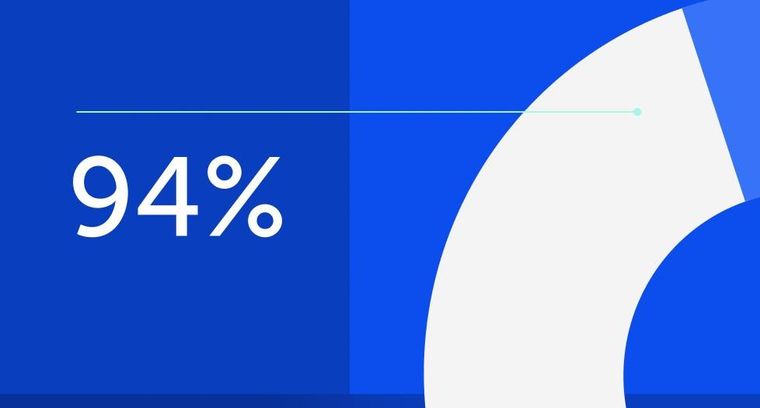
94% of researchers rate our articles as excellent or good
Learn more about the work of our research integrity team to safeguard the quality of each article we publish.
Find out more
REVIEW article
Front. Plant Sci., 29 April 2021
Sec. Crop and Product Physiology
Volume 12 - 2021 | https://doi.org/10.3389/fpls.2021.644528
This article is part of the Research TopicResilience of Grapevine to Climate Change: From Plant Physiology to Adaptation StrategiesView all 24 articles
Climate change has become a topic of increasing significance in viticulture, severely challenged by this issue. Average global temperatures are increasing, but frost events, with a large variability depending on geographical locations, have been predicted to be a potential risk for grapevine cultivation. Grape cold hardiness encompasses both midwinter and spring frost hardiness, whereas the avoidance of spring frost damage due to late budbreak is crucial in cold resilience. Cold hardiness kinetics and budbreak phenology are closely related and affected by bud’s dormancy state. On the other hand, budbreak progress is also affected by temperatures during both winter and spring. Genetic control of bud phenology in grapevine is still largely undiscovered, but several studies have recently aimed at identifying the molecular drivers of cold hardiness loss and the mechanisms that control deacclimation and budbreak. A review of these related traits and their variability in different genotypes is proposed, possibly contributing to develop the sustainability of grapevine production as climate-related challenges rise.
Climate change is a proven reality whose consequences on human activities and natural systems have reached an undeniable magnitude all around the world (IPCC, 2014). Global mean surface temperatures are predicted to increase by 0.3–4.8°C by the end of the 21st century, depending on the trend of anthropogenic greenhouse gas emissions, compared to the reference time-frame 1986–2005 (IPCC, 2014). Many plant species are expected to be unable to shift their geographical range quickly enough to keep up with these changes, and production will be negatively impacted if no adaptation occurs. Rainfall changes are likely to differ depending on the region, whereas radiation and extreme weather events are expected to increase (IPCC, 2019). Agriculture and viticulture, in particular, greatly depend on thermal regimen, soil composition, and water availability, in terms of fruit yield and metabolite composition (van Leeuwen and Darriet, 2016). Grapevine holds great economic value as it can be used fresh (table grape) or dry (raisin) and for winemaking (Delrot et al., 2020). Climate variations in wine-producing regions induce the so-called “vintage effect,” the year-to-year variations in yield, quality, and typicity (van Leeuwen and Darriet, 2016). Grape berry composition also depends on “terroir,” defined as the complete natural environment in which a wine is produced, in which climate plays a major role, with the interplay of human activity (Delrot et al., 2020; Santos et al., 2020). Grapevine phenology and fruit ripening are greatly affected by temperature conditions. Berry composition is key in determining the subsequent quality of wines. The increase in temperature has been shown to cause a rise of berry sugar concentration (Coombe, 1987), whereas some secondary metabolites, such as malic acid or anthocyanins (Kliewer and Torres, 1972), are negatively affected. Higher temperatures produce an advance of phenology, causing earlier harvest dates (van Leeuwen and Darriet, 2016) and decoupling sugar and phenolic compound accumulation at maturity, thus leading to unbalanced wines (Sadras and Moran, 2012; Bonada et al., 2015). High temperatures during the final stages of berry growth, together with high precipitations, can also be the cause of cracks and rots (Molitor et al., 2016). Although rainfall tendencies are difficult to predict, the increase in evapotranspiration caused by temperature increase will cause plants to experience water stress even when rainfall does not directly decrease (van Leeuwen and Darriet, 2016).
The new climate change scenario will lead to increasing difficulty in the production of traditional wines in their areas of origin if no adaptation occurs. Therefore, adaptation measures are necessary as wine quality greatly depends on ripening conditions (Bonada et al., 2015), which in turn are a direct consequence of the timing of several phenological phases starting with budbreak.
Although the impacts of climate change are expected to be diverse in different wine-making regions (Santillán et al., 2019) and among cultivars with different phenological rhythms (McIntyre et al., 1982), several adaptation practices may be able to cope with the short-term effects of climate change and maintain wine typicity, and new training systems could be developed for the middle term (Duchêne, 2016). Remarkably, several variations in training systems and cultural practices have been adopted and tested in recent times with the aim to lower the risk of freezing damage in spring. Trimming, hedging, or pruning has been evaluated in order to mitigate the short-term impacts of climate change (Herrera et al., 2015; Frioni et al., 2016; Palliotti et al., 2017; Abad et al., 2019). In the past, late winter pruning was shown to be effective in delaying bud burst in cool climate areas (Trought et al., 1999), although it could not be applied for grapevine grown in different environments, in which both yield increase (Friend and Trought, 2007) and loss (Frioni et al., 2016) were observed. Recently, a double-pruning approach has shown a potential budburst delay of up to 4 weeks, depending on the timing of the second pruning (Palliotti et al., 2017). As regards the direct avoidance of spring frost damage, several methods, encompassing active and passive types, have been used in the past (Liu and Sherif, 2019). Active approaches include the use of wind machines and helicopters to force the warmer air toward the ground, or heaters and irrigation, to exploit the fusion heat of water. Efficacy of such methods depends greatly on external factors and cannot guarantee a complete avoidance of damage. Moreover, these approaches are costly and environmentally unsustainable and require coordinated action by growers to avoid the rise of production costs and to ensure the effectiveness in the short term (Unterberger et al., 2018). Additionally, the application of chemicals (e.g., Amigo oil, FrostShield, and ProTone) and plant growth regulators (i.e., ethephon) has been shown to delay budbreak, although these results remain inconsistent (Qrunfleh and Read, 2013; Centinari et al., 2018; Kovaleski and Londo, 2019; Liu and Sherif, 2019; Wang and Dami, 2020).
In this context, the genetic improvement of grapevine has been taken into consideration to cope with the effects of climate change in the long run. Cultivated grapevines all around the world are usually grafted, and this adds a layer of complication to the understanding of plant–environment interactions. Moreover, the communication between scion and rootstock is often unclear or unexplored as the connection that is immediately established at grafting may evolve as the plant ages (Delrot et al., 2020). Therefore, despite the numerous aspects to consider, the investigation of unexploited varieties in germplasm collections, for both rootstock and scion, could be an interesting opportunity, strengthened by the continuous evolution of sequencing technologies and gene-mapping approaches. Efficient phenotyping methods also need to be developed to assess the effectiveness of varietal selection and the plasticity of the phenotype in different scion–rootstocks combinations (Warschefsky et al., 2016). As an example, recent studies have shown that different clone–rootstock combinations can influence and level cold hardiness differences among cultivars (Hébert-Haché et al., 2020). However, the possibility that the variability within clones of the Vitis vinifera species might be insufficient to compensate the phenological shifts caused by climate change must be contemplated; the need to introduce new varieties with the abandonment of the traditional ones will eventually arise if no measure is taken (Duchêne, 2016). Moreover, in addition to the already existing varieties, new ones could be generated through traditional breeding approaches or even genetic engineering. In any case, the comparison and analysis of different Vitis species could, first, help in clarifying the molecular regulators and drivers of cold hardiness, deacclimation, and budbreak and, second, allow the identification of targets to optimize clone selection and breeding efforts.
In this review, spring frost frequency and trends for different geographical regions are reported, together with the recent findings about the potential pathways involved in cold deacclimation and budbreak. We aim to provide an update on current status of research regarding the effects of climate change on grapevine phenology, with a focus on cold hardiness dynamics, budbreak, and the key molecular players involved in these processes. This will hopefully help in developing new ways to face current and future climate-related contingencies to allow berry ripening and harvest to be achieved in favorable conditions.
Several studies have assessed the impact of climate change on grapevine phenology and viticulture in the past and in the present (Biasi et al., 2019), and numerous models have been tested to predict future consequences (Caffarra and Eccel, 2011; Bonfante et al., 2017; Alikadic et al., 2019; Costa et al., 2019; Ramos and de Toda, 2020). Agroclimatic indices are considered more reliable than individual climatic variables to describe climate change effects (Santos et al., 2020); these tools allow to closely follow and simulate plant development in different scenarios and can be used to evaluate the potential of different areas for viticulture (Molitor et al., 2014; Blanco-Ward et al., 2019). Redistribution of wine production within continents is a likely perspective, and the change in viticultural suitability for different geographic regions has been calculated, showing agreement among 17 global climate models. Wine-producing regions will possibly decrease by 2050 (mainly in Mediterranean climate area), whereas expanding suitability has been predicted an increase for New Zealand, western North America, and Northern Europe (Hannah et al., 2013).
