electron microscopic image of the bacterial cell after culture for 16 h at 37°C. (b) The phenotype of the leaf, whole plant and root in cotton seedlings 30 days after inoculation of GN03. (c) The biomass of cotton seedlings. Leaf area is measured as the area of the third leaf from the top bud. Statistical significance was evaluated by one-way ANOVA and LSD post hoc test (*p < 0.05, **p < 0.01; ns, not significant, n = 30). (d) Disease symptoms of cotton plants pre-treated with different concentrations of GN03 (0, 104, and 108 cfu/mL) following inoculation with V. dahliae strain V991 at 18 dpi. (e) Disease indexes of the GN03-pre-treated cotton were determined from 3 to 21 dpi. Values are means ± SD; n = 30.
Effect of GN03 on Cotton Seedling Growth and Disease Resistance
To explore the plant growth-promoting effect of GN03, each cotton seedling was inoculated with various concentrations of GN03, ranging from 0 cfu⋅mL–1 as the control (Mock), 104 cfu⋅mL–1 (C1), to 108 cfu⋅mL–1 (C2). The evaluated phenotypic characteristics of cotton significantly improved with the increase in inoculation concentration (C2 > C1 > Mock; Figure 1B). Compared to the Mock group, the C2 group exhibited significantly increased stem length, leaf area, root length, stem width, and leaf number by 85.91, 98.45, 38.42, 22.22, and 61.47%, respectively (Figure 1C). Overall, GN03 inoculation promoted cotton seedling growth in a dose-dependent manner.
After pouring different concentrations (0, 104, and 108 cfu⋅mL–1) of GN03 suspensions into the soil surrounding the roots of the cotton seedling, the seedlings were inoculated with V991 spore suspensions. Different responses to the V991 infection were observed in pre-treated and control seedlings, and the symptoms of wilt and etiolation were much more severe in the control than the pre-treated seedlings at 21 dpi (Mock + V991 > C1 + V991 > C2 + V991; Figure 1D). The disease index increased with prolonged dpi, but disease development was slower in the pre-treated seedlings. At 21 dpi, compared to the Mock + V991 group, the C1 + V991 and C2 + V991 groups exhibited significantly increased Verticillium wilt resistance, and the disease indexes were 90.6, 59.2, and 19.9% for the Mock + V991, C1 + V991, and C2 + V991 groups, respectively (Figure 1E). These results indicated that GN03 inoculation enhanced the disease resistance of cotton seedlings.
Changes in Phytohormones and the Expression of Disease Resistance-Related Genes After GN03 Inoculation
Bacillus association boosts plant growth and immunity to adversity by regulating growth and stress response genes, proteins, plant hormones, and related metabolites (Radhakrishnan et al., 2017; Afzal et al., 2019; Hashem et al., 2019). Accordingly, in cotton seedling roots, the expression of SA, JA, BRs, and GA synthesis-related genes (EDS1, AOC1, BES1, and GA20ox), auxin transporter gene (AUX1), and disease resistance-related genes (NPR1 and PR1) was significantly increased (Figures 2A–C). The changes in plant hormone levels in the seedlings were consistent with the expression of genes related to phytohormone synthesis. Compared to those in the Mock group, the level of IAA, GA, BR, JA, and SA were increased by 19.4, 31.1, 16.6, 28.6, and 44.9% in the C1 group, and that were significantly increased by 58.9, 32.4, 68.7, 38.7, and 31.3% in the C2 group, respectively (Figure 2D).
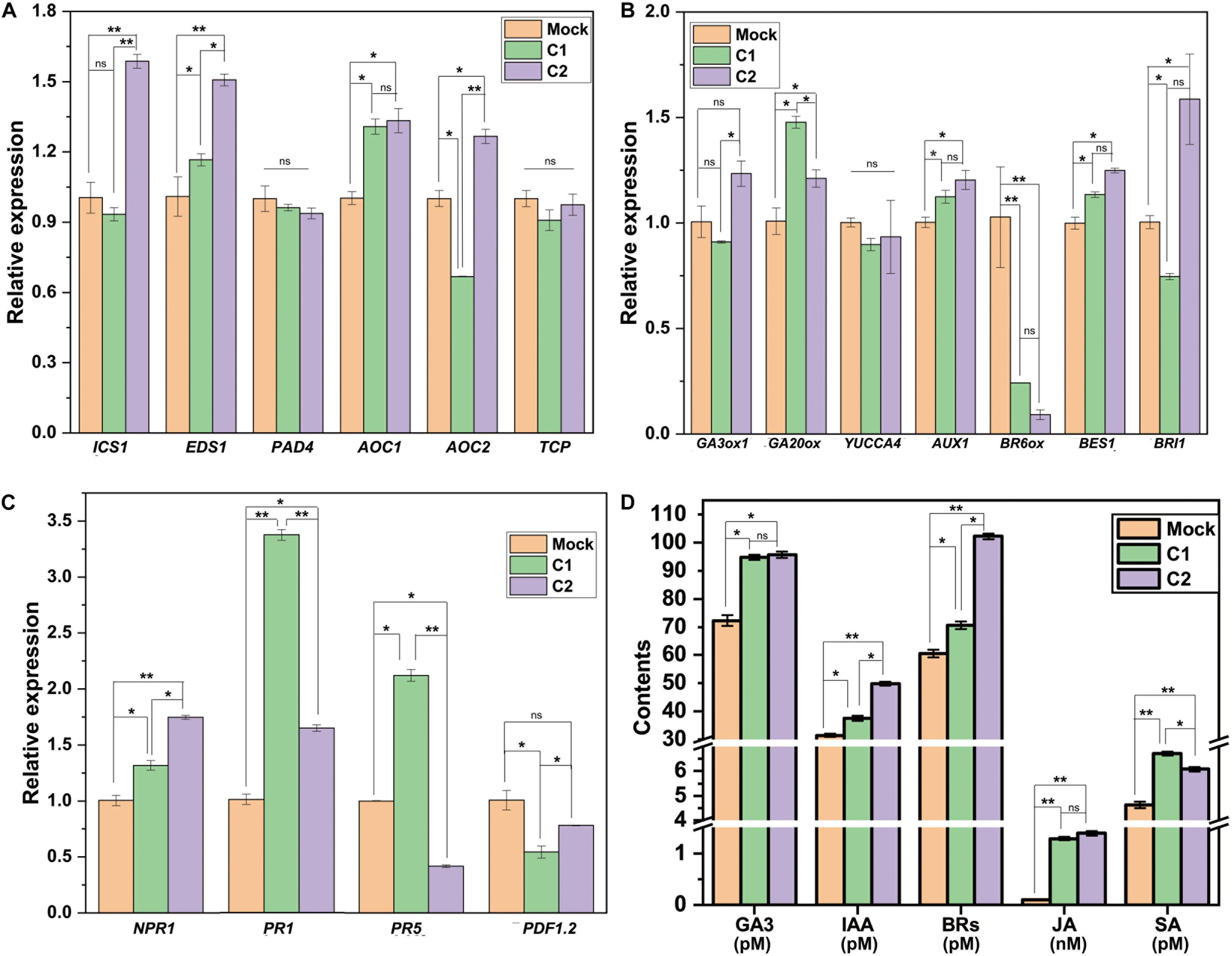
Figure 2. Changes in cotton hormones and related gene expression 30 days after GN03 inoculation. Relative expression of (A) SA and JA synthesis-related gene, (B) IAA, GA, BR synthesis-related genes, and (C) disease resistance-related genes, assessed with RT-qPCR. (D) Hormone content of cotton leaves. In the graphs, mean values with SD are indicated; statistical significance was evaluated by one-way ANOVA and LSD post hoc test (*p < 0.05, **p < 0.01; ns, not significant, n = 5).
Rhizosphere Microbiota Changes After GN03 Inoculation
Thirty days after inoculation, rhizosphere soil samples from each group were extracted to assess the microbial communities based on 16S rDNA amplicon sequencing. The richness and diversity of bacterial species were evaluated using the Chao1 and Shannon indices (Shannon, 1948; Chao, 1984). The results showed no significantly difference between the rhizosphere sample groups (Supplementary Table 5A). Nevertheless, the PCoA (Ramette, 2007) suggested that the microbiota could be divided into two distinct clusters based on the presence or absence of inoculation (Figure 3A). A network diagram (Faust and Raes, 2012) of species association showed very complex bacterial relationships in the rhizosphere, wherein the bacterial species promoted as well as inhibited each other (Supplementary Figure 3). This indicated that the rhizosphere microbiota was large in number and complex in composition, and that the microbial equilibrium in the rhizosphere would not be easily disrupted, thereby retaining a strong ability to recover.