However, commonly bioclimatic indices used in viticulture (e.g., Huglin Index, Winkler Index, Dryness Index, Cool Night Index) are arguably replaced by dynamic crop models (e.g., STICS, BRIN), which combine several indices and integrate phenotype, soil, weather data, and management practices into a more comprehensive picture (Cortázar-atauri et al., 2009; Moriondo et al., 2013; Fraga et al., 2016). Heat requirements, determined in terms of growing-degree days (GDD), represent the climatic constraint that allows grape to successfully complete its annual cycle when met. Distinct phenological phases need different climatic conditions to take place (e.g., release from ecodormancy; Ruml et al., 2016). Higher temperatures lead to an acceleration of plant development, being a potential cause of premature loss of bud cold hardiness (Pagter and Arora, 2013; Londo and Kowaleski, 2017; Kovaleski et al., 2018). In fact, early events such as budbreak and flowering have been shown to be the most sensitive to temperature-driven variations as compared to later phases (Jones et al., 2005). This increases the chances of vulnerable green tissues to be exposed to late spring frost events, which have been known to be the cause of great yield losses in the past (Gu et al., 2008). The timing of budbreak is strictly linked to the end of dormancy, a genetically programmed state of self-arrest in which the bud stops its development to avoid breaking at unfavorable times (Lang et al., 1987; Horvath et al., 2003). Whether the risk of damage due to spring frosts is globally increasing is up to debate, although recent reports suggest the relevance of this phenomenon in several locations (Augspurger, 2013; Ma et al., 2018; Sgubin et al., 2018). Effects are expected to vary, depending on the geographical position, and changes in water availability need to be taken into account together with temperature variations. Great attention has been always given to budbreak timing as early dormancy release in cold winter regions can cause significant crop losses, and frost-protecting measures represent a notable cost for producers. To the contrary, warmer regions can be affected by low rates of budburst and lower productivity due to insufficient chilling during winter, making the use of artificial dormancy-breakers a necessity. Vineyards located in southern Europe (e.g., Italy, Spain, Portugal) are expected to experience increased water stress conditions especially during summer, leading, together with warming, to yield and quality reduction (Fraga et al., 2017; Santillán et al., 2019). Severe dryness is, in fact, the main reason impairing viticulture suitability in these areas. On the other hand, increasing average temperature has been predicted to have positive outcomes on winemaking regions in central and Western Europe and to allow the extension of viticultural areas in the north and east (Gaal et al., 2012; Cardell et al., 2019). This will favor the introduction of new currently inaccessible varieties in colder areas, as frost is expected to decrease and optimal ripening temperatures to be reached (e.g., Northern Europe, North America; Santillán et al., 2019); moreover, wine-producing suitable areas are expected to develop up to the 55°N by 2070 (Fraga et al., 2016).
Dormancy encompasses endodormancy, determined by internal factors, which allows buds to cold acclimate and reach a state of hardiness to survive freezing temperatures during winter. Cold acclimation is a process in which physiological, biochemical, and epigenetic changes driven by cold temperatures confer freezing tolerance (Wisniewski et al., 2018). Exposure to chilling temperatures, with difference depending on cultivar (Anzanello et al., 2018), is required to resume bud responsiveness to environmental signals and avoid growth start if mild temperatures occur during winter (Rohde and Bhalerao, 2007). Internal signals also prevent growth resumption in late summer or early autumn, which would cause the death of the bud in unfavorable environmental conditions (Lang et al., 1987; Horvath et al., 2003).
The productivity of grapevine and temperate plants is related to the capability of buds, both reproductive and vegetative, to tolerate freezing temperatures. Cold hardiness correlation with winter temperatures has been measured (Kovaleski et al., 2018). In general, sudden or recurring warm spells in winter can endanger the survival of woody perennials to freezing temperatures because the deacclimation process, during which cold tolerance is lost, is relatively fast (Pagter and Arora, 2013). Although deacclimation and acclimation cycles seem possible and efficient in several herbaceous plants (Vyse et al., 2019), it appears diverse for woody perennials with cold acclimation being restored only in part (Shin et al., 2015). Various grapevine species have been shown to be differently responsive to temperature variations during dormancy, likely related to the dissimilar chilling requirements that allow the transition from endodormancy to ecodormancy, at distinct timings. In addition, maximal cold hardiness is not reached automatically, and a cold sustained winter is needed (Londo and Kowaleski, 2017). Depending on the species, grapevine buds’ cold hardiness can reach temperatures below −30°C (Londo and Kowaleski, 2017). However, once buds begin to swell and deharden during the deacclimation process, their freezing tolerance quickly reduces, and the observed advancements in phenological timings may possibly increase the exposure of vulnerable plant structures to late frost events.
Late spring frosts have often resulted in great damage to cultivated fruit trees and in important economic losses (Gu et al., 2008; Marino et al., 2011; Ault et al., 2013; Vitasse and Rebetez, 2018). In the bigger picture, these phenomena can alter the ecosystem and evolution of entire populations because of competition among species and parasite opportunism (Inouye, 2000; Reineke and Thiéry, 2016). As previously stated, the vulnerability of plant structures to freezing temperatures differs, depending on their level of cold hardiness, which varies seasonally, and on their intrinsic ability to sustain lower temperatures. Green tissues, flowers, and fruit are, in fact, significantly more susceptible to lower temperatures than wooden tissues as their hydration levels are considerably higher, and their supercooling capabilities lower (Fennell, 2004). Budburst and leafout have been delineated as the most critical, as several trees have been shown to be the most vulnerable at that specific time (Vitasse et al., 2014; Lenz et al., 2016). Moreover, a lower temperature stability is expected during winter in the future, which will require the use of cultivars with a lower response to so-called “false springs” (Londo and Kowaleski, 2019). A “false spring” can be empirically defined as a period of warm temperatures with premature rapid vegetative growth, followed by a freeze (Gu et al., 2008; Ault et al., 2013); several mathematical approaches to evaluate these phenomena have been attempted (Marino et al., 2011). Freezing temperatures following a “false spring” can culminate in more serious damage, which affects photosynthetic tissue and reproductive tissue alike with consequences spread on multiple years of development (Carmona et al., 2008). In general, the influence of climate change on late frost events frequency and distribution remains unclear, and whether risk is increasing for temperate trees remains up for debate. The analysis of remote-sensing data showed that frost day in which the temperature drops below 0°C during the growing season have increased in the Northern Hemisphere (Liu et al., 2018). Concerning Europe, phenological and climate records were used to analyze the evolution of spring frost risk as regards several tree species, between 1950 and 2013, with a focus on determining variations in the frequency of the phenomenon (Ma et al., 2018). These results showed that species whose phenology is more responsive to temperature increases tend to experience a higher risk of being subjected to frost occurrences and damage. Maritime areas in Europe were also more exposed to frost compared to continental ones (Ma et al., 2018). Besides, high-altitude areas could experience decreased risk as the rate of warming seems to be amplified with elevation (Pepin et al., 2015). The effects of late frosts on the distribution of grapevine in Europe were analyzed (Leolini et al., 2018). The results, simulated under future scenarios, described in the AR5 IPCC (2014) report, show that budbreak and flowering advancement are more pronounced in Northeastern Europe compared to the Southwest. The simulations showed that changes in the phenology stages of grapevine might expose it to higher frequency of extreme events, with the effects being strictly linked to the phenological cycle of the considered variety (Leolini et al., 2018). An increased risk of spring frost damage is also predicted in several regions of France, supported by two budburst day simulation models (Sgubin et al., 2018). Similarly, a high probability of spring frost damage for several woody species in Illinois (United States) was reported, by integrating field observations of temperature, phenology, and frost damage over long timeframes (Augspurger, 2013). “False spring” occurrences were reviewed across the United States over the 1920–2013 interval by taking into consideration the trends of vegetation start dates, spring freezes, and a sensitivity analysis, which indicated a decrease in spring frost exposure (Peterson and Abatzoglou, 2014), pointing out distinct tendencies for different geographical locations.
Passive spring frost damage avoidance approaches are used preemptively and are suited to work on the long-term and include breeding and selection of new fitter varieties (Liu and Sherif, 2019). Traditional breeding approaches have been successfully used in the past to select new cultivars with characteristics of economic interest and in a perennial crop such as grapevine the entire traditional breeding procedure and evaluation process can take many years to be completed (Eibach and Töpfer, 2015). As cultivated grapevines are propagated clonally to fix and maintain specific production parameters, somatic variations that can accumulate during clonal propagation are almost the only source of genetic diversity (Carbonell-Bejerano et al., 2017; van Houten et al., 2020), greatly lower than intervarietal diversity (Roach et al., 2018). Clone collections exist and are available worldwide and represent a source that should be accessed to search for interesting genotypes (Duchêne, 2016). A possible adaptation for the current grape-growing areas should consist in the selection of varieties with a later ripening period; such varieties can be obtained from germplasm collections or through breeding processes (Duchêne et al., 2012).
Fruit trees must fulfill a chilling requirement to transition from endodormancy to ecodormancy, a phase of dormancy in which buds are responsive to growth-promoting conditions. The amount of chilling hours required to do so depends on the genotype, and genotypes that require less chilling have been shown to deacclimate earlier. In any case, the models describing winter chill accumulation are purely empirical or based on experiments in controlled conditions, and the physiological processes occurring in plants during winter are still poorly understood (Luedeling and Brown, 2011). The most popular chilling-hours accumulation models estimate effective chilling temperatures to be included in the 0–7.2°C interval (Dokoozlian, 1999), although different models attribute varying effectiveness to specific temperatures or even negative impacts of higher temperatures on previously accumulated chill (Darbyshire et al., 2011). The widely applied and possibly most accurate Dynamic Model also suggests that the same temperatures might have inconsistent effectiveness, depending on which time of the season they are registered, making it difficult to transfer available information from one location to another (Luedeling, 2012). Cultivated grapevines are generally considered low-chilling-requiring species compared to other woody perennials; however, chilling requirements can differ significantly in high‐ and low-chill varieties and fast‐ or slow-burst phenotypes (Londo and Johnson, 2014). Production located at higher latitudes could benefit from the use of grapevines characterized by higher chilling requirements and slower budburst rates, which would allow lowering the risk of spring frost damage (Londo and Johnson, 2014). Wild grapevines presented a continuous range of chilling requirements and budburst rates, making them an interesting source of variability. In detail, Vitis amurensis, Vitis labrusca, and Vitis riparia were classified as low-chill and fast-burst species, whereas Vitis rupestris, Vitis aestivalis, and Vitis vulpina showed higher chilling requirements (>1,000 h) and longer budburst timings (>14 days). Different latitudes were also proposed as seemingly having an adaptive effect. In fact, North-distributed genotypes (V. riparia, V. labrusca, and V. amurensis) were all classified as low-chill, fast-bursting species. On the contrary, southern varieties (V. aestivalis, V. cinerea, V. rupestris, and V. vulpina) were all characterized by higher chilling requirements and slower budburst timings (Londo and Johnson, 2014).
Hybrid crosses were shown to allow lowering the deepest level of cold hardiness, although this could also introduce enhanced midwinter responsiveness in areas where climate warming produces mild winter temperatures (Londo and Kowaleski, 2019). Deacclimation rates were also observed to be much faster in wild varieties V. riparia and V. amurensis, commonly used by breeders to increase freezing tolerance in cultivated varieties. This could contribute to increased risks of deacclimation during warmer winters and of spring frost damage (Kovaleski et al., 2018). These phenomena could be explained by the evolutionary necessity of these varieties to develop rapidly during short growing seasons typical of their area of origin (Ferguson et al., 2014). Paradoxically, this would make the varieties with the deepest levels of cold hardiness also the most vulnerable to spring frost damage (Ferguson et al., 2014), and considering the observed advancement of spring phenology, winter-hardy varieties could display unwanted phenotypes. For these reasons, focusing breeding efforts on the production of delayed growth-start cultivars could be an alternative favorable approach. A prerequisite for this strategy is the gaining of a comprehensive understanding of the biochemical and molecular mechanisms responsible for dormancy establishment and release in grapevine buds.
Rootstocks are traditionally used to protect scions from soil-borne pests and to improve tolerance to various abiotic stresses; however, their effects on the entirety of the plant often remain obscure (Ollat et al., 2016). The breeding of new rootstocks needs to be considered as a long-term strategy to cope with the consequences of climate change as the substitution of traditional scions with new ones is not going to be accepted as easily. The genetic background of commonly used rootstocks can be difficult to understand as their heritage is often mixed (Poczai et al., 2013), but efforts to improve breeding by enhancing the knowledge of genetic markers have been attempted in recent years (Migliaro et al., 2019; Riaz et al., 2019). This information is important and needs to be exploited to improve marker-assisted selection (MAS) of new rootstocks, as their influence on scion signaling molecules, response to several stresses, and even berry quality has been observed (Tramontini et al., 2013; Pagliarani et al., 2017; Martin et al., 2020; Zombardo et al., 2020). Moreover, rootstocks can alter scion development rate possibly because of their different abilities to take up nutrients and water from the soil (Zhang et al., 2016). Additionally, messenger RNA molecules and hormones have been reported to pass through the graft site in a possibly environment‐ and genotype-dependent manner (Nikolaou et al., 2000; Yang et al., 2015). Putative rootstock effects on grapevine phenology and, in particular, on its heat requirements have also been described (Miele, 2019).
A great boost in breeding effort can be attributed to the identification of molecular markers, the introduction of genetic mapping, and genotype–phenotype associations, considerably facilitated by the release of the complete sequence of the V. vinifera genome (Jaillon et al., 2007; Velasco et al., 2007). MAS can help the identification of sequences with different genetic backgrounds, aiding the potential exploitation of wild Vitis species carrying traits of interest (Daldoul et al., 2020).
Monitoring dormancy status of the bud in real time appears really challenging, because of the absence of visual changes during the bud dormancy cycle (Or, 2009), and the use of GDD as a proxy for spring phenology is not always reliable. Therefore, a better knowledge base of the physiological mechanisms underpinning dormancy induction and release can be an important part of predicting the potential effects of global warming on grapevine. A strict correlation between budbreak and loss of winter cold hardiness (deacclimation) has been recently hypothesized, pointing out that a temperature-controlled interplay underpins these phenological changes (Kovaleski and Londo, 2019).
In this context, recent advances in the understanding of cold hardiness and spring budburst mechanisms may contribute to enhance the sustainability of viticulture, especially when acute cold weather events are expected to increase (Kovaleski and Londo, 2019). On the other hand, traditional breeding is also empirical and requires a deep knowledge of the physiological characteristics of the selected cultivars in past and present cultivated areas. Recently introduced molecular approaches allowed new methods of “molecular breeding” to be applied, allowing speedier and refined crosses (Delrot et al., 2020).
Unfortunately, phenological traits, such as budburst, are often regulated by many quantitative trait loci (QTLs), which are highly responsive to environmental factors. For this reason, the mapping and cloning of genes related to phenological traits are really challenging, and the reproducibility of these QTLs remains low (Delrot et al., 2020).
Recently, several works have identified QTLs associated with budbreak. For example, two independent QTLs on chromosomes 4 and 19 were identified using a genetic map build with microsatellites markers on varieties Riesling and Gewurztraminer (Duchêne et al., 2012). The WRKY transcription factor VvWRKY3 was found within the confidence interval on chromosome 19; a similar transcription factor, AtWRKY2 from Arabidopsis, was shown to mediate ABA (abscisic acid) control on seed germination (Jiang and Yu, 2009). Moreover, several genes encoding glutathione S-transferases (GSTs) were also identified on both chromosomes 4 and 19. Increased levels of expression of these genes were registered after both HC (hydrogen cyanamide) application (Or, 2009), a dormancy-breaking agent, and after the natural fulfillment of chilling requirements (Pacey-Miller et al., 2003). Similarly, simple sequence repeats (SSRs) and single nucleotide polymorphisms (SNPs) were used to map another QTL related to budburst on chromosome 15, overlapping on QTLs related to veraison (Grzeskowiak et al., 2013). Genes on chromosome 15 included several transcription factors involved in bud and fruit development (Grzeskowiak et al., 2013).
With regard to cold hardiness control, the progeny resulting from the cross between cold-vulnerable cv. Cabernet Sauvignon and the cold-tolerant hybrid Zuoyouhong was used for the construction of a high-density genetic linkage map on which cold hardiness-related QTLs were mapped (Su et al., 2020). Six QTLs located on chromosomes 2, 3, and 15 were identified, and four cold-responsive candidate genes were proposed. In detail, a dehydration-responsive protein containing a cis-DRE (dehydration responsive) element was identified. CRT (C-repeat)/DRE elements, containing a core CCGAC sequence designated as C-repeat, are present in single or multiple copies in the promoter regions of plant COR (cold-responsive) genes, which are induced by low-temperature exposure (Stockinger et al., 1997). The COP9 signalosome (CSN) subunit 1 was also individuated; CSN was shown to be required for the expression of COR genes in Arabidopsis (Schwechheimer et al., 2002). Additionally, an RRM (RNA recognition motif)–containing protein was found to be putatively involved in cold hardiness as well. RRM modules were found in cold-responsive RNA-binding proteins from cyanobacteria (Maruyama et al., 1999). Lastly, a MYB-related gene’s expression was also reported to be enhanced by cold exposure. Its overexpression in Arabidopsis was previously shown to confer increased tolerance to cold (Sun et al., 2018).