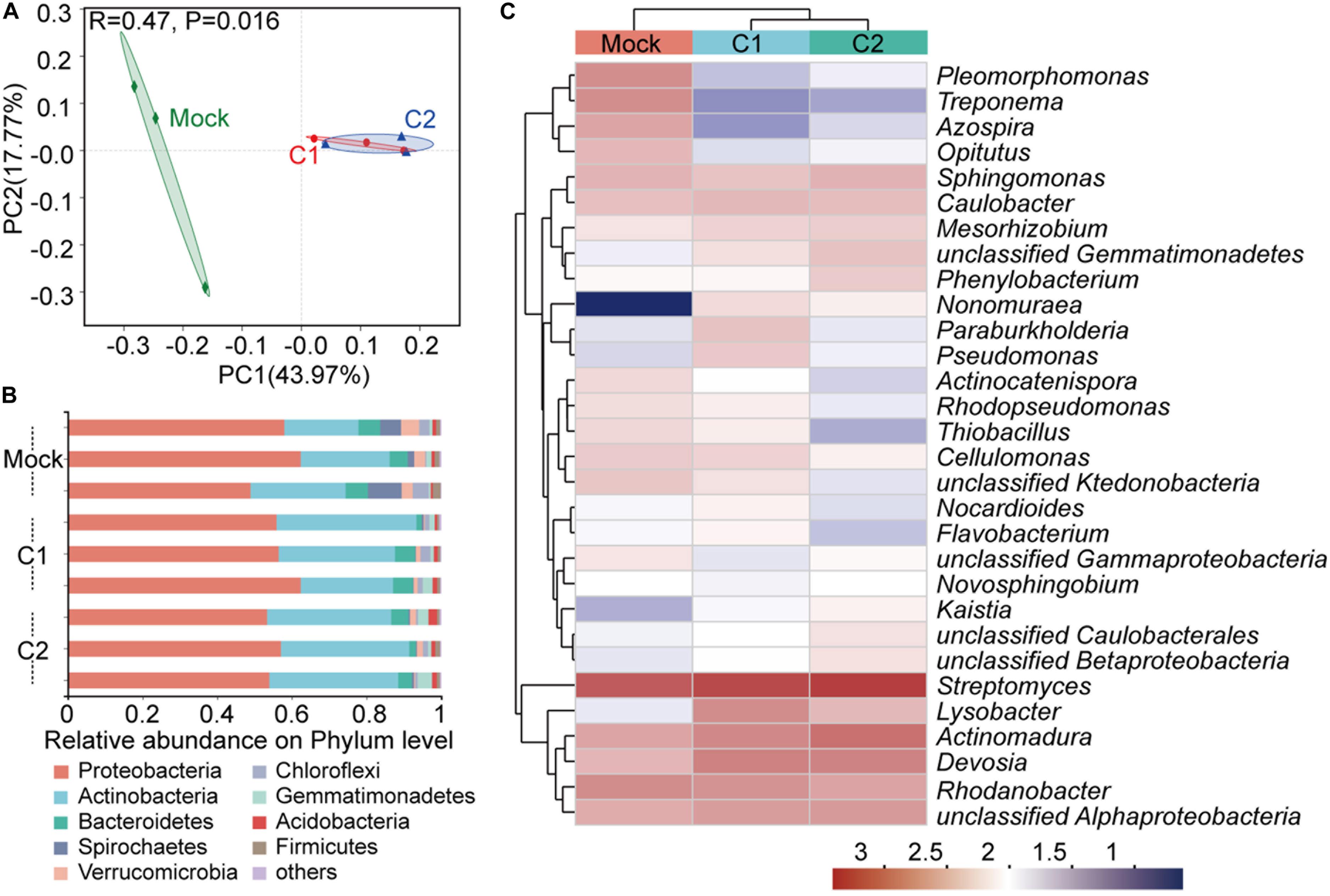
Figure 3. Bacterial community of the rhizosphere 30 days after GN03 inoculation. (A) PCoA plot based on the structure of the microbial community. Mock, green diamonds; C1, red circle; and C2, blue triangles. Variation in communities was based on Bray–Curtis distances and an ANOSIM test with 999 permutations. (B) Relative abundance of bacterial phyla in different samples. (C) Heatmap showing the relative abundance of the dominant taxa with respect to treatment. Hierarchical cluster analysis was based on Bray–Curtis distances using a complete-linkage method. Each sample was measured in triplicate.
With regards to rhizosphere bacterial composition at the phylum level (Figure 3B), the top three phyla were Proteobacteria, Actinomycetes, and Bacteroides. The relative proportion of Proteobacteria was approximately 50–60%. In the C2 group, the proportion of Spirochaetes was decreased by 5.05% but that of Actinobacteria was increased by 11.01%. At the genus level, the proportions of Lysobacter and Herbaspirillum, which have important biocontrol activities and nitrogen fixation abilities (Chubatsu et al., 2012; Exposito et al., 2015), were increased in the rhizosphere after inoculation, while those of Geobacter, Curvibacter, and Methylocysti, which have repair functions (Baani and Liesack, 2008; Gulay et al., 2018), were reduced (Figure 3C and Supplementary Figure 4A).
Endophytic Microbiota Changes After GN03 Inoculation
The cotton seedlings root samples after GN03 inoculation 30 days from each group were extracted to assess the microbial communities. Corresponding to the rhizosphere results, the microbial diversity in plant roots showed the following changes: (1) species richness and diversity increased after inoculation (Figure 4A and Supplementary Table 5B); (2) each treatment formed a separate cluster in the PCoA (Figure 4B); and (3) the structure of the network diagram was very simple, with only Dechloromonas being negatively correlated to the 25 other genera and all others positively correlated to the top 50 genera (Figure 4D). This suggested that the root microbiota was more sensitive to GN03 inoculation than the rhizosphere microbiota. Furthermore, functional prediction also confirmed this result. PICRUSt2 software (Douglas et al., 2019) analysis showed that the clusters of orthologous groups (COG) of protein function did not change significantly in the rhizosphere. However, in post-inoculation plant roots, amino acid transport and metabolism were the most abundant functions, followed by energy production. Furthermore, fatty acid metabolism, the pentose phosphate pathway, and biosynthesis of neomycin, kanamycin, and gentamicin increased following GN03 inoculation, while plant apoptosis and plant disease were inhibited. Further testing was performed to identify the bacteria responsible for this functional change.
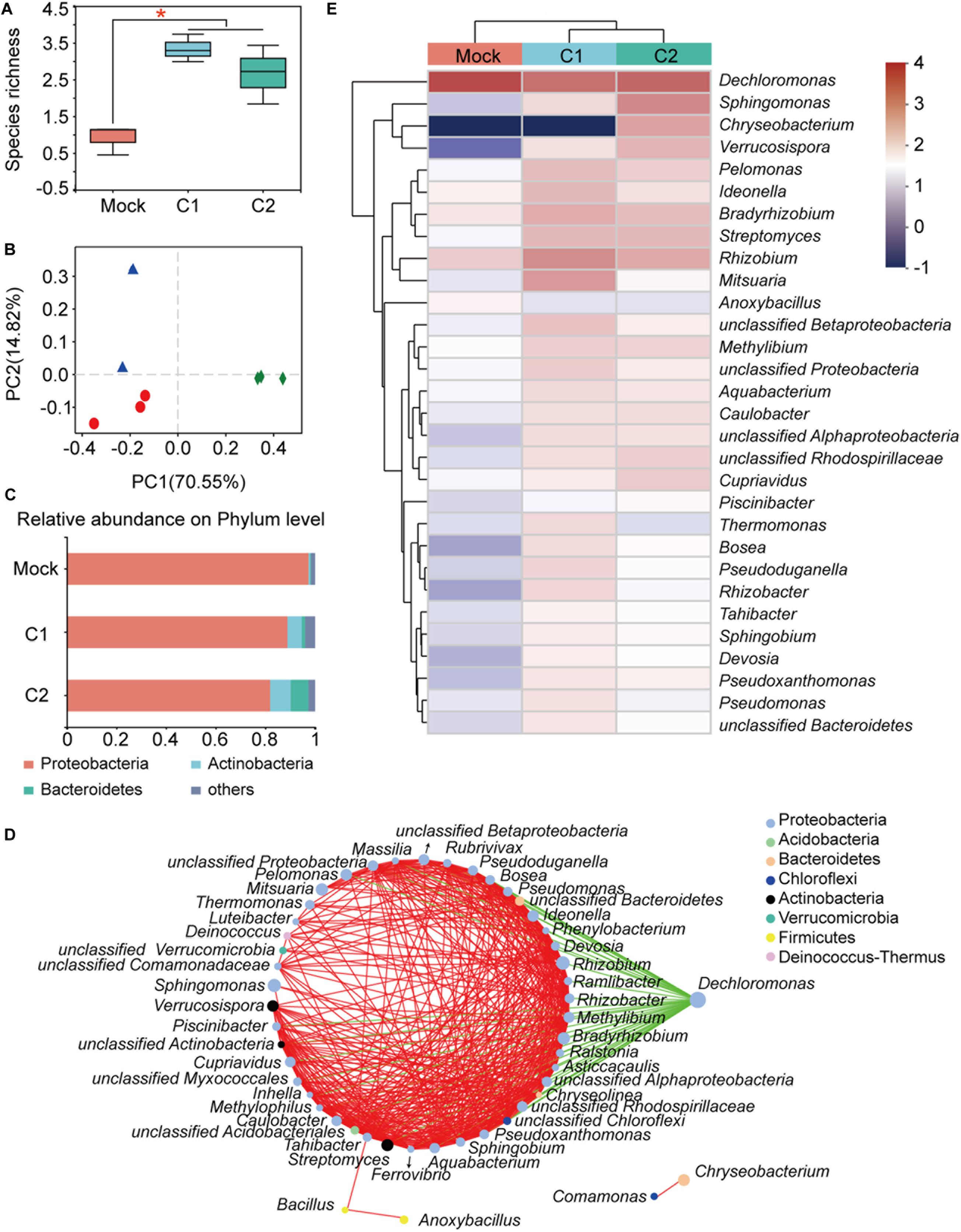
Figure 4. Bacterial community of cotton roots 30 days after GN03 inoculation. (A) Boxplot of species richness (number of OTUs) by Shannon index. The asterisk (*) indicates a significant difference (one-way ANOVA, LSD post hoc test, p < 0.05, see Supplementary Table 5B). (B) PCoA plot based on the structure of the microbial community. Each symbol represents a sample. Mock, green diamonds; C1, red circle; and C2, blue triangles. (C) Relative abundance of bacterial phyla in each group. Each value is the mean of three measurements. (D) Network correlation between plant root bacteria. The nodes on the left side of the ring are positively correlated at all instances (red lines) and the green lines indicate negative correlations between the genera. (E) Heatmap showing the relative abundance of the top 30 dominant taxa at the genus level in cotton roots.