Transcriptomic tools have led to new insights into the gene expression processes that take place in dormant tissues. Dormancy release is regulated by a multitude of independent genes whose mechanisms of action are still unclear, together with their conservation among species (Table 1). Growth resumption happens simultaneously with cold deacclimation, although most hardiness is already lost when new tissue is visible (Kovaleski and Londo, 2019). Growth start is also subordinate to the fulfillment of the chilling requirement and the transition from endodormancy to ecodormany, in which the bud becomes sensitive to favorable environmental conditions. CBFs/DREBs (C-repeat binding factors/dehydration responsive element binding) are important cold-response regulators stimulated by low temperatures. These transcription factors act as a part of a signaling cascade in which they are induced by ICEs (inducers of CBF expression) and activate COR genes by binding to the CRT/DRE cis-elements in their promoter regions and thus conferring freezing tolerance to the plant (Chinnusamy et al., 2010; Thomashow, 2010). Another cold-responsive transcription factor, bHLH, was characterized in both V. vinifera cv. Cabernet Sauvignon and wild V. amurensis with a proposed putative regulatory role in cold stress response in a CBF-dependent way (Xu et al., 2014). Changes in expression levels and timing of VvbHLH and VabHLH were observed, possibly caused by differences in the cis-regulatory elements in their sequence (Xu et al., 2014). CBFs/DREBs have been identified in several woody species as well as Arabidopsis, and their functions are highly conserved (Wisniewski et al., 2014). Several CBFs/DREBs are known in grapevine (Xiao et al., 2006; Tillett et al., 2012; Rubio et al., 2019a) and show increased mRNA expression following exposure to freezing temperatures (Xiao et al., 2006, 2008). The most well-known targets of CBFs/DREBs are DHNs (dehydrins), part of the LEA (late embryogenesis abundant) proteins. DHNs accumulate during dormancy induction and cold acclimation and protect cells from dehydration damage (Wisniewski et al., 2014). Four grape DHNs have been identified (Yang et al., 2012). DHNs were reported to be differently expressed in wild V. riparia and in cultivated variety Chardonnay following cold exposure (Xiao and Nassuth, 2006). Increased freezing tolerance is also observed in case of VvCBFs overexpression (Tillett et al., 2012). Moreover, the synergistic effect of low temperatures and ABA application in stimulating the expression of CBFs/DREBs in grapevine dormant buds has been recently assessed (Rubio et al., 2019a). ABA has a key role in plant dormancy regulation as ABA variations have been correlated to different degrees of seed dormancy (Nambara et al., 2010). ABA’s role in bud dormancy in woody perennials has been hypothesized, although the regulation mechanism is complex and is still obscure. Recently, several studies showed that the highest levels of ABA were reached at the maximum depth of dormancy and started decreasing at the end of endodormancy in grapevine buds (Kovaleski and Londo, 2019; Rubio et al., 2019b). ABA was also observed to promote starch synthesis in dormant buds, thus promoting their sink capacity and regulating dormancy depth this way (Rubio et al., 2019b). Changing ABA balance in the buds is also the mechanism by which dormancy-breaking agents, such as HC, seem to accomplish their effect (Zheng et al., 2015; Rubio et al., 2019b). In detail, the budbreaking effect of HC in grapevine was reported to be exerted by the stimulation of the ABA-degrading enzyme ABA 8′-hydrolase (A8H), encoded by the VvA8H-CYP707A4 gene (Zheng et al., 2015). A8H and ABA catabolite increase was also observed during natural dormancy release (Zheng et al., 2015). Moreover, the reversible ability of ABA to prevent loss of cold hardiness and deacclimation after several days of prolonged application on grapevine buds was observed (Kovaleski and Londo, 2019). Together, these results suggest an important role of ABA in endodormancy maintenance and dormancy release, but not in its induction. More recent studies showed that transgenic vines overexpressing VvA8H-CYP707A4 show both a higher catabolism of ABA and an enhancement of budbreak. Hypoxia and ethylene, which are both considered dormancy release stimulants, enhance the expression of VvA8H-CYP707A4 (Zheng et al., 2018a). Multiple studies have shed light on the role of other hormones in dormancy release and budbreak; for example, a recent work focused on the expression of several genes involved in the gibberellin (GA) biosynthetic pathway and the interaction of GAs with cytokinins (CKs) in grapevine buds (Zheng et al., 2018b). Although further studies are required, the authors propose an inhibitory effect of GA on budbreak that would give account of the low levels of this hormone registered during dormancy. Authors also hypothesize that this inhibition results from the antagonistic effect of GAs on CK responses, which are required for bud meristem reactivation; only following meristem activation higher levels of GA could be required to sustain growth and budbreak (Zheng et al., 2018b). In addition to this, the effects of cold temperatures on the concentration of salicylic acid (SA) and the expression of genes in its biosynthetic pathway in dormant grapevine buds were also explored (Orrantia-Araujo et al., 2020). Buds exposed to longer periods of chilling hours showed a higher content of endogenous SA once transferred in forcing conditions. The expression of genes ICS2 (isochorismate synthase 2), NPR1 (nonexpressor of PR genes 1) and WRKY70 showed variations in buds subjected to cold treatment compared to control ones. ICS2 takes part in the biosynthesis pathway of SA, NPR1 is a master regulator of SA-mediated defense signaling, and WRKY70 participates in both positive and negative regulation of SA signaling. These results indicate that cold accumulation could stimulate the synthesis of SA in grapevine buds and introduce the possibility of a role of SA-mediated defense signaling in bud dormancy release (Orrantia-Araujo et al., 2020).
The discovery and characterization of the EBB1 gene with a role in shoot growth resumption after winter have been carried out both in Populus (Yordanov et al., 2014) and in peach, where RNA-seq analysis confirmed that EBB1 is involved in budbreak by taking part into the regulation of several pathways that act synergistically and involve hormones, cell division, and cell wall modifications (Zhao et al., 2020). The conservation of this AP2/ERF family transcription factor was evidenced by the identification of several homologs in various woody perennial species, among which also is V. vinifera (Busov et al., 2016). Consistently with the EBB1 expression in Poplar, VvEBB1 resulted greatly downregulated during dormancy and upregulated before budbreak.
It is well-known that genomic DNA methylation is a mechanism that influences gene expression. In plants, a subgroup of DNA glycosylase-lyases, known as DEMETER-LIKE DNA demethylases (DMLs), can actively demethylate DNA and have been shown to be involved in abiotic stress responses in Arabidopsis (Le et al., 2014), developmental transitions in tomato (Liu et al., 2015), and nodule development in Medicago truncatula (Satgé et al., 2016). A Populus trichocarpa DML, PtaDML10, was proposed to be responsible for DML-mediated demethylation at the shoot apical meristem in budbreak regulation (Conde et al., 2017). A loss-of-function analysis confirmed the chilling-responsive demethylation performed by DML10 in proximity to dormancy release. RNA-seq combined with methylome data analysis revealed that the DML10 gene targets are genetically associated with budbreak (Conde et al., 2017). Moreover, no overlap was found between the targets of DML10-mediated demethylation and EBB1 targets in poplar. This seemingly confirms that these genes act on separate pathways (Conde et al., 2017). No evidence on the role of DML genes on grapevine dormancy release currently exists, although several DML demethylases have been identified (Shangguan et al., 2020).
Additionally, regulated hypoxia has been found to be a development signal in several stages of plant life (Gibbs et al., 2014; Abbas et al., 2015), and many responses to hypoxia are regulated by group VII of ethylene responsive transcription factors (ERF-VIIs) (Gibbs et al., 2014). For these reasons, the role of oxygen-dependent signaling in transcriptional and metabolic reactivation during budburst in grapevine was investigated (Meitha et al., 2018). The data support that oxygen-dependent signaling through grape ERFs is involved in the transition from dormancy to budburst. Moreover, approximately 20% of grapevine genes presenting a HRPE (hypoxia-responsive promoter element) motif in their promoter were differently expressed in the first 24 h of budburst (Meitha et al., 2018). These results strongly suggest an important developmental function of oxygen-dependent signaling through VvERF-VIIs in determining timing and coordination of budburst in grapevines. Further support of the role of oxidative stress response pathways in grapevine budbreak regulation is provided by Kovaleski and Londo (2019), proposing the expression of RBOHF (respiratory burst oxidase homolog protein F) as a marker for budbreak. RBOHF is involved in ABA and ethylene signaling through H2O2 production (Kwak et al., 2003). In addition to this, two ERF genes from Chinese wild Vitis pseudoreticulata, VpERF2 and VpERF3, were reported to be involved in abiotic stress response pathways including cold exposure (Zhu et al., 2013). Overexpression studies also pointed out a role of these transcription factors in pathogenesis-related proteins accumulation. Moreover, ABA-dependent expression of VpERF2 and SA-dependent expression of VpERF3 were shown through exogenous hormone application on leaves (Zhu et al., 2013).
Recently, dormant buds of several Vitis genotypes, belonging to different species, were observed to sense the stimulus for dormancy release and deacclimation simultaneously when put into the same forcing conditions (Kovaleski and Londo, 2019). The observed differences in budbreak timings would then be attributed to the ability of the specific genotypes to restart growth. In fact, temperature sensing is believed to be the first step toward bud growth. Among the first sensors, membrane CNGCs (cyclic nongated ion channels) are very responsive to temperature changes. These nonselective Ca2+ channels are placed as very first components of the thermosensing pathways in Arabidopsis and Physcomitrella (Finka et al., 2012) and possibly have the ability to sense membrane fluidity changes caused by temperature shifts (Finka and Goloubinoff, 2014). Synchronous downregulation of nuclear-localized CNGC15 and FAD5 (fatty acid desaturase 5) was reported, suggesting a role of nuclear Ca2+ signaling during dormancy in grapevine buds (Kovaleski and Londo, 2019). A role in cold and water stress response of Ca2+ flux sensor VaCPK20 (calcium-dependent protein kinase) from wild V. amurensis vines was also suggested (Dubrovina et al., 2013).
Spring frost damage risk cannot be overlooked in the future in several areas of the world, making the identification of effective adaptive measures an issue of the present. Understanding the molecular mechanisms underlying cold hardiness loss/deacclimation and budbreak is essential for improving crop sustainability and adaptation in the future changing climate. The observations gathered so far on cold deacclimation and dormancy release regulation in grapevine outline a very complex scenario in which many pathways are involved (Figure 1). As chilling requirement, deacclimation dynamics, and budbreak timing appear tightly connected, a major regulatory role can be ascribed to temperature-sensing related genes, common among different genotypes. Hormonal interplay, at times synergistic as well as antagonistic or seemingly independent, should also draw great attention as not only ABA’s expected involvement seems ascertained, but also growth reactivation-related, defense-related, and oxidative stress–related hormones putatively perform actively in the regulation of these phenomena. A third valuable and worthy of notice opportunity concerns epigenetics and epigenetic regulators, which add an extra layer of complexity. Defining the extent of the role and significance of each component of this intricate net of regulators requires further studies.
Figure 1. Schematic representation of the current knowledge on the molecular control of bud dormancy-budbreak transition. Temperature plays a key role in influencing both phenological stages. Most of the gene functions involved at each phenological stage are reported, as well as their interplay with other metabolic and hormonal signaling pathways.
Breeding efforts need to focus on the potential of wild Vitis varieties to bear favorable traits, starting from changing chilling requirements and budburst rates. In this regard, the accuracy of all most popularly used chilling-hours accumulation models needs to be standardized in order to select varieties suitable to changing conditions is specific areas. An intense application of genetic mapping approaches is required to locate and isolate the genetic loci that are responsible for the phenotypic expression of these characteristics so that traditional or new plant breeding techniques can be carried out more swiftly and purposefully (Figure 2). Despite the complexity of the full picture and the uncertainties about the connections among the players, the variety of elements involved allows tackling the problem through a multitude of approaches and should be considered encouraging.
Figure 2. Schematic overview of traditional and new breeding approaches to cope with climate change issues. Natural variability and genetic knowledge are important building blocks of breeding; phenology-related traits are the main target. GWAS, genome-wide association studies; MAS, marker-assisted selection.
All authors listed have made a substantial, direct and intellectual contribution to the work, and approved it for publication.
VDR activity was supported by a grant of the Italian Ministry of Education, Research and University (MIUR).
The authors declare that the research was conducted in the absence of any commercial or financial relationships that could be construed as a potential conflict of interest.
We would like to thank Department of Agricultural, Food, Environmental, and Animal Sciences, University of Udine for funding newly hired researchers.