The top bacterial species at the phylum level of roots, were similar to those in the rhizosphere (Figure 4C). However, their relative abundances varied greatly. For example, the relative proportion of Proteobacteria reached 97.46% within roots, whereas it was 52.63% in the rhizosphere. It should be noted that, as the inoculation concentration increased, the relative proportion of Proteobacteria gradually decreased to 81.94%. In the C2 group, the proportions of Actinobacteria and Bacteroidetes were increased by 7.65 and 6.87%, respectively. At genus level, the proportion of many genera increased after GN03 inoculation, especially in the C1 samples (Figure 4E and Supplementary Figure 4B), including Rhizobium, a bacterium that forms a symbiotic relationship with plant roots and is able to fix nitrogen (Zilli et al., 2020), Rubrivivax, a photosynthetic bacterium (Mekala et al., 2019), Actinomadura, a bacterium with potential use in new antibiotics (Lazzarini et al., 2000), and Labrys, a bacterium capable of chlorobenzene and fluoride biodegradation (Amorim et al., 2014). These results indicated that GN03 regulated the changes in the rhizosphere and endophytic microbiota. After inoculation, the microbial community had a huge change and showed some regularities.
Comparative Genomic Analysis of GN03
The above findings have so far demonstrated that GN03 inoculation can promote cotton seedlings growth, change the microbiota in and around the plant roots, increase phytohormones in cotton seedlings, up-regulate the expression of phytohormone synthesis and disease-resistance related genes. Then, we conducted a genome-wide sequencing analysis followed by a comparative genome analysis. The complete genome sequence of GN03 was deposited in the GenBank database (chromosome and plasmid accession numbers CP053315 and CP053316, respectively). An illustration of the genomic structure of the GN03 chromosome is shown in Figure 5A, and that of the plasmid is shown in Figure 5B. The total genome length was 5,217,129 bp. The mean average GC content was 35.64%, which was similar to the average GC content across B. circulans genomes (length, 5.09 Mbp; mean GC content, 35.5%) recorded by the NCBI. Of interest, GN03 contained a plasmid that was 0.18 Mbp long with a mean GC content of 31.62%. Multiple databases (Nr, COG, KEGG, Swiss-Prot, GO, and CAZy) were integrated to annotate gene function through sequence alignment.
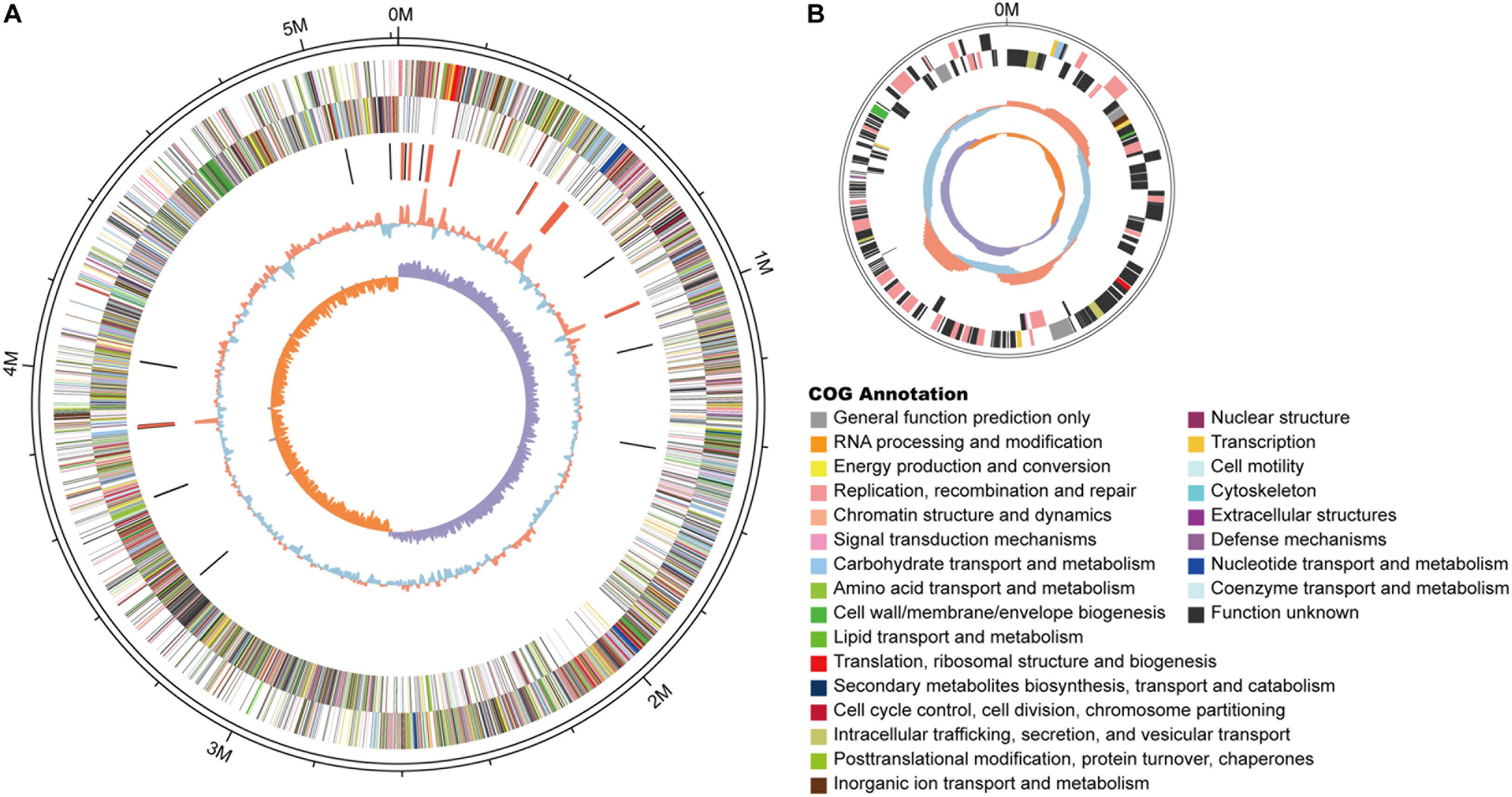
Figure 5. Schematic of the complete GN03 genome. GN03 chromosome (A) and plasmid (B). Rings represent the following features labeled from the outside to the center, where the outermost circle represents the scale in bp: the first ring represents positive strand genes and the second ring represents negative strand genes. Each color patch represents a COG functional classification. The third ring represents ncRNA (black indicates tRNA, red indicates rRNA). The fourth ring represents the GC content (red indicates GC content above the mean, blue indicates GC content below the mean). The innermost ring shows the GC skew (GC skew = [GC]/[G + C]; purple means greater than 0, orange means less than 0). Circos v0.62 (http://circos.ca/) software was used to draw the genomic circle map.
The GN03 genome included clusters of the following: de novo amino acid synthesis and metabolic pathways, de novo sugar synthesis and metabolism (such as starch, sucrose, and other polysaccharide metabolism), and de novo lipid synthesis (such as saturated fatty acids and unsaturated fatty acids synthesis) and metabolism. It also contained gene clusters for folic acid synthesis and for porphyrin, chlorophyll, butyric acid, biotin, inositol phosphate, sulfur, and methane metabolism. GN03 could effectively degrade naphthalene, xylene, ethylbenzene, nitrotoluene, chlorobenzene, and chlorocyclohexane. The genome also contained a comprehensive DNA repair system that could act as a DNA protectant. The functions of plasmid genes were mainly focused on DNA replication, mismatch repair, and phosphonate metabolism. In addition, we predicted the presence of genes that confer resistance by producing antibiotics (streptomycin, surfactin, penicillin, novobiocin, and cephalosporin) and other genes, such as gabD, opuC and opuA and proX, proV, and proW, against stress. These results indicated that GN03 was capable of pathogen resistance and pollutant degradation.
We selected four B. circulans reference strains with or without growth-promoting function (Supplementary Table 3) and performed a comparative genomic analysis. To identify homologous genes and specific gene families among the five bacterial species, homologous genes were identified using multiple databases (with an E-value < 1e-7) and the numbers of genes and gene families were analyzed using a Venn diagram (Figure 6A). Functional annotation data were obtained from multiple databases. The following top three KEGG pathways were shared among all strains: secondary metabolite biosynthesis, ribosome biosynthesis, and aminoacyl-tRNA biosynthesis. The collinear and the molecular evolutionary tree analysis of GN03 with four reference strains (Figures 6B,C) showed that the GN03 strain is most closely matched to the PK3-109 strain, which has also been reported to promote plant growth (Bokhari et al., 2019).
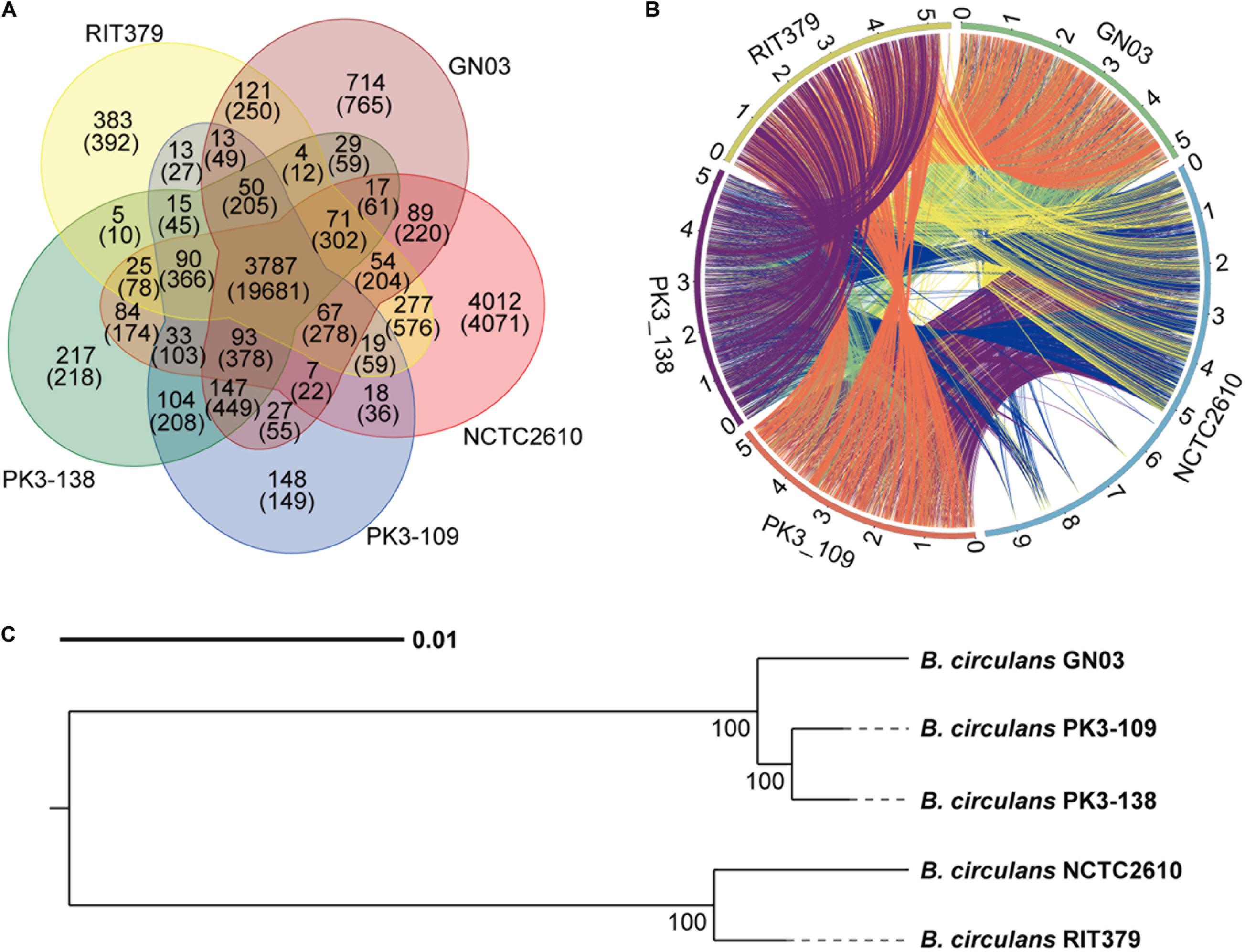
Figure 6. Comparative genomic analysis of GN03 with other four Bacillus stains. (A) Venn diagram showing the number of genes in all five strains. In BLAST alignment, gene pairs with E-values less than 1e-7 are considered to be homologous. OrthoMCL was used to group the homologous genes into the same families. The upper number represents the number of gene families and the lower number represents the number of genes. (B) The collinear circos diagram of the five bacterial strains. The outermost circle represents the genomic sequences of the five bacteria. For example, green in the upper right corner represents the genomic sequence of GN03 bacteria. Every two strains are connected by a line representing a collinear area between the two bacteria. (C) Phylogenetic tree of the five strains. The tree was built using a single copy homologous gene using the neighbor joining method with a bootstrap value of 1,000. Unit: nucleotide substitution rate.
Discussion
GN03 Inoculation Promoted Cotton Seedling Growth and Accumulation of Growth- Related Phytohormones
Plant roots can anchor and absorb nutrients and water in the soil. Lateral roots and root hairs are closely related to nutrient and water use efficiency. Many PGPB strains are known to promote the increase of lateral roots and the formation of root hairs (Zamioudis et al., 2013; Luo et al., 2019). GN03 is a soil-borne bacterium with positive effects on plant growth (Shen et al., 2018). In this study, The GN03 promoted the growth of cotton seedlings, which may be achieved by increasing the length and number of lateral roots (Figures 1B,C) resulting in cotton seedlings with a larger root surface in contact with the soil, thus boosting plants to absorb more nutrients and water. Accordingly, the GN03-treated cotton seedlings showed obvious increases stem length and width, leaf number and area during growth, especially in the C2 group (Figures 1B,C).
The improving effect of PGPB on plant growth is mainly reflected in the production of hormones or the induction of hormone signals (Egamberdieva et al., 2017). Phytohormones, such as IAA, GA, and BRs, can regulate various cellular processes and plant responses to biotic and abiotic stresses with a very low concentration (Ciura and Kruk, 2018). Auxins play an essential role in plant developmental process ranging from the cell division, and cell elongation to morphogenesis (Felten et al., 2009). Brassinosteroids are capable of executing diverse functions in plant growth, development, and stress tolerance (Banerjee and Roychoudhury, 2018). Gibberellins play an indispensable role in stem elongation, leaf expansion, and plant development, while GA deficit results in plant dwarfism (Ciura and Kruk, 2018). In our study, GN03 inoculation led to accumulation of growth-related hormones (IAA, GA, and BRs), as determined with ELISA, in the cotton leaves. Compared to those in the Mock group, the level of IAA, GA, and BR were increased by 19.4, 31.1, and 16.6% in the C1 group, and that were significantly increased by 58.9, 32.4, and 68.7% in the C2 group, respectively (Figure 2D). Accordingly, compared to those in the Mock group, the expression of IAA, BRs, and GA synthesis-related genes (AUX1, BES1, and GA20ox) was significantly increased in cotton seedling roots (Figure 2B). Phytohormones can be transported to different parts of the plant by different sophisticated transporter molecules through the vascular system in plant (Lacombe and Achard, 2016). These hormones may include endogenous hormones produced by the plant and the exogenous hormones produced by rhizosphere and endophytic microbes (Backer et al., 2018). Hence, this may also be the reason for the total amount of hormones accumulated in the leaves.