Abad, F. J., Marín, D., Loidi, M., Miranda, C., Royo, J. B., Urrestarazu, J., et al. (2019). Evaluation of the incidence of severe trimming on grapevine (Vitis vinifera L.) water consumption. Agric. Water Manag. 213, 646–653. doi: 10.1016/j.agwat.2018.10.015
Abbas, M., Berckhan, S., Rooney, D. J., Gibbs, D. J., Vicente Conde, J., Sousa Correia, C., et al. (2015). Oxygen sensing coordinates photomorphogenesis to facilitate seedling survival. Curr. Biol. 25, 1483–1488. doi: 10.1016/j.cub.2015.03.060
Alikadic, A., Pertot, I., Eccel, E., Dolci, C., Zarbo, C., Caffarra, A., et al. (2019). The impact of climate change on grapevine phenology and the influence of altitude: a regional study. Agric. For. Meteorol. 271, 73–82. doi: 10.1016/j.agrformet.2019.02.030
Anzanello, R., Fialho, F. B., and dos Santos, H. P. (2018). Chilling requirements and dormancy evolution in grapevine buds. Cienci Agrotec. 42, 364–371. doi: 10.1590/1413-70542018424014618
Augspurger, C. K. (2013). Reconstructing patterns of temperature, phenology, and frost damage over 124 years: spring damage risk is increasing. Ecology 94, 41–50. doi: 10.1890/12-0200.1
Ault, T. R., Henebry, G. M., de Beurs, K. M., Schwartz, M. D., Betancourt, J. L., and Moore, D. (2013). The false spring of 2012, earliest in north American record. Eos 94, 181–182. doi: 10.1002/2013EO200001
Biasi, R., Brunori, E., Ferrara, C., and Salvati, L. (2019). Assessing impacts of climate change on phenology and quality traits of Vitis vinifera L.: the contribution of local knowledge. Plants 8:121. doi: 10.3390/plants8050121
Blanco-Ward, D., Ribeiro, A., Barreales, D., Castro, J., Verdial, J., Feliciano, M., et al. (2019). Climate change potential effects on bioclimatic indices: a casa study for the Portuguese demarcated Douro region (Portugal). BIO Web Conf. 12:01013. doi: 10.1051/bioconf/20191201013
Bonada, M., Jeffery, D. W., Petrie, P. R., Moran, M. A., and Sadras, V. O. (2015). Impact of elevated temperature and water deficit on the chemical and sensory profiles of Barossa Shiraz grapes and wines. Aust. J. Grape Wine Res. 21, 240–253. doi: 10.1111/ajgw.12142
Bonfante, A., Alfieri, S. M., Albrizio, R., Basile, A., De Mascellis, R., Gambuti, A., et al. (2017). Evaluation of the effects of future climate change on grape quality through a physically based model application: a case study for the Aglianico grapevine in Campania region, Italy. Agric. Syst. 152, 100–109. doi: 10.1016/j.agsy.2016.12.009
Busov, V., Carneros, E., and Yakovlev, I. (2016). EARLY BUD-BREAK1 (EBB1) defines a conserved mechanism for control of BUD-BREAK in woody perennials. Plant Signal. Behav. 11:e1073873. doi: 10.1080/15592324.2015.1073873
Caffarra, A., and Eccel, E. (2011). Projecting the impacts of climate change on the phenology of grapevine in a mountain area. Aust. J. Grape Wine Res. 17, 52–61. doi: 10.1111/j.1755-0238.2010.00118.x
Carbonell-Bejerano, P., Royo, C., Torres-Pérez, R., Grimplet, J., Fernandez, L., Franco-Zorrilla, J. M., et al. (2017). Catastrophic unbalanced genome rearrangements cause somatic loss of berry color in grapevine. Plant Physiol. 175, 786–801. doi: 10.1104/pp.17.00715
Cardell, M. F., Amengual, A., and Romero, R. (2019). Future effects of climate change on the suitability of wine grape production across Europe. Reg. Environ. Chang. 19, 2299–2310. doi: 10.1007/s10113-019-01502-x
Carmona, M. J., Chaïb, J., Martínez-Zapater, J. M., and Thomas, M. R. (2008). A molecular genetic perspective of reproductive development in grapevine. J. Exp. Bot. 59, 2579–2596. doi: 10.1093/jxb/ern160
Centinari, M., Gardner, D. M., Smith, D. E., and Smith, M. S. (2018). Impact of amigo oil and KDL on grapevine postbudburst freeze damage, yield components, and fruit and wine composition. Am. J. Enol. Vitic. 69, 77–88. doi: 10.5344/ajev.2017.17030
Chinnusamy, V., Zhu, J. K., and Sunkar, R. (2010). Gene regulation during cold stress acclimation in plants. Methods Mol. Biol. 639, 39–55. doi: 10.1007/978-1-60761-702-0_3
Conde, D., Le Gac, A. L., Perales, M., Dervinis, C., Kirst, M., Maury, S., et al. (2017). Chilling-responsive DEMETER-LIKE DNA demethylase mediates in poplar bud break. Plant Cell Environ. 40, 2236–2249. doi: 10.1111/pce.13019
Coombe, B. G. (1987). Influence of temperature on composition and quality of grapes. Acta Hortic. 206, 23–36. doi: 10.17660/actahortic.1987.206.1
Cortázar-atauri, I. G. D., Brisson, N., and Gaudillere, J. P. (2009). Performance of several models for predicting budburst date of grapevine (Vitis vinifera L.). Int. J. Biometeorol. 53, 317–326. doi: 10.1007/s00484-009-0217-4
Costa, R., Fraga, H., Fonseca, A., de Cortazar-Atauri, I. G., Val, M. C., Carlos, C., et al. (2019). Grapevine phenology of cv. Touriga Franca and Touriga Nacional in the Douro wine region: Modelling and climate change projections. Agronomy 9:210. doi: 10.3390/agronomy9040210
Daldoul, S., Boubakri, H., Gargouri, M., and Mliki, A. (2020). Recent advances in biotechnological studies on wild grapevines as valuable resistance sources for smart viticulture. Mol. Biol. Rep. 47, 3141–3153. doi: 10.1007/s11033-020-05363-0
Darbyshire, R., Webb, L., Goodwin, I., and Barlow, S. (2011). Winter chilling trends for deciduous fruit trees in Australia. Agric. For. Meteorol. 151, 1074–1085. doi: 10.1016/j.agrformet.2011.03.010
Delrot, S., Grimplet, J., Carbonell-Bejerano, P., Schwandner, A., Bert, P.-F., Bavaresco, L., et al. (2020). “Genetic and genomic approaches for adaptation of grapevine to climate change” in Genomic Designing of Climate-Smart Fruit Crops. ed. C. Kole (Cham: Springer), 157–270.
Dokoozlian, N. K. (1999). Chilling temperature and duration interact on the budbreak of “Perlette” grapevine cuttings. HortScience 34, 1054–1056. doi: 10.21273/hortsci.34.6.1
Dubrovina, A. S., Kiselev, K. V., and Khristenko, V. S. (2013). Expression of calcium-dependent protein kinase (CDPK) genes under abiotic stress conditions in wild-growing grapevine Vitis amurensis. J. Plant Physiol. 170, 1491–1500. doi: 10.1016/j.jplph.2013.06.014
Duchêne, É. (2016). How can grapevine genetics contribute to the adaptation to climate change? Oeno One 50, 113–124. doi: 10.20870/oeno-one.2016.50.3.98
Duchêne, E., Butterlin, G., Dumas, V., and Merdinoglu, D. (2012). Towards the adaptation of grapevine varieties to climate change: QTL and candidate genes for developmental stages. Theor. Appl. Genet. 124, 623–635. doi: 10.1007/s00122-011-1734-1
Eibach, R., and Töpfer, R. (2015). “Traditional grapevine breeding techniques” in Grapevine Breeding Programs for the Wine Industry. ed. A. Reynolds (Woodhead Publishing), 3–22.
Fennell, A. (2004). Freezing tolerance and injury in grapevines. J. Crop Improv. 10, 201–235. doi: 10.1300/J411v10n01_09
Ferguson, J. C., Moyer, M. M., Mills, L. J., Hoogenboom, G., and Keller, M. (2014). Modeling dormant bud cold hardiness and Budbreak in twenty-three Vitis genotypes reveals variation by region of origin. Am. J. Enol. Vitic. 65, 59–71. doi: 10.5344/ajev.2013.13098
Finka, A., Cuendet, A. F. H., Maathuis, F. J. M., Saidi, Y., and Goloubinoff, P. (2012). Plasma membrane cyclic nucleotide gated calcium channels control land plant thermal sensing and acquired thermotolerance. Plant Cell 24, 3333–3348. doi: 10.1105/tpc.112.095844
Finka, A., and Goloubinoff, P. (2014). The CNGCb and CNGCd genes from Physcomitrella patens moss encode for thermosensory calcium channels responding to fluidity changes in the plasma membrane. Cell Stress Chaperones 19, 83–90. doi: 10.1007/s12192-013-0436-9
Fraga, H., de Cortázar Atauri, G. I., Malheiro, A. C., Moutinho-Pereira, J., and Santos, J. A. (2017). Viticulture in Portugal: a review of recent trends and climate change projections. Oeno One 51, 61–69. doi: 10.20870/oeno-one.2017.51.2.1621
Fraga, H., de Cortázar Atauri, I. G., Malheiro, A. C., and Santos, J. A. (2016). Modelling climate change impacts on viticultural yield, phenology and stress conditions in Europe. Glob. Chang. Biol. 22, 3774–3788. doi: 10.1111/gcb.13382
Friend, A. P., and Trought, M. C. T. (2007). Delayed winter spur-pruning in New Zealand can alter yield components of merlot grapevines. Aust. J. Grape Wine Res. 13, 157–164. doi: 10.1111/j.1755-0238.2007.tb00246.x
Frioni, T., Tombesi, S., Silvestroni, O., Lanari, V., Bellincontro, A., Sabbatini, P., et al. (2016). Spur-pruning applied after bud burst limits yield and delayed fruit sugar accumulation in cv. Sangiovese in Central Italy. Am. J. Enol. Vitic. 67, 419–425. doi: 10.5344/ajev.2016.15120
Gaal, M., Moriondo, M., and Bindi, M. (2012). Modelling the impact of climate change on the Hungarian wine regions using random forest. Appl. Ecol. Environ. Res. 10, 121–140. doi: 10.15666/aeer/1002_121140
Gibbs, D. J., MdIsa, N., Movahedi, M., Lozano-Juste, J., Mendiondo, G. M., Berckhan, S., et al. (2014). Nitric oxide sensing in plants is mediated by Proteolytic control of group VII ERF transcription factors. Mol. Cell 53, 369–379. doi: 10.1016/j.molcel.2013.12.020
Grzeskowiak, L., Costantini, L., Lorenzi, S., and Grando, M. S. (2013). Candidate loci for phenology and fruitfulness contributing to the phenotypic variability observed in grapevine. Theor. Appl. Genet. 126, 2763–2776. doi: 10.1007/s00122-013-2170-1
Gu, L., Hanson, P. J., Post, W. M., Kaiser, D. P., Yang, B., Nemani, R., et al. (2008). The 2007 eastern US spring freeze: increased cold damage in a warming world? Bioscience 28, 253–262. doi: 10.1641/B580311
Hannah, L., Roehrdanz, P. R., Ikegami, M., Shepard, A. V., Shaw, M. R., Tabor, G., et al. (2013). Climate change, wine, and conservation. PNAS 110, 6907–6912. doi: 10.1073/pnas.1210127110
Hébert-Haché, A., Inglis, D., Kemp, B., and Willwerth, J. J. (2020). Clone and rootstock interactions influence the cold hardiness of Vitis vinifera cvs. Riesling and sauvignon blanc. Am. J. Enol. Vitic. 72:ajev.2020.20025. doi: 10.5344/ajev.2020.20025
Herrera, J. C., Bucchetti, B., Sabbatini, P., Comuzzo, P., Zulini, L., Vecchione, A., et al. (2015). Effect of water deficit and severe shoot trimming on the composition of Vitis vinifera L. merlot grapes and wines. Aust. J. Grape Wine Res. 21, 254–265. doi: 10.1111/ajgw.12143
Horvath, D. P., Anderson, J. V., Chao, W. S., and Foley, M. E. (2003). Knowing when to grow: signals regulating bud dormancy. Trends Plant Sci. 8, 534–540. doi: 10.1016/j.tplants.2003.09.013
Inouye, D. W. (2000). The ecological and evolutionary significance of frost in the context of climate change. Ecol. Lett. 3, 457–463. doi: 10.1046/j.1461-0248.2000.00165.x
IPCC (2014). Climate Change 2014: Synthesis Report. Contribution of Working Groups I, II and III to the Fifth Assessment Report of the Intergovernmental Panel on Climate Change. eds. Core Writing Team R. K. Pachauri and L. A. Meyer (Geneva: IPCC), 151.
IPCC (2019). Climate Change and Land: An IPCC Special Report on Climate Change, Desertification, Land Degradation, Sustainable Land Management, Food Security, and Greenhouse Gas Fluxes in Terrestrial Ecosystems. eds. P. R. Shukla, J. Skea, E. C. Buendia, V. Masson-Delmotte, H. O. Pörtner, and D. C. Roberts, et al. (In press).
Jaillon, O., Aury, J. M., Noel, B., Policriti, A., Clepet, C., Casagrande, A., et al. (2007). The grapevine genome sequence suggests ancestral hexaploidization in major angiosperm phyla. Nature 449, 463–467. doi: 10.1038/nature06148
Jiang, W., and Yu, D. (2009). Arabidopsis WRKY2 transcription factor mediates seed germination and postgermination arrest of development by abscisic acid. BMC Plant Biol. 9:96. doi: 10.1186/1471-2229-9-96
Jones, G. V., Duchene, E., Tomasi, D., Yuste, J., Braslavka, O., Schultz, H., et al. (2005). “Change in European Winegrape phenology and relationship with climate” in Proceedings of XIV GESCO Symposium. Vol. 1. 55–61.
Kliewer, W. M., and Torres, R. E. (1972). Effect of controlled day and night temperatures on grape coloration. Am. J. Enol. Vitic. 23, 71–77.
Kovaleski, A. P., and Londo, J. P. (2019). Tempo of gene regulation in wild and cultivated Vitis species shows coordination between cold deacclimation and budbreak. Plant Sci. 287:110178. doi: 10.1016/j.plantsci.2019.110178
Kovaleski, A. P., Reisch, B. I., and Londo, J. P. (2018). Deacclimation kinetics as a quantitative phenotype for delineating the dormancy transition and thermal efficiency for budbreak in Vitis species. AoB Plants 10:ply066. doi: 10.1093/aobpla/ply066
Kwak, J. M., Mori, I. C., Pei, Z. M., Leonhard, N., Angel Torres, M., Dangl, J. L., et al. (2003). NADPH oxidase AtrbohD and AtrbohF genes function in ROS-dependent ABA signaling in arabidopsis. EMBO J. 22, 2623–2633. doi: 10.1093/emboj/cdg277
Lang, G. A., Early, J. D., Martin, G. C., and Darnell, R. L. (1987). Endo-, Para-, and ecodormancy: physiological terminology and classification for dormancy research. Hortic. Sci. 22, 371–377.
Le, T. N., Schumann, U., Smith, N. A., Tiwari, S., Khang Au, P. C., Zhu, Q. H., et al. (2014). DNA demethylases target promoter transposable elements to positively regulate stress responsive genes in Arabidopsis. Genome Biol. 15:458. doi: 10.1186/s13059-014-0458-3
Lenz, A., Hoch, G., Körner, C., and Vitasse, Y. (2016). Convergence of leaf-out towards minimum risk of freezing damage in temperate trees. Funct. Ecol. 30, 1480–1490. doi: 10.1111/1365-2435.12623
Leolini, L., Moriondo, M., Fila, G., Costafreda-Aumedes, S., Ferrise, R., and Bindi, M. (2018). Late spring frost impacts on future grapevine distribution in Europe. Field Crop Res. 222, 197–208. doi: 10.1016/j.fcr.2017.11.018
Liu, R., How-Kit, A., Stammitti, L., Teyssier, E., Rolin, D., Mortain-Bertrand, A., et al. (2015). A DEMETER-like DNA demethylase governs tomato fruit ripening. Proc. Natl. Acad. Sci. U. S. A. 112, 10804–10809. doi: 10.1073/pnas.1503362112
Liu, Q., Piao, S., Janssens, I. A., Fu, Y., Peng, S., Lian, X., et al. (2018). Extension of the growing season increases vegetation exposure to frost. Nat. Commun. 9:426. doi: 10.1038/s41467-017-02690-y
Liu, J., and Sherif, S. M. (2019). Combating spring frost with ethylene. Front. Plant Sci. 10:1408. doi: 10.3389/fpls.2019.01408
Londo, J. P., and Johnson, L. M. (2014). Variation in the chilling requirement and budburst rate of wild Vitis species. Environ. Exp. Bot. 106, 138–147. doi: 10.1016/j.envexpbot.2013.12.012
Londo, J. P., and Kowaleski, A. P. (2017). Characterization of wild north American grapevine cold hardiness using differential thermal analysis. Am. J. Enol. Vitic. 68, 203–212. doi: 10.5344/ajev.2016.16090
Londo, J. P., and Kowaleski, A. P. (2019). Deconstructing cold hardiness: variation in supercooling ability and chilling requirements in the wild grapevine Vitis riparia. Aust. J. Grape Wine R. 25, 276–285. doi: 10.1111/ajgw.12389
Luedeling, E. (2012). Climate change impacts on winter chill for temperate fruit and nut production: a review. Sci. Hortic. 144, 218–229. doi: 10.1016/j.scienta.2012.07.011
Luedeling, E., and Brown, P. H. (2011). A global analysis of the comparability of winter chill models for fruit and nut trees. Int. J. Biometeorol. 55, 411–421. doi: 10.1007/s00484-010-0352-y
Ma, Q., Huang, J. G., Hänninen, H., and Berninger, F. (2018). Divergent trends in the risk of spring frost damage to trees in Europe with recent warming. Glob. Chang. Biol. 25, 351–360. doi: 10.1111/gcb.14479
Marino, G. P., Kaiser, D. P., Gu, L., and Ricciuto, D. M. (2011). Reconstruction of false spring occurrences over the southeastern United States 1901-2007: an increasing risk of spring freeze damage? Environ. Res. Lett. 6:024015. doi: 10.1088/1748-9326/6/2/024015
Martin, L., Vila, H., Bottini, R., and Berli, F. (2020). Rootstocks increase grapevine tolerance to NaCl through ion compartmentalization and exclusion. Acta Physiol. Plant. 42:145. doi: 10.1007/s11738-020-03136-7
Maruyama, K., Sato, N., and Ohta, N. (1999). Conservation of structure and cold-regulation of RNA-binding proteins in cyanobacteria: probable convergent evolution with eukaryotic glycine-rich RNA-binding proteins. Nucleic Acids Res. 27, 2029–2036. doi: 10.1093/nar/27.9.2029
McIntyre, G. N., Lider, L. A., and Ferrari, N. L. (1982). The chronological classification of grapevine phenology. Am. J. Enol. Vitic. 33, 80–85.