GN03 Inoculation Promoted Cotton Seedling Disease Resistance and the Expressing of Disease Resistance-Related Genes
The plant hormones JA and SA play key roles in the response of the plant to V. dahliae infection (Glazebrook, 2005; Zhang et al., 2017b). Consistently, we observed significant differences in JA and SA levels (Figure 2D) and changes in the expression of both JA and SA synthetic genes (EDS1 and AOC1, respectively) and disease resistance-related genes (NPR1 and PR1) after inoculation with the GN03 strain. Meanwhile, disease assessment after GN03 pre-treatment revealed significantly increased cotton disease resistance to V. dahliae (Figures 1D,E). We, therefore, presume that this resistance is mediated by GN03-regulating hormones signaling pathways. However, further molecular and genetic evidence is needed to elucidate the resistance mechanisms. Controlling Verticillium wilt is challenging, since the pathogen V. dahliae can survive for many years in the soil in dormant survival structure (microsclerotia) forms, which germinate to produce hyphae approximal to the root exudates of a suitable host (Inderbitzin et al., 2011; Inderbitzin and Subbarao, 2014; Luo et al., 2014; Daayf, 2015; Shaban et al., 2018). Some bacteria, collectively known as PGPB, can be effective when applied as fertilizers. This is of great significance for the development of more environment friendly agricultural practices. Moreover, biological control agents are eco-friendly strategies with great promise for controlling Verticillium wilt in cotton (Markakis et al., 2016; Zhang et al., 2021). B. circulans GN03 can promote upland cotton seedling growth and significantly reduce the incidence of Verticillium wilt. To our knowledge, this study is the first to investigate the growth promotion of B. circulans in cotton and application of B. circulans cells to reduce disease incidence.
Several beneficial bacteria and non-pathogenic rhizobacteria can also cause activation of the SA/JA pathway and lead to hormonal changes (Olanrewaju et al., 2017). After inoculation, the abundance of bacteria showed additional beneficial function in the roots, especially in the C1 group, though the C2 group showed a stronger growth promotion effect than C1. The expression levels of hormone-related genes (endogenous hormones) in plant roots support the theory that plants can reduce their growth to invest in disease resistance. This implies that the activation of plant growth and defense against potential pathogens are opposing processes in that the onset of one often leads to the inhibition of the other (Zhu et al., 2013; Huot et al., 2014).
To infect cotton roots favorably, V. dahliae can selectively steer the local microbiome by its effector proteins, and increasing reports have shown that the microbiome inside and outside a plant plays an crucial role in its health (Snelders et al., 2020). Bacillus species can produce some antibiotics, which can directly strangle the growth of the pathogen or induce systemic resistance in plants (Hasan et al., 2020). In our study, it was found that GN03 possess antibiotics (streptomycin, surfactin, penicillin, novobiocin, and cephalosporin) encoding genes and other genes, such as gabD, opuC and opuA and proX, proV, and proW, against stress. In addition, GN03 inoculation altered the microbiota in and around the plant roots and the expressing of disease resistance-related genes. Finally, the co-production of these antibiotics producing genes and the changes in cotton seedlings after GN03 inoculation may be form a amassing role against V. dahliae.
GN03 Inoculation Changed the Rhizosphere and Endophytic Bacterial Composition
Within the complex soil environment, many biotic and abiotic factors affect the colonization of PGPB. As colonization can be enhanced by multiple inoculations (Benizri et al., 2001; Coy et al., 2014), we tested different inoculation concentrations (C1 and C2), with greater inoculation concentration showing better beneficial effects. Furthermore, it was previously shown that rhizosphere microorganisms can recover after being temporarily affected by PGPB inoculation (Qiao et al., 2017). Our study confirmed that there were no significant differences in rhizosphere, while various plant indicators showed significant differences. We speculate that GN03 exerted a high ecological effect, which significantly influenced other bacterial changes within 30 days, such as increase in the levels of Lysobacter, Rubrivivax, and Rhizobium that are beneficial in promoting plant growth and enhancing disease resistance. Interestingly, obvious change of Bacillus or Firmicutes in the cotton seedling roots was found, while that was not found around the cotton seedling roots (Supplementary Figure 5). This may be due to (1) the time interval of detection is too long (30 days after inoculation); (2) microbial competition in a wide diversity of niches (Snelders et al., 2020). The dynamic changes of bacterial composition (especially beneficial bacteria including Bacillus) discovered in the 30-day period after inoculation need to be further investigated in future research.
Activation of defense against potential pathogens may inhibit growth activation, whereas the abundance of beneficial bacteria results in either the generation or stimulated-release of growth hormones (Backer et al., 2018). In general, plants in the C1 group did not show excessive growth promotion or inhibition which indicates that GN03 concentration is crucial to achieve optimal growth promotion effects.
Genome Analysis of GN03 Promoting Plant Growth and Disease Resistance
Our study demonstrated that GN03 promoted plant growth by regulating the rhizosphere and endophytic microbiota, together with the expression of plant hormones. To further understand the underlying mechanism, we conducted a genome-wide sequencing analysis, followed by a comparative genome analysis, to elucidate this growth promoting effect. Various genes of interest were found to be involved in the potential growth promoting and disease-resistance effects observed with GN03 inoculation. Inorganic phosphate uptake in some beneficial bacteria may be promoted by high-affinity phosphate transport systems regulated altogether by PstB, PstC, PstA, and PstS; PhnC, PhnE, and PhnD (Liu et al., 2016), and the genes cysC, cysI, cysJ, cysH, and cysN, responsible for H2S biosynthesis (Dooley et al., 2013). Of note, some of the trp cluster genes (trpA, trpB, trpD, and trpC) involved in tryptophan biosynthesis might be involved in multiple biological processes, including plant hormone biosynthesis (Gupta et al., 2014). The speB and speE genes encode agmatinase and spermidine synthase to catalyze the transformation of amino acids into plant growth-promoting compounds (Cassan et al., 2009). Furthermore, gabD is responsible for the production of pest/disease inhibiting γ-aminobutyric acid (Gupta et al., 2014). Autoinducer-2 (luxS) is a small molecule, produced by a number of bacterial species, that is implicated in the regulation of biofilm formation, motility, and the production of virulence factors. It has been reported to act directly through quorum sensing or indirectly through modulation of cellular metabolism (Reading and Sperandio, 2006). Lastly, the heat-shock protein genes dnaK and groEL could aid the survival of GN03 in harsh environments (Guazzaroni et al., 2013), whereas opuC and opuA and proX, proV, and proW might be involved in protecting plants against oxidative stress (Gupta et al., 2014).
In conclusion, the GN03 strain of B. circulans has been shown to improve plant growth and disease resistance, but the exact underlying mechanism is unclear. In fact, to our knowledge, very little work has been done on the synergistic relationship of plant root microbiota and B. circulans. This study showed that the application of B. circulans GN03 in cotton seedlings can regulate the microbiota in and around the plant roots, promote cotton seedling growth and disease resistance, induce the production of phytohormones such as IAA, GA, and SA, and up-regulate the expression of phytohormone synthesis-related genes (EDS1, AOC1, AUX1, BES1, and GA20ox) and disease-resistance genes (NPR1 and PR1).
Data Availability Statement
16S rDNA amplicon raw sequencing data were deposited in the NCBI Short Read Archive (SRA) BioProject PRJNA631145 under the accession numbers SRR11735611–SRR11735637. The B. circulans GN03 genome was deposited under accession numbers CP053315 and CP053316 (the latter is for the plasmid).
Author Contributions
LQ designed and performed the experiments. XY conducted the experiments. QC and WJ performed cotton growth measurements. SH, PT, and CX performed RNA extraction, RT-qPCR, and assisted in bioinformatics data analysis. LQ, PT, and QC analyzed the data, wrote the original draft of the manuscript, and performed the lab work. LQ, PT, and XY reviewed and edited the manuscript. XY obtained the funding. All authors read and approved the final manuscript.
Funding
This work was supported by the Basic Research and Frontier Exploration Foundation of Chongqing (cstc2017jcyjBX00078).
Conflict of Interest
The authors declare that the research was conducted in the absence of any commercial or financial relationships that could be construed as a potential conflict of interest.
Supplementary Material
The Supplementary Material for this article can be found online at: https://www.frontiersin.org/articles/10.3389/fpls.2021.644597/full#supplementary-material
Footnotes
- ^ http://www.majorbio.com
- ^ https://ftp.ncbi.nih.gov/blast/db/
- ^ http://uniprot.org
- ^ http://www.genome.jp/kegg/
- ^ http://www.ncbi.nlm.nih.gov/COG
- ^ http://lowelab.ucsc.edu/tRNAscan-SE
- ^ http://www.cbs.dtu.dk/services/RNAmmer
- ^ https://www.ncbi.nlm.nih.gov/biosample/?term=circulans%20NCTC2610
References
Afzal, I., Shinwari, Z. K., Sikandar, S., and Shahzad, S. (2019). Plant beneficial endophytic bacteria: mechanisms, diversity, host range and genetic determinants. Microbiol. Res. 221, 36–49. doi: 10.1016/j.micres.2019.02.001
Ahemad, M., and Kibret, M. (2014). Mechanisms and applications of plant growth promoting rhizobacteria: current perspective. J. King Saud Univ. Sci. 26, 1–20. doi: 10.1016/j.jksus.2013.05.001
Alka, S., Shahir, S., Ibrahim, N., Chai, T. T., Bahari, Z. M., and Abd Manan, F. (2020). The role of plant growth promoting bacteria on arsenic removal: a review of existing perspectives. Environ. Technol. Inno. 17:100602. doi: 10.1016/j.eti.2020.100602
Aloo, B. N., Makumba, B. A., and Mbega, E. R. (2019). The potential of Bacilli rhizobacteria for sustainable crop production and environmental sustainability. Microbiol. Res. 219, 26–39. doi: 10.1016/j.micres.2018.10.011
Amorim, C. L., Moreira, I. S., Maia, A. S., Tiritan, M. E., and Castro, P. M. L. (2014). Biodegradation of ofloxacin, norfloxacin, and ciprofloxacin as single and mixed substrates by Labrys portucalensis F11. Appl. Microbiol. Biotechnol. 98, 3181–3190. doi: 10.1007/s00253-013-5333-8
Araujo, R., Dunlap, C., Barnett, S., Barnett, S., and Franco, C. M. M. (2019). Decoding wheat endosphere–rhizosphere microbiomes in Rhizoctonia solani infested soils challenged by Streptomyces biocontrol agents. Front. Plant Sci. 10:1038. doi: 10.3389/fpls.2019.01038
Baani, M., and Liesack, W. (2008). Two isozymes of particulate methane monooxygenase with different methane oxidation kinetics are found in Methylocystis sp strain SCZ. Proc. Nat. Acad. Sci. U.S.A. 105, 10203–10208. doi: 10.1073/pnas.0702643105
Backer, R., Rokem, J. S., Ilangumaran, G., Lamont, J., Praslickova, D., Ricci, E., et al. (2018). Plant growth-promoting rhizobacteria: context, mechanisms of action, and roadmap to commercialization of biostimulants for sustainable agriculture. Front. Plant Sci. 9:1473. doi: 10.3389/fpls.2018.01473
Banerjee, A., and Roychoudhury, A. (2018). Interactions of brassinosteroids with major phytohormones: antagonistic effects. J. Plant Growth Regul. 37, 1025–1032. doi: 10.1007/s00344-018-9828-5
Benizri, E., Baudoin, E., and Guckert, A. (2001). Root colonization by inoculated plant growth-promoting rhizobacteria. Biocontrol. Sci. Technol. 11, 557–574. doi: 10.1080/09583150120076120
Bokhari, A., Essack, M., Lafi, F. F., Andres-Barrao, C., Jalal, R., Alamoudi, S., et al. (2019). Bioprospecting desert plant Bacillus endophytic strains for their potential to enhance plant stress tolerance. Sci. Rep. 9:18154. doi: 10.1038/s41598-019-54685-y
Buchanan, R., Gibbons, N., and Cowan, S. (1994). Bergey’s Manual of Determinative Bacteriology, 9th Edn. Philadelphia: Williams & Wilkins.
Cassan, F., Maiale, S., Masciarelli, O., Vidal, A., Luna, V., and Ruiz, O. (2009). Cadaverine production by Azospirillum brasilense and its possible role in plant growth promotion and osmotic stress mitigation. Eur. J. Soil Biol. 45, 12–19. doi: 10.1016/j.ejsobi.2008.08.003
Chao, A. (1984). Nonparametric-estimation of the number of classes in a population. Scand. J. Stat. 11, 265–270.
Cheng, F. F., Li, G., Peng, Y. J., Wang, A. Y., and Zhu, J. B. (2020). Mixed bacterial fermentation can control the growth and development of Verticillium dahliae. Biotechnol. Biotech. Eq. 34, 58–69. doi: 10.1080/13102818.2020.1713023
Chubatsu, L. S., Monteiro, R. A., de Souza, E. M., Schuler de Oliveira, M. A., Yates, M. G., Wassem, R., et al. (2012). Nitrogen fixation control in Herbaspirillum seropedicae. Plant Soil 356, 197–207. doi: 10.1007/s11104-011-0819-6
Ciura, J., and Kruk, J. (2018). Phytohormones as targets for improving plant productivity and stress tolerance. J. Plant Physiol. 229, 32–40. doi: 10.1016/j.jplph.2018.06.013
Coy, R. M., Held, D. W., and Kloepper, J. W. (2014). Rhizobacterial inoculants increase root and shoot growth in ‘Tifway’ hybrid bermudagrass. J. Environ. Horticult. 32, 149–154. doi: 10.24266/0738-2898.32.3.149
Daayf, F. (2015). Verticillium wilts in crop plants: pathogen invasion and host defence responses. Can. J. Plant Pathol. 37, 8–20. doi: 10.1080/07060661.2014.989908
Dahmani, M. A., Desrut, A., Moumen, B., Verdon, J., Mermouri, L., Kacem, M., et al. (2020). Unearthing the plant growth-promoting traits of Bacillus megaterium RmBm31, an endophytic bacterium isolated from root nodules of Retama monosperma. Front. Plant Sci. 11:124. doi: 10.3389/fpls.2020.00124
Delcher, A. L., Bratke, K. A., Powers, E. C., and Salzberg, S. L. (2007). Identifying bacterial genes and endosymbiont DNA with Glimmer. Bioinformatics 23, 673–679. doi: 10.1093/bioinformatics/btm009
Dooley, F. D., Nair, S. P., and Ward, P. D. (2013). Increased growth and germination success in plants following hydrogen sulfide administration. PLoS One 8:e0062048. doi: 10.1371/journal.pone.0062048
Douglas, G. M., Maffei, V. J., Zaneveld, J., Yurgel, S. N., Brown, J. R., Taylor, C. M., et al. (2019). PICRUSt2: an improved and extensible approach for metagenome inference. BioRxiv [Preprint] doi: 10.1101/672295
Egamberdieva, D., Wirth, S. J., Alqarawi, A. A., Abd Allah, E. F., and Hashem, A. (2017). Phytohormones and beneficial microbes: essential components for plants to balance stress and fitness. Front. Microbiol. 8:2104. doi: 10.3389/fmicb.2017.02104
Emmanuel, O. C., and Babalola, O. O. (2020). Productivity and quality of horticultural crops through co-inoculation of arbuscular mycorrhizal fungi and plant growth promoting bacteria. Microbiol. Res. 239:126569. doi: 10.1016/j.micres.2020.126569
Erdogan, O., and Benlioglu, K. (2010). Biological control of Verticillium wilt on cotton by the use of fluorescent Pseudomonas spp. under field conditions. Biol. Control 53, 39–45. doi: 10.1016/j.biocontrol.2009.11.011
Exposito, R. G., Postma, J., Raaijmakers, J. M., and De Bruijn, I. (2015). Diversity and activity of Lysobacter species from disease suppressive soils. Front. Microbiol. 6:1255. doi: 10.3389/fmicb.2015.01243