Meitha, K., Agudelo-Romero, P., Signorelli, S., Gibbs, D. J., Considine, J. A., Foyer, C. H., et al. (2018). Developmental control of hypoxia during bud burst in grapevine. Plant Cell Environ. 41, 1154–1170. doi: 10.1111/pce.13141
Miele, A. (2019). Rootstock-scion interaction: 6. Phenology, chilling and heat requirements of cabernet sauvignon grapevine. Rev. Bras. Frutic. 41:e-446. doi: 10.1590/0100-29452019446
Migliaro, D., De Lorenzis, G., Di Lorenzo, G. S., Nardi, B., De Gardiman, M., Failla, O., et al. (2019). Grapevine non-vinifera genetic diversity assessed by simple sequence repeat markers as a starting point for new rootstock breeding programs. Am. J. Enol. Vitic. 70, 390–397. doi: 10.5344/ajev.2019.18054
Molitor, D., Baus, O., Hoffmann, L., and Beyer, M. (2016). Meteorological conditions determine the thermal-temporal position of the annual botrytis bunch rot epidemic on Vitis vinifera L. cv. Riesling grapes. Oeno One 50, 231–244. doi: 10.20870/oeno-one.2016.50.4.36
Molitor, D., Junk, J., Evers, D., Hoffmann, L., and Beyer, M. (2014). A high-resolution cumulative degree day-based model to simulate phenological development of grapevine. Am. J. Enol. Vitic. 65, 72–80. doi: 10.5344/ajev.2013.13066
Moriondo, M., Jones, G. V., Bois, B., Dibari, C., Ferrise, R., Trombi, G., et al. (2013). Projected shifts of wine regions in response to climate change. Clim. Chang. 119, 825–839. doi: 10.1007/s10584-013-0739-y
Nambara, E., Okamoto, M., Tatematsu, K., Yano, R., Seo, M., and Kamiya, Y. (2010). Abscisic acid and the control of seed dormancy and germination. Seed Sci. Res. 20, 55–67. doi: 10.1017/S0960258510000012
Nikolaou, N., Koukourikou, A. M., and Karagiannidis, N. (2000). Effects of various rootstocks on xylem exudates cytokinin content, nutrient uptake and growth patterns of grapevine Vitis vinifera L. cv. Thompson seedless. Agronomie 20, 363–373. doi: 10.1051/agro:2000133
Ollat, N., Bordenave, L., Tandonnet, J. P., Boursiquot, J. M., and Marguerit, E. (2016). Grapevine rootstocks: origins and perspectives. Acta Hortic. 1136, 11–22. doi: 10.17660/ActaHortic.2016.1136.2
Or, E. (2009). “Grape Bud Dormancy Release - The Molecular Aspect” in Grapevine Molecular Physiology and Biotechnology. 2nd Edn. ed. K. A. Roubelakis-Angelakis (Netherlands: Springer), 1–29.
Orrantia-Araujo, M. A., Martínez-Téllez, M. Á., Rivera-Domínguez, M., Hernández-Oñate, M. Á., and Vargas-Arispuro, I. (2020). Changes in the endogenous content and gene expression of salicylic acid correlate with grapevine bud dormancy release. J. Plant Growth Regul. 40, 254–262. doi: 10.1007/s00344-020-10100-9
Pacey-Miller, T., Scott, K., Ablett, E., Tingey, S., Ching, A., and Henry, R. (2003). Genes associated with the end of dormancy in grapes. Funct. Integr. Genomics 3, 144–152. doi: 10.1007/s10142-003-0094-6
Pagliarani, C., Vitali, M., Ferrero, M., Vitulo, N., Incarbone, M., Lovisolo, C., et al. (2017). The accumulation of miRNAs differentially modulated by drought stress is affected by grafting in grapevine. Plant Physiol. 173, 2180–2195. doi: 10.1104/pp.16.01119
Pagter, M., and Arora, R. (2013). Winter survival and deacclimation of perennials under warming climate: physiological perspectives. Physiol. Plant. 147, 75–87. doi: 10.1111/j.1399-3054.2012.01650.x
Palliotti, A., Frioni, T., Tombesi, S., Sabbatini, P., Cruz-Castillo, J. G., Lanari, V., et al. (2017). Double-pruning grapevines as a management tool to delay berry ripening and control yield. Am. J. Enol. Vitic. 68, 412–421. doi: 10.5344/ajev.2017.17011
Pepin, N., Bradley, R. S., Diaz, H. F., Baraer, M., Caceres, E. B., Forsythe, N., et al. (2015). Elevation-dependent warming in mountain regions of the world. Nature Clim. Change 5, 424–430. doi: 10.1038/nclimate2563
Peterson, A. G., and Abatzoglou, J. T. (2014). Observed changes in false springs over the contiguous United States. Geophys. Res. Lett. 41, 2156–2162. doi: 10.1002/2014GL059266
Poczai, P., Hyvönen, J., Taller, J., Jahnke, G., and Kocsis, L. (2013). Phylogenetic analyses of Teleki grapevine rootstocks using three chloroplast DNA markers. Plant Mol. Biol. Report. 31, 371–386. doi: 10.1007/s11105-012-0512-9
Qrunfleh, I. M., and Read, P. E. (2013). Delaying bud break in ‘Edelweiss’ grapevines to avoid spring frost injury by NAA and vegetable oil applications. Adv. Hortic. Sci. 27, 18–24. doi: 10.1400/220118
Ramos, M. C., and de Toda, F. M. (2020). Variability in the potential effects of climate change on phenology and on grape composition of Tempranillo in three zones of the Rjoja DOCa (Spain). Eur. J. Agron. 111:126014. doi: 10.1016/j.eja.2020.126014
Reineke, A., and Thiéry, D. (2016). Grapevine insect pests and their natural enemies in the age of global warming. J. Pest. Sci. 89, 313–328. doi: 10.1007/s10340-016-0761-8
Riaz, S., Pap, D., Uretsky, J., Laucou, V., Boursiquot, J. M., Kocsis, L., et al. (2019). Genetic diversity and parentage analysis of grape rootstocks. Theor. Appl. Genet. 132, 1847–1860. doi: 10.1007/s00122-019-03320-5
Roach, M. J., Johnson, D. L., Bohlmann, J., van Vuuren, H. J. J., Jones, S. J. M., Pretorius, I. S., et al. (2018). Population sequencing reveals clonal diversity and ancestral inbreeding in the grapevine cultivar chardonnay. PLoS Genet. 14:e1007807. doi: 10.1371/journal.pgen.1007807
Rohde, A., and Bhalerao, R. P. (2007). Plant dormancy in the perennial context. Trends Plant Sci. 12, 217–223. doi: 10.1016/j.tplants.2007.03.012
Rubio, S., Noriega, X., and Pérez, F. J. (2019a). Abscisic acid (ABA) and low temperatures synergistically increase the expression of CBF/DREB1 transcription factors and cold-hardiness in grapevine dormant buds. Ann. Bot. 123, 681–689. doi: 10.1093/aob/mcy201
Rubio, S., Noriega, X., and Pérez, F. J. (2019b). ABA promotes starch synthesis and storage metabolism in dormant grapevine buds. J. Plant Physiol. 234–235, 1–8. doi: 10.1016/j.jplph.2019.01.004
Ruml, M., Korać, N., Ćirković, D., Vujadinović, M., and Vuković, A. (2016). Heat requirements for red grapevine cultivars in the wine-producing region of Sremski Karlovci, Serbia. Acta Hortic. 1139, 409–412. doi: 10.17660/ActaHortic.2016.1139.71
Sadras, V. O., and Moran, M. A. (2012). Elevated temperature decouples anthocyanins and sugars in berries of shiraz and cabernet franc. Aust. J. Grape Wine Res. 18, 115–122. doi: 10.1111/j.1755-0238.2012.00180.x
Santillán, D., Iglesias, A., La Jeunesse, I., Garrote, L., and Sotes, V. (2019). Vineyards in transition: a global assessment of the adaptation needs of grape producing regions under climate change. Sci. Total Environ. 657, 839–852. doi: 10.1016/j.scitotenv.2018.12.079
Santos, J. A., Fraga, H., Malheiro, A. C., Moutinho-Pereira, J., Dinis, L.-T., Correia, C., et al. (2020). A review of the potential climate change impacts and adaptation options for European viticulture. Appl. Sci. 10:3092. doi: 10.3390/app10093092
Satgé, C., Moreau, S., Sallet, E., Lefort, G., Auriac, M. C., Remblière, C., et al. (2016). Reprogramming of DNA methylation is critical for nodule development in Medicago truncatula. Nat. Plants. 2:16166. doi: 10.1038/nplants.2016.166
Schwechheimer, C., Serino, G., and Deng, X. W. (2002). Multiple ubiquitin ligase-mediated processes require COP9 signalosome and AXR1 function. Plant Cell 14, 2553–2563. doi: 10.1105/tpc.003434
Sgubin, G., Swingedouw, D., Dayon, G., García de Cortázar-Atauri, I., Ollat, N., Pagé, C., et al. (2018). The risk of tardive frost damage in French vineyards in a changing climate. Agric. For. Meteorol. 250–251, 226–242. doi: 10.1016/j.agrformet.2017.12.253
Shangguan, L., Fang, X., Jia, H., Chen, M., Zhang, K., and Fang, J. (2020). Characterization of DNA methylation variations during fruit development and ripening of Vitis vinifera (cv. ‘Fujiminori’). Physiol. Mol. Biol. Plants 26, 617–637. doi: 10.1007/s12298-020-00759-5
Shin, H., Oh, Y., and Kim, D. (2015). Differences in cold hardiness, carbohydrates, dehydrins and related gene expressions under an experimental deacclimation and reacclimation in Prunus persica. Physiol. Plant. 154, 485–499. doi: 10.1111/ppl.12293
Stockinger, E. J., Gilmour, S. J., and Thomashow, M. F. (1997). Arabidopsis thaliana CBF1 encodes an AP2 domain-containing transcriptional activator that binds to the C-repeat/DRE, a cis-acting DNA regulatory element that stimulates transcription in response to low temperature and water deficit. Proc. Natl. Acad. Sci. U. S. A. 94, 1035–1040. doi: 10.1073/pnas.94.3.1035
Su, K., Xing, H., Guo, Y., Zhao, F., Liu, Z., Li, K., et al. (2020). High-density genetic linkage map construction and cane cold hardiness QTL mapping for Vitis based on restriction site-associated DNA sequencing. BMC Genomics 21:419. doi: 10.1186/s12864-020-06836-z
Sun, X., Matus, J. T., Wong, D. C. J., Wang, Z., Chai, F., Zhang, L., et al. (2018). The GARP/MYB-related grape transcription factor AQUILO improves cold tolerance and promotes the accumulation of raffinose family oligosaccharides. J. Exp. Bot. 69, 1749–1764. doi: 10.1093/jxb/ery020
Thomashow, M. F. (2010). Molecular basis of plant cold acclimation: insights gained from studying the CBF cold response pathway. Plant Physiol. 154, 571–577. doi: 10.1104/pp.110.161794
Tillett, R. L., Wheatley, M. D., Tattersall, E. A. R., Schlauch, K. A., Cramer, G. R., and Cushman, J. C. (2012). The Vitis vinifera C-repeat binding protein 4 (VvCBF4) transcriptional factor enhances freezing tolerance in wine grape. Plant Biotechnol. J. 10, 105–124. doi: 10.1111/j.1467-7652.2011.00648.x
Tramontini, S., Vitali, M., Centioni, L., Schubert, A., and Lovisolo, C. (2013). Rootstock control of scion response to water stress in grapevine. Environ. Exp. Bot. 93, 20–26. doi: 10.1016/j.envexpbot.2013.04.001
Trought, M. C. T., Howell, G. S., and Cherry, N. (1999). Practical Considerations for Reducing Frost Damage in Vineyards. Report to New Zealand Winegrowers.
Unterberger, C., Brunner, L., Nabernegg, S., Steininger, K. W., Steiner, A. K., Stabentheiner, E., et al. (2018). Spring frost risk for regional apple production under a warmer climate. PLoS One 13:e0200201. doi: 10.1371/journal.pone.0200201
van Houten, S., Muñoz, C., Bree, L., Bergamín, D., Sola, C., and Lijavetzky, D. (2020). Natural genetic variation for grapevine phenology as a tool for climate change adaptation. Appl. Sci. 10:5573. doi: 10.3390/app10165573
van Leeuwen, C., and Darriet, P. (2016). The impact of climate change on viticulture and wine quality. J. Wine Econ. 11, 150–167. doi: 10.1017/jwe.2015.21
Velasco, R., Zharkikh, A., Troggio, M., Cartwright, D. A., Cestaro, A., Pruss, D., et al. (2007). A high quality draft consensus sequence of the genome of a heterozygous grapevine variety. PLoS One 2:e1326. doi: 10.1371/journal.pone.0001326
Vitasse, Y., Lenz, A., and Körner, C. (2014). The interaction between freezing tolerance and phenology in temperate deciduous trees. Front. Plant Sci. 5:541. doi: 10.3389/fpls.2014.00541
Vitasse, Y., and Rebetez, M. (2018). Unprecedented risk of spring frost damage in Switzerland and Germany in 2017. Clim. Chang. 149, 233–246. doi: 10.1007/s10584-018-2234-y
Vyse, K., Pagter, M., Zuther, E., and Hincha, D. K. (2019). Deacclimation after cold acclimation‐ a crucial, but widely neglected part of plant winter survival. J. Exp. Bot. 70, 4595–4604. doi: 10.1093/jxb/erz229
Wang, H., and Dami, I. E. (2020). Evaluation of Budbreak-delaying products to avoid spring frost injury in grapevines. Am. J. Enol. Vitic. 71, 181–190. doi: 10.5344/ajev.2020.19074
Warschefsky, E. J., Klein, L. L., Frank, M. H., Chitwood, D. H., Londo, J. P., von Wettberg, E. J. B., et al. (2016). Rootstocks: diversity, domestication, and impacts on shoot phenotypes. Trends Plant Sci. 21, 418–437. doi: 10.1016/j.tplants.2015.11.008
Wisniewski, M., Nassuth, A., and Arora, R. (2018). Cold hardiness in trees: a mini-review. Front. Plant Sci. 9:1394. doi: 10.3389/fpls.2018.01394
Wisniewski, M., Nassuth, A., Teulières, C., Marque, C., Rowland, J., Cao, P. B., et al. (2014). Genomics of cold hardiness in Woody plants. CRC. Crit. Rev. Plant Sci. 33, 92–124. doi: 10.1080/07352689.2014.870408
Xiao, H., and Nassuth, A. (2006). Stress‐ and development-induced expression of spliced and unspliced transcripts from two highly similar dehydrin 1 genes in V. riparia and V. vinifera. Plant Cell Rep. 25, 968–977. doi: 10.1007/s00299-006-0151-4
Xiao, H., Siddiqua, M., Braybrook, S., and Nassuth, A. (2006). Three grape CBF/DREB1 genes respond to low temperature, drought and abscisic acid. Plant Cell Environ. 29, 1410–1421. doi: 10.1111/j.1365-3040.2006.01524.x
Xiao, H., Tattersall, E. A. R., Siddiqua, M. K., Cramer, G. R., and Nassuth, A. (2008). CBF4 is a unique member of the CBF transcription factor family of Vitis vinifera and Vitis riparia. Plant Cell Environ. 31, 1–10. doi: 10.1111/j.1365-3040.2007.01741.x
Xu, W., Zhang, N., Jiao, Y., Li, R., Xiao, D., and Wang, Z. (2014). The grapevine basic helix-loop-helix (bHLH) transcription factor positively modulates CBF-pathway and confers tolerance to cold-stress in Arabidopsis. Mol. Biol. Rep. 41, 5329–5342. doi: 10.1007/s11033-014-3404-2
Yang, Y., He, M., Zhu, Z., Li, S., Xu, Y., Zhang, C., et al. (2012). Identification of the dehydrin gene family from grapevine species and analysis of their responsiveness to various forms of abiotic and biotic stress. BMC Plant Biol. 12:140. doi: 10.1186/1471-2229-12-140
Yang, Y., Mao, L., Jittayasothorn, Y., Kang, Y., Jiao, C., Fei, Z., et al. (2015). Messenger RNA exchange between scions and rootstocks in grafted grapevines. BMC Plant Biol. 15:251. doi: 10.1186/s12870-015-0626-y
Yordanov, Y. S., Ma, C., Strauss, S. H., and Busov, V. B. (2014). EARLY BUD-BREAK 1 (EBB1) is a regulator of release from seasonal dormancy in poplar trees. Proc. Natl. Acad. Sci. U. S. A. 111, 10001–10006. doi: 10.1073/pnas.1405621111
Zhang, L., Marguerit, E., Rossdeutsch, L., Ollat, N., and Gambetta, G. A. (2016). The influence of grapevine rootstocks on scion growth and drought resistance. Theor. Exp. Plant Physiol. 28, 143–157. doi: 10.1007/s40626-016-0070-x
Zhao, X., Han, X., Wang, Q., Wang, X., Chen, X., Li, L., et al. (2020). EARLY BUD BREAK 1 triggers bud break in peach trees by regulating hormone metabolism, the cell cycle, and cell wall modifications. J. Exp. Bot. 71, 3512–3523. doi: 10.1093/jxb/eraa119
Zheng, C., Acheampong, A. K., Shi, Z., Mugzech, A., Halaly-Basha, T., Shaya, F., et al. (2018a). Abscisic acid catabolism enhances dormancy release of grapevine buds. Plant Cell Environ. 41, 2490–2503. doi: 10.1111/pce.13371
Zheng, C., Halaly, T., Acheampong, A. K., Takebayashi, Y., Jikumaru, Y., Kamiya, Y., et al. (2015). Abscisic acid (ABA) regulates grape bud dormancy, and dormancy release stimuli may act through modification of ABA metabolism. J. Exp. Bot. 66, 1527–1542. doi: 10.1093/jxb/eru519
Zheng, C., Kwame Acheampong, A., Shi, Z., Halaly, T., Kamiya, Y., Ophir, R., et al. (2018b). Distinct gibberellin functions during and after grapevine bud dormancy release. J. Exp. Bot. 69, 1635–1648. doi: 10.1093/jxb/ery022
Zhu, Z., Shi, J., Xu, W., Li, H., He, M., Xu, Y., et al. (2013). Three ERF transcription factors from Chinese wild grapevine Vitis pseudoreticulata participate in different biotic and abiotic stress-responsive pathways. J. Plant Physiol. 170, 923–933. doi: 10.1016/j.jplph.2013.01.017
Keywords: Vitis vinifera, chilling requirement, deacclimation, budburst, spring frost, gene expression, demethylation
Citation: De Rosa V, Vizzotto G and Falchi R (2021) Cold Hardiness Dynamics and Spring Phenology: Climate-Driven Changes and New Molecular Insights Into Grapevine Adaptive Potential. Front. Plant Sci. 12:644528. doi: 10.3389/fpls.2021.644528
Received: 21 December 2020; Accepted: 17 March 2021;
Published: 29 April 2021.
Edited by:
Chiara Pastore, University of Bologna, ItalyReviewed by:
Artur Conde, University of Minho, PortugalCopyright © 2021 De Rosa, Vizzotto and Falchi. This is an open-access article distributed under the terms of the Creative Commons Attribution License (CC BY). The use, distribution or reproduction in other forums is permitted, provided the original author(s) and the copyright owner(s) are credited and that the original publication in this journal is cited, in accordance with accepted academic practice. No use, distribution or reproduction is permitted which does not comply with these terms.
*Correspondence: Giannina Vizzotto, Z2lhbm5pbmEudml6em90dG9AdW5pdWQuaXQ=; Rachele Falchi, cmFjaGVsZS5mYWxjaGlAdW5pdWQuaXQ=
Disclaimer: All claims expressed in this article are solely those of the authors and do not necessarily represent those of their affiliated organizations, or those of the publisher, the editors and the reviewers. Any product that may be evaluated in this article or claim that may be made by its manufacturer is not guaranteed or endorsed by the publisher.
Research integrity at Frontiers
Learn more about the work of our research integrity team to safeguard the quality of each article we publish.