Faust, K., and Raes, J. (2012). Microbial interactions: from networks to models. Nat. Rev. Microbiol. 10, 538–550. doi: 10.1038/nrmicro2832
Felten, J., Kohler, A., Morin, E., Bhalerao, R. P., Palme, K., Martin, F., et al. (2009). The ectomycorrhizal fungus Laccaria bicolor stimulates lateral root formation in poplar and Arabidopsis through auxin transport and signaling. Plant Physiol. 151, 1991–2005. doi: 10.1104/pp.109.147231
Gadhave, K. R., Devlin, P. F., Ebertz, A., Ross, A., and Gange, A. C. (2018). Soil inoculation with Bacillus spp. Modifies root endophytic bacterial diversity, evenness, and community composition in a context-specific manner. Microb. Ecol. 76, 741–750. doi: 10.1007/s00248-018-1160-x
Glazebrook, J. (2005). Contrasting mechanisms of defense against biotrophic and necrotrophic pathogens. Annu. Rev. Phytopathol. 43, 205–227. doi: 10.1146/annurev.phyto.43.040204.135923
Goicoechea, N. (2009). To what extent are soil amendments useful to control Verticillium wilt? Pest Manage. Sci. 65, 831–839. doi: 10.1002/ps.1774
Golding, C. G., Lamboo, L. L., Beniac, D. R., and Booth, T. F. (2016). The scanning electron microscope in microbiology and diagnosis of infectious disease. Sci. Rep. 6:26516. doi: 10.1038/srep26516
Guazzaroni, M.-E., Morgante, V., Mirete, S., and Gonzalez-Pastor, J. E. (2013). Novel acid resistance genes from the metagenome of the Tinto River, an extremely acidic environment. Environ. Microbiol. 15, 1088–1102. doi: 10.1111/1462-2920.12021
Gulay, A., Cekic, Y., Musovic, S., Albrechtsen, H.-J., and Smets, B. F. (2018). Diversity of iron oxidizers in groundwater-fed rapid sand filters: evidence of Fe(II)-dependent growth by Curvibacter and Undibacterium spp. Front. Microbiol. 9:2808. doi: 10.3389/fmicb.2018.02808
Gupta, A., Gopal, M., Thomas, G. V., Manikandan, V., Gajewski, J., Thomas, G., et al. (2014). Whole genome sequencing and analysis of plant growth promoting bacteria isolated from the rhizosphere of plantation crops coconut, cocoa and arecanut. PLoS One 9:e0104259. doi: 10.1371/journal.pone.0104259
Hasan, N., Farzand, A., Heng, Z., Khan, I. U., Moosa, A., Zubair, M., et al. (2020). Antagonistic potential of novel endophytic Bacillus strains and mediation of plant defense against Verticillium wilt in upland cotton. Plants (Basel, Switzerland) 9:1438. doi: 10.3390/plants9111438
Hashem, A., Tabassum, B., and Abd Allah, E. F. (2019). Bacillus subtilis: a plant-growth promoting rhizobacterium that also impacts biotic stress. Saudi J. Biol. Sci. 26, 1291–1297. doi: 10.1016/j.sjbs.2019.05.004
Hassan, M. K., McInroy, J. A., and Kloepper, J. W. (2019). The interactions of rhizodeposits with plant growth-promoting rhizobacteria in the rhizosphere: a review. Agriculture 9:142. doi: 10.3390/agriculture9070142
Huot, B., Yao, J., Montgomery, B. L., and He, S. Y. (2014). Growth-defense tradeoffs in plants: a balancing act to optimize fitness. Mol. Plant 7, 1267–1287. doi: 10.1093/mp/ssu049
Inderbitzin, P., Bostock, R. M., Davis, R. M., Usami, T., Platt, H. W., and Subbarao, K. V. (2011). Phylogenetics and taxonomy of the fungal vascular wilt pathogen Verticillium, with the descriptions of five new species. PLoS One 6:e28341. doi: 10.1371/journal.pone.0028341
Inderbitzin, P., and Subbarao, K. V. (2014). Verticillium systematics and evolution: how confusion impedes Verticillium wilt management and how to resolve it. Phytopathology 104, 564–574. doi: 10.1094/phyto-11-13-0315-ia
Kemp, P. F., and Aller, J. Y. (2004). Bacterial diversity in aquatic and other environments: what 16S rDNA libraries can tell us. FEMS Microbiol. Ecol. 47, 161–177. doi: 10.1016/s0168-6496(03)00257-5
Khatri, S., Sharma, R. K., and Shridhar, V. (2020). Influence of cadmium-tolerant and plant growth-promoting rhizobacteria on cadmium accumulation and growth response of wheat seedlings under mountain ecosystem. Agric. Res. 9, 56–65. doi: 10.1007/s40003-019-00407-9
Lacombe, B., and Achard, P. (2016). Long-distance transport of phytohormones through the plant vascular system. Curr. Opin. Plant Biol. 34, 1–8. doi: 10.1016/j.pbi.2016.06.007
Lazzarini, A., Cavaletti, L., Toppo, G., and Marinelli, F. (2000). Rare genera of actinomycetes as potential producers of new antibiotics. Int. J. Gen. Mol. Microbiol. 78, 399–405. doi: 10.1023/a:1010287600557
Lee, S., Trịnh, C. S., Lee, W. J., Jeong, C. Y., Truong, H. A., Chung, N., et al. (2020). Bacillus subtilis strain L1 promotes nitrate reductase activity in Arabidopsis and elicits enhanced growth performance in Arabidopsis, lettuce, and wheat. J. Plant Res. 133, 231–244. doi: 10.1007/s10265-019-01160-4
Liu, W., Wang, Q., Hou, J., Tu, C., Luo, Y., and Christie, P. (2016). Whole genome analysis of halotolerant and alkalotolerant plant growth-promoting rhizobacterium Klebsiella sp D5A. Sci. Rep. 6:26710. doi: 10.1038/srep26710
Lugtenberg, B., and Kamilova, F. (2009). Plant-growth-promoting rhizobacteria. Annu. Rev. Microbiol. 63, 541–556. doi: 10.1146/annurev.micro.62.081307.162918
Luo, X., Tian, T., Tan, X., Zheng, Y., Xie, C., Xu, Y., et al. (2020). VdNPS, a nonribosomal peptide synthetase, is involved in regulating virulence in Verticillium dahliae. Phytopathology 110, 1398–1409. doi: 10.1094/phyto-02-20-0031-r
Luo, X., Xie, C., Dong, J., Yang, X., and Sui, A. (2014). Interactions between Verticillium dahliae and its host: vegetative growth, pathogenicity, plant immunity. Appl. Microbiol. Biotechnol. 98, 6921–6932. doi: 10.1007/s00253-014-5863-8
Luo, Y., Wang, F., Huang, Y., Zhou, M., Gao, J., Yan, T., et al. (2019). Sphingomonas sp. Cra20 increases plant growth rate and alters rhizosphere microbial community structure of Arabidopsis thaliana under drought stress. Front. Microbiol. 10:1221. doi: 10.3389/fmicb.2019.01221
Mahmood, A., and Kataoka, R. (2020). Metabolite profiling reveals a complex response of plants to application of plant growth-promoting endophytic bacteria. Microbiol. Res. 234:126421. doi: 10.1016/j.micres.2020.126421
Markakis, E. A., Tjamos, S. E., Antoniou, P. P., Paplomatas, E. J., and Tjamos, E. C. (2016). Biological control of Verticillium wilt of olive by Paenibacillus alvei, strain K165. BioControl 61, 293–303. doi: 10.1007/s10526-015-9669-0
Mehnaz, S., Baig, D. N., and Lazarovits, G. (2010). Genetic and phenotypic diversity of plant growth promoting rhizobacteria isolated from sugarcane plants growing in Pakistan. J. Microbiol. Biotechnol. 20, 1614–1623. doi: 10.4014/jmb.1005.05014
Mehta, P., Chauhan, A., Mahajan, R., Mahajan, P. K., and Shirkot, C. K. (2010). Strain of Bacillus circulans isolated from apple rhizosphere showing plant growth promoting potential. Curr. Sci. 98, 538–542.
Mehta, P., Walia, A., Kulshrestha, S., Chauhan, A., and Shirkot, C. K. (2015). Efficiency of plant growth-promoting P-solubilizing Bacillus circulans CB7 for enhancement of tomato growth under net house conditions. J. Basic Microbiol. 55, 33–44. doi: 10.1002/jobm.201300562
Mekala, L. P., Mohammed, M., Chinthalapati, S., and Chinthalapati, V. R. (2019). Pyomelanin production: insights into the incomplete aerobic L-phenylalanine catabolism of a photosynthetic bacterium, Rubrivivax benzoatilyticus JA2. Int. J. Biol. Macromol. 126, 755–764. doi: 10.1016/j.ijbiomac.2018.12.142
Nakamura, L. K., and Swezey, J. (1983). Taxonomy of Bacillus-circulans Jordan 1890 - base composition and reassociation of deoxyribonucleic-acid. Int. J. Syst. Bacteriol. 33, 46–52. doi: 10.1099/00207713-33-1-46
Olanrewaju, O. S., Glick, B. R., and Babalola, O. O. (2017). Mechanisms of action of plant growth promoting bacteria. World J. Microbiol. Biotechnol. 33:197. doi: 10.1007/s11274-017-2364-9
Parnell, J. J., Berka, R., Young, H. A., Sturino, J. M., Kang, Y. W., Barnhart, D. M., et al. (2016). From the Lab to the farm: an industrial perspective of plant beneficial microorganisms. Front. Plant Sci. 7:1110. doi: 10.3389/fpls.2016.01110
Polter, S. J., Caraballo, A. A., Lee, Y. P., Eng, W. W. H., Gan, H. M., Wheatley, M. S., et al. (2015). Isolation, identification, whole-genome sequencing, and annotation of four Bacillus species, B.anthracis RIT375, B.circulans RIT379, B. altitudinis RIT380, and B. megaterium RIT381, from internal stem tissue of the insulin plant Costus igneus. Genome Announ 3, e00847–15. doi: 10.1128/genomeA.00847-15
Qiao, J., Yu, X., Liang, X., Liu, Y., Borriss, R., and Liu, Y. (2017). Addition of plant-growth-promoting Bacillus subtilis PTS-394 on tomato rhizosphere has no durable impact on composition of root microbiome. BMC Microbiol. 17:131. doi: 10.1186/s12866-017-1039-x
Radhakrishnan, R., Hashem, A., and Abd Allah, E. F. (2017). Bacillus: a biological tool for crop improvement through bio-molecular changes in adverse environments. Front. Physiol. 8:667. doi: 10.3389/fphys.2017.00667
Ramakrishna, W., Yadav, R., and Li, K. (2019). Plant growth promoting bacteria in agriculture: two sides of a coin. Appl. Soil Ecol. 138, 10–18. doi: 10.1016/j.apsoil.2019.02.019
Ramette, A. (2007). Multivariate analyses in microbial ecology. FEMS Microbiol. Ecol. 62, 142–160. doi: 10.1111/j.1574-6941.2007.00375.x
Reading, N. C., and Sperandio, V. (2006). Quorum sensing: the many languages of bacteria. FEMS Microbiol. Lett. 254, 1–11. doi: 10.1111/j.1574-6968.2005.00001.x
Shaban, M., Miao, Y., Ullah, A., Khan, A. Q., Menghwar, H., Khan, A. H., et al. (2018). Physiological and molecular mechanism of defense in cotton against Verticillium dahliae. Plant Physiol. Biochem. 125, 193–204. doi: 10.1016/j.plaphy.2018.02.011
Shannon, C. E. (1948). A mathematical theory of communication. Bell Sys. Tech. J. 27, 623–656. doi: 10.1002/j.1538-7305.1948.tb00917.x
Shen, H., Wu, B., Li, H. H., Liu, Y. Q., Wu, X. W., and Yang, X. Y. (2018). Screening of plant growth-promoting bacteria from purple soil and growth promoting effects of its complex microbial inoculant on ginger germchit (in Chinese). China Veget 6, 54–59.
Sherzad, Z., and Canming, T. (2020). A new strain of Bacillus velezens is as a bioagent against Verticillium dahliae in cotton: isolation and molecular identification. Egypt. J. Biol. Pest Control 30:118. doi: 10.1186/s41938-020-00308-y
Snelders, N. C., Rovenich, H., Petti, G. C., Rocafort, M., van den Berg, G. C. M., Vorholt, J. A., et al. (2020). Microbiome manipulation by a soil-borne fungal plant pathogen using effector proteins. Nat. Plants 6:1365. doi: 10.1038/s41477-020-00799-5
Tanaka, H., and Watanabe, T. (1995). Glucanases and chitinases of Bacillus circulans WL-12. J. Ind. Microbiol. 14, 478–483. doi: 10.1007/BF01573962
Tao, X. Y., Zhang, H. L., Gao, M. T., Li, M. L., Zhao, T., and Guan, X. Y. (2020). Pseudomonas species isolated via high-throughput screening significantly protect cotton plants against Verticillium wilt. AMB Express 10:193. doi: 10.1186/s13568-020-01132-1
Wang, J., Li, R., Zhang, H., Wei, G., and Li, Z. (2020). Beneficial bacteria activate nutrients and promote wheat growth under conditions of reduced fertilizer application. BMC Microbiol. 20:38. doi: 10.1186/s12866-020-1708-z
Wang, Y., Liang, C., Wu, S., Zhang, X., Tang, J., Jian, G., et al. (2016). Significant improvement of cotton Verticillium wilt resistance by manipulating the expression of Gastrodia antifungal proteins. Mol. Plant 9, 1436–1439. doi: 10.1016/j.molp.2016.06.013
Xu, N., Tan, G., Wang, H., and Gai, X. (2016). Effect of biochar additions to soil on nitrogen leaching, microbial biomass and bacterial community structure. Eur. J. Soil Biol. 74, 1–8. doi: 10.1016/j.ejsobi.2016.02.004
Zamioudis, C., Mastranesti, P., Dhonukshe, P., Blilou, I., and Pieterse, C. M. J. (2013). Unraveling root developmental programs initiated by beneficial Pseudomonas spp. bacteria. Plant Physiol. 162, 304–318. doi: 10.1104/pp.112.212597
Zhang, F., Li, X. L., Zhu, S. J., Ojaghian, M. R., and Zhang, J. Z. (2018). Biocontrol potential of Paenibacillus polymyxa against Verticillium dahliae infecting cotton plants. Biol. Control 127, 70–77. doi: 10.1016/j.biocontrol.2018.08.021
Zhang, J., Yu, X., Zhang, C., Zhang, Q., Sun, Y., Zhu, H., et al. (2021). Pectin lyase enhances cotton resistance to Verticillium wilt by inducing cell apoptosis of Verticillium dahliae. J. Hazard. Mater. 404:124029. doi: 10.1016/j.jhazmat.2020.124029
Zhang, L., Ni, H., Du, X., Wang, S., Ma, X.-W., Nürnberger, T., et al. (2017a). The Verticillium-specific protein VdSCP7 localizes to the plant nucleus and modulates immunity to fungal infections. New Phytol. 215, 368–381. doi: 10.1111/nph.14537
Zhang, L., Tao, Y., Zhao, S., Yin, X., Chen, J., Wang, M., et al. (2020). A novel peroxiredoxin from the antagonistic endophytic bacterium Enterobacter sp. V1 contributes to cotton resistance against Verticillium dahliae. Plant Soil 454, 395–409. doi: 10.1007/s11104-020-04661-7
Zhang, W., Corwin, J. A., Copeland, D., Feusier, J., Eshbaugh, R., Chen, F., et al. (2017b). Plastic transcriptomes stabilize immunity to pathogen diversity: the jasmonic acid and salicylic acid networks within the Arabidopsis/Botrytis pathosystem. Plant Cell 29, 2727–2752. doi: 10.1105/tpc.17.00348
Zhang, Y., Wang, X. F., Ding, Z. G., Ma, Q., Zhang, G. R., Zhang, S. L., et al. (2013). Transcriptome profiling of Gossypium barbadense inoculated with Verticillium dahliae provides a resource for cotton improvement. BMC Genomics 14:637. doi: 10.1186/1471-2164-14-637
Zhou, L., Zhao, J., Guo, W., and Zhang, T. (2013). Functional analysis of autophagy genes via Agrobacterium-mediated transformation in the vascular wilt fungus Verticillium dahliae. J. Genet. Genom. 40, 421–431. doi: 10.1016/j.jgg.2013.04.006
Zhu, Y., Du, B., Qian, J., Zou, B., and Hua, J. (2013). Disease resistance gene-induced growth inhibition is enhanced by rcd1 independent of defense activation in Arabidopsis. Plant Physiol. 161, 2005–2013. doi: 10.1104/pp.112.213363
Keywords: Bacillus circulans, upland cotton (Gossypium hirsutum L.), disease resistance, growth-promoting, phytohormones synthesis, rhizosphere and endophytic microbiota
Citation: Qin L, Tian P, Cui Q, Hu S, Jian W, Xie C, Yang X and Shen H (2021) Bacillus circulans GN03 Alters the Microbiota, Promotes Cotton Seedling Growth and Disease Resistance, and Increases the Expression of Phytohormone Synthesis and Disease Resistance-Related Genes. Front. Plant Sci. 12:644597. doi: 10.3389/fpls.2021.644597
Received: 21 December 2020; Accepted: 23 March 2021;
Published: 14 April 2021.
Edited by:
Jianfei Wang, Anhui University of Science and Technology, ChinaReviewed by:
Christos Zamioudis, Democritus University of Thrace, GreeceLi Xue, Zhejiang Normal University, China
Xingang Zhou, Northeast Agricultural University, China
Copyright © 2021 Qin, Tian, Cui, Hu, Jian, Xie, Yang and Shen. This is an open-access article distributed under the terms of the Creative Commons Attribution License (CC BY). The use, distribution or reproduction in other forums is permitted, provided the original author(s) and the copyright owner(s) are credited and that the original publication in this journal is cited, in accordance with accepted academic practice. No use, distribution or reproduction is permitted which does not comply with these terms.
*Correspondence: Xingyong Yang, eWFuZ3h5OTRAc3d1LmVkdS5jbg==; Hong Shen, c2hlbmhvbmdAc3d1LmVkdS5jbg==
†These authors have contributed equally to this work